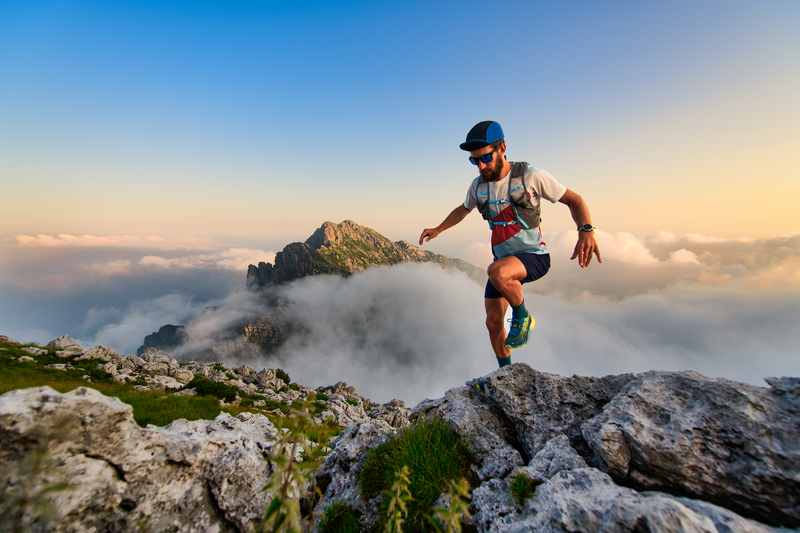
95% of researchers rate our articles as excellent or good
Learn more about the work of our research integrity team to safeguard the quality of each article we publish.
Find out more
REVIEW article
Front. Mar. Sci. , 16 March 2021
Sec. Marine Biotechnology and Bioproducts
Volume 8 - 2021 | https://doi.org/10.3389/fmars.2021.629629
This article is part of the Research Topic Marine Biotechnology, Revealing an Ocean of Opportunities View all 26 articles
Coastal countries have traditionally relied on the existing marine resources (e.g., fishing, food, transport, recreation, and tourism) as well as tried to support new economic endeavors (ocean energy, desalination for water supply, and seabed mining). Modern societies and lifestyle resulted in an increased demand for dietary diversity, better health and well-being, new biomedicines, natural cosmeceuticals, environmental conservation, and sustainable energy sources. These societal needs stimulated the interest of researchers on the diverse and underexplored marine environments as promising and sustainable sources of biomolecules and biomass, and they are addressed by the emerging field of marine (blue) biotechnology. Blue biotechnology provides opportunities for a wide range of initiatives of commercial interest for the pharmaceutical, biomedical, cosmetic, nutraceutical, food, feed, agricultural, and related industries. This article synthesizes the essence, opportunities, responsibilities, and challenges encountered in marine biotechnology and outlines the attainment and valorization of directly derived or bio-inspired products from marine organisms. First, the concept of bioeconomy is introduced. Then, the diversity of marine bioresources including an overview of the most prominent marine organisms and their potential for biotechnological uses are described. This is followed by introducing methodologies for exploration of these resources and the main use case scenarios in energy, food and feed, agronomy, bioremediation and climate change, cosmeceuticals, bio-inspired materials, healthcare, and well-being sectors. The key aspects in the fields of legislation and funding are provided, with the emphasis on the importance of communication and stakeholder engagement at all levels of biotechnology development. Finally, vital overarching concepts, such as the quadruple helix and Responsible Research and Innovation principle are highlighted as important to follow within the marine biotechnology field. The authors of this review are collaborating under the European Commission-funded Cooperation in Science and Technology (COST) Action Ocean4Biotech – European transdisciplinary networking platform for marine biotechnology and focus the study on the European state of affairs.
Marine environments provide a plethora of ecosystem services leading to societal benefits (Townsed et al., 2018). These services are mostly linked to supporting services (primary production and nutrient cycling), provisioning services (such as food) and cultural services, including tourism. The recent advancements of science and technology have facilitated the implementation of marine biotechnology, where marine organisms and their compounds are identified, extracted, isolated, characterized and used for applications in various sectors to benefit the society, ranging from food/feed to pharmaceutical and biomedical industries. Life in marine environments is diverse with wide environmental gradients in the physical, chemical, and hydrological parameters such as temperature, light intensity, salinity, and pressure. Marine organisms have adapted to these diverse environments by developing a broad spectrum of forms, functions, and strategies that play a crucial role for survival, adaptation and thriving in the multitude of these competitive ecosystems.
Among the vast array of evolutionary traits present in extant marine phyla, the production of biomolecules (secondary metabolites, enzymes, and biopolymers) is one of the most stimulating for biotechnology. Biomolecules mediate chemical communication between organisms, act as a protective barrier against adverse environmental conditions, serve as weapons for catching prey or for protection against predators, pathogens, extreme temperature or harmful UV radiation and are primordial in many other life-sustaining processes. Biomolecules have evolved to improve the organisms’ survival performance in their marine habitats and they are usually capable of exerting biological activity even at low concentrations to counteract dilution/dispersion effects occurring in the sea. The unique and complex structures of many marine metabolites enable the discovery of new and innovative applications with a commercial interest. Over 50% of the medicines currently in use originate from natural compounds, and this percentage is much higher for anticancer and antimicrobial treatment agents (Newman and Cragg, 2020). Apart from biomolecules, other properties and functions of marine organisms can also be beneficial and of interest to various industries, including the removal and degradation of individual chemical compounds or organic matter, as well as the development of intricate biochemical processes. However, marine resources remain largely underexplored and undervalorized.
Joint explorations of field and experimental biologists as well as chemists, supported by the recent advancements of techniques to access the ocean, fueled the increase in knowledge levels from the mid 20th century on. By the turn of the century, marine natural products chemistry became a mature and fully established subfield of chemistry with a focus on isolation and structure elucidation of secondary metabolites (Baslow, 1969; Faulkner, 2000; Gerwick et al., 2012).
Besides carrageenans or other polysaccharides that were extracted from seaweeds and widely used as food additives and cosmetic ingredients since the 1930s, modern marine biotechnology expanded after the 1970s with the intensification of research on marine organisms and their secondary metabolites (Rotter et al., 2020a). The first studies focused on natural products isolated from representative taxa inhabiting marine ecosystems like sessile macroorganisms including sponges, cnidarians, bryozoans, and tunicates, revealing a unique chemical diversity of bioactive metabolites (de la Calle, 2017). Multicellular organisms from all types of habitats were also reported to host complex and specialized microbiota (the holobiont concept, Margulis, 1991; Souza de Oliveira et al., 2012; Simon et al., 2019). These symbiotic microbial communities have major impacts on the fitness and function of their hosts and they contribute to the production of several secondary metabolites that play important roles against predators, pathogens or fouling organisms (Wilkins et al., 2019). This is especially true for soft-bodied, sessile organisms such as cnidarians and sponges, which are the best studied invertebrates and the most prolific source of bioactive molecules (Mehbub et al., 2014; Steinert et al., 2018). In fact, microorganisms make up approximately 40–60% of the sponge biomass (Yarden, 2014) and many bioactive molecules have been demonstrated or predicted to have a microbial origin (Gerwick and Fenner, 2013). Moreover, microorganisms represent nearly 90% of the living biomass in the oceans and are fundamental for the function and health of marine ecosystems by managing biogeochemical balances (de la Calle, 2017; Alvarez-Yela et al., 2019). They can produce a plethora of secondary metabolites with less stringent ethical and environmental requirements for research and product scale-up processes. Due to the broad range of manipulation possibilities, microorganisms are gaining importance for sustainable marine biotechnology and almost 60% of the new marine natural products nowadays are derived from microorganisms (Carroll et al., 2019). Nevertheless, macroorganisms still represent an active source and field of research for novel natural metabolites.
A simplified marine biotechnology workflow (Figure 1) depicts that product development from marine organisms is an inherently transdisciplinary and multidimensional task. The scientific community, industry, policy makers and the general public have a role to play in this process. Initially, the scientific community, while adhering to the ethical and legal guidelines, conducts systematic bioprospecting and screenings of marine organisms, elucidates the structure of bioactive molecules and their mechanisms of action, and sets up the protocols for product development. Often the financing of product development is dictated by the societal needs and challenges (such as health, well-being, or environmental protection) and suitable intellectual property (IP) protection. However, due to the transdisciplinary character of any biotechnology research, the co-design of processes and co-creation of knowledge is a necessary step in advancing the biodiscovery pipeline that ensures a faster product uptake. This also involves the collection of information about people’s perceived and actual needs, which is of paramount importance. Outreach activities are therefore conducted to engage the public in protecting the marine environment and promoting its sustainable use. Their feedback to both the scientific and industrial communities, either through directed questionnaires (in case of market research/analyses aiming to provide feedback on novel food, cosmeceuticals, and other products), workshops or other means of communication, often represents a key milestone for increasing consumers’ acceptance of bio-based products. Subsequent steps in new marine product development, e.g., scale-up, delivery format, validation of potency and toxicity, in vivo testing and implementation of statistically powered pre-clinical studies are generally performed by the pharmaceutical, biotechnological, biomedical, and food/nutraceuticals sectors.
Figure 1. A simplified representation of the marine biotechnology pipeline that is intrinsically interdisciplinary, combining basic and applied research with industry and business sectors.
The present article reviews some of the important aspects of marine biotechnology workflow. As marine biotechnology is an important contributor to bioeconomy, this concept is introduced in section “Bioeconomy.” In section “Marine biodiscovery areas,” we elaborate on hotspots of marine biodiscovery, from water column, the seafloor, microbial biofilms, beach wrack, to side streams. Section “Marine organisms and their potential application in biotechnology” introduces the main marine organisms being targeted for biotechnological research. Section “Methodology for exploration of marine bioresources” provides an overview of the general marine biotechnology pipeline and its key elements: organism isolation, data analysis and storage, chemical methods for isolation, and characterization of compounds. Production and scaling-up to guarantee sufficient supply at the industrial level are presented in section “Production upscaling.” Section “Use case scenarios” presents interesting use case scenarios where marine biotechnology can significantly address the societal challenges such as energy production, agronomy, bioremediation, food, feed, cosmetics, bio-inspired materials, and pharmaceuticals. Furthermore, the legislative and ethical issues arising from the development of marine biotechnology should not be overlooked and they are presented in section “Legislation and funding.” Section “Communication and stakeholder engagement in development finalization” concludes with a discussion on the importance of science communication both to raise consumer awareness on new products and establish new collaborations on one hand and implement knowledge transfer channels with stakeholders from the industrial, governmental and public sectors, on the other hand. The establishment of efficient communication that enables productive collaboration efforts is essential for the market entry and successful commercialization of marine biotechnology products. We conclude with an overview of the marine biotechnology roadmap in Europe.
Marine biotechnology is recognized as a globally significant economic growth sector. The field is mostly concentrated in the European Union (EU), North America and the Asia-Pacific (Van den Burg et al., 2019). Some of the globally renowned marine biotechnology centers are in China (e.g., the Institutes of Oceanology, the institutes of the Chinese Academy of Sciences), Japan (e.g., Shimoda Marine Research Center), United States (e.g., Scripps Institute of Oceanography), Australia (e.g., Australian Institute of Marine Science). In other countries, marine biotechnology is an emerging field to address global economic challenges, such as in South America (Thompson et al., 2018), Middle East (Al-Belushi et al., 2015) and Africa (Bolaky, 2020). However, the proper implementation of the field has its limitations: the lack of investment, need for appropriate infrastructure and human capital (Thompson et al., 2017, 2018). To enable the development of the marine bioeconomy, national or global partnerships with the leading research labs are established (Vedachalam et al., 2019), including European participation.
