- 1Department of Biology, University of West Florida, Pensacola, FL, United States
- 2Center for Environmental Diagnostics and Bioremediation, University of West Florida, Pensacola, FL, United States
- 3Bream Fishermen Association, Pensacola, FL, United States
Vibriosis is the general term for human illnesses caused by infection of pathogenic Vibrio species. Vibrio vulnificus (Vv) and parahaemolyticus (Vp) are two problematic waterborne pathogens that have yet to be enumerated in northwest Florida coastal Gulf of Mexico estuaries. In this regionally novel study, we surveyed 43 locations in two subtropical estuarine systems, Perdido Bay and Pensacola Bay, over seven dates in winter 2020. Sampling included three substrate types: surface waters, sediments, and invertebrate biofilms. We determined baseline abundances of presumptive viable Vv and Vp appearing as colonies on CHROMagar (Vv, blue; Vp, purple). Vv was detected in 37 out of 43 water samples, with maximum levels of 3,556 CFU/mL. Vp was only detected in 15 water samples, with a maximum concentration of 8,919 CFU/mL. Sediments contained Vv in all but one sample, with concentrations ranging from 121 to 607,222 CFU/mL. In contrast, Vp were only detected in 33 sediment samples, where concentrations ranged from 28 to 77,333 CFU/mL. Opportunistically-sampled surface swabs (biofilms), collected from shells (either oyster or barnacle) and polychaete worms found in sediment samples, contained on average 7,735 and 1,490 CFU/mL of Vv and Vp, respectively. Surface water Vv abundances covaried with bottom water pH, maximum prior cumulative wind speeds, and tidal coefficient on the day of sampling. Vp surface water abundances negatively correlated with surface water salinity, surface water pH, and bottom water pH and positively correlated with total surface dissolved inorganic and total Kjeldahl nitrogen concentrations, and wind. Spatially, there was large variation in Vibrio densities in surface waters; abundances of both species were strongly correlated with wind, suggesting resuspension was important. Sedimentary abundances of both putative Vv and Vp shared a correlation with one parameter: salinity stratification. Due to the length of this study, temperature was not considered a major factor. This short-term (1 month) study was designed not to enumerate pathogenic Vv or Vp, but rather to establish the first winter baseline of Vibrio abundances for this region. Determination of these baseline winter cultivable putative Vibrio abundances will be valuable in predicting relative risk factors in each waterbody of interest.
Introduction
Warm and salty or brackish waters in Florida and other nearby coastal states are currently subject to increased temperatures and frequency of storm events, changing heterotrophic bacterial communities (Lønborg et al., 2019). The success of specific groups of bacteria in subtropical estuaries and coasts are affected by many factors including eutrophication, sea-level rise, warming temperatures, and changes in trophic states (Montánchez et al., 2019; Krishna et al., 2020; Li et al., 2020). One major concern associated with these factors is increased Vibrio abundances: in the water column, in and on invertebrates, and in the sediments (Hughes et al., 2013). Vibrio vulnificus (Vv) and V. parahaemolyticus (Vp) are two species that are potentially harmful to humans and the marine life on which local economies depend. Vibrio infections in the USA account for approximately 8,000 deaths annually (Pruzzo et al., 2005). Vv mostly causes human disease via entrance of open cuts or wounds, with a case fatality rate as high as 50% (Johnson et al., 2012). Pathogenesis of Vp, on the other hand, occurs through the consumption of raw or undercooked shellfish (especially oysters) and is the leading cause of reported fatalities through gastroenteritis and septicemia due to foodborne illness (Panicker et al., 2004; Johnson et al., 2012). Both species possess specialized genes that contribute to pathogen virulence by promoting phenotypes such as increased epithelial (intestinal) cell adhesion or erythrocyte (red blood cell) lysis (i.e., hemolysis) (Zhang and Austin, 2005).
Although Vp infections are more common in recent years (Lovell, 2017), serious Vv infections are concurrently increasing in frequency (Baker-Austin and Oliver, 2018). These increased pathogenic loads can be attributed to a variety of factors. Temperature is considered to be a driving factor of Vibrio abundances (Johnson, 2013; Wetz et al., 2014) and may even affect the relative severity of vibriosis (Hernández-Cabanyero et al., 2020). However, temperature was not a major factor in this study. In fact, our findings suggest other factors may also be responsible for higher abundances of culturable Vibrio. Water column Vibrio abundances may increase after storm events, possibly from sediment bacteria resuspension (Fries et al., 2008). Increased nutrient loads, often associated with anthropogenic- and climatic-influenced eutrophication, will likely promote higher concentrations of human pathogens such as Vibrio species (Malham et al., 2014). Higher turbidity has been previously related to Vp in subtropical estuaries near coastal northern Gulf of Mexico (GoM) (Zimmerman et al., 2007). Because Vibrio may be associated with diatoms and zooplankton, their abundances are likely positively related to increased turbidity, typically measured as concentrations of total suspended solids (TSS). Similarly, a positive relationship is often observed between Vibrio abundance and phytoplankton biomass, typically measured by chlorophyll a (chl a) (Martinez-Urtaza et al., 2012). Therefore, the combination of terrestrial inputs of nutrients from rain and storm events, sediment resuspension from high wind speeds, and the quite evident rise in global water temperatures may provide optimal environmental conditions for the success of human pathogens such as Vv and Vp (Davis et al., 2017; Froelich et al., 2019).
Invertebrate filter-feeders (such as oysters or barnacles) are also susceptible to minor changes in water and sediment quality, making them common carriers of pathogenic Vp (Aagesen et al., 2013). Oysters in the Pensacola Bay System (PBS), including in polluted waterbodies within, such as Bayou Chico, are common (Caffrey et al., 2016). Barnacles were abundant invertebrates settling on artificial reefs in the nearby GoM shelf in a recent study (Babcock et al., 2020). Often, biofilms on invertebrate shells are considered anoxic, a key consideration in our evaluation of Vibrio on invertebrates. Vv and Vp are aerobes, with most strains functioning as facultative anaerobes (Drake et al., 2007). Biofilms on oyster or barnacle shells are often oxic (Ray et al., 2019), which could promote success of Vv, Vp, and other aerobes in shell biofilms. For example, desiccation or regular air exposure of oysters from nearby northern GoM bays promotes Vibrio growth (Grodeska et al., 2019; Pruente et al., 2020).
In water and sediments or on invertebrates of Florida subtropical estuaries, there is currently little data with respect to Vv or Vp abundances, except in Apalachicola Bay, FL (Williams and LaRock, 1985; Lipp et al., 2001), formerly home to one of the largest oyster fisheries in the United States. It is estimated that warming water temperatures due to climate change may have a more significant effect in temperate waters (Froelich and Daines, 2020). However, abundances in subtropical estuaries are important to assess, not only considering the potentially pathogenic nature of Vv and Vp, but also because of increasing water and sediment temperatures anticipated with climate change (Thomas, 2016). Furthermore, estuaries may be fragile ecosystems, especially so in the current threat of climate change in subtropical regimes. Extreme climate events (droughts, floods, tropical cyclones, heat waves, etc.) have increased in frequency and intensity, coinciding with climate model predictions due to anthropogenic causes. Consequences of warming waters combined with increased intensity or frequency of such extreme events impact a wide range of trophic levels. Terrestrial-derived nutrient influxes into fresh and estuarine systems promote a chain of succession in both primary and secondary producers, leading to changes in the types of heterotrophs. Warming waters expected with global climate change combined with increased terrestrial organic matter inputs exacerbates these changes, in both temperate (Paczkowska et al., 2019) and subtropical (Williams and Quigg, 2019) regions. Clearly, there is cause for investigating the effects of these changes locally in relation to potentially pathogenic Vibrio loads.
Study site. To determine baseline presumptive Vv and Vp loads in winter, we surveyed seven major basins in the Pensacola, FL area. Locations for this survey were chosen within the PBS and the Perdido Bay, located in northwest Florida. The PBS is an excellent model system to examine the responses of subtropical microbial responses to anthropogenically-driven changes in temperature, freshwater flow, and other parameters (Murrell et al., 2018). The PBS is the fourth largest estuary in Florida and is unique in that it is microtidal, nutrient inputs are relatively low (Caffrey and Murrell, 2016), and heterotrophs are substrate-limited (Murrell, 2003). Flow from the Escambia River provides approximately 70% of the freshwater to Escambia Bay, which is part of the PBS (Caffrey and Murrell, 2016). While light availability, nutrient fluxes, primary productivity, and chl a are influenced by riverine nutrient inputs (Caffrey and Murrell, 2016), the impact of freshwater flow on the distribution of Vibrio in the estuary and adjacent basins is unknown. Chl a concentrations range from 1 to 26 μg/mL and are highest in mesohaline portions of the Bay (Murrell and Lores, 2004; Murrell et al., 2007; Caffrey and Murrell, 2016). Bacterial abundances and production are also influenced by freshwater inputs from the Escambia River (Murrell, 2003). Cyanobacteria abundances in these systems are low at the marine ends and undetectable in freshwaters, but dominate in mesohaline zones (Murrell and Caffrey, 2005).
In the PBS, chl a and phytoplankton productivity peak about a month following seasonal maximum freshwater flow from the Escambia River (Murrell et al., 2007). Summer benthic hypoxia occurs during periods of stratification (Hagy and Murrell, 2007), but is moderated by light that supports sub-pycnocline phytoplankton and benthic primary production (Murrell et al., 2009). However, regional strong storm events do result in high particle loads (> 6,000 particles/mL), particularly in the summer. In winter (February 2014 and 2015), total particle abundances ranged from 500 to >3,500 particles/mL, as determined by FlowCam analysis (Sieracki et al., 1998) of Lugols-preserved whole water samples from the Escambia Bay (Michael Murrell, personal communication). Interestingly, the detrital contribution to total particulate matter composition is low (Murrell, 2003), suggesting the majority of particulate matter in the estuary is comprised of zooplankton and phytoplankton. The phytoplankton are diverse, with picocyanobacteria (<5 μm) dominating during summer months, representing 70 to 100% of total chl a (Murrell and Lores, 2004), a pattern found in other GoM estuaries (Murrell and Caffrey, 2005).
Perdido Bay is another shallow estuarine system whose watershed includes southeast Alabama. Waters in this system are generally more turbid than in the PBS as a whole, and major sources of freshwater input include the Perdido River and Elevenmile Creek (Macauley et al., 1995). While Escambia Bay/Pensacola Bay and Perdido Bay are major river-dominated systems with freshwater inflow, Big Lagoon is a mesohaline system connecting the PBS and Perdido Bay, where sediments are more sandy than muddy, light attenuation is generally low, and seagrasses are more abundant than in the Escambia or Perdido Bays (Hester et al., 2016; Murrell et al., 2018). Creeks provide freshwater inputs into the three urban bayous in this study: Bayou Grande, Bayou Texar, and Bayou Chico. All three bayous, adjacent to the city of Pensacola, are the largest bayous in the PBS that are impacted by stormwater runoff (Lewis et al., 2016). Other inputs of freshwater to the bayous include adjacent creeks, but runoff contributes greatly to eutrophication and sedimentation, as well as contamination with heavy metals and pesticides (Lewis et al., 2002; Nature Conservancy, 2014). Septic tank systems within the Bayou Chico and Grande watersheds contribute to eutrophication, and Bayou Chico fails to meet total maximum daily load goals of US and FL standards (Nature Conservancy, 2014). We hypothesized that abundances of Vibrio species in the Perdido and Pensacola Bay Systems would be higher in water column samples with high turbidity. We also expected Vibrio species to thrive where dissolved or total nutrient concentrations were high, especially when salinity and temperature fell within optimal ranges as described by previously-published studies (Kaspar and Tamplin, 1993; Motes et al., 1998; Hsieh et al., 2008). Temperature was not considered a major factor in this short-term study, as all sampling was done within one winter month (February 2020).
