- 1California Academy of Sciences, San Francisco, CA, United States
- 2Caribbean Research and Management of Biodiversity Foundation, Willemstad, Curaçao
- 3Institut de Biologie Intégrative et des Systèmes, Université Laval, Québec, QC, Canada
- 4School of Biological Sciences, The University of Queensland, St Lucia, QLD, Australia
- 5Institute for Biodiversity and Ecosystem Dynamics, University of Amsterdam, Amsterdam, Netherlands
Coral reefs across the world are undergoing rapid deterioration, and understanding the ecological and evolutionary processes that govern these ecosystems is critical to our ability to protect them. Molecular ecological studies have been instrumental in advancing such understanding, and while initially focused primarily on broad-scale patterns, they have gradually uncovered the prevalence of local genetic structuring. Genome-wide sequencing approaches have provided new opportunities to understand both neutral and adaptive contributions to this largely unexplained diversity, but fine-scale assessments have been hampered by challenges associated with aquatic environments, in terms of (geo)referencing, seafloor characterization, and in situ phenotyping. Here, we discuss the potential of “reefscape genomics,” leveraging recent advances in underwater imaging to enable spatially explicit genomic studies on coral reefs. More specifically, we consider how (close-range) photogrammetry approaches enable (1) fine-scale spatial mapping of benthic target organisms, (2) repeatable characterization of the abiotic and biotic reefscape, and (3) simultaneous in situ mass-phenotyping. The spatially explicit consideration of genomic data –combined with detailed environmental and phenotypic characterization– opens up the opportunity for fine-scale landscape genomic approaches on coral reefs (and other marine ecosystems). Such approaches enable assessment of the spatio-temporal drivers and adaptive potential of the extensive genetic structuring and cryptic diversity encountered in benthic invertebrates, such as reef-building corals. Considering the threats that coral reefs are facing worldwide, we believe that reefscape genomics represents a promising advancement of our molecular ecological toolkit to help inform how we can most effectively conserve and restore coral reef ecosystems into the future.
Introduction
Coral reefs are one of the most biodiverse and economically important ecosystems. Yet, they are undergoing an unprecedented decline due to a wide range of anthropogenic stressors (e.g., increasing sea temperatures, ocean acidification, pollution, and overfishing) (Hoegh-Guldberg et al., 2007; McClenachan et al., 2017). Our ability to manage and conserve these vulnerable ecosystems is contingent on our understanding of the fundamental processes underpinning their resilience. Over the past decades, molecular ecology has played a major role in elucidating these processes for reef-building corals (order Scleractinia) (van Oppen and Gates, 2006), by uncovering patterns of dispersal and connectivity (Ayre and Hughes, 2000; van Oppen et al., 2008), contributions of sexual and asexual reproduction (Miller and Ayre, 2004; Foster et al., 2013; Dubé et al., 2017), the prevalence and nature of hybridization (Vollmer and Palumbi, 2002; Combosch et al., 2008), and the endosymbiotic microbial diversity which is critical to their survival (Baums et al., 2014; Boilard et al., 2020). Importantly, the advent of high-throughput genomic approaches (e.g., reduced representation and whole-genome sequencing) has facilitated increasingly sophisticated assessments for non-model organisms (Riginos et al., 2016; Matz, 2018), including the opportunity to study adaptive variation critical to the persistence of coral reefs (Bay and Palumbi, 2014; Dixon et al., 2015). While these genomic advances hold great promise to address knowledge gaps in ecology and evolution, their true potential is ultimately dependent on our ability to couple their outputs with environmental and/or phenotypic information at the relevant spatial scale (Andrew et al., 2013).
Landscape genetics has provided a powerful framework in terrestrial ecosystems to predict population genomic patterns from landscape attributes and processes (Manel et al., 2003; Balkenhol et al., 2016). By extension, landscape genomics is a more recent discipline that queries similar relationships but across both neutral and adaptive parts of the genome (Balkenhol et al., 2017; Li et al., 2017). As its marine counterpart, seascape genomics shares much of the aforesaid theoretical and analytical framework, but is challenged by physical variability of the oceanic environment and the unique life histories of marine organisms (e.g., high dispersal potential and large effective population sizes) (Riginos et al., 2016; Liggins et al., 2019). Recent studies have demonstrated the potential of seascape genomics in the study of reef-building corals, for example by identifying genes associated with thermal adaptation (Jin et al., 2016; Fuller et al., 2020; Selmoni et al., 2020a,b). Nevertheless, there are several major limitations associated with the application of seascape genomics to coral reef environments. Firstly, due to its reliance on remote sensing methods, the environmental characterization mostly focuses on the (upper) ocean surface (i.e., oceanographic features) rather than the benthic landscape (or “benthoscape”). In addition, the spatial resolution (or “grain”) of such remotely sensed methods generally only allows for limited characterization on a within-reef scale. Lastly, spatially explicit, individual-based sampling has been hampered (compared to terrestrial studies) due to the inability of using satellite-based geo-positioning, as radio signals do not propagate sufficiently underwater.
In this perspective, we discuss the potential for “reefscape genomics,” leveraging advances in underwater imaging to enable fine-scale landscape genomic studies on coral reefs. The term “reefscape” has been used loosely in the coral reef literature, mostly as an underwater equivalent to the term landscape (e.g., Arias-González et al., 2006; Urbina-Barreto et al., 2020). Inherently connected to seascape genomics, we define reefscape genomics as spatially explicit studies focused on a within-reef scale that use reefscape attributes and processes as statistical predictors of genomic variation. This follows a recent call to expand seascape characterization to specifically include the benthic component (Van Wynsberge et al., 2017), but we argue the additional value of doing so at a high spatial resolution. Such fine-scale characterization of the reefscape has recently been made possible due to advances in computer vision, and further facilitated by the increased accessibility of the underwater environment (e.g., through autonomous underwater vehicles, dive propulsion vehicles, and closed-circuit rebreathers). In particular, we believe that close-range photogrammetry has the potential to transform seascape genomics by enabling (1) fine-scale spatial mapping benthic components, (2) repeatable characterization of both abiotic and biotic features of the benthoscape, and (3) simultaneous mass-phenotyping of target organisms. We begin by explaining why a reefscape genomics approach is relevant in terms of major knowledge gaps (focusing mostly on reef-building corals), we then elaborate on the types of relevant (meta)data that can be acquired through photogrammetry, and we conclude by illustrating how such data can be integrated into genomic assessments to address the outlined knowledge gaps.
Why Reefscape Genomics?
The choice of spatial scale in molecular ecology is critical as it defines the ability to identify the processes underlying genetic variation (Hellberg, 2007). Given the biphasic life cycle of most marine organisms (i.e., pelagic larval and benthic adult phase), it has been traditionally assumed that neutral genetic patterns are governed by broad-scale larval dispersal processes (Kinlan et al., 2005; Liggins et al., 2013). However, studies have since demonstrated the prevalence of local genetic differentiation within both species with internal (brooders) and external (broadcasters) fertilization. For brooding species, such fine-scale population structure can be linked to strongly localized sperm and larval dispersal (Underwood et al., 2007; Ledoux et al., 2010; Warner et al., 2016), while such patterns for broadcasting species contradict with their broad dispersal capability and with observations of high gene flow over large distances (Ayre and Hughes, 2000; van Oppen et al., 2008; Cros et al., 2020). These non-intuitive population structures are likely the result of the complex interplay and spatio-temporal variability in species attributes, pelagic conditions, and benthic features (Liggins et al., 2019). The perceived chaos in reef-building corals is –at least in part– due to a mismatch in spatial resolution (Cros et al., 2020), a predominance of population-level sampling (Riginos and Liggins, 2013; Liggins et al., 2019), and an almost complete lack of temporal assessments (but see Williams et al., 2014; Underwood et al., 2018). Microsatellite-based studies with exhaustive local sampling have demonstrated the critical relevance of fine-scale, individual-based sampling by revealing the important contributions of clonality and inbreeding (Gorospe and Karl, 2013; Dubé et al., 2017), sperm dispersal and self-fertilization (Warner et al., 2016), co-dispersal of siblings, and self-recruitment (Cros et al., 2020; Dubé et al., 2020). Nonetheless, our understanding of reproduction and dispersal processes in benthic reef organisms is still in its infancy given that spatially explicit, individual-based attempts have been incredibly tedious, and have lacked the ability to characterize and integrate the fine-scale composition and configuration of the reefscape.