The European Commission defines blue bioeconomy as an exciting field of innovation, turning aquatic biomass into novel foods, feed, energy, packaging, and other applications1. This is also reflected in the revised EU Bioeconomy Strategy2, setting several priorities that are relevant for marine biotechnology, such as developing substitutes to plastics and to other fossil-based materials that are bio-based, recyclable and marine-biodegradable. In the European Union, blue economy (including all the sectors) reached €750 billion turnover and employed close to 5 million people in 2018 (European Commission, 2020). Marine biotechnology is a niche within the ocean-based industries. As this is a growing field, it is projected that in 2030 many ocean-based industries will outperform the growth of the global economy as a whole, providing approximately 40 million full-time equivalent jobs (Rayner et al., 2019). Besides creating new jobs, the development of marine biotechnology can contribute to the existing employment structures by diversification of additional income for fishermen or aquaculture specialists. In this aspect, the policy making sector is aware that future marine research priorities should include improved techniques for mass production and processing of marine biomass (European Commission, 2019). This imposes several challenges: (i) the need for harmonization and later standardization of processes, protocols and definitions; (ii) the need to establish ethical guidelines that will be endorsed and respected by national administrative authorities and concern the fair share and use of biological resources; (iii) the need to bridge the collaboration and communication gap between science and industry on one hand, and policy makers on the other. This entails some changes in the mode of action. For example, networking activities such as brokerage events and participatory workshops should be used for the exchange of expertise, opinion and potential co-creation of strategic documents. (iv) There is a need to develop strategies for showcasing individual expertise, such as the creation of open access repositories of experts and their contacts. (v) Finally, there is a need to sustain the investment into ocean observations that provide evidence of regulatory compliance and support the valuation of natural assets and ecosystem services (Rayner et al., 2019). Open science, also through full access to research publications (embraced by Plan S and supported by the EC3) and access to data are of key importance here, enabling fair access to public knowledge.
The marine environment with its unique physico-chemical properties harbors an extraordinary source of yet undiscovered organisms (Figure 2) and their chemical/biochemical compounds to develop commercially interesting bio-based products.
Since the early 21st century, the exploration of marine microbial biodiversity has been driven by the development of high-throughput molecular methods such as High Throughput Sequencing (HTS), and their direct application on intact seawater samples without requiring any prior isolation or cultivation of individual microorganisms (Shokralla et al., 2012; Seymour, 2019). The HTS approach utilizes specific gene regions (barcodes) to provide massive amounts of genetic data on the various microbial communities with continuing improvements in data quality, read length and bioinformatic analyses methods, leading to a better representation of the genetic based taxonomic diversity of the sample. HTS methods detect both the most abundant community members but also the rare species, which cannot otherwise be retrieved by traditional culture-dependent methods. The Global Ocean Sample Expedition (GOS4) led by J. Craig Venter Institute is exemplary for that. Recently, the most important step forward in elucidating world-wide eukaryotic biodiversity were cross-oceanic expeditions such as Malaspina, Tara Oceans, and Biosope (Grob et al., 2007; Claustre et al., 2008; Bork et al., 2015; Duarte, 2015; de Vargas et al., 2015). These studies have also confirmed that much of the eukaryotic plankton diversity in the euphotic zone is still unknown and has not been previously sequenced from cultured strains. The Tara Oceans dataset also provided significant new knowledge to protistan diversity, identifying many new rDNA sequences, both within known groups and forming new clades (de Vargas et al., 2015).
The isolation of DNA from environmental samples – eDNA – is already well established in the field of microbiology and marine monitoring (Diaz-Ferguson and Moyer, 2014; Pawlowski et al., 2018). Metabolic engineering is being used for characterization of bacterial communities in sediments since the 1980’s (Ogram et al., 1987) and monitoring entire microbial populations in seawater samples (Venter et al., 2004). However, this approach has been applied to the analyses of macroorganisms only in recent years (Thomsen et al., 2012; Kelly et al., 2017; Jeunen et al., 2019). The study of eDNA in the water column (Keuter et al., 2015) or in seafloor sediments (Keuter and Rinkevich, 2016) has received considerable attention for high-throughput biomonitoring using metabarcoding of multiple target gene regions, as well as for integrating eDNA metabarcoding with biological assessment of aquatic ecosystems (Pawlowski et al., 2018). In addition, targeted detection of species using quantitative Polymerase Chain Reaction (qPCR) assays have been implemented for both micro- and macroorganisms (Gargan et al., 2017; Hernández-López et al., 2019) in seawater samples. The potential for using eDNA in aquatic ecosystems has been the topic of several concerted actions (like DNAqua-Net COST Action5; Leese et al., 2016) and there have been considerable efforts to standardize DNA approaches to supplement or replace the existing methods, often dictated by regulatory frameworks such as the European Union Water Framework Directive– EU WFD [Directive 2000/60/EC] and the Marine Strategy Framework Directive – EU MSFD [Directive 2008/56/EC]. In-depth knowledge on the biodiversity of marine microorganisms has been substantially improved with the adaptation of various DNA barcoding protocols and approaches (Leese et al., 2016; Paz et al., 2018; Weigand et al., 2019). Although metagenomic data generated from eDNA samples can provide vast amounts of new information, datasets from complex microbial communities are difficult to process. Metagenome assemblies and their functional annotations are challenging (Dong and Strous, 2019). A large fraction (>50%) of the detected genes has no assigned function. The use of functional metagenomics applications and tools (including metagenomics coupled with bioactivity screening/enzyme screening, meta-transcriptomics, meta-proteomics, meta-metabolomics), is key to solve this problem. One of the most used applications of functional metagenomics is the activity-based screening of metagenomics libraries. Enzyme discovery is currently the largest field of its use, including marine enzymes (Hårdeman and Sjöling, 2007; Di Donato et al., 2019).
High throughput sequencing offers a culture-independent characterization of microbial diversity, but the biotechnological exploitation of marine microorganisms often requires their cultivation in pure cultures and the optimization of production yield for the compounds of interest. It is estimated that over 85% of microorganisms are unculturable under the current laboratory cultivation techniques (Wade, 2002; Lloyd et al., 2018). This is the “great plate anomaly,” a consequence of the difficulties in mimicking the complexity of natural habitats in the laboratory (i.e., cultivations under finely tuned conditions for various parameters, such as temperature, salinity, oxygen, agitation and pressure, and use of species-specific growth substrates with trace elements). Additionally, cell-to-cell interactions, occurring both between symbiotic and competing organisms in the natural environment may be absent when pure cultures (axenic) are grown in laboratory conditions (Joint et al., 2010). Other strategies, like mimicking in situ nutritional composition and physico-chemical conditions as well as the addition of signaling molecules are used to increase the diversity of isolated marine microorganisms (Bruns et al., 2003). It is however important to realize the limitations of methods and tools used in culture-based techniques. For example, the sampling and isolation of bacteria from seawater relies on filters with specific retention characteristics (e.g., 0.22 micron pore size), that typically fail to capture the smaller bacteria (Hug et al., 2016; Ghuneim et al., 2018). Hence, the establishment of pure microbial cultures remains one of the main hurdles in the discovery of bioactive constituents in microorganisms. To increase the number of harnessed microbes, a new generation of culture approaches has been developed that mimic the proximity of cells in their natural habitat and exploit interspecific physiological interactions. Several techniques, such as diffusion chambers (Kaeberlein et al., 2002), the iChip (Nichols et al., 2010), as well as the recently developed miniaturized culture chips (Chianese et al., 2018) have been employed to address microbial cultivation problems. Another reason preventing the wider valorization of marine microorganisms is that some strains cannot exist in nature without their symbionts: often microorganisms that are associated or in symbiosis with marine macroorganisms are the true metabolic source of marine natural products (McCauley et al., 2020). To uncover the potential of these species, many microbiological studies are focusing on bulk-community dynamics and how the performance of ecosystems is supported and influenced by individual species and time-species interaction (Kouzuma and Watanabe, 2014). Such advancements in the field of microbial ecology provide robust background knowledge for biotechnological exploitation. Hence, as natural communities are complex and difficult to assess, characterize and cultivate in artificial conditions, researchers use synthetic microbial communities of reduced complexity in their laboratory studies. These artificial communities are prepared by retaining only the microorganisms carrying out a specific biosynthetic process, as well as those providing culture stability and performance, to enable their use in biotechnological applications (Großkopf and Soyer, 2014).
To maximize the biotechnological potential of our oceans it is essential to exploit microbial communities with their complex networking systems engaged in cooperation and competition. Currently, the integrative omics approach provides comprehensive information describing the community through sophisticated analyses from genes to proteins and metabolites. Shotgun metagenome analysis allows the sequencing of genomes of the dominant organisms to link the enzymes of interest directly to organisms. Functional metagenomics is an excellent tool for studying gene function of mixed microbial communities, with a focus on genomic analysis of unculturable microbes and correlation with their particular functions in the environment (Lam et al., 2015). The conventional approach to construction and screening of metagenomic libraries, which led to the discovery of many novel bioactive compounds as well as microbial physiological and metabolic features, has been improved by sequencing of complete microbial genomes from selected niches. Metatranscriptomics and metaproteomics, important for further functional analysis of microbial community composition, may indicate their role in many crucial processes such as carbon metabolism and nutrition acquisition (Shi et al., 2009).
The development of sequencing, computational and storage capacities reduced the financial and time investment needed for new discoveries of biotechnological interest. This is now enabled with genome mining, the process of extracting information from genome sequences to detect biosynthetic pathways of bioactive natural products and their possible functional and chemical interactions (Trivella and de Felicio, 2018; Albarano et al., 2020). It is used to investigate key enzymes of biosynthetic pathways and predict the chemical products encoded by biosynthetic genes. This can reveal the locked bioactive potential of marine organisms by utilizing the constantly evolving sequencing technologies and software tools in combination with phenotypic in vitro assays. This approach can facilitate natural products discovery by identifying gene clusters encoding different potential bioactivities within genera (Machado et al., 2015). These bioactive molecules are usually synthesized by a series of proteins encoded by biosynthetic gene clusters (BGCs) which represent both a biosynthetic and an evolutionary unit. The complete genome sequence of several marine microorganisms revealed unidentified BGCs even in highly explored species, indicating that the potential of microorganisms to produce natural products is much higher than that originally thought (Lautru et al., 2005). BGCs are mostly classified based on their product as: saccharides, terpenoids, polyketide synthases (PKSs), non-ribosomal peptide synthetases (NRPSs), and ribosomally synthesized and post-translationally modified peptides (RiPPs). PKS and NRPS have long been attractive targets in genome mining for natural products with applications in medicine. For example, salinilactam A was the first compound spotted by genome mining from a marine-derived actinomycete (Zerikly and Challis, 2009) and the angucyclinones, fluostatins M–Q, produced by Streptomyces sp. PKU-MA00045 were isolated from a marine sponge (Jin J. et al., 2018). In parallel, a recent increase in discoveries of novel RiPP classes revealed their bioactive potential in the biomedical field, e.g., as novel antibiotics (Hudson and Mitchell, 2018). Thus, different methodologies are currently available for RiPP genome mining, focused on known and/or novel classes finding, including those based on identifying RiPP BGCs, or identifying RiPP precursors using machine-learning classifiers (de los Santos, 2019; Kloosterman et al., 2021).
With the development of in situ molecular tools (such as fluorescence in situ hybridization – FISH), it is possible to quantify and follow the dynamics of specific bacterial groups (Pizzetti et al., 2011; Fazi et al., 2020). Such tools may also help to uncover whether microbial communities with explicit characteristics can favor biosynthesis processes for specific compounds of interest.
