- 1Third Institute of Oceanography, Ministry of Natural Resources, Xiamen, China
- 2Fujian Provincial Key Laboratory of Marine Ecological Conservation and Restoration, Xiamen, China
- 3Fujian Provincial Station for Field Observation and Research of Island and Coastal Zone, Zhangzhou, China
- 4State Key Laboratory of Marine Environmental Science, College of Ocean and Earth Sciences, Xiamen University, Xiamen, China
- 5Marine Genomics and Biotechnology Program, Institute of Marine Science and Technology, Shandong University, Qingdao, China
- 6National Center of Ocean Standard and Metrology, Tianjin, China
The supply of metabolites from symbionts to scleractinian corals is crucial to coral health. Members of the Symbiodiniaceae can enhance coral calcification by providing photosynthetically fixed carbon (PFC) and energy, whereas dinitrogen (N2)-fixing bacteria can provide additional nutrients such as diazotrophically-derived nitrogen (DDN) that sustain coral productivity especially when alternative external nitrogen sources are scarce. How these mutualistic associations benefit corals in the future acidifying ocean is not well understood. In this study, we investigated the possible effects of ocean acidification (OA; pHs 7.7 and 7.4 vs. 8.1) on calcification in the hermatypic coral Galaxea fascicularis with respect to PFC and DDN assimilation. Our measurements based on isotopic tracing showed no significant differences in the assimilation of PFC among different pH treatments, but the assimilation of DDN decreased significantly after 28 days of stress at pH 7.4. The decreased DDN assimilation suggests a nitrogenous nutrient deficiency in the coral holotiont, potentially leading to reduced coral calcification and resilience to bleaching and other stressful events. This contrasting impact of OA on carbon and N flux demonstrates the flexibility of G. fascicularis in coping with OA, apparently by sustaining a largely undamaged photosystem at the expense of N2 fixation machinery, which competes with coral calcification for energy from photosynthesis. These findings shed new light on the critically important but more vulnerable N cycling in hospite, and on the trade-off between coral hosts and symbionts in response to future climate change.
Introduction
Nutritional interactions are a key reason why scleractinian corals, the main reef builders in the oceans, thrive in the oligotrophic tropical waters where nitrogen (N) sources are particularly scarce (Muscatine and Porter, 1977; Cardini et al., 2014; Peixoto et al., 2017). The obligate relationship between corals and Symbiodiniaceae is the basis of a functioning reef ecosystem. The coral host provides the endosymbionts with inorganic nutrients for photosynthesis, and in turn acquires photosynthetically fixed carbon (PFC) from the symbionts in the form of glycerol, glucose, amino acids, and other organic compounds that the host cannot synthesize independently (Rowan, 1998). Symbiodiniaceae, hence, play a central role in the trophic foundation of coral reef ecosystems (Silveira et al., 2017) and in the formation of the physical reef structure (Tambutté et al., 2011).
Other microbes are increasingly being recognized as important to the health of the corals and in coral reef biogeochemistry, particularly diazotrophs including dinitrogen (N2)-fixing bacteria and archaea (Lema et al., 2014; Peixoto et al., 2017). N2 fixation is one of the main sources of new N in the ocean necessary to sustain marine primary productivity, particularly in coral reef ecosystems where the availability of nitrogenous nutrients can be low (Benavides et al., 2017). Diazotrophs are able to fix N2 utilizing the nitrogenase enzyme, which cleaves the triple bond of the N2 molecule to form bioavailable ammonium (NH4+; Zehr and Kudela, 2011). In this context, endosymbiotic photosynthesis-dependent diazotrophic cyanobacteria were discovered in the coral tissue of Montastraea cavernosa (Lesser et al., 2004), and N2 fixation activity has been detected in several coral species (Lesser et al., 2007). High throughput sequencing has subsequently identified diverse communities of non-cyanobacterial diazotrophs associated with numerous scleractinian corals from varying geographical regions (Fiore et al., 2010; Olson and Lesser, 2013), exemplifying their importance in meeting nutritional demand of corals (Fiore et al., 2010; Cardini et al., 2014). At the ecosystem level, up to 11% of the N used in coral reef primary production is provided by diazotrophs in supporting the productivity of that ecosystem (Cardini et al., 2014; Lema et al., 2016). The ability of corals to overcome N limitation may determine their success in oligotrophic waters, ultimately influencing the distribution and abundance of coral reefs (Fiore et al., 2010). Additionally, Symbiodiniaceae and associated bacteria form calcifying structures termed symbiolites (Frommlet et al., 2015). This bacterial–algal calcification, induced by Symbiodiniaceae photosynthesis through the assimilation of CO2 and HCO3– and the release of hydroxyl ion from carbon (C) concentrating mechanism, implies that symbiotic bacteria might also facilitate coral calcification (Frommlet et al., 2018).
As a result of anthropogenically-driven increase in the ocean concentration of carbon dioxide (CO2), tropical coral reefs are highly vulnerable to ocean acidification (OA). This is because the ecosystem structure depends on calcium carbonate-secreting organisms, which are subject to negative impacts of changing ocean carbonate chemistry associated with OA (Byrne et al., 2013; Leung et al., 2017, 2020; Mollica et al., 2018). It has been estimated that coral reefs may transition from net calcium carbonate accretion to net dissolution by the end of this century (Hoegh-Guldberg et al., 2007; Dove et al., 2013; Enochs et al., 2016; Eyre et al., 2018). OA not only inhibits calcification, reduces photosynthesis and species diversity directly, but also indirectly impacts coral-associated microbes, thereby potentially disrupting the normal function of the coral holobiont. This loss of function may in turn impact coral reef ecosystems as a whole (Moya et al., 2012; Kaniewska et al., 2015). However, N2 fixation activities in the coral holobiont may moderate the host’s response to stress (Rädecker et al., 2015). The increased transfer of diazotrophically-derived nitrogen (DDN) to endosymbiotic Symbiodiniaceae occurs particularly under conditions of low availability of external nutrients or during stress (Bednarz et al., 2017, 2019). Moreover, N2 fixation may be particularly important for providing nutrients to the host under stressful conditions, especially when Symbiodiniaceae are lost from host tissues (Fine and Loya, 2002). Therefore, N2 fixation may play a key role in regulating coral–Symbiodiniaceae symbiosis.
However, investigations into the coral nutrient were mainly focused on C fixation (Tremblay et al., 2012; Hoadley et al., 2015), and few on N2 fixation (Bednarz et al., 2017; Lesser et al., 2019). The effects of OA on these processes remain largely unknown and there is simply one study on N2 fixation response to OA according to the best of our current knowledge (Rädecker et al., 2014). N2 fixation rates can be detected through the acetylene reduction assay (Capone, 1993) and the 15N2-tracer method (Montoya et al., 1996). The former is an indirect method, converting acetylene produced to overall rates of N2 fixation but usually overestimating the rates. Instead, the latter allows measurement of the net N2 fixation directly, simply by adding filtered seawater enriched with 15N2 gas to the incubation to ensure consistency of 15N2 concentrations (White et al., 2020). In this study, we used H13CO3– and 15N2 as tracers and performed indoor experiments to mimic OA conditions (pHs 7.7 and 7.4) relative to the ambient condition in the field (pH 8.1 as control) to investigate: (i) Whether OA would affect PFC and DDN assimilation in the ecologically important scleractinian coral G. fascicularis; (ii) Is there a correlation between the C/N flux and coral calcification and how they interact with each other to cope with OA.
Materials and Methods
Experimental Setup
Twelve colonies of healthy, adult G. fascicularis with surface areas ranging from 10 to 15 cm2 were collected from Changjiang (110°65′E, 19°25′N), Hainan, China, at noon in July 2015. The corals were cultivated in an aquarium with approximately 1,000 L of recirculated artificial seawater for up to 3 months of acclimation. The coral genotype was identified as the mt-L1 type of G. fascicularis (Lin et al., 2017). A detailed description of the aquarium setup and water quality has been reported elsewhere (Zheng et al., 2018a). Photosynthetically active radiation (PAR) of 150 ± 25 μmol photons m–2 s–1 was provided on a 12 h/12 h photoperiod using metal halide lamps (Phillips, Amsterdam, Netherlands). The live rock containing denitrifying bacteria was used to stabilize the water quality by removing dissolved inorganic nitrogen (DIN; Li et al., 2017). The DIN and dissolved inorganic phosphorus (DIP) concentrations were all < 0.05 μM.