Materials and Methods
Field Sampling
Forty-three stations were chosen, representing a wide range of salinities, stratification, eutrophication, and freshwater influence. We surveyed surface waters and sediments on seven dates between 02/03/20 and 03/02/20 (Supplementary Figure 1). All sampling and in situ measurements were performed from small vessels. Hydrographic data (surface water and bottom water) were measured with a YSI multimeter (Xylem, Yellow Springs, OH). Parameters measured in situ at all stations included surface and bottom water column readings of salinity (PSU), temperature (°C), and dissolved oxygen (mg/L). Surface and bottom water pH were determined with a ProDSS pH sensor, which became available on 02/21/20; thus, surface and bottom pH were reported only for Bayou Grande (02/21/20), Big Lagoon (02/28/20), and Perdido Bay (03/02/20). Statistical analyses (Supplementary Table 4) for pH data reflect the correct sample size (n = 20). Light attenuation coefficient (Kd, m–1) was calculated from the slope of attenuation of photosynthetic photon flux fluence rate (PPFFR) at 0.5-meter intervals measured with a LiCor 4II spherical underwater quantum sensor. Total water column depth (meters) was measured with the line on a Secchi disk and with the vessel’s on-board instrumentation. Total precipitation and cumulative maximum wind speeds were recorded in the three days leading up to sampling, including the mornings of sampling events in which windy conditions delayed the start of sampling. Precipitation and maximum wind speed data were obtained from the NOAA Climate Online Data station ID: GHCND:USW00013899, 30.47° N, 87.2° W located at Pensacola Regional Airport (WUnderground, 2020; Supplementary Table 4). Tidal coefficient (m) at Lora Point (Escambia Bay, 30.5178° N, 87.1700° W), also within our sampling area and in one of the seven basins, was also recorded for each sampling date (TidalCoefficient, 2020). Since precipitation, wind speed, and tidal coefficient were the same within basins, values for each were considered reflective of the entire basin. Therefore, sample size for these parameters was n = 7.
Forty-three surface water samples were collected via bucket cast and held in an acid-washed 1-L Nalgene bottle (one for each site) in coolers maintained at in situ temperature. Sediment samples were collected using a PONAR grab sampler. Forty-two sediment samples (0.5 to 2.0 mL of superficial sediment) were collected from the top 0 to 30 cm with sterile, single-use plastic spatulas and resuspended in 0.5 mL of phosphate-buffered saline (1X solution contains 0.137M NaCl, 0.0027M KCl, 0.0119M phosphates, pH 7.4) (Fisher BioReagents, Pittsburgh, PA), and held in sterile 50-mL centrifuge tubes in coolers maintained at in situ temperature. An additional 50 mL of sediment was collected for analysis of sediment water content, organic matter content (loss on ignition), sediment chl a and phaeopigment concentrations. Surface water was filtered on-site through Whatman GF/F filters to collect filtrates which were deposited into acid-washed bottles and immediately preserved in coolers on ice and later stored at the laboratory at −20°C for subsequent dissolved nutrient analyses. Filters were also immediately cooled on site and preserved at −20°C for subsequent chl a analyses. Whole water was preserved with H2SO4 as per EPA method 351.2 and held at 4°C for total Kjeldahl nitrogen (TKN) analyses. Where invertebrates were present (on marker poles or in sediment grabs), we collected invertebrate surface (presumptive biofilm) samples via surface-swab, henceforth referred to as biofilm or biofilm swab samples. A total of 13 samples of these biofilms, approximately 1 cm2 surface area each, were collected with sterile cotton swabs, resuspended in 0.1 mL of sterile phosphate-buffered saline in centrifuge tubes and held at in situ temperature until processed.
All surface water samples were analyzed using the following methods: dissolved inorganic phosphate (DIP) as in (Parsons et al., 1984), NH4+ as in (Holmes et al., 1999), and NO3– + NO2– as in (Schnetger and Lehners, 2014). Dissolved inorganic nitrogen (DIN) is the sum of NH4+, NO3–, and NO2–. Whole water was preserved with acid and analyzed for TKN with EPA method 351.2 on a Lachat Quikchem FIA Model autoanalyzer. Samples for chl a were extracted in 90% acetone for 24 hours and analyzed as in (Welschmeyer, 1994). Sediment samples were evaluated for biomass of benthic microalgae using the acidified chl method to determine concentrations (μg/g) of sediment chl a and phaeopigment as in (Parsons et al., 1984). Sediment aliquots were also processed for water content and organic carbon (ash content) percentages. Ash-free dry weight combustion was at 500°C. Surface water concentrations of TSS were determined using EPA method 160.2.
Viable Putative Vibrio Abundances
To determine abundances of presumptive viable Vv and Vp, all samples (surface water, sediment suspension, and biofilm surface-swab suspension) were plated on CHROMagarTM-Vibrio (DRG International, Inc., Springfield, NJ), an agar medium with chromogenic substrates (15.0 g/L agar, 8.0 g/L peptone and yeast extract, 51.4 g/L salts, 0.3 g/L chromogenic mix, pH 9.0). In winter (January) and summer (June) of 2019, we had performed preliminary end-point PCR testing to narrow color ranges of presumptive Vv (bright blue, with early color development before 24 h) and Vp (bright mauve, again with early color development). To assess accuracy of presumptive Vv colored colonies on CHROMagar, vvhA was assayed using primer pair F-vvh785/R-vvh990 as previously described (Panicker et al., 2004; Panicker and Bej, 2005). Presumptive Vp, appearing as bright pink, not purple, were confirmed as Vp with either tdh- or trh-positive end-point PCR, employing primer pairs tdh-F/R and trh-F/R as previously described (Nordstrom et al., 2007). All samples of colony biomass were also tested by end-point PCR for prokaryotic 16S rRNA (BACT1369 + PROK1541R), to verify there were no false-negatives. None of the 2019 preliminary data (abundances based on colony counts or end-point PCR results) are presented here, since data collection in 2019 did not include environmental data. In the preliminary work, depending on the body of water sampled, the percent of bright blue colonies scoring vvhA-positive ranged from ∼50 to 85%, with an average of 75% among all summer and winter 2019 samples for Vv (bright blue colony, vvhA-positive) and Vp (bright pink colony, tdh- or tlh-positive.) The same person (L. Waidner) who observed vvhA-positive and trh- or tdh-positive colonies was also the one who counted colonies in the winter 2020 data presented in this study.
In this study, to assess presumptive Vv and Vp abundances, water samples were plated undiluted on CHROMagarTM-Vibrio; fresh sediment samples were diluted 2-, 3-, and 4-log-fold with phosphate-buffered saline before plating, and biofilm surface-swab samples were similarly diluted 1- and 2-log-fold. Subsequent to dilutions and plating, exact volumes of each sediment sample were determined. Each abundance calculation accounted for the variability in volume of sediments collected in the field. Presumptive biofilm, sediment, and undiluted surface water samples were vigorously shaken (water) or mixed via vortex (sediment and biofilm) prior to plating. Three aliquots (0.15 mL each) of each dilution of each sample, in triplicate, were plated on Petri plates as previously described (Oliver, 2003; Huq et al., 2012; Thomas et al., 2014; Yeung and Thorsen, 2016). Plating was followed by incubation in the dark at room temperature (24°C, chosen since this was most similar to in situ temperature) for 24, 48, and 72 h. At each 24-hour interval, the number of each of the colored colony types was recorded and reported as CFU of presumptive Vv or Vp. The abundances of each type of Vibrio were calculated in the same manner for all sample types and included all abundances of dilutions that could be accurately assessed (between 3 and 300 CFU/plate). Abundances lower than the limit of detection (15 CFU/mL) were recorded as zero. For presumptive abundance determination across all samples, abundances at 48 hours were used, and average cell abundances were normalized to milliliters (mL), since abundances per cm3 are equivalent to abundances per volume (mL). We did not use molecular methods to confirm Vv and Vp (i.e., bright blue and bright pink colonies, respectively) in this study.
Statistical Analyses
Mathematical relationships and each corresponding significance were performed using the “Analyze” function of the Statistical Package for the Social Sciences (SPSS). All surface water and sedimentary Vibrio abundances were log-transformed to accommodate normality for Pearson (parametric) correlation analysis. SPSS was used to generate one-way analysis of variance (ANOVA) tests to determine differences between log-transformed Vibrio abundances and environmental parameters among all seven basins. Parameters that showed relatively strong evidence (r >0.30) for a difference among basins were followed with multiple comparison procedures (Tukey’s Post Hoc) to determine wherein the seven basins lies the difference. In addition to basin-by-basin comparisons, one-way ANOVAs were also generated to determine differences between open and enclosed basins. Pearson correlation coefficients were calculated in the statistical package Plymouth Routines in Multivariate Ecological Research (PRIMER) version 7. Box- and surface-plots showing the distribution of measured abundances and ecological parameters across all basins were also generated using PRIMER.
Results
Overview of Bodies of Water Sampled
In all stations of all basins surveyed, the minimum depth was approximately 0.4 m, with a maximum depth at any station of 6.1 m. The average surface water temperature of all stations was 15.4°C, and temperatures ranged from 12.3 to 22.2°C (Table 1A). Surface water salinities ranged from 0.9 to 18.2 PSU. Among all seven basins, Bayou Chico had the greatest average water chl a (8.6 ± 5.4 μg/L), TKN (53.5 ± 16.7 μM), and DIN (39.0 ± 38.7 μM) concentrations. Of all basins examined, the surface waters’ temperature and salinity in Bayou Chico were overall the highest and lowest, respectively (Figure 1). Additionally, salinity stratification was high at two of the five locations sampled within Bayou Chico, V-20 and V-23, with surface and bottom values of 9.4 and 21, respectively (Supplementary Table 4). Perdido Bay had the lowest average water chl a concentration (1.9 ± 1.0 μg/L), and Big Lagoon had the lowest average TKN (24.2 ± 3.0 μM) and DIN (1.7 ± 0.5 μM) concentrations. DIP concentrations were negligible or undetectable in surface water samples (Table 1A and Figure 2).

Figure 1. Boxplots of surface water salinity and temperature. Open circles denote extreme values; box-tops and -bottoms show the 25th and 75th percentiles; lines within boxes mark mean values; and whiskers indicate minimums and maximums.
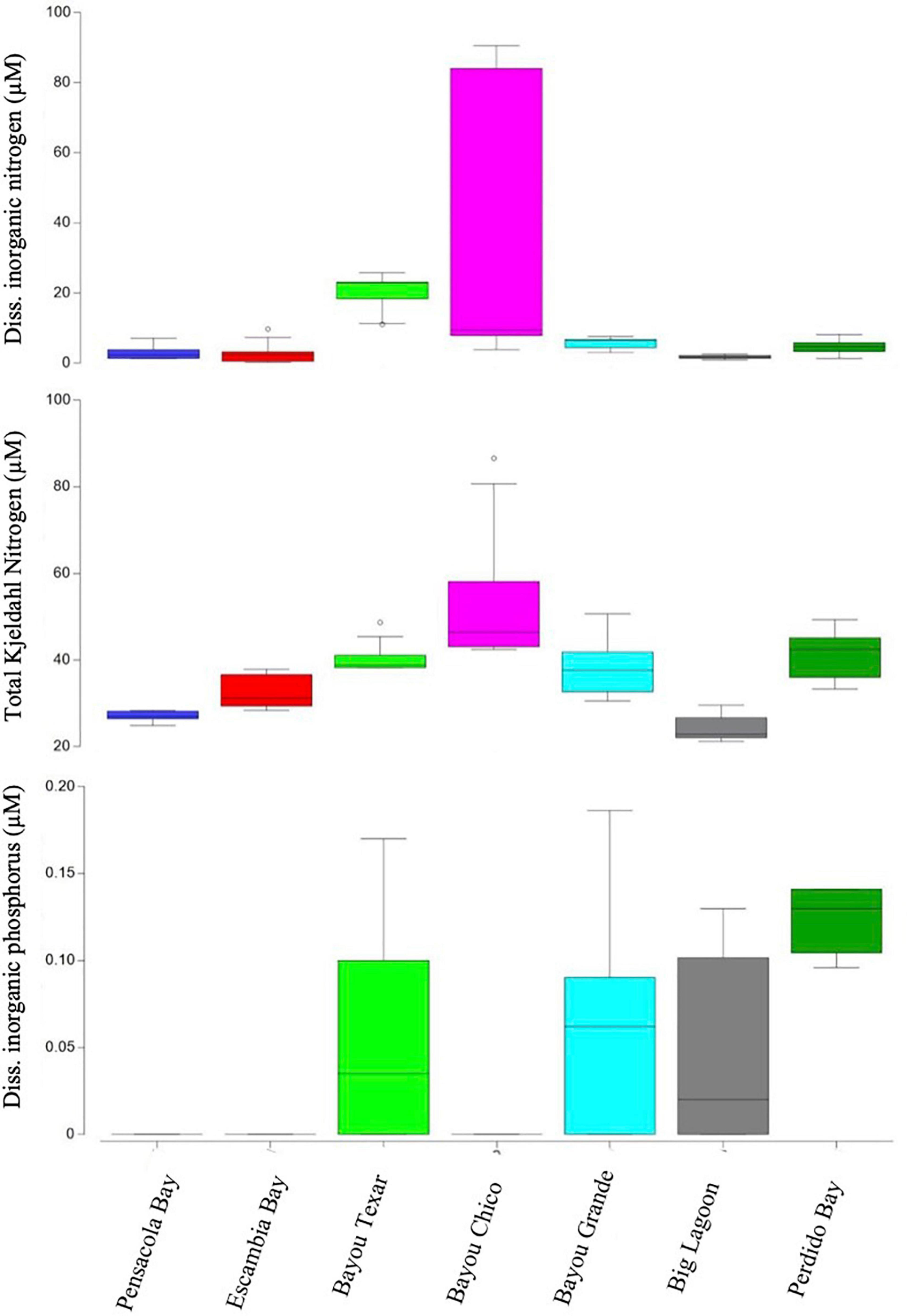
Figure 2. Boxplots of dissolved inorganic nitrogen and phosphorous (DIN, DIP) and total Kjeldahl nitrogen (TKN) concentrations. Open circles denote extreme values; box-tops and -bottoms show the 25th and 75th percentiles; lines within boxes mark mean values; and whiskers indicate minimums and maximums.
