- 1National Oceanography Centre, Southampton, United Kingdom
- 2Ocean and Earth Science, National Oceanography Centre, University of Southampton, Southampton, United Kingdom
- 3Department of Marine Science, College of Science, Kuwait University, Kuwait City, Kuwait
- 4Centre for Environment, Fisheries and Aquaculture Science (Cefas), Weymouth, United Kingdom
Copepods can feed on, and may regulate, the blooms of harmful algae (HA), and may also facilitate dinoflagellate blooms by inducing toxin production and through selective grazing. However, exposure to HA may also cause mortality and reproductive impairment in copepods, with detrimental effects at the population-scale. Here we present the toxin profile of the dinoflagellate, Alexandrium catenella (formerly Alexandrium tamarense), and examine how it affects the survival and reproduction of the cosmopolitan marine copepod, Acartia tonsa. Healthy adult copepods were exposed to mono-specific diets of toxic and non-toxic strains of A. catenella (1119/27 and 1119/19, respectively) and non-toxic Rhodomonas sp. for 10 days alongside unfed controls to examine how their survival was influenced by likely HA bloom conditions. Additional 2-day experiments examined how their egg production rate and hatching success were affected by food deprivation, toxic A. catenella, a non-toxic alternative and a mixture of toxic and non-toxic prey, at high and low concentrations. Survival of A. tonsa declined over the 10-day experiment in all treatments but was not significantly lower in the toxic A. catenella treatment; mortality was only significantly enhanced in the unfed animals, which showed 100% mortality after 9 days. Egg production rates and hatching success from females in the unfed and toxic A. catenella treatments were all significantly lower than values observed in females fed Rhodomonas sp. or non-toxic A. catenella. Animals offered 1,000 μg C L–1 of Rhodomonas sp. and a 50:50 mixture of toxic A. catenella and Rhodomonas sp. produced significantly more eggs than animals fed toxic A. catenella alone. These results were not apparent at prey concentrations of 100 μg C L–1. The percentages of eggs to successfully hatch from females offered mono-specific diets of toxic A. catenella were always close to zero. Collectively, our results indicate that adult female A. tonsa can acquire sufficient energy from toxic A. catenella to survive, but suffer reproductive impairment when feeding on this prey alone.
Introduction
In the past five decades, harmful algal (HA) blooms, which are caused by toxic or noxious species, have increased in frequency, duration, and distribution, resulting in major environmental and economic impacts (Hallegraeff, 1993; Anderson et al., 2012). Regardless of whether or not these increases are due to changing observational frequencies, HA blooms can be toxigenic, creating blooms that cause disease and death in a number of marine species including fish, seabirds, and mammals (Hallegraeff, 1993; Anderson et al., 2012). The impacts of HA on marine life and humans due to consuming intoxicated shellfish might result in illness or mortality (Wang, 2008). However, the interactions between toxic algae and potential grazer species are complex, inconsistent, and difficult to compare between studies due to different concentrations of HA used as food, the level of toxins in the food and the various responses of the species examined.
The dinoflagellate, Alexandrium catenella [formerly reported in this area as Alexandrium tamarense Group I, reassigned taxonomically by John et al. (2014) and Fraga et al. (2015), acknowledged in Prud’homme van Reine, 2017] can be found in the United Kingdom waters and some strains (such as 1119/27) are associated with shellfish toxicity and produce paralytic shellfish poisoning toxins (PSP), while others can be found naturally non-toxic (such as 1119/19). PSP toxin derivatives are divided into three main groups: carbamate-, N- sulfocarbamoyl-, and decarbamoyl- toxins. Compared to other PSP toxins, the carbamate toxins saxitoxin (STX) and neosaxitoxin (NEO) are considered as the most potent. Gonyautoxins 1 to 4 (GTX1, GTX2, GTX3, and GTX4) are other highly potent analogs, whereas N-sulfocarbamoyl (C) toxins and some of the decarbamoyl (dc) are considered less potent (Cook et al., 2010). Saxitoxins have mainly neurological effects through the blockage of sodium channels (Luckas et al., 2015). Consumption of shellfish contaminated with PSP toxins can cause breathing difficulties, gastrointestinal problems, and a sense of dissociation followed by complete paralysis in humans and other vertebrates (Wang, 2008). However, it remains unclear how exposure to and/or consumption of PSP toxins affect invertebrate fitness.
Zooplankton, and particularly copepods, are major grazers of phytoplankton and can influence phytoplankton dynamics (Runge, 1988; Mann, 1993; Mitra and Flynn, 2006). Copepods dominate the zooplankton communities in most marine water bodies worldwide, and play an important role in the transfer of carbon from phytoplankton to higher trophic levels such as fish and marine mammals (Mann, 1993; Dam, 2013). Toxic algae may affect the survival, feeding and fitness of copepods, and lead to lower growth and reproduction rates in their populations (Barreiro et al., 2007; Jiang et al., 2009; Waggett et al., 2012). These impacts would ultimately result in less food available to higher trophic levels, and may therefore have negative ecological effects (Dam, 2013; Turner, 2014).
In some cases, copepods are reported to show negative effects when feeding on neurotoxic dinoflagellates, with symptoms consistent with toxicity (Dutz, 1998; Xu and Kiørboe, 2018; Xu et al., 2018; Prevett et al., 2019) and decreased fitness (Cohen et al., 2007; Jiang et al., 2009). In other cases, neurotoxic dinoflagellates were consumed by copepods without obvious adverse effects (Teegarden and Cembella, 1996; Abdulhussain et al., 2020). Dinoflagellates that are not known to produce neurotoxins may also adversely affect copepod fitness, e.g., by reducing egg production and hatching success (Ianora et al., 2004). Copepods may produce detoxification enzymes to reduce the impact of toxins (Dutz, 1998; Costa et al., 2008). However, a recent gene expression study that examined the impact of the toxic dinoflagellate, A. catenella (reported as Alexandrium fundyense), on the marine copepod, Calanus finmarchicus, showed that detoxification is not the main component of the response (Roncalli et al., 2016). Rather, the main genes that responded were related to digestion, suggesting that toxins may reduce the absorption of food and therefore the reproductive output without an impact on survival. It is generally recognized that there is no single obvious relationship between phytoplankton neurotoxicity and effects on plankton grazers, and that experiments involving sympatric grazers and prey species are necessary to understand trophic interactions in the plankton (Smayda, 1997; Turner and Tester, 1997).
The resistance of marine copepods to HA has been well documented (Colin and Dam, 2002, 2005, 2007; Jiang et al., 2011; Zheng et al., 2011). Copepods with a history of co-occurrence with HA were found to have higher survival and ingestion rates when exposed to HA blooms (Turner et al., 2000, 2005; Maneiro et al., 2005; Doucette et al., 2006). They may have evolved tolerance to HA species that co-occur in the same region. Some copepods have a discrete toxin-resistant phenotype that suggests a simple genetic mechanism evolving to adapt and tolerate Paralytic Shellfish Poisoning (PSP) toxins during HA blooms (Avery and Dam, 2007). For example, the fitness of Acartia hudsonica increased for several generations after feeding on A. catenella (reported as A. fundyense), which indicates that adaptation occurred (Finiguerra et al., 2014). Mutations of the sodium channels have been suggested as a possible mechanism (Avery and Dam, 2007; Chen et al., 2015). However, exposure of A. hudsonica to HA did not induce an increased proportion of mutant isoforms (Finiguerra et al., 2014), which suggests that mutation in the sodium channel was not responsible.
The majority of studies examining how copepods respond to HA exposure have been conducted over approximately 1 day (Turner, 2014). This duration is sufficient to quantify grazing and discern patterns of feeding selectivity, but may be insufficient to determine any deleterious effects of the HA on grazers since there is a considerable delay in the effect of grazers’ response to toxins, and acclimatization may also take time to occur (Ianora and Miralto, 2010; Turner et al., 2012), e.g., due to the time required to produce detoxification enzymes (Kozlowsky-Suzuki et al., 2009). Short-term exposure to toxic algae might not result in significantly increased mortality compared to non-toxic algae. However, extended periods of exposure to toxic algae may cause substantial mortality within a grazers’ population (Prince et al., 2006; Barreiro et al., 2007; Jiang et al., 2009; Drillet et al., 2015). Results from short-term, e.g., 24 h, experiments may therefore hinder our ability to understand how copepods are affected by exposure to natural blooms of HA, which can last from a few days up to a few months (Bresnan et al., 2008).
