- 1Faculty of Fisheries, Kagoshima University, Kagoshima, Japan
- 2Graduate School of Fisheries, Kagoshima University, Kagoshima, Japan
- 3EPHE-UPVD-CNRS, USR 3278 CRIOBE BP 1013, PSL Research University, Moorea, French Polynesia
The euryhaline copepod Pseudodiaptomus inopinus play important roles in coastal waters as vectors of docosahexanoic (DHA) and eicosapentaenoic (EPA) acids for larval fish. While DHA and EPA in polar lipids (PLs) are more effective for fish larval development than non-polar lipid forms (NLs), there is little knowledge how much these lipids are accumulated in copepods from microalgae and are effective for early development of fish larvae. We report PLs fatty acid profiles of P. inopinus fed DHA-poor microalgae and evaluate its significance as a food source for larvae development of Pagrus major, compared with DHA-enriched rotifers. Copepods and rotifers were fed a mixed diet of three algal species (Phaeodactylum tricornutum, Tisochrysis lutea, and Pavlova lutheri), in addition of DHA-supplemented Super Fresh Chlorella (SFC) for rotifers. Compared with SFC, the algal mixture had higher EPA but lower DHA. Copepods had higher DHA and EPA in total lipids than rotifers fed each diet. Copepod PLs were specifically enriched with DHA and their contents were higher than both rotifers. On the other hand, PLs EPA contents were comparable between preys, indicating that copepods selectively fortified the PLs. Fish culture experiment showed that larvae fed copepods had higher growth than those fed SFC-enriched rotifers. Principal component analysis for each organism fatty acid composition emphasized trophic modification of DHA by copepods toward larval fish. This study highlighted that P. inopinus contribute to enhanced growth of coastal larval fish by efficiently transferring DHA via copepod fatty acid metabolism.
Introduction
Based on the critical period hypothesis (Hjort, 1914), food availability during initial feeding periods is known as a crucial factor for early development of fish larvae and its recruitment. As match-mismatch hypothesis (Cushing, 1990) states, prey quantity well contributes to the recruitment in fish stocks, while laboratory experiments, especially those for aquaculture application, has pointed the importance of prey quality (i.e., nutrition) to explain fish stocks. Marine finfish lack the ability to synthesize n-3 highly unsaturated fatty acids (n-3 HUFAs), such as docosahexaenoic acid (DHA) and eicosapentaenoic acid (EPA), and therefore must supplement these essential fatty acids from diets for their growth and survival (Tocher, 2003). Indeed, larval fish reared under sufficient amounts of diets further enhance their growth, survival, and development when the diets are rich in n-3 HUFA (especially DHA; Watanabe et al., 1989; Nćss et al., 1995; Shields et al., 1999; Evjemo et al., 2003; Karlsen et al., 2015). Larvae inhabiting in the coastal areas, also exhibit higher growth rate when the ambient zooplankton contain higher n-3 HUFAs (Paulsen et al., 2014). These facts suggest the necessity to investigate how fatty acids are transferred toward larval fish for further understanding of fish recruitment.
Coastal and estuarine waters are known as a nursery area for various larval species and support their abundance (Borges et al., 2007). The euryhaline calanoid Pseudodiaptomus inopinus is a very common copepod species in such ecosystems around East Asia, Russia, and the United States (Sakaguchi and Ueda, 2011). The genus Pseudodiaptomus has a tolerance to a broad range of salinity (Beyrend-Dur et al., 2011), and high turbidity (Hwang et al., 2010), so that it is often the most abundant prey for larval fish. This copepod is reported to have high DHA and/or EPA in total lipids (Toledo et al., 1999; Rayner et al., 2015). Thus, P. inopinus with rich fatty acids can play important roles for larval development in coastal ecosystems.
On the other hand, in coastal and estuary waters, the dominant primary producers of n-3 HUFA are often diatoms (Kleppel, 1993; Trigueros and Orive, 2001; Ansotegui et al., 2003). They lack DHA despite having specifically high EPA (Kates and Volcani, 1966; Volkman et al., 1989). Indeed, DHA was relatively low in spring blooms where diatoms are dominated (Sargent et al., 1985). On the diatom–copepod–larval fish food chain, little is known how P. inopinus contributes to early development of larval fish by transferring DHA. To deal with this issue, fatty acid profiles, particularly polar lipids (PLs) of copepods, require further investigation (Olsen et al., 2014). For larval fish, dietary n-3 HUFA distributed to the PLs can be more efficiently absorbed than those distributed to the non-polar lipids (NLs; Salhi et al., 1999), resulting in enhanced larval growth and survival (Gisbert et al., 2005; Wold et al., 2009). However, the distribution of DHA and EPA in P. inopinus PLs is unknown.
Our objective is to evaluate the fatty acid profiles of PLs in P. inopinus cultivated using DHA-poor microalgae and their significance in early development of fish larvae. Rather than copepods, successful larviculture is well established using artificially DHA-enriched Brachionus rotifers as the diet (Koven et al., 1990; Castell et al., 2003; Thépot et al., 2016). In this study, we used such rotifers fed on a commercial DHA enrichment diet as a standard for evaluating copepod fatty acids. We also cultured P. major larvae with copepods, and compared the performances with those cultured using enriched rotifers. P. major is a widely distributed fish in coastal waters of Japan (Shoji et al., 2007), and is of commercial importance to Japan, Korea, and China (Hossain et al., 2016). We then discuss fatty acid trophic transfer in coastal ecosystems, and the significance of copepods on early development of coastal fish larvae.
Materials and Methods
Cultivation of Rotifers
The L-type rotifer Brachionus plicatilis species complex (Obama strain) was used as control diet for larval fish. As an experimental control, artificially enriched rotifers were prepared by feeding Chlorella vulgaris containing DHA (Super Fresh Chlorella V-12, SFC; Chlorella industry, Tokyo, Japan). SFC is produced by heterotrophic cultivation of C. vulgaris with fish oil DHA as a carbon source (Hayashi et al., 2001). Following the procedure of rotifer enrichment described by Waqalevu et al. (2018), SFC was fed to rotifers at 46 × 103 cells per rotifer per a day. Seawater for the culture medium was filtered through 100 μm cartridge filters (Micro-Cilia; Roki Techno Co. Ltd., Japan); water temperature was maintained at 25°C and salinity at 17‰. Taurine, an essential amino acid for red sea bream larvae (Chen et al., 2004), was also enriched in rotifers using Aquaplus ET (Marubeni Nisshin Feed Co, Ltd., Japan) following manufacturer instructions (60 mg L–1). The enriched rotifers were prepared daily during larval rearing experiment (for 20 days) and harvested to directly supply to larvae. In addition, for fatty acid analysis, the rotifers were directly harvested from three randomly-selected cultures, washed with distilled water, and then freeze-dried and preserved at −80°C until measurement. Starvation periods to make rotifer digestive tracts empty were not set before harvesting them.
To compare the fatty acid composition of rotifers and copepods fed the same diet, the rotifers were also fed same algal mixture as the copepods. Rotifers were fed the mixture daily, cultivated at 20°C water temperature and salinity 17‰. When rotifer density reached 800–1,000 individuals ml–1 they were harvested and preserve for later fatty acid analysis (these rotifers were not used for fish larval diet). Such rotifer samples were prepared in triplicate as SFC-enriched ones.
Cultivation of Microalgae
Copepods were fed on the diatom Phaeodactylum tricornutum, with the haptophytes Pavlova lutheri and Tisochrysis lutea (originated from Isochrysis affinis galbana Tahiti strain; Bendif et al., 2013) as a mixture. We supplemented the diatom with the two haptophytes for mass production of copepod, since monoalgal diatom is well reported to suppress copepod egg productivity and survival (Støttrup and Jensen, 1990; Schipp et al., 1999; Shields et al., 2005; Ohs et al., 2010). The strains of P. tricornutum and P. lutheri were supplied by National Research Institute of Aquaculture, Fisheries Research Agency (Mie, Japan); and T. lutea strain was from the Graduate School of Fisheries and Environmental Science, Nagasaki University, Japan. Each microalga was incubated in 5 L polycarbonate bottles containing a seawater culture medium at 20°C with continuous 0.5 L min–1 aeration and irradiance of 120 μmol photons m–2 s–1. Seawater for the microalgae culture medium was filtered through a GF/F filter (Whatman), diluted to salinity 17‰, autoclaved, and enriched with KW21 medium (Daiichi Seimo Co. Ltd., Japan). Fresh cultures for each species were prepared weekly and maintained for about 1 week. Cells of the three algal species were counted every day using a Thoma hematocytometer (0.1 mm depth; Sunlead Glass Co. Ltd., Tokyo, Japan). Cells were harvested for a diet and fatty acid analysis once they reached the end of logarithmic growth phase or the beginning of stationary phase. Microalgae were directly used as a diet without temporary storage. Three samples of each algal species for fatty acid analysis were twice washed with 0.5 M ammonium formate, and then freeze-dried and preserved at −80°C.
Cultivation of Copepods
Copepods (P. inopinus) were collected using a plankton net (mesh 63 μm) from the surface of Yakugachi River (Kagoshima, Japan), in waters of 19.7°C and salinity 11.5‰. Seawater for the copepod culture medium was pumped from Kagoshima Bay (Kagoshima, Japan), filtered through a graded series of 100, 25, and 10 μm pore sized cartridge filters (Micro-Cilia; Roki Techno Co. Ltd., Japan), before being subjected to ultraviolet irradiance (UVF-1000; Iwaki Co. Ltd., Japan). Copepods were cultivated at 20°C, salinity 17‰, and photoperiod of 12L:12D, initially using 5-L plastic beakers or 50-L polycarbonate tanks as a container. As the copepod population grew, the cultures were transferred to larger polycarbonate tanks (100 and 200 L). Aeration was gently performed via a glass tube at 1 ml min–1 for <50 L of medium, 3 ml min–1 for <100 L of medium, and 6 ml min–1 for <200 L of medium. Microalgae were supplied to copepods as a mixture every 2 days to achieve the following dry mass-based concentrations (Phaeodactylum, 31 μg ml–1, Pavlova, 20 μg ml–1, and Tisochrysis 12 μg ml–1, at the ratio of 5:3:2), where cell concentrations were 400 × 103, 200 × 103, and 400 × 103 cells ml–1, respectively. The medium for copepod culture was fully exchanged every 2–3 days. Generations of the copepods were repeatably incubated for a year before the present experiment, and the species was confirmed to be P. inopinus from the subsamples collected monthly. Except for the 200-L culture of copepods used as a diet for larval fish, three 50-L culture tanks were prepared for copepod fatty acid analysis. Protocols for copepod harvest, wash, and preservation were the same as those for rotifers.
Larval Rearing
Naturally fertilized and spawned red sea bream eggs were obtained from Ogata Suisan Inc. (Amakusa, Japan). The eggs were placed into black 100-L polyethylene tanks at a stocking density of 2,000 eggs per tank. The number of hatched larvae was estimated using a volumetric method described by Kotani et al. (2017), to be 1,900 individuals in each tank. Rearing tanks (100 L) were maintained under 12L:12D light conditions, with water quality monitored twice daily; pH and dissolved oxygen (DO) were measured by portable pH (D-51; Horiba Co. Ltd., Kyoto, Japan) and DO (FDO925; WTW, Bavaria, Germany) meters. Temperature was maintained at 21.8 ± 1.5°C via a 1 kW titanium heater (Nittokizai Co, Ltd., Saitama, Japan); salinity was maintained at 33 ± 1‰. Rearing tank aeration was initially supplied at 15 ml min–1, and this gradually increased with larval growth. The first water exchange occurred 5 days post hatching (dph), after which water was exchanged at 3.5 ml s–1 via a siphoning system.
Larvae were reared until 20 dph following two prey conditions: SFC-enriched rotifers (control), and algal mixture-fed copepods (Figure 1). Both preys were sufficiently fed to fish larvae based on the dry mass, twice a day (at 08:00 and 16:00) from 3 dph until 19 dph. On critical days 3 and 4 post-hatching, to achieve a successful first feeding under copepod supply, the number of nauplii fed to fish larvae was increased to that of rotifers (ca. 5 inds ml–1). Larval rearing was terminated at 20 dph morning before feeding. Twenty fish larvae in each treatment were sampled at 0, 5, 10, 15, and 20 dph. All fish larvae surviving in control and copepod treatments at 20 dph morning were sampled, washed with distilled water, and separately pooled as 1 sample to detect their fatty acids. Larvae for fatty acid analysis were freeze-dried and preserved at −80°C until lipid measurement.