Bottom water temperatures and salinities ranged from 13.0 to 22.0°C and 1.9 to 28 PSU, respectively (Table 1B). Bayou Texar had the greatest average sediment chl a concentration (3.7 ± 2.4 μg/g). Escambia Bay had the greatest average concentration of sediment phaeopigment (4.7 ± 1.5 μg/g) and average sediment water content (58.0 ± 22.7%). Perdido Bay had the greatest average sediment organic content (8.3 ± 6.0%), with Pensacola Bay as the lower bound (1.8 ± 2.8%).
Overview of Putative Vibrio in Surface Waters
Overall, in surface waters, Vv was more ubiquitous than Vp. In seven of the 43 surface water samples, putative Vv was below detection limits of the chromogenic agar assay (Table 2). In the remaining 36 locations, Vv concentrations ranged from 15 to 3,556 CFU/mL, with a median concentration of 44 CFU/mL. In contrast, putative Vp abundance was below the limit of detection at 28 of the 43 stations (Table 3). In the 15 surface water samples where it was detected, Vp concentrations ranged from 15 to 8,919 CFU/mL, with a median concentration of 104 CFU/mL. Bayou Texar greatly outnumbered the other basins with regards to Vp abundances, with Pensacola Bay and Perdido Bay having the lowest surface water Vp abundances (Table 3). Conversely, Bayou Chico had the greatest abundance of surface water Vv of the seven basins, as well as the largest range in Vv surface water abundance at the sites within the basin (Figure 3).
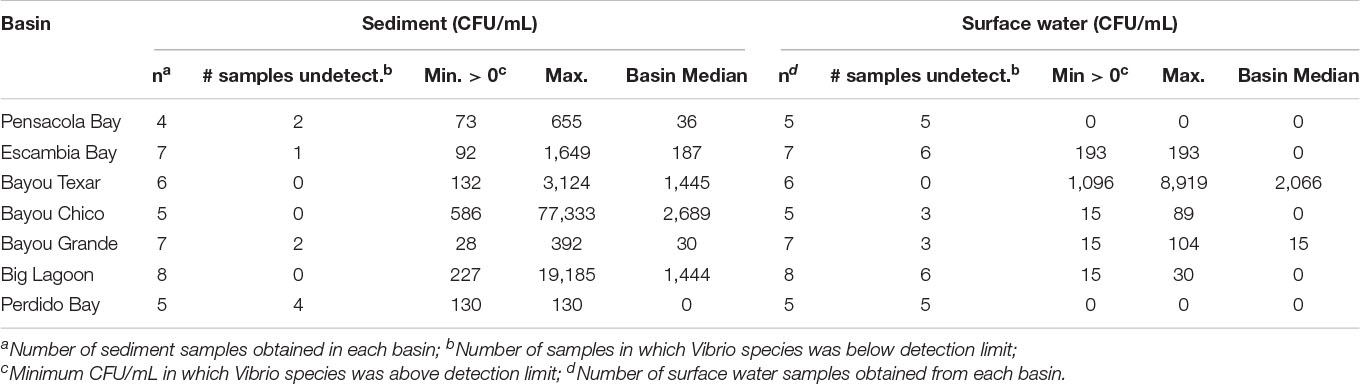
Table 3. Abundances of culturable Vp in sediments and surface waters. Medians and ranges in each basin are provided.
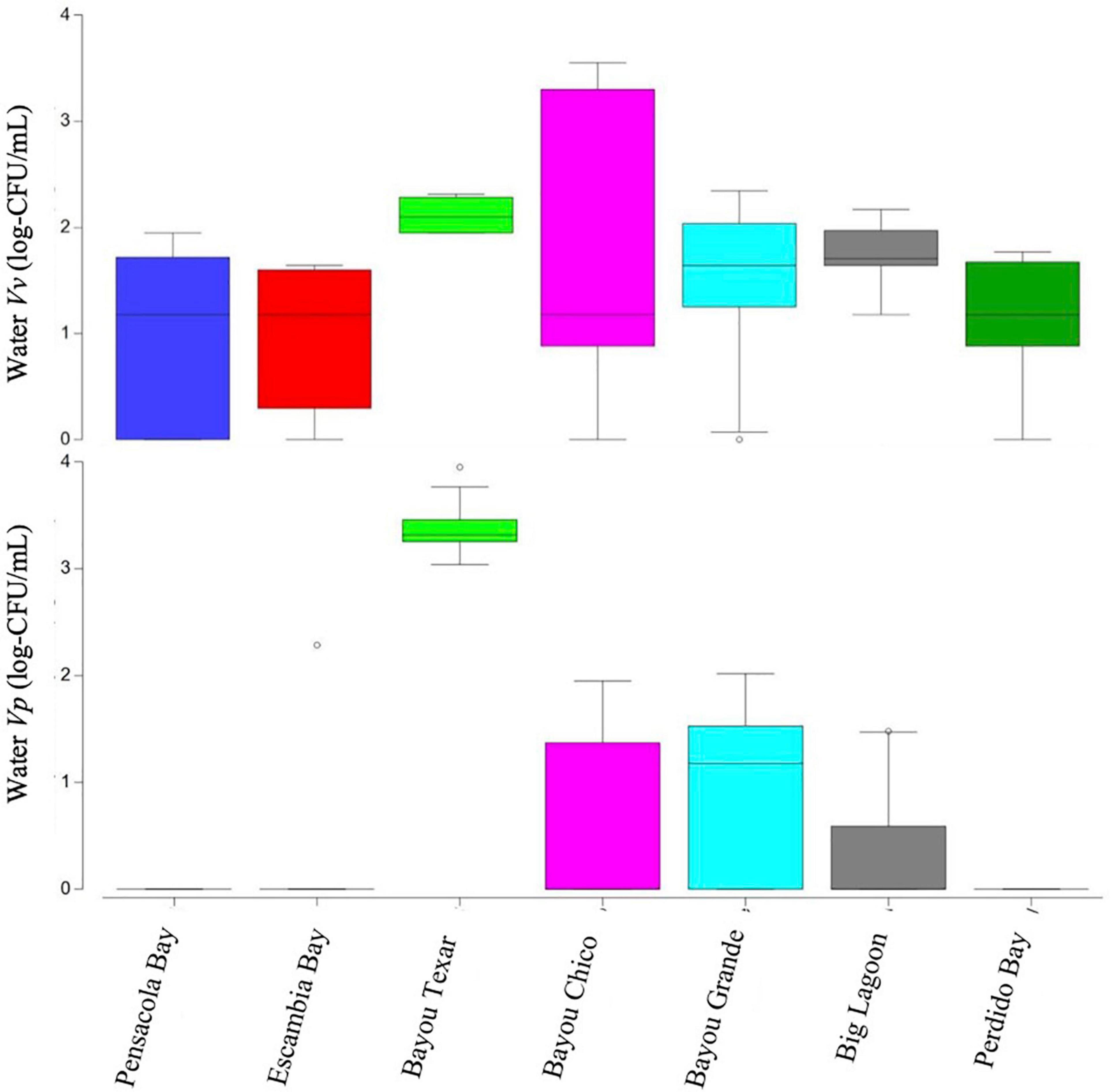
Figure 3. Boxplots of surface water Vibrio abundances. Open circles denote extreme values; box-tops and -bottoms show the 25th and 75th percentiles; lines within boxes mark mean values; and whiskers indicate minimums and maximums.
Overview of Putative Vibrio in Sediment Samples
Basin-wide analyses indicated there was no body of water with low concentrations of Vibrio in sediments. At the 43 locations sampled, 42 sediment samples were obtained. Sediment types ranged from sand to sand-mud mix to mud. When Vv was detected, concentrations ranged from 169 to 607,222 CFU/mL, with a median concentration of 18,160 CFU/mL (Table 2). In three of the seven major basins, there were exceptionally high Vv concentrations in at least one location each. In Bayous Chico and Texar, Vv was found in some locations at concentrations > 300,000 CFU/mL, and in one location of Big Lagoon at > 600,000 CFU/mL (Table 2). In contrast, Vp was generally less concentrated in the sediments and was below detection limits in nine of the sediment samples (Table 3). In the remaining 33 stations, Vp concentrations ranged from 28 to 77,333 CFU/mL, with a median concentration of 652 CFU/mL. The highest densities of Vp were observed in the sediments of Bayou Chico (77,333 CFU/mL) and in Big Lagoon (19,185 CFU/mL). In four of the five Perdido Bay sites, Vp was undetectable. In Bayou Grande, Vp was undetectable in two of the seven locations. In the Pensacola Bay basin (Pensacola Bay and Escambia Bay), we collected 11 sediment samples. Of those, three contained undetectable levels of Vp, one site had 1,649 CFU/mL, and the remaining eight sites contained fewer than 655 CFU/mL in the sediments (Table 3).
The variation in sediment Vibrio abundance, both among the seven basins and within each of them, was high. The upper bound of Vv was similar across all basins, with Pensacola Bay and Perdido Bay having the largest range (Figure 4). Vp, however, was more abundant in Bayou Chico, with Bayou Texar and Big Lagoon following closely behind. Sites within basins Pensacola Bay and Bayou Grande generally had the lowest abundance of Vp in sediments. Given that the average concentration of total active aerobic bacterial cells present per mL of estuarine, riverine, or coastal marine sediments is 1 to 500 × 106 (Glavin et al., 2004; Kirchman, 2018; Luna et al., 2002; Proctor and Souza, 2001), the data suggest Vv make up from 0.2 to 60% of total sediment bacteria; whereas Vp could comprise 0.003 to 0.2% of all bacteria in sediments. On average, Vv outnumbered Vp by approximately 18-fold in all sediments. Notably, very high abundances of Vv and Vp in sediments were found in stations V-24 and V-25, two sites in Bayou Grande. In these locations, bottom pH and stratification were closely tied and showed the greatest variation among all sites (Figure 5).