Fragments (∼3 cm in diameter) of G. fascicularis were grown in the aquarium until coral tissue entirely covered the skeleton. Ninety coral fragments were randomly and equally assigned to three 100-L experimental tanks (30 fragments per tank), each maintained at a specific pH (8.1, 7.7, and 7.4). The carbonate chemistry was manipulated by bubbling pure CO2 gas (99% purity) into the artificial seawater in the experimental tanks to maintain the pH (total scale, pHT) levels (Supplementary Table 1) with a pH-stat system (CO2SYS ZB-LS 3.0; Xiamen, China), which monitors and regulates the pH every second with an accuracy of ± 0.02 pH unit (Zheng et al., 2018b). The pH in each tank was recorded every 5 min by the CO2SYS ZB-LS 3.0 (Supplementary Figure 1).
The three tanks were maintained at a salinity of approximately 34 ppt and a temperature of 27°C, and irradiated on a 12 h/12 h photoperiod with 150 μmol photons m–2 s–1 of PAR provided by T5HO lamps (ATI, Hamm, Germany). Submersible pumps (EHEIM, Deizisau, Germany) were used to ensure a high level of seawater circulation and easy dissolution of the CO2 gas into the seawater. A protein skimmer (Bubble Magus, Jiangmen, China) was used to maintain the inorganic nutrients at low levels (Supplementary Table 1).
While parallel, independent replicate tanks were not used per each pH treatment, the water quality was well maintained over the course of experiment and no statistical differences were found among the tanks for all the environmental parameters measured except the pH (Supplementary Table 1). Given that the number of replicate coral fragments in each tank was high and comparable to those in previous studies (Rädecker et al., 2014; Kurman et al., 2017), the resulting data should be regarded as reliable as in other OA studies that also adopted a single tank for each pH treatment (Barkley et al., 2017; Comeau et al., 2017; Kurman et al., 2017; Coronado et al., 2019). Additionally, the tanks were rigorously cleaned weekly to eliminate “tank” effects and ensure stable water quality throughout the experiment.
Monitoring of Water Quality and Carbonate Chemistry
A total of 300 mL seawater was sampled weekly for the measurement of inorganic nutrient concentrations (PO43–, NO3–, NH4+, and SiO32–) and carbonate chemistry (pH and total alkalinity: TA). Saturated mercuric chloride (7 mg mL–1) was added to preserve the water samples. An aliquot of 100 mL seawater was used for the measurement of inorganic nutrients using a 7,230 Spectrophotometer (Jingmi, Shanghai, China). The pH was measured using a 3,430 portable meter (WTW, Munich, Germany) that was calibrated daily, and TA was measured by potentiometric titration following the standard procedure (Dickson et al., 2007). Changes in seawater carbonate chemistry were calculated from pHT and TA using the CO2 Sys Excel Macro (Lewis et al., 1998).
Measurement of Photochemical Efficiency
The photochemical efficiency of the algal symbiont was assessed using an underwater chlorophyll fluorometer (Diving-PAM; Walz, Effeltrich, Germany) that measured the quantum yield of chlorophyll a fluorescence. Measurements of the maximum quantum yield (Fv/Fm) and effective quantum yield (ΔF/Fm′) of photosystem II (PSII) were conducted 2 h into the dark and light periods, respectively, in every 14 days (n = 6).
Measurement of PFC and DDN Assimilation
The 13C and 15N stable isotopic tracing experiment was carried out to quantify the rates of PFC and DDN assimilation in the coral holobiont. The DDN and PFC assimilation rates were measured using the dissolution method (Großkopf et al., 2012). In summary, 15N2 pre-dissolved seawater was made with 15N2 gas (98.9 atom%, Cambridge Isotope Laboratories) following the procedure of Shiozaki et al. (2015). Seawater was filtered through 0.2 μm membrane, degassed using Sterapore membrane unit (20 M 1,500 A: Mitsubishi Rayon Co., Ltd., Tokyo, Japan), and filled into 2-L Tedlar bags. Twenty milliliter, of 15N2 was injected into each seawater-filled bag, which was tapped slightly until complete dissolution of the gas. The coral fragments were incubated, with one fragment per 1-L polycarbonate bottle (Nalgene, Rochester, NY, United States, the actual volume is ∼1,225 mL) filled with 100 mL of the 15N2 pre-dissolved seawater and 1,125 mL seawater from the corresponding experimental tanks (pHs 8.1, 7.7, and 7.4). The 13C-labeled sodium bicarbonate (99 atom% 13C; Cambridge Isotope Laboratories) was added in parallel with 15N2 at a final tracer concentration of 70 μmol⋅L–1. The incubation lasted 24 h (12 h light/12 h dark; 150 μmol photons m–2⋅s–1). Coral fragments were sampled at the start and end of the isotope tracer experiment to assess the PFC and DDN assimilation rates. Since OA has negligible effect on coral respiration (Comeau et al., 2017; Van der Zande et al., 2020), a 24-h incubation represents the net assimilation of PFC, which has subtracted the respiration of consumed metabolites. The samples collected prior to the incubation were also used to assess the natural isotope ratios δ13C and δ15N. Samples were immediately snap-frozen in liquid nitrogen followed by storage at −80°C until further processing.
Coral tissue was detached from the skeleton using a WaterPik, filtered onto pre-combusted (450°C, 4 h) 0.3 μm GF-75 filters (Advantec, Taiwan, China) and dried at 60°C overnight. The isotopic values were determined using a Delta V plus isotope ratio mass spectrometer (Thermo Fisher Corporation, Carthage, MO, United States) interfaced with a Flash HT 2000 elemental analyzer (Thermo Fisher Corporation, Carthage, MO, United States). International reference material (USGS40) with a different amount of C/N and certified δ13C and δ15N values of −4.5 and −26.2‰, respectively, was inserted every 5 samples to check the drift and to ensure the accuracy of the measurements. The reproducibility for δ13C and δ15N measurements were both better than 0.3‰. The PFC and DDN assimilation rates were calculated by using equations proposed by Hama et al. (1983) and Montoya et al. (1996), respectively.
Measurement of Coral Calcification
The coral calcification rates were determined using the buoyant weight method measuring increase in skeletal mass, as reported previously (Davies, 1989). Briefly, the coral fragments were suspended in seawater beneath an analytical balance that weighs to an accuracy of 0.01 mg. Optimal weighing conditions were reached when the air temperature was stable and close to that of the seawater. A total of eight replicates (n = 8) of coral fragments were measured at two timepoints (day = 14 and 28).
Statistical Analysis
Differences in water quality (pH, TA, pCO2, and inorganic nutrients) and all parameters among the pH treatments were compared using one-way analysis of variance (ANOVA). The effects of pH and time on the physiological parameters (ΔF/Fm′, Fv/Fm, calcification) were tested using two-way ANOVA. The post-eriori Tukey’s test was followed when the differences were significant (p < 0.05). Data were checked for normality and homogeneity (Shapiro-Wilk and Levene tests, respectively), and when necessary were transformed to achieve ANOVA assumptions. All data were expressed as an average value ± standard error (SE). Statistical analysis was performed using IBM SPSS Statistics 23 (IBM Corp., Armonk, NY, United States).
Results
Water Quality and Carbonate Chemistry of Seawater
The results of the water quality and carbonate chemistry analyses of seawater are shown in Supplementary Table 1. Over the 28-day experimental period, temperature and salinity were kept at 27 ± 0.3°C and 34 ± 0.1 ppt, respectively. No differences in the water temperature and salinity were found among the pH treatments. The inorganic nutrient concentrations were low and comparable to those in the field, with approximate concentrations of 0.03−0.05 μM for phosphate (PO43+), 0.45−0.58 μM for nitrite (NO2–), < 0.05 for nitrate (NO3–), 0.18−0.51 μM for silicate (SiO32–), and 0.35−0.84 μM for NH4+. Although the average values were higher at pH 7.4, there were no significant differences among the three pH treatments (Supplementary Table 1; p = 0.287 for PO43+, p = 0.964 for NO2–, p = 0.563 for SiO32–, and p = 0.665 for NH4+).
Data from a total of 8,064 measurements recorded in each tank by the CO2SYS ZB-LS 3.0 system indicated that each treatment pH was very stable during the entire experimental period (Supplementary Table 1 and Supplementary Figure 1). The standard error was ± 0.01 at pHs 7.7 and 8.1, and ± 0.02 at pH 7.4 (Supplementary Table 1). The TA was approximately 2,600 μM, and there were no significant differences among treatments (Supplementary Table 1; df = 2, F = 1.655, p = 0.244). Because of the relatively high TA (relative to 2,300 μM in the field), the aragonite saturation state (Ωarag) value at pH 7.7 reached 3.29, but rapidly reduced to 1.79 at pH 7.4. The concentration of carbonate (CO32–) declined under OA conditions (Supplementary Table 2; df = 2, F = 3,337, p < 0.001) but other carbonate parameters, including pCO2, CO2, and HCO3–, increased (p < 0.001).