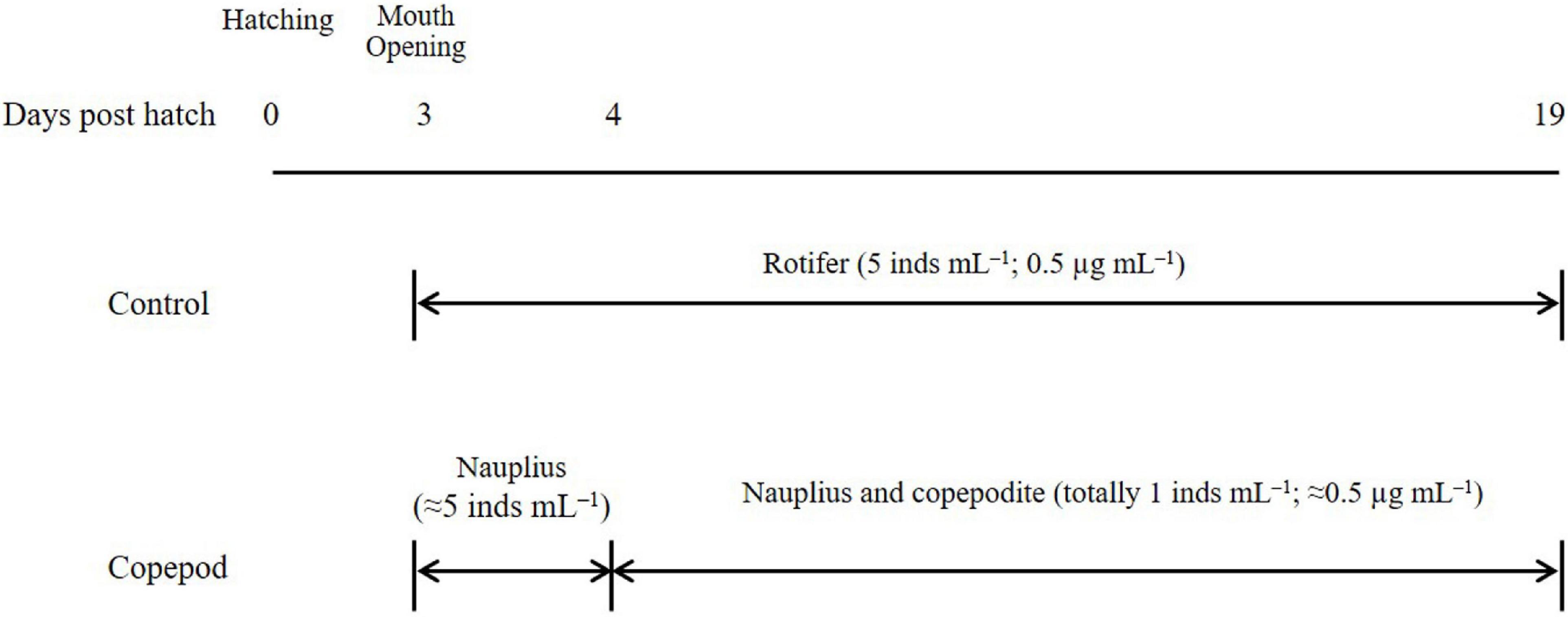
Figure 1. Temporal change in prey items of larval red sea bream Pagrus major in experimental treatments (rotifers and copepods). Values in parentheses present densities based on individual number (ind. mL–1) and dry mass (μg mL–1). Rotifers were prepared by feeding docosahexaenoic acid-supplemented Super Fresh Chlorella (SFC); and the copepods fed algal mixture were used. Rotifer: Brachionus plicatilis sp. complex. Copepod: Pseudodiaptomus inopinus.
Length and Dry Mass of Rotifers and Copepods
Lengths of rotifers and copepods were measured using a micro-ruler under microscopy (SMZ800; Nikon Co, Ltd., Tokyo, Japan) after fixation in acidic Lugol’s solution. The length of rotifer lorica (from crown spines to the lower extremity; Fu et al., 1991) was measured. Four categories of copepods were measured: nauplius (stages I–VI), copepodite (stages I–V), and adult male and female (copepodite stage VI). Adult males were distinguished from other stages by their asymmetrically developed first antennae; females were deemed adult when they possessed egg sacs. Body length was measured for nauplii (n = 40), and prosome length for copepodites (n = 30) and adults (n = 20).
Rotifer dry mass was measured by rinsing samples from three cultures with distilled water, freeze-dried, and weighed using a semi-micro balance (readability of 0.01 mg; GH-202; A and D, Japan). Dry mass of individual copepod was estimated from body length (μm) and prosome length (μm) using the equation adapted by Uye et al. (1983), with a factor (0.45) to convert carbon content to dry mass (Omori and Ikeda, 1984):
Larval Growth and Gut Contents
Fish standard length (SL) was measured after fixation in 10% formalin-seawater solution. Fifteen larvae sampled 2 h after feeding (5, 10, and 15 dph) were used for gut content analysis. In gut contents, rotifers and copepods at each developmental stage (nauplius, copepodite, and adult) were identified by microscopy (SMZ800; Nikon Co, Ltd., Tokyo, Japan) and counted. Total dry mass (μg larva–1) and DHA mass (ng larva–1) of ingested preys were calculated from the number of prey items in larval guts multiplied by their dry mass (μg ind–1) and DHA content (mg g–1).
Fatty Acid Analysis
After homogenization of dried samples, total lipids were extracted following the method of Folch et al. (1957). Extracted lipids were separated into NLs and PLs fractions by column chromatography using a Sep-Pak silica cartridge (Waters, SA, United States; Juaneda and Rocquelin, 1985), with a chloroform-methanol mixture (98:2, v/v) for NLs and methanol for PLs. Methyl-esterification of fatty acids for total lipids, NLs, and PLs, was conducted as described by Matsui et al. (2019). Gas chromatography (GC-2010 Plus; Shimadzu, Kyoto, Japan) equipped with a hydrogen flame ionizing type detector (260°C) and Omegawax capillary column (30 m length, 0.32 mm internal diameter, 0.25 μm film; Supelco, PA, United States) was used for detection of each fatty acid. Each fatty acid species was determined according to the equivalent chain length (ECL), and its proportion was calculated from the peak area. Quantification of fatty acid content was carried out based on the content of non-adecanoic acid (C19:0) analyzed together as an internal standard. Except for microalgae, rotifers, and copepods (n = 3), replicates for lipid analysis are not made for larval fish, due to difficulties in mass production of copepods used for later larviculture. Since fatty acid compositions for Phaeodactylum, Tisochrysis, and Pavlva microalgae have already been well reported (Volkman et al., 1989; Reitan et al., 1994a), each species was analyzed once (n = 1) and added to this study only as supplementary data for algal mixture.
Statistical Analysis
Statistical analysis was undertaken using Sigma-Plot ver 11.0 software (Systat Software Inc., CA, United States) and JMP version 11.0 software (SAS Institute Inc., NC, United States), p = 0.05. The length and dry mass of copepods at different stages were non-parametrically analyzed by Kruskal–Wallis test, followed by Steel–Dwass test, since Shapiro–Wilk’s normality test and Bartlett’s test both failed. A Student’s t-test was conducted to compare total lipid fatty acid species of SFC and algal mixtures. Between rotifer fed on SFC and algal mixture, and copepods, the fatty acid proportions and contents for total lipids, NLs, and PLs were compared by one-way analysis of variance (ANOVA), then subjected to a Tukey pairwise multiple comparison post hoc test if significant differences were observed. An arcsine transformation was performed for proportions of fatty acids in each lipid before being subjected to a one-way ANOVA or Student’s t-test. Larval growth rate was compared between feeding treatments (control and copepod) and between rearing times using two-way ANOVA. Total lipid fatty acid proportions of microalgae, rotifers, copepods, and larvae were analyzed using a principal component analysis (PCA) to characterize fatty acid profiles of each trophic level organism.
Results
Length and Dry Mass of Copepod Stages
The length of copepods increased ontogenetically (Figure 2A). Nauplii showed similar dry mass but larger body length than those for rotifers (p < 0.05; Figure 2B). Length and dry mass were significantly higher in copepodites and adults than nauplii (p < 0.05). The length and dry mass were larger for adult females than adult males (p < 0.05).
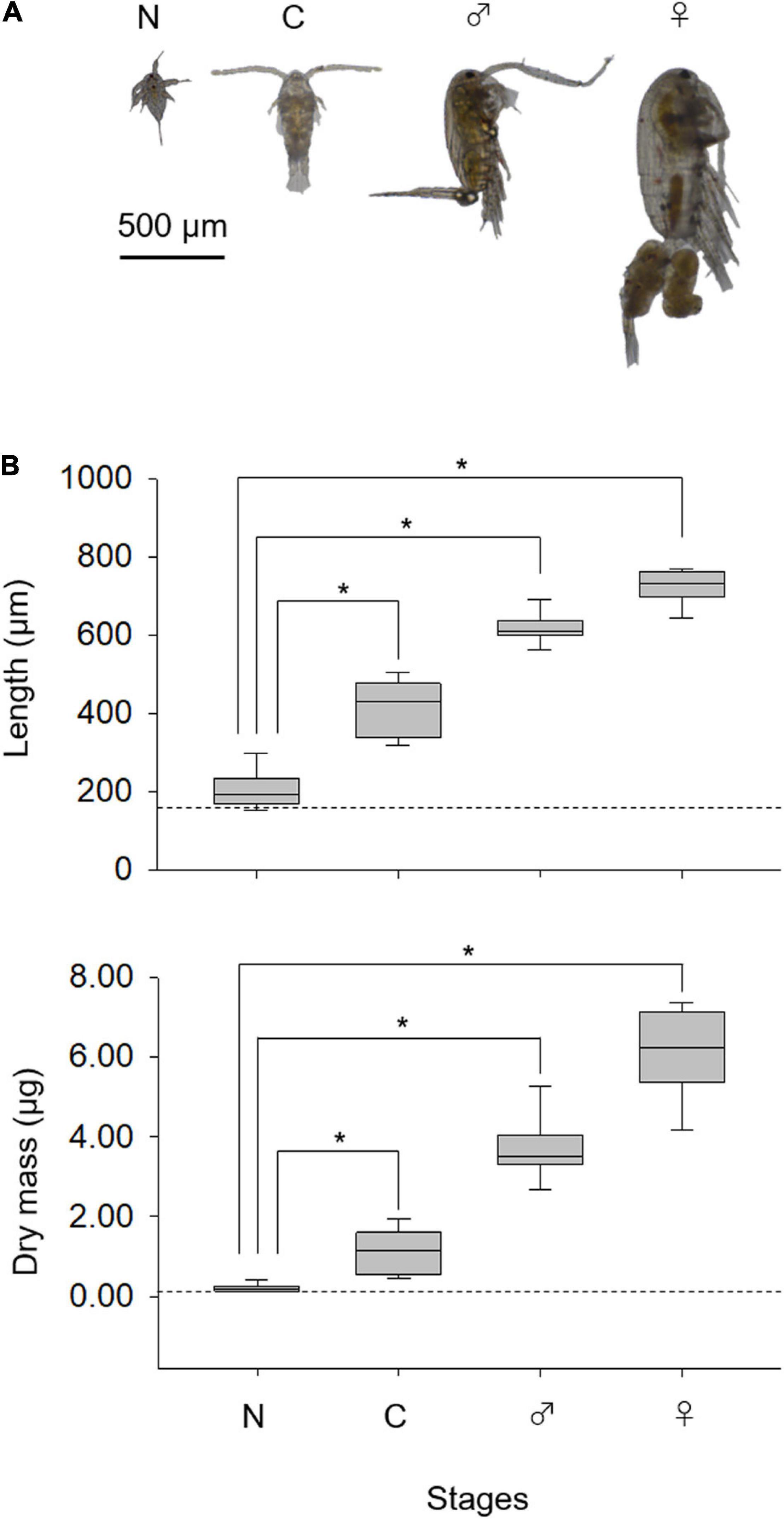
Figure 2. Developmental changes in Pseudodiaptomus inopinus (A) and the lengths and dry mass (B). Nauplius (N), copepodite (C), adult male (♂), and female (♀). Nauplius length is expressed as body length; and copepodite and adult length are expressed as prosome length. Broken lines depict average rotifer lorica length (upper) and dry mass (lower). For box plots, the central line represents the median value, and the box limits are the 25 and 75% quartiles; whiskers cover 5–95% of the data. Asterisks denote significant differences in values (Steel–Dwass test, p < 0.05).
Fatty Acid Profiles of Microalgae
Total lipid fatty acid compositions of the SFC and algal mixture (three species) were determined (Table 1). SFC had peaks of C16:0, C16:2n-4, C18:2n-6, and C18:3n-3 fatty acids, and DHA, and the C18:2n-6 was highly enriched (19.57%). The fatty acid composition of the algal mixture was characterized by peaks of C14:0, C16:1n-7, C16:3n-4, and C18:4n-3 fatty acids, and EPA. These peaks in the algal mixture contributed to total saturated fatty acids (Σ SFA), monounsaturated fatty acids (Σ MUFA), and n-3 polyunsaturated fatty acids (Σ n-3) being higher than those of SFC (p < 0.05). EPA was higher in the algal mixture (p < 0.05) because of the high proportion of fatty acids in P. lutheri (24.44%) and P. tricornutum (23.74%). While the algal mixture also contained DHA, mainly due to the fatty acids supplied from T. lutea (11.85%) and P. lutheri (9.62%), the DHA was lower than SFC (p < 0.05). The sum of the EPA and DHA (n-3 HUFAs) were occupied at approximately 20% of total fatty acids in both SFC and algal mixture. However, there was remarkable difference in DHA/EPA ratio between SFC (2.21) and algal mixture (0.35; p < 0.05). Arachidonic acid (ARA) was scarce (<1%) in all microalgae.
