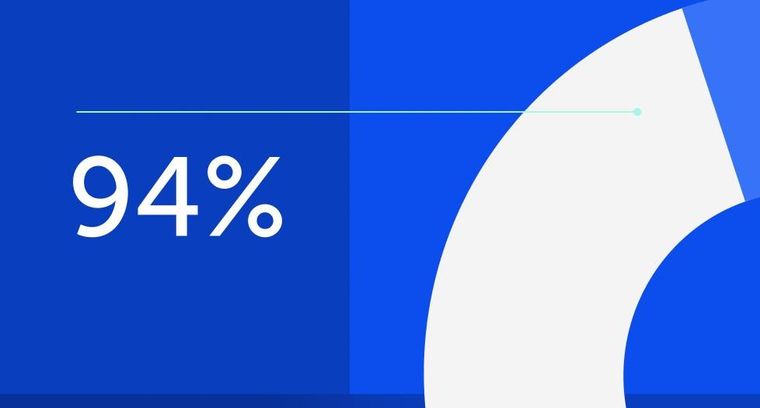
94% of researchers rate our articles as excellent or good
Learn more about the work of our research integrity team to safeguard the quality of each article we publish.
Find out more
ORIGINAL RESEARCH article
Front. Mar. Sci., 15 April 2021
Sec. Coral Reef Research
Volume 8 - 2021 | https://doi.org/10.3389/fmars.2021.651601
Mesophotic coral ecosystems (MCEs) are light-dependent coral-associated communities found at 30–150 m depth. Corals inhabiting these deeper reefs are often acclimatized to a limited and blue-shifted light environment, enabling them to maintain the relationship with their photosynthetic algal symbionts (family Symbiodiniaceae) despite the seemingly suboptimal light conditions. Among others, fluorescent proteins produced by the coral host may play a role in the modulation of the quality and spectral distribution of irradiance within the coral tissue through wavelength transformation. Here we examined the bio-optical properties and photosynthetic performances of different fluorescence morphs of two mesophotic coral species Goniopora minor and Alveopora ocellata, in order to test the photosynthesis enhancement hypothesis proposed for coral fluorescence. The green morph of G. minor and the low fluorescence morph of A. ocellata exhibit, in their natural habitats, higher abundance. The morphs also presented different spectral reflectance and light attenuation within the tissue. Nevertheless, chlorophyll a fluorescence-based, and O2 evolution measurements, revealed only minor differences between the photosynthetic abilities of three fluorescence morphs of the coral G. minor and two fluorescence morphs of A. ocellata. The fluorescence morphs did not differ in their algal densities or chlorophyll concentrations and all corals harbored Symbiodiniaceae from the genus Cladocopium. Thus, despite the change in the internal light quantity and quality that corals and their symbionts experience, we found no evidence for the facilitation or enhancement of photosynthesis by wavelength transformation.
Stony corals are sessile organisms and are considered to be highly dependent on the photosynthates derived from their algal symbionts (family Symbiodiniaceae; LaJeunesse et al., 2018) as their main energy source (Muscatine, 1990). Sustaining high rates of photosynthesis in the underwater light environment is challenging as (1) light attenuates rapidly with depth (Kirk, 2011a), (2) wave movement creates a flickering effect that causes rapid and extreme localized changes in light intensity (Walsh and Legendre, 1983), (3) the macro-structure of the reef may shade coral colonies (Stimson, 1985; Kaniewska et al., 2008), and (4) certain substrates may be highly reflective consequently contributing to downwelling irradiance (Kirk, 2011b). Scleractinian corals in mesophotic coral ecosystems (MCEs; 30–150 m) experience light intensities that are up to 99% lower than those experienced by their shallow counterparts (0–30 m; Kahng and Kelley, 2007; Lesser et al., 2009; Tamir et al., 2019). Additionally, MCEs are also exposed to a restricted light spectrum centered around the blue region of the spectrum (Jerlov, 1968; Kahng et al., 2019). Coral photoacclimatization to MCEs is manifested at the coral-host level as changes in colony morphology (Kaniewska et al., 2008; Nir et al., 2011), skeletal features that modify the internal light environment (Enríquez et al., 2005; Kahng et al., 2012), changes in the populations of endosymbiotic Symbiodiniaceae expressed as a shift in their genetic identity (Cooper et al., 2011; Bongaerts et al., 2013; Einbinder et al., 2016) and modifications in the composition of photosynthetic pigments and structure of the photosynthetic complex (Einbinder et al., 2016). These changes, among others, potentially assist corals in maintaining a successful symbiosis with Symbiodiniaceae. Accordingly, deeper corals will usually present a higher maximal quantum yield of photosystem II (Einbinder et al., 2016; Ben-Zvi et al., 2020), a lower Symbiodiniaceae density accompanied by higher chlorophyll concentration within algal cells (Mass et al., 2007), and a reduced capacity to manage excess light (Einbinder et al., 2016; Ben-Zvi et al., 2020). Additionally, mesophotic corals may rely more on heterotrophy rather than autotrophy as their main strategy for acquiring energy (Mass et al., 2007; Lesser et al., 2010).
Corals are recognized for being extremely colorful under short-wavelength lighting conditions, which is attributed to the phenomenon of fluorescence (Kawaguti, 1944; Matz et al., 1999). Fluorescence refers to the conversion of light wavelength, usually from short wavelengths into longer ones. In corals, fluorescence results from proteins belonging to the green fluorescent protein (GFP)-like family, that are produced by the coral host. The fluorescent proteins (FPs) may exhibit a diversity of excitation and emission peaks (Alieva et al., 2008), and an individual coral may possess a single or multiple FPs (Dove et al., 2001; Ben-Zvi et al., 2014, 2019; Eyal et al., 2015). Fluorescence polymorphism within the same coral species has been previously described as resulting either from a difference in the expression levels of a single protein (Gittins et al., 2015; Takahashi-Kariyazono et al., 2018), or the presence of different FPs (Eyal et al., 2015; Ben-Zvi et al., 2019).
One of the suggested functional roles for coral fluorescence is that of photosynthesis enhancement. Earlier studies have contended that fluorescence may enhance photosynthesis where light is limited, as in deeper habitats, by conversion of short wavelengths into longer wavelengths, capable of absorption by the photosynthetic pigments of the algal symbiont using host associated cyan FPs (Schlichter et al., 1986, 1994; Schlichter and Fricke, 1990). However, this notion is not fully supported since a conversion of blue light, which is abundant in deeper habitats, usually results in green wavelengths that are the least efficient for photosynthesis in Symbiodiniaceae (Scott and Jitts, 1977; Kühl et al., 1995). Another mechanism for photosynthesis enhancement was suggested to rely on electron transport through fluorescence resonance energy transfer (FRET) which depends on a close proximity between the donor of electrons and the recipient (Förster, 1955). Despite some evidences indicating that FRET is occurring between FPs (Cox et al., 2007), there is currently a lack of sufficient support for electron transfer between FPs and the photosynthetic apparatus (Gilmore et al., 2003; Cox and Salih, 2005). Nevertheless, recent studies suggest that FPs may alter (by wavelength conversion) and disperse (by scattering) the available light more efficiently through the tissue, enabling it to reach the Symbiodiniaceae residing in the deeper tissue layers of the coral host (Smith et al., 2017), and that symbiotic algae are attracted to green light (Hollingsworth et al., 2005) and green fluorescence (Aihara et al., 2019). The enhanced reflectance and scattering of light are also supported by direct measurements and is correlated with higher FP fluorescence (Salih et al., 2000; Lyndby et al., 2016).