Here we exposed adult female A. tonsa to mono-specific diets of toxic and non-toxic strains of A. catenella and non-toxic Rhodomonas sp. for 10 days alongside unfed controls to examine how their survival was impacted by probable conditions experienced during a HA bloom. In addition, 2-day experiments examined how their egg production rate and hatching success were influenced by the type of food available and its concentration. Our results are presented alongside a full toxin profile for A. catenella in each of the experiments conducted.
Materials and Methods
Culture Condition
All phytoplankton were grown in a culture cabinet at 15°C with a 16:8 h (Light:Dark) photoperiod, using seawater collected from the Western Channel Observatory monitoring site L4 off Plymouth, United Kingdom1, amended with either F/2 (Rhodomonas sp.) or L1 (A. catenella) medium (Guillard and Ryther, 1962; Guillard and Hargraves, 1993). Acartia tonsa were purchased from Reefshotz, Cardiff, United Kingdom, where they have been cultured for >10 years, and were subsequently cultured in artificial seawater (Tropic Marin Sea salt, Classic Meersalz; salinity 31) at room temperature (21 ± 2°C) for >15 months prior to experimentation. Details of the culturing conditions for all phytoplankton species and for A. tonsa can be found elsewhere (Abdulhussain et al., 2020). The toxic A. catenella cultured was strain 1119/27 (CCAP, Loch Ainort, Scotland), and the non-toxic A. catenella was strain 1119/19 (CCAP Port Ellen, Islay, Scotland). A. tonsa cultures were fed Rhodomonas sp. (59.0 ± 7.8 pg C cell–1) before experiments 1 and 2 and Isochrysis galbana (25.5 ± 0.3 pg C cell–1) before experiment 3 at a concentration of 60,000 cells ml–1 (2,000 ± 1,000 μg C L–1) three times a week.
Influence of Toxic A. catenella, Starvation, and Non-toxic Prey Alternatives on the Survival of A. tonsa
Experiment 1 examined how the survival of A. tonsa was affected by a 10-day exposure to either food deprivation (starved) or one of three different types of food: Rhodomonas sp. (RHO), toxic A. catenella (TAC) and non-toxic A. catenella (NAC). Prey cells were offered at a nominal concentration of 1,000 μg carbon (C) L–1 to ensure that feeding conditions were always saturating (Berggreen et al., 1988; Abdulhussain et al., 2020). Individual adult females were incubated in 15 ml glass dram vials with 10 ml of sterile filtered L4 seawater (0.2 μm; SFSW hereafter) for 10 days at 19°C with a 12:12 h (L:D) photoperiod. Each of the four treatment levels was replicated fifteen times (total = 60 bottles). Nominal prey concentrations were achieved by determining the algal stock culture concentration using a Beckman Multisizer 3 Coulter Counter equipped with a 70 μm aperture and subsequently diluting it with an appropriate volume of SFSW. Each copepod was carefully transferred into a petri dish at the end of every 24 h incubation period and examined for survival, determined by movement after mechanical stimulus. Surviving copepods were subsequently pipetted into clean 15 ml vials of fresh SFSW containing the same species and concentration of prey cells that they had previously been exposed to. Algal concentrations in the stock cultures were counted every other day using the Coulter Counter. Triplicate 5 ml samples from the stock cultures of each of the three prey types (RHO, TAC, and NAC) were collected onto pre-combusted 13 mm glass fiber filters (Whatman GF/F) on the start of days 1 and 9 of the experiment, dried overnight at 40°C and stored in a desiccator prior to determining their carbon and nitrogen content (Carlo Erba EA-1108 elemental analyzer). Triplicate 30 ml samples of the TAC stock culture were collected at the outset of the experiment, centrifuged at 3,000 rpm for 20 min, and the resulting pellets were transferred into 2 ml Eppendorf tubes and stored frozen at −80°C until toxin profile analysis (see section “Toxin Analysis”).
Influence of Toxic A. catenella, Starvation, and Non-toxic Prey Alternatives on the Egg-Production and Hatching Success of A. tonsa
Experiment 2 examined how the egg production rate of A. tonsa was affected by different types of food: RHO, TAC, and NAC. Prey cells were offered at a nominal concentration of 1,000 μg C L–1. Four adults (three females and one male) were incubated in 150 ml LPDE plastic bottles with an inner mesh compartment of 120 μm (to separate the adult from the eggs produced during the experiment) filled with 100 ml of SFSW for 2 days at 19°C with a 12:12 h (L:D) photoperiod. Each of the four treatment levels (starved, RHO, TAC, NAC: 12 bottles) was replicated three times. The experiment was run over 2 days, with the first day being considered as an acclimation period (Breier and Buskey, 2007; Cohen et al., 2007; Jiang et al., 2009; Waggett et al., 2012; Abdulhussain et al., 2020). Hatching success was therefore only calculated for eggs produced on day 2. After the first day, the mesh compartments containing copepods were transferred to another LPDE plastic bottle with fresh SFSW containing the same species and concentration of prey cells that they had previously been exposed to. The eggs left in the LPDE plastic bottles from day 1 were counted under a stereomicroscope (to observe the response of A. tonsa to TAC during the acclimation period: see Supplementary Material). At the end of day 2, copepods in each treatment were carefully transferred into a petri dish and examined for survival (see section “Influence of Toxic A. catenella, Starvation, and Non-toxic Prey Alternatives on the Survival of A. tonsa”). Eggs produced on day 2 were counted and then transferred into petri dishes for 48 h at 19°C with a 12:12 h (L:D) photoperiod before counting the eggs hatched for each group, and calculating percentage hatching success (eq. 1):
Samples to determine the toxin- and elemental-content of the algal cultures were collected on the start of day 2 (see section “Influence of Toxic A. catenella, Starvation, and Non-toxic Prey Alternatives on the Survival of A. tonsa”).
Influence of Toxic A. catenella, Starvation, and Mixed Diet Prey Alternatives on the Egg-Production and Hatching Success of A. tonsa at High and Low Concentrations
Experiment 3 was conceptually similar to experiment 2, but examined how the egg production rate and hatching success of A. tonsa was affected by low (100 μg C L–1) and high (1,000 μg C L–1) concentrations of: RHO, TAC, and a diet where the carbon content was derived from 50% RHO to 50% TAC (MIX hereafter). Each of the seven treatment levels (starved, low RHO, high RHO, low TAC, high TAC, low MIX, and high MIX) was replicated three times (two times for low MIX; total = 20 bottles). Samples to determine the toxin- and elemental-content of the algal cultures were collected at the start of the experiment (after the acclimation period) (see section “Influence of Toxic A. catenella, Starvation, and Non-toxic Prey Alternatives on the Survival of A. tonsa”).
Toxin Analysis
The frozen pellets of A. catenella from each experiment were mixed with 1.5 ml of 1% acetic acid before being vortex-mixed for 90 s at 2500 rpm. Solutions were subjected to probe sonication (Sonic Dismembrator, Fisher Scientific) set to 30% power for 1.5 min per sample. After sonication, vials were re-mixed, and 400 μL taken for desalting clean-up using carbon solid phase extraction (SPE). Details of the toxin extraction, column, mobile phases, chromatographic separation, and HILIC-MS/MS analysis conditions are described elsewhere (Turner et al., 2015, 2019; Abdulhussain et al., 2020).
Data Analyses
The effects of 10 days of exposure to food deprivation, RHO, TAC, and NAC on the survival of A. tonsa were examined using Kaplan Meier survival analysis:
Where S is the Survival probability; t is time in days; N is the number of A. tonsa that survived; and D is the number of A. tonsa that died. Curve comparisons were achieved using the Log-rank test. Standard errors are presented to show the deviation from the population mean.
The influence of feeding conditions (starved, RHO, TAC, and NAC) on the egg production of A. tonsa in experiment 2 was examined using 1-way ANOVA. Egg hatching success was examined using a 1-way ANOVA. The influence of food type (starved, TAC, MIX) and food concentration (100 or 1,000 μg C L–1) on the egg production of A. tonsa in experiment 3 were examined using 2-way ANOVA. Egg hatching success was examined using a 2-way ANOVA. All explanatory variables were treated as categories. Post hoc comparisons were conducted using Tukey’s test with alpha = 0.05. Standard errors are presented for the egg production and hatching experiments to show the variation within each treatment. All statistical analyses were carried out using Prism Graphpad (v.8.4) software.