Patterns of adaptive variation in marine environments often occur at local scales, with selection contributing to spatial genetic structuring regardless of the extent of gene flow (Liggins et al., 2019). Habitat-specific sampling has demonstrated how local genetic structure in reef-building corals can reflect divergence across environmentally distinct but spatially adjacent reef habitats (Benzie et al., 1995; Bongaerts et al., 2010, 2011; van Oppen et al., 2018), with parallel patterns observed in coral endosymbionts (Frade et al., 2008; Bongaerts et al., 2010; Pantos et al., 2015; Hernandez-Agreda et al., 2018; van Oppen et al., 2018). Such findings highlight the importance of environment-associated selection and the potential for ecological barriers to gene flow. However, substantial genetic and phenotypic diversity in nominal species is being uncovered within reef habitats (Dubé et al., 2017; Gélin et al., 2017; Forsman et al., 2020), with much of that diversity remaining unexplained. While advances in omics-based approaches have shown great potential (Riginos et al., 2016; Matz, 2018), our ability to understand such diversity has been limited by the difficulty of gathering high-resolution data on the corresponding phenotypes and associated environments. Understanding the adaptive potential of this genetic and phenotypic variation (e.g., tolerance to warming or eutrophication) and its nature or origin (e.g., standing genetic variation, hybridization, somatic mutations, or epigenetic), is becoming increasingly important to predict how corals may persist into the future. It is also critical for coral reef conservation and restoration efforts, to ensure that the adaptive potential of protected, translocated, or restored populations is maximized to promote survival under rapidly changing environmental conditions.
Reefscape Characterization Through Close-Range Photogrammetry
The logistical difficulty of fine-scale underwater mapping and (geo)referencing has long hampered spatially explicit coral reef studies and thereby direct coupling of genetic data with environmental, ecological, and phenotypic data. However, recent advances in photogrammetry –in particular Structure from Motion (SfM)– now permit fine-scale 3D characterization based on consumer-grade cameras and non-expert software (Figueira et al., 2015; Burns and Delparte, 2017; DeBell et al., 2019). In contrast to stereophotogrammetry that usually relies on calibrated image pairs, SfM can approximate camera position and angle from highly overlapping photographs to generate a “sparse point cloud” (Westoby et al., 2012). This can be further processed using multi-view stereo algorithms into a “dense point cloud” (Iglhaut et al., 2019), from which 2D (orthoprojection/mosaic), 2.5D (digital elevation model), or 3D (textured 3D mesh) products can be generated (Figure 1). SfM has been widely adopted in geoscience for topographical surveys (Westoby et al., 2012; Fonstad et al., 2013; Smith et al., 2016), including subaerial forest, wetland and coastal characterization (Kalacska et al., 2017; Iglhaut et al., 2019), but its close-range ability overcomes persistent underwater light attenuation and scattering issues, making SfM particularly suited for fine-scale benthoscape characterization.
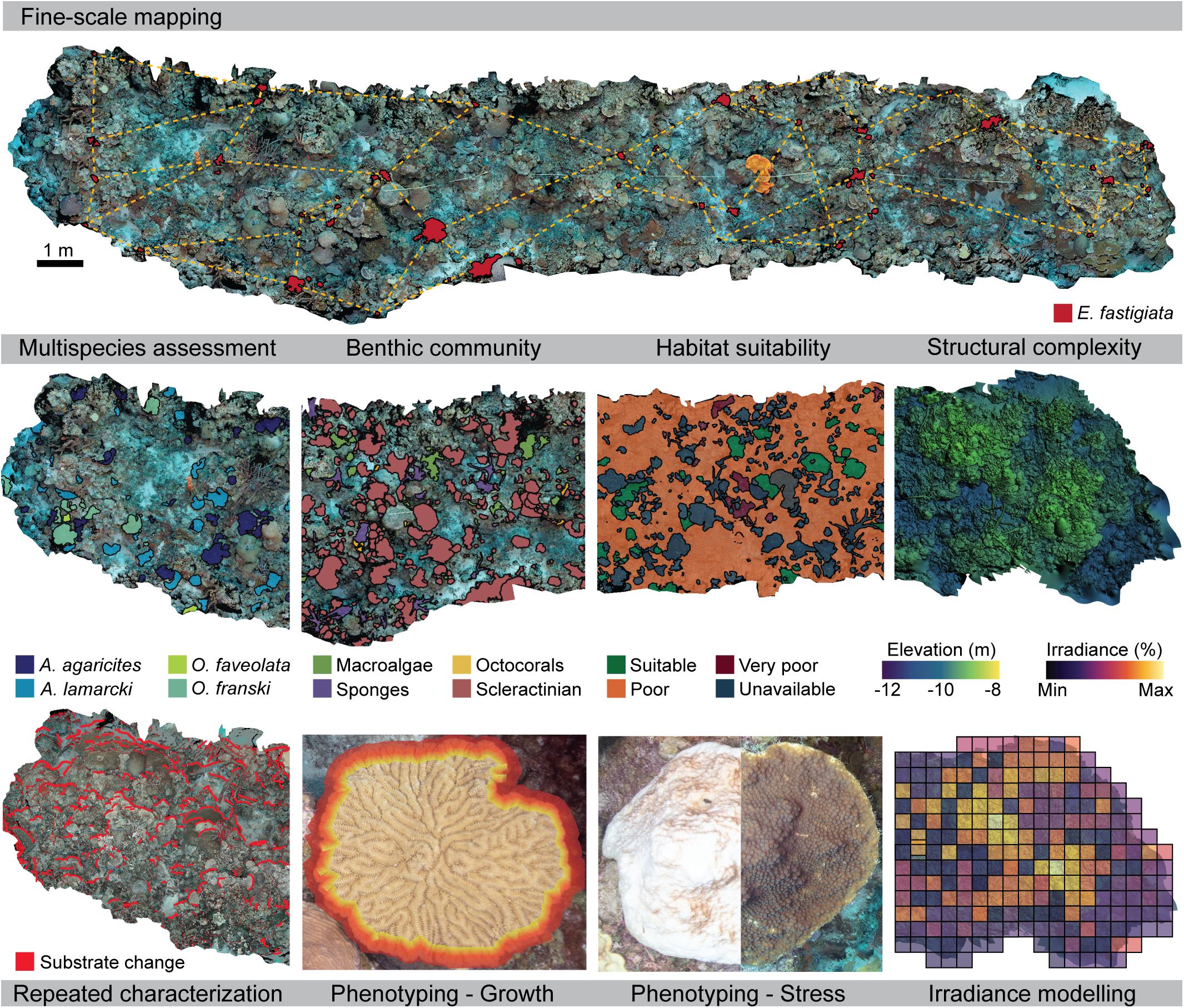
Figure 1. Conceptual diagram summarizing conceptual diagram summarizing different SfM-enabled characterization examples that can be utilized in reefscape genomic studies. The orthoprojection (25 × 4 m) depicts a Caribbean coral reef community at 20 m depth (from the CoralScape project, Curaçao, Southern Caribbean). Fine-scale mapping allows for accurate positioning of target organisms within the reefscape and a priori established sampling designs (depicted here for Eusmilia fastigiata). It also enables spatially explicit, multi-species assessments (e.g., to characterize broader patterns related to life history or the occurrence and consequences of hybridization). Image-based biotic/abiotic characterization of the reefscape can help elucidate the interaction of dispersal, recruitment, and selection processes with the environment (e.g., considering competitive or mutualistic relationships or habitat suitability). Structural complexity or 3D positioning can be similarly considered or used for environmental or biophysical modeling (e.g., incident irradiance, water flow, sedimentation, or larval dispersal). Repeated characterization allows for spatio-temporal consideration of the reefscape and target organisms, as well as mass-phenotyping of the latter (e.g., growth rates or stress responses).