Spatial and temporal distribution patterns, as well as the actual abundance of phytoplankton, bacterioplankton and viruses are defined by a variety of physico-chemical parameters (e.g., light availability, nutrient supply, temperature, water column stratification) and biological processes (e.g., microbial activity, competition, zooplankton grazing pressure, viral lysis) (Field et al., 1998; Kirchman, 2000; Mousing et al., 2016). Seafloor provides diverse habitats for organisms. Besides forming stromatolites in the sediments surface, cyanobacteria can secrete or excrete extracellular polymeric substances (EPS) (Golubic et al., 2000) that can further stabilize sediments and protect them from antimicrobial compounds and other various biotic and abiotic stressors (Costa et al., 2018). Ocean sediments cover the greatest portion of the Earth’s surface and host numerous bacterial phyla and individual species, the biosynthetic capabilities of which are largely unknown. This is particularly true for the poorly accessible deep seas. As an example, phylogenetic studies from the ultra-oligotrophic eastern Mediterranean Sea have shown that sediments down to almost 4,400 m depth host a vast diversity of bacteria and archaea (Polymenakou et al., 2015). More importantly, a large fraction of 16S rDNA sequences retrieved from the same environment (∼12%) could not be associated with any known taxonomic division, implying the presence of novel bacterial species (Polymenakou et al., 2009). Moreover, the OceanTreasures expedition revealed new and diverse actinobacteria strains adapted to life in the ocean (i.e., marine obligates) with biotechnological potential and other bacteria that are capable of producing exopolysaccharides, obtained from sediments collected off the Madeira Archipelago, Portugal, down to 1,310 m in depth (Prieto-Davó et al., 2016; Roca et al., 2016).
Given that the world’s ocean average depth is 2,000 m, the advent of diving and vessel-operated sampling equipment and techniques, such as dredges, collectors and remotely operated vehicles (ROVs), allowed exploring previously inaccessible environments (such as hydrothermal vents and deep sea), opening new prospects and horizons for marine biotechnology. The exploration of these extreme environments requires specific equipment, skills, and resources for long cruises, which include systematic mapping, especially in the deep ocean and seabed.
Submarine caves were recently recognized as biodiversity hotspots (Gerovasileiou and Voultsiadou, 2012; Gerovasileiou et al., 2015), with most of them being largely unexplored. The organisms hosted in these environments are of particular biotechnological interest. A range of mesophilic and thermotolerant microorganisms have been reported from submarine caves and cavities, characterized by elevated concentrations of hydrogen sulfide (Canganella et al., 2006), while methanogenic and sulfate reducing microbial species with potential applications in biogas production and bioremediation have been isolated from similar environments (Polymenakou et al., 2018). Besides biogas production, the biotechnological interest of bacteria originating from unique submarine caves could be even greater in terms of their secondary metabolites.
Biofilm formation is an important life strategy for microorganisms to adapt to the wide range of conditions encountered within aquatic environments (Figure 2). Biofilms are complex and dynamic prokaryotic and eukaryotic microbial communities. Besides being a novel species bank of hidden microbial diversity and functional potential in the ocean, biofilms are increasingly being recognized as a source of diverse secondary metabolites (Battin et al., 2003; Zhang W. et al., 2019). Scarcity of some microbial species in seawater prevents their capture in global sequencing efforts and they can only be detected when their relative abundance increases during biofilm formation. The biofilm microbiome can be analyzed by metagenomic approaches such as Illumina and visualized by microscopic imaging techniques such as CARDFISH (Parrot et al., 2019). Mass spectrometry imaging (MSI) techniques, such as DESI-MSI allows the identification and spatial distribution and localization of marine microbial natural products in the biofilm (Papazian et al., 2019; Parrot et al., 2019).
The main component of biofilms, accounting for up to 90% of the dry biomass, are EPS, which comprise polysaccharides, proteins, extracellular DNA, lipids and other substances, forming a complex 3-D structure that holds the cells nearby (Flemming and Wingender, 2010). This matrix of EPS keeps the biofilm attached to the colonized surface (Battin et al., 2007; Flemming and Wingender, 2010). Moreover, the dense EPS matrix provides a protective envelope against biocides and antibiotics produced by other (micro)organisms, and physical stress. Exopolysaccharides can remain bound to the cell surface or be directly released into the aquatic environment. The assessment of the distribution of specific microbes within the biofilm and their exopolysaccharide composition is therefore of crucial importance for biotechnological applications (Pereira et al., 2009; Lupini et al., 2011; Fazi et al., 2016). EPS composition varies greatly depending on the microorganisms present and environmental conditions (e.g., shear forces, temperature, nutrient availability, Di Pippo et al., 2013). This compositional variability suggests the contribution of various biosynthetic mechanisms, that are, in turn, dependent on environmental and growth conditions (De Philippis and Vincenzini, 2003; Pereira et al., 2009). EPS are responsible for adhesion of microorganisms to surfaces, aggregation of microbial cells, cohesion within the biofilm, retention of water, protection, and enzymatic activity (Žutić et al., 1999). Furthermore, they are important for the absorption of organic and inorganic compounds, exchange of genetic material, storage of excess carbon and they can serve as a nutrient source (Flemming and Wingender, 2010). These features can be exploited in different applications. The biodegradable and sustainable carbohydrate-based materials in biofilms provide mechanical stability and can be exploited as packaging materials, films, prosthetics, food stabilizers and texturizers, industrial gums, bioflocculants, emulsifiers, viscosity enhancers, medicines, soil conditioners, and biosorbents (Li et al., 2001; Roca et al., 2016). Furthermore, the extracellular proteins and enzymes can find interesting applications in medical biofilm dispersion or bioconversion processes. In addition, EPS contain biosurfactants with antimicrobial activity and extracellular lipids with surface-active properties that can disperse hydrophobic substances, which can be useful for enhanced oil recovery or bioremediation of oil spills (Baldi et al., 1999; Flemming and Wingender, 2010). Understanding the molecular mechanisms behind various secondary metabolites that are used for interspecies communication within biofilms will also be useful in combating the biofilm-forming pathogenic bacteria (Barcelos et al., 2020).
Biofilms are involved in marine biofouling and defined as the accumulation of microorganisms, algae and aquatic animals on biotic and abiotic surfaces, including human-made structures that are immersed in seawater (Amara et al., 2018). The environmental impact of biofouling is significant due to the reduction of the water flow and the increase of debris deposition below the aquaculture farms (Fitridge et al., 2012). Biofouling can seriously affect the environmental integrity and consequently impact the worldwide economy. Ships’ hulls can increase fuel consumption by up to 40% due to the increased drag and weight of the ships (Amara et al., 2018) and can also increase ship propulsion up to 70% (Trepos et al., 2014), resulting in a rise in carbon dioxide and sulfur dioxide emissions by 384 and 3.6 million tons per year, respectively (Martins et al., 2018). The transport delays, the hull repairs and the biocorrosion cost an additional 150 billion dollars per year (Schultz, 2007; Hellio et al., 2015). Moreover, ships might transport invasive fouling species, thus threatening indigenous aquatic life forms (Martins et al., 2018). The use of antifouling paints incorporating biocides like heavy metals and tributyltin was the chemical answer to the fouling issue, but these substances are known to leach into the water and pose deleterious effects to many non-target species (Yebra et al., 2004; Amara et al., 2018). Marine natural products, originating from bacteria as well as higher organisms, can represent an environmentally friendly alternative to synthetic biocides (Eguía and Trueba, 2007; Adnan et al., 2018; Bauermeister et al., 2019) and an economically advantageous solution to foul-release polymers (Trepos et al., 2014; Chen and Qian, 2017; Pereira et al., 2020). In particular, the compounds that inhibit quorum sensing signals that regulate the microbial colonization and formation of aggregates could mitigate the impact of biofilms (de Carvalho, 2018; Salehiziri et al., 2020).
Biofilms are useful as a core part of the nitrification bioreactors, operated in recirculating aquaculture systems and are called biofilters, as they convert excreted ammonia from animals to nitrates. This restores healthy conditions for farmed fish and shrimp. Aquaculture biofilms have a complex microbiome, the composition of which varies depending on the farmed species and culture conditions. However, these biofilms can also harbor pathogens that can be harmful for farmed fish. Therefore, careful manipulation of microbial communities associated with fish and their environment can improve water quality at farms and reduce the abundance of fish pathogens (Bentzon-Tilia et al., 2016; Bartelme et al., 2017; Brailo et al., 2019).
Beach wrack (Figure 2) consists of organic material (mainly seagrasses and seaweeds) washed ashore by storms, tides, and wind. According to the European Commission Regulation (EC) No 574/2004 and the European Waste Catalog (EWC), waste from beaches is defined as “municipal wastes not otherwise specified” (Wilk and Chojnacka, 2015). Therefore, specific regulations within each country are implemented concerning the collection of municipal solid waste (Guillén et al., 2014) which typically results in large amounts of unexploited beach wrack. Moreover, the removal of wrack eliminates valuable nutrients that may affect sandy beach and dune ecosystem’s food chains, and it can cause a reduction in species abundance and diversity (Defeo et al., 2009). For example, the role of Posidonia oceanica “banquettes” (dead leaves and broken rhizomes with leaf bundles) is fundamental in protecting beaches from erosion, and its removal can have a dramatic negative impact on P. oceanica ecosystem services, including the conservation of beaches (Boudouresque et al., 2016). On the other hand, a high deposition of beach cast can be encountered from the blooms of opportunistic macroalgae as a result of elevated nutrient loading. In such cases, a removal of beach cast is seen as a mitigation action to remove excess nutrients from the marine environments (Weinberger et al., 2019). The collected beach wrack and its chemical constituents could find use in interesting biotechnological applications, but such harvesting interventions should also take into account the ecological role that algae and plant debris play in the coastal ecosystems (Guillén et al., 2014). Collection and handling methods should therefore be tailored to fit the characteristics of each coastal system, including the type of the dominant species and the quantities of biomass. Solutions should then be verified from economical, technical, and environmental perspectives.
As beach wrack often includes contaminants, the decay of this biomass can lead to the reintroduction of toxic pollutants into the aquatic environment. Before the implementation of industrial agriculture practices in the 20th century, beach wrack was used in Europe as a fertilizer (Emadodin et al., 2020). The high salt content was reduced by leaving the biomass on the shore for weeks to months so that the salt could be washed out by the rain. The implementation of adapted recycling methods should be included for further processing of wrack biomass before its removal from the coastal ecosystem. Besides high salt content, sand removal is another obstacle for the immediate utilization of beach wrack. New technologies, including the magnetic modification of marine biomass with magnetic iron oxide nano- and microparticles and its subsequent application for the removal of important pollutants can be considered (Angelova et al., 2016; Safarik et al., 2016, 2020).
Coastal biomass displays good biotransformation potential for biogas, which is comparable to higher plants containing high amounts of lignin. Besides biogas production, the bioactive substances of this biomass (mainly exopolysaccharides) can be used to develop cosmetics, as well as for pharmaceutical and biomedical products (Barbot et al., 2016). This biomass may also be used for the removal of various types of contaminants by biosorption (Mazur et al., 2018). Hence, there is interest in the adoption of circular economy principles for the sustainable management of coastal wrack biomass. This can enable the production of valuable solutions, while at least partially substituting the use of fossil fuels, the use of electricity at a biogas plant and the use of chemical fertilizers (using degassed or gasified/torrefied biomass), thus avoiding “dig and dump” practices and landfill emissions. The implementation of these methods can also contribute to local small-scale energy unit development in coastal regions (Klavins et al., 2019).