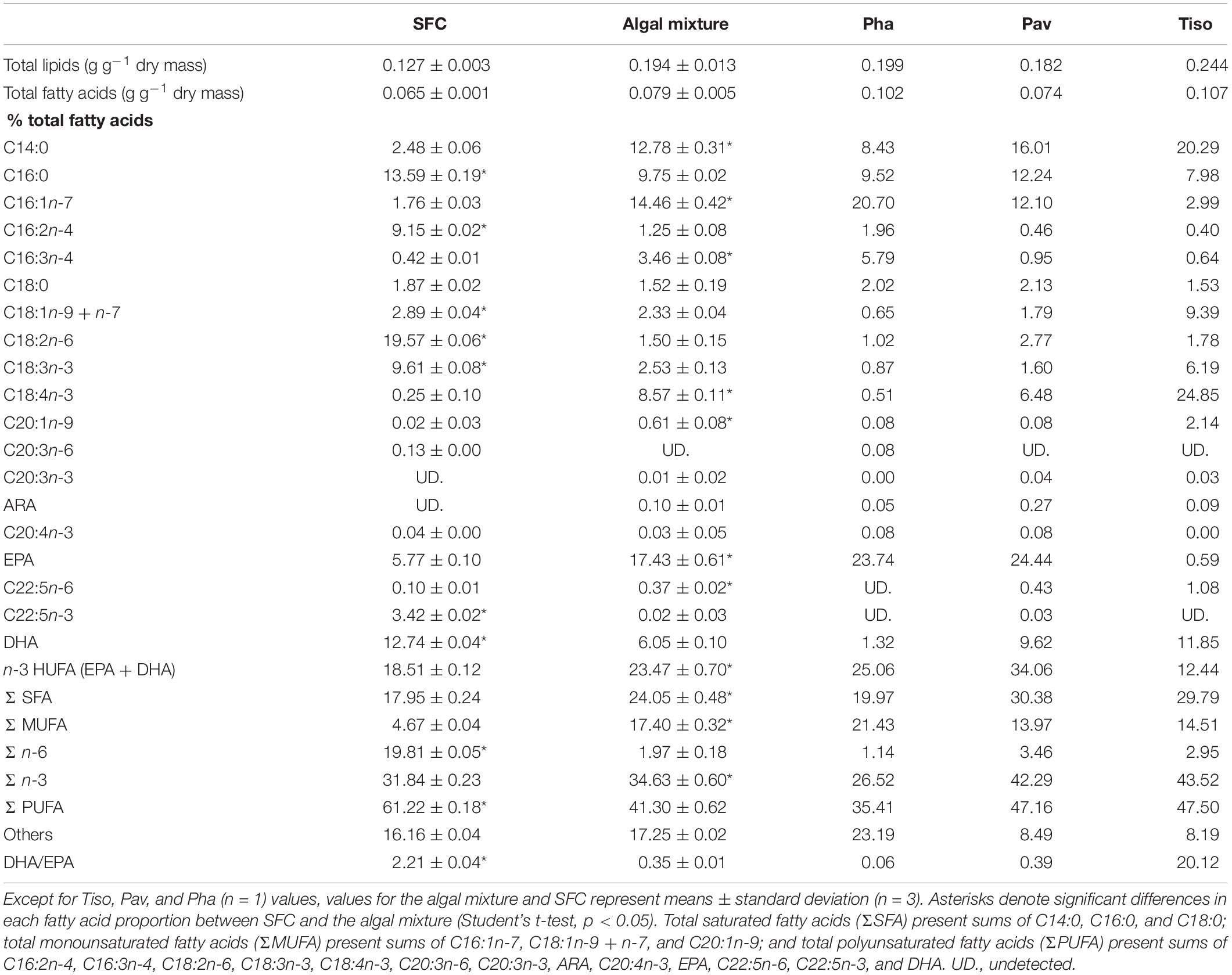
Table 1. Total lipids and their fatty acid proportions in docosahexaenoic acid-supplemented Super Fresh Chlorella (SFC), algae Phaeodactylum (Pha), Pavlova (Pav), and Tisochrysis (Tiso), and their mixture (Algal mixture).
Fatty Acid Profiles of Rotifers and Copepods
The total lipid fatty acid composition of rotifers fed an algal mixture was compared with that of control diet (rotifers fed SFC; Table 2). The fatty acids C16:0, C18:1 (n-9 + n-7), and C18:2n-6 were high in total lipids of rotifers fed SFC. Rotifers fed SFC also exhibited EPA and DHA at 4.66 and 6.40%, respectively, resulting in DHA/EPA ratio of 1.38. When rotifers were fed an algal mixture, the C14:0, C16:1n-7, and C18:4n-3 fatty acid proportions increased significantly, and tended to have more EPA compared with those fed SFC (although the difference was not significant). On the contrary, the 18:2n-6 fatty acid was drastically decreased in rotifers fed an algal mixture (p < 0.05). The DHA was almost half as much as that of rotifers fed SFC. The fatty acid compositions of the two rotifer groups (those fed SFC, and those fed an algal mixture) corresponded with fatty acid peaks in SFC and the algal mixture (Table 1).
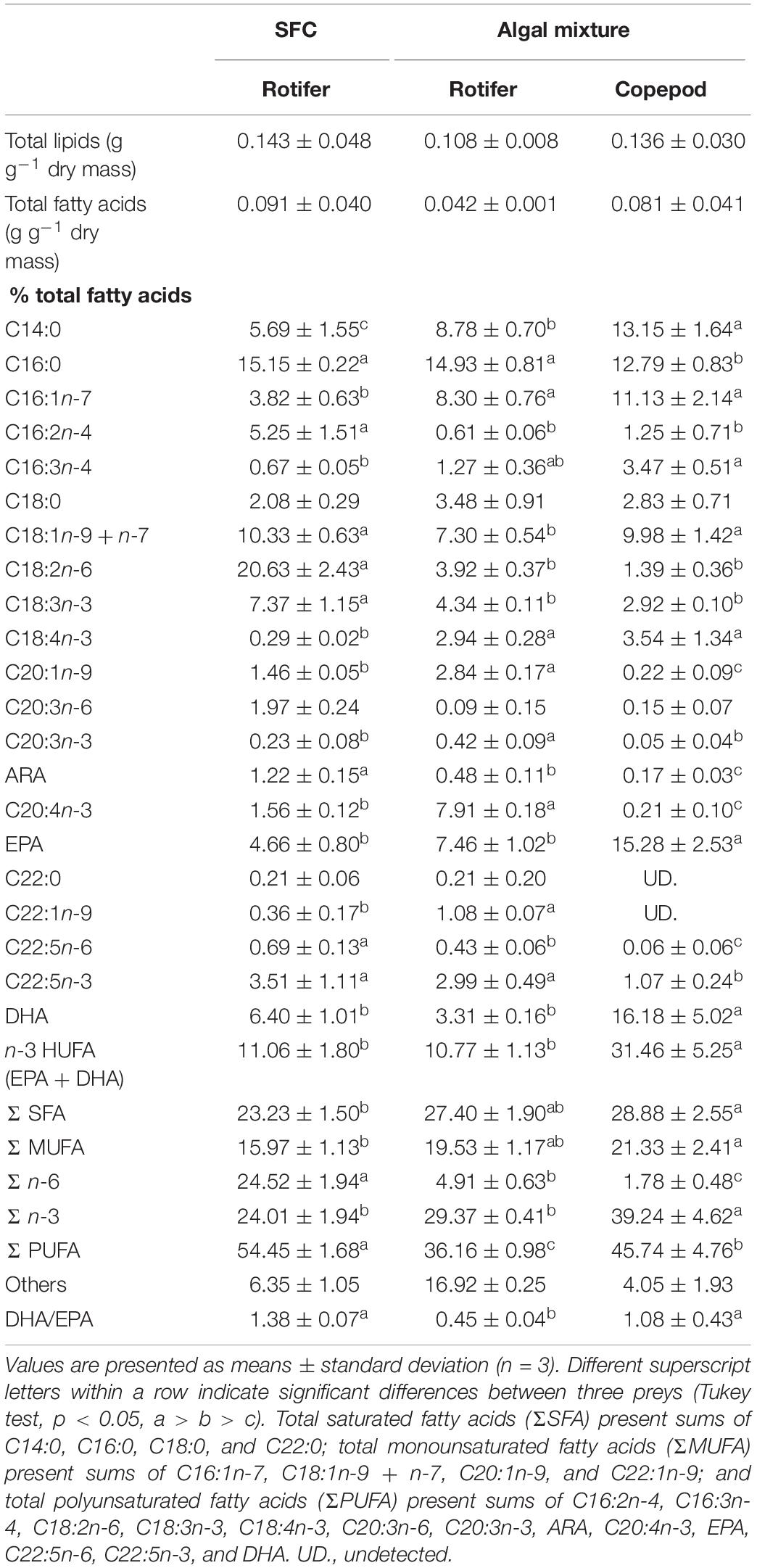
Table 2. Fatty acid composition in total lipids of preys enriched with docosahexaenoic acid-supplemented Super fresh chlorella (SFC), or algal mixture.
Compared with rotifers fed SFC, copepods had higher proportions of C14:0, C16:1n-7, C16:3n-4, and C18:4n-3 fatty acids, and lower proportions of C16:2n-4, C18:2n-6, and C18:3n-3 (p < 0.05; Table 2). Trends of such C14–18 fatty acids were consistently observed in comparison between rotifers fed SFC and algal mixture. However, except for EPA and DHA, copepods had lower proportions of C20–22 fatty acids than rotifers fed SFC or the algal mixture (p < 0.05). Conversely, EPA and DHA in copepods were specifically higher than rotifers fed SFC (p < 0.05). Copepod EPA and DHA accounted for 15.28 and 16.18% of total fatty acids, suggesting that it was a main component of the Σ n-3. In particular, copepods had higher DHA, despite being fed an algal mixture which contained lower DHA than SFC (Table 1) and decreased DHA in rotifers (Table 2). Copepods had a comparable DHA/EPA ratio to rotifers fed SFC (p > 0.05). The ratio was higher compared with rotifers fed algal mixture (p < 0.05).
The NLs and PLs of fatty acids among rotifers and copepods were further compared to evaluate the distributions of EPA and DHA (Tables 3, 4). Compared with rotifers fed SFC, rotifers fed the algal mixture had NLs containing no significantly different EPA, but a lower DHA. Copepod NLs had a higher proportion of EPA than rotifers fed SFC or the algal mixture (p < 0.05; Table 3). DHA in NLs of copepods was, however, lower than in rotifers enriched by SFC (p < 0.05). Conversely, an extremely high proportion of DHA in copepods was distributed to PLs (27.97%; Table 4). DHA in PLs for copepods was five times higher than rotifers fed SFC, and 10 times higher than in those fed the algal mixture (p < 0.05). A comparison of dry mass-based contents of their fatty acids (mg g–1) revealed that copepod EPA and DHA were remarkably higher in NLs and PLs, respectively, (Figure 3). The PLs-EPA of copepods were comparable to those of rotifers fed both SFC and the algal mixture (p > 0.05). A comparison of DHA between the rotifers fed SFC and the algal mixture revealed that they presented comparable PLs-DHA contents (p > 0.05) unlike NLs-DHA contents. Accordingly, rotifers fed SFC had higher NLs-DHA, while copepods had higher PLs-DHA.
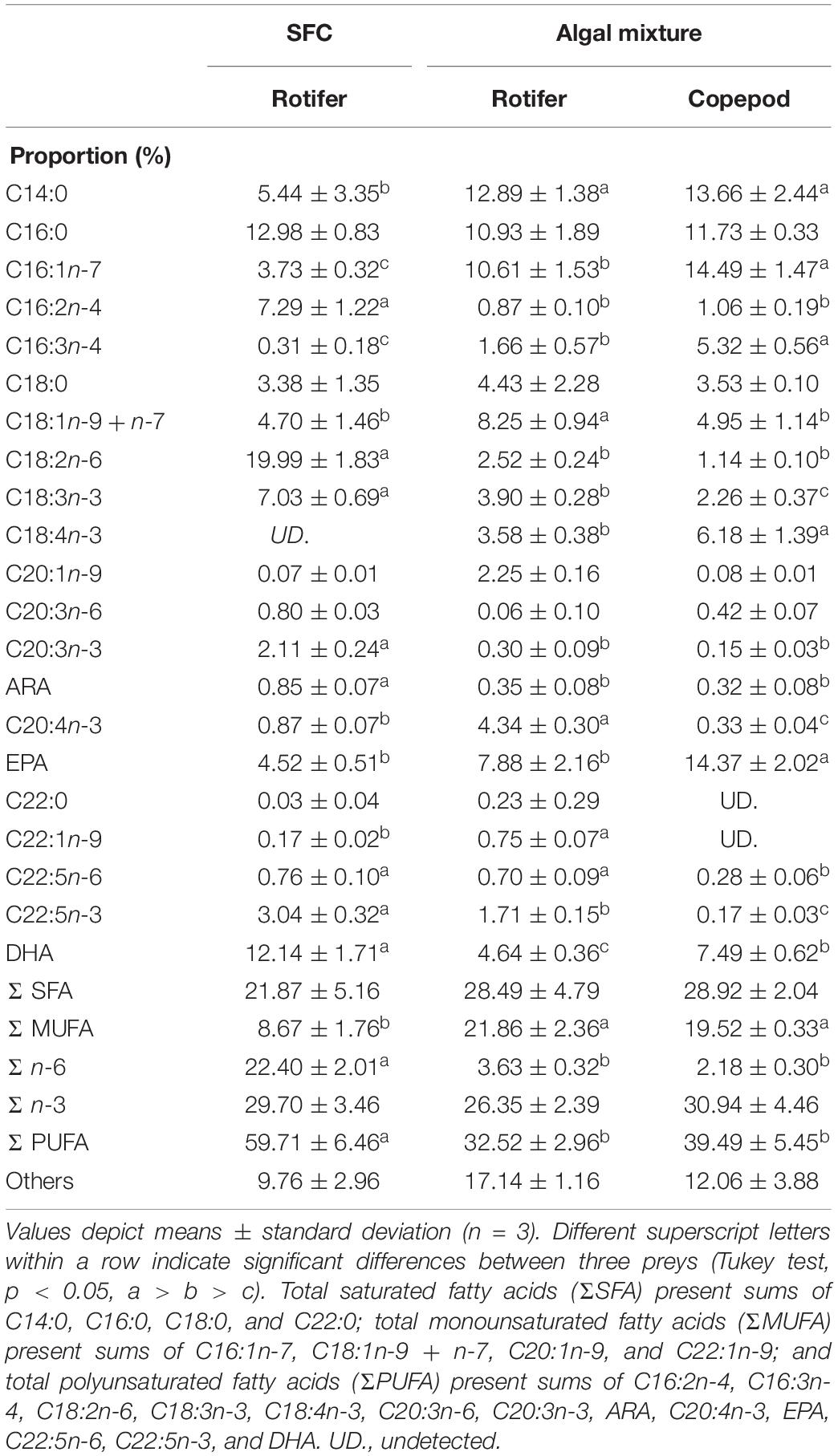
Table 3. Fatty acid proportions in non-polar lipids of preys enriched with docosahexaenoic acid-supplemented Super Fresh Chlorella (SFC), or algal mixture.