SfM has rapidly become a critical tool in benthic ecology studies on coral reefs (Burns et al., 2015; Leon et al., 2015; Ferrari et al., 2016b; Edwards et al., 2017; González-Rivero et al., 2017), where the requirement of a static environment (throughout the imaging process) is largely satisfied by the dominance of reef-building corals. Depending on camera resolution and altitude (i.e., distance to seafloor), current modeling abilities roughly span a grain range of ∼0.1–10 mm and spatial extent range of 0.01–1 ha (Figure 2), with a trade-off between grain size and spatial extent. Using scale references, a highly accurate local coordinate system of the generated model can be created (mm to cm accuracy; Ledoux et al., 2010), enabling underwater mapping with unprecedented resolution, efficiency, and repeatability compared to traditional methods using transect tapes, depth gages, and/or compasses (Foster et al., 2013; Gorospe and Karl, 2013; Williams et al., 2014; Gélin et al., 2017; Dubé et al., 2017, 2020). Such local coordinates can be converted to real-world coordinates by georeferencing the model using, e.g., ground control points (GCPs) or by integrating acoustic positioning and orientation sensors on the imaging platform [e.g., ultrashort-baseline (USBL), Doppler velocity log (DVL), and attitude and heading reference systems (AHRS)]. In both cases, the accuracy of georeferencing will be determined by that of the surface-based global navigation satellite system (GNSS) receiver, and the method used to determine positioning relative to that of the receiver (e.g., a simple vertical upline or sophistic multisensory navigation system).
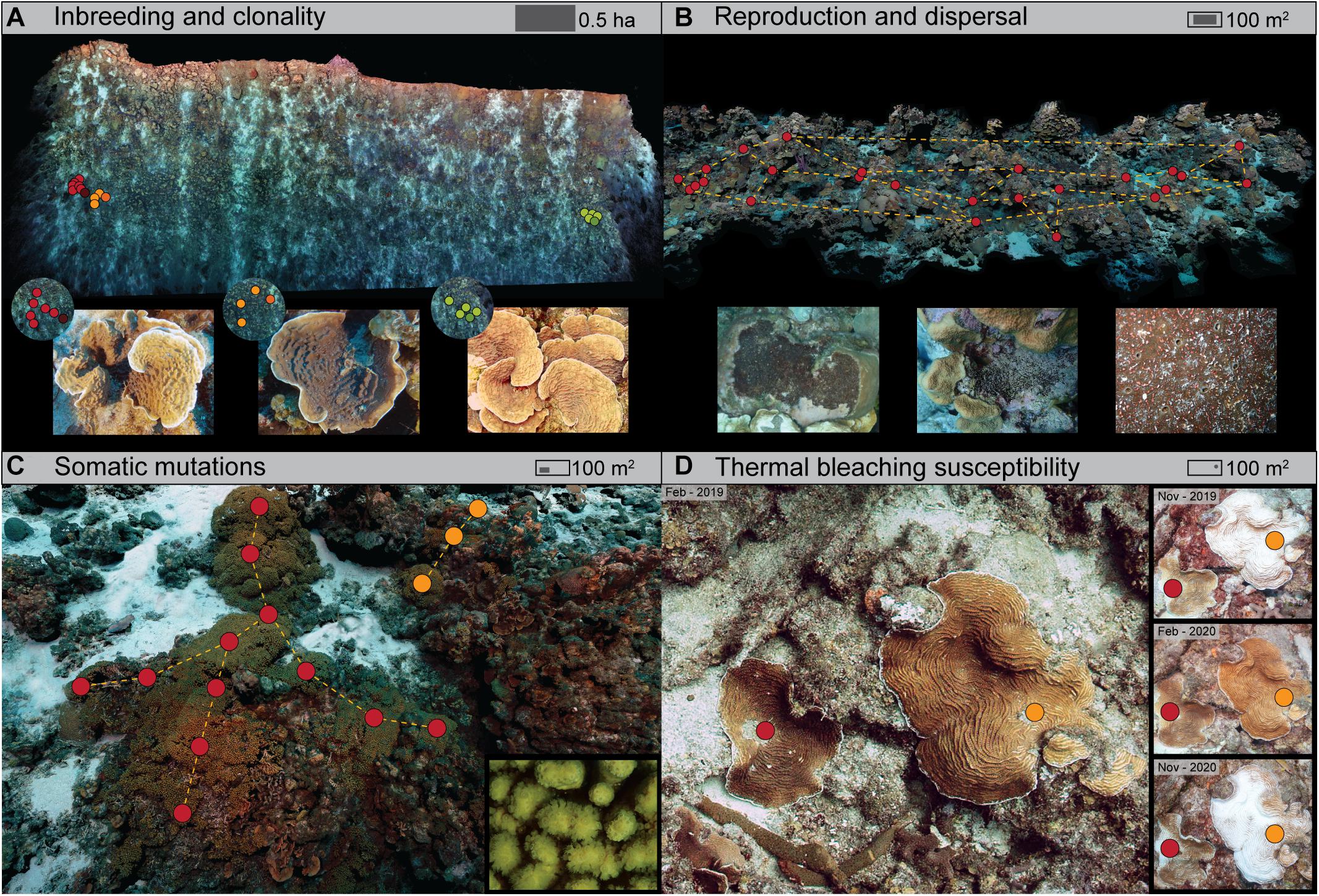
Figure 2. Four example applications of reefscape genomics, conducted as part of the CoralScape project in Curaçao (Southern Caribbean). This project monitors large-area plots (0.5–1 ha per plot) and focal plots (100 m2) covering a range of 5–60 m depth at eight different locations along the leeward shore. The focal plots are reimaged twice a year (using a Canon 5DsR with an EF 24 mm f1/4 prime lens and four Inon Z330 strobes) and incrementally sampled (for different taxa). Collected samples are referenced to the 3D model using video from a head-mounted camera, with the diver carrying a 96-well rack with numbered 0.5 mL tubes bungee-mounted to their forearm. (A) Broad-scale imaging (>0.5 ha per plot) to assess the impact of rapid population decline on the reproduction and genomic variation in the coral Helioseris cucullata, and to evaluate extinction risk and appropriate conservation strategies (Hernandez-Agreda et al., unpublished data). (B) Medium-scale imaging (100 m2 per plot) to understand the roles of niche partitioning and asexual reproduction in the fast expansion of coral-eroding sponges in the Cliona viridis species complex (Achlatis et al., unpublished data). Inset photographs show different growth forms and a close-up. (C) Medium-scale imaging (100 m2 per plot) to track the spread of somatic mutations in the coral Madracis mirabilis, and assess their contributions to genetic diversity in large monospecific stands (Bongaerts et al., unpublished data). (D) Medium-scale imaging (100 m2 per plot) to disentangle the role of environment and genotype in bleaching response and the overall effect of bleaching on population genetic diversity within the coral genus Agaricia (Prata et al., unpublished data). Circles represent samples of target organisms (as depicted in close-up photos) colored by genotype (except for in B).