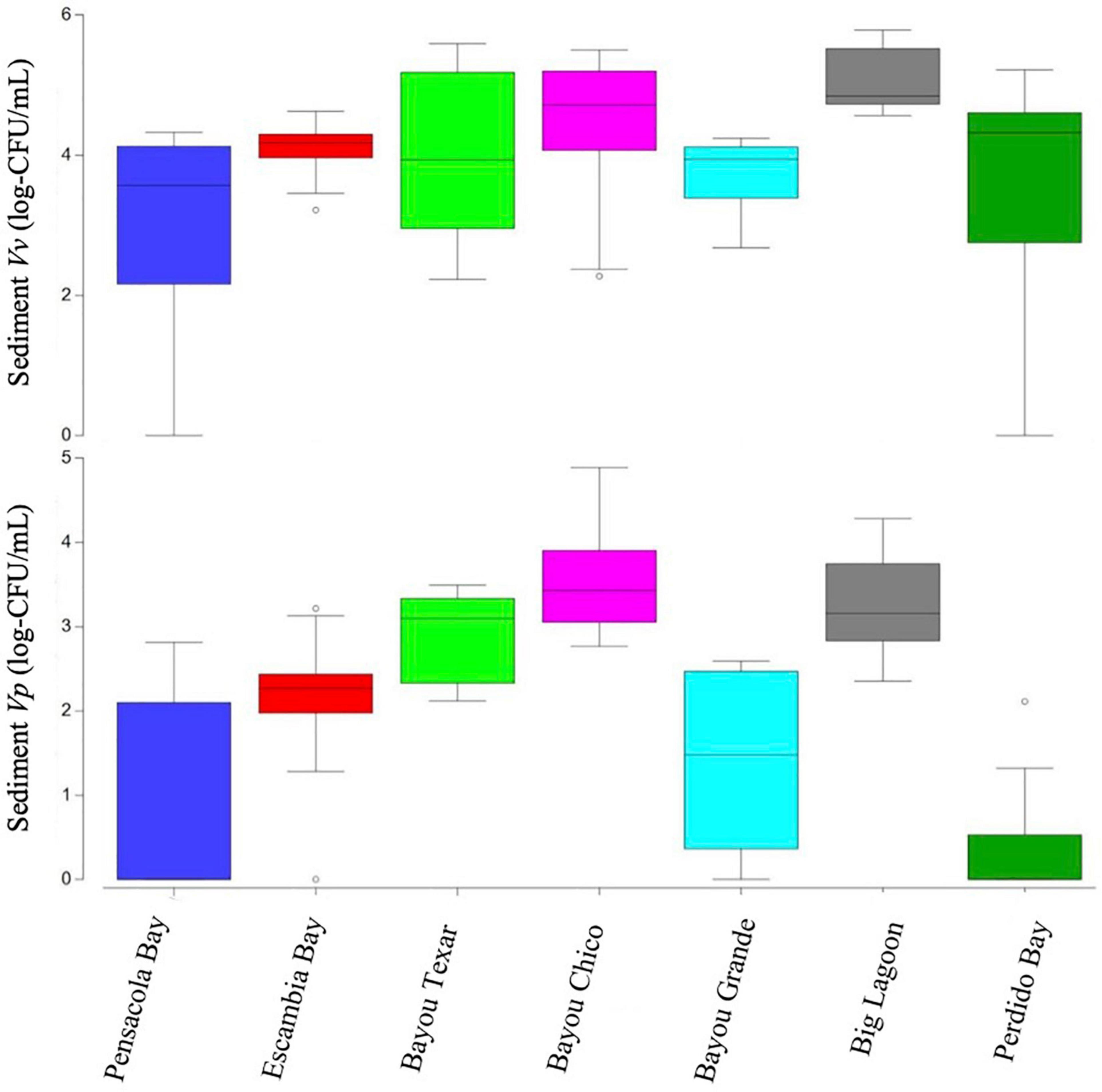
Figure 4. Boxplots of Vibrio abundances in sediments. Open circles denote extreme values; box-tops and -bottoms show the 25th and 75th percentiles; lines within boxes mark mean values; and whiskers indicate minimums and maximums.
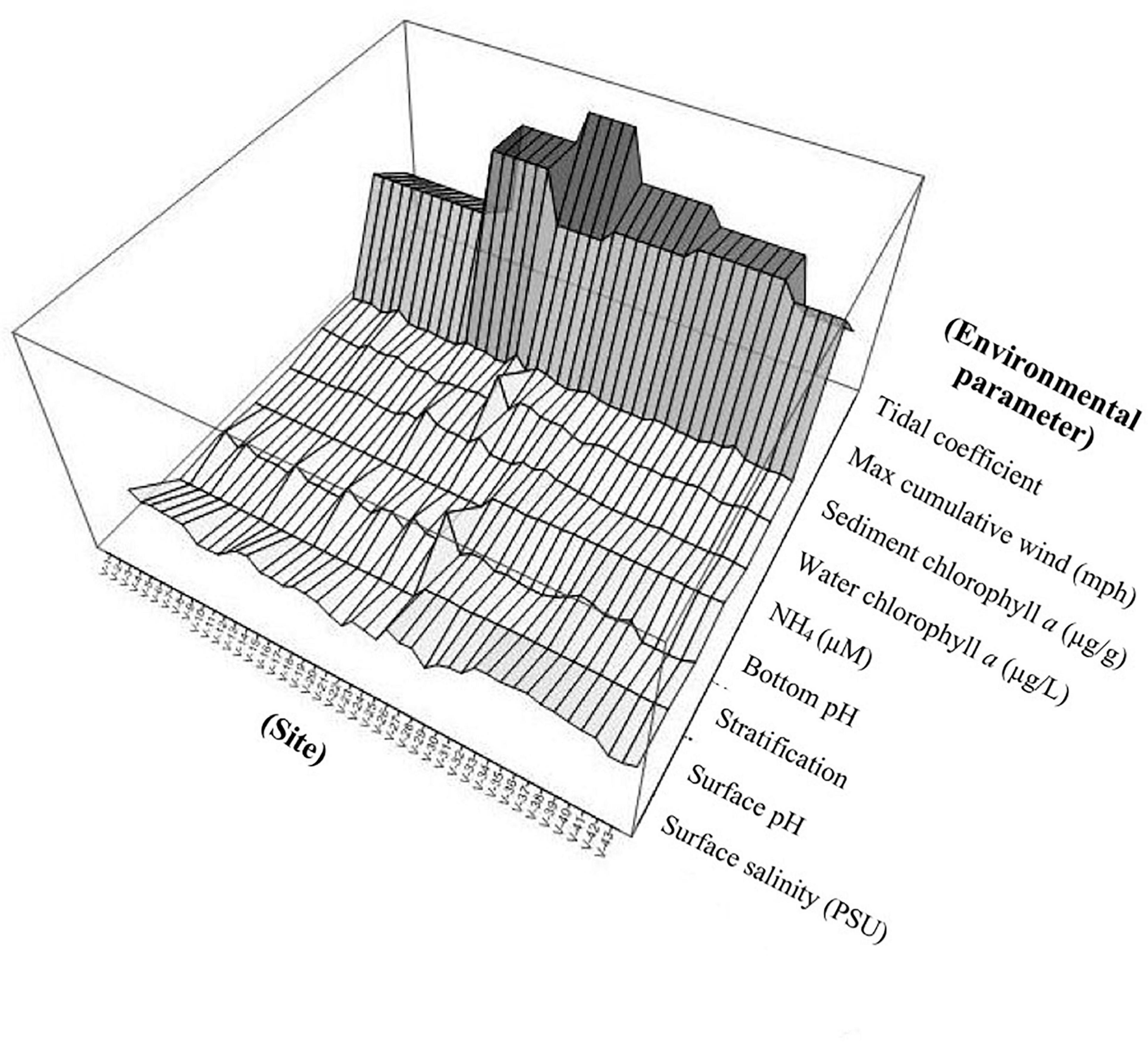
Figure 5. Surface plot illustrating site-to-site variation of the most influential environmental parameters covarying with Vibrio abundances.
Putative Vibrio in Biofilm Samples
The median concentrations of Vv and Vp in all presumptive biofilms were 2,281 and 163 CFU/mL, respectively (Table 4). Only two invertebrate biofilm samples resulted in undetectable Vibrio – one from a polychaete worm in Pensacola Bay, and the other from an oyster located at Bayou Grande. In the remaining 11 samples, mostly from swabbed oyster or barnacle shells, putative Vv abundances ranged from 81 to 28,844 CFU/mL, and putative Vp from 81 to 14,422 CFU/mL. There was not a statistical difference between oysters (n = 5) and barnacles (n = 6) for Vv (t-test, p = 0.750) nor Vp (t-test, p = 0.560) (Table 4).
Correlation of Abundances With Abiotic Environmental Parameters
Vibrios were generally most abundant in Bayou Chico (with the exception of Vp water abundances, which were greatest in Bayou Texar) (Tables 2, 3 and Figure 3). Prior wind speeds showed strong evidence for a positive correlation between surface water abundances of both Vv (r = 0.84, p < 0.05) and Vp (r = 0.83, p < 0.05), but not with sedimentary Vibrio abundances (Table 5). Water Vv abundances were also positively correlated with bottom water pH (r = 0.46, p < 0.05) and tidal coefficient (r = 0.86, p < 0.01) and moderately correlated with average sediment chl a concentrations (r = 0.29, p = 0.057) (Table 5). Surface water Vv did not covary strongly with salinity. Surface water Vp abundances correlated with seven parameters, the greatest number of correlations among the four calculated species abundances. Parameters that showed negative correlations with surface water Vp included surface salinity (r = −0.36, p < 0.05), surface pH (r = −0.45, p < 0.05), and bottom pH (r = −0.47, p < 0.05). Positive correlations with surface Vp included total DIN (r = 0.32, p < 0.05) and TKN (r = 0.36, p < 0.05) concentrations. Maximum cumulative wind speed (r = 0.83, p < 0.05) also showed evidence for a positive correlation with water Vp (Table 5).
In sediments, putative Vv abundances positively correlated with both surface (r = 0.50, p < 0.05) and bottom (r = 0.52, p < 0.05) pH (Table 5). Sedimentary Vp was correlated with average sediment chl a concentrations (r = 0.31, p < 0.05) and tidal coefficient (r = 0.77, p < 0.05). Interestingly, both Vv and Vp sediment abundances shared a correlation with one parameter: salinity stratification. Although winter levels of salinity stratification among all basins were generally low, stratification positively correlated with sedimentary abundances of both Vv (r = 0.31, p < 0.05) and Vp (r = 0.33, p < 0.05). Within the salinity values measured at different locations in this study, there was no optimal salinity range where Vv or Vp was found to be more abundant (Supplementary Figure 3 and Supplementary Table 4). To further examine the relationship of salinity with these presumed Vv and Vp abundances, we also examined Vibrio abundances within a subset of stations that had salinities only between 5-25 PSU. Five stations (V-7, V-19, V-20, V-23, V-27) with surface or bottom water salinities outside this range were excluded from this additional correlation analysis. Even when salinity did fall within the optimal range, there still was no significant relationship with abundance, whether in the water column (Vv; r = −0.0089, p = 0.958, n = 38) or in the sediments (Vv; r = 0.0875, p = 0.596, n = 39 and Vp; r = 0.1107, p = 0.542, n = 39). Only surface Vp showed a strong relationship with optimal salinity conditions, but it did so negatively (r = −0.435, p < 0.01, n = 38) (Supplementary Table 4).
Water column Vibrio abundances were not correlated with concentrations of TSS, dissolved oxygen, DIP, chl a, or Kd. Sedimentary Vibrio abundances were not correlated with the following sediment characteristics: percent water, percent organic content, and sediment phaeophyte pigments. Additionally, neither presumptive Vv nor Vp abundances were correlated with water column Kd.
Analysis of Variance (ANOVA) Among Basins
ANOVA tables were generated for each environmental parameter and subsequently used to determine factors that indicated strong evidence for a difference among basins (Supplementary Table 1). Bottom pH (F = 16.548), bottom salinity (F = 7.771), bottom water temperature (F = 33.328), log-DIN concentrations (F = 10.684), log-Vp sediment abundances (F = 9.421), log-Vp water abundances (F = 19.735), surface pH (F = 21.012), surface salinity (F = 13.750), surface water temperature (F = 96.284), and TKN concentrations (F = 9.732) were different at a < 0.001. Additionally, TSS (F = 3.225, p = 0.01), water chl a (F = 4.791, p = 0.001), DIP (F = 5.210, p = 0.001), and Kd (F = 4.817, p = 0.001) showed strong evidence for a difference among basins.