Thus far, hypotheses regarding the role of coral fluorescence have mostly been tested on shallow corals (DeSalvo et al., 2008; Dove et al., 2008; D’Angelo et al., 2012) and among coral morphs that display different expression levels of the same FP but not among morphs that differ in the FPs they contain (Gittins et al., 2015; Roth et al., 2015). In MCEs, the phenomenon of fluorescence polymorphism is common and multiple species can exhibit several color morphs occurring side by side at the same site and depth (Eyal et al., 2015; Ben-Zvi et al., 2019).
Here we sought to investigate the photosynthetic and bio-optical properties of different fluorescence morphs of two mesophotic coral species which differ either in the levels of expression (i.e., Alveopora ocellata) or by the emission peak of their FPs (i.e., Goniopora minor), in order to determine the potential links between the coral host’s fluorescent pigments and coral photosynthesis, in the unique mesophotic environment.
Ten colonies of the mesophotic scleractinian coral A. ocellata were collected from the reef in front of the Interuniversity Institute for Marine Sciences in Eilat (IUI; 29°30′16″N, 34°55′7″E) and seven colonies of G. minor were collected from the Dekel Beach site (29°32′17″N, 34°56′56″E), Gulf of Eilat/Aqaba (GoE/A), northern Red Sea. All corals were collected at 45 m depth using open-circuit technical diving. Corals were transferred to a running seawater system at the IUI in dark containers and were subsampled and preserved by dipping the fragments in liquid nitrogen and storing them at −80°C until analyses (for Symbiodiniaceae density and genetic identification, and chlorophyll concentration analyses). The remaining corals (used for the scalar irradiance, chlorophyll fluorescence, and O2 evolution measurements) were kept for further analyses under a lighting filter (“Lagoon blue,” Lee Filters, United States) providing a light regime similar to that of the natural mesophotic reefs at Eilat (Dishon et al., 2012; Ben-Zvi et al., 2021).
Eight line transects (each 10 m long) were deployed at 45 m depth at each collection site (i.e., IUI and Dekel Beach; total of 160 m). Each colony crossed by the transect was classified as either a high or low fluorescence morph (for A. ocellata), or as green, yellow, or red morph (for G. minor), based on their appearance under ambient light. A given morph’s abundance was calculated by dividing the number of colonies from each morph by the total number of colonies from the specific species it belongs to, that were found along the transect.
Representative fragments from each morph were imaged with a SONY RX100 camera under white illumination (for the non-fluorescent images) and under a blue excitation light source (BlueStar, NightSea, United States) and a commercial yellow barrier filter (Y12, Tiffen, United States) mounted on the camera (for the fluorescent images). Host fluorescence was excited by a light source peaking at 450 nm (BlueStar, NightSea, United States) positioned horizontally to the coral colony and emission spectra were recorded with a flat cut 600 μm core UV-Visible fiber (QP600-2-UV-VIS, Ocean Optics, United States) equipped with a long pass barrier filter (cut-off <500 nm) positioned at a 45° angle to the excitation light and connected to a spectrometer (JAZ, Ocean Optics, United States).
G. minor fragments were placed in a black acrylic flow chamber supplied with fresh seawater. Incident irradiance was provided by a tungsten halogen lamp (Schott ACE 1, Germany) equipped with a collimating lens. Measurements (n = 3 for each morph) of scalar irradiance (E0) were collected using an 80 μm spherical light microprobe (Zenzor, Denmark) connected to a spectrometer (AvaSpec-UL2048XL, Avantes, United States). The microprobe was oriented at 45° relative to the vertical incident light and carefully positioned above the coral polyp mouth (Wangpraseurt et al., 2012). Light gradients were measured through the coral gastrovascular cavity until reaching the skeleton at 100 μm increments, using a computer-controlled micromanipulator (Wangpraseurt et al., 2012). The scalar irradiance measurements were normalized to the downwelling spectral irradiance (Ed) provided by the collimated light, measured from a non-reflective black surface (Wangpraseurt et al., 2012). Integrated photon irradiance was calculated individually for wavelengths of interest (i.e., around the fluorescence emission peaks measured for each fluorescence morph; 500–530 nm, 530–560 nm, and 560–590 nm) by calculating the area under the curve of E0 and Ed, using the “MESS” package in R software (R Core Team, 2013) and equation 1, where λa and λb are the wavelengths of interest:
We used an imaging-pulse amplitude modulation fluorometer (imaging-PAM; blue maxi-version Walz GmbH, Germany) to perform rapid light curves (RLCs) and measure photosystem II (PSII) chlorophyll fluorescence on the intact corals. The photosynthetic quantum yield of PSII (ΦPSII), relative electron transport rate (rETR) and non-photochemical quenching (NPQ) were calculated following Kramer et al. (2004) as:
Where Fm′ is the steady-state maximal fluorescence yield, PAR is the photosynthetically active radiation, F0 is the fluorescence yield, Fm is the maximal fluorescence followed by a saturating pulse, and qL is the fraction of open PSII centers. It should be noted that rETR calculation is based on the PAR absorbance (0.84) and photosystems ratio (1:1) of terrestrial plants (Björkman and Demmig, 1987), as it has not yet been determined for the selected coral species in this study.
Following a 30 min dark incubation, measurements were taken using a saturation pulse intensity of 2,700 μmol photons m–2 s–1 for 800 ms after 2.5 min incubation at each light intensity (0, 20, 55, 110, 185, 280, 335, 395, 460, 530, 610, and 700 μmol photons m–2 s–1). The following PAM settings were used: measuring light intensity = 1, measuring light frequency = 1, actinic light intensity = 1, gain = 1. The initial slope (α), relative maximal electron transport rate (rETRmax), and minimum saturating irradiance (Ek) were calculated from a fitted double exponential decay function following Platt et al. (1980).
Coral fragments were incubated in 85 mL acrylic closed jacket respiration metabolic chambers containing 0.45 μ filtered seawater. O2 concentration inside the experimental chambers was measured using a FireSting pro meter (FSPRO-4, Pyroscience, Germany) connected to fiber-optic oxygen sensors (OPROB3, Pyroscience, Germany) placed at the top of each chamber, calibrated using 1-point calibration of 100% O2 saturated water. Photosynthesis-irradiance (P-E) curves were performed by incubating coral fragments for 10 min under increasing light intensities (0, 5, 23, 36, 50, 78, 150, 250, 350, 450, and 550 μmol photons m–2 s–1). The incident downwelling irradiance was provided by a computer-controlled array of light-emitting diodes (LEDs) measured with a LI-1400 light meter (LI-COR, United States) equipped with a cosine-corrected quantum sensor (LI-190R, LI-COR, United States). The same procedure was also performed for blue light illumination with the LED array covered with a spectral filter (“lagoon blue,” Lee Filters, United States) mimicking the light spectrum at mesophotic reefs (45 m) in Eilat (Ben-Zvi et al., 2021). During measurements, the experimental water was constantly stirred and kept at 25°C as measured by a temperature probe (TDIP15, Pyroscience, Germany). O2 concentrations were normalized to coral surface area that was determined from top-view images and to the volume of water in the chambers. Net and gross photosynthesis were fitted using a double exponential decay following Platt et al. (1980), and α, maximal photosynthesis rate (Pmax), compensation irradiance (Ec), Ek, and dark respiration (Rd) were extracted from the fitted models.