Fish represent the main commodity among marine resources; of the 179 million tons of global production over 85% was used for direct human consumption in 2018 (FAO, 2020). Due to the lack of adequate infrastructures and services to ensure proper handling and preservation of the fish products throughout the whole value chain, 20–80% of marketed fish biomass is discarded or is wasted between landing and consumption (Gustavsson et al., 2011; Ghaly et al., 2013). In addition, large quantities of marine by-products are generated mainly as a result of fish and shellfish processing by industrial-scale fisheries and aquaculture (Ferraro et al., 2010; Rustad et al., 2011; Radziemska et al., 2019). Besides fish, the phycocolloid industry also generates considerable amounts of seaweed by-products that are good sources of plant protein, cellulosic material and contain taste-active amino acids. They can also be used in food flavoring (Laohakunjit et al., 2014), animal feed (Hong et al., 2015) or as feedstock biomass for bioenergy (Ge et al., 2011).
The by-products are often treated as waste, despite containing valuable compounds. Direct composting and combustion should be avoided in favor of recovery of valuable compounds. The biorefinery concept integrates biomass conversion processes and equipment to produce value-added chemicals, fuels, power and heat from various types of side stream (waste) biomass. In this context, marine biorefinery employs recycling/reutilization of marine waste biomaterials to produce higher-value biologically active ingredients/components, such as minerals, proteins, peptides, lipids, long-chain ω-3 fatty acids, enzymes, and polysaccharides (Nisticò, 2017; Kratky and Zamazal, 2020; Kumar et al., 2020; Prabha et al., 2020). The processed leftovers from fish and shellfish typically include trimmings, skins and chitin residues from crustacean species, heads, frames (bone with attached flesh) and viscera. Only a small portion of these cut-offs is further processed, mostly into fish meal and fish oil, while a part is processed to extract value-added compounds that can be used in the pharmaceutical and nutraceutical products (Senevirathne and Kim, 2012) for prevention and management of various disease conditions, such as those associated with metabolic syndromes (Harnedy and FitzGerald, 2012). Extracted collagen can be used for wound healing dressings, drug delivery, tissue engineering, nutritional supplement, as an antibiofilm agent, or as an ingredient in cosmetics and pharmaceutical products (Abinaya and Gayathri, 2019; Shalaby et al., 2019). Gelatin and chondroitin have applications in the food, cosmetic and biomedical sectors. Chitin and chitosan resulting from shellfish are other examples of marine by-products with applications in the food, agriculture and biomedicine sectors (Kim and Mendis, 2006). Hydroxyapatite derived from fish bone has demonstrated relevance for dental and medical applications (Kim and Mendis, 2006) and can also be used as feed or fertilizers in agriculture or horticultural use. Cephalopod ink sac is considered a by-product that is discharged by most processing industries (Hossain et al., 2019). However, the ink from squid and cuttlefish can be a potential source of bioactive compounds, such as antioxidant, antimicrobial, or chemopreventive agents (Smiline Girija et al., 2008; Zhong et al., 2009; Shankar et al., 2019). Moreover, microbes that live in fishes’ slimy mucus coating can be explored as drug-leads for the pharmaceutical industry (Estes et al., 2019). The main challenge for the valorization of all these by-products is their very short shelf-life, implying that the processing steps should be fast enough to prevent oxidation and microbial degradation.
All groups of marine organisms have the potential for biotechnological valorization (Figure 2). Table 1 presents the different groups of marine organisms and their main biotechnological applications that are currently being developed, while a more detailed discussion on this topic follows in subsequent sections.
Table 1. A non-exhaustive example list of the most prominent marine taxa, their use and challenges toward production scale-up.
Most initial studies on marine natural products focused on marine metazoans such as sponges, cnidarians, gastropods and tunicates, as they were representative organisms of the studied marine ecosystems and relatively easy to collect by scuba diving (reviewed in Molinski et al., 2009). These sessile organisms with limited motility tend to produce a vast assemblage of complex compounds with defensive (e.g., antipredatory, antifouling) or other functional properties such as communication or chemoreception (Bakus et al., 1986). The first described drugs from the sea that were used in clinical trials decades after their discoveries were vidarabine (ara-A®) and cytarabine (ara-C®), two chemical derivatives of ribo-pentosyl nucleosides extracted in the 1950s from a Caribbean marine sponge, Tectitethya crypta (Bergmann and Feeney, 1951). Other early drugs originating from the sea were ziconotide, a synthetic form of ω-conotoxin, extracted from the Pacific cone snail Conus magus and commercialized under the trade name Prialt® for the treatment of chronic pain (Olivera et al., 1985; Jones et al., 2001), ω-3 acid ethyl esters (Lovaza®) from fish for hyperlipidemia conditions, the anticancer drugs Eribulin Masylate (E7389) macrolide marketed as Halaven®, and Monomethyl auristatin E derived from dolastatin peptides isolated from marine shell-less mollusk Dolabella auricularia commercialized as Adcetris® (Jimenez et al., 2020). In 2007, about 40 years after its initial extraction from the Caribbean ascidian Ecteinascidia turbinata, ecteinascidin-743 (ET-743), also known as trabectedin or its trade name Yondelis®, was the first marine-derived drug to be approved for anticancer treatments (Rinehart et al., 1990; Corey et al., 1996; Martinez and Corey, 2000; Aune et al., 2002). Multiple strategies were tested to produce the drug at the industrial scale, including mariculture and total synthesis, and eventually a semisynthetic process starting from cyanosafracin B, an antibiotic obtained by fermentation of Pseudomonas fluorescens, solved the problem (Cuevas et al., 2000; Cuevas and Francesch, 2009). Since then, the discovery of marine-derived compounds extracted from metazoans is expanding (Molinski et al., 2009; Rocha et al., 2011) and sponges and cnidarians are the most prominent as far as novel marine natural products discovery is concerned (Qi and Ma, 2017; Blunt et al., 2018; Carroll et al., 2020). The annual number of new compounds reported for each is consistently high at approximately 200 along the past decade, while the respective number for other widely investigated marine phyla, such as mollusks, echinoderms and tunicates (subphylum Chordata), is limited between 8 and 50 for the same period (Carroll et al., 2020). Bryozoans are acknowledged as an understudied group of marine metazoans with regard to metabolites production, with the newly discovered natural products fluctuating from zero to slightly over 10 in the last few years (Blunt et al., 2018; Carroll et al., 2020). Those pronounced differences in marine product discovery either reflect an innate trend toward the production of complex secondary metabolites (Leal et al., 2012) or the study effort toward a phylum or another taxonomic group, often due to the lack of taxonomic expertise for some groups. Yet, the differences in discovered marine products do not appear to reflect the biodiversity included within each group, since sponges, cnidarians, bryozoans, and echinoderms each include a comparable number (6,289–11,873) of accepted species (Costello and Chaudhary, 2017; WoRMS Editorial Board, 2020). At the same time, mollusks are one of the richest marine metazoan phyla in terms of biodiversity with 48,803 extant species (WoRMS Editorial Board, 2020) but a poor source of novel marine products (17 new metabolites in 2017, according to Carroll et al., 2020). While sponges and cnidarians, in particular corals, can be cultured on a large-scale to support drug discovery (Duckworth and Battershill, 2003; Leal et al., 2013), the provision of marine invertebrates in sufficient quantities for implementing a battery of activity tests on their natural products is challenging as sustainable supply has always been a bottleneck.
Sponges are prominent candidates for bioproduction-oriented cultivation, due to their simple body plan and regenerative capacity, and their richness in bioactive substances. While sponge farming in the open sea has been proposed (and often accomplished) as an effective strategy to resolve the supply bottleneck and ensure sustainable production of sponge-derived compounds (Duckworth, 2009), it is still limited by the necessity to destructively collect primary material from wild populations in various phases of the cultivation. Little progress has been made toward “closed life cycle cultivation,” i.e., successfully inducing reproduction and recruitment of larvae to the aquaculture (Abdul Wahab et al., 2012). Cell culturing can provide advantages in terms of control of desirable biological characteristics and fine-tuning of production (Sipkema et al., 2005), while recent advances have shown potential for the establishment of sponge cell lines and the development of sustainable processes to produce sponge metabolites (Pérez-López et al., 2014; Conkling et al., 2019).
Since many marine invertebrates host massive associations with microbes, the origin of detected metabolites can be the organism itself, its symbionts (e.g., bacteria) or the food source (e.g., algae) of the metazoan (Loureiro et al., 2018). Hence, metazoans can act as concentrators of natural compounds. Symbionts can produce molecules of biotechnological interest, e.g., enzymes or polyhydroxyalkanoates – PHAs (Bollinger et al., 2018). The antitumor depsipeptide kahalalide F (Shilabin and Hamann, 2011; Salazar et al., 2013), produced by the alga Bryopsis spp. at very low concentrations, was originally identified from the sea slug Elysia rufecens that feed on Bryopsis algae and accumulate this natural compound at 5,000 fold higher concentrations (Hamann et al., 1996). The cultivation of particular metazoan-associated microorganisms is thus becoming a promising opportunity to discover new metabolites (Esteves et al., 2013).
Seaweeds, or marine macroalgae gained wide interest in recent years due to the opening of new applications and markets (i.e., biofuel, feed and food supplements, food ingredients, nutraceuticals, and cosmetics), which contributed to the development of aquaculture on several species (Stévant et al., 2017). The term “seaweed” includes macroscopic and multicellular marine red, green, and brown algae. Seaweeds are a rich source of proteins, minerals, iodine, vitamins, non-digestible polysaccharides, and bioactive compounds with potential health benefits. They are present in almost all shallow water habitats. Like phytoplankton, the distribution patterns of seaweed species are controlled by several abiotic environmental factors and biological interactions. Dense beds of large brown seaweeds (i.e., Fucus and Sargassum species) are frequently found together with red and green algae of smaller size. The competition for space between species is strong, and the removal of one species can impact the structure of the whole community. However, invasive species can be valorized for biotechnological applications and in some areas this has showed promising results and has been already used for skincare products (Rotter et al., 2020b). By harvesting invasive seaweeds, the adverse effects on local biodiversity can also be mitigated. In addition to their nutritional value, seaweeds exhibit antimicrobial, immunostimulatory and antioxidant properties (Gupta and Abu-Ghannam, 2011). Due to the growing demand for marine proteins and lipids in the fishfeed industry over the last 20 years, the use of seaweed extracts as prophylactic and therapeutic agents in shellfish and fish aquaculture has become increasingly popular (Vatsos and Rebours, 2015). There are also other macroalgal biomolecules (mainly polysaccharides and carotenoids) that can be used as functional food, nutraceuticals and cosmeceuticals. In 2016 alone, over 32.8 million tons of seaweed were produced from capture and aquaculture worldwide, and the seaweed production increased around 10% annually in the last 3 years, mainly due to an increase in the aquaculture sector (European Commission, 2020). Globally, over 95% of seaweeds are produced through aquaculture (FAO, 2020) with Asian countries being the leaders in this activity, whereas the European seaweed production is primarily based on the harvesting of natural resources (Ferdouse et al., 2018; FAO, 2020), primarily kelp (Laminariales) and in smaller volumes knotted wrack (Ascophyllum nodosum) for production of alginates and seaweed meals or extracts, respectively. Saccharina latissima is presently the species that is cultivated in Europe on a large scale. The yield of the active substances extracted from seaweed is ranging from less than 1% up to 40% of the dry algal mass, depending on various factors, such as target metabolite, species and season (Pereira and Costa-Lotufo, 2012). To increase the value of commercial seaweeds it is important to study the remaining biomass after the extraction of the target substances and find new strategies for its further valorization, for example with the Gelidium sesquipedale and Pterocladiella capillacea biomass remaining after agar extraction (Matos et al., 2020). Understanding the physiological effects of seaweeds or seaweed extracts is a complicated task; similarly to other marine organisms their properties differ depending on the geographical origin and season of harvest. The extraction method of the bioactive components can affect the efficacy of the final extracts. The feasibility of using any of these extracts on a commercial scale needs to be examined to define the extraction cost and reveal how the extracts can be delivered under intensive farming conditions. It is finally worth mentioning that seaweeds can act as habitats for endophytes (Flewelling et al., 2015; Manomi et al., 2015; Mandelare et al., 2018). Endophytes are microorganisms (bacteria and fungi) that live in the plant tissue and in many cases support the plant immunity and growth under extreme or unfavorable conditions and against pathogens and pests (Liarzi and Ezra, 2014; Bacon and White, 2016; Gouda et al., 2016). Endophytes are a rich source of secondary metabolites with potential use in medical, agricultural and industrial applications (Liarzi and Ezra, 2014; Gouda et al., 2016).
