- 1Departamento de Oceanografía and Centro de Investigación Oceanográfica COPAS Sur-Austral, Universidad de Concepción, Concepción, Chile
- 2Centro FONDAP de Investigación en Dinámica de Ecosistemas Marinos de Altas Latitudes (IDEAL), Valdivia-Punta Arenas, Chile
- 3Instituto de Ciencias Marinas y Limnológicas, Universidad Austral de Chile, Valdivia, Chile
In southern Patagonia, the Beagle Channel shows very low production during winter but simultaneously sustains very dense aggregations of the pelagic stage of squat lobster (Munida gregaria), a benthic decapod whose pelagic juveniles have the largest body size within the chitinous pelagic community. To assess the coexistence of the mesozooplankton community and the pelagic M. gregaria stage under the harsh feeding winter conditions, we conducted a research cruise at two locations connected to the Beagle Channel, Yendegaia Bay (land terminating-glacier) and Pia Fjord (marine-terminating glacier). Our results showed that the zooplankton communities were similar in these two fjords, that a single pelagic group dominated in terms of biomass (pelagic Munida gregaria), and that differences in vertical distribution existed between most of the principal crustacean zooplankton and pelagic M. gregaria. All groups showed consumption of terrestrially derived organic matter, as revealed by their δ13C values. However, the isotopic composition, trophic positions (TP), and isotopic niche areas of the groups separated pelagic M. gregaria, presenting some of the lowest δ15N and the highest δ13C values, and the narrowest isotopic niche width. Pelagic M. gregaria was dominated by a single body size class along the 0–100 m water column, with no diel changes in vertical distribution, remained mostly in the upper layers (0–50 m), and benefited from the slightly higher phytoplankton concentrations at shallower depths as revealed by their higher δ13C values and low trophic position. In contrast, the other groups, including zoea M. gregaria stages, developed changes in distribution between day and night or remained deeper in the water column. These groups showed higher δ15N values, higher TP, and lower δ13C values, most of which probably fed on a nanoheterotrophs and terrestrial particulate organic matter mixture at deeper layers. Thus, the different vertical distributions, different trophic level food sources, and slightly different organic carbon sources apparently reduced any potential competence for food resources and form part of the feeding strategy that may facilitate the coexistence of the different large pelagic crustaceans under harsh feeding winter conditions in this high latitude austral region.
Introduction
Austral Patagonia has recently received attention with regard to global climate change because it contains some of the largest freshwater reserves in continental areas (other than Antarctica) in the form of extensive ice fields and glaciers (González et al., 2016; RGI, 2017). Recent reports have documented the retrieval of these ice masses during the last two decades associated with changes in nival and rain precipitation (Climate Change, 2007, 2013; Rivera et al., 2007; Casassa et al., 2010; Marín et al., 2013). These variations, in turn, have induced changes in the amount of water received in the coastal and fjord environments and the amount of terrestrial originated debris conducted toward the adjacent marine system (Dussaillant et al., 2009; Meerhoff et al., 2019; Ross et al., 2020).
The Beagle Channel, one of the only two channels that connects the Pacific and Atlantic oceans in South America, is one of these high-latitude environments in which numerous fjords and glaciers contribute to glacier melted water and runoff, particularly in spring and summer. Because of the difficulties in oceanographic research in winter, most studies on pelagic components of the community and trophic functioning in these areas have been conducted in warmer months (spring and summer) or other seasons in the Atlantic side of the Beagle Channel (Almandoz et al., 2011; Aguirre et al., 2012; Riccialdelli et al., 2017; Diez et al., 2018). Information on the origin of the organic carbon that sustains the pelagic trophic web and how it is trespassed along the different components, thus, remains undocumented for the coldest months (winter), particularly to the Pacific Ocean branches of the Beagle Channel. Previous studies in the northern Chilean Patagonia (42–46°S) have proposed that, in contrast to the spring season, when primary production in the coastal areas is diatom-based, winter production is fueled by the microbial community (González et al., 2010, 2011). Other studies further south (Magellan Strait, 53°S) in Patagonia have documented the important role of the filter feeding euphausiid species (Euphausia vallentini) in channelizing a large amount of phytoplanktonic carbon production in spring, the main source of organic carbon entering the fjords and channels (González et al., 2016). Finally, most recent studies carried out in spring on the Atlantic side of the Beagle Channel (BC; 55°S) report that the carbon flux in the area is based on combined marine phytoplankton, macroalgae, and terrestrially derived plant debris with the largest fraction provided by these latter two sources that reach the higher trophic levels of the food web (Riccialdelli et al., 2017).
Because stable isotope enrichment occurs along the trophic web, stable isotope analyses are currently widely utilized to study the structure and fluxes along food webs (Vander Zanden and Rasmussen, 2001; Post, 2002). In marine ecosystems, for instance, the nitrogen stable isotope 15N has been utilized to indicate the trophic position of certain species along the food web to determine whether this position is shared by other organisms in the same location or if changes occur between seasons or among environments (Lindsay et al., 1998; Tanaka et al., 2008; Malzahn and Boersma, 2009). The carbon stable isotope 13C, in turn, has been utilized to identify the sources of organic carbon in coastal environments because 13C values change if they proceed from terrestrial environments or from marine sources (phytoplankton or macroalgae) (Vander Zanden and Rasmussen, 1999, 2001; Vargas et al., 2011). Stable isotopes were also utilized to determine changes in feeding habits when organisms grow or changed their distribution or depths of residence to feed on prey with different isotopic content (Overman and Parrish, 2001; Laiz-Carrion et al., 2011; Montecinos et al., 2016; Bernal et al., 2020).
Given its high abundance and because its role as an important detritivore species that returns organic matter from the surface sediments to higher level marine predators, such as sea lions, seals, birds, squid, fishes and even whales, the galatheid squat lobster Munida gregaria is considered a key species in Patagonian channels and fjords (Tapella et al., 2002; Vinuesa and Varisco, 2007; Diez et al., 2012; Betti et al., 2020). The species has two morphs, a benthic adult morph, originally named M. gregaria, and a post-larval-juvenile pelagic morph, originally named M. subrugosa, that can reach the size of adults while still in the water column (Pérez-Barros et al., 2008, 2010, 2011). While the depth distributions of both morphs have been reported, there are insufficient studies on shorter-term (diel, seasonal) variations in distributions of different sized pelagic stages in the water column (Meerhoff et al., 2013, 2014), with most reports on the zoea stages of development (Castro et al., 2011, 2019). For these stages, ontogenetic migration from the inner channel zones of zoea release to offshore zones and back to inner areas as megalopa or juveniles, has been documented in northern Chilean Patagonian channels (León et al., 2008; Meerhoff et al., 2013, 2014; Mujica et al., 2013). Whether the juvenile stages change in vertical distribution as they grow or if they carry out shorter-term changes (seasonal, diel vertical migrations) remain to be determined.
Pelagic M. gregaria is a large-sized crustacean in the water column of Patagonian fjords and channels. Other large crustacean zooplankton, usually found in most high-latitude Patagonian channel areas, are euphausiids (Euphausia vallentini), amphipods (Eurythemistos sp.), some large decapods such as Sergestes arcticus, and some mid- to large-sized copepods, such as Calanus australis or Rhincalanus sp. Other holozooplankton groups, but small, often collected in large numbers, are ostracods, cladocerans, and small-sized copepods (Rosenberg and Palma, 2003; Biancalana et al., 2007; Zagami et al., 2011; Aguirre et al., 2012; Giesecke et al., 2019). How these crustacean assemblages cope to survive in a common environment during the winter low production season in these high-latitude areas remains to be determined.
In the present study, we utilized stable isotope analyses and stratified plankton sampling to explore whether the most abundant and large-sized crustacean pelagic groups in the BC develop differential feeding and/or vertical distributions conducive to favor their coexistence during the austral winter. Because most crustacean zooplankton are essentially omnivorous (although some are often classified as mostly herbivores (e.g., E. vallentini, calanoid copepods, ostracods), carnivores, or detritivores (e.g., amphipods, M. gregaria, Sergestes sp.) and because of the scarce winter food availability, we expected most groups would probably develop an opportunistic feeding mode without a clear separation in food items ingested and thereafter, a wide overlay in isotopic trophic niches. At the same time, because of the low winter marine primary production, the different groups would either subsist feeding on microzooplankton and hence reach mid- to high trophic positions, feed on allochthonous organic matter from terrestrial origin or, finally, partition the food resources by residing in different depth layers. Accordingly, the objectives of the present study were to assess (a) whether the largest body size and more abundant zooplankton crustacean groups were the same in different fjords, (b) whether their organic carbon source was primarily of marine origin (autochthonous) or terrestrially (allochthonous) derived, (c) whether the trophic positions and isotopic niche widths differed among crustacean groups, and finally, (d) to evaluate whether potentially different feeding behavior (determined from isotopic composition) along with potential differences in vertical distributions might constitute a way of partitioning the environment to facilitate the maintenance of different groups within this low winter production high-latitude system. Particular emphasis is given to juvenile M. gregaria because it is a species that has been reported to expand its high abundance toward lower latitude channels and fjords (Diez et al., 2016, 2018).
Materials and Methods
Field work. From July 19 to 26, 2017, was conducted a research cruise on board the Motorboat “Forrest” to the BC. Two locations connected to the BC were studied for three days in the austral winter: Yendegaia Bay (central BC, land-terminating-glaciers) and Pia fjord (northwestern arm BC, marine-terminating glaciers). The Yendegaia Bay, ∼10 km long, ∼2.5 km wide and with a maximum depth of ∼220 m, is connected to the Yendegaia River in its head, which incorporates melted water from the Serka (also called Stopanni) glacier located ∼11 km inland. The western arm of Pia fjord, ∼14 km long, 1.25 km wide and with a maximum depth of ∼200 m, receives freshwater directly from the Guilcher glacier in its inner flank (Figure 1).