Frozen fragments of G. minor and A. ocellata were thawed and tissue was removed in the presence of 0.22 μ filtered seawater using an artist’s air brush into 50 ml tubes. Surface area was determined using the single dip wax method for normalization (Veal et al., 2010). Coral tissue was mechanically broken using a motorized homogenizer and centrifuged at 5,000 rpm for 5 min to separate the host (i.e., coral) supernatant from the algal pellet. The host fraction was discarded, and the algal pellet was used for the quantification of chlorophyll and Symbiodiniaceae cell density. A small subsample from each algal pellet was taken for Symbiodiniaceae genetic identification. Algal cells were counted in triplicates using a hemocytometer under a light microscope. The remaining algal pellet was used for chlorophyll analysis. Chlorophyll was extracted in the presence of 100% cold acetone for 15 h at 4°C and chlorophyll a and c2 concentrations (pg chlorophyll cell–1) were determined spectrometrically as in Jeffrey and Humphrey (1975).
DNA was extracted using the DNeasy Blood and Tissue kit (Qiagen, Germany) from the Symbiodiniaceae sub-samples following the manufacturer’s protocol. A ∼700 bp sequence fragment of the internal transcribed spacer 2 (ITS2) was PCR amplified using the primers SYM_VAR_FWD and SYM_VAR_REV following the procedure of Hume et al. (2013). PCR products were purified by ExoSAP-IT (Thermo Fisher Scientific, United States) and bi-directionally sequenced. Individual sequences were aligned using Geneious, and a consensus sequence was constructed for comparison against the GeoSymbio database (Franklin et al., 2012).
All statistical analyses were performed using R software (R Core Team, 2013). Data were tested for normality using the Shapiro–Wilk test and homogeneity of variance with Levene’s test. G. minor data was tested using PERMANOVA (for repeated measure ANOVA in the light curves analyses), ANOVA (for normally distributed data), or permutational ANOVA when data did not follow a normal distribution. A. ocellata data was tested with PERMANOVA (for repeated measure ANOVA in the light curves analyses), t-tests (for normally distributed data) or Wilcoxon signed-rank test (for non-normal data). Results were considered significant if p < 0.05. Where appropriate, data were further examined using a Tukey’s post hoc test.
Fluorescence morphs of A. ocellata and G. minor visually differ from one another under white illumination, under blue light illumination, and in their fluorescence emission peak (λem; Figure 1). Three distinct fluorescence morphs are described for G. minor; a red morph (Figure 1A; λem = 580 nm), a green morph (Figure 1B; λem = 515 nm), and a yellow morph (Figure 1C; λem = 515 and 545 nm). Two fluorescence morphs are described for A. ocellata: a low fluorescence morph (Figure 1D; λem = 520 nm) and a high fluorescence morph (Figure 1E; λem = 520 nm), both of which present the same green emission peak, with the distinction that the low fluorescence morph appears red under both illuminations due to chlorophyll fluorescence of its symbionts (λem = 680 nm). The green morph of G. minor presents the typical, brownish color of corals under white light (Figure 1B, top image), commonly attributed to the symbiotic algae, yet exhibits a green fluorescence under the excitation light (Figure 1B, middle image); while the yellow morph displays a yellowish appearance under white light illumination (Figure 1C, top image) and exhibits a bright green glow under blue illumination (Figure 1C, middle image).
Figure 1. Fluorescence diversity of mesophotic corals. Representative non-fluorescent images (top image in each panel); fluorescent images (middle image in each panel); and fluorescence emission spectra of Goniopora minor (A–C) and Alveopora ocellata (D,E) fluorescence morphs. Fluorescence emission peaks are indicated by arrows.
For G. minor, out of 63 surveyed colonies, the green morph was more prevalent compared to the yellow morph (74 ± 14.7% and 26 ± 14.7%, respectively). The red morph of G. minor was extremely rare and was not crossed in our field surveys. For A. ocellata, we found that out of 40 colonies, 84 ± 17.4% (mean ± SD) presented low fluorescence and only 15.15 ± 17.43% of them displayed high fluorescence.
Scalar irradiance (E0) measurements revealed a strong light gradient within the coral tissue (Figure 2 and Supplementary Figure 1). At the tissue surface, irradiance is 1.5-fold higher than the incident irradiance for visible light (PAR; 400–700 nm) and at the tissue-skeleton interface, available light is greatly reduced to 50% of the incident light for PAR. While this surface light enhancement is prominent between 400 and 700 nm for the yellow and green morphs, it only occurs above 580 nm for the red morph (the λem of this morph). Light absorbance by photosynthetic pigments (i.e., chlorophyll a) can be observed as a drop around 680 nm in all fluorescence morphs, and in the yellow morph the contribution of the host fluorescent proteins can be observed as a shoulder between 500 and 600 nm. The scattering of light at wavelengths above 700 nm was found to be 11–14% higher in the green morph compared to the other morphs. Comparing the integrated photon irradiance (Figure 2B) revealed significant differences in all morphs between the two areas in which the measurement was taken (i.e., tissue surface and tissue-skeleton interface; permutational ANOVA, p < 0.0001). When examining the differences among morphs within each location we found that the yellow morph differed from the green and red morphs in the surface measurements (permutational ANOVA, p < 0.01), while the green and red morphs did not (permutational ANOVA, p = 0.71). Near the skeleton the green morph differed form the two other morphs (permutational ANOVA, p < 0.05) while the red and yellow did not (permutational ANOVA, p = 0.89).
Figure 2. In vivo spectral scalar irradiance of three fluorescence morphs of the mesophotic coral Goniopora minor. (A) Scalar irradiance (E0) was measured at the coral tissue surface (solid lines) and at the tissue-skeleton interface (dotted lines) of red (colored red), green (colored green), and yellow (colored yellow) fluorescence morphs of the mesophotic coral G. minor. Dashed black line indicates 100% of the incident irradiance (Ed). Colored lines represent the mean relative scalar irradiance (n = 3) of each morph and confidence intervals are represented by transparent corresponding areas. Fluorescence emission peaks are indicated by arrows. The solid box indicates an area of interest corresponding to (B) the integrated photon irradiance (n = 3) for specific wave bands (500–530 nm, 530–560 nm, and 560–590 nm) for the three fluorescence morphs. Boxes represent the upper and lower quartile, center lines represent medians, and whiskers extend to data measurements that are less than 1.5∗IQR (interquartile range) away from first/third quartile.
Relative electron transport rate values did not differ significantly between morphs of A. ocellata (Figures 3A,C; PERMANOVA, F = 0.85, p = 0.37) or G. minor (PERMANOVA, F = 0.41, p = 0.7). In A. ocellata, we found a significant effect of the fluorescence morph on ΦPSII (Figure 3B; PERMANOVA, F = 5.68, p = 0.04), being higher in the low fluorescence morph, while in G. minor it was found to be lowest in the green morph (Figure 3E; PERMANOVA, F = 58.13, p = 0.01). The maximum quantum yield of PSII (Fv/Fm; or ΦPSII measured after a dark incubation) was found to be similar among the fluorescence morph of A. ocellata (Figure 3B and Supplementary Figure 3A; t-test, t = −1.37, p = 0.18) and among morphs of G. minor (Figure 3E and Supplementary Figure 2B; permutational ANOVA, p = 0.18).
Figure 3. Rapid light curves of two mesophotic coral species. Fitted (solid lines) and mean (circles) ± SE (error bras) of rETR values (A,D), effective quantum yield [ΦPSII; (B,E)], and non-photochemical quenching [NPQ; (C,F)] of high fluorescence (dark green; n = 5) and low fluorescence (light green; n = 5) morphs of Alveopora ocellata (A–C), and green (green; n = 2), red (red; n = 1), and yellow (yellow; n = 2) fluorescence morphs of Goniopora minor (D–F).