ANOVA Between Open and Enclosed Waterbodies
Statistical analysis was also performed to determine evidence of a difference between open estuaries (Pensacola Bay, Escambia Bay, and Perdido Bay) and enclosed estuaries (Bayou Texar, Bayou Chico, Bayou Grande, and Big Lagoon). Log-Vv water abundance (F = 9.973, p = 0.003), log-Vp water abundance (F = 9.662, p = 0.003), log-Vp sediment abundance (F = 17.271, p < 0.001), surface temperature (F = 7.838, p = 0.008), bottom temperature (F = 24.197, p < 0.0001), salinity stratification (F = 4.257, p = 0.045), log-DIN concentrations (F = 8.305, p = 0.006), and tidal coefficient (F = 25.809, p = 0.004) were different between open and enclosed estuaries (Supplementary Table 2). In surface waters, Vv median abundance was approximately 2-fold greater in open basins than in the enclosed basins. The difference was more striking for Vp; in only three of the 25 stations, Vp abundances were at levels above the minimum detection limit in open basins (12% of samples), whereas this species was found in surface waters in 12 of the 18 locations in enclosed waterbodies (67% of samples). Median Vv and Vp in sediments, however, did not follow the same pattern of surface water Vibrio abundances. In open basins, the median Vv sediment abundance exceeded that of enclosed waterbodies by approximately 1.8-fold, while median sediment Vp was approximately 2-fold greater in enclosed systems than in open systems.
Discussion
Salinity, among others, is often considered a predictive variable for Vibrio in the water column, with optimal conditions ranging from 5 to 25 PSU (Kaspar and Tamplin, 1993; Motes et al., 1998; Hsieh et al., 2008). However, this was not the case in this study. In the seven basins examined, locations chosen for the survey represented waterbodies with a wide range of salinities and stratification (Figure 5). Within the salinity values measured at these different locations, there was no optimal salinity range where presumptive Vv or Vp was found to be more abundant (Supplementary Figure 3 and Supplementary Table 4). Moreover, bottom pH and stratification also correlated with one another, though not as dramatically (r = 0.4541, p = 0.044). This could have been attributed to the lower sample size (n = 20), as pH measurements were only taken for approximately half of all basins (Bayou Grande, Big Lagoon, and Perdido Bay). Vv abundances can increase with depth, particularly in bottom waters within shallow estuaries (Wetz et al., 2014). This trend may be attributed to variation in salinity and/or temperature at different depths, suggesting covariation between temperature and salinity in regards to estimating Vibrio abundance. However, in our study, putative abundances of Vv and Vp did not significantly vary with total water column depth, and there was no consistent pattern of depth, salinity, and Vibrio abundances. In some basins, surface water Vibrio abundances may have been greater than usual due to high wind speeds, which likely promoted mixing in the water column and resuspension of sediments. Therefore, it would have been unlikely to see a relationship between Vibrio abundances and depth. However, we did notice a strong relationship between Vibrio abundances and salinity stratification, which does in fact align with findings in previous studies, especially for shallow estuaries (Wetz et al., 2014). We also chose to sample from a diverse group of waterbodies, varying greatly in salinity, stratification, eutrophication, and freshwater influence. Although the method employed here assesses only abundances based on viable CFU, this broad range of data is useful in determining baseline abundances in the region.
Correlations of Vibrio abundance with temperature are often linked to seasons (Hsieh et al., 2008; Wetz et al., 2014). For example (Wetz et al., 2014), reported seasonal data over a four-year period for environmental parameters that may influence surface and bottom water abundances of Vv within the Neuse River Estuary (NRE). Certainly, the length of this survey capacitates the reporting of seasonal data. Although the NRE and the PBS are both shallow, river-dominated estuarine systems, and despite the contrast in duration of the study, actual relationships between water or sedimentary Vibrio abundances and environmental parameters differ depending on the influence of physicochemical factors (i.e., runoff, mixing, resuspension). Evidently, seasonal data reported for temperature, salinity, turbidity, and chl a all showed strong correlations to Vv abundances, whereas none of the four covaried with Vibrio abundances in our study (Wetz et al., 2014). In fact, surface water temperatures in this winter survey were less than optimal for maximum concentrations of Vv or Vp, presumed to fall within the range of 20 and 37°C (Tantillo et al., 2004; Johnson et al., 2012; Johnson, 2013). Maximum abundances of surface water putative Vv were in Bayou Chico, the one basin with the highest overall Vv surface water abundances. In this basin, surface water salinities were lowest of all, but temperatures (Figure 1) and salinity stratification (Supplementary Table 3) were the highest. It was surprising to see very high winter abundances in surface waters, sediments, or both, in particular basins of this study.
Surface water presumptive Vp were maximal in Bayou Texar where total DIN concentrations were high, and sedimentary presumptive Vp was greatest in Bayou Chico where chl a concentrations were high – factors that are both associated with eutrophication suspected to promote Vibrio success (Wetz et al., 2008; Turner et al., 2009; Froelich et al., 2019; Han et al., 2020). It is feared that eutrophication will promote higher concentrations of pathogens such as Vibrio species (Malham et al., 2014). Estuarine eutrophication alone, often measured by chl a concentrations, does not necessarily assess the number of higher trophic levels (Van Meerssche and Pinckney, 2019). Genomic evaluation of Vv and Vp suggests most are chitinolytic (Grimes et al., 2009), and Vibrio may be attached to zooplankton or eukaryotic phytoplankton (Takemura et al., 2014). Abundances of particle-associated estuarine Vibrio may be strongly correlated with chl a, but this relationship may vary seasonally or from year to year (Main et al., 2015). These data suggest much of the particulate material in these estuaries, terrestrial-derived and autochthonous, drives and is associated with primary production. If Vibrios in subtropical estuaries are largely associated with diatoms or zooplankton (Martinez-Urtaza et al., 2012), one might expect a positive relationship of Vibrio abundances with increased turbidity and phytoplankton biomass (chl a). However, surface water Vibrio abundances were not significantly correlated with TSS or chl a in this study. A lack of, or negative, correlation of abundance with chl a and/or TSS is consistent with findings of previous temperate estuarine studies (Wetz et al., 2014; Deeb et al., 2018). However, culture-dependent methods of total Vibrio counts do suggest their abundances are strongly and positively correlated with turbidity and DIN (Froelich et al., 2019). Here, we observed culturable presumptive Vp positively correlating with DIN and TKN, similar to the findings of (Froelich et al., 2019) in a temperate estuary. However, neither surface water putative Vv nor sedimentary putative Vp or Vv significantly covaried with nitrogen. Additionally, an earlier study (Wetz et al., 2008) of culture-dependent enumeration of total Vibrio found abundances strongly correlated with temperature during two significant storms, but abundances were correlated with salinity and TSS in only one of the two events. These findings were explained by the different wind directions and levels of sediment resuspension. Therefore, in addition to nutrients, turbidity, and phytoplankton biomass, estuarine dynamics such as tidal influence, salinity stratification, and wind-driven mixing may also be important considerations.
Salinity and stratification, both affected by wind and/or rain events, can affect Vibrio concentrations (Fries et al., 2008). Therefore, we evaluated the effect of maximum cumulative wind speeds recorded in the Pensacola metropolitan area in the three days leading up to and on the mornings of our sampling events. Wind mixing introduces sediment-dwelling bacteria into surface waters and may partly explain increased Vibrio concentrations in surface waters, particularly in Bayou Texar (02/07/20), the basin with the highest cumulative wind speeds (84 MPH). While wind-driven resuspension could explain the very high numbers of presumptive Vp in all stations sampled there, corresponding water abundances of Vv do not support wind as the sole cause for high Vp numbers on that sampling date. For example, in Bayou Texar, Vv water levels were between 89 and 207 CFU/mL, while Vp concentrations ranged from ∼1,000 to 9,000 CFU/mL. In contrast, concentrations of both species in sediments showed the opposite trend. In two of the six locations sampled, putative Vv in sediments roughly equaled those of putative Vp. At the remaining four stations, Vv exceeded Vp by at least eight-fold. It is clear that other factors, in addition to wind, are likely responsible for high Vibrio concentrations in the water column and within the sediments.
In sediments of subtropical coasts or temperate estuaries, Vp are generally more prevalent than Vv (Pfeffer et al., 2003; Vezzulli et al., 2009), but this overall pattern was not seen in this study. The relative abundances of these two species in sediments varied widely, with an approximate 1500:1 ratio of presumptive Vv:Vp in Perdido Bay, but approximately 50:1 in all other basins, except Bayou Chico, where Vv:Vp was only 6:1 (Figure 4 and Tables 2, 3). Multiple comparison procedures (Tukey’s Post Hoc) determined Vp sedimentary abundances in Pensacola and Perdido Bays were significantly lower than in the other basins. Interestingly, Vibrio abundances covaried with tidal coefficient and maximum wind speeds, indicating the influence of sediment resuspension. In this study, bottom water oxygen concentrations and putative Vibrio abundances in the sediments were not correlated. Generally, there is a lack of hypoxia in bottom waters of these basins in winter (Caffrey and Murrell, 2016), which was also found in winter 2020 (Supplementary Table 3). Since all types of putative Vibrio we enumerated except sediment Vv were significantly correlated with prior cumulative wind speeds, resuspension may be a contributing factor in explaining Vibrio in the surface waters of the Pensacola and Perdido Bay water basins.
To determine the effects of rain events prior to our sampling dates, we evaluated precipitation with respect to Vibrio abundances and environmental parameters. In all basins, total precipitation did not positively covary with turbidity as expected (Supplementary Figure 2 and Supplementary Table 4). Kd, not unexpectedly, was positively correlated with TSS (r = 0.30, p = 0.05) and chl a (r = 0.73, p < 0.0001). Pensacola Bay cyanobacterial abundances in these waters are lowest in winter (Murrell and Lores, 2004; Murrell and Caffrey, 2005), and while we did observe different chl a concentrations among the basins examined, there was no significant relationship with surface or sedimentary Vibrio abundances (Supplementary Table 4). Although we anticipated increased Vibrio densities in waters with higher turbidity, as observed previously with Vp in subtropical waters of coastal northern GoM (Zimmerman et al., 2007), abundances of Vv and Vp may not consistently covary with turbidity, where patterns are either site- or season-specific (Zimmerman et al., 2007; Blackwell and Oliver, 2008; Johnson et al., 2012). Our findings suggest terrestrial inputs of nutrients concomitant with mixing and increased turbidity are alone not sufficient to explain Vibrio success, as previously suggested for temperate estuaries (Davis et al., 2017; Froelich et al., 2019).
For the purposes of initial surveys to determine baseline levels, culture-dependent methods can provide notable ecological insights, yet also be limited in scope. For example, the false-positive rate of identifying Vv on CHROMagar as a bright blue colony was previously tested from a low-salinity body of water (Lake Ponchartrain) in the same approximate latitude as the waterbodies surveyed here (Nigro and Steward, 2015). In this typically oligohaline environment, with a salinity in the range of our study sites Bayous Texar and Chico (Supplementary Table 3), winter abundances of Vv and Vp may be overestimated by 50% (Nigro and Steward, 2015). Additionally, enumeration of Vv and Vp using the colony method likely underestimates the count, as a single particle may contain numerous Vibrio cells but still appear as a single colony (Joux et al., 2015).
False-positives may also be due to cross-reactivity of the substrate, such as blue colonies presenting as putative Vv confirmed by other methods to be V. cholerae or V. mimicus (Hara-Kudo et al., 2001). In our preliminary testing (winter and summer of 2019) of similar bodies of water, molecular method verification indicated that Vv presented not only as bright blue on this solid medium, but also vvhA-positive in end-point colony PCR, using established primers for the vvhA gene, a species-marker for Vv. Similarly, bright pink (not purple, which may be Aeromonas species) were determined to be either tdh- or trh-positive (data not shown). Molecular analyses (either end-point PCR of colonies or qPCR on DNA extracted directly from the sample) may also provide over- or underestimates of Vv abundance data, due to possible cross-reactivity of vvhA PCR primers with related Vibrio species (Canizalez-Roman et al., 2011; Klein et al., 2014). However, this is not the case for Vp-specific PCR primers, such that they do not cross-react with other species of Vibrio (e.g., V. mimivus, V. fluvialis, V. cholerae).