The power of SfM in resolving 3D structure has enabled the study of structural complexity in relation to species diversity, competition, and coexistence. Structural complexity can be characterized through various metrics: linear and surface rugosity (Dustan et al., 2013; Ferrari et al., 2016a, 2018), fractal dimension (Tokeshi and Arakaki, 2012; Leon et al., 2015; Young et al., 2017), crevice or refuge density (González-Rivero et al., 2017; Agudo-Adriani et al., 2019; Oakley-Cogan et al., 2020), viewshed (González-Rivero et al., 2017; Urbina-Barreto et al., 2020), and surface height range (Torres-Pulliza et al., 2020). Broader-scale environmental parameterization (e.g., through the deployment of sensors) has the potential to enable fine-scale modeling of further abiotic variables that cannot be inferred directly from the imagery, such as temperature, irradiance, water flow, and sedimentation across the reefscape (Figure 1). As SfM is imagery-based, detailed characterization (2D/3D) of the seafloor can be undertaken through point-based annotation or semantic segmentation, with promising automation potential through machine learning (Alonso et al., 2019; Williams et al., 2019; Pavoni et al., 2020). Species-level identifications and recruit detection can be facilitated by the pairing of individual points to the original photographs (usually having greater resolution than the constructed dense point cloud), with such characterizations rapidly providing new insights into coral demographics (Edwards et al., 2017; Brito-Millán et al., 2019; Pedersen et al., 2019). The simultaneous documentation of the abiotic and biotic reefscape holds particular promise for landscape community genomic approaches focusing on the interaction of environmental and community effects on genomic variation (Hand et al., 2015).
Another major advantage of SfM characterization is its suitability for repeat surveys, describing how target species populations and interfering biotic and abiotic reefscapes change over time. SfM also opens up the opportunity for simultaneous in situ phenotyping of focal organisms through the obtained imagery. Although certain morphological (e.g., gross morphology) and ecological (e.g., symbiotic state) aspects can be extracted from a single time-point, repeated characterization allows for the determination of growth rates (surface or linear expansion; Holmes et al., 2008) or susceptibility to stressors (Chow et al., 2016; Miller et al., 2016; Precht et al., 2016; Page et al., 2017; Gintert et al., 2018; Johnston et al., 2019). The third dimension that photogrammetry adds significantly enhances all aspects of phenotyping; for example, growth traits of corals and other invertebrates are more accurately determined from 3D surface areas and volumes (Lavy et al., 2015; Gutiérrez-Heredia et al., 2016; Ferrari et al., 2017; Olinger et al., 2019), as are other colony-level and polyp-level morphological traits (Kruszyński et al., 2007; Gutiérrez-Heredia et al., 2015).
Opportunities Enabled by Reefscape Genomics
Photogrammetric approaches uniquely enable both fine-scale mapping and simultaneous characterization of the focal organism and surrounding reefscape, and will provide a step change in our ability to conduct landscape genomic assessments in marine environments. Such approaches have the potential to overcome pervasive sampling biases associated with underwater population genetic studies (Gorospe et al., 2015; Riginos, 2015) in that rigorous sampling designs can be established based on a priori characterized positioning, micro-environment, and phenotypes of organisms across the reefscape (Figure 1). As the spatial extent and grain of the reefscape characterization can vary per imaging platform (diver-based or autonomous underwater vehicle) and strategy (low or high altitude), reefscape genomic approaches allow for spatially explicit assessments from fine-scale (e.g., assessing the spread of somatic mutations or distribution of endosymbiotic associations within/between colonies), to medium-scale (e.g., patterns of genetic variation, kinship, and clonality within/across reef habitats), and broad-scale (e.g., in conservation genomics assessments of rare and threatened species at the scale of hectares) (Figure 2). Currently, these assessments can be conducted across multiple locations to enable parallel comparisons, or they can be incorporated within a hierarchical seascape genomics framework. Ultimately, they may converge with broader seascape-scale assessments as technologies advance. The explicit consideration of the benthoscape opens up the novel opportunity to assess the effect of the fine-scale biotic and abiotic composition, configuration, and traversability of the underwater landscape on gene flow and dispersal through the use of spatial correlation analyses (e.g., Moran’s Eigenvector Maps; Dray et al., 2006) and analyses that identify gene flow pathways (e.g., resistance-based; McRae, 2006; Petkova et al., 2016). Overall, by enabling repeatable surveys and eliminating constraints on grain size (previously imposed through shipboard, aerial, or orbital characterization), we can now effectively assess the fine-scale spatiotemporal drivers of the extensive unexplained diversity and the hierarchical genetic structuring on coral reefs.
Selection is expected to play a dominant role in shaping the genetic variation of coral reef inhabitants due to the marked environmental heterogeneity occurring between and within reef habitats. Existing approaches investigating adaptive variation can be divided into those that identify genetic signatures of selection resulting from environmental conditions [e.g., outlier tests and genetic-environment association (GEA); Rellstab et al., 2015] and those that identify associations between genotypes and phenotypic traits [e.g., quantitative trait loci (QTL mapping); Stinchcombe and Hoekstra, 2008, genome-wide association studies (GWAS); Korte and Farlow, 2013, and genome-wide selection (GS); Meuwissen et al., 2001]. However, in coral reef invertebrates, GEAs have almost exclusively been explored in relation to either broad-scale oceanographic settings or discrete reef habitats. Characterization of the reefscape now opens the opportunity to investigate the role of fine-scale and biotic selective pressures in population genetic structuring (Gorospe and Karl, 2013), and to explore whether the “sympatric” distribution of morphologically cryptic lineages (Warner et al., 2015) may have overlooked niche partitioning across micro-environments. Moreover, the difficulty of conducting large-scale phenotypic characterization through aquarium-based (due to collection impact concerns) or natural experiments (due to challenges of the underwater environment) has hindered the ability to detect genetic-phenotypic associations. As photogrammetry offers the opportunity of repeated characterization of target organisms, it has the potential to scale up phenotyping efforts of critical traits. Large sample sizes are particularly important for the detection of polygenic signals (i.e., where the phenotype is influenced by more than one locus), such as those identified in relation to thermal bleaching susceptibility (Bay and Palumbi, 2014; Jin et al., 2016; Fuller et al., 2020). Overall, the most promising advance of reefscape genomics is the ability to simultaneously consider the interaction of genotype, (micro-)environment, and phenotype. Disentangling this interaction could elucidate fundamental but poorly understood processes affecting natural evolutionary trajectories, such as cryptic diversification, hybridization, and heritable changes in gene expression (epigenetics). Considering this interaction would also have substantial benefits in terms of restoration and assisted evolution efforts, through more informed identification of resilient natural genotypes and selection of suitable outplanting/transplantation environments (as described in van Oppen et al., 2015; Baums et al., 2019).
Conclusion
As advances in genomics have offered the opportunity to transition from few neutral markers to genome-wide assessments, advances in underwater imaging now unlock the full potential of these assessments in benthic marine ecosystems by enabling spatially explicit (individual-based) sampling integrated with fine-scale biotic and abiotic characterization. As discussed in this perspective, this provides the unprecedented potential to apply fine-scale landscape genomics approaches to coral reef environments, allowing us to address fundamental knowledge gaps regarding the role of neutral and adaptive processes in the structuring of coral reef biodiversity. Additional methodological advantages are the opportunities for simultaneous mass-phenotyping (e.g., growth and thermal susceptibility), repeatable surveys (e.g., explaining how demographic changes contribute to changing allele frequencies), cumulative data gathering (e.g., revisit and expand sampling to additional individuals or species), efficient characterization of difficult-to-access environments (e.g., mesophotic habitats), and robust sampling design planning (e.g., based on a priori mapped individuals). Although close-range photogrammetry is uniquely suited to document the static structures of reef-building corals, a “benthoscape genomics” approach (to use a more inclusive term) is equally applicable to other marine benthic habitats (e.g., deep-sea bioherms, mangroves, or rocky reefs) where the requirement of a largely static environment can be met. Studying fine-scale patterns and processes in marine ecosystems will be critical in advancing our understanding of contradictory metapopulation structures, our ability to accurately analyze and interpret broader-scale patterns, and ultimately, our capacity to effectively conserve these ecosystems into the future.