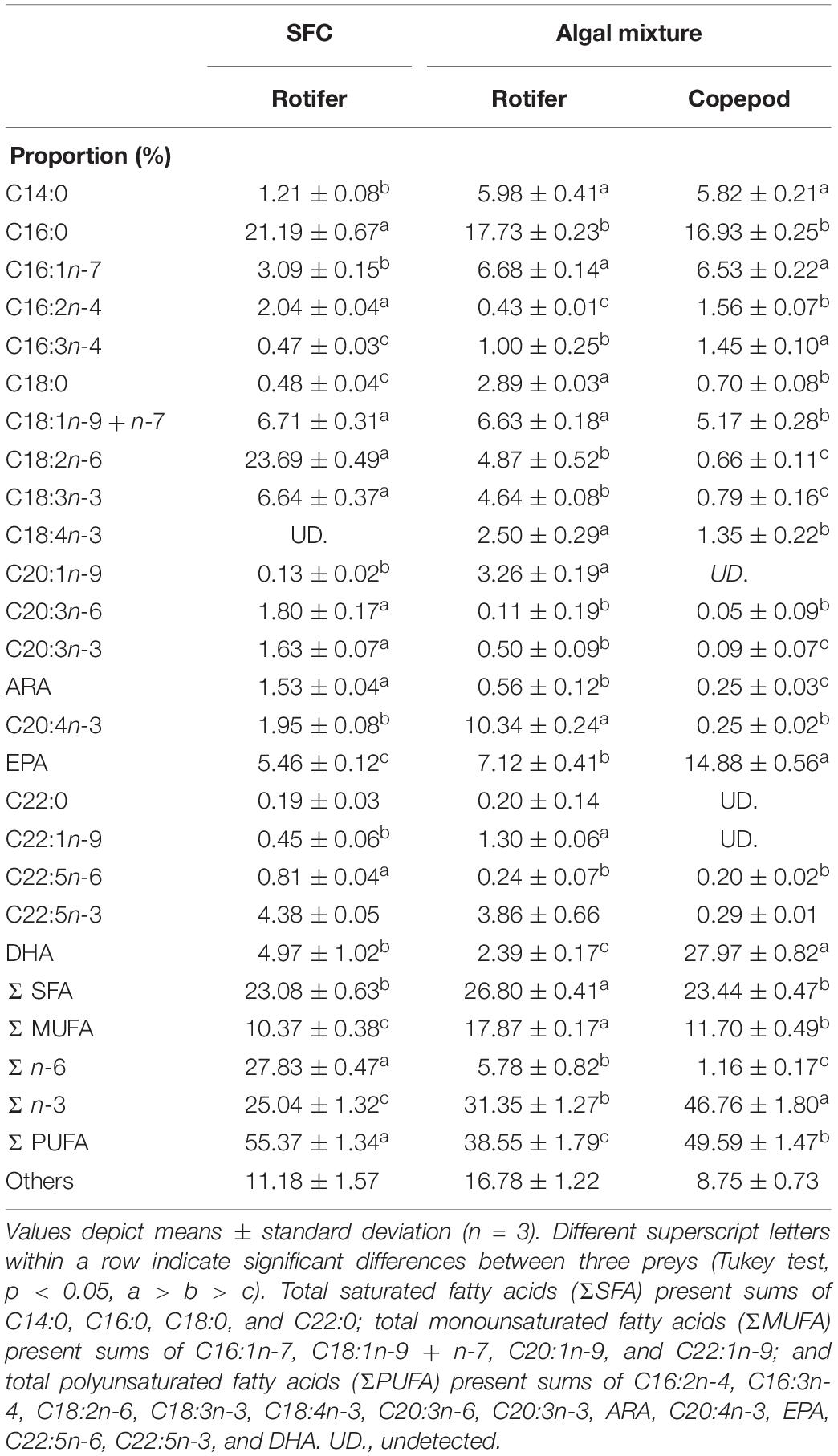
Table 4. Fatty acid proportions in polar lipids of preys enriched with docosahexaenoic acid-supplemented Super Fresh Chlorella (SFC), or algal mixture.
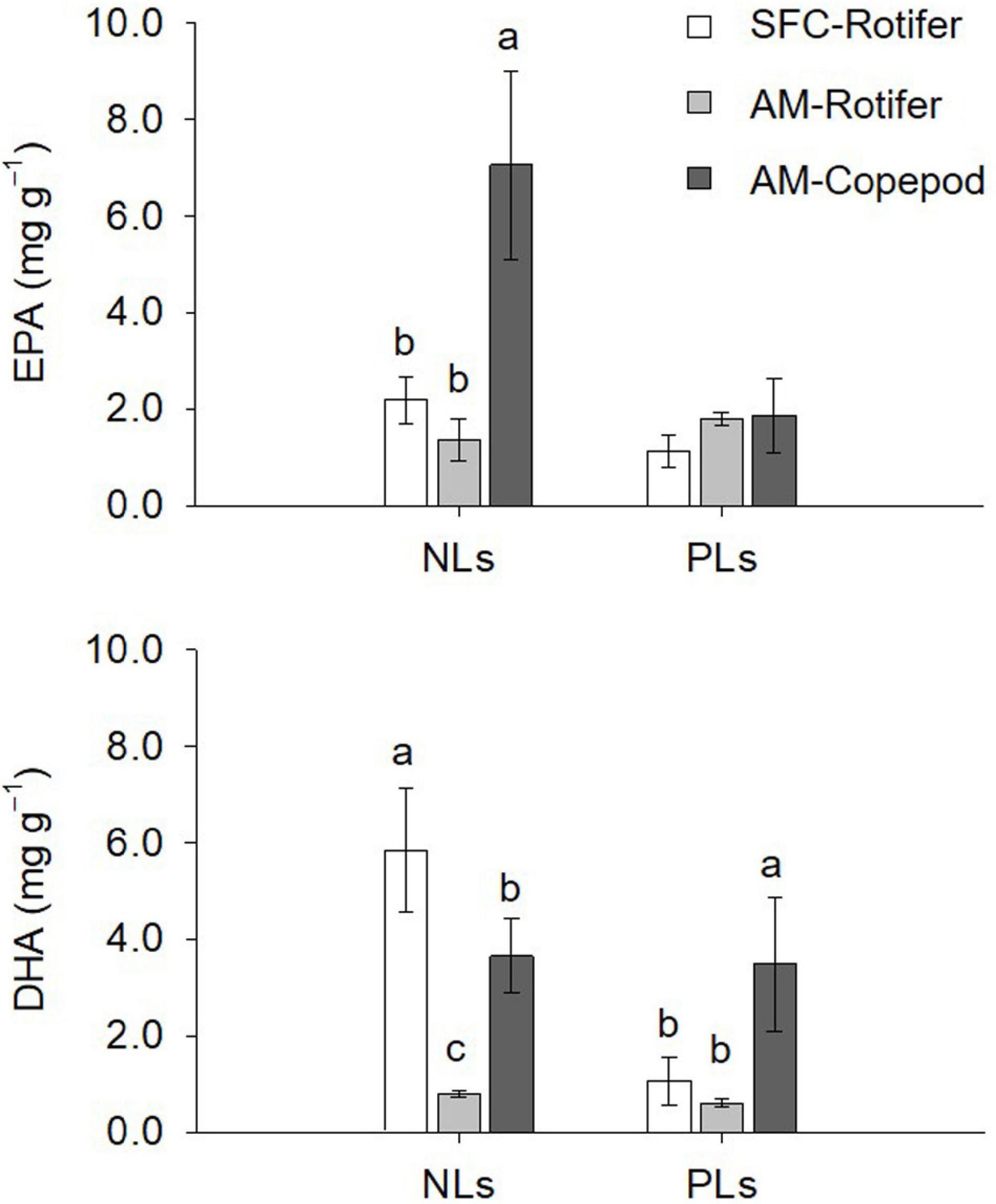
Figure 3. Dry mass-based contents of eicosapentaenoic acid (EPA) and docosahexaenoic acid (DHA) for non-polar lipids (NLs) and polar lipids (PLs) in enriched preys. SFC-Rotifer represents rotifers fed docosahexaenoic acid-supplemented Super Freshwater Chlorella (SFC). AM-Rotifer and AM-Copepod represent rotifers and copepods fed algal mixture, respectively. Values represent means ± standard deviation (n = 3). Letters above bars indicate significant differences (Tukey test, p < 0.05, a > b > c).
Larviculture Performance of Red Sea Bream
Fish larvae fed rotifers and copepods exhibited exponential growth curves (p < 0.05; Figure 4A). SL at 20 dph was greater in fish larvae fed copepods (p < 0.05; Figure 4B). The growth coefficient of larvae fed copepods was higher than that for rotifer feeding treatment (p < 0.05; Table 5).
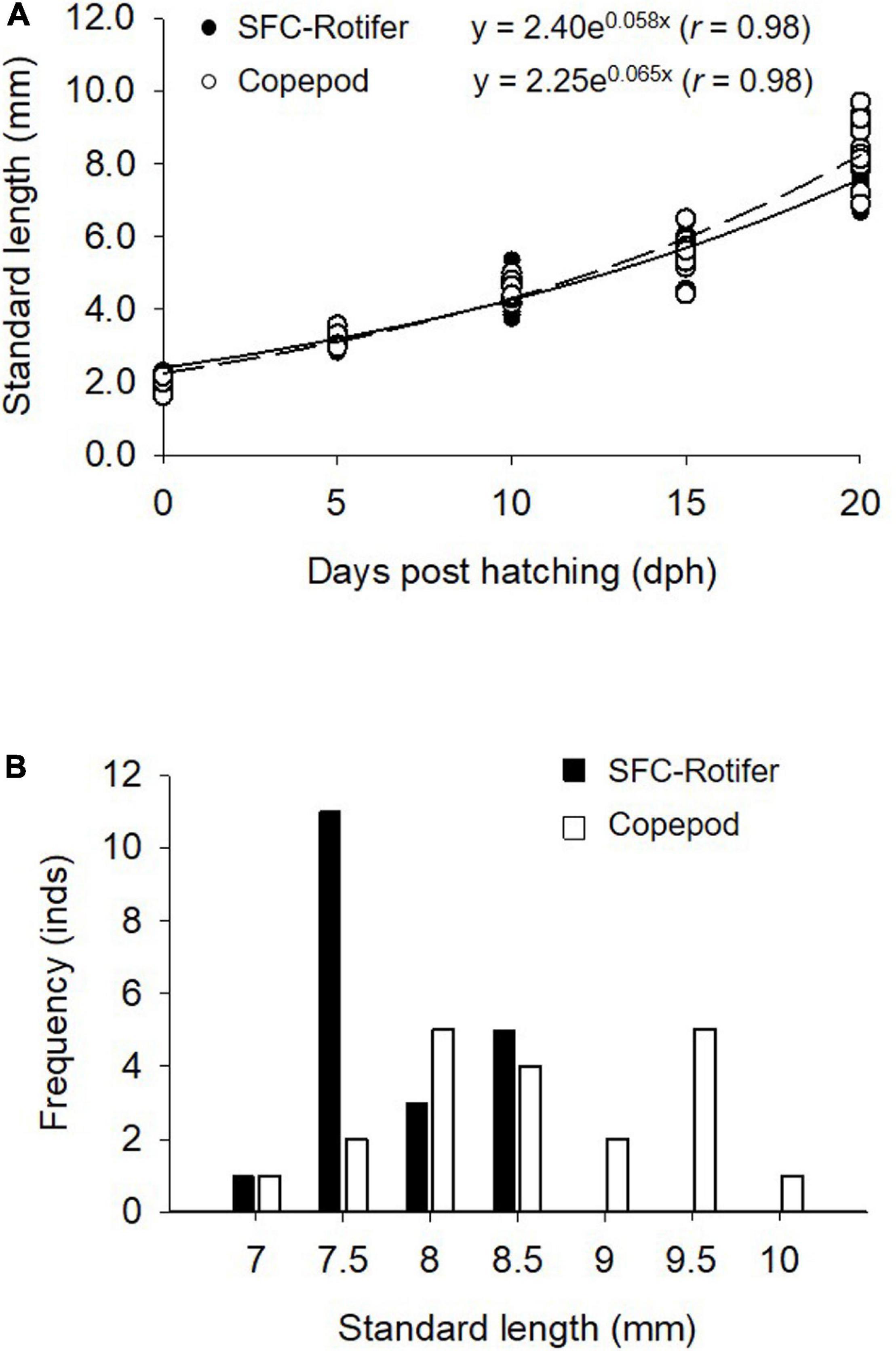
Figure 4. Growth curve for Pagrus major larvae reared under different feeding regimes (A) and a histogram of larval standard length at the end of rearing periods (20 days post hatching) (B). The curve for larvae fed rotifers (SFC-Rotifer; solid line) and those fed copepods (broken line) is fitted based on data from 20 samples obtained at 0, 5, 10, 15, and 20 dph.