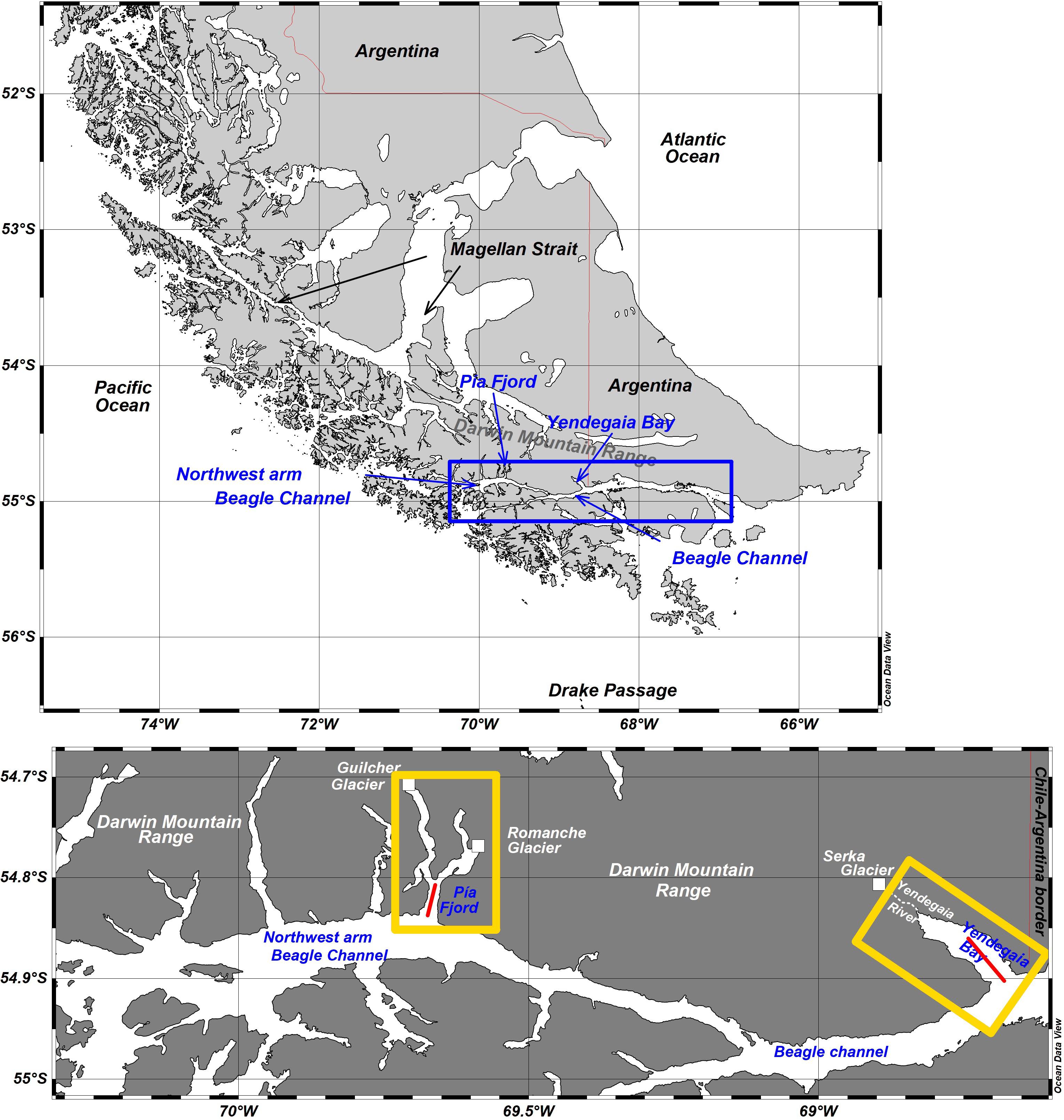
Figure 1. Map of the Southern Patagonia showing the Beagle channel (upper panel) and both sampling locations: Pia fjord and Yendegaia bay (lower panel). Red lines in each location indicates the zooplankton sampling track.
At each location, a longitudinal transect was conducted, in which hydrographic profiles were obtained with a Seabird 25 CTD equipped with a fluorometer and turbidity sensor. Absolute salinity and conservative temperature were derived by applying the algorithms proposed in the Thermodynamic Equation of Seawater 2010 according to the new standards of seawater properties adopted by the Intergovernmental Oceanographic Commission (Valladares et al., 2011). Along the center of the transects, zooplankton profiles of stratified samplings were conducted at each location four times during a 24 h cycle. The profiles included stratified zooplankton samples collected with a 0.5 × 0.5 m mouth opening Tucker trawl (300 μm mesh, equipped with a GO flowmeter) at three depth strata: 0–10, 10–50, 50–100 m. The profiles were obtained during the day and at night. Once onboard, the samples were split and one fraction was preserved in 5% formalin, and the other was drained and then frozen at −20°C for later isotope (δ13C, δ15N) analyses. Additional zooplankton samples (0–50 m depth) were collected at night to increase the number of organisms for isotopic analyses. Once in the laboratory, all samples for isotope analyses were transferred to −80°C. Seawater samples along the water column were also collected using 5 L Niskin bottles from six depths (0, 5, 10, 20, 50, and 100 m) both during the day and at night. Seawater samples (0.4 L) were filtered (Whatman GF/F) for chlorophyll-a determinations and 0.5–1.0 L were also filtered (GFF 0.7 μm pore precombusted filters) for stable isotopes in particulate organic matter (POM) analyses. All samples were stored on board initially at −20°C and then transferred to −80°C in the laboratory.
Laboratory work. In the laboratory, formalin-preserved zooplankton samples were identified as functional group levels and counted. Munida gregaria were classified as either zoea or pelagic M. gregaria (megalopa, juveniles). All zooplankton groups were measured (cephalothorax length and total length). Biomass (wet weight) measurements were conducted on larger-sized zooplankton groups on a high-resolution analytical scale (±0.000001 g), and then individual wet weight (WW) values were transformed into dry weight (DW) following Wiebe (1988):
For the other smaller-sized or less abundant zooplankton groups, biomass was estimated by calculating their individual volume and then utilizing Wiebe (1988) displaced volume (DV) to dry weight (DW) relationship:
From the frozen zooplankton samples, the largest crustaceans (M. gregaria pelagic, E. vallentini, Sergestes arcticus, mysid larvae) and the most abundant zooplankton groups (copepods and ostracods) were sorted for isotope analyses and for total carbon and nitrogen determinations. The number of individuals in each crustacean group used for the isotope analyses was estimated from the weight per sample as described in the Stable Isotope Facility web page at the University of California at Davis.1 Only the abdominal muscular tissue from E. vallentini and pelagic M. gregaria were used for isotopic analyses. The 13C and 15N isotopes in the samples were determined using an Elementar Vario EL Cube elemental analyzer (Elementar Analysensysteme GmbH, Hanau, Germany) interfaced with an Isoprime VisION IRMS (Elementar UK Ltd, Cheadle, United Kingdom) isotope ratio mass spectrometer, or a PDZ Europa ANCA-GSL elemental analyzer interfaced to a PDZ Europa 20-20 isotope ratio mass spectrometer (Sercon Ltd., Cheshire, United Kingdom). The final delta values are expressed relative to the international standards Vienna Pee Dee Belemnite and Air for carbon and nitrogen, respectively.
Data analyses. The trophic positions of the different groups were estimated based on isotopic 15N enrichment, following Vander Zanden (1997):
where δ15Norganism is the isotopic value measured, 3.4 is the average de δ15N enrichment per trophic level (Post, 2002), and δ15N base is usually the δ15N from organisms that show an herbivorous feeding mode. In this study, we used the same approach used by Riccialdelli et al. (2017), which is the mean δ15N value reported for all benthic filter feeders (Notochthamalus scabrosus δ15N = 12.7‰; Aulacomya atra δ15N = 12.0‰, Brachydontes purpuratus δ15N = 11.3‰; Mytilus chilensis δ15N = 11.9‰) from the same region (BC), assuming they were all herbivorous. λ Represents the reference value (TL = 2) in the food web.
Bayesian standard ellipse areas (SEAB) were also calculated to determine the isotopic niche width (Jackson et al., 2011) of the largest body size and most abundant crustacean groups (pelagic Munida gregaria, E. vallentini adults, Sergestes arcticus, ostracods, and copepods). To estimate niche width, we used the estimate function in the Stable Isotope Bayesian Ellipses in R (SIBER) v3.6.1. The program first estimates a Bayesian standard ellipse that describes the data in a δ13C–δ15N space for each group and can also perform an arithmetic correction for a small number (n < 30) of samples (standard ellipse area corrected, SEAC) (Young et al., 2017).
We calculated the relative importance of terrestrial particulate organic carbon (POC) in the diet of all crustacean major groups by applying a two-source mixing model (Bianchi, 2007):
where δ13C sample is the mean isotopic composition of each crustacean group, δ13Cmarine is the marine end-member, and δ13Cterrestrial is the terrestrial end-member. Particulate organic matter has been used as an endmember in some trophic studies (Post, 2002; Benstead et al., 2006; Vargas et al., 2011). However, POM is a mixture of terrestrial and marine organic matter in this zone (Riccialdelli et al., 2017); hence, our POM isotope values could not be used as marine end members [in fact, our 13C POM = −24.51 ± 0.53‰ were very similar with C2 plants (δ13C = −23.9‰) reported by Riccialdelli et al., 2017]. Accordingly, we utilized δ13C values from C2 plants as terrestrial end members and macroalgae values (chlorophyta, rhodophyta, phaeophyta) as marine end members, all reported by Riccialdelli et al. (2017) for the BC.
Statistical analyses included a Kolmogorov-Smirnov test with the Lilliefors correction to explore whether the zooplankton group abundance and the δ13C and δ15N data series followed normal distributions. Since none of the series followed this distribution (p < 0.05), Mann-Whitney U-tests non-parametric tests were then utilized to look for differences in total abundance between locations in the larger crustacean groups, and median tests were utilized to determine whether statistical differences existed between day and night samples. Potential differences in vertical distributions during the day and night among sampled depths were evaluated using the interaction term (day/night × depths) in PERMANOVA. Lineal regressions were utilized to assess the potential relationships between the isotope composition and C/N with M. gregaria body length (cephalothorax length). The stable isotope composition in M. gregaria was also compared among the depths of collection using Kruskal-Wallis non-parametric tests. All analyses were carried out in R (v. 3.5.1) using the Lillie.test function (to test for normality) and lm (linear models).
Results
Hydrographic Conditions
The hydrographic vertical section of Yendegaia Bay shows a well mixed 0–200 m deep water column with conservative temperature values varying between 6.5 and 7.3°C and absolute salinity values fluctuating between 31 and 32 g/kg (Figure 2). A very narrow surface layer (<5 m) was observed, with low salinity (30.5–31.5 g/kg), low temperature (<6.5°C), and maximum fluorescence and turbidity values (0.2 mg/m3 fluorescence and turbidity values of approximately 1.5 NTU). The highest temperature (7–7.3°C) occurred deeper than 100 m near the bottom at the inner part of the fjord, where salinity did not reach higher than 32 g/kg.