Photochemical Efficiency of the Algal Symbiont
Statistical analysis showed no significant pH × time interactions for Fv/Fm (Table 1; df = 4, F = 0.726, p = 0.579), and no significant differences among the pH treatments (Table 1; df = 2, F = 1.403, p = 0.257) or times (Table 1; df = 2, F = 1.775, p = 0.182). The trend for ΔF/Fm′ was similar to that for Fv/Fm, but the values of ΔF/Fm′ declined significantly from 0.58 ± 0.01 at pH 8.1 to 0.49 ± 0.02 at pH 7.4 after 28-day stress (Figure 1, Table 1, and Supplementary Table 3; df = 2, F = 3.351, p = 0.043).
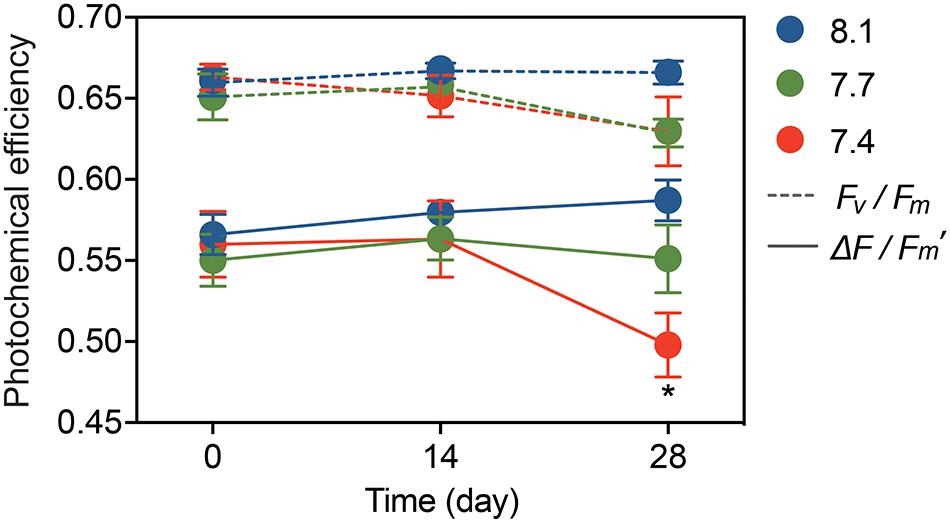
Figure 1. Photochemical efficiency of G. fascicularis over the course of the experiment: the maximum quantum yield (Fv/Fm) and effective quantum yield (ΔF/Fm′) of PSII. Values are expressed as mean ± SE from six determinations (n = 6). The asterisk (*) represents significant difference in the ΔF/Fm′ between pH 7.4 and other pH treatments (p < 0.05).
PFC and DDN Assimilation Rates
Galaxea fascicularis exhibited detectable gross PFC and DDN assimilation under control and OA conditions (Figure 2). At day 0, the assimilation of PFC and DDN were similar among all treatments (6.55–6.80 nmol Ctracer μmol Ctissue–1 d–1 for PFC assimilation, and 0.16–0.17 nmol Ntracer μmol Ntissue–1 d–1 for DDN assimilation; Supplementary Table 3 and Figure 2). The OA treatments did not affect PFC assimilation rates (Figure 2A and Table 2; F = 0.884, p = 0.461), but the DDN assimilation rates decreased at pH 7.4 (0.03 ± 0.02 nmol Ntracer μmol Ntissue–1 d–1) compared with those at pH 8.1 after 28-day stress (Figure 2B and Table 2; F = 7.454, p = 0.024). For natural isotope ratio, no significant effect was found for δ13C values (Figure 2C and Table 2; F = 2.053, p = 0.223), but the δ15N values decreased significantly at pH 7.4 compared with those at pH 8.1 after 28-day stress (Figure 2C and Table 2; F = 7.313, p = 0.033).
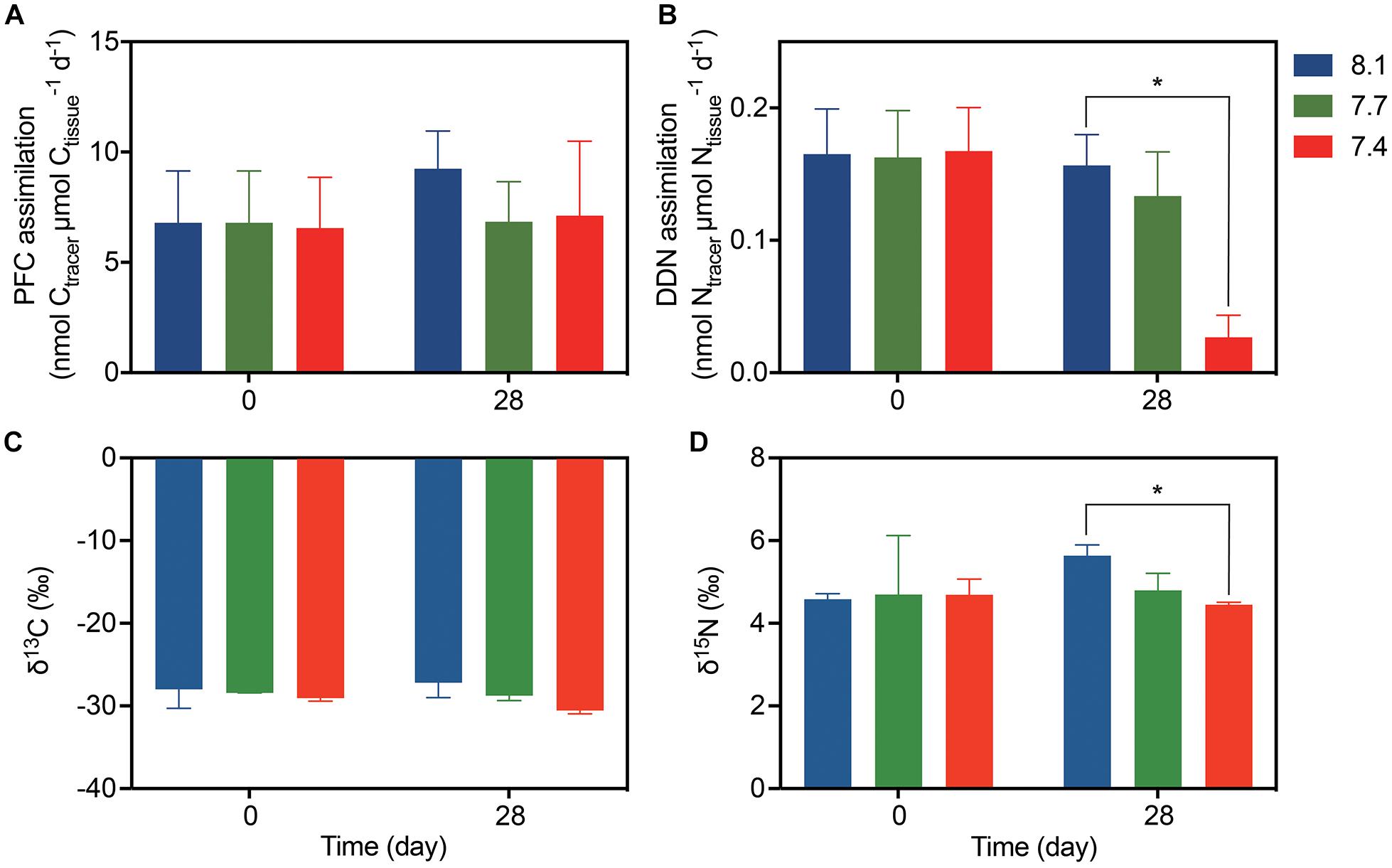
Figure 2. The assimilation rates and isotope values of G. fascicularis (mean ± SE) over the course of the experiment. (A) The PFC and (B) DDN assimilation rates at the start and end of the different pH treatments. (C) Carbon and (D) nitrogen stable isotope values for the coral holobiont at the start and end of the different pH treatments. Values are expressed as mean ± SE of three determinations (n = 3). The asterisk (*) represent significant difference between pH 7.4 and pH 8.1 (p < 0.05).