Non-photochemical quenching values were also similar between morphs of A. ocellata (Figure 3C; PERMANOVA, F = 0.1, p = 0.74), and G. minor (Figure 3F; PERMANOVA, F = 0.06, p = 0.09).
The initial slope (α), and relative maximal electron transport rate (rETRmax) calculated from the RLC were found to be similar among A. ocellata morphs (Supplementary Table 1; t-test, t = −0.05, p = 0.96 and t = −0.38, p = 0.71, respectively), while the minimum saturating irradiance (Ek) was slightly higher in the high fluorescence morph (t-test, t = −2.17, p = 0.06). In G. minor, the fluorescence morph had no significant effect on any of the parameters (Supplementary Table 1; permutational ANOVA, p < 0.05).
O2 evolution differed when measured under white or blue illuminations (Supplementary Figure 3, Table 1, and Supplementary Table 2; PERMANOVA, p = 0.03). Since P-E curves performed under blue illumination resulted in fully extended polyps, smoother curves (i.e., higher R2), and had been previously suggested to be more appropriate for mesophotic corals (Mass et al., 2010), we present in Figure 4 and Supplementary Figure 4 measurements, and in Table 1 parameters derived from the curves performed under blue light (parameters derived from the white illuminated curves can be found in Supplementary Table 2).
Table 1. Photosynthetic parameters derived from photosynthesis-irradiance (P-E) curves under blue illumination.
Figure 4. Net photosynthesis of two mesophotic corals fluorescence morphs. Fitted (solid lines) and mean (circles) ± SE (error bars) values of O2 evolution (μmol O2 cm–2 hr–1) measured under blue light at increasing intensities (0, 5, 23, 36, 50, 78, 150, 250, 350, 450, and 550 μmol photons m–2 s–1) of Alveopora ocellata [(A), n = 1 and 4 for high and low fluorescence morphs, respectively] and Goniopora minor [(B), n = 2, 1, and 3 for green, red, and yellow morphs, respectively].
We did not find differences in the P-E derived parameters between species (permutational ANOVA, p > 0.05) or between A. ocellata and G. minor morphs (t-test for A. ocellata or permutational ANOVA for G. minor, p > 0.05).
Symbiodiniaceae density and chlorophyll (a and c2) concentrations did not significantly differ between species (Figure 5; permutational ANOVA, p < 0.05). The mean (±SD) Symbiodiniaceae density was 2.6 × 107 ± 1.4 × 107 cells cm–2. Moreover, we did not find a significant effect of the fluorescence morph, of both species, on Symbiodiniaceae density (Figure 5A, t-test, t = −0.54, p = 0.6, and permutational ANOVA, p = 0.88 for A. ocellata and G. minor, respectively), chlorophyll a concentration (Figure 5B; Wilcoxon test, W = 7, p = 0.35 and ANOVA, F = 0.53, p = 0.61 for A. ocellata and G. minor, respectively), and chlorophyll c2 (Figure 5C; Wilcoxon test, W = 7, p = 0.35 and ANOVA, F = 0.86, p = 0.47 for A. ocellata and G. minor, respectively).
Figure 5. Photobiology of fluorescence morphs of the mesophotic corals Alveopora ocellata and Goniopora minor. Areal Symbiodiniaceae density (A), cellular chlorophyll a (B), and cellular chlorophyll c2 (C) of low fluorescence (LF) and high fluorescence (HF) morphs of A. ocellata and green, yellow, and red fluorescence morphs of G. minor. Boxes represent the upper and lower quartile, center lines represent medians, and whiskers extend to data measurements that are less than 1.5*IQR away from first/third quartile. Outliers are represented by dots. Sample size (n) is indicated below each box.
Genetic identification based on the ITS2 region revealed that all our coral samples harbored Symbiodiniaceae from the genus Cladocopium (formerly known as clade C; LaJeunesse et al., 2018), regardless of species or fluorescence morph. Based on the ITS2 sequences we found that A. ocellata harbored Cladocopium types C3.10 (n = 7), C101 (n = 1), C3 (n = 1), and C66b (n = 1), and G. minor harbored types C1 (n = 4), C78 (n = 1), C40 (n = 1), and C3 (n = 1). We found no effect of the fluorescence morph on Symbiodiniaceae types.
The spectral analyses revealed a range of fluorescence emission peaks for two mesophotic coral species (Figure 1). While, A. ocellata presented one fluorescence emission peak (at 520 nm), G. minor presented three (515, 545, and 580 nm). In the GoE/A, A. ocellata mostly inhibits mesophotic depths (Eyal-Shaham et al., 2016), whereas G. minor is a depth generalist and can be found also in the shallower parts of the reefs (Tamir et al., 2019). The differences in the zonation characteristics of these species may explain why G. minor possesses a range of FPs. A broader FPs arsenal covers a broader spectrum of emission peaks which potentially correspond to a wider range of excitations that may mediate excess light (at shallow depths) or provide wavelengths that are absent (at mesophotic depths). A. ocellata, presents only one fluorescence emission which will have a narrower excitation range. Field surveys revealed that for A. ocellata the low fluorescence morph was the dominant one, while for G. minor the dominant morph was the green one. Despite the reported higher abundance of red FPs in deeper habits compared to shallower ones, and their suggested advantage in the dispersal of light deeper into the coral tissue (Smith et al., 2017), the red morph of G. minor is extremely rare.
Host fluorescence is known to play a critical role in the modification of irradiance intensity and the spectral tuning of in-hospite irradiance environment (Salih et al., 2000; Mazel and Fuchs, 2003; Wangpraseurt et al., 2012; Lyndby et al., 2016; Smith et al., 2017; Quick et al., 2018; Wangpraseurt et al., 2019; Bollati et al., 2020). Our light microsensors measurements indicate that the optical environment within the coral tissue is influenced by the presence of different FPs (Figure 2 and Supplementary Figure 1). Despite the bright appearance of G. minor under white light (in the red and yellow morph; Figures 1A,C) and under blue excitation light (all morphs), the contribution of the FPs to the spectral signature of the morphs was not as strong as expected and previously documented (Mazel and Fuchs, 2003; Wangpraseurt et al., 2012). This may be explained by the light source used for these measurements which is poor in blue, FP-exciting photons, or by the absorbance of light by the photosynthetic pigments sharing these emission peaks of host fluorescence. For example, the yellow morph of G. minor has a fluorescence emission peak at 545 nm (Figure 1C), while peridinin has an absorbance peak at 540 nm (Niedzwiedzki et al., 2014). Additionally, coral-associated cyanobacteria may also contain phycoerythrin with absorption bands around 505 and 571 nm (Lesser et al., 2004), which correspond to the emission peaks of several FPs and may confound the interpretation of fluorescence spectra. Nonetheless, the in-hospite irradiance differed among the morphs (Figure 2). The yellow morph presented the greatest scalar irradiance enhancement at the shorter wavelengths (i.e., 400–700 nm), and the green morph showed greater light enhancement at longer wavelengths (700–800 nm). The dominance of certain morphs over others and the differences found in the internal light environment within the coral tissue, led us to hypothesize that certain FPs may be advantageous for photosynthesis within the mesophotic light environment.