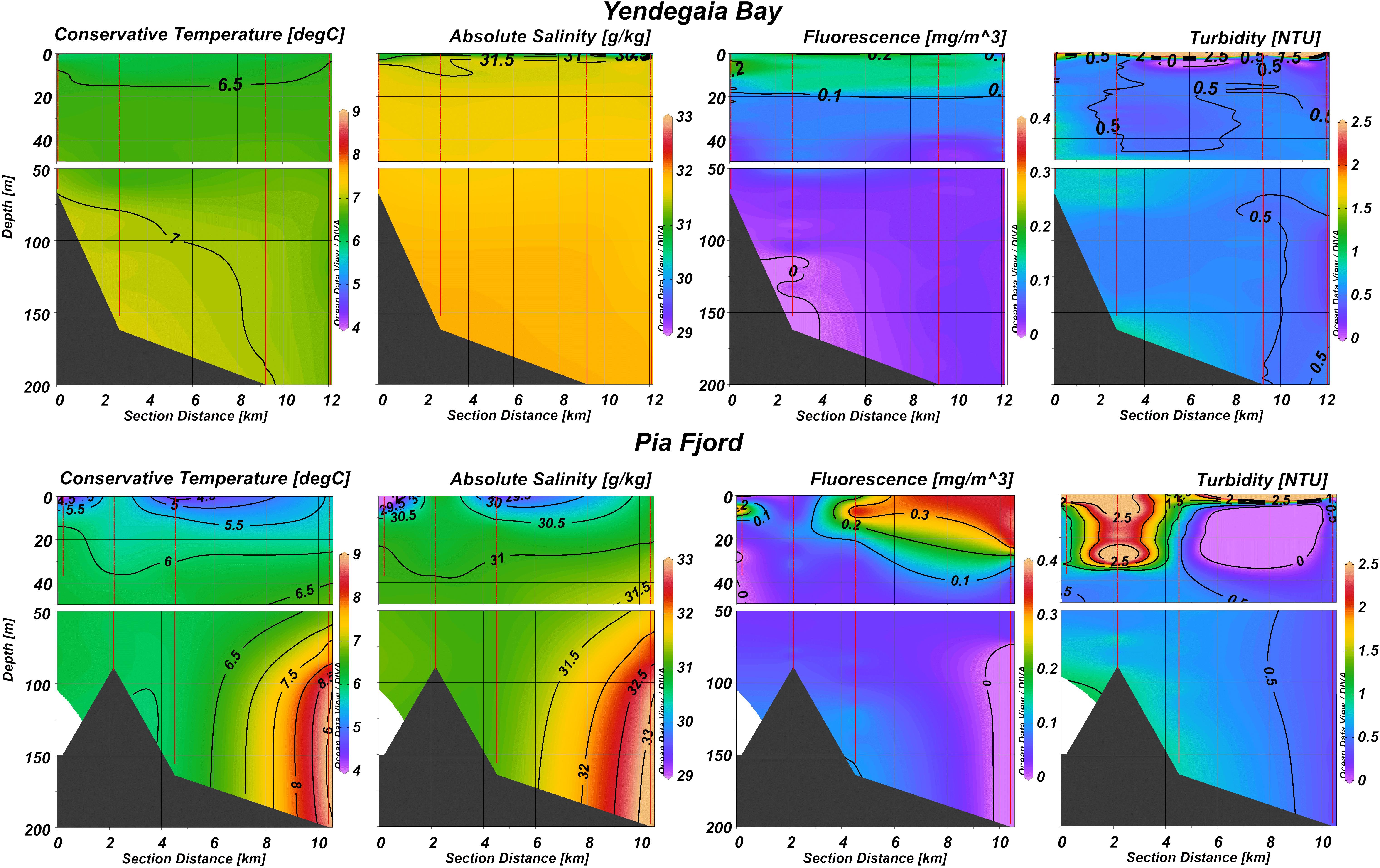
Figure 2. Vertical section of hydrographic characteristics (conservative temperature, absolute salinity, fluorescence and turbidity) along the sampling track located from the center of Yendegaia bay and Pia fjord toward the Beagle channel.
In the Pia fjord, the water column was less mixed in terms of temperature and salinity, particularly at the outer side of the fjord (Figure 2). Compared with the Yendegaia bay, surface water was colder (<5°C), less saline (29.5–30.5 g/kg), and showed higher fluorescence values (>0.3 mg/m3) down to 25 m deep toward the mouth of the fjord, and higher turbidity values (2.5 NTU) toward the head of the fjord. Deeper than 100 m, toward the entrance of the fjord, the seawater was warmer (>8°C) and saltier (>32 g/kg), evidencing a mixture with saltier water entering the BC from the oceanic area.
Zooplankton Groups Abundance and Vertical Distribution
A similar number of zooplankton functional groups were identified in the plankton samples: 20 in Yendegaia Bay and 22 in Pia Fjord (Table 1). The mean abundance of individuals collected in all groups were very low in both locations (mean per group: Yendegaia bay = 0.44 ± 1.37 ind./m3; Pia fjord = 0.70 ± 2.05 ind./m3), and the 5 numerically most abundant groups (copepods, ostracods, E. vallentini juveniles, E. vallentini adults, pelagic M. gregaria) were the same. Copepods and ostracods represented approximately 90% of all individuals collected in each zone (Figure 3), and both showed higher abundances in Pia fjord (copepods Mann-Whitney U: 27; p = 0.03; ostracods: Mann-Whitney U: 27; p = 0.03). E. vallentini juveniles and adults together accounted for ca. in 3.1% of all individuals in all groups in Yendegaia Bay and 1.5% Pia fjord and showed no difference in abundance between locations (E. vallentini juveniles: Mann-Whitney U: 45; p = 0.346; E. vallentini adults: Mann-Whitney U: 58,5; p = 0.923). Zoea and pelagic M. gregaria stage together represented ca. 1% in Yendegaia Bay and 0.2% in Pia Fjord, and both showed higher abundance in Yendegaia Bay (Zoea M. gregaria: Mann-Whitney U: 18; p = 0.004; juvenile M. gregaria: Mann-Whitney U: 25; p = 0.021), while the other comparatively large body sized zooplankton groups, Sergestes sp. and mysid larvae, reached low abundance representing less than 1% in each location and both showed a low frequency (≤50%) in all zooplankton profiles and no difference in abundance between locations (Sergestes sp.: Mann-Whitney U: 46; p = 0.381; mysis larvae: Mann-Whitney U: 49; p = 0.497).
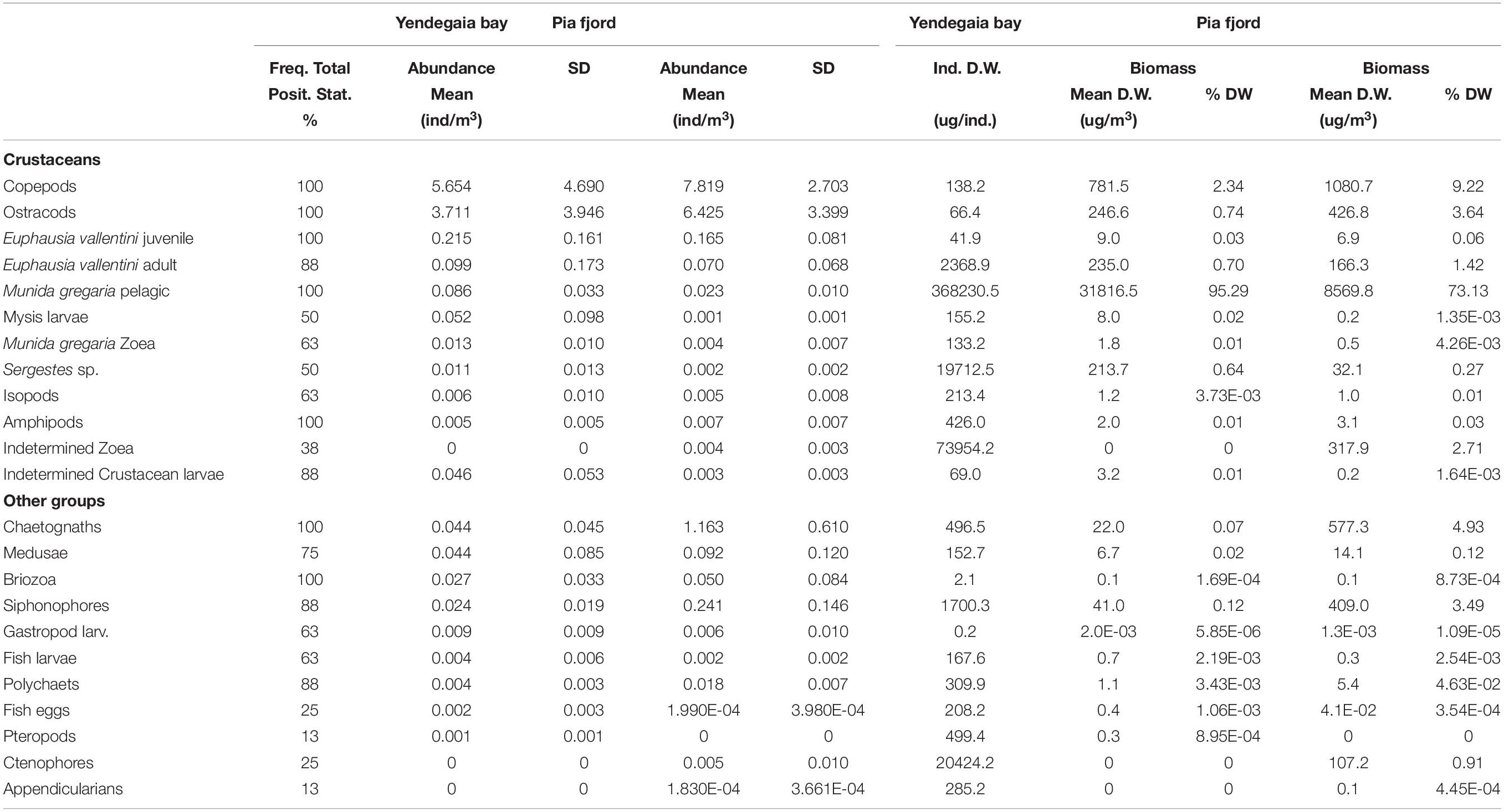
Table 1. Abundance (ind./m3) and biomass (g/m3 dry weight, DW) of the different zooplankton groups collected during the winter cruise 2017 to Yendegaia bay and Pia fjord in the Beagle channel, indicating the percentage of positive stations (Posit. Stat.), mean abundance (±standard deviation), individual dry weights, total biomass (±standard deviation) and percentage of the total biomass corresponding to each group at each location.
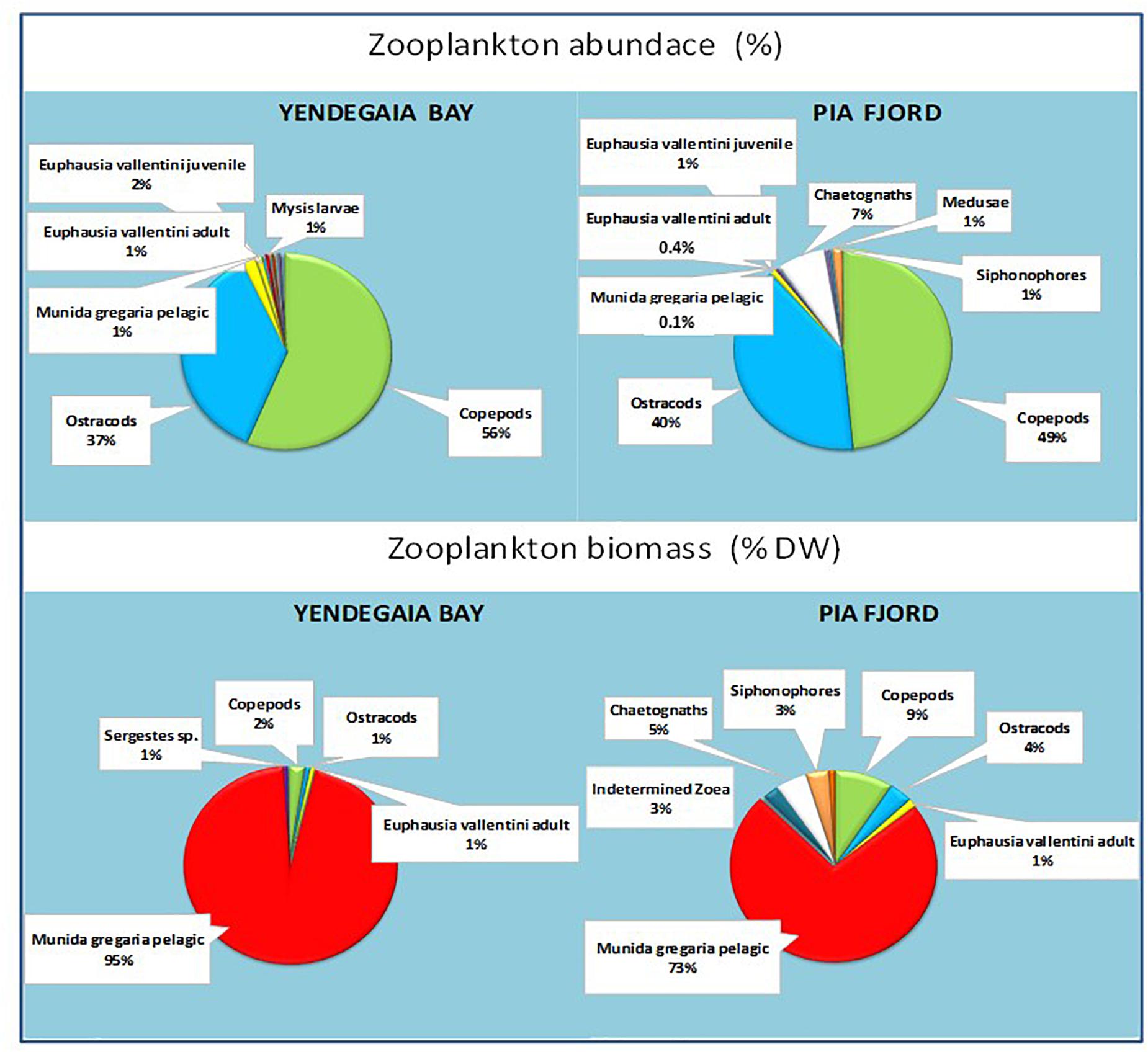
Figure 3. Zooplankton abundance (%) and biomass (dry weight; %) of the most abundant groups in Yendegaia bay and Pia fjord in winter 2017. Pelagic Munida gregaria, copepods and ostracods are highlighted because of their relative importance both numerically and in terms of biomass.