Seagrasses are higher plants (angiosperms) that live in marine environments, and along with macroalgae, they play a significant role in coastal ecosystems as they provide food, habitat, and are nursery areas for numerous vertebrates and invertebrates. When harvested sustainably, they can be a potential source to produce bioactive compounds as seagrasses are very resistant to decomposition (Grignon-Dubois and Rezzonico, 2013). Seagrasses are rich in secondary metabolites like polyphenols, flavonoids, and fatty acids, which are the key factors involved in the adaptation to biotic and abiotic environments and for the defense mechanism (Custódio et al., 2016). Many different bioactivities including antioxidant, antiviral and antifungal activities are frequently found in extracts of marine seagrasses such as Halodule uninervis or Posidonia oceanica (Bibi et al., 2018; Benito-González et al., 2019). Seagrasses have potential cytotoxic, antimicrobial, and antifouling activity (Zidorn, 2016; Lamb et al., 2017). Luteolin, diosmetin, and chrysoeriol have been often detected in seagrass tissues (Guan et al., 2017). Zosteric acid from the genus Zostera is one of the most promising natural antifoulants due to its low bioaccumulation and no ecotoxicity effects (Vilas-Boas et al., 2017). Seagrass secondary metabolites are often produced by their associated microorganisms such as fungi or bacteria (Panno et al., 2013; Petersen et al., 2019).
A recent study demonstrated that the presence of seagrasses might contribute to a reduction of 50% in the relative abundance of bacterial pathogens that can cause disease in humans and marine organisms (Lamb et al., 2017). Their preservation can therefore benefit both humans and other organisms in the environment. Achamlale et al. (2009) analyzed the content of rosmarinic acid, a phenolic compound with economic interest, from Zostera noltii and Z. marina beach wrack. Its high amount obtained from seagrass flotsam represents a high value-added potential for this raw material and a real economical potential for industries such as cosmetic and herbal ones. Additionally, chicoric acid, another phenolic compound with demonstrated therapeutic applications and high value on the nutraceutical market, has been found in high amounts in Cymodocea nodosa detrital leaves (Grignon-Dubois and Rezzonico, 2013). Considering the rare occurrence of this compound in the plant kingdom, this makes the abundant beach-cast seagrass biomass of interest for dietary and pharmaceutical applications. However, to maintain the ecological stability, novel approaches need to be designed, such as chemical synthesis of seagrass biomolecules, to prevent the over-harvesting of these protected species.
Microalgae have high growth rates and short generation times and can double their biomass more than once per day in the fastest growing species. Microalgal biomass yields have been reported to be up to 20 kg/m2/year for microalgal cultures (Varshney et al., 2015) and they have the potential of transforming 9–10% of solar energy into biomass with a theoretical yield of about 77 g of biomass/m2/day, which is about 280 ton/ha/year (Melis, 2009; Formighieri et al., 2012), however practical yields in large scale cultivation are much lower (e.g., 23 g/m2/day, Novoveská et al., 2016). As their specific growth rate is 5–10 times higher than those of terrestrial plants, the interest in microalgal biotechnology has increased over the last decades. Microalgal biomass could be considered as genuine “cell factories” for the biological synthesis of bioactive substances used in the production of food, feed, high-value chemicals, bioenergy, and other biotechnological applications (Skjånes et al., 2013; de Morais et al., 2015; de Vera et al., 2018). Large-scale cultivation of marine microalgae is usually performed on land, although near-shore cultivation facilities have been tested (Wiley et al., 2013). Saltwater is pumped from the ocean and introduced into ponds or bioreactors where marine microalgae are cultivated on a larger scale. While land is still needed for microalgae cultivation, this does not have to be the high-quality arable land required for agricultural activities. Microalgal production may utilize wastewater as a nutrient source and/or recycled CO2 from industrial facilities or geothermal power plants (Sayre, 2010; Hawrot-Paw et al., 2020).
Microalgae-based production combines several bioprocesses: cultivation, harvesting, extraction and isolation of active components. Parameters to be controlled in cultures include levels of carbon dioxide, light, oxygen, temperature, pH, and nutrients as they have a crucial effect on biomass activity and productivity. The control of predators in industrial scale microalgae production must also be considered (Rego et al., 2015). The biochemical composition of microalgae can be manipulated by optimizing parameters or by applying specific environmental stress conditions, such as variations in nitrate concentration, light spectrum and intensity or salinity, to induce microalgae to produce a high concentration of target bioactive compounds (Markou and Nerantzis, 2013; Yu X. et al., 2015; Vu et al., 2016; Chokshi et al., 2017; Smerilli et al., 2017).
Microalgal species can produce valuable compounds including antioxidants, enzymes, polymers, lipids, polyunsaturated fatty acids, peptides, vitamins, toxins, and sterols (Moreno-Garcia et al., 2017; Vignesh and Barik, 2019). Microalgae also contain pigments such as chlorophylls, carotenoids, keto-carotenoids, and phycobiliproteins (Zullaikah et al., 2019). Due to their contents, microalgae are considered as a promising feedstock for renewable and sustainable energy, cosmetics, food/feed industry both as whole biomass and as nutritional components, colorants or stabilizing antioxidants and for the synthesis of antimicrobial, antiviral, antibacterial and anticancer drugs (Suganya et al., 2016; Moreno-Garcia et al., 2017). Microalgal biomass can also be used in bio-surfactants, bio-emulsifiers, and bioplastics. Unused biomass can be utilized as bio-fertilizer or it can be digested for biomethane or biohydrogen production (Skjånes et al., 2008, 2013). Among microalgae, marine dinoflagellates are efficient producers of bioactive substances, including biotoxins, some of the largest, most complex and powerful molecules known in nature (Daranas et al., 2001). Biotoxins are of great interest not only due to their negative impact on seafood safety, but also for their potential uses in biomedical and pharmaceutical applications (de Vera et al., 2018). Nonetheless, the supply of these metabolites remains challenging due to their low cellular abundance and the tremendous difficulties involved in large-scale microalgae production (Gallardo-Rodríguez et al., 2012; Molina-Miras et al., 2018).
Currently, the most lucrative large-scale production systems focus on pigments (Novoveská et al., 2019), for example, the production of β-carotene from genus Dunaliella. Nannochloropsis species rich in ω-3 fatty acids are used for eicosapentaenoic acid (EPA) and docosahexaenoic acid (DHA) production. The cultivation of Haematococcus pluvialis in large quantities for the commercial production of astaxanthin has also attracted worldwide interest, as this compound is considered to be a “super antioxidant” with numerous applications, ranging from human nutraceuticals and cosmetics to aquaculture feed additives and beverages (Kim et al., 2016; Shah et al., 2016). Many other marine microalgae are being investigated as they are genetically adapted to a variety of environmental conditions. The basis of these adaptations and/or acclimation mechanisms are still being uncovered (Mühlroth et al., 2013; Brembu et al., 2017a,b; Mühlroth et al., 2017; Alipanah et al., 2018; Sharma et al., 2020). Consequently, several research groups have been bioprospecting new microalgal strains that accumulate bioactive compounds of interest (Nelson et al., 2013; Bohutskyi et al., 2015; Erdoǧan et al., 20166).
Selection and breeding of marine algae lag some 10,000 years behind similar activities for terrestrial plants. Recent developments in the fields of molecular biology, genomics and genetic engineering have enabled the implementation of detailed functional studies and precise editing of traits/properties of marine microalgae (Nymark et al., 2016, 2017; Kroth et al., 2018; Sharma et al., 2018; Serif et al., 2018; Slattery et al., 2018; Nymark et al., 2019; Sharma et al., 2020). The molecular biology and genomics tool-box for marine microalgae is growing rapidly and we will soon be able to optimize growth, stack properties and transfer complete metabolic pathways from one species to another or from other types of organisms (Noda et al., 2016; Slattery et al., 2018; Nymark et al., 2019; Sharma et al., 2020).
Marine environments are known to host unique forms of microbial life (Poli et al., 2017). So far, an increasing number of thermophilic, halophilic, alkalophilic, psychrophilic, piezophilic, and polyextremophilic microorganisms (mostly bacteria and archaea) have been isolated. They are capable of proliferating even under extreme conditions as a result of their adaptation strategies involving diverse cellular metabolic mechanisms. As the survival strategies of extremophiles are often novel and unique, these microorganisms produce secondary metabolites or enzymes of biotechnological interest. An example is the enzymes of thermophilic or psychrophilic bacteria that are capable of degrading polysaccharides, proteins and lipids for various applications in the food industry or modifying exopolysaccharides for tissue engineering (Poli et al., 2017). In a recent study, bacteria were isolated across the water column of the CO2-venting Kolumbo submarine volcano (Northeast Santorini, Greece) and the strains originating from the hydrothermally active zone in the crater’s floor (500 m depth) were found to be several-fold more tolerant to acidity, antibiotics and arsenic, compared to the strains isolated from overlying surface waters (Mandalakis et al., 2019).
Many archaea are extremophiles. Enzymes derived from extremophiles (extremozymes) are superior to the traditional catalysts because they can perform industrial processes even under harsh conditions, where conventional proteins are completely denatured (Egorova and Antranikian, 2005). Hyperthermophiles, whose preferred growth temperatures lie above 80°C, consist mostly of archaea. Ideal extremozymes for application in a sustainable biorefinery with lignocellulosic waste material as feedstock should exhibit high specific activities at high temperatures combined with superior thermostability (Krüger et al., 2018). Over 120 genomes of hyperthermophiles have been completely sequenced and are publicly available. Of these, interesting strains belonging to extremophilic archaea that grow at elevated temperatures have been studied in detail such as the genera Pyrococcus, Thermococcus, or Thermotoga found in the marine environment (Krüger et al., 2018). Thermostable archaeal enzymes were reported to have higher stability toward high pressure, detergents, organic solvents and proteolytic degradation (Sana, 2015). However, marine organisms, especially those in biofilms or adapted to live in extreme environments, such as hyperthermophiles, may be recalcitrant to typical lysis methods, hindering protein extraction or modifying the yield of this important step. The hyperthermophile and radiotolerant archaeon Thermococcus gammatolerans, which was isolated about 2,000 m deep in the Pacific Ocean, is a typical example of such a difficult non-model organism (Zivanovic et al., 2009; Hartmann et al., 2014). Chitin is the second most abundant polysaccharide after cellulose, but its use as a feedstock is limited by the inability to hydrolyze it into simple sugars. Several chitinases have been characterized by extremely thermophilic archaea from marine biotopes (Straub et al., 2018; Chen et al., 2019). Recently, the first thermophilic chitinase able to hydrolyze the reducing end of chitin was reported in Thermococcus chitonophagus, potentially expanding the opportunities for using this material (Andronopoulou and Vorgias, 2004; Horiuchi et al., 2016).