Chlorophyll fluorescence-based measurements revealed that A. ocellata and G. minor fluorescence morphs mostly did not differ in the quantum yield of PSII (Figure 3A,B and Supplementary Figure 2). Likewise, Roth et al. (2015), did not find differences in ΦPSII between high and low fluorescence morphs of Leptoseris spp. at mesophotic depths. Although it has been shown that changes in the in-hospite irradiance environment can affect absolute electron transport rates in corals (Wangpraseurt et al., 2014), we found no differences in rETR values among the examined morphs (Figures 3A,D) despite an enhanced irradiance measured within the tissue of G. minor’s yellow morph (Figure 2). When the photoprotective role of FPs was examined in mesophotic Euphyllia paradivisa, no differences in the amount of UVR-induced DNA damage were found between fluorescence morphs (Ben-Zvi et al., 2019). However, this does not exclude the putative photoprotective role of FPs as NPQ values were slightly (but not significantly) higher for the red and yellow morphs of G. minor compared to the green morph (Figure 3F), indicating their potentially greater capability in mediating excess light and preventing damage to the photosynthetic apparatus.
Respiration and photosynthesis data from mesophotic corals are very limited and there is currently no agreed protocol for performing these measurements. Although Mass et al. (2010) provided evidence for the chromatic dependence of photosynthetic performance on the light provided during measurements, ex-situ measurements on mesophotic corals are still commonly performed under white light (Cooper et al., 2011; Ben-Zvi et al., 2020). Since corals are known to photoacclimatize to their natural light conditions, mesophotic corals are most likely acclimatize to blue light, as this is the prominent wavelength at depths of 30–150 m (Kahng et al., 2019). Moreover, the wavelength-dependent absorption cross-section for Symbiodiniaceae has already been established as being more efficient at shorter wavelengths than at longer ones (Szabó et al., 2014). Furthermore, as corals FPs are largely excited by blue light, the FPs will in turn affect the internal light environment. We therefore compared measurements taken under white and blue light (Supplementary Figure 3 and Supplementary Table 2) and indeed found differences in coral response. Under blue light corals expanded their tentacles, while under white light they contracted them. Conducting the metabolic measurements under blue light resulted in smoother P-E curves (Supplementary Figure 3), which may represent a more natural performance of mesophotic corals. Most of the previously reported values for P-E derived parameters that we were able to compare to our measurement aligned with the current results (Cooper et al., 2011; Nir et al., 2014; Eyal et al., 2019). The results indicate that mesophotic corals usually present relatively low compensation irradiance ranging between 15 and 96 μmol photons m–2 s–1 as well as low saturating irradiances, ranging between 28 and 80 μmol photons m–2 s–1 leaving a narrow window of light intensities which enable photosynthesis.
Klueter et al. (2006) found that a highly fluorescent morph of Montipora digitata had higher Symbiodiniaceae densities and chlorophyll a concentration compared to a low fluorescent morph. We examined the Symbiodiniaceae densities as well as chlorophyll a and c2 concentrations in all our studied morphs but found no differences among our samples (Figure 5). Our measured values of Symbiodiniaceae density are higher than previously reported values in mesophotic corals (Bongaerts et al., 2011; Cooper et al., 2011), however this parameter can greatly vary between species, depth, and light availability [reviewed by Roth (2014)]. Chlorophyll concentration values determined in this study, align with previously reported values (Lesser et al., 2010; Cooper et al., 2011; Eyal et al., 2019). The latter result also indicates that the brighter color of the yellow morph of G. minor is probably the result of a higher expression of FPs rather than a low algal density or low chlorophyll concentration. Similarly, in A. ocellata, the red/brown appearance of the low fluorescence morph compared to the green appearance of the high fluorescence morph may also be a result of a higher concentrations of the host FP and not of a change in the algal symbionts.
Aihara et al. (2019) demonstrated that coral fluorescence may serve as an attractive signal for symbiotic algae, and specific Symbiodiniaceae genera/species were found to be correlated with “redder” juveniles of Acropora millepora (Quigley et al., 2018). We therefore sought to determine whether a specific fluorescent signal would indeed attract symbionts that differ genetically. The genetic identity of Symbiodiniaceae revealed no significant effect of the fluorescence morph, and all corals harbored Symbiodiniaceae from the genus Cladocopium, as previously described in other coral species at the mesophotic reefs of the GoE/A (Nir et al., 2011; Einbinder et al., 2016; Eyal et al., 2019), as well as at other mesophotic reefs worldwide (Ziegler et al., 2015; Goulet et al., 2019). We therefore conclude that despite the possibly of serving as general Symbiodiniaceae attractant, specific fluorescence emissions do not attract specific Symbiodiniaceae genotypes. Since all the species and morphs we examined share the same habitat and are found in close proximity to each other, and hence experience similar environmental conditions, there may not be an apparent reason to attract different symbionts, which may or may not have an advantage in their photosynthetic performances or in their tolerance to stressors, such as temperature.
In this study, we investigated the bio-optical properties and photosynthetic performances of mesophotic corals exhibiting different host fluorescence emissions resulting from differential expression of the same FP (A. ocellata) or multiple FPs (G. minor). Our results, showing only negligible differences in the photobiology of the different coral fluorescence morphs, do not support any of the prevailing mechanisms that have been suggested to enhance photosynthesis in coral by means of FPs in deeper habitats. Nevertheless, the bio-optical properties revealed changes among morphs, indicating that fluorescence may mediate the internal light environment of corals, potentially affecting other aspects of coral physiology through cellular mechanisms that are light-regulated such as circadian clocks entrainment (Levy et al., 2007), spawning (Sweeney et al., 2011), or growth (Roth et al., 1982). Future research should focus on depicting the pathways, such as gene regulation and expression, by which the effect of the diverse internal light regimes found among morphs may play a significant role.
The raw data supporting the conclusions of this article will be made available by the authors, without undue reservation.
OB-Z and YL conceived the study. GE and OB-Z collected the coral samples and conducted the field surveys. DW performed the bio-optical measurements. OB-Z performed all other analyses, visualized all data, and wrote the first draft of the manuscript. OB-Z, DW, and OB analyzed the data. All authors reviewed, commented, and approved the manuscript.
This research was funded by the Israel Science Foundation (ISF) grant agreement No. 1191/16 to YL, Ministry of Science, Technology and Space doctoral fellowship grant agreement No. 3-18487 to OB-Z, Assemble Plus Consortium grant to DW, and European Union’s Horizon 2020 Research and Innovation Program under the Marie Skłodowska-Curie grant agreement No. 796025 to GE.
The authors declare that the research was conducted in the absence of any commercial or financial relationships that could be construed as a potential conflict of interest.
We would like to thank O. Levy for the use of the Imaging-PAM and oxygen evolution measurement system, T. Treibitz for the use of the JAZ system, and N. Paz for editing the manuscript. We would also like to thank the Interuniversity Institute for Marine Sciences in Eilat and its staff for making their facilities available for us and for all the YL group for their ongoing support.