Among the larger-body-sized zooplankton groups, the pelagic M. gregaria stage was the largest (>40 mm total length, TL) and the highest total dry weight (DW) biomass, accounting for more than 70% of the total zooplankton biomass (95% in Yendegaia Bay; 73% in Pia Fjord; Figure 3). In individual size basis, one 2.9 cm total length body size pelagic M. gregaria (0.37 g/ind DW) was equivalent to 2663 2.5-mm prosome length copepods (0.000138 g/ind.DW) (Table 1), with copepods being the most abundant zooplankton group and, after the pelagic M. gregaria stage, the following crustacean group in terms of biomass contribution.
The five more abundant zooplankton groups occurred in both the day and night samplings (Figure 4). Of these, the zoea and pelagic M. gregaria stage did not show differences in abundance between day and night (Median tests, p > 0.05; Table 2). These results contrast with the other groups (E. vallentini juveniles and adults, copepods, ostracods, Sergestes sp.), which showed higher abundance along the water column during the night than during the day (Median tests, p < 0.05, Table 2), suggesting that the incorporation of individuals from deeper layers (>100 m) at night was associated with vertical migrations.
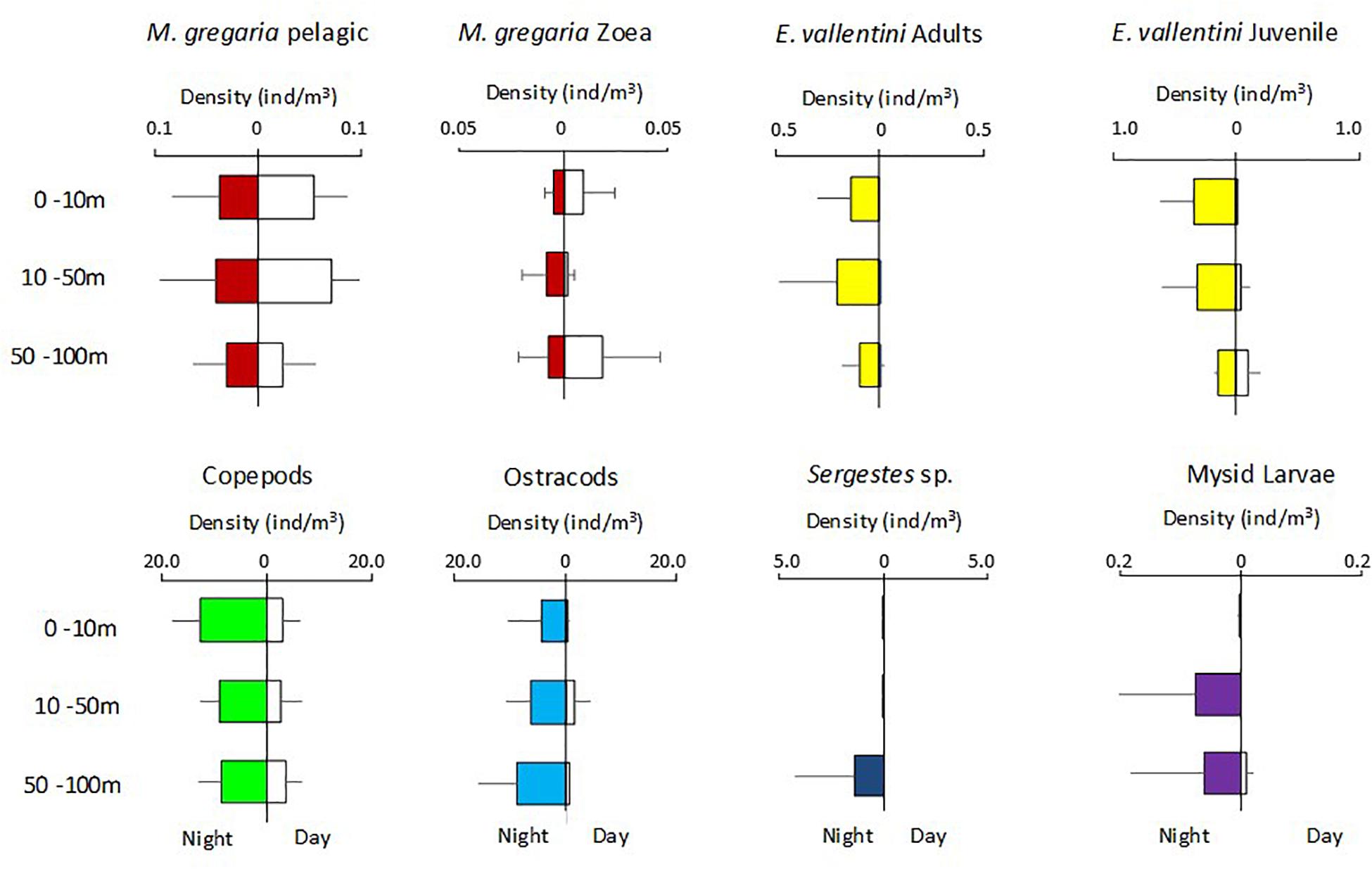
Figure 4. Vertical distribution of the most abundant zooplankton crustacean groups at day (white) and night time (colored) collected at both locations (pooled data). Abundance are expressed as density of individuals at each sampled stratum (ind./m3).
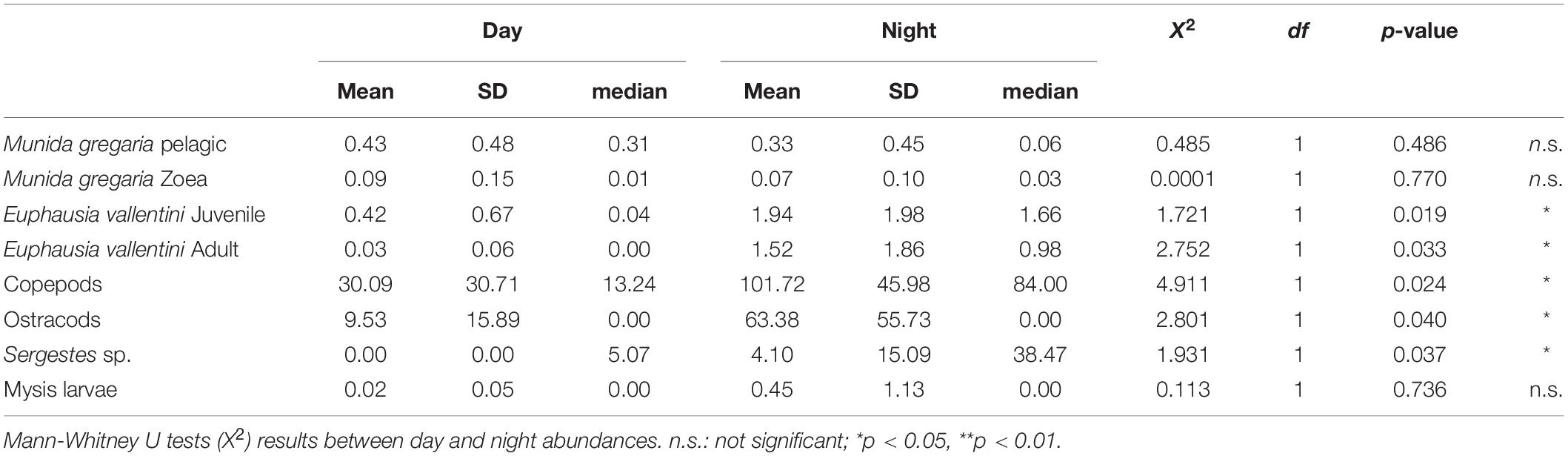
Table 2. Mean abundance (ind/10 m3), standard deviations (SD) and medians of the main pelagic crustacean functional groups at day and night time.
Pelagic M. gregaria stage occurred mostly in the 0–50 m depth range and showed no statistical difference in vertical distribution between day and night (PERMANOVA, depths × day/night: F = 1.786; p = 0.718). M. gregaria zoea stages were more abundant at the deepest layer (50–100 m) during the day. At night, they dispersed along the water column, increasing their mean abundance tenfold in the intermediate layer (zoea d/n 10–50m = 0.1). Copepods, a group that showed a high number of individuals (>2 ind/m3) at the three layers sampled day and at night, showed significant day-night differences in vertical distribution (PERMANOVA, depths × day/night; F = 0.128; p = 0.048). The other groups, with minimum abundance at the three layers sampled during the daytime, did not show statistical differences (p > 0.05) in day-night distributions, probably because their daytime aggregations were deeper than our samplings. At night, though, most of the large-sized crustacean groups studied here shared the 0–50 m shallower depth range at night, except for Sergestes sp., which occurred mostly at the deepest layer (50–100 m) at night (Figure 4).
Stable Isotopes, Trophic Positions, and Isotopic Niche Width in Crustacean Groups
The range of mean δ13C values for all crustacean groups was narrow (1.7‰), with the highest value for adult E. vallentini (−19.50 ± 1.22‰) and the lowest for mysid larvae (−21.27 ± 1.07‰) (Table 3 and Figure 5A). The M. gregaria pelagic stage showed the lowest δ15N values (12.43 ± 0.74‰) and Sergestes sp. the highest (15.32 ± 1.11‰). The C/N ratio revealed a clear distinction between M. gregaria and E. vallentini lower values (M. gregaria = 4.08 ± 1.43‰; E. vallentini 4.73 ± 1.68‰) compared with the higher values of the other four groups (range: 7.02–9.97‰) (Table 3).

Table 3. Stable isotopes in the major pelagic crustaceans collected in the winter 2017 cruise to the Beagle channel, indicating number of samples analyzed (n), δ13C (±standard deviation), δ15N (±standard deviation), C/N ratio, trophic position (TP), corrected Stable Isotope Bayesian ellipse area (niche width; SEAc) and terrestrial organic carbon content (TOC) in the different groups.