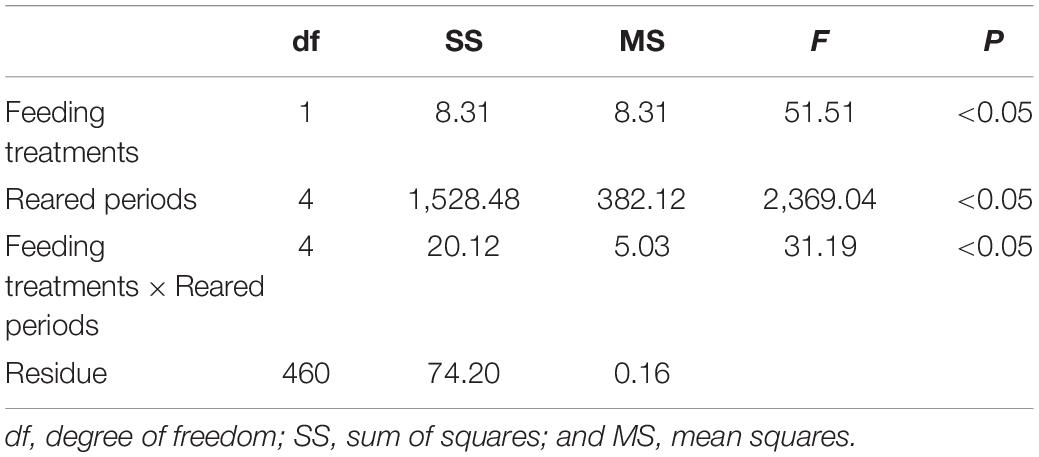
Table 5. The result of two-way analysis of variance (ANOVA) for the exponential growth coefficient of Pagrus major larvae reared during 20 days.
Based on gut contents at 5 dph (Figures 5A,B), fish larvae fed rotifers ingested an average of 2.0 ± 1.3 rotifers larva–1, while those fed copepods ingested an average of 1.0 ± 1.2 nauplii larva–1. Larval ingestion rates increased ontogenetically in both rotifer- and copepod-feeding treatments. Copepodites occurred in the guts of fish larvae from 10 dph. The total dry mass of ingested prey (μg larva–1) was estimated from the number of preys in the gut by multiplying each dry mass (Figure 5C). Total dry mass ingested did not differ between fish larvae fed rotifers or copepods at 5 dph, although the difference in total dry matter was greater in fish larvae fed copepods at both 10 dph (1.7 ± 1.8 μg larva–1) and 15 dph (5.1 ± 3.6 μg larva–1; p < 0.05). Estimates of the total DHA mass ingested by fish larvae (ng larva–1) based on their dry mass-based contents in preys (Figure 5D) showed that copepod-fed fish larvae ingested more PLs-DHA at 5 dph (0.77 ± 0.89 ng larva–1), 10 dph (5.85 ± 6.20 ng larva–1), and 15 dph (17.87 ± 12.47 ng larva–1) than fish larvae fed rotifers. The NLs-DHA mass ingested was comparable between fish larvae fed copepods and rotifers irrespective of their ages.
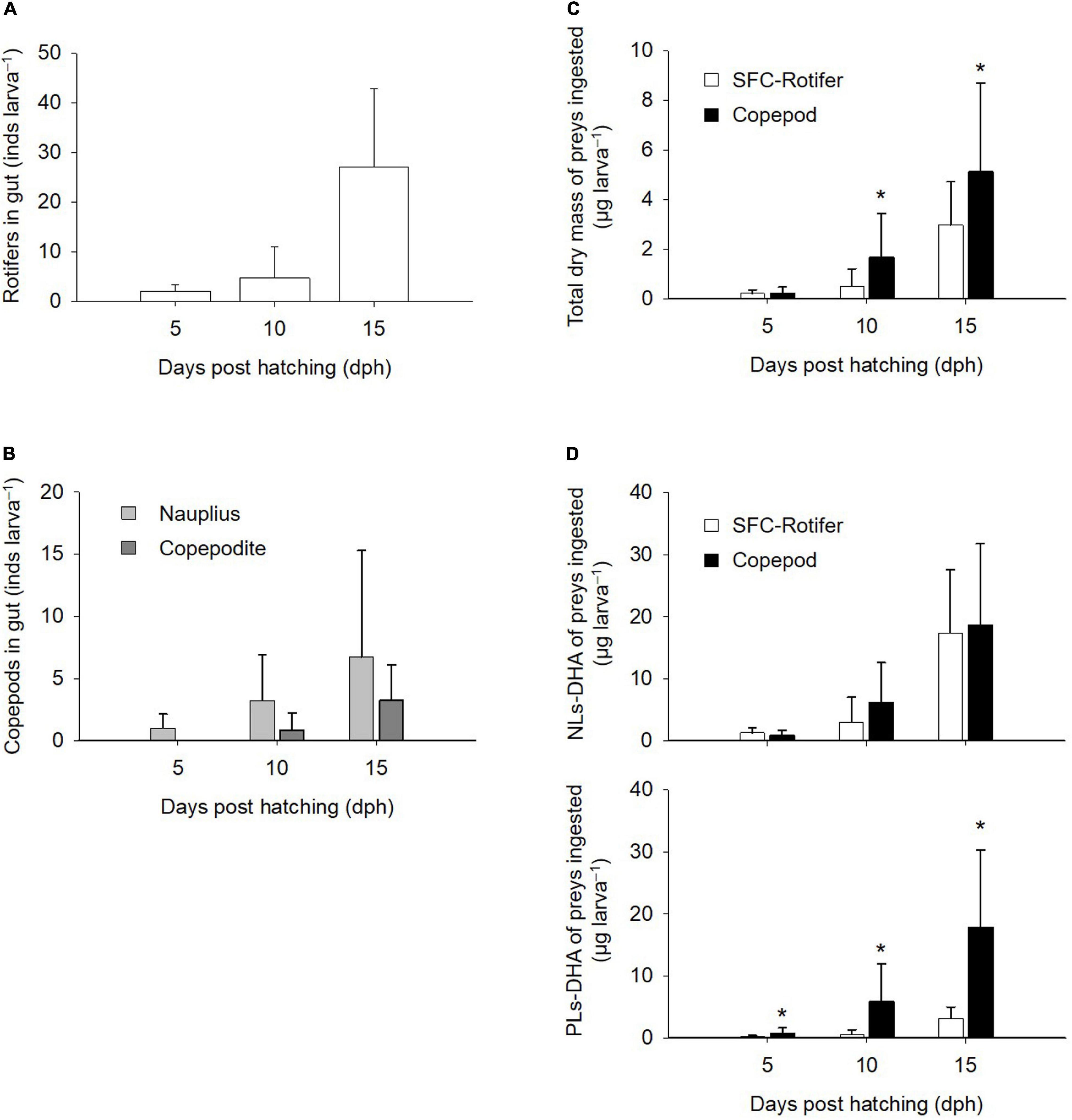
Figure 5. Gut contents of larval Pagrus major based on the number of preys (A,B), total dry mass (C), and docosahexaenoic acid (DHA) mass (D). Ingested mass of DHA is presented for non-polar (NLs-DHA) and polar (PLs-DHA) lipid forms. Values represent means ± standard deviation (n = 15). Asterisks depict significant differences in dry and DHA masses between rotifers fed docosahexaenoic acid-supplemented Super Fresh Chlorella treatment (SFC-Rotifer) and copepod treatments (Student’s t-test, p < 0.05).
Fatty Acid Profiles of Fish Larvae
Fish larvae fed rotifers and copepods had relatively higher values of Σ n-3: 32.45% of total fatty acids in larvae fed rotifers, and 39.39% of total fatty acids in larvae fed copepods (Table 6), comprising mainly DHA (larvae fed rotifers, 20.26%; larvae fed copepods, 27.52%). The DHA/EPA ratios for fish larval total lipids were 4.50 (rotifer-fed fish larvae) and 3.10 (copepod-fed fish larvae)—in both cases about three times higher in larvae than in their food (Table 3). When fish larvae total lipids were separated into NLs and PLs, the NLs-DHA of those fed copepods were about four times higher (26.62%) than those fed rotifers (8.74%). Larval PLs were similarly enriched with DHA (those fed rotifers, 22.34%; those fed copepods, 28.26%).
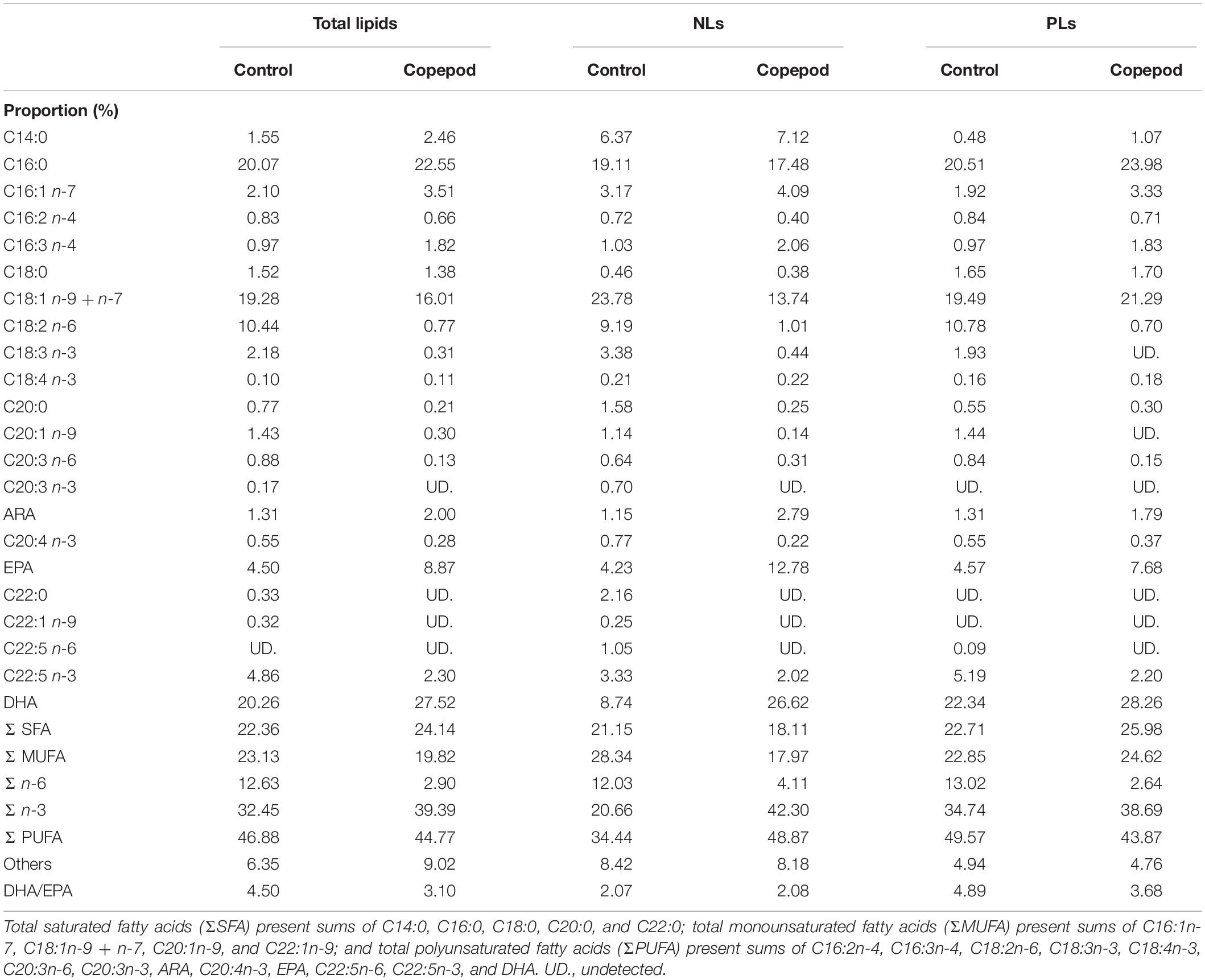
Table 6. Fatty acid composition of total lipids, non-polar lipids (NLs), and polar lipids (PLs) of Pagrus major larvae at the end of rearing periods (20 days post hatching).