Halophilic archaea (haloarchaea) comprise a group of microorganisms from hypersaline environments, such as solar salterns, salt lakes and salt deposits. These microorganisms can synthesize and accumulate both C40 and C50 carotenoids (Giani et al., 2019). The bioactivity of the carotenoid extracts of some haloarchaea indicates their antioxidant, antihemolytic, and anticancer activity (Galasso et al., 2017; Hou and Cui, 2018). The main haloarchaeal carotenoid, bacterioruberin, a C50 carotenoid, presents higher antioxidant capacity when compared to other commercially available carotenoids, such as ß-carotene (Yatsunami et al., 2014).
Some groups of bacteria, like actinobacteria (commonly named actinomycetes) and cyanobacteria, stand out for their capacity to synthesize secondary metabolites. About 660 new natural products from marine bacteria were discovered between 1997 and 2008, with 33 and 39% of them originating from cyanobacteria and actinobacteria (Williams, 2009). Since then, the new hits from marine bacteria exhibited an accelerated increase. The number of marine bacterial natural products identified each year from 2010 to 2012 was approximately 115, from 2013 to 2015 was 161, while it increased to 179 in 2016 (Blunt et al., 2018). It is estimated that 10% of all marine bacteria are actinomycetes (Subramani and Aalbersberg, 2013; Subramani and Sipkema, 2019). With less than 1% of the actinomycetes isolated and identified so far, the marine environment represents a highly promising resource in the field of biodiscovery. Between 2007 and 2017, 177 new actinobacterial species belonging to 29 novel genera and three novel families were described (Subramani and Sipkema, 2019). The Gram-positive actinobacteria are a major chemically prolific source of bioactive metabolites with anticancer, antimicrobial, antiparasitic, anti-inflammatory, antibiofilm activities, and antifouling properties, among others (Prieto-Davó et al., 2016; Bauermeister et al., 2018, 2019; Cartuche et al., 2019, 2020; Girão et al., 2019; Pereira et al., 2020). There are several marine-derived actinomycete metabolites described in the literature. Marinone and neomarinone, a pair of sesquiterpene napthoquinones, generated considerable interest in the biosynthesis community because terpenes are extremely rare in bacteria and because the biosynthetic gene cluster could represent a unique mechanism to produce hybrid polyketides through combinatorial biology (Pathirana et al., 1992). Cyclomarin A, a cyclic heptapeptide, also generated interest due to its uniquely modified amino acids and potent anti-inflammatory activity (Renner et al., 1999; Lee and Suh, 2016). The importance of these microorganisms (Potts et al., 2011) is clearly illustrated by the obligate marine actinomycetes from species Salinispora tropica that produce salinosporamide A (marizomib), a unique and highly potent β-lactone-γ-lactam proteasome inhibitor currently in Phase III trials as anticancer agents (Mincer et al., 2002; Feling et al., 2003; Fenical et al., 2009). Marinomycins A-D are also chemically unique bioactive metabolites with potent cytotoxicity toward cancer cell lines, possessing unprecedented polyol-polyene carbon skeletons with unique biological activities evaluated in vivo tumor models (Kwon et al., 2006), and currently being evaluated in clinical trials. Streptomyces sp. are a source of novel anticancer compounds as they exhibit significant in vitro cytotoxicity and pronounced selectivity in a diversity of cancer cell lines (Hughes et al., 2009a,b) and show potent antibiotic activity against several human pathogenic strains (Nam et al., 2010; Jang et al., 2013). However, as non-medical applications require less strict bioassays for certification, a recent trend for Streptomyces-derived antimicrobial compounds is their use in antifouling and antibiofilm products due to their capacity to inhibit the growth of biofilm-forming species (Cheng et al., 2013; Bauermeister et al., 2019; Pereira et al., 2020).
Cyanobacteria are found in a wide variety of environments and are prolific producers of bioactive secondary metabolites. Cyanometabolites are characterized by antimicrobial, anti-inflammatory, antioxidant, anticoagulant, anticancer, antiprotozoal, and antiviral activities. Therefore, cyanobacteria are suitable sources of bioactive compounds for medical, food, and cosmetics applications (Silva et al., 2018; Demay et al., 2019; Kini et al., 2020). To date, 2,031 cyanobacterial metabolites have been described, among which 65% are peptides (Jones et al., 2020). Based on the similarities of their structure and biological activity, they have been organized in 55 unique bioactive classes, such as cyanopeptolins, anabaenopeptins, aerucyclamides, aeruginosines, and microginins (Welker and van Döhren, 2006; Huang and Zimba, 2019; Janssen, 2019). Frequently a single strain will produce a diverse cocktail of peptides (Supplementary Figure 1). The peptides are highly diverse often containing unusual amino acids such as the heptapeptides, the microcystins which contain an unusual hydrophobic amino acid, Adda (2S,3S,8S,9S)-3-amino-9-methoxy-2,6,8-trimethyl-10-phenyldeca-4,6-dienoic acid. Related pentapeptides, nodularins are the only other compounds to contain this amino acid. A high number of cyanopeptides was isolated from cyanobacteria previously classified to the polyphyletic genus Lyngbya. After taxonomic revision, the new genus – Moorea – being the source of more than 40% of all reported marine cyanobacterial natural products, was described. These include curacin, apratoxins, cryptophycins, and dolastatins. A total synthesis of several bioactive cyanopeptides has been successfully elaborated, which opened new opportunities for structure-activity studies and better evaluation of their potential (White et al., 1997; Chen et al., 2014; Yokosaka et al., 2018).
Cyanobacteria synthesize low molecular weight toxins (cyanotoxins), such as microcystins, anatoxins, saxitoxins, β-methylamino-L-alanine (BMAA), and cylindrospermopsin (Janssen, 2019). Amongst cyanotoxins, microcystins are the most geographically widespread and are not restricted to any climatic zone or other geographic range (Welker and van Döhren, 2006; Overlingė et al., 2020). To date, over 279 microcystin variants have been identified and structurally characterized (Spoof and Catherine, 2017). Microcystins are potent inhibitors of type 1 and type 2A protein phosphatases (Bouaïcha et al., 2019). Modified nodularin and microcystins compounds comprise the cytotoxic agents and can be used for the therapy of various diseases (Enke et al., 2020).
Marine firmicutes and proteobacteria have been also shown to produce diverse bioactive compounds, like indole derivatives, alkaloids, polyenes, macrolides, peptides, and terpenoids (Soliev et al., 2011). Firmicutes represent 7% of the bioactive secondary metabolites produced by microorganisms. The genus Bacillus is a relevant representative of the Firmicutes phylum and is a common dweller of the marine environment. It shows high thermal tolerance and rapid growth in liquid media (Stincone and Brandelli, 2020). Species of the genus Bacillus are a prolific source of structurally diverse classes of secondary metabolites and among them, macrolactins, cyclic macrolactones consisting of 24-membered ring lactones, stand out due to their antimicrobial, anticancer, antialgal and antiviral activities (Gustafson et al., 1989; Li et al., 2007; Azumi et al., 2008; Berrue et al., 2009; Mondol et al., 2013).
Although Proteobacteria is the most abundant phylum in the ocean, representing 55% of ocean bacteria (Sunagawa et al., 2015), these bacteria are scarcely explored in terms of their biotechnological potential while holding many biosynthetic gene clusters in their genomes, potentially linked with the production of bioactive compounds (Buijs et al., 2019). More than 15% of their genome is dedicated to natural products biosynthesis with structural features that include halogenation, sulfur-containing heterocycles, non-ribosomal peptides and polyketides with unusual biosynthetic pathways (Timmermans et al., 2017). Thus, proteobacteria are promising cell factories and remain an attractive source of new drug leads (Buijs et al., 2019). For example, more than 20 biosynthetic gene clusters are found in a single genome of pigmented Pseudoalteromonas strains (Paulsen et al., 2019), comparable to the prolific phylum Actinobacteria. Apart from their important ecological role in marine ecosystems, marine members of Pseudoalteromonas have proven to be important producers of various antibiotics (Bowman, 2007; Fehér et al., 2010; Chen et al., 2012). A Pseudoalteromonas strain isolated from Huon Estuary in southern Tasmania, Australia also shows potent algicidal activity on harmful algae species implicated in bloom events (Lovejoy et al., 1998). Additionally, these microorganisms have also been found to produce several pigmented compounds with antifouling activity (Egan et al., 2001, 2002; Holmström et al., 2002).
The establishment of (pure) cultures of all bacterial divisions still remains the main challenge and this is an essential prerequisite for the development of marine biodiscovery. While genomics allows access to the genetic information of uncultured microorganisms, the availability of the organisms is essential to develop their full potential (Joint et al., 2010).
Marine fungi are widespread in the oceans and colonize different ecological niches; they are found associated with organisms of all trophic levels and can act as saprobes, symbionts and parasites (Wang et al., 2012; Raghukumar, 2017; Poli et al., 2018). Despite the increasing effort of marine mycologists to contribute to the discovery of new species (Abdel-Wahab et al., 2017; Bovio et al., 2018; Devadatha et al., 2018; Poli et al., 2017, 2020), marine fungi are still an understudied group compared to other marine microorganisms (Tisthammer et al., 2016). Jones et al. (2015) estimated that about 10,000 species of marine fungi are still waiting to be described. Marine fungal strains can be isolated from different substrates, such as invertebrates, decaying wood, seawater, sediment, seaweeds, and mangrove detritus. Many factors influence the occurrence and distribution of marine fungi including hydrogen ion activity, hydrostatic pressure, ionic composition and concentration, osmotic response, oxygen availability, salinity, tidal exposure, temperature, availability of substrates for growth (Pang et al., 2016). Since fungi are major marine decomposers, their distribution and seasonal variability typically follow the abundance of the organic matter and its seasonal variability. The highest levels of fungal biomass are encountered in coastal environments and the upper 30 m of the sea surface, rather than in deep seawaters (Wang et al., 2012). On the contrary, deep-sea sediments represent the sink for organic matter creating a habitat where fungi are the dominant eukaryotic microbes (Nicoletti and Andolfi, 2018; Amend et al., 2019). Around 120 fungal species have been retrieved from sediments of deep-sea hydrothermal vents (Xu et al., 2018). Several other substrates have been investigated for the isolation of marine fungi, however, due to the increasing interest in natural products, the most studied fungal communities are those associated with invertebrates, algae, and plants (Garzoli et al., 2015; Bovio et al., 2017; Gnavi et al., 2017; Raghukumar, 2017; Garzoli et al., 2018; Marchese et al., 2020). Nevertheless, the uncultivable fungi, described by using HTS techniques, still represent the major component of the marine fungal community (Comeau et al., 2016; Rämä et al., 2017; Xu et al., 2018). Marine fungi can produce hydrolytic and/or oxidative enzymes including alginate lyase, amylase, cellulase, chitinase, glucosidase, inulinase, keratinase, ligninase, lipase, nuclease, phytase, protease, and xylanase (Bonugli-Santos et al., 2015). These enzymes can have their optimum activity at temperatures ranging from 35 to 70 °C, and at pH values spanning from 3 to 11. The ability of marine fungi to adapt to high saline conditions and extreme pH represents a major biological advantage over terrestrial fungi, and it gives them a higher versatility in biotechnological applications.