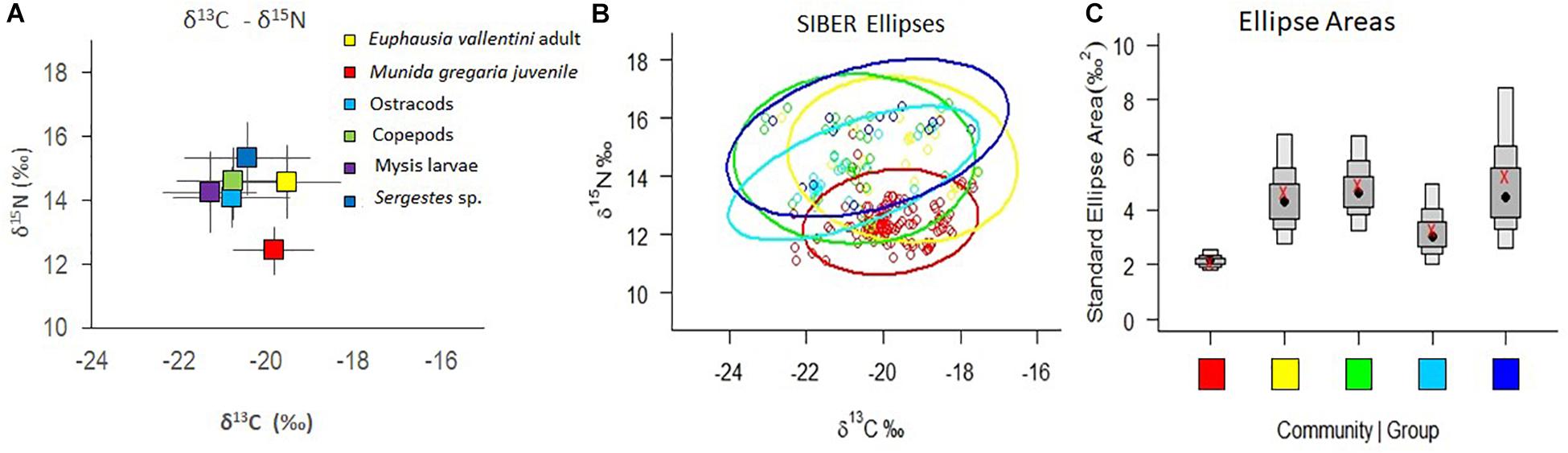
Figure 5. Stable isotopes δ13C and δ15N values of the most abundant crustacean zooplankton groups in both locations (pooled data) sampled in winter 2017. (A) δ13C and δ15N plot of mean values (bars are standard deviations). (B) Stable Isotope Bayesian ellipses showing the width of the isotopic niche of each of the crustacean groups. (C) Box and whiskers plot of ellipse areas of each group showing the median, 50, 75, and 95% limits, and corrected mean value (x). Mysid larvae were not included in ellipses nor box and whiskers diagrams because of the low number of replicates available (<5 samples).
The Trophic positions (TP) estimated for all groups showed that Sergestes sp. had the highest position (TP = 3), followed by E. vallentini adults and copepods (TP = 2.8), mysid larvae (TP = 2.7), ostracods (TP = 2.6), and the pelagic M. gregaria stage with the lowest trophic position (TP = 2.1). The isotopic ellipses diagram shows a great degree of overlap in isotopic niche width among most groups except pelagic M. gregaria that occurred at the bottom of the ellipses diagram and that overlapped only partially with the other groups (Figure 5B). While pelagic M. gregaria showed the narrowest ellipse areas, Sergestes sp. showed the widest area (Figure 5C). Adult E. vallentini, and copepods, in turn showed similar ellipse areas, as revealed by the mean SEAc values, and ostracods showed values between the later groups and pelagic M. gregaria (Table 3). The relative importance of terrestrial organic carbon (TOC) in the diet of all crustaceans, as depicted by the δ13C content, indicates that all groups analyzed were deeply dependent on terrestrial carbon, with percentages of terrestrial contribution varying from 41% in E. vallentini to 54% in mysid larvae (Table 3). Within the crustacean assemblage, pelagic M. gregaria (43%) and Sergestes sp. (42%) showed a lower proportion of terrestrial organic carbon in their tissues, compared with ostracods and copepods (50%).
Munida gregaria Stable Isotopes at Different Body Lengths and at Different Depths
Pelagic M. gregaria showed a wide range of body lengths (11.9–40.8 mm total length, TL), particularly at the surface layer where the size range was wider than that at other depths (Figure 6). Along the water column, however, their length distribution was dominated by a narrow body size range (15–20 mm TL) at all depths.
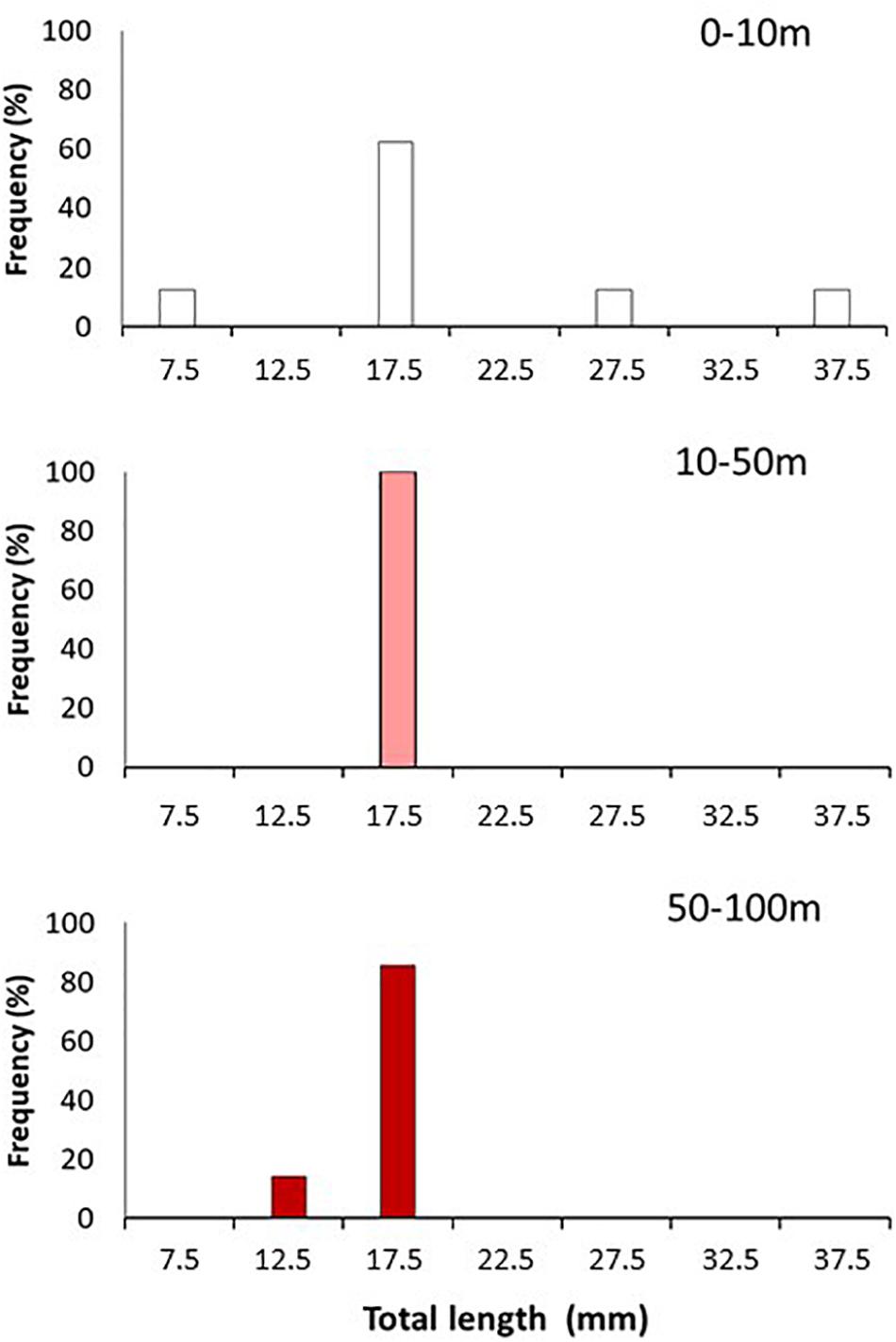
Figure 6. Total length frequency distribution (%) of pelagic Munida gregaria collected at the three sampled strata (0–10 m, 10–50 m and 50–100 m depth) in both locations (pooled data) in winter 2017.
Over the length range of pelagic M. gregaria utilized for isotope and C/N analyses (6.9–21.5 cephalothorax length, CL), very small isotopic changes were observed in δ13C (linear model, n = 44, p = 0.0104) and δ15N (lineal model, n = 44, p = 0.0213) with cephalothorax length but with very low coefficients of determination (R2 = 0.14 and R2 = 0.12 for δ13C and δ15N, respectively) (Figure 7). No significant relationship was observed between C/N and pelagic M. gregaria cephalothorax length (linear model, n = 44, p = 0.3408, R2 = 0.02).
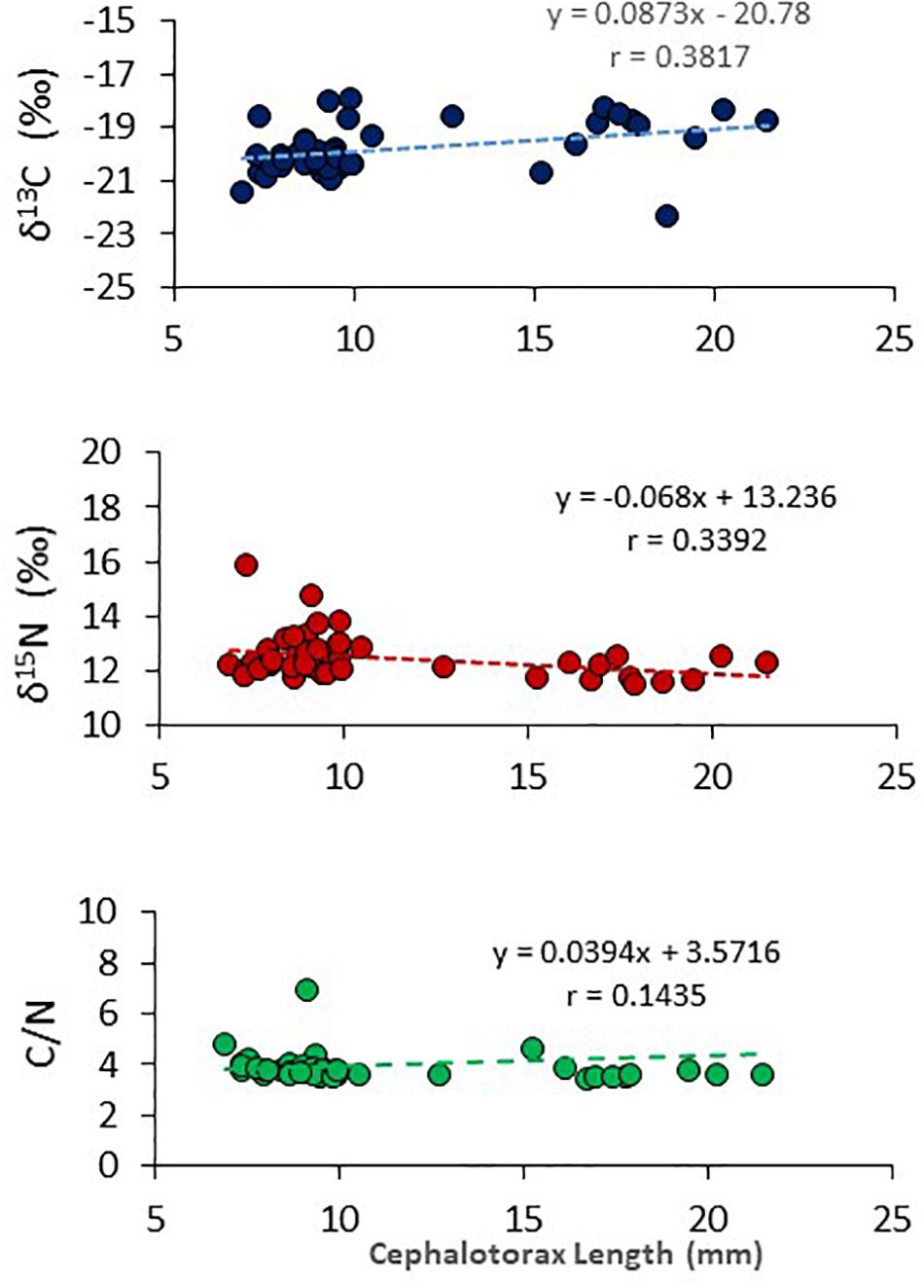
Figure 7. Stable isotope values (δ13C, upper panel; δ15N, middle panel) and C/N ratio (bottom panel) in different body length (cephalothorax length, mm) pelagic Munida gregaria collected in winter 2107. Linear model regressions are also shown in both panels.