Results
The carbon and nitrogen contents of the prey cells offered in the different experiments are presented in Table 1. TAC toxin profile concentrations, and their saxitoxin (STX) equivalent, are presented in Table 2.
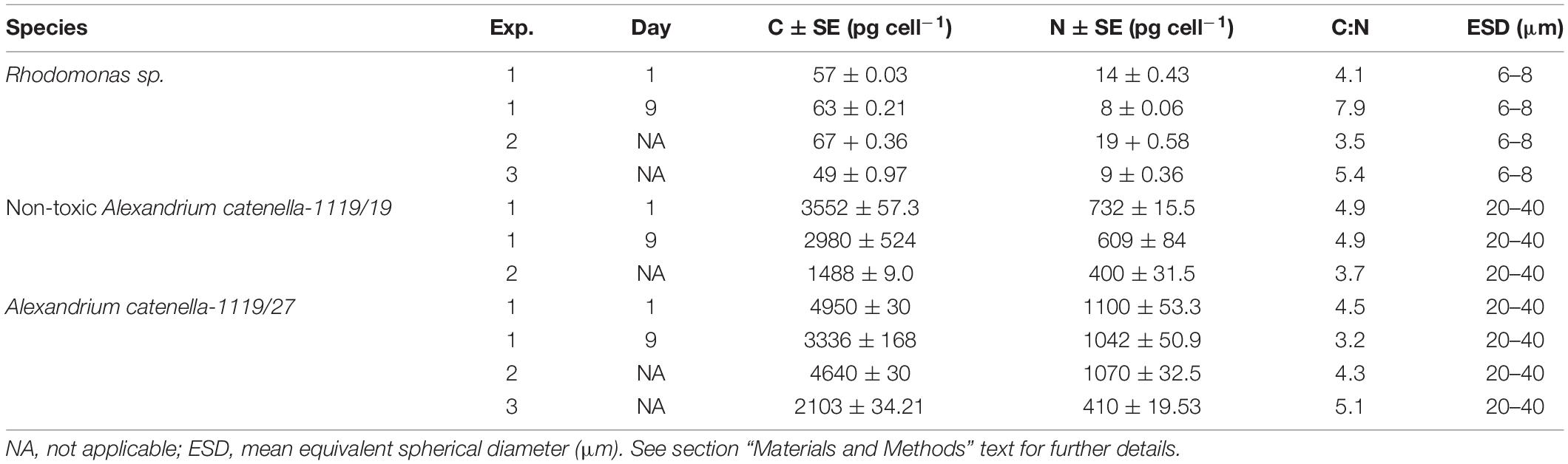
Table 1. The concentrations of carbon (C) and nitrogen (N) (pg cell–1) ± standard error (SE) in the different prey cells offered to Acartia tonsa in the three experiments (Exp.).
Influence of Toxic A. catenella, Starvation, and Non-toxic Prey Alternatives on the Survival of A. tonsa
The survival of female A. tonsa declined significantly across the 10-day duration of the experiment (Figure 1; Log-rank test, Chi square = 29, df = 3, p < 0.001). However, survival was only significantly reduced in the starving group, where there was 100% mortality by day 9 (Figure 1; Log-rank test, Chi square = 29, df = 3, p < 0.001). Survival of the females offered RHO (73%), TAC (80%), and NAC (60%) at nominal concentrations of 1,000 μg C L–1 were statistically indistinguishable, with an average survival of 71 ± 10% across these treatments (Figure 1; Log-rank test, Chi square = 1, df = 2, p = 0.525).
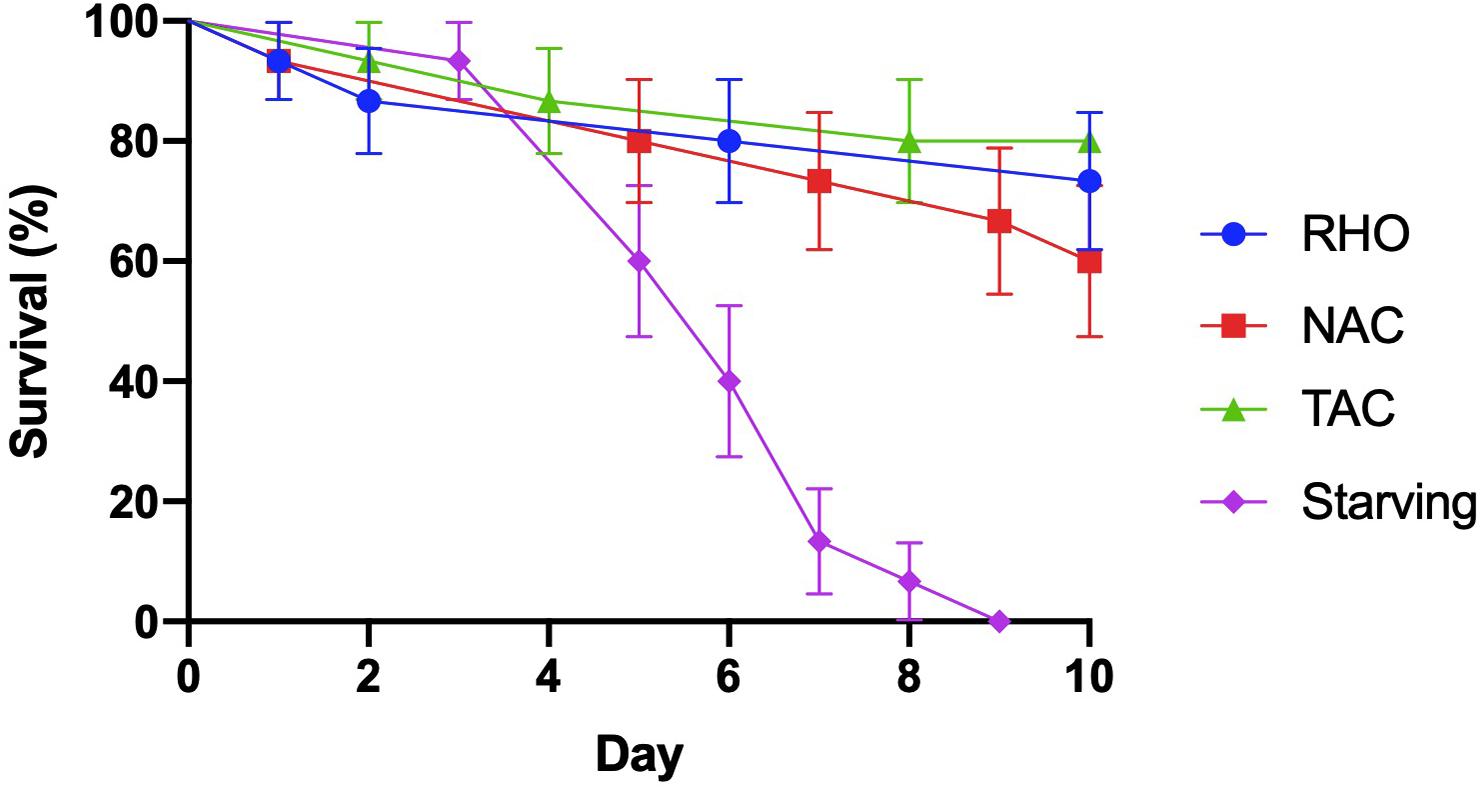
Figure 1. Acartia tonsa survival when exposed to RHO (Rhodomonas sp.; blue circles), TAC (toxic Alexandrium catenella 1119/27; green triangles), and NAC (non-toxic A. catenella 1119/19; red squares) at a concentration of 1,000 μg C L− 1 or when starved (purple diamond). Dots (N = 15) are mean value, and error bars show the standard error (SE).
Influence of Toxic A. catenella, Starvation, and Non-toxic Prey Alternatives on the Egg Production and Hatching Success of A. tonsa
The egg production rate of A. tonsa was affected by the type of food they received (Figure 2A; 1-way ANOVA, F(3,8) = 13.24, p ≤ 0.0115). The females offered RHO and NAC produced significantly more eggs (17.7 ± 4.9 and 14.1 ± 5.7 eggs female–1 day–1, respectively) than those offered TAC (2.4 ± 2.3 eggs female–1 day–1) and starved (1.0 ± 0.6 eggs female–1 day–1) (Figure 2A). The hatching success of eggs produced on day 2 was influenced by the maternal feeding conditions (1-way ANOVA, F(3,7) = 11.53, p = 0.0042). Hatching success of the eggs from females that had received RHO (60 ± 9.7%) and NAC (56 ± 23.9%) was higher than the eggs from females fed TAC (0%) or starved (7 ± 11.5%) (Figure 2B).