Another limitation of the plating method may be a potential underestimate of the Vibrio abundance due to incubation temperatures or other factors affecting bacterial growth on solid agar. Conversely, there is a possibility that not all putative Vv and Vp detected on plates may contain the established marker genes (e.g., tdh, trh for Vp or vvhA for Vv). Therefore, a combination of plating and molecular methods is ideal for enumerating putative Vibrio species in the water column or sediments. For example, in a study of Vibrio abundances responding to hurricanes, low percentages of the colonies detected on plates of water or sediment samples contained genes associated with virulence (vcgC and tdh), and none of the sediment or water colonies were positive for trh. Notably, in that study, abundances of sedimentary and water column Vv and Vp, detected with culture methods, were not significantly impacted by wind or freshening due to the storm (Shaw et al., 2014). In contrast, in our study, putative Vibrio, based on only the culture method employed here, did significantly covary with prior wind speeds. Cultivable Vv and Vp abundance determination is dependent on culture substrate, time and temperature of incubation, among other factors. In a GoM estuary near our study site, for instance, Vibrio abundances associated with finfish or shellfish were previously determined using simultaneous culture-dependent and -independent methods (Givens et al., 2014). The abundances determined by both approaches were similar, but it should be noted that molecular methods may be more sensitive when attempting to enumerate all Vibrio, culturable and non-culturable, or when enumerating only pathogenic species (Gutierrez West et al., 2013).
Additionally, both Vv and Vp can be underestimated by culture-dependent techniques as compared to molecular methods (Kirchman et al., 1982; Froelich et al., 2012; Williams et al., 2017). There was variability of putative Vibrio abundances among the few surface swab samples collected (Table 4), but this could be explained by the diversity of strains enumerated, some of which may have missed being counted due to slow recovery after plating. Recovery of Vv, Vp, or other Vibrio on various types of culture media is variable (Nordstrom and DePaola, 2003; Warner and Oliver, 2007) and possibly dependent on the nutrient state of the environment from which the sample was derived (Warner and Oliver, 2007). In this study, low abundances from swab samples may have been rectified by a longer period of incubation on agar plates or use of a different medium (Nordstrom and DePaola, 2003; Warner and Oliver, 2007). We enumerated colonies after 24, 48, and 72 h, but there were no significant increases in numbers of Vv nor Vp colonies after 48 h. Therefore, the variability of Vibrio in the opportunistically-sampled biofilms in this study may be more dependent on the abundances of Vv and Vp in the surrounding water column, but this was not seen in oysters and water samples in Tampa Bay, FL (Chase et al., 2015).
Consistent with other studies that examined subtropical water column and sediments, Vv and Vp in sediments outnumbered those in surface waters by at least 10-fold. In surface waters of temperate or subtropical estuaries, Vv distributions are highly variable (Heidelberg et al., 2002; Franco et al., 2012; Givens et al., 2014), but this may be explained by underestimates in the culture method, due to overwintering, in which Vv enter a “viable but not culturable” (VBNC) state when conditions are not optimal, such as in cooler months (Oliver and Bockian, 1995; Foster, 2004; Franco et al., 2012). In temperate systems, culture-dependent abundances in winter sediments are low compared to those in surface waters, also suggesting overwintering (Pfeffer et al., 2003), but bottom water temperatures in systems such as those examined here are generally warmer. Evaluation of subtropical Mediterranean coastal lagoon sediments did not yield cultivable Vv, but incubations were performed at higher temperatures (41°C) (Cantet et al., 2013) than in this study.
The design of this study was not to enumerate pathogenic Vv and Vp, but rather to determine baseline bacterial loads in winter for a set of waterbodies yet to be examined for Vibrio. Although the method employed here assesses only “presumptive” Vv and Vp abundances based on viable CFU, this broad range of data is useful in determining baseline abundances in the region. Cultivable abundance estimates, previously shown to be representative of consistent sample-to-sample comparison (Zimmerman et al., 2007), are valuable in and of themselves for determining relative risk factors in each waterbody of interest. Pathogenic strains’ abundances vary extensively – within samples, between sampling dates in the same location, among samples with different source salinities, among seasons, or after significant storm events (Kaufman et al., 2003; Zimmerman et al., 2007; Nigro et al., 2011; Liu et al., 2016). Therefore, to confirm estimated loads of pathogenic strains of either species measured with culture-dependent analyses, future efforts will be directed toward species-specific enumeration, with additional methods using culture-independent analyses of Vibrio species abundances.
Data Availability Statement
The raw data supporting the conclusions of this article will be made available by the authors, without undue reservation.
Author Contributions
LW and JC conceptualized the research design, executed or oversaw the laboratory assays, and analyzed the data. LW, JC, and WJ acquired the funding. LW and TP wrote the manuscript. JC donated lab resources in collaboration with the efforts of MS and MR for water quality lab analyses. WJ created the map figures. LW, JC, WJ, and BA each provided efforts in their areas of expertise. TP, JC, MS, CD, BA, and LW all assisted with field sampling and processing. All authors contributed to the article and approved the final version.
Funding
This project was supported by the Administrator of Escambia County, FL, through the University of West Florida.
Conflict of Interest
The authors declare that the research was conducted in the absence of any commercial or financial relationships that could be construed as a potential conflict of interest.
Acknowledgments
We would like to thank Gina Rodriguez, Lacey Bowman, and Julianna O’Bar for technical assistance in the lab. Field operations and sample collection would not have been possible without the help of Nine Henriksson and DJ Johnson. Advice on statistical analyses was graciously provided by Chris Pomory. Many thank are due to Erika Headrick and Mark Prousalis for lab assistance and field sampling in our preliminary (2019) method development. General information on particle loads in the estuary system studied was provided in several fruitful discussions with Mike Murrell. The revised manuscript was improved by the incorporation of many helpful comments and guidance from three reviewers.
Supplementary Material
The Supplementary Material for this article can be found online at: https://www.frontiersin.org/articles/10.3389/fmars.2021.645755/full#supplementary-material
References
Aagesen, A. M., Phuvasate, S., Su, Y.-C., and Häse, C. C. (2013). Persistence of Vibrio parahaemolyticus in the Pacific Oyster, Crassostrea gigas, is a multifactorial process involving Pili and Flagella but Not Type III secretion systems or phase variation. Appl. Environ. Microbiol. 79, 3303–3305. doi: 10.1128/aem.00314-13
Babcock, K. K., Cesbron, F., Patterson, W. F., Garner, S. B., Waidner, L. A., and Caffrey, J. M. (2020). Changing biogeochemistry and invertebrate community composition at newly deployed artificial reefs in the Northeast Gulf of Mexico. Estuar. Coasts 43, 680–692. doi: 10.1007/s12237-020-00713-4
Baker-Austin, C., and Oliver, J. D. (2018). Vibrio vulnificus: new insights into a deadly opportunistic pathogen. Environ. Microbiol. 20, 423–430. doi: 10.1111/1462-2920.13955
Blackwell, K. D., and Oliver, J. D. (2008). The ecology of Vibrio vulnificus, Vibrio cholerae, and Vibrio parahaemolyticus in North Carolina Estuaries. J. Microbiol. 46, 146–153. doi: 10.1007/s12275-007-0216-2
Caffrey, J. M., and Murrell, M. C. (2016). “A historical perspective on eutrophication in the Pensacola Bay Estuary, FL, USA,” in Aquatic Microbial Ecology and Biogeochemistry: A Dual Perspective, eds P. Glibert and T. Kana (Cham: Springer), 199–213. doi: 10.1007/978-3-319-30259-1_16
Caffrey, J. M., Hollibaugh, J. T., and Mortazavi, B. (2016). Living oysters and their shells as sites of nitrification and denitrification. Mar. Pollut. Bull. 112, 86–90. doi: 10.1016/j.marpolbul.2016.08.038
Canizalez-Roman, A. C.-R., Flores-Villaseñor, H. F.-V., Zazueta-Beltran, J. Z.-B., Muro-Amador, S. M.-A., and León-Sicairos, N. L.-S. (2011). Comparative evaluation of a chromogenic agar medium – PCR protocol with a conventional method for isolation of Vibrio parahaemolyticus strains from environmental and clinical samples. Can. J. Microbiol. 57, 136–142. doi: 10.1139/w10-108026
Cantet, F., Hervio-Heath, D., Caro, A., Le Mennec, C., Monteil, C., Quéméré, C., et al. (2013). Quantification of Vibrio parahaemolyticus, Vibrio vulnificus and Vibrio cholerae in French Mediterranean coastal lagoons. Res. Microbiol. 164, 867–874. doi: 10.1016/j.resmic.2013.06.005
Chase, E., Young, S., and Harwood, V. J. (2015). Sediment and vegetation as reservoirs of Vibrio vulnificus in the Tampa Bay Estuary and Gulf of Mexico. Appl. Environ. Microbiol. 81, 2489–2494. doi: 10.1128/aem.03243-14
Davis, B. J. K., Jacobs, J. M., Davis, M. F., Schwab, K. J., DePaola, A., and Curriero, F. C. (2017). Environmental determinants of Vibrio parahaemolyticus in the Chesapeake Bay. Appl. Environ. Microbiol. 83:e01147-17.
Deeb, R., Tufford, D., Scott, G. I., Moore, J. G., and Dow, K. (2018). Impact of climate change on Vibrio vulnificus abundance and exposure risk. Estuaries and Coasts 41, 2289–2303. doi: 10.1007/s12237-018-0424-5
Drake, S. L., DePaola, A., and Jaykus, L.-A. (2007). An overview of Vibrio vulnificus and Vibrio parahaemolyticus. Compr. Rev. Food Sci. Food Saf. 6, 120–144. doi: 10.1111/j.1541-4337.2007.00022.x
Foster, J. W. (2004). Cold-Induced Hibernation of Marine Vibrios in the Gulf of Mexico: A Study of Cell-Cell Communication and Dormancy in Vibrio vulnificus | Research Project Database | Grantee Research Project | ORD. Washington, DC: EPA.