Along the water column, δ13C in particulate matter (POM) ranged from −25.6 to −23.6‰ and did not show increases in mean values from the surface layer (δ13C POM0–10 m = −24.8 ± 0.4‰) to deeper layers (δ13C POM100 m = −24.0 ± 0.4‰). No significant difference was observed in the mean isotopic values of δ13C in pelagic M. gregaria (Kruskal-Wallis, p = 0.237), suggesting that they eat terrestrial plant carbon and phytoplankton/macroalga-derived carbon throughout the column. However, a slight increase in δ15N in pelagic M gregaria toward higher depths was observed (Figure 8), which coincided with the slightly higher trophic positions toward deeper waters (TP0–10m = 2.1, TP10–50m = 2.13, TP50–100m = 2.17). The δ15N difference among depths was not statistically significant when the mean values were compared (Kruskal-Wallis, p = 0.126). Means C/N values among depths were not significantly different (Kruskal-Wallis, p = 0.444).
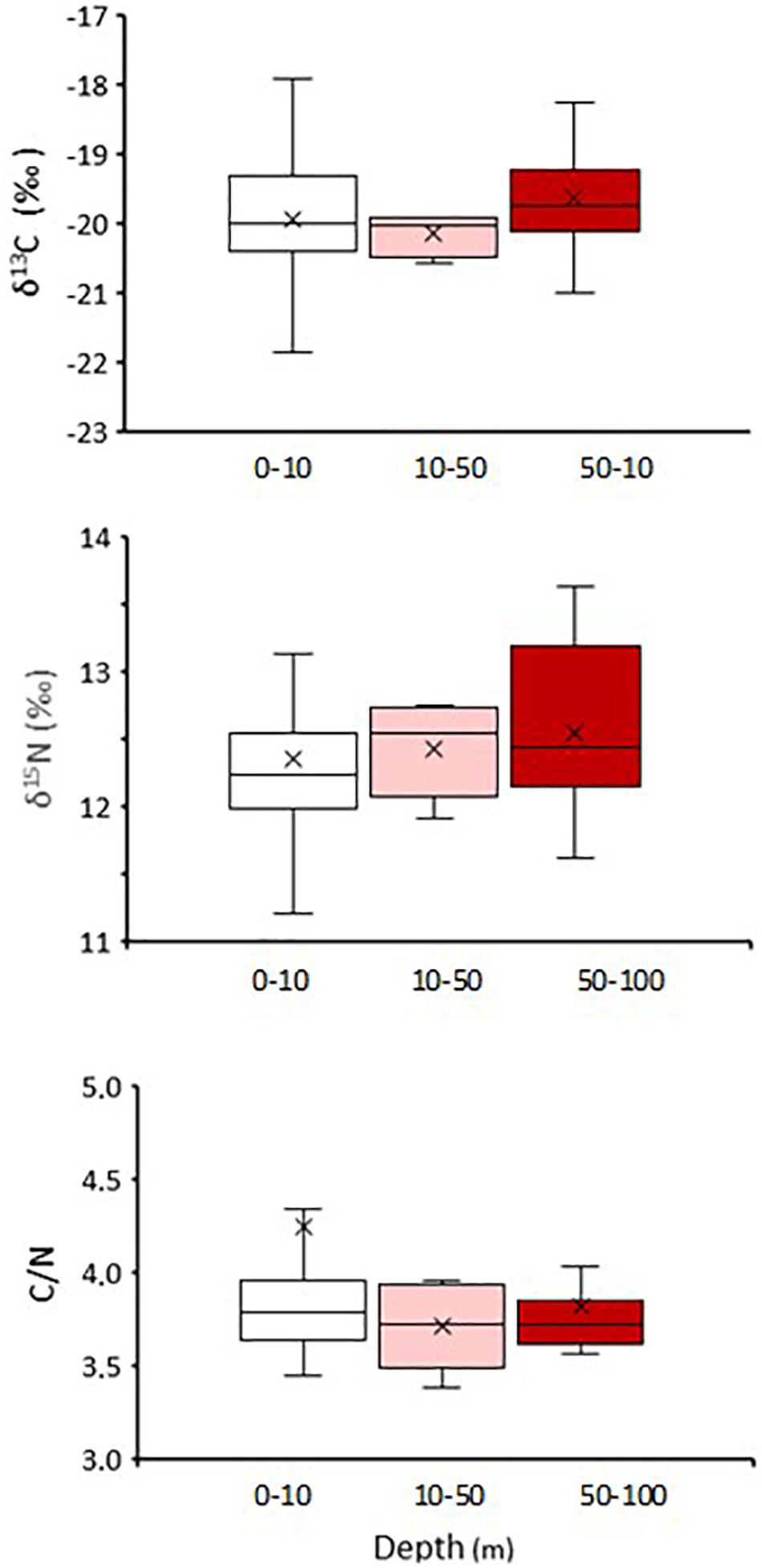
Figure 8. Stable isotope (δ13C, upper panel; δ15N middle panel) and C/N ratio (bottom panel) in abdominal muscle of pelagic Munida gregaria at the three depth of collection (0–10 m, 10–50 m and 50–100 m depth).
Discussion
The general objective of this study was to assess how the dominant chitinous zooplankton groups in the BC survive during the winter low production season in this high latitude region. Our results show that the zooplankton community was similar in two fjords connected to the BC, where a single pelagic group dominated in terms of biomass (pelagic Munida gregaria), and that differences in vertical distribution occurred between most principal crustacean zooplankton groups and M. gregaria, which was partially explained by apparent shifts in vertical distribution between day and night in the former groups. All groups showed a high dependence on terrestrially derived organic matter, as revealed by their δ13C values. However, the isotopic composition, TP estimates, and isotopic niche widths of the groups clearly separated pelagic M. gregaria from the others, presenting the lowest δ15N and highest δ13C values, and a narrower niche width. This group was dominated by a single body size class along the 0–100 m water column and remained during the day and at night, mostly in the upper layers (0–50 m), apparently benefiting from the higher phytoplankton concentrations (compared to deeper layers), as revealed by their higher δ13C values and low TP. In contrast, the other groups changed in vertical distribution between day and night or remained deeper in the water column during the day, and showed slightly higher δ15N values, higher TP, and lower δ13C values, probably feeding on a microzooplankton-terrestrial POM mixture at deeper layers. Trophic flows through microbial loops from heterotrophic nanoflagellates and ciliates toward crustacean zooplankton have been reported during the winter season in Nord-Patagonian fjords (González et al., 2010).
The zooplankton community characteristics in both locations were similar despite local hydrographic differences between fjords exerted by different water exchange and freshening effects given by marine and land-terminating glaciers. The Pia fjord, closer to the Pacific Ocean connection than Yendegaia Bay, showed lower temperature and salinity at the surface, a large input of oceanic water at depth, and a more stratified water column (Figure 2). The chlorophyll a concentrations were very low (range: 0.03–0.28 mg/m3) in both locations reaching values between one and two orders of magnitude lower than those observed in the same area in spring (Giesecke et al., 2019). The mesozooplankton community in this fjord, with abundances of organisms also one to two orders of magnitude lower than in spring (Giesecke et al., 2019), included a relatively similar number of taxa (20–22 functional groups) and a similar total number of organisms per group compared with Yendegaia Bay, but showed some gelatinous organisms not present in Yendegaia (apendicularia and ctenophores). The five numerically most abundant groups (copepods, ostracods, E. vallentini juveniles, E. vallentini adults, and pelagic M. gregaria) were the same in both locations, copepods and ostracods representing 90% of the zooplankton abundance in each zone, and were the most frequent and abundant mesozooplankton groups reported previously in the BC but in other seasons (Biancalana et al., 2007; Aguirre et al., 2012; Giesecke et al., 2019). However, when transformed into biomass estimates (D/W), the relative importance of most zooplankton groups in our winter study significantly decreased, and pelagic M. gregaria became the most important group with biomass representing from ∼70–90% of the total zooplankton biomass in both locations (Figure 3).
The vertical distributions of the larger-sized crustacean groups differed between day and night, except for pelagic M. gregaria. While most groups showed an increase in abundance at all sampled layers during the night, apparently after a diel residence of most individuals deeper than our maximum depth sampled (e.g., E. vallentini 150 m diurnal mean weight depth; Hamame and Antezana, 2010), suggesting a diel vertical migration, pelagic M. gregaria remained mostly in the shallower 0–50 m layer in both periods. Diel vertical migration has been reported for most of these groups in other locations, including the Patagonian region, but in other seasons (Castro et al., 1993; Hamame and Antezana, 2010; Valle-Levinson et al., 2014). In M. gregaria zoea diel changes in vertical distribution have been documented mainly for the earlier stages in northern Patagonia and, depending on the location, in synchrony with semidiurnal tides (Castro et al., 2011, 2019). The vertical distribution of the pelagic M. gregaria in our study coincides with previous observations of post-larval stages in the Baker basin (47°S) or the inner sea of Chiloé (42°S), where their position has been associated with the halocline apparently to favor horizontal transport (Castro et al., 2019; Meerhoff et al., 2019). This also coincides with acoustic reports of juvenile M. gregaria in the BC where it reached maximum depths deeper than 80 m but that situated this species denser aggregations between 21 and 28 m depth (Diez et al., 2016, 2018). In neither of these studies in the BC further north in the northern and central Chilean Patagonian zone (Castro et al., 2019; Meerhoff et al., 2019), diel vertical migrations were documented for the post-larval (juvenile or megalopa) stages.