Author Contributions
PB conceived of the presented idea with input from the other authors. JG created the figures. All authors helped develop the concepts and contributed equally to the writing of the manuscript.
Funding
This work was funded by the Hope for Reefs Initiative at the California Academy of Sciences.
Conflict of Interest
The authors declare that the research was conducted in the absence of any commercial or financial relationships that could be construed as a potential conflict of interest.
Acknowledgments
We would like to thank the teams of the 100 Island Challenge at Scripps Institution of Oceanography (SIO), the Australian Centre for Field Robotics (ACFR), the Marine Imaging Lab at the University of Haifa, and the Australian Institute of Marine Science (AIMS) for collaborative input on photogrammetry methods. We would also like to thank our Diving Operations team at the California Academy of Sciences for supporting the realization of these ideas in the field.
References
Agudo-Adriani, E. A., Cappelletto, J., Cavada-Blanco, F., and Cróquer, A. (2019). Structural complexity and benthic cover explain reef-scale variability of fish assemblages in Los Roques National Park, Venezuela. Front. Mar. Sci. 6:690. doi: 10.3389/fmars.2019.00690
Alonso, I., Yuval, M., Eyal, G., Treibitz, T., and Murillo, A. C. (2019). CoralSeg: learning coral segmentation from sparse annotations. J. Field Robot. 36, 1456–1477. doi: 10.1002/rob.21915
Andrew, R. L., Bernatchez, L., Bonin, A., Buerkle, A. C., Carstens, B. C., Emerson, B. C., et al. (2013). A road map for molecular ecology. Mol. Ecol. 22, 2605–2626. doi: 10.1111/mec.12319
Arias-González, J., Done, T., Page, C., Cheal, A., Kininmonth, S., and Garza-Pérez, J. (2006). Towards a reefscape ecology: relating biomass and trophic structure of fish assemblages to habitat at Davies Reef, Australia. Mar. Ecol. Prog. Ser. 320, 29–41. doi: 10.3354/meps320029
Ayre, D. J., and Hughes, T. P. (2000). Genotypic diversity and gene flow in brooding and spawning corals along the Great Barrier Reef, Australia. Evolution 54, 1590–1605. doi: 10.1111/j.0014-3820.2000.tb00704.x
Balkenhol, N., Cushman, S. A., Waits, L. P., and Storfer, A. (2016). “Current status, future opportunities, and remaining challenges in landscape genetics,” in Landscape Genetics Concepts, Methods, Applications, First Edition, eds N. Balkenhol, S. A. Cushman, A. T. Storfer, and L. P. Waits (New York: John Wiley & Sons, Ltd), 247–256. doi: 10.1002/9781118525258.ch14
Balkenhol, N., Dudaniec, R. Y., Krutovsky, K. V., Johnson, J. S., Cairns, D. M., Segelbacher, G., et al. (2017). “Landscape genomics: understanding relationships between environmental heterogeneity and genomic characteristics of populations,” in Population Genomics, ed. O. Rajora (Cham, CH: Springer), 261–322. doi: 10.1007/13836_2017_2
Baums, I. B., Baker, A. C., Davies, S. W., Grottoli, A. G., Kenkel, C. D., Kitchen, S. A., et al. (2019). Considerations for maximizing the adaptive potential of restored coral populations in the western Atlantic. Ecol. Appl. 29:e01978. doi: 10.1002/eap.1978
Baums, I. B., Devlin−Durante, M. K., and LaJeunesse, T. C. (2014). New insights into the dynamics between reef corals and their associated dinoflagellate endosymbionts from population genetic studies. Mol. Ecol. 23, 4203–4215. doi: 10.1111/mec.12788
Bay, R. A., and Palumbi, S. R. (2014). Multilocus adaptation associated with heat resistance in reef-building corals. Curr. Bio. 24, 2952–2956. doi: 10.1016/j.cub.2014.10.044
Benzie, J. A. H., Haskell, A., and Lehman, H. (1995). Variation in the genetic composition of coral (Pocillopora damicornis and Acropora palifera) populations from different reef habitats. Mar. Biol. 121, 731–739. doi: 10.1007/BF00349309
Boilard, A., Dubé, C. E., Gruet, C., Mercière, A., Hernandez-Agreda, A., and Derome, N. (2020). Defining coral bleaching as a microbial dysbiosis within the coral holobiont. Microorganisms 8:1682. doi: 10.3390/microorganisms8111682
Bongaerts, P., Riginos, C., Hay, K. B., van Oppen, M. J. H., Hoegh-Guldberg, O., and Dove, S. (2011). Adaptive divergence in a scleractinian coral: physiological adaptation of Seriatopora hystrix to shallow and deep reef habitats. BMC Evol. Biol. 11:303. doi: 10.1186/1471-2148-11-303
Bongaerts, P., Riginos, C., Ridgway, T., Sampayo, E. M., van Oppen, M. J. H., Englebert, N., et al. (2010). Genetic divergence across habitats in the widespread coral Seriatopora hystrix and its associated Symbiodinium. PLoS One 5:e10871. doi: 10.1371/journal.pone.0010871
Brito-Millán, M., Werner, B. T., Sandin, S. A., and McNamara, D. E. (2019). Influence of aggregation on benthic coral reef spatio-temporal dynamics. R. Soc. Open Sci. 6:181703. doi: 10.1098/rsos.181703
Burns, J., Delparte, D., Gates, R., and Takabayashi, M. (2015). Integrating structure-from-motion photogrammetry with geospatial software as a novel technique for quantifying 3D ecological characteristics of coral reefs. PeerJ. 3:e1077. doi: 10.7717/peerj.1077
Burns, J. H. R., and Delparte, D. (2017). Comparison of commercial structure-from-motion photogrammetry software used for underwater three-dimensional modeling of coral reef environments. Int. Arch. Photogramm. Remote Sens. Spatial Inform. Sci. XLII-2/W3, 127–131. doi: 10.5194/isprs-archives-xlii-2-w3-127-2017
Chow, M. H., Tsang, R. H. L., Lam, E. K. Y., and Ang, P. Jr. (2016). Quantifying the degree of coral bleaching using digital photographic technique. J. Exp. Mar. Biol. Ecol. 479, 60–68. doi: 10.1016/j.jembe.2016.03.003
Combosch, D. J., Guzman, H. M., Schuhmacher, H., and Vollmer, S. V. (2008). Interspecific hybridization and restricted trans−Pacific gene flow in the Tropical Eastern Pacific Pocillopora. Mol. Ecol. 17, 1304–1312. doi: 10.1111/j.1365-294X.2007.03672.x
Cros, A., Toonen, R. J., and Karl, S. A. (2020). Is post-bleaching recovery of Acropora hyacinthus on Palau via spread of local kin groups? Coral Reefs 39, 687–699. doi: 10.1007/s00338-020-01961-3
DeBell, L., Duffy, J. P., McKinley, T. J., and Anderson, K. (2019). Species and habitat mapping in two dimensions and beyond. Structure-from-Motion multi-view stereo photogrammetry for the conservation community. bioRxiv [Preprint]. doi: 10.1101/2019.12.16.878033