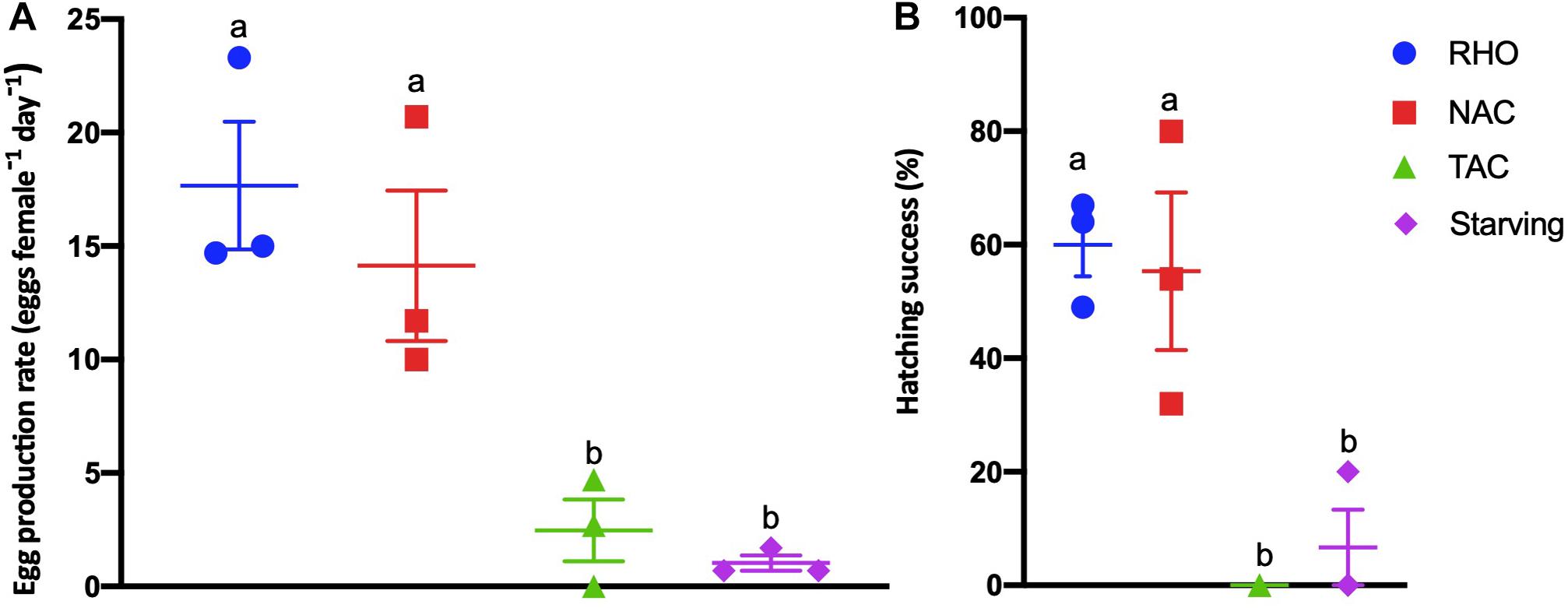
Figure 2. Egg production and hatching success of Acartia tonsa fed on RHO (Rhodomonas sp.; blue circles), TAC (toxic Alexandrium catenella 1119/27; green triangles), and NAC (non-toxic A. catenella 1119/19; red squares) at a concentration of 1,000 μg C L− 1 or when starved (purple diamond). Dots are replicates and bars show the mean ± standard error (SE). (A) Eggs produced (24–48 h) after acclimation period. (B) Nauplii hatched (48–96 h). The a and b letters above bars show the post hoc comparisons between the treatments with shared letters denoting no significant difference.
Influence of Toxic A. catenella, Starvation, and a Mixed Diet on the Egg-Production and Hatching Success of A. tonsa at High and Low Food Concentrations
The egg production rate of A. tonsa was affected by food type and food quantity (Figure 3A, 2-way ANOVA, food type: F(3,15) = 40.0; food quantity F(1,15) = 25.0, p ≤ 0.002 in both cases). Copepods that were fed on MIX at 1,000 μg C L–1 produced significantly fewer eggs (4.7 ± 1.2 eggs female–1 day–1) than in the RHO treatment at the same concentration (9.0 ± 1.3 eggs female–1 day–1) but significantly more than the females that received TAC (0.3 ± 0.3 eggs female–1 day–1) (Figure 3A). Egg production rates in the 100 μg C L–1 treatments were all similarly low (Figure 3A). The hatching success of the eggs produced was influenced by the food type and food quantity (Figure 3B, 2-way ANOVA, F(3,15) = 15.39 and 6.32, respectively, p ≤ 0.0238 in both cases). The hatching success of eggs from females that were fed 1,000 μg C L–1 of RHO (58 ± 14%) and MIX (56 ± 25%) were both higher than TAC (0%) and starved (0%) (Figure 3B), whereas the hatching success of eggs from females fed at 100 μg C L–1 of RHO (67 ± 18%), MIX (60 ± 28%) and TAC (62 ± 28%) were all similar.
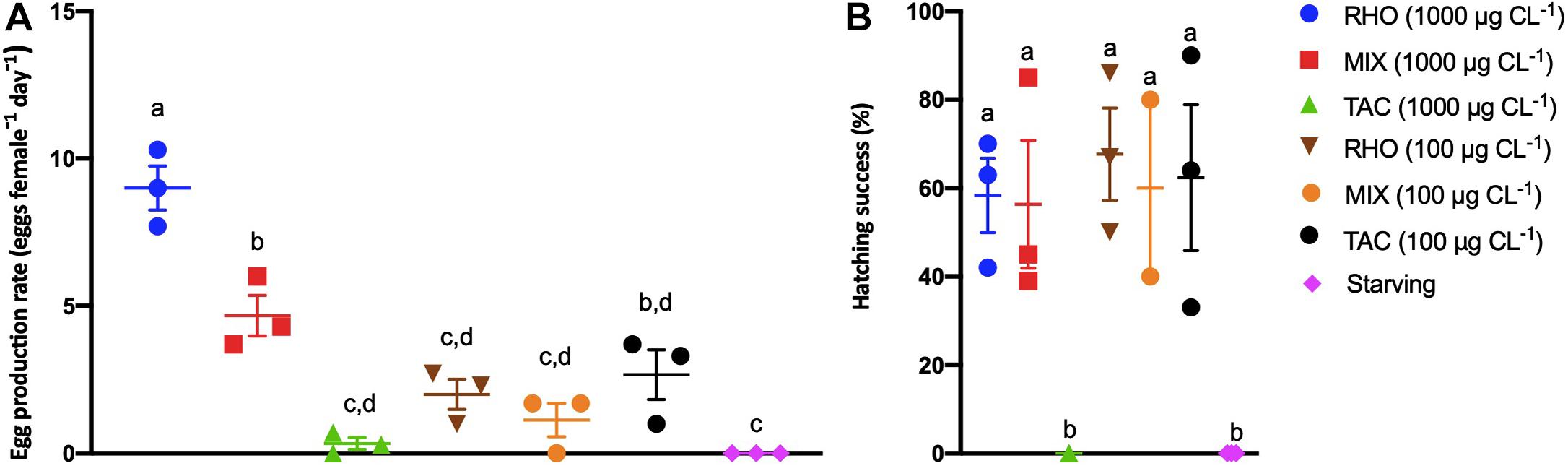
Figure 3. Egg production and hatching success of Acartia tonsa fed on RHO (Rhodomonas sp.; brown inverted triangles and blue circles, 100 and 1,000 μg C L− 1, respectively), TAC (toxic Alexandrium catenella 1119/27; black circles and green triangles, 100 and 1,000 μg C L− 1, respectively), and MIX (50:50 mixture of both cell types, orange circles and red squares, 100 and 1,000 μg C L− 1, respectively) at two concentrations: 100 and 1,000 μg C L− 1 or when starved (purple diamond). The dots are replicates, and bars show the mean ± standard error (SE). (A) Eggs produced (24–48 h) after acclimation period. (B) Nauplii hatched (48–96 h). The a and b letters above bars show the post hoc comparisons between the treatments with shared letters denoting no significant difference.