Marine fungi produce diverse bioactive molecules (Silber et al., 2016). Besides, their biotechnological potential is still incontestable: among the 1,277 new natural products described in 2016, marine fungi account for 36% of these newly described molecules (Blunt et al., 2018). In comparison, the respective percentage of molecules originating from marine bacteria was only 14%. In 5 years (2010–2015), 285 antibacterial and antifungal compounds were isolated from marine fungi (Nicoletti and Andolfi, 2018). The first reported group of bioactive compounds from marine fungi were cephalosporins, a class of β-lactam antibiotics originally isolated from the Acremonium chrysogenum (which was previously known as Cephalosporium) by Giussepe Brotzu in 1945. Most of the published work on secondary metabolites of marine fungi has focused on a few genera, mainly Penicillium, Aspergillus, Fusarium, and Cladosporium (Imhoff, 2016; Marchese et al., 2020). Marine fungi are found to be a promising source of pharmacologically active metabolites (Imhoff, 2016) with novel anticancer, antibacterial, antiviral, anti-plasmodial, anti-inflammatory, but rarely antifouling, activities (Rajasekar et al., 2012; Bovio et al., 2019a). They are also useful in the production of biosurfactants (Cicatiello et al., 2016; Pitocchi et al., 2020), enzymes (Nicoletti and Andolfi, 2018), and bioremediation (Bovio et al., 2017). Interestingly, endophytic fungi that are associated with macroalgae produce biologically active secondary metabolites with antibacterial, antifungal anticancer and other beneficial properties (Mathan et al., 2013; de Felício et al., 2015; Teixeira et al., 2019). Furthermore, marine fungal enzymes can be used for cleaning, textile, leather, biofuel, pulp, and paper industries; for food and beverages; for animal feed; for environmental, pharmaceutical and cosmetic applications (Bonugli-Santos et al., 2015).
The thraustochytrids represent a unique protist group of eukaryotic microorganisms that provides bioactive compounds including antimicrobial agents. In contrast to their common referral as microalgae, these marine heterotrophic protists (a fungus-like clade of Stramenopiles, class Labyrinthula of the Chromista kingdom) are not microalgae because they are not photosynthetic and lack plastids (Leyland et al., 2017). Under culture conditions that are different for various species, each one of the thraustochytrid taxa develops ectoplasmic networks generated by a unique organelle termed the sagenogen (or sagenogenetosome). Some growing cells exhibit gliding mobility associated with the ectoplasmic networks. Their reproduction activities involve the formation of heterokont, biflagellate zoospores (Porter, 1990). Yet, the level of diversity of thraustochytrids remains to be uncovered since an increasing number of strains and species are being discovered. Thraustochytrid species are usually characterized either by their developmental modes, sorus form or their spore type (Porter, 1990). However, these traditional systematic approaches are insufficient, as only a limited list of morphological characters is available. This facilitated the development of molecular markers, of which the most common are the 18S rDNA sequences (Mo et al., 2002). Yet, due to the limitation of 18S rRNA clone library construction and the emerged cultivation-dependent approaches, the diversity of thraustochytrid species is far from elucidated (Liu et al., 2017).
The thraustochytrids have been isolated from a wide range of coastal, open sea (where they were collected by “pollen traps”) and deep-sea habitats that are often rich in organic materials (Raghukumar, 2002). They are major colonizers of mangrove environments, feeding on decaying materials (Morabito et al., 2019), while playing an important ecological role as active degraders of organic materials and primary consumers (Mo and Rinkevich, 2001). In fact, thraustochytrids, as other heterotrophic marine protists, can consume dissolved organic matter and particulate organic matter as energy sources, and as a result, they are considered to share a distinct ecological niche in marine ecosystems with specific roles in marine biogeochemical cycles (Liu et al., 2017).
Thraustochytrids, considered as oleaginous microorganisms, are developing into an increasingly important marine source of polyunsaturated fatty acids (PUFAs) for a wide range of biotechnological applications (primarily for the industrial production of the ω-3 fatty acid DHA). Some thraustochytrids including species of Schizochytrium and Aurantiochytrium produce long chain polyunsaturated lipids like DHA (C22:5 n3) and docosapentaenoic acid (DPA, C22:5 n6) (Heggeset et al., 2019). To produce their DHA, thraustochytrids use a sophisticated system that differs from the classical fatty acid synthase system (synthesized by a polyketide synthase, instead by the standard fatty acid synthesis), yet, very little has been developed regarding process optimization and their optimal use (Aasen et al., 2016). They are also known to be pathogens of edible invertebrates and common contaminants of marine invertebrate cell cultures (Rinkevich and Rabinowitz, 1993; Ilan et al., 1996; Bowels et al., 1999; Rinkevich, 1999; Rabinowitz et al., 2006) as they are found on surfaces and within the bodies of most marine organisms. Based on these characteristics, the thraustochytrids are considered as an alternative to fish oil and an eco-friendly solution to overfishing. In addition, they are further known to produce saturated fatty acids which are renewable sources of biofuels, for biodiesel, and as a potential source of squalene and carotenoids, two other commercially important compounds that show an increasing market potential (Aasen et al., 2016).
Thraustochytrids also have novel extracellular lipases, for which sequences have not yet been elucidated (Ishibashi et al., 2019). Thraustochytrid strains produce enzymes with multiple hydrolytic activities and a wide range of them is being secreted constitutively, including agarases, amylases, pectinases, chitinases, and carrageenases. They thus have potential in diverse industrial applications (Shirodkar et al., 2017). They are probably the only eukaryotic group that may digest tarballs. Further, the oil from one strain of thraustochytrids (Schizochytrium sp.) has been designated safe for human and for animal consumption by the United States Food and Drug Administration (US FDA7). Thus, it is not surprising that 731 patents on thraustochytrids were published between 1999 and 2018 (US and Eurasia are top in the list), with most patents targeting the use of their chemicals for human well-being purposes, especially the use of ω-3 oils (Colonia et al., 2020).
The development of the research on thraustochytrids has largely facilitated the exploration of novel thraustochytrid strains and species from various marine habitats. Since the beginning of this century, research efforts are involved in fermentative trials, to select appropriate thraustochytrid strains for the industry and for the optimization of culture conditions to obtain high yields (Rabinowitz et al., 2006; Xie et al., 2017).
While viruses are identified as pathogens in many marine organisms, little research effort has been put toward their presumed role as associated organisms. There are an estimated 1030 virioplankton in the world’s oceans, the majority of which are bacteriophages (Parsons et al., 2012). Viruses can live as virioplankton, which is a dynamic component of the marine environment, with a turnover time of 2–4 days, or associated to macro- or microorganisms encompassing enormous genetic diversity and serving as a reservoir of genes for prokaryotic communities (Angly et al., 2006; Dinsdale et al., 2008). They contribute to the global carbon cycling as well as influence pathways of their host’s metabolism (Hernandes Coutinho et al., 2017, 2018, 2019). The presence of viruses and virus-like particles (VLPs) in association with corals (Blackall et al., 2015), sea cucumbers (Nerva et al., 2019a) and sponges (Webster and Taylor, 2011) has been reported using morphological and molecular approaches. In the sponge phylum, elaborate microscopical studies have demonstrated a variety of morphological entities that can be hosted in different body compartments of the host, such as within the cells of the sponge or the associated microbes, or the extracellular matrix and the epithelium (Pascelli et al., 2018). While functional, mutualistic roles have been envisaged for viruses and VLPs associated with corals (van Oppen et al., 2009), relevant studies have been lacking in sponges. Specifically, most marine viruses are cyanophages and are important players in biogeochemical cycles and drivers of the evolution of their hosts (Brussaard et al., 2008) by influencing microbial population size through their lytic capacity, altering their metabolic output and providing an immensely diverse pool of genetic material available for horizontal gene transfer. Only recently, viruses infecting and replicating in marine fungi were reported and many new mycoviruses were identified in a handful of studies (Nerva et al., 2016, 2017, 2019b). Contrary to most bacterial viruses, mycoviruses do not cause lysis of the fungal host cell and accumulate to high levels without specific cytotoxic effects as persistent and often cryptic infections creating multi-level interactions with their hosts: they can modulate host behavior to successfully spread and survive in the environment and provide adaptive advantages (Mehle et al., 2012; Selman et al., 2012; Son et al., 2015). Very recently it has also been highlighted how mycoviruses can modulate the production of secondary metabolites for example mycotoxins (Nerva et al., 2019a). Based on this evidence, the search for mycoviruses within each fungal isolate and the investigation of their contribution to biosynthetic processes is of high biotechnological interest, and it may open a new avenue in the discovery of unique natural products.
By some estimations, bacteriophages are the most abundant entities on this planet (Harada et al., 2018). Their valorization in early anti-infection trials was hampered by the discovery of antibiotics but it is being reintroduced due to the global emergence of antibiotic resistance (Coffey et al., 2010; Fernández-Ruiz et al., 2018). Bacteriophages can be used as an alternative to antibiotics, either against resistant pathogens (Mattey and Spencer, 2008) or for steering aquaculture processes that rely on massive use of antibiotics (Culot et al., 2019). Additionally, bacteriophage biotechnology is directed to control bacterial pathogens in important crops and to limit the risk of pathogens reaching food chains by decontamination of livestock (Harada et al., 2018). Bacteriophages can also be used as cost-efficient, highly stable and specific biosensors to effective detection of pathogenic bacteria (Singh S. et al., 2020).
Classical taxonomy, based on morphological traits, is still the basis of the (macro)species description. However, omics approaches have become increasingly popular in recent decades due to decreasing costs of DNA sequencing, the availability of mass spectrometry (metabolomics, lipidomics, and proteomics) and the development of bioinformatics. The major limitations that prevent taking the full advantage of the rapidly growing volumes of biological data from omics technologies, are the lack of standardization and/or description of experimental/sampling conditions, as well as lack of big data analytics experts. Other limitations are the lack of user friendly bioinformatic annotation pipeline tools and well curated and populated sequence and data repositories (Glöckner and Joint, 2010). Being coupled with subsequent network analyses, bioinformatics and biostatistics, these tools would enable faster detection of new marine species and their biomolecules and faster adoption of molecular protocols. Typically, biodiversity data is generated from long-term monitoring campaigns or novel exploratory expeditions and are presented as lists of species presence and (relative) abundance. As these lists can be lengthy, their manual inspection is time-consuming and limits access to important pieces of information. Visualizing these data using network analysis tools can uncover important associations and relations between species in a specific location (Orlando-Bonaca and Rotter, 2018; Mozetič et al., 2019). Importantly, other traits can be included in the biodiversity datasets (i.e., chemodiversity, physical parameters), which can uncover complex network associations of marine organisms and their compounds and can help to determine the target organisms for biotechnological exploitation. Bioinformatics approaches are typically developed and used for assembly, alignment, gene/genome annotation, function prediction, and data integration. After determining the species and compounds of interest, the next step is to compare the organism growth/metabolic engineering conditions, productivity and yields of the compound(s) of interest. Novel approaches such as the use of Solid-Phase Microextraction (SPME) have been recently demonstrated as a successful means of non-invasive untargeted metabolome screening of marine organisms (Bojko et al., 2019). This method comprises the use of specially coated probes that combine metabolite sampling and extraction in a single step, thus allowing in vivo metabolomic screening in the marine environment, while allowing both polar and non-polar metabolites to be extracted efficiently (Reyes-Garcés et al., 2018), thus emerging as a useful field tool to discover novel compounds of interest from complex marine holobionts. Statistical models are then often used for the determination of differential expression of genes or metabolites of interest and determination of optimization protocols. These are necessary steps for the development of scale-up production protocols.