Dixon, G. B., Davies, S. W., Aglyamova, G. V., Meyer, E., Bay, L. K., and Matz, M. V. (2015). Genomic determinants of coral heat tolerance across latitudes. Science 348, 1460–1462. doi: 10.1126/science.1261224
Dray, S., Legendre, P., and Peres-Neto, P. R. (2006). Spatial modelling: a comprehensive framework for principal coordinate analysis of neighbour matrices (PCNM). Ecol. Model. 196, 483–493. doi: 10.1016/j.ecolmodel.2006.02.015
Dubé, C. E., Boissin, E., Maynard, J. A., and Planes, S. (2017). Fire coral clones demonstrate phenotypic plasticity among reef habitats. Mol. Ecol. 26, 3860–3869. doi: 10.1111/mec.14165
Dubé, C. E., Boissin, E., Mercière, A., and Planes, S. (2020). Parentage analyses identify local dispersal events and sibling aggregations in a natural population of Millepora hydrocorals, a free−spawning marine invertebrate. Mol. Ecol. 29, 1508–1522. doi: 10.1111/mec.15418
Dustan, P., Doherty, O., and Pardede, S. (2013). Digital reef rugosity estimates coral reef habitat complexity. PLoS One 8:e57386. doi: 10.1371/journal.pone.0057386
Edwards, C. B., Eynaud, Y., Williams, G. J., Pedersen, N. E., Zgliczynski, B. J., Gleason, A. C., et al. (2017). Large-area imaging reveals biologically driven non-random spatial patterns of corals at a remote reef. Coral Reefs 36, 1291–1305. doi: 10.1007/s00338-017-1624-3
Ferrari, R., Figueira, W. F., Pratchett, M. S., Boube, T., Adam, A., Kobelkowsky-Vidrio, T., et al. (2017). 3D photogrammetry quantifies growth and external erosion of individual coral colonies and skeletons. Sci. Rep. 7:16737. doi: 10.1038/s41598-017-16408-z
Ferrari, R., Malcolm, H. A., Byrne, M., Friedman, A., Williams, S. B., Schultz, A., et al. (2018). Habitat structural complexity metrics improve predictions of fish abundance and distribution. Ecography 41, 1077–1091. doi: 10.1111/ecog.02580
Ferrari, R., McKinnon, D., He, H., Smith, R., Corke, P., González-Rivero, M., et al. (2016b). Quantifying multiscale habitat structural complexity: a cost-effective framework for underwater 3D modelling. Remote Sens. 8:113. doi: 10.3390/rs8020113
Ferrari, R., Bryson, M., Bridge, T. C. L., Hustache, J., Williams, S. B., Byrne, M., et al. (2016a). Quantifying the response of structural complexity and community composition to environmental change in marine communities. Glob. Chang. Biol. 22, 1965–1975. doi: 10.1111/gcb.13197
Figueira, W., Ferrari, R., Weatherby, E., Porter, A., Hawes, S., and Byrne, M. (2015). Accuracy and precision of habitat structural complexity metrics derived from underwater photogrammetry. Remote Sens. 7, 16883–16900. doi: 10.3390/rs71215859
Fonstad, M. A., Dietrich, J. T., Courville, B. C., Jensen, J. L., and Carbonneau, P. E. (2013). Topographic structure from motion: a new development in photogrammetric measurement. Earth Surf. Process. Landf. 38, 421–430. doi: 10.1002/esp.3366
Forsman, Z. H., Ritson-Williams, R., Tisthammer, K., Knapp, I. S. S., and Toonen, R. J. (2020). Host-symbiont coevolution, cryptic structure, and bleaching susceptibility, in a coral species complex (Scleractinia; Poritidae). Sci. Rep. 10:16995. doi: 10.1038/s41598-020-73501-6
Foster, N. L., Baums, I. B., Sanchez, J. A., Paris, C. B., Chollett, I., Agudelo, C. L., et al. (2013). Hurricane-driven patterns of clonality in an ecosystem engineer: the caribbean coral Montastraea annularis. PLoS One 8:e53283. doi: 10.1371/journal.pone.0053283
Frade, P. R., De Jongh, F., Vermeulen, F., Van Bleijswijk, J., and Bak, R. P. M. (2008). Variation in symbiont distribution between closely related coral species over large depth ranges. Mol. Ecol. 17, 691–703. doi: 10.1111/j.1365-294X.2007.03612.x
Fuller, Z., Mocellin, V. J. L., Morris, L. A., Cantin, N., Shepherd, J., Sarre, L., et al. (2020). Population genetics of the coral Acropora millepora: toward genomic prediction of bleaching. Science 369:eaba4674. doi: 10.1126/science.aba4674
Gélin, P., Fauvelot, C., Bigot, L., Baly, J., and Magalon, H. (2017). From population connectivity to the art of striping Russian dolls: the lessons from Pocillopora corals. Ecol. Evol. 8, 1411–1426. doi: 10.1002/ece3.3747
Gintert, B. E., Manzello, D. P., Enochs, I. C., Kolodziej, G., Carlton, R., Gleason, A. C. R., et al. (2018). Marked annual coral bleaching resilience of an inshore patch reef in the Florida Keys: a nugget of hope, aberrance, or last man standing? Coral Reefs 37, 533–547. doi: 10.1007/s00338-018-1678-x
González-Rivero, M., Harborne, A. R., Herrera-Reveles, A., Bozec, Y.-M., Rogers, A., Friedman, A., et al. (2017). Linking fishes to multiple metrics of coral reef structural complexity using three-dimensional technology. Sci. Rep. 7:13965. doi: 10.1038/s41598-017-14272-5
Gorospe, K. D., Donahue, M. J., and Karl, S. A. (2015). The importance of sampling design: spatial patterns and clonality in estimating the genetic diversity of coral reefs. Mar. Biol. 162, 917–928. doi: 10.1007/s00227-015-2634-8
Gorospe, K. D., and Karl, S. A. (2013). Genetic relatedness does not retain spatial pattern across multiple spatial scales: dispersal and colonization in the coral, Pocillopora damicornis. Mol. Ecol. 22, 3721–3736. doi: 10.1111/mec.12335
Gutiérrez-Heredia, L., Benzoni, F., Murphy, E., and Reynaud, E. G. (2016). End to end digitisation and analysis of three-dimensional coral models, from communities to corallites. PLoS One 11:e0149641. doi: 10.1371/journal.pone.0149641
Gutiérrez-Heredia, L., D’Helft, C., and Reynaud, E. G. (2015). Simple methods for interactive 3D modeling, measurements, and digital databases of coral skeletons. Limnol. Oceanogr. Methods 13:178–193. doi: 10.1002/lom3.10017
Hand, B. K., Lowe, W. H., Kovach, R. P., Muhlfeld, C. C., and Luikart, G. (2015). Landscape community genomics: understanding eco-evolutionary processes in complex environments. Trends Ecol. Evol. 30, 161–168. doi: 10.1016/j.tree.2015.01.005
Hellberg, M. E. (2007). Footprints on water: the genetic wake of dispersal among reefs. Coral Reefs 26, 463–473. doi: 10.1007/s00338-007-0205-2
Hernandez-Agreda, A., Leggat, W., Bongaerts, P., Herrera, C., and Ainsworth, T. D. (2018). Rethinking the coral microbiome: simplicity exists within a diverse microbial biosphere. mBio 9, e00812–18.