Difference in Fatty Acid Characteristics of Microalgae, Zooplankton, and Fish Larvae
Fatty acid compositions of microalgae, zooplankton, and larval fish were summarized using PCA with the first two PC axes (PC1 and PC2). The PC1 and PC2 explained 66.5% and 20.4% of all variations, respectively. On PC1, the score was correlated with almost all fatty acids (C14:0, C16:1n-7, C16:2n-4 C16:3n-4, C18:2n-6, C18:3n-3, C18:4n-3, EPA, and C22:5n-3), but not with C16:0 and DHA. On the other hand, C16:0 and DHA were negatively correlated with the PC2 score.
According to PCA biplot (Figure 6), the PC1 distinguished two groups depending on the origin of dietary fatty acids (i.e., SFC or algal mixture). The most negative scores on PC1 were found for SFC and the scores of rotifers fed SFC and larvae fed those rotifers were close to the SFC scores. On the contrary, algal mixture had the most positive score on PC1. The scores of rotifers fed algal mixture were close to zero, and copepods fed algal mixture had positive scores between algal mixture and the rotifers. Larvae fed those copepods had relatively higher score than larvae fed SFC-enriched rotifers, although their scores were commonly negative.
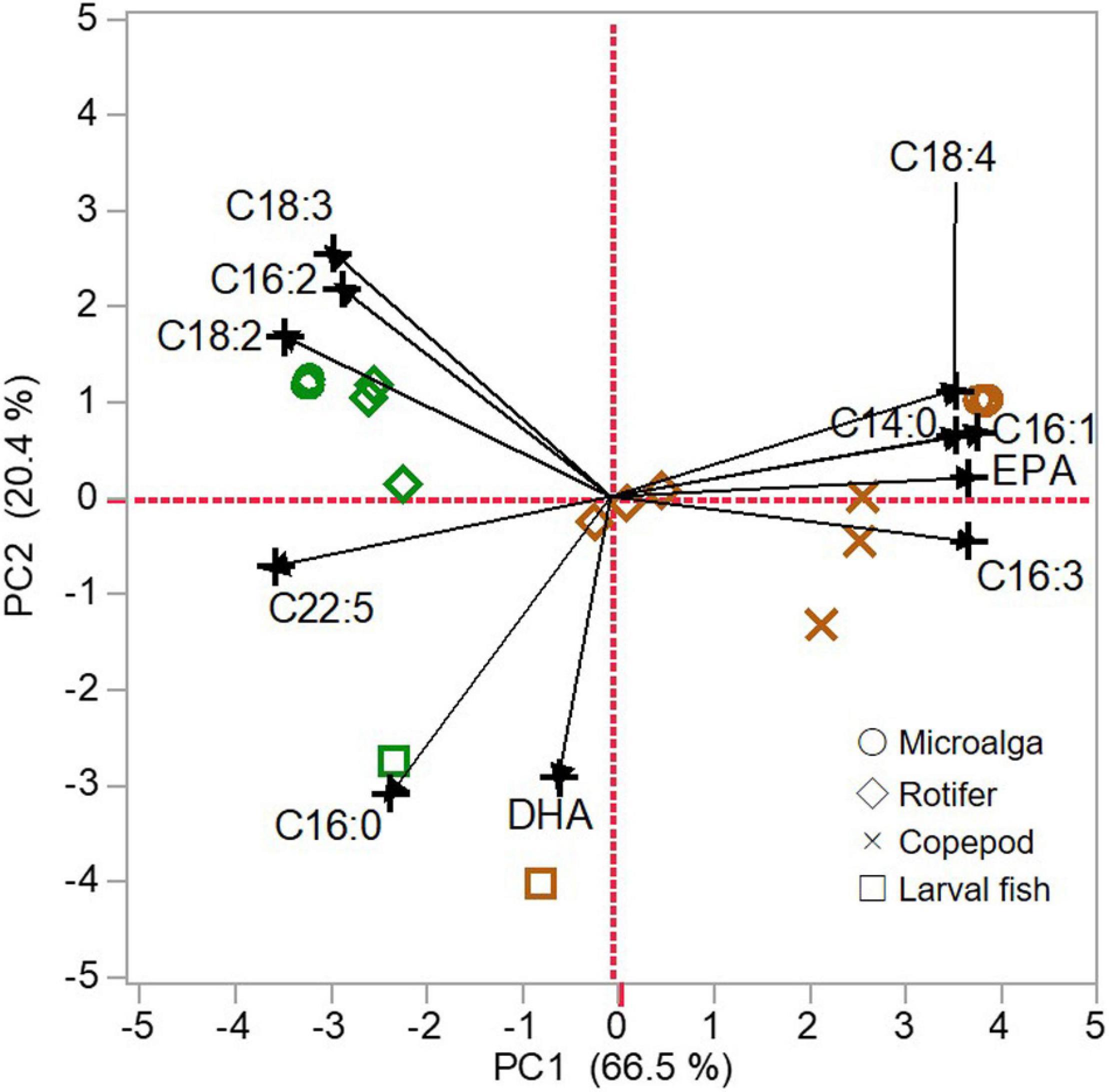
Figure 6. Principal component analysis (PCA) of total lipid fatty acid compositions of microalgae (circle), zooplankton (rotifers, diamond; copepods, cross), and larval fish (square). Green: docosahexaenoic acid-supplemented Super Fresh Chlorella (SFC). Brown: algal mixture. The PCA was calculated using proportions of C14:0, C16:0, C16:1, C16:2, C16:3, C18:2, C18:3, C18:4, EPA, C22:5, and DHA, which were significant differences of one or more percent between SFC and algal mixture. The PC1 scores were correlated with C14:0 (r = 0.913), C16:1 (r = 0.973), C16:2 (r = 0.708), C16:3 (r = 0.944), C18:2 (r = 0.860), C18:3 (r = 0.733), C18:4 (r = 0.909), EPA (r = 0.892), C22:5 (r = 0.892); and the PC2 ones were correlated with C16:0 (r = 0.783) and DHA (r = 0.742).
The PC2 mainly separated larvae from the lower trophic level organisms. Irrespective of the diets, larvae were commonly positioned at the most negative scores. On the contrary, SFC and algal mixture had the most positive scores. The scores of rotifers fed each diet little varied within the positive side, unlike those for PC1. Compared with rotifers, copepods modified the PC2 scores of microalgae toward those of larvae.
Discussion
Accumulation of DHA in PLs of Copepods
The result of PCA for total lipid fatty acid proportions of each trophic level revealed the followings; (1) fatty acid compositions of microalgae (algal mixture and SFC) were commonly the most different from those for larval fish, and (2) compared with microalgae, P. inopinus and rotifers had closer characteristics of fatty acids toward larvae based on DHA and the others (except for C16:0), respectively, (Figure 6). In particular, P. inopinus had PLs specifically enriched with DHA despite its diet containing low DHA relative to EPA (Tables 1, 4). Such selection of fatty acids to accumulate in the PLs can support the function of P. inopinus as a vector of DHA to larval fish.
Fraser et al. (1989) reported wild-caught Calanus finmarchicus, Pseudocalanus sp., and Temora longicornis copepods to have abundant DHA in PLs (30.9, 31.8, and 31.9%, respectively), which is consistent with our copepod species, P. inopinus (Table 4). Each copepod contained little DHA in triacylglycerol and wax esters, the main components of NLs (Fraser et al., 1989). It stresses the importance of DHA as a preferential component in the PLs of copepods. PLs, including phospholipids, mainly form lipid bilayer of plasma and organelle membranes in animals (Spector and Yorek, 1985). An abundance of DHA in C. finmarchicus eggs and nauplii was reported by Sargent and Falk-Petersen (1988). Egg production and hatching success for T. longicornis are enhanced with increased DHA concentration in the diet (Evjemo et al., 2008; Jónasdóttir et al., 2009). Considering specific abundance of DHA in PLs, the DHA in copepods probably functions as a key membrane component in the gonad and embryo during development.
On the other hand, proportions of DHA in PLs of artificially enriched rotifers were lower than in C. finmarchicus (Bell et al., 2003; Olsen et al., 2014), which is consistent with our comparison (Table 4). Rotifers fed SFC had DHA enriched in NLs but not in PLs (Figure 3). There was no significant difference in PLs-DHA of rotifers fed SFC and the algal mixture irrespective of differences in their microalgae DHA (Table 1). Thus, rotifers possibly have PLs with relatively limited capacities for DHA (Li et al., 2015). Rotifers are reported to use DHA mainly as an energy source (Rainuzzo et al., 1994), which is a different DHA metabolic strategy to copepods.
In this study, it was unclear how P. inopinus could retain high concentrations of DHA in their PLs. In mammals and fish, phospholipase A2 and phospholipid acyltransferase function to modify fatty acid composition belonging to PLs (Sun et al., 1979; Balsinde, 2002; Tocher et al., 2008). Such enzymatic activities can also be investigated in copepods to reveal how DHA is preferentially accumulated in their PLs. In addition, copepods can biosynthesize DHA via fatty acid desaturation and elongation even when they cannot ingest diets including DHA (Monroig et al., 2013; Nielsen et al., 2019; Lee et al., 2020). In fact, DHA is detected in Pseudodiaptomus annandalei adults and nauplii fed non-DHA possessing microalga Tetraselmis chuii (Rayner et al., 2017). It indicates that both activities (i.e., accumulation of DHA in the PLs, and fatty acid biosynthesis) can support copepods to maintain DHA in their PLs.
Significance of Copepod Fatty Acid Profiles to Fish Larvae
Larvae fed rotifers and copepods had extremely high DHA relative to EPA (at the ratio of 4.50 and 3.10; Table 6), indicating the preference of DHA for larvae. In particular, PLs in larval fish fed both copepods and rotifers were commonly enriched with DHA regardless of dietary differences in total lipid DHA (Table 2). DHA is highly concentrated in the larval fish brain; it is a key membrane component, and functions in development of the nervous system (Furuita et al., 1998; Masuda et al., 1999). In this case the DHA is often paired with C16:0 (Farkas et al., 2000), explaining why C16:0 was also presentative fatty acid for larvae (Figure 6). Because of the limited capacity of n-3 HUFA biosynthesis in marine fish (Tocher, 2003), red sea bream might preferentially retain DHA obtained from its diet in its PLs. In this study, although there are no replicates in the fatty acid analysis for larvae, NLs-DHA in larvae fed copepods is quite high and therefore non-negligible (Table 6). Dietary DHA is efficiently incorporated into marine finfish larvae when the fatty acid is formed in the PLs (Salhi et al., 1999; Gisbert et al., 2005; Wold et al., 2009). In fact, P. inopinus had high PLs-DHA, while rotifers fed SFC had high DHA in NLs but not in PLs (Figure 3). Larvae also ingested considerably more DHA mass from copepod PLs (Figure 5D). Our results suggest that accumulation of DHA in copepod PLs functions to efficiently transfer DHA to fish larvae.
In addition to larval DHA, the growth was enhanced by feeding copepods compared with rotifers (Figure 4). Our results indicate that the higher growth is achieved by an increase in the ingested copepod mass (mainly copepodite stage) and by more nutrition derived from copepods. Busch et al. (2010) suggest that nutritional status of larvae is a key to explain the differences in growth of larval fish fed rotifers and copepods. Larval Atlantic cod Gadus morhua growth was positively correlated with proportions of DHA (Cutts et al., 2006). Thus, higher DHA in P. inopinus-fed larvae (especially in those NLs) can be linked to the enhanced growth.
As the other n-3 HUFA, EPA is essential fatty acid to further enhanced growth and survival of larvae (Ganuza et al., 2008; Eryalçın et al., 2013). In fact, an increase in concentrations of dietary n-3 HUFA resulted in higher larval growth as long as the DHA/EPA ratio is appropriate (Rodríguez et al., 1998). On the contrary, excess EPA over DHA (i.e., low DHA/EPA ratio) negatively affects larval growth and development (Reitan et al., 1994b; Rodríguez et al., 1997). P. inopinus, despite extremely low DHA/EPA in their diet (Table 1), increased that ratio of total lipids until larvae predated the preys (Table 2). In particular, P. inopinus accumulated excess EPA in the NLs (Figure 4), which might be consumed as an energy source like NLs DHA for rotifers. They indicate that P. inopinus contribute to larval growth by transferring DHA and/or excluding EPA from microalgae.
Roles of P. inopinus in Coastal Regions in Transferring n-3 HUFA to Larvae
In the debate on fish recruitment, contribution of microalgae fatty acids to early development of larval fish has been discussed (Bell and Sargent, 1996). In marine ecosystems, specific fatty acids in microalgae are sufficiently transferred via mainly copepods to wild fish larvae so that they are used as biomarker for microalgae (Klungsøyr et al., 1989; Rossi et al., 2006). Especially in coastal and estuarine regions like P. inopinus habitats, microalgae diatoms are often dominated (Ubertini et al., 2012) and such blooms had higher proportions of EPA than DHA (Sargent et al., 1985). Sediments, which can be fed by the genus Pseudodiaptomus copepods (Jacoby and Greenwood, 1988), also include diatoms as a main component (mainly benthic microalgae; Grippo et al., 2010). However, larvae experienced on diatom-fed copepods showed lower growth than those on copepods fed dinoflagellate with higher DHA and lower EPA (St. John et al., 2001). They can hypothesize that fatty acid distribution in coastal microalgae is not suited for larval growth. According to the result of PCA on each fatty acid species for three trophic levels, however, two kinds of microalgae with distinct fatty acid compositions did not regulate larval DHA (Figure 6). Compared with rotifers, P. inopinus shifted algal fatty acid compositions much more toward DHA peak where larvae were positioned, and furthermore, enhanced larval growth (Figure 4). Our results suggest that what feeds on microalgae is more important factors than microalgae to determine larval growth. On the classical food chain in coastal waters, P. inopinus can function as a buffer of microalgae fatty acids for enhanced larval recruitment.