Discussion
A. catenella Toxin Composition and Concentrations
The elemental content, and composition and concentrations of toxins, of A. catenella (1119/27) were all variable (Tables 1, 2), despite being grown under near identical conditions. For example, the total toxin concentration was 501.1 STX eq cell–1 for experiments 1 and 2, and 3575.5 STX eq cell–1 for experiment 3. Some of the factors that can change the toxin levels within a HA bloom are turbulence (Juhl et al., 2001), salinity (Grzebyk et al., 2003), and nutrient conditions (Turner et al., 1998; John and Flynn, 2002; Leong et al., 2004). Toxin levels can also vary with growth phase in cultured algae (Hamasaki et al., 2001; Cook et al., 2010). We suggest that the differences in toxicity in our laboratory-based study were caused by differences in the nutrient concentrations of the seawater that was used for culturing TAC: Experiment 1 and 2 were conducted during January 2020, and experiment 3 during July 2019, when surface nitrate and phosphate concentrations were close to their highest and lowest, respectively2. Moreover, in response to nutrient stress and chemical signals from copepods, HA can dramatically increase the development of PSP toxins (Wohlrab et al., 2010; Selander et al., 2015; Griffin et al., 2019). A group of predator cues called copepodamides (also known as taurine-containing lipids; Mayor et al., 2015), which are exuded by three species of calanoid copepods (Centropages typicus, Pseudocalanus sp., and Calanus sp.) have been found to trigger increased PSP toxins in dinoflagellates, as well as amnesic shellfish poisoning (ASP) toxins in diatoms (Selander et al., 2019). It is therefore possible that the toxin content of the prey cells increased during our incubations and the concentrations presented in Table 2 should be considered minimal. These differences highlight the importance of reporting toxin profile concentrations, particularly in the context of copepod survival, grazing, and egg production studies. This makes it easier to robustly compare between different studies.
Survival of A. tonsa Exposed to Toxic A. catenella
Exposure of A. tonsa to a toxic strain of TAC for 10 days did not significantly affect their survival relative to the NAC treatment. Rather, increased mortality was only observed in the starved treatment (Figure 1). These findings suggest that A. tonsa is able to feed and survive on TAC over the likely duration of a typical bloom of TAC (Bresnan et al., 2008), regardless of their toxicity. Previous 24 h experiments showed that A. tonsa can feed upon TAC and survive blooms two orders of magnitude higher than the maximum densities of A. catenella recorded in natural systems (Abdulhussain et al., 2020), possibly by producing detoxification enzymes (Dutz, 1998; Costa et al., 2008) or by reducing food absorption efficiency (Asselman et al., 2012; Roncalli et al., 2016). Bloom concentrations of TAC are generally low and typically last for only a few days (Bresnan et al., 2005), although more persistent blooms are reported (Bresnan et al., 2008). Our findings therefore support the understanding that Acartia sp. tolerate exposure to TAC for the typical duration of naturally occurring blooms without negative impacts on the survival of adults (Liu and Wang, 2002; Estrada et al., 2008). However, exposure to high densities of HA for an extended period may cause substantial mortality within Acartia spp. populations (Prince et al., 2006; Barreiro et al., 2007; Jiang et al., 2009). Thus, the response of Acartia spp. appears to vary between different studies, possibly because of different toxin concentrations (section “A. catenella Toxin Composition and Concentrations”). Higher survival rates of A. tonsa in this study compared to other survival experiments could also be a result of reduced encounter rates between individuals as each experimental vial contained only one female per treatment replicate. However, in denser cultures, elevated encounter rates between individuals may increase levels of stress and cannibalism, potentially resulting in higher rates of mortality (Drillet et al., 2015).
Egg Production and Hatching Success of A. tonsa Exposed to Toxic A. catenella
The average egg production rate of A. tonsa feeding on RHO in our 2-day exposure experiments, ∼17 eggs female–1 day–1 (Figure 2), agrees with values previously reported for the genus Acartia sp. (range = 9–76 eggs female–1 day–1; Prince et al., 2006; Barreiro et al., 2007; Breier and Buskey, 2007; Jiang et al., 2009; Waggett et al., 2012). Our results reveal that egg production and hatching success were affected by the types of food available and the concentration at which it was offered (Figures 2, 3), with the observed values being significantly lower in the TAC treatments relative to those in the NAC and RHO treatments (Figure 2). Egg production rates by females fed RHO, NAC and MIX (50% RHO, 50% TAC) at 1,000 μg C L–1 were also significantly higher than those fed TAC alone. By contrast, the rates of females fed TAC were not statistically different to those of the starved animals (Figures 2, 3). Furthermore, the percentages of eggs to successfully hatch from females offered mono-specific diets of toxic A. catenella at concentration of 1,000 μg C L–1 were always close to zero. These results are consistent with the observation that reproduction in Acartia clausi is negatively affected when consuming toxic Alexandrium minutum, and that these effects can be alleviated by offering mixed diets (Barreiro et al., 2006, 2007).
Acartia tonsa can discriminate between different species of Alexandrium with different toxin contents, showing preference for the least toxic species (Xu et al., 2018; Abdulhussain et al., 2020). Copepods are thought to be capable of rejecting toxic prey cells (Cook et al., 2010; Abdulhussain et al., 2020) or consume them at lower rates than a similarly sized non-toxic cells (Xu and Kiørboe, 2018). It is therefore possible that the reduced egg production rate in the TAC treatment could be caused by starvation. However, we know that A. tonsa is capable of surviving and feeding on TAC when alternative prey are not available (Abdulhussain et al., 2020). This understanding is further supported by the current study, where survival of A. tonsa in TAC treatment was significantly higher than in the starved treatment (section “Survival of A. tonsa Exposed to Toxic A. catenella”). The significantly lower egg production rates observed in the TAC treatments of both experiments is therefore unlikely attributable to a starvation effect. The negative effects of TAC on the observed egg production rates and hatching success may have occurred because (a) the females were directly impeded by the toxins, (b) the females needed to produce detoxification enzymes, and therefore had to channel energy and other resources away from producing viable eggs, (c) the presence of toxins reduced the efficiency with which food is absorbed, and therefore reduced the amount of energy and other resources available for producing viable eggs, or (d) TAC were in some way nutritionally inferior to the other diets offered.
Nutritionally deficient food and the presence of toxins have both been suggested to cause reduced egg production in Acartia sp. (Dutz, 1998; Collumb and Buskey, 2004; Prince et al., 2006; Costa et al., 2008). The survival of females exposed to TAC was not significantly lower than females exposed to the non-toxic prey treatments, apparently suggesting that TAC toxins do not necessitate additional metabolic costs associated with the production of detoxification enzymes to the extent that they have a discernible, negative effect on the overall physiological performance of A. tonsa. However, copepods may increase their ingestion rates when feeding on toxic dinoflagellates in order to compensate for the increased energy required for detoxification (Dutz, 1998; Costa et al., 2008) or reduce the efficiency with which they absorb food (Asselman et al., 2012; Roncalli et al., 2016). For example, ingestion rates of A. tonsa were higher when fed on toxic A. catenella relative to animals fed on non-toxic Rhodomonas sp. at a food saturating concentration of 1,000 μg C L–1 (Abdulhussain et al., 2020). Compensatory feeding may also be necessary if the nutritional value of HA is lower than other non-toxic food (Collumb and Buskey, 2004; Prince et al., 2006). Our survival experiments were conducted at a food saturating concentration of 1000 μg C L–1 (Berggreen et al., 1988; Abdulhussain et al., 2020) and we cannot therefore exclude the possibility that the females increased their ingestion rates to offset any additional metabolic costs. Nevertheless, even if compensatory feeding did occur, it was clearly insufficient to mitigate the negative effects on reproduction associated with ingesting TAC. Egg production rates of females fed NAC, which we assume to have a highly similar nutritional make up to TAC, were indistinguishable from those fed on RHO, a food source that is widely assumed to support optimal reproductive output in Acartia spp. (Støttrup et al., 1986; Berggreen et al., 1988). We therefore suggest that the reduced rates observed in the TAC treatment (Figure 2) were not attributable to the absence of nutritive compounds; they occurred because of the presence of toxins, although the specific mechanism cannot be elucidated here.
The negative effects of consuming TAC were less apparent when prey cells were offered at 100 μg C L–1, where egg production rates were similarly low across all treatments (Figure 3A). This agrees with the understanding that lower food concentrations limit growth, reproduction, and survival (Berggreen et al., 1988). Interestingly, the hatching success of the few eggs produced by females fed TAC at 100 μg C L–1 was similarly high when compared to the values observed in the other, non-toxic treatments. This may suggest that there is a toxin-threshold level, beyond which eggs do not hatch.