Calcification Rate
There were no significant pH × time interactions for coral calcification (Table 1; df = 2, F = 0.426, p = 0.656), and no significant differences among times (Table 2; df = 2, F = 2.586, p = 0.115), but significant differences among pH treatments were found (Table 1; df = 2, F = 5.968, p = 0.005). At pH 8.1, G. fascicularis maintained stable calcification rates, averaging 0.22 ± 0.05% week–1 and 0.18 ± 0.05% week–1 during the first and the second 14 days of growth, respectively (Supplementary Table 3 and Figure 3A). The calcification rates at pH 8.1 were significantly higher than those at pH 7.4 during the first (−0.01 ± 0.03% week–1) and the second 14 days (−0.14 ± 0.13% week–1), and significant carbonate dissolution was found at pH 7.4 (Figure 3A). The coral calcification rate decreased at pH 7.7, but was not significantly different from that at pH 8.1 (Figure 3A). Generalized linear models showed a significant positive relationship between the calcification rate and Ωarag over the experimental period (Figure 3B).
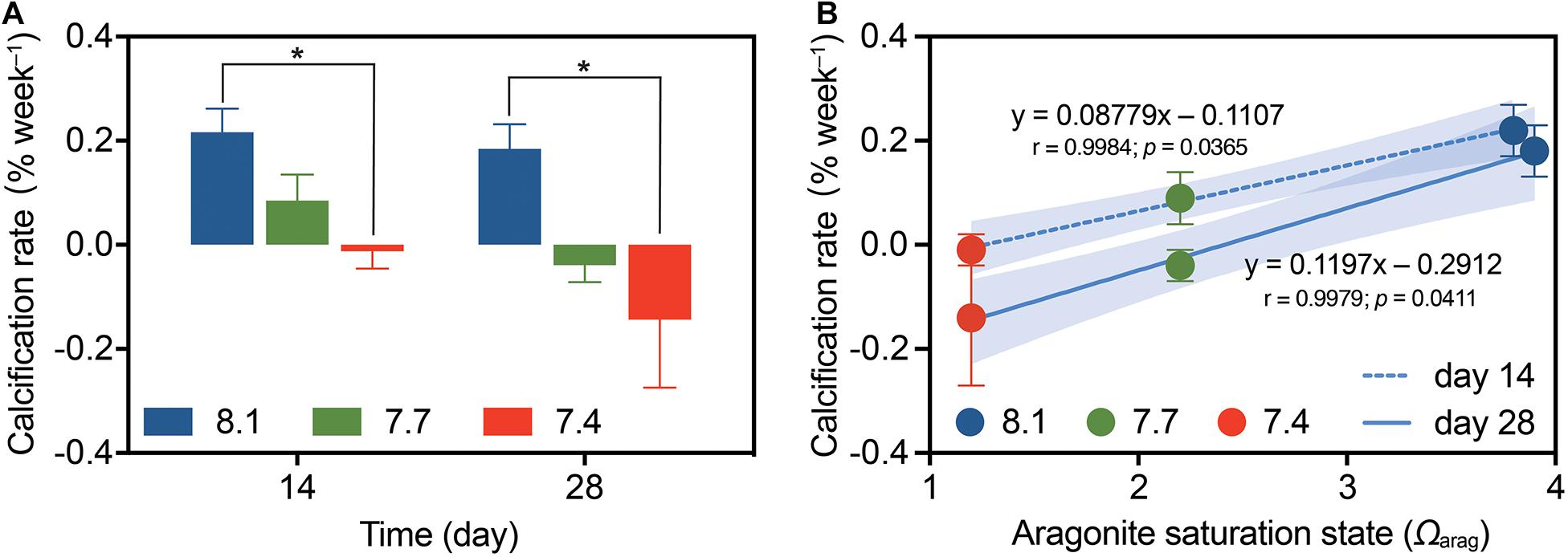
Figure 3. The calcification rates of G. fascicularis (mean ± SE) over the course of the experiment. (A) Values are expressed as mean ± SE from eight determinations (n = 8); The asterisk (*) represent significant difference between pH 7.4 and pH 8.1 (p < 0.05). (B) Relationship between coral calcification rate and the aragonite saturation state (Ωarag) based on the measurements after 14 days (dotted) or 28 days (solid) of different pH treatments. The p-values associated with each generalized linear model are shown. Shades surrounding the trend lines indicate 95% confidence level.
Discussion
Our measurements revealed that the effective quantum yield of the algal symbiont in G. fascicularis decreased significantly at pH 7.4 after 28 days of OA stress, but the algal maximum quantum yield and the coral assimilation of PFC did not appear to be affected by OA (Figures 1, 2A). Conflicting results of OA impact on algal photosynthesis have been reported, ranging from decreased photochemical efficiency in Acropora millepora at pH 7.6−7.7 and pH 7.8−7.9 (Kaniewska et al., 2012) and negative effects of long-term OA on coral productivity (Anthony et al., 2008), unaffected photosynthesis in A. digitifera at pH 7.56 (Takahashi and Kurihara, 2013), to enhanced net photosynthesis in Porites spp., A. millepora, and Pocillopora damicornis around volcanic CO2 seeps (pH 7.8) (Strahl et al., 2015). For many plants, acidification has a “fertilizing effect” on photosynthesis, as high pCO2 alleviates C limitation in the Calvin cycle, and facilitates greater rates of photosynthesis compared with those under ambient conditions (Vogel et al., 2015). The absence of the “fertilizing effect” of OA on photosynthesis in G. fascicularis suggests that this phenomena might be species-specific and maximum carbon fixation in the coral may have already attained under normal ambient pH condition. This could also be due to the fact that Symbiodiniaceae are located within the gastroderm of the coral host (i.e., not in direct contact with seawater) and thereby cannot efficiently sequester CO2 in acidified seawater. As a result, host respiration probably provides some CO2 that is easier to accesses than the external dissolved inorganic carbon to Symbiodiniaceae for photosynthesis (Furla et al., 2000). Moreover, the virtually unaffected assimilation of PFC in corals could be tied to the stable Symbiodiniaceae community. Symbiodiniaceae have been shown to be able to thrive in low pH and a stable Symbiodiniaceae community under OA may be expected to provide PFC to the coral holobiont (Noonan et al., 2013). This was further confirmed by the results of δ13C values showing no difference among different pH treatments (Figure 2C). The natural C isotope abundance has been used to understand the balance of autotrophy and heterotrophy in hermatypic corals (Wall et al., 2020), with the δ13C values positively relating to the photosynthate or autotrophic input (Tremblay et al., 2015; Allgeier et al., 2020). Therefore, the similar δ13C values among different pH treatments suggest stable PFC assimilation.
Microbes involved in N cycling may be fundamental to coral resilience to climate change (Rädecker et al., 2014, 2015). Rapid and significant decrease in DDN assimilation rates under OA (pH 7.71 with pCO2 1,080 μatm) has been reported in Seriatopora hystrix based on the acetylene reduction assay (Rädecker et al., 2014). In this study, the rates of DDN assimilation were differentially affected depending on the severity of the OA stress, with a non-significant decrease at pH 7.7 and a significant sharp decline at pH 7.4 (Figure 2B). The unaffected DDN assimilation at pH 7.7 may be attributed to the homeostasis of microbial, particularly diazotrophic community, whereas the marked decrease of DDN assimilation at pH 7.4 may be related to the disordered symbiosis with diazotrophs. Evidences that corals maintain high bacterial compositional stability under mild OA conditions (pH 7.9) have been reported in A. millepora and S. hystrix (Webster et al., 2016; Glasl et al., 2019). In contrast, a break in the coral–bacterial symbiosis and marked changes in bacterial abundance and diversity were found under severe OA (Morrow et al., 2015; Zaneveld et al., 2017). The abundance of cyanobacterial symbionts and Endozoicomonas sp., which were proposed to contribute to N cycling, was significantly reduced in the coral microbiome at a CO2 seep (Morrow et al., 2015). These findings imply that OA may disrupt the community structure of microbes involved in N cycling, which poses a threat to coral growth and calcification. The significant decrease in coral calcification rates under OA in the present study (Figure 3A) may also be attributed to the disordered processes associated with calcifying bacteria, such as the recently discovered photosynthesis-induced, bacterial—algal calcification (Frommlet et al., 2015, 2018), or changes in the community structure of some symbiotic cyanobacteria that promote calcium carbonate formation (Durak et al., 2019; Nitschke et al., 2020).