Franco, S. L. M., Swenson, G. J., and Long, R. A. (2012). Year round patchiness of Vibrio vulnificus within a temperate Texas bay. J. Appl. Microbiol. 112, 593–604. doi: 10.1111/j.1365-2672.2011.05229.x
Fries, J. S., Characklis, G. W., and Noble, R. T. (2008). Sediment–water exchange of Vibrio sp. and fecal indicator bacteria: implications for persistence and transport in the Neuse River Estuary, North Carolina, USA. Water Res. 42, 941–950. doi: 10.1016/j.watres.2007.09.006
Froelich, B. A., and Daines, D. A. (2020). In hot water: effects of climate change on Vibrio–human interactions. Environ. Microbiol. 22, 4101–4111. doi: 10.1111/1462-2920.14967
Froelich, B., Gonzalez, R., Blackwood, D., Lauer, K., and Noble, R. (2019). Decadal monitoring reveals an increase in Vibrio spp. concentrations in the Neuse River Estuary, North Carolina, USA. PLoS One 14:e0215254. doi: 10.1371/journal.pone.0215254
Froelich, B. A., Williams, T. C., Noble, R. T., and Oliver, J. D. (2012). Apparent loss of Vibrio vulnificus from North Carolina oysters coincides with a drought-induced increase in salinity. Appl. Environ. Microbiol. 78, 3885–3889. doi: 10.1128/aem.07855-11
Givens, C. E., Bowers, J. C., DePaola, A., Hollibaugh, J. T., and Jones, J. L. (2014). Occurrence and distribution of Vibrio vulnificus and Vibrio parahaemolyticus–potential roles for fish, oyster, sediment and water. Lett. Appl. Microbiol. 58, 503–510. doi: 10.1111/lam.12226
Glavin, D. P., Cleaves, H. J., Schubert, M., Aubrey, A., and Bada, J. L. (2004). New method for estimating bacterial cell abundances in natural samples by use of sublimation. Appl. Environ. Microbiol. 70, 5923–5928. doi: 10.1128/aem.70.10.5923-5928.2004
Grimes, D. J., Johnson, C. N., Dillon, K. S., Flowers, A. R., Noriea, N. F., and Berutti, T. (2009). What genomic sequence information has revealed about Vibrio ecology in the ocean–a review. Microb. Ecol. 58, 447–460. doi: 10.1007/s00248-009-9578-9
Grodeska, S. M., Jones, J. L., Walton, W. C., and Arias, C. R. (2019). Effects of desiccation practices and ploidy in cultured oysters, Crassostrea virginica, on Vibrio spp. abundances in Portersville Bay (Alabama, USA). Aquaculture 507, 164–171. doi: 10.1016/j.aquaculture.2019.03.060
Gutierrez West, C. K., Klein, S. L., and Lovell, C. R. (2013). High frequency of virulence factor genes tdh, trh, and tlh in Vibrio parahaemolyticus strains isolated from a pristine estuary. Appl. Environ. Microbiol. 79, 2247–2252. doi: 10.1128/aem.03792-12
Hagy, J. D., and Murrell, M. C. (2007). Susceptibility of a northern Gulf of Mexico estuary to hypoxia: an analysis using box models. Estuar. Coast. Shelf Sci. 74, 239–253. doi: 10.1016/j.ecss.2007.04.013
Han, X., Schubert, C. J., Fiskal, A., Dubois, N., and Lever, M. A. (2020). Eutrophication as a driver of microbial community structure in lake sediments. Environ. Microbiol. 22, 3446–3462. doi: 10.1111/1462-2920.15115
Hara-Kudo, Y., Nishina, T., Nakagawa, H., Konuma, H., Hasegawa, J., and Kumagai, S. (2001). Improved method for detection of Vibrio parahaemolyticus in seafood. Appl. Environ. Microbiol. 67, 5819–5823. doi: 10.1128/aem.67.12.5819-5823.2001
Heidelberg, J. F., Heidelberg, K. B., and Colwell, R. R. (2002). Seasonality of Chesapeake Bay bacterioplankton species. Appl. Environ. Microbiol. 68, 5488–5497. doi: 10.1128/aem.68.11.5488-5497.2002
Hernández-Cabanyero, C., Sanjuán, E., Fouz, B., Pajuelo, D., Vallejos-Vidal, E., Reyes-López, F. E., et al. (2020). The effect of the environmental temperature on the adaptation to host in the zoonotic pathogen Vibrio vulnificus. Front. Microbiol. 11:489. doi: 10.3389/fmicb.2020.00489
Hester, C. M., Smith, H. M., Head, M. E., Langsten, H., Linder, S., Manor, E., et al. (2016). Comparing productivity and biogeochemistry of native and transplanted thalassia testudinum and halodule beaudettei in big Lagoon, Florida, USA. Gulf Mex. Sci. 1, 14–25.
Holmes, R., Aminot, A., Kerouel, R., Hooker, B. A., and Peterson, B. J. (1999). A simple and precise method for measuring ammonium in marine and freshwater ecosystems. Can. J. Fish. Aquat. Sci. 56, 1801–1808. doi: 10.1139/f99-128
Hsieh, J. L., Fries, J. S., and Noble, R. T. (2008). Dynamics and predictive modelling of Vibrio spp. in the Neuse River Estuary, North Carolina, USA. Environ. Microbiol. 10, 57–64.
Hughes, S. N., Greig, D. J., Miller, W. A., Byrne, B. A., Gulland, F. M. D., and Harvey, J. T. (2013). Dynamics of Vibrio with virulence Genes detected in Pacific Harbor seals (Phoca vitulina richardii) Off California: implications for marine mammal health. Microb. Ecol. 65, 982–994. doi: 10.1007/s00248-013-0188-1
Huq, A., Haley, B. J., Taviani, E., Chen, A., Hasan, N. A., and Colwell, R. R. (2012). Detection, isolation, and identification of Vibrio cholerae from the environment. Curr. Protoc. Microbiol 26, 6A.5.1–6A.5.51.
Johnson, C. N. (2013). Fitness factors in vibrios: a mini-review. Microb. Ecol. 65, 826–851. doi: 10.1007/s00248-012-0168-x
Johnson, C. N., Bowers, J. C., Griffitt, K. J., Molina, V., Clostio, R. W., Pei, S., et al. (2012). Ecology of Vibrio parahaemolyticus and Vibrio vulnificus in the Coastal and Estuarine Waters of Louisiana, Maryland, Mississippi, and Washington (United States). Appl. Environ. Microbiol. 78, 7249–7257. doi: 10.1128/aem.01296-12
Joux, F., Bertrand, J.-C., De Wit, R., Grossi, V., Intertaglia, L., Lebaron, P., et al. (2015). “Methods for studying microorganisms in the environment,” in Environmental Microbiology: Fundamentals and Applications: Microbial Ecology, eds J.-C. Bertrand, P. Caumette, P. Lebaron, R. Matheron, P. Normand, and T. Sime-Ngando (Dordrecht: Springer Netherlands), 757–829.
Kaspar, C. W., and Tamplin, M. L. (1993). Effects of temperature and salinity on the survival of Vibrio vulnificus in seawater and shellfish. Appl. Environ. Microbiol. 59, 2425–2429. doi: 10.1128/aem.59.8.2425-2429.1993
Kaufman, G. E., Bej, A. K., Bowers, J., and DePAOLA, A. (2003). Oyster-to-Oyster variability in levels of Vibrio parahaemolyticus. J. Food Prot. 66, 125–129. doi: 10.4315/0362-028x-66.1.125
Kirchman, D., Sigda, J., Kapuscinski, R., and Mitchell, R. (1982). Statistical analysis of the direct count method for enumerating bacteria. Appl. Environ. Microbiol. 44, 376–382. doi: 10.1128/aem.44.2.376-382.1982
Klein, S. L., Gutierrez West, C. K., Mejia, D. M., and Lovell, C. R. (2014). Genes Similar to the Vibrio parahaemolyticus virulence-related genes tdh, tlh, and vscC2 Occur in other vibrionaceae species isolated from a pristine estuary. Appl. Environ. Microbiol. 80, 595–602. doi: 10.1128/aem.02895-13
Krishna, K., Veettil, V. P., Anas, A., and Nair, S. (2020). Hydrological regulation of Vibrio dynamics in a tropical monsoonal estuary: a classification and regression tree approach. Environ. Sci. Pollut. Res. 28, 724–737. doi: 10.1007/s11356-020-10486-9
Lewis, M., Kirschenfeld, J., and Goodhart, T. (2016). Environmental Quality of the Pensacola Bay System: Retrospective Review for Future Resource Management and Rehabilitation. Washington, D.C: Environmental Protection Agency.
Lewis, M. A., Weber, D. L., and Moore, J. C. (2002). An evaluation of the use of colonized periphyton as an indicator of wastewater impact in near-coastal areas of the Gulf of Mexico. Arch. Environ. Contam. Toxicol. 43, 11–18. doi: 10.1007/s00244-001-0054-x
Li, N., Dong, K., Jiang, G., Tang, J., Xu, Q., Li, X., et al. (2020). Stochastic processes dominate marine free-living Vibrio community assembly in a subtropical gulf. FEMS Microbiol. Ecol. 96:fiaa198.
Lipp, E. K., Rodriguez-Palacios, C., and Rose, J. B. (2001). Occurrence and distribution of the human pathogen Vibrio vulnificus in a subtropical Gulf of Mexico estuary. Hydrobiologia 460, 165–173. doi: 10.1007/978-94-017-3284-0_15
Liu, B., Liu, H., Pan, Y., Xie, J., and Zhao, Y. (2016). Comparison of the effects of environmental parameters on the growth variability of Vibrio parahaemolyticus coupled with strain sources and genotypes analyses. Front. Microbiol. 7:994. doi: 10.3389/fmicb.2016.00994
Lønborg, C., Baltar, F., Carreira, C., and Morán, X. A. G. (2019). Dissolved organic carbon source influences tropical coastal heterotrophic bacterioplankton response to experimental warming. Front. Microbiol 10:2807. doi: 10.3389/fmicb.2019.02807
Lovell, C. R. (2017). Ecological fitness and virulence features of Vibrio parahaemolyticus in estuarine environments. Appl. Microbiol. Biotechnol. 101, 1781–1794. doi: 10.1007/s00253-017-8096-9
Luna, G. M., Manini, E., and Danovaro, R. (2002). Large fraction of dead and inactive bacteria in coastal marine sediments: comparison of protocols for determination and ecological significance. Appl. Environ. Microbiol. 68, 3509–3513. doi: 10.1128/aem.68.7.3509-3513.2002
Macauley, J. M., Engle, V. D., Summers, J. K., Clark, J. R., and Flemer, D. A. (1995). An assessment of water quality and primary productivity in Perdido Bay, a Northern Gulf of Mexico Estuary. Environ. Monit. Assess. 36, 191–205. doi: 10.1007/bf00547901
Main, C. R., Salvitti, L. R., Whereat, E. B., and Coyne, K. J. (2015). Community-level and species-specific associations between phytoplankton and particle-associated Vibrio species in delaware’s inland bays. Appl. Environ. Microbiol. 81, 5703–5713. doi: 10.1128/AEM.00580-15
Malham, S. K., Rajko-Nenow, P., Howlett, E., Tuson, K. E., Perkins, T. L., Pallett, D. W., et al. (2014). The interaction of human microbial pathogens, particulate material and nutrients in estuarine environments and their impacts on recreational and shellfish waters. Environ. Sci. Process. Impacts 16, 2145–2155. doi: 10.1039/c4em00031e
Martinez-Urtaza, J., Blanco-Abad, V., Rodriguez-Castro, A., Ansede-Bermejo, J., Miranda, A., and Rodriguez-Alvarez, M. X. (2012). Ecological determinants of the occurrence and dynamics of Vibrio parahaemolyticus in offshore areas. ISME J. 6, 994–1006. doi: 10.1038/ismej.2011.156
Montánchez, I., Ogayar, E., Plágaro, A. H., Esteve-Codina, A., Gómez-Garrido, J., Orruño, M., et al. (2019). Analysis of Vibrio harveyi adaptation in sea water microcosms at elevated temperature provides insights into the putative mechanisms of its persistence and spread in the time of global warming. Sci. Rep. 9:289.
Motes, M. L., DePaola, A., Cook, D. W., Veazey, J. E., Hunsucker, J. C., Garthright, W. E., et al. (1998). Influence of water temperature and salinity on Vibrio vulnificus in Northern Gulf and Atlantic Coast oysters (Crassostrea virginica). Appl. Environ. Microbiol. 64, 1459–1465. doi: 10.1128/aem.64.4.1459-1465.1998
Murrell, M. C. (2003). Bacterioplankton dynamics in a subtropical estuary: evidence for substrate limitation. Aquat. Microb. Ecol. 32, 239–250. doi: 10.3354/ame032239
Murrell, M., and Caffrey, J. (2005). High cyanobacterial abundance in three Northeastern Gulf of Mexico Estuaries. Gulf Caribb. Res. 17, 95–106.
Murrell, M. C., and Lores, E. M. (2004). Phytoplankton and zooplankton seasonal dynamics in a subtropical estuary: importance of cyanobacteria. J. Plankton Res. 26, 371–382. doi: 10.1093/plankt/fbh038
Murrell, M. C., Hagy, J. D., Lores, E. M., and Greene, R. M. (2007). Phytoplankton production and nutrient distributions in a subtropical estuary: importance of freshwater flow. Estuar. Coasts 30, 390–402. doi: 10.1007/bf02819386
Murrell, M. C., Campbell, J. G., Hagy, J. D., and Caffrey, J. M. (2009). Effects of irradiance on benthic and water column processes in a Gulf of Mexico estuary: Pensacola Bay, Florida, USA. Estuar. Coast. Shelf Sci. 81, 501–512. doi: 10.1016/j.ecss.2008.12.002
Murrell, M. C., Caffrey, J. M., Marcovich, D. T., Beck, M. W., Jarvis, B. M., and Hagy, J. D. (2018). Seasonal oxygen dynamics in a warm temperate estuary: effects of hydrologic variability on measurements of primary production, respiration, and net metabolism. Estuar. Coasts 41, 690–707. doi: 10.1007/s12237-017-0328-9
Nature Conservancy. (2014). Pensacola Bay Community-Based Watershed Plan 2014 Report. Maitland, FL: The Nature Conservancy.