Prey cell size can influence the outcome of copepod feeding experiments. However, in our study, differences between Rhodomonas (6–8 μm ESD) and I. galbana (4–6 μm ESD) were trivial, suggesting that these are unlikely to cause differences in the feeding rates of our experimental animals. Indeed, previous work has shown that female A. tonsa have an optimal prey size of 14.8 μm ESD, but are able to achieve high clearance rates when feeding on both Rhodomonas and on the dinoflagellate A. catenella (ESD = 20–40 μm) (Berggreen et al., 1988; Abdulhussain et al., 2020). Moreover, despite differences in the feeding histories of the animals in experiments 2 and 3, the outcomes of these two experiments were qualitatively and quantitatively the same: EPR and hatching success in the TAC and starved treatments in both experiments were similarly low (not different to zero); EPR and hatching success in the RHO treatments in both experiments were always higher than in the TAC and starved treatments (Figures 2, 3); ERP in the RHO treatments were not statistically different between the two experiments (Paired t-tests: t = 3.965, df = 2, p = 0.058). These results would not have occurred if any feeding history effects had not been accounted for within the 24 h acclimation period.
Finally, in the real environment, there are usually more than one species of algae available for copepods to feed on. The availability of alternative prey suggests that the impact of HA on copepod egg production may be very low due to availability of other algae. Nevertheless, it is clear that further work is required to understand the energetic and nutritional requirements of A. tonsa and their developing embryos, and how these are directly and indirectly affected when ingesting toxin-producing prey species.
Summary
The survival of A. tonsa exposed to monospecific diets of either toxic and non-toxic A. catenella or Rhodomonas sp. declined over a 10-day incubation experiment. However, mortality only increased significantly in unfed copepods, which showed 100% mortality after 9 days. Egg production and hatching success rates of A. tonsa exposed to 1,000 μg C L–1 toxic A. catenella were not significantly different from starved animals, but were significantly lower than animals fed 1,000 μg C L–1 of non-toxic A. catenella, Rhodomonas sp., or a 50:50 mix of toxic A. catenella and Rhodomonas sp. Egg production and hatching success rates of eggs produced by females that were fed either Rhodomonas sp., toxic A. catenella or a 50:50 mix of toxic A. catenella and Rhodomonas sp. at 100 μg C L–1 were not significantly different. Further studies are required to understand the physiological basis of why female A. tonsa can feed and survive on toxic A. catenella, but display reproductive impairment as a result.
Data Availability Statement
The raw data supporting the conclusions of this article will be made available by the authors, without undue reservation.
Author Contributions
AA, DM, and KC contributed to conception and design of the study. AA conducted the experiments, performed the statistical analyses, and wrote the manuscript. AA, AT, and AL contributed to toxin extraction and analyses. All authors contributed to manuscript revision, read, and approved the submitted version.
Funding
This work was funded by a Ph.D. scholarship awarded to AA from Kuwait University.
Conflict of Interest
The authors declare that the research was conducted in the absence of any commercial or financial relationships that could be construed as a potential conflict of interest.
Acknowledgments
We thank Angus Atkinson for assistance in organizing seawater collection, and John Gittins, Nicola Pratt, Robbie Robinson, and George Clarke for mass algae culturing. We also thank the reviewers for their insightful comments, all of which helped improve an earlier version of this manuscript.
Supplementary Material
The Supplementary Material for this article can be found online at: https://www.frontiersin.org/articles/10.3389/fmars.2021.652225/full#supplementary-material
Footnotes
- ^ https://www.westernchannelobservatory.org.uk/
- ^ https://www.westernchannelobservatory.org.uk/l4_nutrients.php
References
Abdulhussain, A. H., Cook, K. B., Turner, A. D., Lewis, A. M., Elsafi, M. A., and Mayor, D. J. (2020). The influence of the toxic producing dinoflagellate, Alexandrium catenella (1119/27) on the feeding and survival of the marine copepod Acartia tonsa. Harmful Algae 98:101890. doi: 10.1016/j.hal.2020.101890
Anderson, D. M., Cembella, A. D., and Hallegraeff, G. M. (2012). Progress in understanding harmful algal blooms: paradigm shifts and new technologies for research, monitoring, and management. Ann. Rev. Mar. Sci. 4, 143–176. doi: 10.1146/annurev-marine-120308-081121
Asselman, J., De Coninck, D. I. M., Glaholt, S., Colbourne, J. K., Janssen, C. R., Shaw, J. R., et al. (2012). Identification of pathways, gene networks, and paralogous gene families in Daphnia pulex responding to exposure to the toxic cyanobacterium Microcystis aeruginosa. Environ. Sci. Technol. 46, 8448–8457. doi: 10.1021/es301100j
Avery, D. E., and Dam, H. G. (2007). Newly discovered reproductive phenotypes of a marine copepod reveal the costs and advantages of resistance to a toxic dinoflagellate. Limnol. Oceanogr. 52, 2099–2108. doi: 10.4319/lo.2007.52.5.2099
Barreiro, A., Guisande, C., Frangópulos, M., Gonzalez-Fernandez, A., Munoz, S., Perez, S., et al. (2006). Feeding strategies of the copepod Acartia clausi on single and mixed diets of toxic and non-toxic strains of the dinoflagellate Alexandrium minutum. Mar. Ecol. Prog. Ser. 316, 115–125. doi: 10.3354/meps316115
Barreiro, A., Guisande, C., Maneiro, I., Vergara, A. R., Riveiro, I., and Iglesias, P. (2007). Zooplankton interactions with toxic phytoplankton: some implications for food web studies and algal defence strategies of feeding selectivity behaviour, toxin dilution and phytoplankton population diversity. Acta Oecol. 32, 279–290. doi: 10.1016/j.actao.2007.05.009
Berggreen, U., Hansen, B., and Kiørboe, T. (1988). Food size spectra, ingestion and growth of the copepod Acartia tonsa during development: implications for determination of copepod production. Mar. Biol. 99, 341–352. doi: 10.1007/BF02112126
Breier, C. F., and Buskey, E. J. (2007). Effects of the red tide dinoflagellate, Karenia brevis, on grazing and fecundity in the copepod Acartia tonsa. J. Plankton Res. 29, 115–126. doi: 10.1093/plankt/fbl075
Bresnan, E., Fryer, R., Hart, M., and Percy, P. (2005). Correlation Between Algal Presence in Water and Toxin Presence in Shellfish. Fisheries Research Services Contract Report 04/05. Aberdeen: Fisheries Research Services.
Bresnan, E., Turrell, E. A., and Fraser, S. (2008). “Monitoring PSP and Alexandrium hotspots in Scottish waters,” in Proceedings of the 12th International Conference on Harmful Algae, eds Ø Moestrup, G. Doucette, H. Enevoldson, A. Godhe, G. Hallegraeff, B. Luckas, et al. (Copenhagen: Intergovernmental Oceanographic Commission of UNESCO).
Chen, L., Zhang, H., Finiguerra, M., Bobkov, Y., Bouchard, C., Avery, D. E., et al. (2015). A novel mutation from gene splicing of a voltage-gated sodium channel in a marine copepod and its potential effect on channel function. J. Exp. Mar. Bio. Ecol. 469, 131–142. doi: 10.1016/j.jembe.2015.04.003
Cohen, J. H., Tester, P. A., and Forward, R. B. Jr. (2007). Sublethal effects of the toxic dinoflagellate Karenia brevis on marine copepod behavior. J. Plankton Res. 29, 301–315. doi: 10.1093/plankt/fbm016
Colin, S. P., and Dam, H. G. (2002). Latitudinal differentiation in the effects of the toxic dinoflagellate Alexandrium spp. on the feeding and reproduction of populations of the copepod Acartia hudsonica. Harmful Algae 1, 113–125. doi: 10.1016/S1568-9883(02)00007-0
Colin, S. P., and Dam, H. G. (2005). Testing for resistance of pelagic marine copepods to a toxic dinoflagellate. Evol. Ecol. 18, 355–377. doi: 10.1007/s10682-00402369-3
Colin, S. P., and Dam, H. G. (2007). Comparison of the functional and numerical responses of resistant versus non-resistant populations of the copepod Acartia hudsonica fed the toxic dinoflagellate Alexandrium tamarense. Harmful Algae 6, 875–882. doi: 10.1016/j.hal.2007.05.003
Collumb, C. J., and Buskey, E. J. (2004). “Effects of the toxic red tide dinoflagellate (Karenia brevis) on survival, fecal pellet production and fecundity of the copepod Acartia tonsa,” in Harmful Algae 2002, eds K. A. Steidinger, J. H. Landsberg, C. R. Thomas, and G. A. Vargo (Tallahassee, FL: Florida Fish and Wildlife Commission), 44–46. doi: 10.1093/plankt/fbl075
Cook, K. B., Bresnan, E., and Turrell, E. A. (2010). The influence of naturally occurring algal biotoxins on the biology of pelagic copepods: a review of the literature and data currently available for Scottish waters. Scottish Mar. Freshw. Sci. 1:71.