Hoegh-Guldberg, O., Mumby, P. J., Hooten, A. J., Steneck, R. S., Greenfield, P., Gomez, E., et al. (2007). Coral reefs under rapid climate change and ocean acidification. Science 318, 1737–1742. doi: 10.1126/science.1152509
Holmes, G., Ortiz, J., Kaniewska, P., and Johnstone, R. (2008). Using three-dimensional surface area to compare the growth of two Pocilloporid coral species. Mar. Biol. 155, 421–427. doi: 10.1007/s00227-008-1040-x
Iglhaut, J., Cabo, C., Puliti, S., Piermattei, L., O’Connor, J., and Rosette, J. (2019). Structure from motion photogrammetry in forestry: a review. Curr. For. Rep. 5, 155–168. doi: 10.1007/s40725-019-00094-3
Jin, Y. K., Lundgren, P., Lutz, A., Raina, J. B., Howells, E. J., Paley, A. S., et al. (2016). Genetic markers for antioxidant capacity in a reef-building coral. Sci. Adv. 2:e1500842. doi: 10.1126/sciadv.1500842
Johnston, M. A., Hickerson, E. L., Nuttall, M. F., Blakeway, R. D., Sterne, T. K., Eckert, R. J., et al. (2019). Coral bleaching and recovery from 2016 to 2017 at East and West Flower Garden Banks, Gulf of Mexico. Coral Reefs 38, 787–799. doi: 10.1007/s00338-019-01788-7
Kalacska, M., Chmura, G. L., Lucanus, O., Bérubé, D., and Arroyo-Mora, J. P. (2017). Structure from motion will revolutionize analyses of tidal wetland landscapes. Remote Sens. Environ. 199, 14–24. doi: 10.1016/j.rse.2017.06.023
Kinlan, B. P., Gaines, S. D., and Lester, S. E. (2005). Propagule dispersal and the scales of marine community process. Divers. Distrib. 11, 139–148. doi: 10.1111/j.1366-9516.2005.00158.x
Korte, A., and Farlow, A. (2013). The advantages and limitations of trait analysis with GWAS: a review. Plant Methods 9:29. doi: 10.1186/1746-4811-9-29
Kruszyński, K. J., Kaandorp, J. A., and van Liere, R. (2007). A computational method for quantifying morphological variation in scleractinian corals. Coral Reefs 26, 831–840. doi: 10.1007/s00338-007-0270-6
Lavy, A., Eyal, G., Neal, B., Keren, R., Loya, Y., and Ilan, M. (2015). A quick, easy and non−intrusive method for underwater volume and surface area evaluation of benthic organisms by 3D computer modelling. Methods Ecol. Evol. 6, 521–531. doi: 10.1111/2041-210x.12331
Ledoux, J.-B., Garrabou, J., Bianchimani, O., Drap, P., Féral, J.-P., and Aurelle, D. (2010). Fine-scale genetic structure and inferences on population biology in the threatened Mediterranean red coral, Corallium rubrum. Mol. Ecol. 19, 4204–4216. doi: 10.1111/j.1365-294x.2010.04814.x
Leon, J. X., Roelfsema, C. M., Saunders, M. I., and Phinn, S. R. (2015). Measuring coral reef terrain roughness using ‘Structure-from-Motion’ close-range photogrammetry. 242, 21–28. doi: 10.1016/j.geomorph.2015.01.030
Li, Y., Zhang, X.-X., Mao, R.-L., Yang, J., Miao, C.-Y., Li, Z., et al. (2017). Ten years of landscape genomics: challenges and opportunities. Front. Plant Sci. 8:2138. doi: 10.3389/fpls.2017.02136
Liggins, L., Treml, E. A., and Riginos, C. (2013). Taking the plunge: an introduction to undertaking seascape genetic studies and using biophysical models. Geogr. Compass 7, 173–196. doi: 10.1111/gec3.12031
Liggins, L., Treml, E. A., and Riginos, C. (2019). “Seascape genomics: contextualizing adaptive and neutral genomic variation in the ocean environment,” in Population Genomics Marine Organisms. Population Genomics, eds M. Oleksiak and O. Rajora (Cham, CH: Springer), 171–218. doi: 10.1007/13836_2019_68
Manel, S., Schwartz, M. K., Luikart, G., and Taberlet, P. (2003). Landscape genetics: combining landscape ecology and population genetics. Trends Ecol. Evol. 18, 189–197. doi: 10.1016/s0169-5347(03)00008-9
Matz, M. V. (2018). Fantastic beasts and how to sequence them: ecological genomics for obscure model organisms. Trends Genet. 34, 121–132. doi: 10.1016/j.tig.2017.11.002
McClenachan, L., O’Connor, G., Neal, B. P., Pandolfi, J. M., and Jackson, J. B. (2017). Ghost reefs: nautical charts document large spatial scale of coral reef loss over 240 years. Sci. Adv. 3:e1603155. doi: 10.1126/sciadv.1603155
Meuwissen, T. H., Hayes, B. J., and Goddard, M. E. (2001). Prediction of total genetic value using genome-wide dense marker maps. Genetics 157, 1819–1829. doi: 10.1093/genetics/157.4.1819
Miller, K. J., and Ayre, D. J. (2004). The role of sexual and asexual reproduction in structuring high latitude populations of the reef coral Pocillopora damicornis. Heredity 92, 557–568. doi: 10.1038/sj.hdy.6800459
Miller, M. W., Karazsia, J., Groves, C. E., Griffin, S., Moore, T., Wilber, P., et al. (2016). Detecting sedimentation impacts to coral reefs resulting from dredging the Port of Miami, Florida USA. PeerJ. 4:e2711. doi: 10.7717/peerj.2711
Oakley-Cogan, A., Tebbett, S. B., and Bellwood, D. R. (2020). Habitat zonation on coral reefs: structural complexity, nutritional resources and herbivorous fish distributions. PLoS One 15:e0233498. doi: 10.1371/journal.pone.0233498
Olinger, L. K., Scott, A. R., McMurray, S. E., and Pawlik, J. R. (2019). Growth estimates of Caribbean reef sponges on a shipwreck using 3D photogrammetry. Sci. Rep. 9:18398. doi: 10.1038/s41598-019-54681-2
Page, C. A., Field, S. N., Pollock, F. J., Lamb, J. B., Shedrawi, G., and Wilson, S. K. (2017). Assessing coral health and disease from digital photographs and in situ surveys. Environ. Monit. Assess. 189:18. doi: 10.1007/s10661-016-5743-z
Pantos, O., Bongaerts, P., Dennis, P. G., Tyson, G. W., and Hoegh-Guldberg, O. (2015). Habitat-specific environmental conditions primarily control the microbiomes of the coral Seriatopora hystrix. ISME J. 9, 1916–1927. doi: 10.1038/ismej.2015.3
Pavoni, G., Corsini, M., and Cignoni, P. (2020). A state of the art technology in large scale underwater monitoring. ERCIM News 2020:121.