The δ15N values is positively correlated with the availability of N nutrient or heterotrophic input in coral holobiont (Donovan et al., 2020), therefore, the declined δ15N values at lowed pH in the present study (Figure 2D) suggest reduced N utilization and heterotrophy in G. fascicularis under OA conditions. In N-replete corals, acquisition and translocation of PFC were significantly higher and thus resulted in enhanced host calcification (Langdon and Atkinson, 2005; Béraud et al., 2013). Conversely, N-deficient corals showed reduced metabolism and nutrient limitation, leading to reduced calcification rates and decreased symbiont growth and density (Wiedenmann et al., 2013; Ezzat et al., 2015). The combined reduction of DDN assimilation rates and δ15N natural isotope abundance under OA in G. fascicularis (Figure 2) potentially may have compromised the coral calcification, although interpreting this in symbiotic corals is difficult because calcification is affected by various processes (Karcher et al., 2020).
Corals have the capacity to maintain elevated pH levels in calcifying fluids despite decreased seawater pH (Mcculloch et al., 2012). However, the upregulation of calcifying fluid pH is believed to be an energy-requiring process that involves removal of protons from the calcifying fluid by Ca2+ ATPases (Tambutté et al., 2011). In this study, the coral calcification rates decreased linearly with prolonged acidification and were negatively correlated with the corresponding Ωarag of the seawater. Hence, a lowering of the Ωarag makes the calcification process more energy consuming (Hohn and Merico, 2012). Furthermore, the N cycle is driven by complex and unique microbial transformations that are energetically expensive. For example, N2 fixation requires at least 16 ATPs to reduce N2 to NH3 (Benavides et al., 2017). It has been found in corals that N2 fixation was inhibited when photosynthesis was blocked, but could be recovered if glucose was added (Shashar et al., 1994), suggesting that N2 fixation strongly depends on photosynthesis to meet its energetic demands (Garcia et al., 2013). As calcification and N2 fixation are both energy-intensive processes, they probably compete for energy within the coral holobiont. Because PFC assimilation was not increased under OA condition, the increased energy demand to cope with OA stress must create an energy deficit and result in decreased rates of DDN assimilation and calcification.
Based on our measurements and published modeling data (Benavides et al., 2017), we propose a conceptual model of C and N fluxes in the coral holobiont under non-OA vs. OA conditions (Figure 4). Under non-OA control condition, fixed inorganic nitrogen, mostly in the form of NH4+, is primarily available to the Symbiodiniaceae, whereas the ingestion and digestion of diazotrophic cells provides organic nitrogen (e.g., in the form of amino acids) to the coral host. The release of organic carbon and energetic substrates from Symbiodiniaceae thus enhances diazotrophic growth and subsequent DDN assimilation, actively promoting coral calcification. On the contrary, OA triggers decreases in the overall assimilation of DDN, leading to insufficient organic nutrient supply to the growth and metabolism of the coral host, further exacerbating the coral calcification. To cope with this limited nutrient availability and imbalance between organic and inorganic nutrients, the coral appears to have evolved a trade-off mechanism to conserve and recycle the remaining fixed inorganic nitrogen to temporarily satisfy the demand of Symbiodiniaceae for barely unaffected assimilation of PFC and energy supply to the holobiont. The contrasting impacts of OA on PFC and DDN assimilation strongly suggests the flexibility of corals to sustain a largely undamaged photosystem and coral–algal symbiosis at the expense of N2 fixation machinery and/or change in the coral–bacterial symbiosis. While this strategic trade-off might work temporarily, it should be noted that long-term loss of bioavailable N would limit Symbiodiniaceae growth and photosynthesis, ultimately suppressing coral resilience to OA (Anthony et al., 2008). Taken together, the findings and proof-of-concept model presented in our study highlight the importance of N cycling and nutrient dynamics for calcifying organisms such as corals to adapt and acclimatize to future acidifying oceans (Thomsen et al., 2013; Leung et al., 2019). In this light, we emphasize the need for research focusing on other essential elements, such as N, which have important roles in addition to C in shaping marine symbioses.
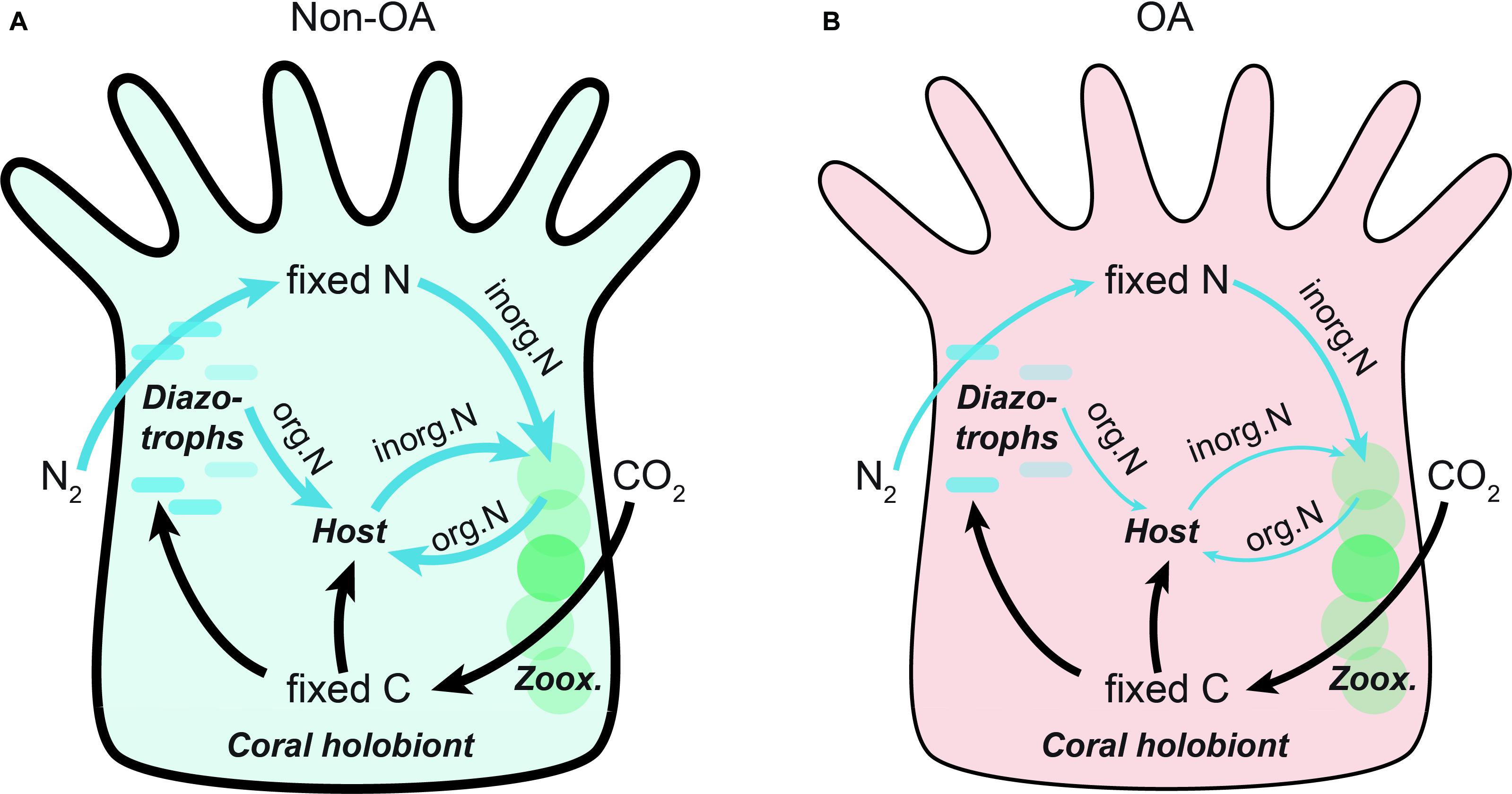
Figure 4. Schematic diagram illustrating the effects of OA on translocation and flux of fixed carbon and nitrogen in the coral holobiont. Shown are conceptual models under (A) Non-OA and (B) OA conditions. The diazotrophs provide fixed inorganic nitrogen to the Symbiodiniaceae, whereas direct ingestion and digestion of diazotrophic cells is a primary pathway of organic nitrogen to the coral host. The fluxes of carbon (black) and nitrogen (blue) are represented as arrows, the width of which corresponds to the levels of the flux. The thickness of coral epidermis corresponds to the resulting coral calcification rates at different conditions. Zoox., zooxanthellae; inorg.N, inorganic nitrogen; org.N, organic nitrogen.
Data Availability Statement
The original contributions presented in the study are included in the article/Supplementary Material, further inquiries can be directed to the corresponding author/s.