Costa, R. M., Pereira, L. C. C., and Fernández, F. (2008). Effects of toxic Alexandrium minutum strains on the feeding and survival rates of pelagic marine copepods Acartia grani and Euterpina acutifrons. Hydrobiologia 614, 55–63. doi: 10.1007/s10750-008-9536-4
Dam, H. G. (2013). Evolutionary adaptation of marine zooplankton to global change. Ann. Rev. Mar. Sci. 5, 349–370. doi: 10.1146/annurev-marine-121211-172229
Doucette, G. J., Cembella, A. D., Martin, J. L., Michaud, J., Cole, T. V. N., and Rolland, R. M. (2006). Paralytic shellfish poisoning (PSP) toxins in North Atlantic right whales Eubalaena glacialis and their zooplankton prey in the Bay of Fundy. Canada. Mar. Ecol. Prog. Ser. 306, 303–313. doi: 10.3354/meps306303
Drillet, G., Rais, M., Novac, A., Jepsen, P. M., Mahjoub, M. S., and Hansen, B. W. (2015). Total egg harvest by the calanoid copepod Acartia tonsa (Dana) in intensive culture–effects of high stocking densities on daily egg harvest and egg quality. Aquac. Res. 46, 3028–3039. doi: 10.1111/are.12459
Dutz, J. (1998). Repression of fecundity in the neritic copepod Acartia clausi exposed to the toxic dinoflagellate Alexandrium lusitanicum: relationship between feeding and egg production. Mar. Ecol. Prog. Ser. 175, 97–107. doi: 10.1111/are.12459
Estrada, M. M., Sala, M. M., van Lenning, K., Alcaraz, M., Felipe, J., and Veldhuis, M. J. W. (2008). Biological interactions in enclosed plankton communities including Alexandrium catenella and copepods: role of phosphorus. J. Exp. Mar. Bio. Ecol. 355, 1–11. doi: 10.1016/j.jembe.2007.10.017
Finiguerra, M., Avery, D. E., and Dam, H. G. (2014). No evidence for induction or selection of mutant sodium channel expression in the copepod Acartia husdsonica challenged with the toxic dinoflagellate Alexandrium fundyense. Ecol. Evol. 4, 3470–3481. doi: 10.1002/ece3.1197
Fraga, S., Sampedro, N., Larsen, J., and Moestrup, Ø, and Calado, A. J. (2015). Arguments against the proposal 2302 by John & al. to reject the name Gonyaulax catenella (Alexandrium catenella). Taxon 64, 634–635. doi: 10.12705/643.15
Griffin, J. E., Park, G., and Dam, H. G. (2019). Relative importance of nitrogen sources, algal alarm cues and grazer exposure to toxin production of the marine dinoflagellate Alexandrium catenella. Harmful Algae 84, 181–187. doi: 10.1016/j.hal.2019.04.006
Grzebyk, D., Béchemin, C., Ward, C. J., Vérité, C., Codd, G. A., and Maestrini, S. Y. (2003). Effects of salinity and two coastal waters on the growth and toxin content of the dinoflagellate Alexandrium minutum. J. Plankton Res. 25, 1185–1199. doi: 10.1093/plankt/fbg088
Guillard, R. R. L., and Hargraves, P. E. (1993). Stichochrysis immobilis is a diatom, not a chrysophyte. Phycologia 32, 234–236. doi: 10.2216/i0031-8884-32-3-234.1
Guillard, R. R. L., and Ryther, J. H. (1962). Studies of marine planktonic diatoms. I. Cyclotella nana Hustedt and Detonula confervaceae (Cleve) Gran. Can. J. Microbiol. 8, 229–239. doi: 10.1139/m62-029
Hallegraeff, G. M. (1993). A review of harmful algal blooms and their apparent global increase. Phycologia 32, 79–99. doi: 10.2216/i0031-8884-32-2-79.1
Hamasaki, K., Horie, M., Tokimitsu, S., Toda, T., and Taguchi, S. (2001). Variability in toxicity of the dinoflagellate Alexandrium Tamarense isolated from Hiroshima Bay, Western Japan, as a reflection of changing environmental conditions. J. Plankton Res. 23, 271–278. doi: 10.1093/plankt/23.3.271
Ianora, A., and Miralto, A. (2010). Toxigenic effects of diatoms on grazers, phytoplankton and other microbes: a review. Ecotoxicology 19, 493–511. doi: 10.1007/s10646-009-0434-y
Ianora, A., Turner, J. T., Esposito, F., Carotenuto, Y., d’Ippolito, G., Romano, G., et al. (2004). Copepod egg production and hatching success is reduced by maternal diets of a non-neurotoxic strain of the dinoflagellate Alexandrium tamarense. Mar. Ecol. Prog. Ser. 280, 199–210. doi: 10.3354/meps280199
Jiang, X., Lonsdale, D. J., and Gobler, C. J. (2011). Rapid gain and loss of evolutionary resistance to the harmful dinoflagellate Cochlodinium polykrikoides in the copepod Acartia tonsa. Limnol. Oceanogr. 56, 947–954. doi: 10.4319/lo.2011.56.3.0947
Jiang, X., Tang, Y., Lonsdale, D. J., and Gobler, C. J. (2009). Deleterious consequences of a red tide dinoflagellate Cochlodinium polykrikoides for the calanoid copepod Acartia tonsa. Mar. Ecol. Prog. Ser. 390, 105–116. doi: 10.3354/meps08159
John, E. H., and Flynn, K. J. (2002). Modelling changes in paralytic shellfish toxin content of dinoflagellates in response to nitrogen and phosphorus supply. Mar. Ecol. Prog. Ser. 225, 147–160. doi: 10.3354/meps225147
John, U., Litaker, R. W., Montresor, M., Murray, S., Brosnahan, M. L., and Anderson, D. M. (2014). Formal revision of the Alexandrium tamarense species complex (Dinophyceae) taxonomy: the introduction of five species with emphasis on molecular-based (rDNA) classification. Protist 165, 779–804. doi: 10.1016/j.protis.2014.10.001
Juhl, A. R., Trainer, V. L., and Latz, M. I. (2001). Effect of fluid shear and irradiance on population growth and cellular toxin content of the dinoflagellate Alexandrium fundyense. Limnol. Oceanogr. 46, 758–764. doi: 10.4319/lo.2001.46.4.0758
Kozlowsky-Suzuki, B., Koski, M., Hallberg, E., Wallén, R., and Carlsson, P. (2009). Glutathione transferase activity and oocyte development in copepods exposed to toxic phytoplankton. Harmful Algae 8, 395–406. doi: 10.1016/j.hal.2008.08.025
Leong, S. C. Y., Murata, A., Nagashima, Y., and Taguchi, S. (2004). Variability in toxicity of the dinoflagellate Alexandrium tamarense in response to different nitrogen sources and concentrations. Toxicon 43, 407–415. doi: 10.1016/j.toxicon.2004.01.015
Liu, S., and Wang, W.-X. (2002). Feeding and reproductive responses of marine copepods in South China Sea to toxic and nontoxic phytoplankton. Mar. Biol. 140, 595–603. doi: 10.1007/s00227-001-0714-4
Luckas, B., Erler, K., and Krock, B. (2015). Analysis of marine biotoxins using LC-MS/MS. Methods Mol. Biol. 1308, 277–297.