Stable isotope results show a narrow range in δ13C (−21.2 to −19.5‰) and δ15N (12.4–15.4‰) mean values across all groups. These low δ13C values highlight the importance of terrestrial organic matter in sustaining the winter trophic web in the area (TOC = 41–54%). A relatively similar range of mean δ13C values (−20.8 ± 3.8‰) was reported for zooplankton in spring in the same BC by Riccialdelli et al. (2017). However, among all groups in our study, pelagic M. gregaria (−19.81 ± 0.93‰) along with E. vallentini (−19.50 ± 1.22‰) showed the highest δ13C values suggesting that marine particulate organic matter might also be an important part of their diet, at least in these two taxa. The δ15N values and isotopic ellipses indicate that most groups superpose their isotopic niche, except for pelagic M. gregaria, which showed the lowest δ15N values (12.43 ± 0.74‰) and TP (2.1) and a narrower isotopic niche, suggesting some differences in feeding resources compared to the other crustacean groups. The δ15N values determined were within the range reported for this species: 10–12‰ in northern Patagonia (Meerhoff et al., 2019) and 14.1 ± 0.7‰ in the same BC in spring (Riccialdelli et al., 2017). Interestingly, δ13C and δ15N stable isotope values were similar at different M. gregaria body lengths in our study.
Slight differences in trends were observed in δ13C and δ15N values in pelagic M. gregaria toward deeper layers. In δ13C, no clear differences were observed among depths, suggesting that a mixture of terrestrial and marine POM was ingested along the water column. Increasing values were observed in δ15N toward higher depths, which resulted in a slightly higher TP deeper in the water column. Dissimilar trends in δ13C and δ15N in pelagic M. gregaria tissues with depth apparently resulted from a combination of processes that include, first, terrestrial organic carbon entering at the sea surface plus phytoplankton, and secondly, in addition to the sinking of organic matter from those sources in shallow waters, the potential addition of sinking fecal pellets, microzooplankton, or zooplankton debris into the pelagic M. gregaria diets at higher depths, which would result in similar δ13C values but higher δ15N and higher trophic levels deeper in the water column. Sediment trap samples deployed at 100 and 200 m depth from the study area (BC) showed a high amount of fecal material throughout the year (González, unpublished data). These sequential processes highlight the role of terrestrial and marine phytoplankton at the surface layers and of the sinking matter and microzooplankton at deeper layers, which determine a similar but significant terrestrial organic matter signal along the pelagic M. gregaria vertical distribution but with different trophic positions at different depths. The higher δ15N and TP values of the other zooplankton or deeper resident crustacean groups coincide with the higher δ15N and TP of those pelagic M. gregaria present in deeper waters, supporting this hypothesis, which suggests that feeding in deeper environments may modify the TP of organisms and hence induce separate feeding patterns among groups.
This study aimed to assess whether the more abundant and largest-body-sized crustacean pelagic groups in the BC develop differential feeding strategies conducive to favoring their coexistence during the austral low production winter season. Our overall results showed that two major groups could be identified in terms of feeding strategies: the first group included a mixture of some of the most abundant holoplankton organisms (>90% numerically) plus zoea M. gregaria stages, that reside deeper in the water column and move vertically during the day. The holoplanktonic group may feed on sinking organic matter or microzooplankton at depth and hence showed slightly higher trophic levels than the other group. The second group contained a single species (pelagic M. gregaria), which constitutes the dominant pelagic group in terms of biomass (>70%), resides mostly at the shallower 50 m of the water column, rich in terrestrially derived organic matter and some marine phytoplankton that constitutes its food, and which infringes them a lower trophic position and a slightly different terrestrial organic carbon signature in their tissues, compared with organisms in the other zooplankton groups. Pelagic M. gregaria did not change in vertical distribution during the day (at least not detected by our sampling methods) and so overlapped its distribution only with those vertical migrating zooplankton groups at night, reducing the potential competence for food resources. The different (i) vertical distributions, (ii) trophic level food sources, and (iii) slightly different original organic carbon sources, seem to be part of the adaptive feeding strategy that facilitates the coexistence of different pelagic crustaceans under harsh feeding winter conditions at this high latitude region.
Data Availability Statement
The original contributions presented in the study are included in the article/supplementary material, further inquiries can be directed to the corresponding author/s.
Author Contributions
LC (zooplankton), HG (POM), and JG-V (hydrographic data) collaborated in the samples collection at sea and data analyses at the laboratory. PB also worked on the zooplankton analyses and stable isotope samples preparation. All authors participated in the manuscript writing.
Funding
This study was supported by the National Agency for Scientific Research and Development (ANID) through the project FONDAP-IDEAL Center (grant number 15150003). Additional funding was provided by the COPAS Sur Austral Center (ANID PIA APOYO CCTE AFB170006), which partially financed zooplankton analyses and LC and PB.
Conflict of Interest
The authors declare that the research was conducted in the absence of any commercial or financial relationships that could be construed as a potential conflict of interest.
Acknowledgments
The authors acknowledge the collaboration of E. Menschel and J. Martin and the Forrest vessel crew for their help at sea and S. Soto for assistance with statistical analyses.
Footnotes
References
Aguirre, G. E., Capitanio, F. L., Lovrich, G. A., and Esnal, G. B. (2012). Seasonal variability of metazooplankton in coastal sub-Antarctic waters (Beagle Channel). Mar. Biol. Res. 8, 341–353. doi: 10.1080/17451000.2011.627922
Almandoz, G. O., Hernando, M. P., Ferreyra, G. A., Schloss, I. R., and Ferrario, M. E. (2011). Seasonal phytoplankton dynamics in extreme southern South America (Beagle Channel, Argentina). J. Sea Res. 66, 47–57. doi: 10.1016/j.seares.2011.03.005
Benstead, J. P., March, J. G., Fry, B., Ewel, K. C., and Pringle, C. M. (2006). Testing isosource: Stable isotope analysis of a tropical fishery with diverse organic matter sources. Ecology 87, 326–333. doi: 10.1890/05-0721
Bernal, A., Castro, L. R., and Costalago, D. (2020). Elucidating trophic pathways of the most abundant fish larvae in Northern Patagonia using δ13C and δ15N isotopes. Mar. Ecol. Progr. Ser. 650, 253–267. doi: 10.3354/meps13374
Betti, F., Castro, L. R., Bavestrello, G., Enrichetti, F., and Daneri, G. (2020). Distribution, abundance and ecological requirements of the benthic phase of Munida gregaria (Anomura; Munididae) in the Puyuhuapi Fjord (Chilean Patagonia). Reg. Stud. Mar. Sci. 40:101534. doi: 10.1016/j.rsma.2020.101534
Biancalana, F., Barría, M. S., and Hoffmeyer, M. S. (2007). Micro and mesozooplankton composition during winter in Ushuaia and Golondrina bays (Beagle channel, Argentina). Braz. J. Oceanogr. 55, 83–95. doi: 10.1590/s1679-87592007000200002
Casassa, G., Wendt, J., Wendt, A., Lopez, P., Schuler, T., Maas, H., et al. (2010). Outburst floods of glacial lakes in Patagonia: is there an increasing trend? Geophys. Res. Abstr. 12:12821.
Castro, L. R., Bernal, P. A., and Troncoso, V. A. (1993). Coastal intrusion of copepods: mechanisms and consequences in the population biology of Rhincalanus nasutus. J. Plankton Res. 15, 501–515. doi: 10.1093/plankt/15.5.501
Castro, L. R., Cáceres, M. A., Silva, N., Muñoz, M. I., León, R., Landaeta, M. F., et al. (2011). Short-term variations in mesozooplankton, ichthyoplankton, and nutrients associated with semi-diurnal tides in a Patagonian Gulf. Cont. Shelf Res. 31, 282–292. doi: 10.1016/j.csr.2010.09.005
Castro, L. R., Soto, S., and González-Saldías, F. (2019). Ontogenetic and short-term fluctuations in the residence depth of young pelagic stages of Munida gregaria in different zones of northern Patagonia. Progr. Oceanogr. 174, 173–184. doi: 10.1016/j.pocean.2018.09.018
Climate Change (2007). Synthesis Report. Contribution of Working Groups I, II and III to the Fourth Assessment Report of the Intergovernmental Panel on Climate Change, eds Core Writing Team, R. K. Pachauri, and A. Reisinger (Geneva, Switzerland: IPCC), 104.
Climate Change (2013). The Physical Science Basis. Contribution of Working Group I to the Fifth Assessment Report of the Intergovernmental Panel on Climate Change, eds T. F. Stocker, D. Qin, G.-K. Tignor, M. Plattner, S. K. Allen, J. Boschung, A. Nauels, Y. Xia, V. Bex, and P. M. Midgley (Cambridge, United Kingdom and New York, NY, USA: Cambridge University Press), 1535.
Diez, M. J., Cabreira, A. G., Madirolas, A., and Lovrich, G. A. (2016). Hydroacoustical evidence of the expansion of pelagic swarms of Munida gregaria (Decapoda, Munididae) within the Beagle Channel and the Argentine Patagonian Shelf, and its relationship with habitat features. J. Sea Res. 114, 1–12. doi: 10.1016/j.seares.2016.04.004
Diez, M. J., Cabreira, A. G., Madirolas, A., Martin, J. N., Scioscia, G., Schiavini, A., et al. (2018). Winter is cool: spatio-temporal patterns of squat lobster Munida gregaria and the Fuegian sprat Sprattus fuegensis in a sub-Antarctic estuarine environment. Polar Biol. 41, 2591–2605. doi: 10.1007/s00300-018-2394-2
Diez, M. J., Pérez-Barros, P., Romero, M. C., Scioscia, G. F., Tapella, F., Cabreira, A. G., et al. (2012). Pelagic swarms and beach strandings of the squat lobster Munida gregaria (Anomura: Munididae) in the Beagle Channel. Tierra del Fuego. Polar Biol. 35, 973–983. doi: 10.1007/s00300-011-1144-5
Dussaillant, A., Benito, G., Buytaert, W., Carling, P., Meier, C., and Espinoza, F. (2009). Repeated glacial-lake outburst floods in Patagonia: an increasing hazard? Nat. Hazards 54, 469–481. doi: 10.1007/s11069-009-9479-8
Giesecke, R., Höfer, J., Vallejosa, T., and González, H. E. (2019). Death in southern Patagonian fjords: copepod community structure and mortality in land- and marine-terminating glacier-fjord systems. Progr. Oceanogr. 174, 162–172. doi: 10.1016/j.pocean.2018.10.011
González, H. E., Calderón, M. J., Castro, L., Clement, A., Cuevas, L. A., Daneri, G., et al. (2010). Primary production and its fate in the pelagic food web of the Reloncaví Fjord and plankton dynamics of the Interior Sea of Chiloé, Northern Patagonia, Chile. Mar. Ecol. Progr. Ser. 402, 13–30. doi: 10.3354/meps08360
González, H. E., Castro, L., Daneri, G., Iriarte, J. L., Silva, N., Vargas, C. A., et al. (2011). Seasonal plankton variability in Chilean Patagonia Fjords: carbon flow through the pelagic food web of the Aysen Fjord and plankton dynamics in the Moraleda Channel basin. Cont. Shelf Res. 31, 225–243. doi: 10.1016/j.csr.2010.08.010
González, H. E., Graeve, M., Kattner, G., Silva, N., Castro, L., Iriarte, J. L., et al. (2016). Carbon flow through the pelagic food web in southern Chilean Patagonia: relevance of Euphausia vallentini as key species. Mar. Ecol. Progr. Ser. 557, 91–110. doi: 10.3354/meps11826
Hamame, M., and Antezana, T. (2010). Migración vertical diurno-nocturna y alimentación de Euphausia vallentini en los fiordos del sur de Chile. Deep Sea Res. II 57, 642–650.