Conclusion
We artificially recreated a series of trophic levels in the coastal ecosystem using the cultured copepod P. inopinus, its prey (DHA-poor algae), and predatory fish larvae. Fatty acid profiles of P. inopinus and fish larvae fed these copepods were compared with those fed DHA-enriched rotifers. Copepods upgraded DHA levels compared with the dietary fatty acids and selectively fortify their PLs. Concentrations of PLs-DHA were considerably higher in copepods compared with rotifers. Red sea bream larvae fed copepods had higher trends in NLs-DHA and enhanced the growth performance. We suggest that P. inopinus play an important ecological role as a carrier of the essential fatty acid DHA in PLs to coastal finfish larvae.
Data Availability Statement
The original contributions presented in the study are included in the article/supplementary material, further inquiries can be directed to the corresponding author/s.
Ethics Statement
The animal study was reviewed and approved by Animal Experimental Committee, Kagoshima University.
Author Contributions
HM, TS, and TKot designed the study and experiments. HM and TS conducted the experiments and collected the data. TKob identified copepod species. HM prepared a draft of the article and revised it with TKob and VW. MI provided the methodologies for GC analysis. HM performed data analyses. TKot supervised the studies. All the authors read and approved the final manuscript.
Funding
This work was supported by the Grant-in-Aid for Scientific Research (C) from Japan Society for the Promotion of Science (#24580275). Also, we received Functional Enhancement Expenses in Kagoshima University Operating Cost Grant “Advanced research infrastructure of biology and physiology of eel species inhabiting in Southern Kyushu, Japan, Taiwan, and South East Asia and Creation of Industry-academia-government network to induce the social implementation of those research result.”
Conflict of Interest
The authors declare that the research was conducted in the absence of any commercial or financial relationships that could be construed as a potential conflict of interest.
Acknowledgments
We would like to thank the members of the Laboratory of Larval Rearing Management, Faculty of Fisheries, Kagoshima University, for their assistance in performing the larviculture experiment. Also, we would like to thank Dr. Hikaru Endo for the discussion on statistical analysis. We would also thank Steve O’Shea, Ph.D., from Edanz Group (https://en-author-services.edanzgroup.com/ac) for editing the draft of this manuscript.
Abbreviations
ANOVA, Analysis of variance; NLs, Non-polar lipids; PLs, Polar lipids; SFC, Super Fresh Chlorella.
References
Ansotegui, A., Sarobe, A., Trigueros, J. M., Urrutxurtu, I., and Orive, E. (2003). Size distribution of algal pigments and phytoplankton assemblages in a coastal–estuarine environment: contribution of small eukaryotic algae. J. Plankton Res. 25, 341–355. doi: 10.1093/plankt/25.4.341
Balsinde, J. (2002). Roles of various phospholipases A2 in providing lysophospholipid acceptors for fatty acid phospholipid incorporation and remodelling. Biochem. J. 364, 695–702. doi: 10.1042/bj20020142
Bell, J. G., McEvoy, L. A., Estevez, A., Shields, R. J., and Sargent, J. R. (2003). Optimising lipid nutrition in first-feeding flatfish larvae. Aquaculture 227, 211–220. doi: 10.1016/s0044-8486(03)00504-0
Bell, M. V., and Sargent, J. R. (1996). Lipid nutrition and fish recruitment. Mar. Ecol. Prog. Ser. 134, 315–316. doi: 10.3354/meps134315
Bendif, E. M., Probert, I., Schroeder, D. C., and de Vargas, C. (2013). On the description of Tisochrysis lutea gen. nov. sp. nov. and Isochrysis nuda sp. nov. in the Isochrysidales, and the transfer of Dicrateria to the Prymnesiales (Haptophyta). J. Appl. Phycol. 25, 1763–1776. doi: 10.1007/s10811-013-0037-0
Beyrend-Dur, D., Kumar, R., Ramakrishna Rao, T., Souissi, S., Cheng, S.-H., and Hwang, J.-S. (2011). Demographic parameters of adults of Pseudodiaptomus annandalei (Copepoda: Calanoida): temperature-salinity and generatino effects. J. Exp. Mar. Biol. Ecol. 404, 1–14. doi: 10.1016/j.jembe.2011.04.012
Borges, R., Ben-Hamadou, R., Chícharo, M. A., Ré, P., and Gonçalves, E. J. (2007). Horizontal spatial and temporal distribution patterns of nearshore larval fish assemblages at a temperate rocky shore. Estuar. Coast. Shelf Sci. 71, 412–428. doi: 10.1016/j.ecss.2006.08.020
Busch, K. E. T., Falk-Petersen, I.-B., Peruzzi, S., Rist, N. A., and Hamre, K. (2010). Natural zooplankton as larval feed in intensive rearing systems for juvenile production of Atlantic cod (Gadus morhua L.). Aquac. Res. 41, 1727–1740. doi: 10.1111/j.1365-2109.2009.02450.x
Castell, J., Blair, T., Neil, S., Howes, K., Mercer, S., Reid, J., et al. (2003). The effect of different HUFA enrichment emulsions on the nutritional value of rotifers (Brachionus plicatilis) fed to larval haddock (Melanogrammus aeglefinus). Aquacult. Int. 11, 109–117.
Chen, J.-N., Takeuchi, T., Takahashi, T., Tomoda, T., Koiso, M., and Kuwada, H. (2004). Effect of rotifers enriched with taurine on growth and survival activity of red sea bream Pagrus major larvae. Nippon Suisan Gakkaishi 70, 542–547. doi: 10.2331/suisan.70.542
Cushing, D. H. (1990). Plankton production and year-class strength in fish populations: an update of the match/mismatch hypothesis. Adv. Mar. Biol. 26, 249–293. doi: 10.1016/s0065-2881(08)60202-3
Cutts, C. J., Sawanboonchun, J., Mazorra, de Quero, C., and Bell, J. G. (2006). Diet-induced differences in the essential fatty acid (EFA) compositions of larval Atlantic cod (Gadus morhua L.) with reference to possible effects of dietary EFAs on larval performance. ICES J. Mar. Sci. 63, 302–310. doi: 10.1016/j.icesjms.2005.11.002
Eryalçın, K. M., Roo, J., Saleh, R., Atalah, E., Benítez, T., Betancor, M., et al. (2013). Fish oil replacement by different microalgal products in microdiets for early waning of gilthead sea bream (Sparus aurata, L.). Aquacult. Res. 44, 819–828. doi: 10.1111/j.1365-2109.2012.03237.x
Evjemo, J. O., Reitan, K. I., and Olsen, Y. (2003). Copepods as live food organisms in the larval rearing of halibut larvae (Hippoglossus hippoglossus L.) with special emphasis on the nutritional value. Aquaculture 227, 191–210. doi: 10.1016/s0044-8486(03)00503-9
Evjemo, J. O., Tokle, N., Vadstein, O., and Olsen, Y. (2008). Effect of essential dietary fatty acids on egg production and hatching success of the marine copepod Temora longicornis. J. Exp. Mar. Bio. Ecol. 365, 31–37. doi: 10.1016/j.jembe.2008.07.032
Farkas, T., Kitajka, K., Fodor, E., Csengeri, I., Lahdes, E., Yeo, Y. K., et al. (2000). Docosahexaenoic acid-containing phospholipid molecular species in brains of vertebrates. Proc. Natl. Acad. Sci. 97, 6362–6366. doi: 10.1073/pnas.120157297
Folch, J., Lees, M., and Sloan Stanley, G. (1957). A simple method for the isolation and purification of total lipids from animal tissues. J. Biol. Chem. 226, 497–509. doi: 10.1016/s0021-9258(18)64849-5
Fraser, A. J., Sargent, J. R., and Gamble, J. C. (1989). Lipid class and fatty acid composition of Calanus finmarchicus (Gunnerus), Pseudocalanus sp. and Temora longicornis Muller from a nutrient-enriched seawater enclosure. J. Exp. Mar. Bio. Ecol. 130, 81–92. doi: 10.1016/0022-0981(89)90020-8
Fu, Y., Hirayama, K., and Natsukari, Y. (1991). Morphological differences between two types of the rotifer Brachionus plicatilis O.F. Müllér. J. Exp. Mar. Biol. Ecol. 151, 29–41. doi: 10.1016/0022-0981(91)90013-m
Furuita, H., Takeuchi, T., and Uematsu, K. (1998). Effects of eicosapentaenoic and docosahexaenoic acids on growth, survival and brain development of larval Japanese flounder (Paralichthys olivaceus). Aquaculture 161, 269–279. doi: 10.1016/s0044-8486(97)00275-5
Ganuza, E., Benítez-Santana, T., Atalah, E., Vega-Orellana, O., Ganga, R., and Izquierdo, M. S. (2008). Crypthecodinium cohnii and Schizochytrium sp. as potential substitutes to fisheries-derived oils from seabream (Sparus aurata) microdiets. Aquaculture 277, 109–116. doi: 10.1016/j.aquaculture.2008.02.005
Gisbert, E., Villeneuve, L., Zambonino-Infante, J. L., Quazuguel, P., and Cahu, C. L. (2005). Dietary phospholipids are more efficient than neutral lipids for long-chain polyunsaturated fatty acid supply in European sea bass Dicentrarchus labrax larval development. Lipids 40, 609–618. doi: 10.1007/s11745-005-1422-0
Grippo, M. A., Fleeger, J. W., Rabalais, N. N., Condrey, R., and Carman, K. R. (2010). Contribution of phytoplankton and benthic microalgae to inner shelf sediments of the north-central Gulf of Mexico. Cont. Shelf Res. 30, 456–466. doi: 10.1016/j.csr.2009.12.015
Hayashi, M., Yukino, T., Maruyama, I., Kido, S., and Kitaoka, S. (2001). Uptake and accumulation of exogenous docosahexaenoic acid by Chlorella. Biosci. Biotechnol. Biochem. 65, 202–204. doi: 10.1271/bbb.65.202
Hjort, J. (1914). Fluctuations in the great fisheries of northern Europe viewed in the light of biological research. Rapports et Procés-Verbaux des Réunions du Conseil Permanent. International Pour l’Exploration de la Mer 20, 1–228. doi: 10.15578/ifrj.3.1.1997.1-7
Hossain, M. S., Koshio, S., Ishikawa, M., Yokoyama, S., and Sony, N. M. (2016). Dietary effects of adenosine monophosphate to enhance growth, digestibility, innate immune responses and stress resistance of juvenile red sea bream, Pagrus major. Fish Shellfish Immunol. 56, 523–533. doi: 10.1016/j.fsi.2016.08.009
Hwang, J. S., Kumar, R., Hsieh, C. W., Kuo, A. Y., Souissi, S., Hsu, M. H., et al. (2010). Patterns of zooplankton distribution along marine, estuarine and riverine portion of Danshuei ecosystem, northern Taiwan. Zool. Stud. 49, 335–352.
Jacoby, C. A., and Greenwood, J. G. (1988). Spatial, temporal, and behavioral patterns in emergence of zooplankton in the lagoon of heron reef, great barrier reef, Australia. Mar. Biol. 97, 309–328. doi: 10.1007/bf00397762
Jónasdóttir, S., Visser, A., and Jespersen, C. (2009). Assessing the role of food quality in the production and hatching of Temora longicornis eggs. Mar. Ecol. Prog. Ser. 382, 139–150. doi: 10.3354/meps07985
Juaneda, P., and Rocquelin, G. (1985). Rapid and convenient separation of phospholipids and non phosphorus lipids from rat heart using silica cartridges. Lipids 20, 40–41. doi: 10.1007/bf02534360
Karlsen, Ø, van der Meeren, T., Rønnestad, I., Mangor-Jensen, A., Galloway, T. F., Kjørsvik, E., et al. (2015). Copepods enhance nutritional status, growth and development in Atlantic cod (Gadus morhua L.) larvae – can we identify the underlying factors? Peer J. 3:e902. doi: 10.7717/peerj.902
Kates, M., and Volcani, B. E. (1966). Lipid components of diatoms. Biochim. Biophys. Acta 116, 264–278. doi: 10.1016/0005-2760(66)90009-9
Kleppel, G. (1993). On the diets of calanoid copepods. Mar. Ecol. Prog. Ser. 99, 183–195. doi: 10.3354/meps099183
Klungsøyr, J., Tilseth, S., Wilhelmsen, S., Falk-Petersen, S., and Sargent, J. R. (1989). Fatty acid composition as an indicator of food intake in cod larvae Gadus morhua from Lofoten, Northern Norway. Mar. Biol. 102, 183–188. doi: 10.1007/bf00428278
Kotani, T., Haraguchi, T., Yamazaki, Y., Doi, T., Matsui, H., Yokoyama, S., et al. (2017). Effect of the duration of nutritional enrichment on the fatty acid composition of commonly used rotifers Brachionus plicatilis sp. complex and lar viculture performance of red sea bream Pagrus major. Aquac. Sci. 65, 133–144.