Pedersen, N. E., Edwards, C. B., Eynaud, Y., Gleason, A. C., Smith, J. E., and Sandin, S. A. (2019). The influence of habitat and adults on the spatial distribution of juvenile corals. Ecography 42, 1703–1713. doi: 10.1111/ecog.04520
Petkova, D., Novembre, J., and Stephens, M. (2016). Visualizing spatial population structure with estimated effective migration surfaces. Nat. Genet. 48, 94–100. doi: 10.1038/ng.3464
Precht, W. F., Gintert, B. E., Robbart, M. L., Fura, R., and van Woesik, R. (2016). Unprecedented disease-related coral mortality in Southeastern Florida. Sci. Rep. 6:31374. doi: 10.1038/srep31374
Rellstab, C., Gugerli, F., Eckert, A. J., Hancock, A. M., and Holderegger, R. (2015). A practical guide to environmental association analysis in landscape genomics. Mol. Ecol. 24, 4348–4370. doi: 10.1111/mec.13322
Riginos, C. (2015). Clones in space—how sampling can bias genetic diversity estimates in corals: editorial comment on the feature article by Gorospe et al. Mar. Biol. 162, 913–915. doi: 10.1007/s00227-015-2638-4
Riginos, C., Crandall, E. D., Liggins, L., Bongaerts, P., and Treml, E. A. (2016). Navigating the currents of seascape genomics: how spatial analyses can augment population genomic studies. Curr. Zool. 62, 581–601. doi: 10.1093/cz/zow067
Riginos, C., and Liggins, L. (2013). Seascape genetics: populations, individuals, and genes marooned and adrift. Geogr. Compass 7, 197–216. doi: 10.1111/gec3.12032
Selmoni, O., Rochat, E., Lecellier, G., Berteaux−Lecellier, V., and Joost, S. (2020b). Seascape genomics as a new tool to empower coral reef conservation strategies: an example on north−western Pacific Acropora digitifera. Evol. Appl. 13, 1923–1938. doi: 10.1111/eva.12944
Selmoni, O., Lecellier, G., Magalon, H., Vigliola, L., Benzoni, F., Peignon, C., et al. (2020a). Seascape genomics reveals candidate molecular targets of heat stress adaptation in three coral species. bioRxiv [Preprint]. doi: 10.1101/2020.05.12.090050,
Smith, M. W., Caick, J. L., and Quincey, D. J. (2016). Structure from motion photogrammetry in physical geography. Prog. Phys. Geogr. 40, 247–275. doi: 10.1177/0309133315615805
Stinchcombe, J. R., and Hoekstra, H. E. (2008). Combining population genomics and quantitative genetics: finding the genes underlying ecologically important traits. Heredity 100, 158–170. doi: 10.1038/sj.hdy.6800937
Tokeshi, M., and Arakaki, S. (2012). Habitat complexity in aquatic systems: fractals and beyond. Hydrobiologia 685, 27–47. doi: 10.1007/s10750-011-0832-z
Torres-Pulliza, D., Dornelas, M. A., Pizarro, O., Bewley, M., Blowes, S. A., Boutros, N., et al. (2020). A geometric basis for surface habitat complexity and biodiversity. Nat. Ecol. Evol. 4, 1495–1501. doi: 10.1038/s41559-020-1281-8
Underwood, J. N., Richards, Z. T., Miller, K. J., Puotinen, M. L., and Gilmour, J. P. (2018). Genetic signatures through space, time and multiple disturbances in a ubiquitous brooding coral. Mol. Ecol. 27, 1586–1602. doi: 10.1111/mec.14559
Underwood, J. N., Smith, L. D., van Oppen, M. J. H., and Gilmour, J. P. (2007). Multiple scales of genetic connectivity in a brooding coral on isolated reefs following catastrophic bleaching. Mol. Ecol. 16, 771–784. doi: 10.1111/j.1365-294X.2006.03187.x
Urbina-Barreto, I., Chiroleu, F., Pinel, R., Fréchon, L., Mahamadaly, V., Elise, S., et al. (2020). Quantifying the shelter capacity of coral reefs using photogrammetric 3D modeling: from colonies to reefscapes. Ecol. Indic. 121:107151. doi: 10.1016/j.ecolind.2020.107151
van Oppen, M. J. H., Bongaerts, P., Frade, P., Peplow, L. M., Boyd, S. E., Nim, H. T., et al. (2018). Adaptation to reef habitats through selection on the coral animal and its associated microbiome. Mol. Ecol. 27, 2956–2971. doi: 10.1111/mec.14763
van Oppen, M. J. H., and Gates, R. D. (2006). Conservation genetics and the resilience of reef-building corals. Mol. Ecol. 15, 3863–3883. doi: 10.1111/j.1365-294x.2006.03026.x
van Oppen, M. J. H., Lutz, A., De’ath, G., Peplow, L., and Kininmonth, S. (2008). Genetic traces of recent long-distance dispersal in a predominantly self-recruiting coral. PLoS One 3:e3401. doi: 10.1371/journal.pone.0003401
van Oppen, M. J. H., Oliver, J. K., Putnam, H. M., and Gates, R. D. (2015). Building coral reef resilience through assisted evolution. Proc. Natl. Acad. Sci. U. S. A. 112, 2307–2313. doi: 10.1073/pnas.1422301112
Van Wynsberge, S., Andréfouët, S., Gaertner-Mazouni, N., Tiavouane, J., Grulois, D., Lefèvre, J., et al. (2017). Considering reefscape configuration and composition in biophysical models advance seascape genetics. PLoS One 12:e0178239. doi: 10.1371/journal.pone.0178239
Vollmer, S. V., and Palumbi, S. R. (2002). Hybridization and the evolution of reef coral diversity. Science 296, 2023–2025. doi: 10.1126/science.1069524
Warner, P. A., van Oppen, M. J. H., and Willis, B. L. (2015). Unexpected cryptic species diversity in the widespread coral Seriatopora hystrix masks spatial-genetic patterns of connectivity. Mol. Ecol. 24, 2993–3008. doi: 10.1111/mec.13225
Warner, P. A., Willis, B. L., and van Oppen, M. J. H. (2016). Sperm dispersal distances estimated by parentage analysis in a brooding scleractinian coral. Mol. Ecol. 25, 1398–1415. doi: 10.1111/mec.13553
Westoby, M. J., Brasington, J., Glasser, N. F., Hambrey, M. J., and Reynolds, J. M. (2012). ‘Structure-from-Motion’ photogrammetry: a low-cost, effective tool for geoscience applications. Geomorphology 179, 300–314. doi: 10.1016/j.geomorph.2012.08.021
Williams, D. E., Miller, M. W., and Baums, I. B. (2014). Cryptic changes in the genetic structure of a highly clonal coral population and the relationship with ecological performance. Coral Reefs 33, 595–606. doi: 10.1007/s00338-014-1157-y
Williams, I. D., Couch, C. S., Beijbom, O., Oliver, T. A., Vargas-Angel, B., Schumacher, B. D., et al. (2019). Leveraging automated image analysis tools to transform our capacity to assess status and trends of coral reefs. Front. Mar. Sci. 6:222. doi: 10.3389/fmars.2019.00222
Keywords: reefscape genomics, seascape genomics, coral reefs, photogrammetry, structure from motion, landscape genomics
Citation: Bongaerts P, Dubé CE, Prata KE, Gijsbers JC, Achlatis M and Hernandez-Agreda A (2021) Reefscape Genomics: Leveraging Advances in 3D Imaging to Assess Fine-Scale Patterns of Genomic Variation on Coral Reefs. Front. Mar. Sci. 8:638979. doi: 10.3389/fmars.2021.638979
Received: 08 December 2020; Accepted: 16 June 2021;
Published: 15 July 2021.
Edited by:
Manuel Gonzalez-Rivero, Australian Institute of Marine Science (AIMS), AustraliaReviewed by:
Lida Teneva, Independent Researcher, Sacramento, CA, United StatesRenata Ferrari, Australian Institute of Marine Science (AIMS), Australia
Copyright © 2021 Bongaerts, Dubé, Prata, Gijsbers, Achlatis and Hernandez-Agreda. This is an open-access article distributed under the terms of the Creative Commons Attribution License (CC BY). The use, distribution or reproduction in other forums is permitted, provided the original author(s) and the copyright owner(s) are credited and that the original publication in this journal is cited, in accordance with accepted academic practice. No use, distribution or reproduction is permitted which does not comply with these terms.
*Correspondence: Pim Bongaerts, cGJvbmdhZXJ0c0BjYWxhY2FkZW15Lm9yZw==
†These authors have contributed equally to this work