The Supplementary Material for this article can be found online at: https://www.frontiersin.org/articles/10.3389/fmars.2021.651601/full#supplementary-material
Aihara, Y., Maruyama, S., Baird, A. H., Iguchi, A., Takahashi, S., and Minagawa, J. (2019). Green fluorescence from cnidarian hosts attracts symbiotic algae. Proc. Nat. Acad. Sci. U.S.A. 116, 2118–2123. doi: 10.1073/pnas.1812257116
Alieva, N. O., Konzen, K. A., Field, S. F., Meleshkevitch, E. A., Hunt, M. E., Beltran-Ramirez, V., et al. (2008). Diversity and evolution of coral fluorescent proteins. PLoS One 3:e2680. doi: 10.1371/journal.pone.0002680
Ben-Zvi, O., Eyal, G., and Loya, Y. (2014). Light-dependent fluorescence in the coral Galaxea fascicularis. Hydrobiologia 759, 15–26. doi: 10.1007/s10750-014-2063-6
Ben-Zvi, O., Eyal, G., and Loya, Y. (2019). Response of fluorescence morphs of the mesophotic coral Euphyllia paradivisa to ultra-violet radiation. Sci. Rep. 9:5245. doi: 10.1038/s41598-019-41710-3
Ben-Zvi, O., Ofer, E., Eyal, G., and Loya, Y. (2021). Experimental evidence of temperature-induced bleaching in two fluorescence morphs of a Red Sea mesophotic coral. Coral Reefs 40, 187–199. doi: 10.1007/s00338-020-02027-0
Ben-Zvi, O., Tamir, R., Keren, N., Tchernov, D., Berman-Frank, I., Kolodny, Y., et al. (2020). Photophysiology of a mesophotic coral 3 years after transplantation to a shallow environment. Coral Reefs 39, 903–913. doi: 10.1007/s00338-020-01910-0
Björkman, O., and Demmig, B. (1987). Photon yield of O2 evolution and chlorophyll fluorescence characteristics at 77K among vascular plants of diverse origins. Planta 170, 489–504. doi: 10.1007/BF00402983
Bollati, E., D’angelo, C., Alderdice, R., Pratchett, M., Ziegler, M., and Wiedenmann, J. (2020). Optical feedback loop involving dinoflagellate symbiont and scleractinian host drives colorful coral bleaching. Curr. Biol. 30, 2433–2445.e3. doi: 10.1016/j.cub.2020.04.055
Bongaerts, P., Riginos, C., Hay, K. B., Van Oppen, M. J., Hoegh-Guldberg, O., and Dove, S. (2011). Adaptive divergence in a scleractinian coral: physiological adaptation of Seriatopora hystrix to shallow and deep reef habitats. BMC Evol. Biol. 11:303. doi: 10.1186/1471-2148-54011-303
Bongaerts, P., Frade, P. R., Ogier, J. J., Hay, K. B., Van Bleijswijk, J., Englebert, N., et al. (2013). Sharing the slope: depth partitioning of agariciid corals and associated Symbiodinium across shallow and mesophotic habitats (2-60 m) on a Caribbean reef. BMC Evol. Biol. 13:205. doi: 10.1186/1471-2148-13-205
Cooper, T. F., Ulstrup, K. E., Dandan, S. S., Heyward, A. J., Kühl, M., Muirhead, A., et al. (2011). Niche specialization of reef-building corals in the mesophotic zone: metabolic trade-offs between divergent Symbiodinium types. Proc. Royal Soc. B 278, 1840–1850. doi: 10.1098/rspb.2010.2321
Cox, G., Matz, M., and Salih, A. (2007). Fluorescence lifetime imaging of coral fluorescent proteins. Microsc. Res. Tec. 70, 243–251. doi: 10.1002/jemt.20410
Cox, G., and Salih, A. (2005). “Fluorescence lifetime imaging of symbionts and fluorescent proteins in reef corals,” in Biomedical Optics 2005: International Society for Optics and Photonics, (San Jose, CA: SPIE), 162–170. doi: 10.1117/12.600708
D’Angelo, C., Smith, E., Oswald, F., Burt, J., Tchernov, D., and Wiedenmann, J. (2012). Locally accelerated growth is part of the innate immune response and repair mechanisms in reef-building corals as detected by green fluorescent protein (GFP)-like pigments. Coral Reefs 31, 1045–1056. doi: 10.1007/s00338-012-0926-8
DeSalvo, M., Voolstra, C., Sunagawa, S., Schwarz, J., Stillman, J., Coffroth, M., et al. (2008). Differential gene expression during thermal stress and bleaching in the Caribbean coral Montastraea faveolata. Mol. Ecol. 17, 3952–3971. doi: 10.1111/j.1365-294X.2008.03879.x
Dishon, G., Dubinsky, Z., Fine, M., and Iluz, D. (2012). Underwater light field patterns in subtropical coastal waters: a case study from the Gulf of Eilat (Aqaba). Isr. J. Plant Sci. 60, 265–275. doi: 10.1560/IJPS.60.1-2.265
Dove, S., Hoegh-Guldberg, O., and Ranganathan, S. (2001). Major colour patterns of reef-building corals are due to a family of GFP-like proteins. Coral Reefs 19, 197–204. doi: 10.1007/PL00006956
Dove, S. G., Lovell, C., Fine, M., Deckenback, J., Hoegh-Guldberg, O., Iglesias-Prieto, R., et al. (2008). Host pigments: potential facilitators of photosynthesis in coral symbioses. Plant Cell Environ. 31, 1523–1533. doi: 10.1111/j.1365-3040.2008.01852.x
Einbinder, S., Gruber, D. F., Salomon, E., Liran, O., Keren, N., and Tchernov, D. (2016). Novel adaptive photosynthetic characteristics of mesophotic symbiotic microalgae within the reef-building coral, Stylophora pistillata. Front. Mar. Sci. 3:195. doi: 10.3389/fmars.2016.00195
Enríquez, S., Méndez, E. R., and Prieto, R. I. (2005). Multiple scattering on coral skeletons enhances light absorption by symbiotic algae. Limnol. Oceanogr. 50, 1025–1032. doi: 10.4319/lo.2005.50.4.1025
Eyal, G., Cohen, I., Eyal-Shaham, L., Ben-Zvi, O., Tikochinski, Y., and Loya, Y. (2019). Photoacclimation and induction of light-enhanced calcification in the mesophotic coral Euphyllia paradivisa. R. Soc. Open Sci. 6:180527. doi: 10.1098/rsos.180527
Eyal, G., Wiedenmann, J., Grinblat, M., D’angelo, C., Kramarsky-Winter, E., Treibitz, T., et al. (2015). Spectral diversity and regulation of coral fluorescence in a mesophotic reef habitat in the Red Sea. PLoS One 10:e0128697. doi: 10.1371/journal.pone.0128697
Eyal-Shaham, L., Eyal, G., Tamir, R., and Loya, Y. (2016). Reproduction, abundance and survivorship of two Alveopora spp. in the mesophotic reefs of Eilat. Red Sea Sci. Rep. 6:20964. doi: 10.1038/srep20964
Förster, T. (1955). Intermolecular Energy Transfer and Fluorescence. Ottawa, ON: National Research Council of Canada.