Ethics Statement
Corals were collected under permit number 2014003 for the Domestication and Breeding of Aquatic Wild Animals, issued by Department of Ocean and Fisheries of Hainan Province of the People’s Republic of China.
Author Contributions
XZ, TS, and LY conceived and designed the study. CW performed the experiments, with the help from HS for isotopic measurements, XD for water quality assessment, and GN for statistical analysis. XZ, CW, and TS interpreted the data and wrote the manuscript. All authors read and approved the final version of the manuscript.
Funding
This work was supported by the National Key Research and Development Program of China (Grant Nos. 2016YFA0601203 and 2020YFA0607602), the National Natural Science Foundation of China (Grant Nos. 41876119 and 41976127), the Scientific Research Foundation from the Fujian Provincial Station for Field Observation and Research of Island and Coastal Zone, administered by the Third Institute of Oceanography, Ministry of Natural Resources of China (Grant No. TIO 2019017).
Conflict of Interest
The authors declare that the research was conducted in the absence of any commercial or financial relationships that could be construed as a potential conflict of interest.
Acknowledgments
We are grateful to Yuanchao Li and Shiquan Chen from Hainan Academy of Ocean and Fisheries Sciences for assistance in coral sampling, and Yan Li and Wencong Huang for help with aquaria maintenance.
Supplementary Material
The Supplementary Material for this article can be found online at: https://www.frontiersin.org/articles/10.3389/fmars.2021.644965/full#supplementary-material
References
Allgeier, J. E., Andskog, M. A., Hensel, E., Appaldo, R., Layman, C., and Kemp, D. W. (2020). Rewiring coral: anthropogenic nutrients shift diverse coral-symbiont nutrient and carbon interactions toward symbiotic algal dominance. Glob. Chang. Biol. 26, 5588–5601. doi: 10.1111/gcb.15230
Anthony, K. R. N., Kline, D. I., Diazpulido, G., Dove, S., and Hoeghguldberg, O. (2008). Ocean acidification causes bleaching and productivity loss in coral reef builders. Proc. Natl. Acad. Sci. U.S.A 105, 17442–17446. doi: 10.1073/pnas.0804478105
Barkley, H. C., Cohen, A. L., McCorkle, D. C., and Golbuu, Y. (2017). Mechanisms and thresholds for pH tolerance in Palau corals. J. Exp. Mar. Biol. Ecol. 489, 7–14. doi: 10.1016/j.jembe.2017.01.003
Bednarz, V. N., Grover, R., Maguer, J. F., Fine, M., and Ferrier-Pagès, C. (2017). The assimilation of diazotroph-derived nitrogen by Scleractinian corals depends on their metabolic status. MBio 8:e02058–16. doi: 10.1128/mBio.02058-16
Bednarz, V. N., van de Water, J. A., Rabouille, S., Maguer, J. F., Grover, R., and Ferrier-Pagès, C. (2019). Diazotrophic community and associated dinitrogen fixation within the temperate coral Oculina patagonica. Environ. Microbiol. 21, 480–495. doi: 10.1111/1462-2920.14480
Benavides, M., Bednarz, V. N., and Ferrier-Pagès, C. (2017). Diazotrophs: overlooked key players within the coral symbiosis and tropical reef ecosystems? Front. Mar. Sci. 4:10. doi: 10.3389/fmars.2017.00010
Béraud, E., Gevaert, F., Rottier, C., and Ferrier-Pagès, C. (2013). The response of the scleractinian coral Turbinaria reniformis to thermal stress depends on the nitrogen status of the coral holobiont. J. Exp. Biol. 216, 2665–2674. doi: 10.1242/jeb.085183
Byrne, M., Lamare, M., Winter, D., Dworjanyn, S. A., and Uthicke, S. (2013). The stunting effect of a high CO2 ocean on calcification and development in sea urchin larvae, a synthesis from the tropics to the poles. Philos. Trans. R Soc. Lond. B Biol. Sci. 368:20120439. doi: 10.1098/rstb.2012.0439
Capone, D. G. (1993). “Determination of nitrogenase activity in aquatic samples using the acetylene reduction procedure,” in Handbook of Methods in Aquatic Microbial Ecology, eds P. F. Kemp, J. J. Cole, B. F. Sherr, and E. B. Sherr (London: CRC Press), 621–631. doi: 10.1201/9780203752746-74
Cardini, U., Bednarz, V. N., Foster, R. A., and Wild, C. (2014). Benthic N2 fixation in coral reefs and the potential effects of human-induced environmental change. Ecol. Evol. 4, 1706–1727. doi: 10.1002/ece3.1050
Comeau, S., Carpenter, R. C., and Edmunds, P. J. (2017). Effects of pCO2 on photosynthesis and respiration of tropical scleractinian corals and calcified algae. ICES J. Mar. Sci. 74, 1092–1102. doi: 10.1093/icesjms/fsv267
Coronado, I., Fine, M., Bosellini, F. R., and Stolarski, J. (2019). Impact of ocean acidification on crystallographic vital effect of the coral skeleton. Nat. commun. 10, 1–9. doi: 10.1038/s41467-019-10833-6
Davies, P. S. (1989). Short-term growth measurements of corals using an accurate buoyant weighing technique. Mar. Biol. 101, 389–395. doi: 10.1007/BF00428135
Dickson, A. G., Sabine, C. L., and Christian, J. R. (2007). Guide to Best Practices for Ocean CO2 Measurements. Sidney, BC: North Pacific Marine Science Organization.
Donovan, M. K., Adam, T. C., Shantz, A. A., Speare, K. E., Munsterman, K. S., Rice, M. M., et al. (2020). Nitrogen pollution interacts with heat stress to increase coral bleaching across the seascape. Proc. Natl. Acad. Sci. U.S.A. 117, 5351–5357. doi: 10.1073/pnas.1915395117
Dove, S. G., Kline, D. I., Pantos, O., Angly, F. E., Tyson, G. W., and Hoeghguldberg, O. (2013). Future reef decalcification under a business-as-usual CO2 emission scenario. Proc. Natl. Acad. Sci. U.S.A. 110, 15342–15347. doi: 10.1073/pnas.1302701110
Durak, G. M., Laumann, M., Wolf, S. L. P., Pawar, A., Gebauer, D., and Böttcher, T. (2019). Pseudo-biomineralization: complex mineral structures shaped by microbes. ACS Biomater. Sci. Eng. 5, 5088–5096. doi: 10.1021/acsbiomaterials.9b00387
Enochs, I. C., Manzello, D. P., Kolodziej, G., Noonan, S. H., Valentino, L., and Fabricius, K. E. (2016). Enhanced macroboring and depressed calcification drive net dissolution at high-CO2 coral reefs. Proc. R. Soc. B Biol. Sci. 283:20161742. doi: 10.1098/rspb.2016.1742
Eyre, B. D., Cyronak, T., Drupp, P., De Carlo, E. H., Sachs, J. P., and Andersson, A. J. (2018). Coral reefs will transition to net dissolving before end of century. Science 359, 908–911. doi: 10.1126/science.aao1118
Ezzat, L., Maguer, J. F., Grover, R., and Ferrier-Pages, C. (2015). New insights into carbon acquisition and exchanges within the coral-dinoflagellate symbiosis under NH4+ and NO3– supply. Proc. Biol. Sci. 282, 20150610. doi: 10.1098/rspb.2015.0610
Fine, M., and Loya, Y. (2002). Endolithic algae: an alternative source of photoassimilates during coral bleaching. Proc. Biol. Sci. 269, 1205–1210. doi: 10.1098/rspb.2002.1983
Fiore, C. L., Jarett, J. K., Olson, N. D., and Lesser, M. P. (2010). Nitrogen fixation and nitrogen transformations in marine symbioses. Trends Microbiol. 18, 455–463. doi: 10.1016/j.tim.2010.07.001
Frommlet, J. C., Sousa, M. L., Alves, A., Vieira, S. I., Suggett, D. J., and Serôdio, J. (2015). Coral symbiotic algae calcify ex hospite in partnership with bacteria. Proc. Natl. Acad. Sci. U.S.A. 112, 6158–6163. doi: 10.1073/pnas.1420991112
Frommlet, J. C., Wangpraseurt, D., Sousa, M. L., Guimaraes, B., Medeiros da Silva, M., Kuhl, M., et al. (2018). Symbiodinium-induced formation of microbialites: mechanistic insights from in vitro experiments and the prospect of its occurrence in nature. Front. Microbiol. 9:998. doi: 10.3389/fmicb.2018.00998
Furla, P., Galgani, I., Durand, I., and Allemand, D. (2000). Sources and mechanisms of inorganic carbon transport for coral calcification and photosynthesis. J. Exp. Biol. 203, 3445–3457.