Maneiro, I., Iglesias, P., Guisande, C., Riveiro, I., Barreiro, A., Zervoudaki, S., et al. (2005). Fate of domoic acid ingested by the copepod Acartia clausi. Mar. Biol. 148, 123–130. doi: 10.1007/978-1-4939-2684-8_18
Mann, K. H. (1993). Physical oceanography, food chains, and fish stocks: a review. ICES J. Mar. Sci. 50, 105–119. doi: 10.1006/jmsc.1993.1013
Mayor, D. J., Sommer, U., Cook, K. B., Davidson, R. L., and Viant, M. (2015). The metabolomic response of marine copepods to environmental warming, ocean acidification and food deprivation. Nat. Sci. Rep. 5:13690. doi: 10.1038/srep13690
Mitra, A., and Flynn, K. J. (2006). Promotion of harmful algal blooms by zooplankton predatory activity. Biol. Lett. 2, 194–197. doi: 10.1098/rsbl.2006.0447
Prevett, A., Lindström, J., Xu, J., Karlson, B., and Selander, E. (2019). Grazer-induced bioluminescence gives dinoflagellates a competitive edge. Curr. Biol. 29, R564–R565. doi: 10.1016/j.cub.2019.05.019
Prince, E. K., Lettieri, L., McCurdy, K. J., and Kubanek, J. (2006). Fitness consequences for copepods feeding on a red tide dinoflagellate: deciphering the effects of nutritional value, toxicity, and feeding behavior. Oecologia 147, 479–488. doi: 10.1007/s00442-005-0274-2
Prud’homme van Reine, W. F. (2017). Report of the nomenclature committee for algae: 15. Taxon 66, 191–192. doi: 10.12705/654.16
Roncalli, V., Cieslak, M., and Lenz, P. (2016). Transcriptomic responses of the calanoid copepod Calanus finmarchicus to the saxitoxin producing dinoflagellate Alexandrium fundyense. Sci. Rep. 6:25708. doi: 10.1038/srep25708
Runge, J. A. (1988). Should we expect a relationship between primary production and fisheries? The role of copepod dynamics as a filter of trophic variability. Hydrobiologia 167, 61–71.
Selander, E., Berglund, E. C., Engström, P., Berggren, F., Eklund, J., Harðardóttir, S., et al. (2019). Copepods drive large-scale trait-mediated effects in marine plankton. Sci. Adv. 5:eaat5096. doi: 10.1126/sciadv.aat5096
Selander, E., Kubanek, J., Hamberg, M., Andersson, M. X., Cervin, G., and Pavia, H. (2015). Predator lipids induce paralytic shellfish toxins in bloom-forming algae. Proc. Nat. Acad. Sci. U.S.A. 112, 6395–6400. doi: 10.1073/pnas.1420154112
Smayda, T. J. (1997). Harmful algal blooms: their ecophysiology and general relevance to phytoplankton blooms in the sea. Limnol. Oceanogr. 42, 1137–1153. doi: 10.4319/lo.1997.42.5_part_2.1137
Støttrup, J. G., Richardson, K., Kirkegaard, E., and Pihl, N. J. (1986). The cultivation of Acartia tonsa Dana for use as live food source for marine fish larvae. Aquaculture 52, 87–96. doi: 10.1016/0044-8486(86)90028-1
Teegarden, G. J., and Cembella, A. D. (1996). Grazing of toxic dinoflagellates, Alexandrium spp., by adult copepods of coastal Maine: implications for the fate of paralytic shellfish toxins in marine food webs. J. Exp. Mar. Bio. Ecol. 196, 145–176. doi: 10.1016/0022-0981(95)00128-X
Turner, A. D., Dhanji-Rapkova, M., Fong, S. Y. T., Hungerford, J., McNabb, P. S., Boundy, M. J., et al. (2019). Ultra-high performance hydrophilic interaction liquid chromatography with tandem mass spectrometry method for the determination of paralytic shellfish toxins and tetrodotoxin in mussels, oysters, cockles and scallops: collaborative study. J. AOAC Int. 103, 533–562. doi: 10.5740/jaoacint.19-0240
Turner, A. D., McNabb, P. S., Harwood, D. T., Selwood, A. I., and Boundy, M. J. (2015). Single-laboratory validation of a multitoxin ultra-performance LC-hydrophilic interaction LC-MS/MS method for quantitation of paralytic shellfish toxins in bivalve shellfish. J. AOAC Int. 98, 609–621. doi: 10.5740/jaoacint.14-275
Turner, J. T., and Tester, P. A. (1997). Toxic marine phytoplankton, zooplankton grazers, and pelagic food webs. Limnol. Oceanogr. 42, 1203–1214. doi: 10.4319/lo.1997.42.5_part_2.1203
Turner, J. T., Doucette, G. J., Keafer, B. A., and Anderson, D. M. (2005). ECOHAB-Gulf of Maine. Trophic accumulation of PSP toxins in zooplankton during Alexandrium fundyense blooms in Casco Bay, Gulf of Maine, April-June 1998. II. Zooplankton abundance and size-fractionated community composition. Deep Sea Res. Part II Top. Stud. Oceanogr. 52, 2784–2800. doi: 10.1016/j.dsr2.2005.06.012
Turner, J. T., Doucette, G. J., Powell, C. L., Kulis, D. M., Keafer, B. A., and Anderson, D. M. (2000). Accumulation of red tide toxins in larger size fractions of zooplankton assemblages from Massachusetts Bay, USA. Mar. Ecol. Prog. Ser. 203, 95–107. doi: 10.3354/meps203095
Turner, J. T., Hopcroft, R. R., Lincoln, J. A., Huestis, C. S., Tester, P. A., and Roff, J. C. (1998). Zooplankton feeding ecology: Grazing by marine copepods and cladocerans upon phytoplankton and cyanobacteria from Kingston Harbour, Jamaica. Mar. Ecol. Della Stn. Zool. Di Napoli I 19, 195–208. doi: 10.1111/j.1439-0485.1998.tb00462.x
Turner, J. T., Roncalli, V., Ciminiello, P., Aversano, C., Fattorusso, E., Tartaglione, L., et al. (2012). Biogeographic effects of the Gulf of Mexico ted tide dinoflagellate Karenia brevis on mediterranean copepods. Harmful Algae 16, 63–73. doi: 10.1016/j.hal.2012.01.006
Waggett, R. J., Hardison, D. R., and Tester, P. A. (2012). Toxicity and nutritional inadequacy of Karenia brevis: synergistic mechanisms disrupt top-down grazer control. Mar. Ecol. Prog. Ser. 444, 15–30. doi: 10.3354/meps09401
Wang, D.-Z. (2008). Neurotoxins from marine dinoflagellates: a brief review. Mar. Drugs 6, 349–371. doi: 10.3390/md20080016
Wohlrab, S., Iversen, M. H., and John, U. (2010). A molecular and co-evolutionary context for grazer induced toxin production in Alexandrium tamarense. PLoS One 5:e15039. doi: 10.1371/journal.pone.0015039
Xu, J., and Kiørboe, T. (2018). Toxic dinoflagellates produce true grazer deterrents. Ecology 99, 2240–2249. doi: 10.1002/ecy.2479
Xu, J., Nielsen, L. T., and Kiørboe, T. (2018). Foraging response and acclimation of ambush feeding and feeding-current feeding copepods to toxic dinoflagellates. Limnol. Oceanogr. 63, 1449–1461. doi: 10.1002/lno.10782
Keywords: phytoplankton, harmful algal bloom, egg production, paralytic shellfish poisoning, saxitoxin, copepod survival
Citation: Abdulhussain AH, Cook KB, Turner AD, Lewis AM, Bibby TS and Mayor DJ (2021) The Influence of the Toxin-Producing Dinoflagellate, Alexandrium catenella (1119/27), on the Survival and Reproduction of the Marine Copepod, Acartia tonsa, During Prolonged Exposure. Front. Mar. Sci. 8:652225. doi: 10.3389/fmars.2021.652225
Received: 12 January 2021; Accepted: 17 March 2021;
Published: 07 April 2021.
Edited by:
Stelios Katsanevakis, University of the Aegean, GreeceReviewed by:
Per Meyer Jepsen, Roskilde University, DenmarkErik Selander, University of Gothenburg, Sweden
Isabella Buttino, Higher Institute for Environmental Protection and Research (ISPRA), Italy
Copyright © 2021 Abdulhussain, Cook, Turner, Lewis, Bibby and Mayor. This is an open-access article distributed under the terms of the Creative Commons Attribution License (CC BY). The use, distribution or reproduction in other forums is permitted, provided the original author(s) and the copyright owner(s) are credited and that the original publication in this journal is cited, in accordance with accepted academic practice. No use, distribution or reproduction is permitted which does not comply with these terms.
*Correspondence: Ali H. Abdulhussain, YWhhMmcxNUBzb3Rvbi5hYy51aw==