Franklin, E. C., Stat, M., Pochon, X., Putnam, H. M., and Gates, R. D. (2012). GeoSymbio: a hybrid, cloud-based web application of global geospatial bioinformatics and ecoinformatics for Symbiodinium–host symbioses. Mol. Ecol. Resour. 12, 369–373. doi: 10.1111/j.1755-0998.2011.03081.x
Gilmore, A. M., Larkum, A. W. D., Salih, A., Itoh, S., Shibata, Y., Bena, C., et al. (2003). Simultaneous time resolution of the emission spectra of fluorescent proteins and zooxanthellar chlorophyll in reef-building corals. Photochem. Photobiol. 77, 515–523. doi: 10.1562/0031-865520030770515STROTE2.0.CO2
Gittins, J. R., D’angelo, C., Oswald, F., Edwards, R. J., and Wiedenmann, J. (2015). Fluorescent protein-mediated colour polymorphism in reef corals: multicopy genes extend the adaptation/acclimatization potential to variable light environments. Mol. Ecol. 24, 453–465. doi: 10.1111/mec.13041
Goulet, T. L., Lucas, M. Q., and Schizas, N. V. (2019). “Symbiodiniaceae genetic diversity and symbioses with hosts from shallow to mesophotic coral ecosystems,” in Mesophotic Coral Ecosystems, eds Y. Loya, K. A. Puglise, and T. C. L. Bridge (Cham: Springer International Publishing), 537–551. doi: 10.1007/978-3-319-92735-0_30
Hollingsworth, L. L., Kinzie, R. A., Lewis, T. D., Krupp, D. A., and Leong, J. A. C. (2005). Phototaxis of motile zooxanthellae to green light may facilitate symbiont capture by coral larvae. Coral Reefs 24, 523–523. doi: 10.1007/s00338-005-0063-8
Hume, B., D’angelo, C., Burt, J., Baker, A. C., Riegl, B., and Wiedenmann, J. (2013). Corals from the Persian/Arabian Gulf as models for thermotolerant reef-builders: prevalence of clade C3 Symbiodinium, host fluorescence and ex situ temperature tolerance. Mar. Pollut. Bull. 72, 313–322. doi: 10.1016/j.marpolbul.2012.11.032
Jeffrey, S. W., and Humphrey, G. F. (1975). New spectrophotometric equations for determining chlorophylls a1, b1, c1 and c2 in higher plants, algae and natural phytoplankton. Biochem. Physiol. Pflanz 167, 191–194. doi: 10.1016/S0015-3796(17)30778-3
Kahng, S. E., Akkaynak, D., Shlesinger, T., Hochberg, E. J., Wiedenmann, J., Tamir, R., et al. (2019). “Light, temperature, photosynthesis, heterotrophy, and the lower depth limits of mesophotic coral ecosystems,” in Mesophotic Coral Ecosystems, eds Y. Loya, K. A. Puglise, and T. C. L. Bridge (Cham: Springer International Publishing), 801–828. doi: 10.1007/978-3-319-92735-0_42
Kahng, S. E., Hochberg, E. J., Apprill, A., Wagner, D., Luck, D. G., Perez, D., et al. (2012). Efficient light harvesting in deep-water zooxanthellate corals. Mar. Ecol. Prog. Ser. 455, 65–77. doi: 10.3354/meps09657
Kahng, S. E., and Kelley, C. D. (2007). Vertical zonation of megabenthic taxa on a deep photosynthetic reef (50–140 m) in the Au’au Channel, Hawaii. Coral Reefs 26, 679–687. doi: 10.1007/s00338-007-0253-7
Kaniewska, P., Anthony, K. R. N., and Hoegh-Guldberg, O. (2008). Variation in colony geometry modulates internal light levels in branching corals, Acropora humilis and Stylophora pistillata. Mar. Biol. 155, 649–660. doi: 10.1007/s00227-008-1061-5
Kawaguti, S. (1944). On the physiology of reef corals VI. Study on the pigments. Palao Trop. Biol. Stn. Stud. 2, 617–674.
Kirk, J. T. (2011a). “Absorption of light within the aquatic medium,” in Light and Photosynthesis in Aquatic Ecosystems, 3 Edn, ed. J. T. O. Kirk (New York, NY: Cambridge University Press), 50–97.
Kirk, J. T. (2011b). “The nature of the underwater light field,” in Light and Photosynthesis in Aquatic Ecosystems, ed. J. T. O. Kirk (New York, NY: Cambridge University Press), 153–198.
Klueter, A., Loh, W., Hoegh-Guldberg, O., and Dove, S. (2006). Physiological and genetic properties of two fluorescent colour morphs of the coral Montipora digitata. Symbiosis 42, 123–134.
Kramer, D. M., Johnson, G., Kiirats, O., and Edwards, G. E. (2004). New fluorescence parameters for the determination of QA redox state and excitation energy fluxes. Photosynth. Res. 79:209. doi: 10.1023/B:PRES.0000015391.99477.0d
Kühl, M., Cohen, Y., Dalsgaard, T., Jørgensen, B. B., and Revsbech, N. P. (1995). Microenvironment and photosynthesis of zooxanthellae in scleractinian corals studied with microsensors for O2, pH and light. Mar. Ecol. Prog. Ser. 117, 159–172. doi: 10.3354/meps117159
LaJeunesse, T. C., Parkinson, J. E., Gabrielson, P. W., Jeong, H. J., Reimer, J. D., Voolstra, C. R., et al. (2018). Systematic revision of Symbiodiniaceae highlights the antiquity and diversity of coral endosymbionts. Curr. Biol. 28, 2570–2580. doi: 10.1016/j.cub.2018.07.008
Lesser, M. P., Mazel, C. H., Gorbunov, M. Y., and Falkowski, P. G. (2004). Discovery of symbiotic nitrogen-fixing cyanobacteria in corals. Science 305, 997–1000. doi: 10.1126/science.1099128
Lesser, M. P., Slattery, M., and Leichter, J. J. (2009). Ecology of mesophotic coral reefs. J. Exp. Mar. Biol. Ecol. 375, 1–8. doi: 10.1016/j.jembe.2009.05.009
Lesser, M. P., Slattery, M., Stat, M., Ojimi, M., Gates, R. D., and Grottoli, A. (2010). Photoacclimatization by the coral Montastraea cavernosa in the mesophotic zone: light, food, and genetics. Ecology 91, 990–1003. doi: 10.1890/09-0313.1
Levy, O., Appelbaum, L., Leggat, W., Gothlif, Y., Hayward, D. C., Miller, D. J., et al. (2007). Light-responsive cryptochromes from a simple multicellular animal, the coral Acropora millepora. Science 318, 467–470. doi: 10.1126/science.1145432
Lyndby, N. H., Kühl, M., and Wangpraseurt, D. (2016). Heat generation and light scattering of green fluorescent protein-like pigments in coral tissue. Sci. Rep. 6:26599. doi: 10.1038/srep26599
Mass, T., Einbinder, S., Brokovich, E., Shashar, N., Vago, R., Erez, J., et al. (2007). Photoacclimation of Stylophora pistillata to light extremes: metabolism and calcification. Mar. Ecol. Prog. Ser. 334, 93–102. doi: 10.3354/meps334093
Mass, T., Kline, D. I., Roopin, M., Veal, C. J., Cohen, S., Iluz, D., et al. (2010). The spectral quality of light is a key driver of photosynthesis and photoadaptation in Stylophora pistillata colonies from different depths in the Red Sea. J. Exp. Biol. 213, 4084–4091. doi: 10.1242/jeb.039891
Matz, M. V., Fradkov, A. F., Labas, Y. A., Savitsky, A. P., Zaraisky, A. G., Markelov, M. L., et al. (1999). Fluorescent proteins from nonbioluminescent Anthozoa species. Nat. biotechnol. 17, 969–973. doi: 10.1038/13657
Mazel, C. H., and Fuchs, E. (2003). Contribution of fluorescence to the spectral signature and perceived color of corals. Limnol. Oceanogr. 48, 390–401. doi: 10.4319/lo.2003.48.1_part_2.0390
Muscatine, L. (1990). “The role of symbiotic algae in carbon and energy flux in reef corals,” in Ecosystems of the World, ed. Z. Dubinsky (Amsterdam: Elsevier), 75–87.