Garcia, N. S., Fu, F.-X., and Hutchins, D. A. (2013). Colimitation of the unicellular photosynthetic diazotroph Crocosphaera watsonii by phosphorus, light, and carbon dioxide. Limnol. Oceanogr. 58, 1501–1512. doi: 10.4319/lo.2013.58.4.1501
Glasl, B., Smith, C. E., Bourne, D. G., and Webster, N. S. (2019). Disentangling the effect of host-genotype and environment on the microbiome of the coral Acropora tenuis. PeerJ 7:e6377. doi: 10.7717/peerj.6377
Großkopf, T., Mohr, W., Baustian, T., Schunck, H., Gill, D., Kuypers, M. M., et al. (2012). Doubling of marine dinitrogen-fixation rates based on direct measurements. Nature 488, 361–364. doi: 10.1038/nature11338
Hama, T., Miyazaki, T., Ogawa, Y., Iwakuma, T., Takahashi, M., Otsuki, A., et al. (1983). Measurement of photosynthetic production of a marine phytoplankton population using a stable 13C isotope. Mar. Biol. 73, 31–36. doi: 10.1007/BF00396282
Hoadley, K. D., Rollison, D., Pettay, D. T., and Warner, M. E. (2015). Differential carbon utilization and asexual reproduction under elevated pCO2 conditions in the model anemone, Exaiptasia pallida, hosting different symbionts. Limnol. Oceanogr. 60, 2108–2120. doi: 10.1002/lno.10160
Hoegh-Guldberg, O., Mumby, P. J., Hooten, A. J., Steneck, R. S., Greenfield, P., Gomez, E., et al. (2007). Coral reefs under rapid climate change and ocean acidification. Science 318, 1737–1742. doi: 10.1126/science.1152509
Hohn, S., and Merico, A. (2012). Effects of seawater pCO2 changes on the calcifying fluid of scleractinian corals. Biogeosci. Discuss. 9, 2655–2689. doi: 10.5194/bgd-9-2655-2012
Kaniewska, P., Campbell, P. R., Kline, D. I., Rodriguezlanetty, M., Miller, D. J., Dove, S., et al. (2012). Major cellular and physiological impacts of ocean acidification on a reef building coral. PLos One 7:e34659. doi: 10.1371/journal.pone.0034659
Kaniewska, P., Chan, C. K. K., Kline, D., Ling, E. Y. S., Rosic, N., Edwards, D., et al. (2015). Transcriptomic changes in coral holobionts provide insights into physiological challenges of future climate and ocean change. PLos One 10:e0139223. doi: 10.1371/journal.pone.0139223
Karcher, D. B., Roth, F., Carvalho, S., El-Khaled, Y. C., Tilstra, A., Kurten, B., et al. (2020). Nitrogen eutrophication particularly promotes turf algae in coral reefs of the central Red Sea. PeerJ 8:e8737. doi: 10.7717/peerj.8737
Kurman, M. D., Gomez, C. E., Georgian, S. E., Lunden, J. J., and Cordes, E. E. (2017). Intra-specific variation reveals potential for adaptation to ocean acidification in a cold-water coral from the Gulf of Mexico. Front. Mar. Sci. 4:111. doi: 10.3389/fmars.2017.00111
Langdon, C., and Atkinson, M. J. (2005). Effect of elevated pCO2 on photosynthesis and calcification of corals and interactions with seasonal change in temperature/irradiance and nutrient enrichment. J. Geophys. Res. Oceans 110:C09S07. doi: 10.1029/2004JC002576
Lema, K. A., Clode, P. L., Kilburn, M. R., Thornton, R., Willis, B. L., and Bourne, D. G. (2016). Imaging the uptake of nitrogen-fixing bacteria into larvae of the coral Acropora millepora. ISME J. 10:1804. doi: 10.1038/ismej.2015.229
Lema, K. A., Willis, B. L., and Bourne, D. G. (2014). Amplicon pyrosequencing reveals spatial and temporal consistency in diazotroph assemblages of the Acropora millepora microbiome. Environ. Microbiol. 16, 3345–3359. doi: 10.1111/1462-2920.12366
Lesser, M. P., Falcón, L. I., Rodríguez-Román, A., Enríquez, S., Hoegh-Guldberg, O., and Iglesias-Prieto, R. (2007). Nitrogen fixation by symbiotic cyanobacteria provides a source of nitrogen for the scleractinian coral Montastraea cavernosa. Mar. Ecol. Prog. Ser. 346, 143–152. doi: 10.3354/meps07008
Lesser, M. P., Mazel, C. H., Gorbunov, M. Y., and Falkowski, P. G. (2004). Discovery of symbiotic nitrogen-fixing cyanobacteria in corals. Science 305, 997–1000. doi: 10.1126/science.1099128
Lesser, M. P., Morrow, K. M., and Pankey, M. S. (2019). N2 fixation, and the relative contribution of fixed N, in corals from Curaçao and Hawaii. Coral Reefs 38, 1145–1158. doi: 10.1007/s00338-019-01863-z
Leung, J. Y. S., Chen, Y., Nagelkerken, I., Zhang, S., Xie, Z., and Connell, S. D. (2020). Calcifiers can adjust shell building at the nanoscale to resist ocean acidification. Small 16:e2003186. doi: 10.1002/smll.202003186
Leung, J. Y. S., Connell, S. D., Nagelkerken, I., and Russell, B. D. (2017). Impacts of near-future ocean acidification and warming on the shell mechanical and geochemical properties of gastropods from intertidal to subtidal zones. Environ. Sci. Technol. 51, 12097–12103. doi: 10.1021/acs.est.7b02359
Leung, J. Y. S., Doubleday, Z. A., Nagelkerken, I., Chen, Y., Xie, Z., and Connell, S. D. (2019). How calorie-rich food could help marine calcifiers in a CO2-rich future. Proc. Biol. Sci. 286:20190757. doi: 10.1098/rspb.2019.0757
Lewis, E., Wallace, D., and Allison, L. J. (1998). Program Developed for CO2 System Calculations. Upton, NY: Brookhaven National Lab.