Jackson, A., Inger, R., Parnell, A. C., and Bearshop, S. (2011). Comparing isotopic niche widths among and within communities: SIBER–Stable Isotope Bayesian ellipses in R. J. Anim. Ecol. 80, 595–602. doi: 10.1111/j.1365-2656.2011.01806.x
Laiz-Carrion, R., Quintanilla, J. M., Mercado, J. M., and García, A. (2011). Combined study of daily growth variability and nitrogen-carbon isotopic signature analysis of schooling Sardina pilchardus larvae. J. Fish Biol. 79, 896–914. doi: 10.1111/j.1095-8649.2011.03048.x
León, R., Castro, L. R., and Cáceres, M. (2008). Dispersal of Munida gregaria (Decapoda: Galatheidae) larvae in Patagonian channels of southern Chile. ICES J. Mar. Sci. 65, 1131–1143. doi: 10.1093/icesjms/fsn093
Lindsay, D. J., Minagawa, M., Mitani, I., and Kawaguchi, K. (1998). Trophic shift in the Japanese anchovy Engraulis japonicus in its early life history stages as detected by stable isotope ratios in Sagami Bay, Central Japan. Fish. Sci. 64, 403–410. doi: 10.2331/fishsci.64.403
Malzahn, A. M., and Boersma, M. (2009). Trophic flexibility in larvae of two fish species (lesser sand eel, Ammodites marinus and dab, Limanda limanda) Advances in the early life history study of fish. Sci. Mar. 73, 131–139. doi: 10.3989/scimar.2009.73s1131
Marín, V. H., Tironi, A., Parades, M. A., and Contreras, M. (2013). Modeling suspended solids in a Northern Chilean Patagonia glacier-fed fjord: GLOF scenarios under climate change conditions. Ecol. Model. 264, 7–16. doi: 10.1016/j.ecolmodel.2012.06.017
Meerhoff, E., Castro, L., and Tapia, F. (2013). Influence of freshwater discharges and tides on the abundance and distribution of larval and juvenile Munida gregaria in the Baker river estuary, Chilean Patagonia. Cont. Shelf Res. 61, 1–11. doi: 10.1016/j.csr.2013.04.025
Meerhoff, E., Castro, L. R., Tapia, F. J., and Pérez-Santos, I. (2019). Hydrographic and biological impacts of a Glacial Lake Outburst Flood (GLOF) in a Patagonian Fjord. Estuaries Coasts 42, 132–143. doi: 10.1007/s12237-018-0449-9
Meerhoff, E., Tapia, F., and Castro, L. R. (2014). Spatial structure of the meroplankton community along a Patagonian fjord – the role of changing freshwater inputs. Prog. Oceanogr. 129, 125–135. doi: 10.1016/j.pocean.2014.05.015x
Montecinos, S., Castro, L. R., and Neira, S. (2016). Stable isotope (δ13C and δ15N) and trophic position of Patagonian sprat (Sprattus fuegensis) from the Northern Chilean Patagonia. Fish. Res. 179, 139–147. doi: 10.1016/j.fishres.2016.02.014
Mujica, A., Nava, M. L., Saavedra, M., Pereira, J., and Vargas, A. (2013). Distribution and abundance of larvae of Munida gregaria (Fabricius, 1793), Sergestes arcticus Kroyer, 1855 and Neotrypea uncinata (H. Milne-Edwards, 1837) between Puerto Montt (41°30’S) and Laguna San Rafael (46°30’S), southern Chile. Lat. Am. J. Aquat. Res. 41, 828–838. doi: 10.3856/vol41-issue5-fulltext-3
Overman, N. C., and Parrish, D. L. (2001). Stable isotope composition of walleye: δ15N accumulation with age and area-specific differences in δ13C. Can. J. Fish. Aquat. Sci. 58, 1253–1260. doi: 10.1139/f01-072
Pérez-Barros, P., Romero, M. C., Calcagno, J. A., and Lovrich, G. A. (2010). Similar feeding habits of two morphs of Munida gregaria (Decapoda) evidence the lack of trophic polymorphism. Rev. Biol. Mar. Oceanog. 45, 461–470. doi: 10.4067/s0718-19572010000300011
Pérez-Barros, P., Calcagno, J. A., and Lovrich, G. A. (2011). Absence of a prezygotic behavioral barrier to gene flow between the two sympatric morphs of the squat lobster Munida gregaria (Fabricius, 1793) (Decapoda: Anomura: Galatheidae). Helgoland Mar. Res. 65, 513–523. doi: 10.1007/s10152-010-0240-1
Pérez-Barros, P., D’Amato, M. E., Guzman, N. V., and Lovrich, G. A. (2008). Taxonomic status of two South American sympatric squat lobsters, Munida gregaria and Munida subrugosa (Crustacea: Decapoda: Galatheidae), challenged by DNA sequence information. Biol. J. Linn. Soc. Lond. 94, 421–434. doi: 10.1111/j.1095-8312.2008.00987.x
Post, D. M. (2002). Using stable isotopes to estimate trophic position: models, methods and assumptions. Ecology 83, 703–718. doi: 10.1890/0012-9658(2002)083[0703:usitet]2.0.co;2
RGI (2017). Randolph Glacier Inventory–A Dataset of Global Glacier Outlines: Version 6.0: Technical Report. Boulder, CO: Global Land Ice Measurements from Space, doi: 10.7265/N5-RGI-60
Riccialdelli, L., Newsome, S. D., Fogel, M. L., and Fernández, D. A. (2017). Trophic interactions and food web structure of a sub Antarctic marine food web in the beagle channel: Bahía Lapataia, Argentina. Polar Biol. 40, 807–821. doi: 10.1007/s00300-016-2007-x
Rivera, A., Benham, T., Casassa, G., Bamber, J., and Dowdeswell, A. J. (2007). Ice elevation and areal changes of glaciers from the Northern Patagonia Ice field, Chile. Glob. Planet. Chang. 59, 126–137. doi: 10.1016/j.gloplacha.2006.11.037
Rosenberg, P., and Palma, S. (2003). Cladocerans in Patagonian fjords and channels, between the Penas Gulf and Strait of Magellan. Invest. Mar. Valparaíso 31, 15–24.
Ross, L., Pérez-Santos, I., Parady, B., Castro, L., Valle-Levinson, A., and Schneider, W. (2020). Glacial lake outburst flood (GLOF) events and water response in a Patagonian fjord. Water Quality and Ecosystems. Water 12:248. doi: 10.3390/w12010248
Tanaka, H., Takasuka, A., Aoki, I., and Ohshimo, S. (2008). Geographical variations in the trophic ecology of Japanese anchovy, Engraulis japonicus, inferred from carbon and nitrogen stable isotope ratios. Mar. Biol. 154, 557–568. doi: 10.1007/s00227-008-0949-4
Tapella, F., Romero, M. C., Lovrich, G. A., and Chizzini, A. (2002). “Life history of the galatheid crab Munida subrugosa in sub Antarctic waters of the Beagle Channel, Argentina,” in Crabs in Cold Water Regions: Biology, Management and Economics, eds A. J. Paul, E. G. Dawe, R. Elner, G. S. Jamieson, G. H. Kruse, R. S. Otto, et al. (Fairbanks, AK: University of Alaska Sea Grant), 115–133.
Valladares, J., Fennel, W., and Morozov, E. G. (2011). Announcement: replacement of EOS-80 with the International Thermodynamic Equation of Seawater—2010 (TEOS-10). Deep Sea Res. 58:978. doi: 10.1016/j.dsr.2011.07.005
Valle-Levinson, A., Castro, L. R., Pizarro, O., and Cáceres, M. (2014). Twilight vertical migrations of zooplankton in a Chilean fjord. Progr. Oceanogr. 129, 114–124. doi: 10.1016/j.pocean.2014.03.008
Vander Zanden, M. J., and Rasmussen, J. B. (1999). Primary consumer delta C-13 and delta N-15 and the trophic position of aquatic consumers. Ecology 80, 1395–1404. doi: 10.1890/0012-9658(1999)080[1395:pccana]2.0.co;2
Vander Zanden, M. J., and Rasmussen, J. B. (2001). Variation in delta N-15 and delta C-13 trophic fractionation: implications for aquatic food web studies. Limnol. Oceanogr. 46, 2061–2066. doi: 10.4319/lo.2001.46.8.2061
Vander Zanden, M. J. (1997). Comparing trophic position of freshwater fish calculated using stable nitrogen ratios (δ15N) and literature dietary data. Can. J. Fish. Aquat. Sci. 54, 1142–1158. doi: 10.1139/f97-016
Vargas, C. A., Martínez, R. A., San Martin, V., Aguayo, M., Silva, N., and Torres, R. (2011). Allochthonous subsidies of organic matter across a lake-river-fjord landscape in the Chilean Patagonia: implications for marine zooplankton in inner fjord areas. Cont. Shelf Res. 31, 187–201. doi: 10.1016/j.csr.2010.06.016
Vinuesa, J. H., and Varisco, M. (2007). Trophic ecology of the lobster krill Munida gregaria in San Jorge Gulf, Argentina. Investig. Mar. 35, 25–34.
Wiebe, P. H. (1988). Functional regression equations for zooplankton displacement volume, wet weight, dry weight, and carbon: a correction. Fish. Bull. (U. S.) 86, 833–835.
Young, H., Nigro, K., Mccauley, D. J., Ballance, L. T., Oleson, E. M., and Baumann-Pickering, S. (2017). Limited trophic partitioning among sympatric delphinids off a tropical oceanic atoll. Plos One 12:e0181526. doi: 10.1371/journal.pone.0181526
Keywords: Beagle channel, stable isotopes, Munida gregaria, Euphausia vallentini, zooplankton, Patagonia
Citation: Castro LR, González HE, Garcés-Vargas J and Barrientos P (2021) Separate Feeding Between the Pelagic Stage of the Squat Lobster Munida gregaria and the Larger Sized Zooplankton Crustacean Groups in the Beagle Channel as Revealed by Stable Isotopes. Front. Mar. Sci. 8:635190. doi: 10.3389/fmars.2021.635190
Received: 30 November 2020; Accepted: 10 May 2021;
Published: 09 June 2021.
Edited by:
Eduardo Joel Quiroga Jamett, Catholic University of Valparaíso, ChileReviewed by:
Mauricio F. Landaeta, Universidad de Valparaiso, ChileKusum Komal Karati, Centre for Marine Living Resources and Ecology (CMLRE), India
Copyright © 2021 Castro, González, Garcés-Vargas and Barrientos. This is an open-access article distributed under the terms of the Creative Commons Attribution License (CC BY). The use, distribution or reproduction in other forums is permitted, provided the original author(s) and the copyright owner(s) are credited and that the original publication in this journal is cited, in accordance with accepted academic practice. No use, distribution or reproduction is permitted which does not comply with these terms.
*Correspondence: Leonardo R. Castro, bGVjYXN0cm9Ab2NlYW5vZ3JhZmlhLnVkZWMuY2w=