Nigro, O. D., and Steward, G. F. (2015). Differential specificity of selective culture media for enumeration of pathogenic vibrios: advantages and limitations of multi-plating methods. J. Microbiol. Methods 111, 24–30. doi: 10.1016/j.mimet.2015.01.014
Nigro, O. D., Hou, A., Vithanage, G., Fujioka, R. S., and Steward, G. F. (2011). Temporal and spatial variability in culturable pathogenic Vibrio spp. in Lake Pontchartrain, Louisiana, following Hurricanes Katrina and Rita. Appl. Environ. Microbiol. 77, 5384–5393. doi: 10.1128/aem.02509-10
Nordstrom, J. L., and DePaola, A. (2003). Improved recovery of pathogenic Vibrio parahaemolyticus from oysters using colony hybridization following enrichment. J. Microbiol. Methods 52, 273–277. doi: 10.1016/s0167-7012(02)00188-4
Nordstrom, J. L., Vickery, M. C. L., Blackstone, G. M., Murray, S. L., and DePaola, A. (2007). Development of a multiplex real-time PCR assay with an internal amplification control for the detection of total and pathogenic Vibrio parahaemolyticus bacteria in oysters. Appl. Environ. Microbiol. 73, 5840–5847. doi: 10.1128/aem.00460-07
Oliver, J. D. (2003). “Chapter 17 Culture media for the isolation and enumeration of pathogenic Vibrio species in foods and environmental samples,” in Progress in Industrial Microbiology, eds J. E. L. Corry, G. D. W. Curtis, and R. M. Baird (Amsterdam: Elsevier), 249–269. doi: 10.1016/s0079-6352(03)80020-6
Oliver, J. D., and Bockian, R. (1995). In vivo resuscitation, and virulence towards mice, of viable but nonculturable cells of Vibrio vulnificus. Appl. Environ. Microbiol. 61, 2620–2623. doi: 10.1128/aem.61.7.2620-2623.1995
Paczkowska, J., Rowe, O. F., Figueroa, D., and Andersson, A. (2019). Drivers of phytoplankton production and community structure in nutrient-poor estuaries receiving terrestrial organic inflow. Mar. Environ. Res. 151:104778. doi: 10.1016/j.marenvres.2019.104778
Panicker, G., and Bej, A. K. (2005). Real-Time PCR detection of Vibrio vulnificus in oysters: comparison of oligonucleotide primers and probes targeting vvhA. Appl. Environ. Microbiol. 71, 5702–5709. doi: 10.1128/aem.71.10.5702-5709.2005
Panicker, G., Myers, M. L., and Bej, A. K. (2004). Rapid detection of Vibrio vulnificus in shellfish and Gulf of Mexico water by Real-Time PCR. Appl. Environ. Microbiol. 70, 498–507. doi: 10.1128/aem.70.1.498-507.2004
Parsons, T. R., Maita, Y., and Lalli, C. M. (1984). A Manual of Chemical & Biological Methods for Seawater Analysis. Amsterdam: Elsevier.
Pfeffer, C. S., Hite, M. F., and Oliver, J. D. (2003). Ecology of Vibrio vulnificus in estuarine waters of eastern North Carolina. Appl. Environ. Microbiol. 69, 3526–3531. doi: 10.1128/aem.69.6.3526-3531.2003
Proctor, L. M., and Souza, A. C. (2001). Method for enumeration of 5-cyano-2,3-ditoyl tetrazolium chloride (CTC)-active cells and cell-specific CTC activity of benthic bacteria in riverine, estuarine and coastal sediments. J. Microbiol. Methods 43, 213–222. doi: 10.1016/s0167-7012(00)00218-9
Pruente, V. L., Jones, J. L., Steury, T. D., and Walton, W. C. (2020). Effects of tumbling, refrigeration and subsequent resubmersion on the abundance of Vibrio vulnificus and Vibrio parahaemolyticus in cultured oysters (Crassostrea virginica). Int. J. Food Microbiol. 335:108858. doi: 10.1016/j.ijfoodmicro.2020.108858
Pruzzo, C., Huq, A., Colwell, R. R., and Donelli, G. (2005). “Pathogenic Vibrio species in the marine and estuarine environment,” in Oceans and Health: Pathogens in the Marine Environment, eds S. Belkin and R. R. Colwell (Boston, MA: Springer US), 217–252. doi: 10.1007/0-387-23709-7_9
Ray, N. E., Henning, M. C., and Fulweiler, R. W. (2019). Nitrogen and phosphorus cycling in the digestive system and shell biofilm of the eastern oyster Crassostrea virginica. Mar. Ecol. Prog. Ser. 621, 95–105. doi: 10.3354/meps13007
Schnetger, B., and Lehners, C. (2014). Determination of nitrate plus nitrite in small volume marine water samples using vanadium(III)chloride as a reduction agent. Mar. Chem. 160, 91–98. doi: 10.1016/j.marchem.2014.01.010
Shaw, K. S., Jacobs, J. M., and Crump, B. C. (2014). Impact of Hurricane Irene on Vibrio vulnificus and Vibrio parahaemolyticus concentrations in surface water, sediment, and cultured oysters in the Chesapeake Bay, MD, USA. Front. Microbiol 5:204. doi: 10.3389/fmicb.2014.00204
Sieracki, C. K., Sieracki, M. E., and Yentsch, C. S. (1998). An imaging-in-flow system for automated analysis of marine microplankton. Mar. Ecol. Prog. Ser. 168, 285–296. doi: 10.3354/meps168285
Takemura, A. F., Chien, D. M., and Polz, M. F. (2014). Associations and dynamics of Vibrionaceae in the environment, from the genus to the population level. Front. Microbiol 5:38. doi: 10.3389/fmicb.2014.00038
Tantillo, G. M., Fontanarosa, M., Pinto, A. D., and Musti, M. (2004). Updated perspectives on emerging vibrios associated with human infections. Lett. Appl. Microbiol. 39, 117–126. doi: 10.1111/j.1472-765x.2004.01568.x
Thomas, F. (2016). Of life and limb: the failure of Florida’s water quality criteria to test for Vibrio Vulnificus in coastal waters and the need for enhanced criteria, regulation, and notification to protect public health. Sea Grant Law Policy J. 7, 1–45. doi: 10.1002/9780470518328.ch1
Thomas, P., Mujawar, M. M., Sekhar, A. C., and Upreti, R. (2014). Physical impaction injury effects on bacterial cells during spread plating influenced by cell characteristics of the organisms. J. Appl. Microbiol. 116, 911–922. doi: 10.1111/jam.12412
TidalCoefficient (2020). Tidal Coefficient Data. Available online at: https://tides4fishing.com/tides/tidal-coefficient (accessed January 15, 2020).
Turner, J. W., Good, B., Cole, D., and Lipp, E. K. (2009). Plankton composition and environmental factors contribute to Vibrio seasonality. ISME J. 3, 1082–1092. doi: 10.1038/ismej.2009.50
Van Meerssche, E., and Pinckney, J. L. (2019). Nutrient loading impacts on estuarine phytoplankton size and community composition: community-based indicators of eutrophication. Estuar. Coasts 42, 504–512. doi: 10.1007/s12237-018-0470-z
Vezzulli, L., Pezzati, E., Moreno, M., Fabiano, M., Pane, L., Pruzzo, C., et al. (2009). Benthic ecology of Vibrio spp. and pathogenic Vibrio species in a coastal Mediterranean environment (La Spezia Gulf, Italy). Microb. Ecol. 58, 808–818. doi: 10.1007/s00248-009-9542-8
Warner, E., and Oliver, J. D. (2007). Refined medium for direct isolation of Vibrio vulnificus from oyster tissue and seawater. Appl. Environ. Microbiol. 73, 3098–3100. doi: 10.1128/aem.02245-06
Welschmeyer, N. A. (1994). Fluorometric analysis of chlorophyll a in the presence of chlorophyll b and pheopigments. Limnol. Oceanogr. 39, 1985–1992. doi: 10.4319/lo.1994.39.8.1985
Wetz, J. J., Blackwood, A. D., Fries, J. S., Williams, Z. F., and Noble, R. T. (2008). Trends in total Vibrio spp. and Vibrio vulnificus concentrations in the eutrophic Neuse River Estuary, North Carolina, during storm events. Aquat. Microb. Ecol. 53, 141–149. doi: 10.3354/ame01223
Wetz, J. J., Blackwood, A. D., Fries, J. S., Williams, Z. F., and Noble, R. T. (2014). Quantification of Vibrio vulnificus in an estuarine environment: a multi-year analysis using QPCR. Estuar. Coasts 37, 421–435. doi: 10.1007/s12237-013-9682-4
Williams, A. K., and Quigg, A. (2019). Spatiotemporal variability in autotrophic and heterotrophic microbial plankton abundances in a subtropical estuary (Galveston Bay, Texas). J. Coast. Res. 35, 434–444. doi: 10.2112/jcoastres-d-18-00004.1
Williams, L. A., and LaRock, P. A. (1985). Temporal occurrence of vibrio species and aeromonas hydrophila in estuarine sediments. Appl. Environ. Microbiol. 50, 1490–1495. doi: 10.1128/aem.50.6.1490-1495.1985
Williams, T. C., Froelich, B. A., Phippen, B., Fowler, P., Noble, R. T., and Oliver, J. D. (2017). Different abundance and correlational patterns exist between total and presumed pathogenic Vibrio vulnificus and V. parahaemolyticus in shellfish and waters along the North Carolina coast. FEMS Microbiol. Ecol 93: fix071.
WUnderground (2020). Weather Underground Data. Available online at: www.wunderground.com/history/daily/us/fl/pensacola/KPNS/date/ (accessed January 20, 2020).
Yeung, M., and Thorsen, T. (2016). Development of a more sensitive and specific chromogenic agar medium for the detection of Vibrio parahaemolyticus and other vibrio species. J. Vis. Exp. 117:e54493. doi: 10.3791/54493
Zhang, X.-H., and Austin, B. (2005). Haemolysins in Vibrio species. J. Appl. Microbiol. 98, 1011–1019. doi: 10.1111/j.1365-2672.2005.02583.x
Keywords: Vibrio parahaemolyticus, Vibrio vulnificus, estuary, water column, sediment, viable, resuspension, stratification
Citation: Potdukhe TV, Caffrey JM, Rothfus MJ, Daniel CE, Swords ME III, Albrecht BB, Jeffrey WH and Waidner LA (2021) Viable Putative Vibrio vulnificus and parahaemolyticus in the Pensacola and Perdido Bays: Water Column, Sediments, and Invertebrate Biofilms. Front. Mar. Sci. 8:645755. doi: 10.3389/fmars.2021.645755
Received: 23 December 2020; Accepted: 15 April 2021;
Published: 07 June 2021.
Edited by:
Robinson W (Wally) Fulweiler, Boston University, United StatesReviewed by:
Jessica L. Jones, United States Food and Drug Administration, United StatesZelam Kaluskar, Louisiana State University, United States
Kristin M. Burkholder, University of New England, United States
Jessica Vorse, University of New England, United States, in collaboration with reviewer KB
Copyright © 2021 Potdukhe, Caffrey, Rothfus, Daniel, Swords, Albrecht, Jeffrey and Waidner. This is an open-access article distributed under the terms of the Creative Commons Attribution License (CC BY). The use, distribution or reproduction in other forums is permitted, provided the original author(s) and the copyright owner(s) are credited and that the original publication in this journal is cited, in accordance with accepted academic practice. No use, distribution or reproduction is permitted which does not comply with these terms.
*Correspondence: Lisa A. Waidner, bHdhaWRuZXJAdXdmLmVkdQ==