Li, Y., Zheng, X., Yang, X., Ou, D., Lin, R., and Liu, X. (2017). Effects of live rock on removal of dissolved inorganic nitrogen in coral aquaria. Acta Oceanologica Sin. 36, 87–94. doi: 10.1007/s13131-017-1092-1
Lin, Z., Chen, M., Dong, X., Zheng, X., Huang, H., Xu, X., et al. (2017). Transcriptome profiling of Galaxea fascicularis and its endosymbiont Symbiodinium reveals chronic eutrophication tolerance pathways and metabolic mutualism between partners. Sci. Rep. 7:42100. doi: 10.1038/srep42100
Mcculloch, M., Trotter, J., Montagna, P., Falter, J., Dunbar, R., Freiwald, A., et al. (2012). Resilience of cold-water scleractinian corals to ocean acidification: boron isotopic systematics of pH and saturation state up-regulation. Geochim. Cosmochim. Acta 87, 21–34. doi: 10.1016/j.gca.2012.03.027
Mollica, N. R., Guo, W., Cohen, A. L., Huang, K.-F., Foster, G. L., Donald, H. K., et al. (2018). Ocean acidification affects coral growth by reducing skeletal density. Proc. Natl. Acad. Sci. U.S.A. 115, 1754–1759. doi: 10.1073/pnas.1712806115
Montoya, J. P., Voss, M., Kahler, P., and Capone, D. G. (1996). A simple, high-precision, high-sensitivity tracer assay for N2 fixation. Appl. Environ. Microbiol. 62, 986–993. doi: 10.3389/fmars.2017.00010
Morrow, K. M., Bourne, D. G., Humphrey, C., Botte, E. S., Laffy, P., Zaneveld, J., et al. (2015). Natural volcanic CO2 seeps reveal future trajectories for host-microbial associations in corals and sponges. ISME J. 9, 894–908. doi: 10.1038/ismej.2014.188
Moya, A., Huisman, L., Ball, E., Hayward, D., Grasso, L., Chua, C., et al. (2012). Whole transcriptome analysis of the coral Acropora millepora reveals complex responses to CO2-driven acidification during the initiation of calcification. Mol. Ecol. 21, 2440–2454. doi: 10.1111/j.1365-294X.2012.05554.x
Muscatine, L., and Porter, J. W. (1977). Reef corals: mutualistic symbioses adapted to nutrient-poor environments. Bioscience 27, 454–460. doi: 10.2307/1297526
Nitschke, M. R., Fidalgo, C., Simoes, J., Brandao, C., Alves, A., Serodio, J., et al. (2020). Symbiolite formation: a powerful in vitro model to untangle the role of bacterial communities in the photosynthesis-induced formation of microbialites. ISME J. 14, 1533–1546. doi: 10.1038/s41396-020-0629-z
Noonan, S. H. C., Fabricius, K. E., and Humphrey, C. (2013). Symbiodinium community composition in Scleractinian corals is not affected by life-long exposure to elevated carbon dioxide. PLos One 8:e63985. doi: 10.1371/journal.pone.0063985
Olson, N. D., and Lesser, M. P. (2013). Diazotrophic diversity in the Caribbean coral, Montastraea cavernosa. Arch. Microbiol. 195, 853–859. doi: 10.1007/s00203-013-0937-z
Peixoto, R. S., Rosado, P. M., Leite, D. C. D. A., Rosado, A. S., and Bourne, D. G. (2017). Beneficial microorganisms for corals (BMC): proposed mechanisms for coral health and resilience. Front. Microbiol. 8:341. doi: 10.3389/fmicb.2017.00341
Rädecker, N., Meyer, F. W., Bednarz, V. N., Cardini, U., and Wild, C. (2014). Ocean acidification rapidly reduces dinitrogen fixation associated with the hermatypic coral Seriatopora hystrix. Mar. Ecol. Prog. Ser. 511, 297–302. doi: 10.3354/meps10912
Rädecker, N., Pogoreutz, C., Voolstra, C. R., Wiedenmann, J., and Wild, C. (2015). Nitrogen cycling in corals: the key to understanding holobiont functioning? Trends Microbiol. 23, 490–497. doi: 10.1016/j.tim.2015.03.008
Rowan, R. (1998). Diversity and ecology of zooxanthellae on coral reefs. J. Phycol. 34, 407–417. doi: 10.1046/j.1529-8817.1998.340407.x
Shashar, N., Cohen, Y., Loya, Y., and Sar, N. (1994). Nitrogen fixation (acetylene reduction) in stony corals: evidence for coral-bacteria interactions. Mar. Ecol. Prog. Ser. 111, 259–264. doi: 10.3354/meps111259
Shiozaki, T., Nagata, T., Ijichi, M., and Furuya, K. (2015). Nitrogen fixation and the diazotroph community in the temperate coastal region of the northwestern North Pacific. Biogeosciences 12, 4751–4764. doi: 10.5194/bg-12-4751-2015
Silveira, C. B., Cavalcanti, G. S., Walter, J. M., Silva-Lima, A. W., Dinsdale, E. A., Bourne, D. G., et al. (2017). Microbial processes driving coral reef organic carbon flow. FEMS Microbiol. Rev. 41, 575–595. doi: 10.1093/femsre/fux018
Strahl, J., Stolz, I., Uthicke, S., Vogel, N., Noonan, S., and Fabricius, K. (2015). Physiological and ecological performance differs in four coral taxa at a volcanic carbon dioxide seep. Comp. Biochem. Physiol. A Mol. Integr. Physiol. 184, 179–186. doi: 10.1016/j.cbpa.2015.02.018
Takahashi, A., and Kurihara, H. (2013). Ocean acidification does not affect the physiology of the tropical coral Acropora digitifera during a 5-week experiment. Coral Reefs 32, 305–314. doi: 10.1007/s00338-012-0979-8
Tambutté, S., Holcomb, M., Ferrier-Pagès, C., Reynaud, S., Tambutté, É, Zoccola, D., et al. (2011). Coral biomineralization: from the gene to the environment. J. Exp. Mar. Biol. Ecol. 408, 58–78. doi: 10.1016/j.jembe.2011.07.026
Thomsen, J., Casties, I., Pansch, C., Kortzinger, A., and Melzner, F. (2013). Food availability outweighs ocean acidification effects in juvenile Mytilus edulis: laboratory and field experiments. Glob. Chang. Biol. 19, 1017–1027. doi: 10.1111/gcb.12109
Tremblay, P., Grover, R., Maguer, J. F., Legendre, L., and Ferrier-Pagès, C. (2012). Autotrophic carbon budget in coral tissue: a new 13C-based model of photosynthate translocation. J. Exp. Biol. 215, 1384–1393. doi: 10.1242/jeb.065201
Tremblay, P., Maguer, J. F., Grover, R., and Ferrier-Pagès, C. (2015). Trophic dynamics of scleractinian corals: stable isotope evidence. J. Exp. Biol. 218, 1223–1234. doi: 10.1242/jeb.115303
Van der Zande, R. M., Achlatis, M., Bender-Champ, D., Kubicek, A., Dove, S., and Hoegh-Guldberg, O. (2020). Paradise lost: end-of-century warming and acidification under business-as-usual emissions have severe consequences for symbiotic corals. Glob. Chang. Biol. 26, 2203–2219. doi: 10.1111/gcb.14998
Vogel, N., Fabricius, K. E., Strahl, J., Noonan, S. H. C., Wild, C., and Uthicke, S. (2015). Calcareous green alga Halimeda tolerates ocean acidification conditions at tropical carbon dioxide seeps. Limnol. Oceanogr. 60, 263–275. doi: 10.1002/lno.10021
Wall, C. B., Kaluhiokalani, M., Popp, B. N., Donahue, M. J., and Gates, R. D. (2020). Divergent symbiont communities determine the physiology and nutrition of a reef coral across a light-availability gradient. ISME J. 14, 945–958. doi: 10.1038/s41396-019-0570-1
Webster, N., Negri, A., Botté, E., Laffy, P., Flores, F., Noonan, S., et al. (2016). Host-associated coral reef microbes respond to the cumulative pressures of ocean warming and ocean acidification. Sci. Rep. 6:19324. doi: 10.1038/srep19324
White, A. E., Granger, J., Selden, C., Gradoville, M. R., Potts, L., Bourbonnais, A., et al. (2020). A critical review of the 15N2 tracer method to measure diazotrophic production in pelagic ecosystems. Limnol. Oceanogr. Methods 18, 129–147. doi: 10.1002/lom3.10353
Wiedenmann, J., D’Angelo, C., Smith, E. G., Hunt, A. N., Legiret, F., Postle, A. D., et al. (2013). Nutrient enrichment can increase the susceptibility of reef corals to bleaching. Nat. Clim. Chang. 3, 160–164. doi: 10.1038/NCLIMATE1661
Zaneveld, J. R., McMinds, R., and Thurber, R. V. (2017). Stress and stability: applying the Anna Karenina principle to animal microbiomes. Nat. Microbiol. 2:17121.
Zehr, J. P., and Kudela, R. M. (2011). Nitrogen cycle of the open ocean: from genes to ecosystems. Annu. Rev. Mar. Sci. 3, 197–225. doi: 10.1146/annurev-marine-120709-142819
Zheng, X., Li, Y., Chen, S., and Lin, R. (2018a). Effects of calcium ion concentration on calcification rates of six stony corals: a mesocosm study. Aquaculture 497, 246–252. doi: 10.1016/j.aquaculture.2018.07.041
Keywords: ocean acidification, coral calcification, carbon fixation, nitrogen fixation, symbiosis, photosynthesis
Citation: Zheng X, Wang C, Sheng H, Niu G, Dong X, Yuan L and Shi T (2021) Effects of Ocean Acidification on Carbon and Nitrogen Fixation in the Hermatypic Coral Galaxea fascicularis. Front. Mar. Sci. 8:644965. doi: 10.3389/fmars.2021.644965
Received: 22 December 2020; Accepted: 30 March 2021;
Published: 22 April 2021.
Edited by:
Jonathan Y. S. Leung, University of Adelaide, AustraliaReviewed by:
Zhi Zhou, Hainan University, ChinaXiangcheng Yuan, South China Sea Institute of Oceanology, Chinese Academy of Sciences, China
Copyright © 2021 Zheng, Wang, Sheng, Niu, Dong, Yuan and Shi. This is an open-access article distributed under the terms of the Creative Commons Attribution License (CC BY). The use, distribution or reproduction in other forums is permitted, provided the original author(s) and the copyright owner(s) are credited and that the original publication in this journal is cited, in accordance with accepted academic practice. No use, distribution or reproduction is permitted which does not comply with these terms.
*Correspondence: Lingling Yuan, yuanoo81@163.com; Tuo Shi, tuoshi@sdu.edu.cn
†These authors share first authorship