Niedzwiedzki, D. M., Jiang, J., Lo, C. S., and Blankenship, R. E. (2014). Spectroscopic properties of the Chlorophyll a–Chlorophyll c2–peridinin-protein-complex (acpPC) from the coral symbiotic dinoflagellate Symbiodinium. Photosynth. Res. 120, 125–139. doi: 10.1007/s11120-013-9794-5
Nir, O., Gruber, D. F., Einbinder, S., Kark, S., and Tchernov, D. (2011). Changes in scleractinian coral Seriatopora hystrix morphology and its endocellular Symbiodinium characteristics along a bathymetric gradient from shallow to mesophotic reef. Coral Reefs 30:1089. doi: 10.1007/s00338-011-0801-z
Nir, O., Gruber, D. F., Shemesh, E., Glasser, E., and Tchernov, D. (2014). Seasonal mesophotic coral bleaching of Stylophora pistillata in the northern Red Sea. PLoS One 9:e84968. doi: 10.1371/journal.pone.0084968
Platt, T., Gallegos, C., and Harrison, W. G. (1980). Photoinhibition of photosynthesis in natural assemblages of marine phytoplankton. J. Mar. Res. 38, 687–701.
Quick, C., D’angelo, C., and Wiedenmann, J. (2018). Trade-offs associated with photoprotective green fluorescent protein expression as potential drivers of balancing selection for color polymorphism in reef corals. Front. Mar. Sci. 5:11. doi: 10.3389/fmars.2018.00011
Quigley, K. M., Strader, M. E., and Matz, M. V. (2018). Relationship between Acropora millepora juvenile fluorescence and composition of newly established Symbiodinium assemblage. PeerJ 6, e5022. doi: 10.7717/peerj.5022
R Core Team (2013). R: A Language and Environment for Statistical Computing. Vienna: R Foundation for Statistical Computing.
Roth, A. A., Clausen, C. D., Yahiku, P. Y., Clausen, V. E., and Cox, W. W. (1982). Some effects of light on coral growth. Pac. Sci. 36, 65–81.
Roth, M. S. (2014). The engine of the reef: photobiology of the coral–algal symbiosis. Front. Microbiol. 5:422. doi: 10.3389/fmicb.2014.00422
Roth, M. S., Padilla-Gamiño, J. L., Pochon, X., Bidigare, R. R., Gates, R. D., Smith, C. M., et al. (2015). Fluorescent proteins in dominant mesophotic reef-building corals. Mar. Ecol. Prog. Ser. 521, 63–79. doi: 10.3354/meps11108
Salih, A., Larkum, A., Cox, G., Kühl, M., and Hoegh-Guldberg, O. (2000). Fluorescent pigments in corals are photoprotective. Nature 408, 850–853. doi: 10.1038/35048564
Schlichter, D., and Fricke, H. W. (1990). Coral host improves photosynthesis of endosymbiotic algae. Naturwissenschaften 77, 447–450. doi: 10.1007/BF01135950
Schlichter, D., Fricke, H. W., and Weber, W. (1986). Light harvesting by wavelength transformation in a symbiotic coral of the Red Sea twilight zone. Mar. Biol. 91, 403–407. doi: 10.1007/BF00428634
Schlichter, D., Meier, U., and Fricke, H. (1994). Improvement of photosynthesis in zooxanthellate corals by autofluorescent chromatophores. Oecologia 99, 124–131. doi: 10.1007/bf00317092
Scott, B. D., and Jitts, H. R. (1977). Photosynthesis of phytoplankton and zooxanthellae on a coral reef. Mar. Biol. 41, 307–315. doi: 10.1007/BF00389097
Smith, E. G., D’angelo, C., Sharon, Y., Tchernov, D., and Wiedenmann, J. (2017). Acclimatization of symbiotic corals to mesophotic light environments through wavelength transformation by fluorescent protein pigments. Proc. R. Soc. B 284:1858. doi: 10.1098/rspb.2017.0320
Stimson, J. (1985). The effect of shading by the table coral Acropora hyacinthus on understory corals. Ecology 66, 40–53. doi: 10.2307/1941305
Sweeney, A. M., Boch, C. A., Johnsen, S., and Morse, D. E. (2011). Twilight spectral dynamics and the coral reef invertebrate spawning response. J. Exp. Biol. 214, 770–777. doi: 10.1242/jeb.043406
Szabó, M., Wangpraseurt, D., Tamburic, B., Larkum, A. W. D., Schreiber, U., Suggett, D. J., et al. (2014). Effective light absorption and absolute electron transport rates in the coral Pocillopora damicornis. Plant Physiol. Biochem 83, 159–167. doi: 10.1016/j.plaphy.2014.07.015
Takahashi-Kariyazono, S., Terai, Y., and Sakai, K. (2018). Presence–absence polymorphisms of highly expressed FP sequences contribute to fluorescent polymorphisms in Acropora digitifera. Genome Biol. Evol. 10, 1715–1729. doi: 10.1093/gbe/evy122
Tamir, R., Eyal, G., Kramer, N., Laverick, J. H., and Loya, Y. (2019). Light environment drives the shallow-to-mesophotic coral community transition. Ecosphere 10:e02839. doi: 10.1002/ecs2.2839
Veal, C. J., Carmi, M., Fine, M., and Hoegh-Guldberg, O. (2010). Increasing the accuracy of surface area estimation using single wax dipping of coral fragments. Coral Reefs 29, 893–897. doi: 10.1007/s00338-010-0647-9
Walsh, P., and Legendre, L. (1983). Photosynthesis of natural phytoplankton under high frequency light fluctuations simulating those induced by sea surface waves. Limnol. Oceanogr. 28, 688–697. doi: 10.4319/lo.1983.28.4.0688
Wangpraseurt, D., Larkum, A., Ralph, P., and Kühl, M. (2012). Light gradients and optical microniches in coral tissues. Front. Microbiol. 3:316. doi: 10.3389/fmicb.2012.00316
Wangpraseurt, D., Lichtenberg, M., Jacques, S. L., Larkum, A. W. D., and Kühl, M. (2019). Optical properties of corals distort variable chlorophyll fluorescence measurements. Plant Physiol. 179, 1608–1619. doi: 10.1104/pp.18.01275
Wangpraseurt, D., Tamburic, B., Szabó, M., Suggett, D., Ralph, P. J., and Kühl, M. (2014). Spectral effects on Symbiodinium photobiology studied with a programmable light engine. PLoS One 9:e112809. doi: 10.1371/journal.pone.0112809
Keywords: photosynthesis, mesophotic coral ecosystems (MCEs), Symbiodiniaceae, fluorescence, microsensors
Citation: Ben-Zvi O, Wangpraseurt D, Bronstein O, Eyal G and Loya Y (2021) Photosynthesis and Bio-Optical Properties of Fluorescent Mesophotic Corals. Front. Mar. Sci. 8:651601. doi: 10.3389/fmars.2021.651601
Received: 15 January 2021; Accepted: 24 March 2021;
Published: 15 April 2021.
Edited by:
Noga Stambler, Bar-Ilan University, IsraelReviewed by:
Michael P. Lesser, University of New Hampshire, United StatesCopyright © 2021 Ben-Zvi, Wangpraseurt, Bronstein, Eyal and Loya. This is an open-access article distributed under the terms of the Creative Commons Attribution License (CC BY). The use, distribution or reproduction in other forums is permitted, provided the original author(s) and the copyright owner(s) are credited and that the original publication in this journal is cited, in accordance with accepted academic practice. No use, distribution or reproduction is permitted which does not comply with these terms.
*Correspondence: Or Ben-Zvi, b3JienZpQGdtYWlsLmNvbQ==
Disclaimer: All claims expressed in this article are solely those of the authors and do not necessarily represent those of their affiliated organizations, or those of the publisher, the editors and the reviewers. Any product that may be evaluated in this article or claim that may be made by its manufacturer is not guaranteed or endorsed by the publisher.
Research integrity at Frontiers
Learn more about the work of our research integrity team to safeguard the quality of each article we publish.