Koven, W. M., Tandler, A., Kissil, C. W. M., Sklan, D., Friezlander, O., and Harel, M. (1990). The effect of dietary (n-3) polyunsaturated fatty acids on growth, survival and swim bladder development in Sparus aurata larvae. Aquaculture 91, 131–141. doi: 10.1016/0044-8486(90)90182-m
Lee, M. C., Choi, H., Park, J. C., Yoon, D. S., Lee, Y., Hagiwara, A., et al. (2020). A comparative study of food selectivity of the benthic copepod Tigriopus japonicus and the pelagic copepod Paracyclopina nana: a genome-wide identification of fatty acid conversion genes and nitrogen isotope investigation. Aquaculture 521:734930. doi: 10.1016/j.aquaculture.2020.734930
Li, K., Kjørsvik, E., Bergvik, M., and Olsen, Y. (2015). Manipulation of the fatty acid composition of phosphatidylcholine and phosphatidylethanolamine in rotifers brachionus nevada and brachionus cayman. Aquacult. Nutr. 21, 85–97. doi: 10.1111/anu.12140
Masuda, R., Takeuchi, T., Tsukamoto, K., Sato, H., Shimizu, K., and Imaizumi, K. (1999). Incorporation of dietary docosahexaenoic acid into the central nervous system of the yellowtail Seriola quinqueradiata. Brain. Behav. Evol. 53, 173–179. doi: 10.1159/000006592
Matsui, H., Anraku, K., and Kotani, T. (2019). Spectrophotometry can monitor changes in algal metabolism triggered by nutrient deficiency in Nannochloropsis oculata cultured under various light-emitting diode light regimes. Fish. Sci. 85, 167–176. doi: 10.1007/s12562-018-1261-y
Monroig, Ó, Tocher, D., and Navarro, J. (2013). Biosynthesis of polyunsaturated fatty acids in marine invertebrates: recent advances in molecular mechanisms. Mar. Drugs 11, 3998–4018. doi: 10.3390/md11103998
Nćss, T., Germain-Henry, M., and Naas, K. E. (1995). First feeding of Atlantic halibut (Hippoglossus hippoglossus) using different combinations of Artemia and wild zooplankton. Aquaculture 130, 235–250. doi: 10.1016/0044-8486(94)00323-g
Nielsen, B. L. H., Gøtterup, L., Jørgensen, T. S., Hansen, B. W., Hansen, L. H., Mortensen, J., et al. (2019). n-3 PUFA biosynthesis by the copepod Apocyclops royi documented using fatty acid profile analysis and gene expression analysis. Biol. Open 8:bio038331. doi: 10.1242/bio.038331
Ohs, C. L., Chang, K. L., Grabe, S. W., DiMaggio, M. A., and Stenn, E. (2010). Evaluation of dietary microalgae for culture of the calanoid copepod Pseudodiaptomus pelagicus. Aquaculture 307, 225–232. doi: 10.1016/j.aquaculture.2010.07.016
Olsen, Y., Evjemo, J. O., Kjørsvik, E., Larssen, H., Li, K., Overrein, I., et al. (2014). DHA content in dietary phospholipids affects DHA content in phospholipids of cod larvae and larval performance. Aquaculture 42, 203–214. doi: 10.1016/j.aquaculture.2014.03.002
Omori, M., and Ikeda, T. (1984). Methods in Marine Zooplankton Ecology. New York, NY: John Wiley & Sons, 332.
Paulsen, M., Hammer, C., Malzahn, A. M., Polte, P., von Dorrien, C., and Clemmesen, C. (2014). Nutritional situation for larval Atlantic herring (Clupea harengus L.) in two nursery areas in the western Baltic sea. ICES J. Mar. Sci. 71, 991–1000. doi: 10.1093/icesjms/fst168
Rainuzzo, J. R., Reitan, K. I., and Olsen, Y. (1994). Effect of short- and long-term lipid enrichment on total lipids, lipid class and fatty acid composition in rotifers. Aquacult. Int. 2, 19–32. doi: 10.1007/bf00118530
Rayner, T. A., Hwang, J.-S., and Hansen, B. W. (2017). Minimizing the use of fish oil enrichment in live feed by use of a self-enriching calanoid copepod Pseudodiaptomus annandalei. J. Plankton Res. 39, 1004–1011. doi: 10.1093/plankt/fbx021
Rayner, T. A., Jørgensen, N. O. G., Blanda, E., Wu, C.-H., Huang, C.-C., Mortensen, J., et al. (2015). Biochemical composition of the promising live feed tropical calanoid copepod Pseudodiaptomus annandalei (Sewell 1919) cultured in Taiwanese outdoor aquaculture ponds. Aquaculture 441, 25–34. doi: 10.1016/j.aquaculture.2015.01.034
Reitan, K. I., Rainuzzo, J. R., and Olsen, Y. (1994a). Effect of nutrient limitation on fatty acid and lipid content of marine microalgae. J. Phycol. 30, 972–979. doi: 10.1111/j.0022-3646.1994.00972.x
Reitan, K. I., Rainuzzo, J. R., and Olsen, Y. (1994b). Influence of lipid composition of live feed on growth, survival and pigmentation of turbot larvae. Aquacult. Int. 2, 33–48. doi: 10.1007/bf00118531
Rodríguez, C., Pérez, J. A., Díaz, M., Izquierdo, M. S., Fernández-Palacios, H., and Lorenzo, A. (1997). Influence of the EPA/DHA ratio in rotifers on gilthead seabream (Sparus aurata) larval development. Aquaculture 150, 77–89. doi: 10.1016/s0044-8486(96)01472-x
Rodríguez, C., Pérez, J. A., Badía, P., Izquierdo, M. S., Fernández-Palacios, H., and Lorenzo, A. (1998). The n-3 highly unsaturated fatty acids requirements of gilthead seabream (Aparus aurata L.) larvae when using an appropriate DHA/EPA ratio in the diet. Aquaculture 169, 9–23. doi: 10.1016/s0044-8486(98)00328-7
Rossi, S., Sabatés, A., Latasa, M., and Reyes, E. (2006). Lipid biomarkers and trophic linkages between phytoplankton, zooplankton and anchovy (Engraulis encrasicolus) larvae in the NW Mediterranean. J. Plankton Res. 28, 551–562. doi: 10.1093/plankt/fbi140
Sakaguchi, S. O., and Ueda, H. (2011). Morphological divergence of Pseudodiaptomus inopinus Burckhardt, 1913 (Copepoda: Calanoida) between the Japan sea and Pacific coasts of western Japan. Plankt. Benthos Res. 6, 124–128. doi: 10.3800/pbr.6.124
Salhi, M., Hernández-Cruz, C. M., Bessonart, M., Izquierdo, M. S., and Fernández-Palacios, H. (1999). Effect of different dietary polar lipid levels and different n-3 HUFA content in polar lipids on gut and liver histological structure of gilthead seabream (Sparus aurata) larvae. Aquaculture 179, 253–263. doi: 10.1016/s0044-8486(99)00194-5
Sargent, J. R., and Falk-Petersen, S. (1988). The lipid biochemistry of calanoid copepods. Hydrobiologia 16, 101–114. doi: 10.1007/978-94-009-3103-9_9
Sargent, J. R., Eilertsen, H. C., Falk-Petersen, S., and Taasen, J. P. (1985). Carbon assimilation and lipid production in phytoplankton in northern Norwegian fjords. Mar. Biol. 85, 109–116. doi: 10.1007/bf00397428
Schipp, G. R., Bosmans, J. M. P., and Marshall, A. J. (1999). A method for hatchery culture of tropical calanoid copepods, Acartia spp. Aquaculture 174, 81–88. doi: 10.1016/s0044-8486(98)00508-0
Shields, R. J., Bell, J. G., Luizi, F. S., Gara, B., Bromage, N. R., and Sargent, J. R. (1999). Natural copepods are superior to enriched Artemia nauplii as feed for halibut larvae (Hippoglossus hippoglossus) in terms of survival, pigmentation and retinal morphology: relation to dietary essential fatty acids. J. Nutr. 129, 1186–1194. doi: 10.1093/jn/129.6.1186
Shields, R. J., Kotani, T., Molnar, A., Marion, K., Kobashigawa, J., and Tang, L. (2005). “Intensive cultivation of a subtropical Paracalanid copepod, Parvocalanus sp., as prey for small marine fish larvae,” in Copepods in Aquaculture, eds L. Cheng-Sheng, P. J. O’Bryen, and N. H. Marcus (Oxfort: Blackwell Publishing), 209–223. doi: 10.1002/9780470277522.ch16
Shoji, J., Sakiyama, K., Hori, M., Yoshida, G., and Hamaguchi, M. (2007). Seagrass habitat reduces vulnerability of red sea bream Pagrus major juveniles to piscivorous fish predator. Fish. Sci. 73, 1281–1285.
Spector, A. A., and Yorek, M. A. (1985). Membrane lipid composition and cellular function. J. Lipid Res. 26, 1015–1034. doi: 10.1016/s0022-2275(20)34276-0
St. John, M. A., Clemmesen, C., Lund, T., and Köster, T. (2001). Diatom production in the marine environment: implications for larval fish growth and condition. ICES J. Mar. Sci. 58, 1106–1113. doi: 10.1006/jmsc.2001.1089
Støttrup, J. G., and Jensen, J. (1990). Influence of algal diet on feeding and egg-production of the calanoid copepod Acartia tonsa Dana. J. Exp. Mar. Biol. Ecol. 141, 87–105. doi: 10.1016/0022-0981(90)90216-y
Sun, G. Y., Su, K. L., Der, O. M., and Tang, W. (1979). Enzymic regulation of arachidonate metabolism in brain membrane phosphoglycerides. Lipids 14, 229–235. doi: 10.1007/bf02533874
Thépot, V., Mangott, A., and Pirozzi, I. (2016). Rotifers enriched with a mixed algal diet promote survival, growth and development of barramundi larvae. Lates calcarifer (Bloch). Aquacult. Rep. 3, 147–158. doi: 10.1016/j.aqrep.2016.02.003
Tocher, D. R. (2003). Metabolism and functions of lipids and fatty acids in teleost fish. Rev. Fish. Sci. 11, 107–184. doi: 10.1080/713610925
Tocher, D. R., Bendiksen, E. Å, Campbell, P. J., and Bell, J. G. (2008). The role of phospholipids in nutrition and metabolism of teleost fish. Aquaculture 280, 21–34. doi: 10.1016/j.aquaculture.2008.04.034
Toledo, J. D., Golez, M. S., Doi, M., and Ohno, A. (1999). Use of copepod nauplii during early feeding stage of grouper Epinephelus coioides. Fish. Sci. 65, 390–397. doi: 10.2331/fishsci.65.390
Trigueros, J. M., and Orive, E. (2001). Seasonal variations of diatoms and dinoflagellates in a shallow, temperate estuary, with emphasis on neritic assemblages. Hydrobiologia 444, 119–133.
Ubertini, M., Lefebvre, S., Gangnery, A., Grangeré, K., Le Gendre, R., and Orvain, F. (2012). Spatial variability of benthic-pelagic coupling in an estuary ecosystem: consequences for microphytobenthos resuspension phenomenon. PLoS One 7:e44155.
Uye, S., Iwai, Y., and Kasahara, S. (1983). Growth and production of the inshore marine copepod Pseudodiaptomus marinus in the central part of the inland sea of Japan. Mar. Biol. 73, 91–98.
Volkman, J. K., Jeffrey, S. W., Nichols, P. D., Rogers, G. I., and Garland, C. D. (1989). Fatty acid and lipid composition of 10 species of microalgae used in mariculture. J. Exp. Mar. Bio. Ecol. 128, 219–240.
Waqalevu, V., Honda, A., Matsui, H., Shiozaki, K., and Kotani, T. (2018). Proteolytic digestive enzyme response in Japanese flounder larvae Paralichthys olivaceus fed two types of rotifers Brachionus plicatilis species complex. Fish. Sci. 84, 1037–1049.
Watanabe, T., Izquiredo, M. S., Takeuchi, T., Satoh, S., and Kitajima, C. (1989). Comparison between eicosapentaenoic and docosapentaenoic acids in terms of essential fatty acid efficacy in larval red seabream. Nippon Suisan Gakkaishi 55, 1635–1640.
Keywords: coastal waters, copepod, DHA, fish larvae, lipids, microalgae, Pseudodiaptomus, rotifer
Citation: Matsui H, Sasaki T, Kobari T, Waqalevu V, Kikuchi K, Ishikawa M and Kotani T (2021) DHA Accumulation in the Polar Lipids of the Euryhaline Copepod Pseudodiaptomus inopinus and Its Transfer to Red Sea Bream Pagrus major Larvae. Front. Mar. Sci. 8:632876. doi: 10.3389/fmars.2021.632876
Received: 24 November 2020; Accepted: 06 January 2021;
Published: 09 February 2021.
Edited by:
Yen-Ju Pan, National Taiwan Ocean University, TaiwanReviewed by:
Kamil Mert Eryalçın, Istanbul University, TurkeyFrancisco Hontoria, Torre de la Sal Aquaculture Institute (IATS), Spain
Copyright © 2021 Matsui, Sasaki, Kobari, Waqalevu, Kikuchi, Ishikawa and Kotani. This is an open-access article distributed under the terms of the Creative Commons Attribution License (CC BY). The use, distribution or reproduction in other forums is permitted, provided the original author(s) and the copyright owner(s) are credited and that the original publication in this journal is cited, in accordance with accepted academic practice. No use, distribution or reproduction is permitted which does not comply with these terms.
*Correspondence: Tomonari Kotani, a290YW5pQGZpc2gua2Fnb3NoaW1hLXUuYWMuanA=