Bioprospecting of marine microorganisms using state-of-the-art molecular methods generates vast amounts of data. By digitalizing these data, scientists have now access to biodiversity data that can be integrated, thus providing an organic link between species distribution and genome diversity (La Salle et al., 2016). However, not all data are open access, as data providers may have their own policies concerning privacy and confidentiality, which limits scientists to share their experience and collaboration. Importantly, any data provider should develop internal metadata guidelines that provide minimal information about the data stored that will enable their use in the future. In 2011, the European Commission (EC) launched a new Open Data Strategy for Europe after realizing that data generated by the public sectors are vast and although financed by the public funds, data are not always available and accessible. Hence, the EC highlighted the unused potential of public sector data to spur innovation, economic growth, and answer to societal challenges such as food security and healthcare8. As a result, Europe now hosts at least two infrastructure initiatives, publicly hosting many marine microbial strains: MIRRI (Microbial resource research infrastructure9) and EMBRC (the European marine biological resource center10). MIRRI is an infrastructure initiative for preserving microbes that can be cultured for future exploitation and providing coordinated actions to facilitate access to genetic resources – data, microbial strains, and expertise. EMBRC and LIFEWATCH (European Infrastructure Consortium11) connect marine stations that provide direct access to the sea, marine resources, and different services. A review of the past and current European initiatives is provided in the Supplementary Table 1 within Rotter et al. (2020a). Also useful for marine biotechnology development are databases of chemical structure: ChemSpider, databases of carbohydrate structures and other glycobiology-related fields (e.g., Glycosmos, Glycomics at Expasy, GlyGen, and CarboMet), MarinLit, a database of marine natural products literature that has been active since the 1970s, CyanoMetDB, a comprehensive database dedicated to cyanobacterial secondary metabolites (Jones et al., 2020), and the newly developed Natural Products Atlas, an open access database for microbial natural products discovery (van Santen et al., 2019). These databases (Table 2) play an important role in facilitating track and trace of the specimen and the chemical compounds they synthesize, thus enabling a rapid dereplication (Blunt et al., 2012; Collins, 2019). Importantly, the policy making and scientific community are supporting the public availability of all information and materials from these databases to avoid the fragmentation of expertise, unbalanced database access, and the estimated $10 billion annual losses due to incorrect reference material (Collins, 2019).
There is a wide range of marine natural products, from relatively small primary and secondary metabolites, with molecular weight usually lower than 1,500 Da, which comprise around 34,000 compounds according to the latest version of the marine natural products database MarinLit (Table 2) with a wide range of biological activities, to macromolecules such as enzymes and polysaccharides. Proteins, carbohydrates, and lipids from marine organisms are crucial in our diet but can also be involved in the development of novel processes for the production of higher value-added products. A wide range of applications has been described for marine enzymes in the food industry and human health, and marine polysaccharides have also found multiple biomedical and tissue engineering uses (Fernandes, 2014; Eswara Rao et al., 2017; Joshi et al., 2019). However, only a fraction of the enzymes derived from marine organisms has been isolated and characterized. Hence, enzymes as biocatalysts from marine organisms are a relatively untapped resource for discoveries.
Dereplication is a crucial step in any natural product discovery used to identify known metabolites in complex and heterogeneous matrices with a broad concentration of bioactive molecules, preventing the re-isolation and re-characterization of known bioactive compounds (Gaudêncio and Pereira, 2015; Kildgaard et al., 2017). The last two decades have seen a revolution in the development of new dereplication strategies. These usually consist of the combination of analytical techniques: chromatography (usually high pressure liquid chromatography, HPLC or UHPLC) with a detection method, mass spectrometry (MS) in the high resolution (HRMS) mode and/or with ion fragmentation (MS/MS), or nuclear magnetic resonance (NMR). The remarkable advances in analytical instrumentation along with the development of suitable databases have enabled the development of the fast dereplication processes required in current drug discovery programs based on natural products (Pérez-Victoria et al., 2016). The use of LC-MS/MS based molecular network workflows enable the unearthing of the real chemical inventory of chemical extracts and downstream fractions, and significantly increase the annotation rate of metabolites (Oppong-Danquah et al., 2018), allowing the targeted isolation of new metabolites (Li et al., 2018). Furthermore, biological activities can be mapped into such networks, facilitating the rapid discovery of (new) compounds that are responsible for bioactivity (Fan et al., 2019).
Open-access knowledge bases containing tandem mass (MS/MS) spectrometry data such as the Global Natural Products Social Molecular Networking (GNPS, Table 2) or structures of microbial natural products (The Natural Products Atlas, Table 2) have been greatly enhancing the efficiency of dereplication processes leading to the identification of new molecules and natural product scaffolds (Wang et al., 2016). Small Molecule Accurate Recognition Technology (SMART), using NMR data, constitutes a step forward in the automatic identification or classification of new natural products, especially when combined with GNPS. Metabolite identification based on high resolution – HR-MS/MS and NMR along with freely accessible and commercial databases was reviewed by Wolfender et al. (2019). The next steps are: (i) the use of ZINC, Reaxys, ChEMBL, PubChem or StreptomeDB 3.0 databases, and/or NMR databases for virtual bioactivity screening (Table 2). These use either structure-based or ligand-based computational approaches to predict quantitative structure-activity relationship (QSAR), based on active and inactive known molecule, or to predict binding sites based on the chemical similarity to known ligands to identify protein targets of bioactive molecules (Pereira et al., 2014, 2015; Sterling and Irwin, 2015; Cockroft et al., 2019; Cruz et al., 2019; Dias et al., 2019). Additionally, (ii) the use genomic data in combination with molecular networks enables dereplication also at the frontier between chemistry and biology (van der Hooft et al., 2020), (iii) the creation of a globally available NMR raw data bank (Gaudêncio and Pereira, 2015; McAlpine et al., 2019), and (iv) the creation of computer based tools to compare and integrate such global NMR data bank can all be used for advancing the automated identification of chemical structures (Zhang et al., 2020a).
The efficiency and rate of secondary metabolite production by microorganisms are heavily dependent on their culture/growth conditions and potential for up-scaling. Indeed, one of the driving forces for the production of specific metabolites, compared to the constitutive ones that are usually produced in any conditions, is the simulation of the marine microbes-native environment, including the interactions with other microorganisms as usually occurs in nature (Takahashi et al., 2013; Vallet et al., 2017). Consequently, standard conditions do not always support fungal or bacterial production of interesting secondary metabolites (Reich and Labes, 2017). To increase the availability of microbial biomass and minimize the environmental damage impacts of organismal collections, new strategies are needed to develop sustainable in vitro approaches for the cultivation of whole organisms or of individual cell types from targeted marine species (Rinkevich, 1999, 2005, 2011; Barnay-Verdier et al., 2013; Ventura et al., 2018; Maristem COST Action12). This is also of importance for sponges and corals, which host endosymbiotic intracellular microorganisms. The appropriate cultivation settings should provide the optimal conditions for the holobionts (Rinkevich, 1999).
When cultured, microorganisms do not always continue to produce the same metabolites (Wijffels, 2008). To mimic the naturally occurring interactions, the co-culture (solid media) or mixed fermentation (liquid media) with other organisms have been demonstrated to stimulate the production of secondary metabolites (Romano et al., 2018), some modulating the quorum sensing, with application both in antibiotics and anticancer pharma sectors (Bertrand et al., 2014). The interactions can be modulated to mimic the natural environment for chemical-ecological study, including symbiosis studies. For example, specific antagonistic organisms can be introduced to promote the synthesis of antibiotics (Bertrand et al., 2014; Romano et al., 2018; Bovio et al., 2019b). Enhanced lipid production in microalgae has been documented in co-cultivation of microalgae and bacteria, including cyanobacteria (Ferro et al., 2019; Gautam et al., 2019; Toyama et al., 2019), as well as in co-cultivation of microalgae and fungi (Arora et al., 2019). Growth-enhancement in microalgae has been documented in co-cultivation of microalgae with other microalgae (Ishika et al., 2019), as well as with fungi (Li H. et al., 2019; Wang et al., 2019) and protozoa (Peng et al., 2016).
Some studies have shown that bacterial genomic data reveal an inconsistency between the number of gene clusters identified using bioinformatic approaches as potentially producing secondary metabolites and the number of chemically characterized secondary metabolites produced under standard fermentation conditions (Reen et al., 2015; Romano et al., 2018). Therefore, cultivation-based techniques have been developed aimed at stimulating expression of these “silent” genes. In 2002, Bode and collaborators highlighted that the modification of cultivation parameters such as temperature or salinity can activate silent genes that determine the synthesis of new secondary metabolites; this method is called OSMAC approach (One Strain – Many Compounds, Bode et al., 2002). The OSMAC strategy is a powerful tool that can activate many silent biogenetic gene clusters in microorganisms to produce more natural products or to induce the expression of poorly expressed bioactive metabolites (Abdelmohsen et al., 2015; Pan et al., 2019). This activation can be achieved in several ways. (i) By co-cultivation of different species, antibiotics can be produced as a response to a bacterial challenge (Cueto et al., 2001). (ii) Elicitation with microbial lysates or with microbial cell components can be applied. (iii) Chemical elicitation by the addition of compounds of non-biological origin. This results in changes in the metabolomic profiles, e.g., the increase in the production of the antibiotic jadomycin B, in Streptomyces venezuelae after the addition of ethanol or the alteration of the antibiotic production of Bacillus circulans and Bacillus polymyxa after the addition of DMSO to the fermentation media (Dull et al., 1994; Chen et al., 2000; Pettit, 2011; Zhu et al., 2014; Abdelmohsen et al., 2015). Also, other epigenetic modifiers such as 5-azacytidine and suberoyl bis-hydroxamic acid, are widely recognized as effective tools to stimulate the production of secondary metabolites and to find new bioactive molecules in microorganisms (Adpressa and Loesgen, 2016; González-Menéndez et al., 2018; Romano et al., 2018). Therefore, once the marine bacteria strains exhibit promising activities, it is important to focus on different cultivation media and conditions to enhance the production of the desired metabolites.
There is a great need for exploring new extraction protocols to identify bioactive ingredients. Extraction is the first step needed to obtain natural products from organisms. Several extractions methods can be applied, depending on the organisms and the compounds of interest. At lab scale, maceration, or percolation with solvents (organic or water) at room temperature are the most commonly used methods for macroorganisms. The selection of the solvent depends on the solubility of the metabolites of interest, but there are other aspects such as cost and safety that are considered. To increase efficiency, avoid the use of large amounts of solvents and reduce extraction time, some greener and automated extraction methods have been developed such as super-critical fluid extraction – SFC (Essien et al., 2020), subcritical water extraction – SWE (Zhang et al., 2020b), a moderate electric field – MEF (Gavahian et al., 2018), ultrasound (Kumari et al., 2018), pressurized liquid extraction – PLE, microwave assisted extraction – MAE and enzyme-assisted extraction – EAE (Grosso et al., 2015). They all share the advantages of time reduction, optimized solvent consumption, reproducibility and selectivity, rendering these procedures suitable for both analytical and industrial processes.
Microorganisms that grow in liquid media require several steps to obtain biomass for biochemical extraction. Biorefinery is costly and requires a long biomass processing that involves multiple unit operations, including cultivation, harvesting, product extraction and stabilization. All of these contribute to the commercial competitiveness of bioproducts. One major bottleneck is the initial amount of the biomass of organisms available. Another issue is the differences in bioactive molecules produced by the same organisms but grown under different environmental conditions or different seasons. Homogenization can take place only if they can be cultivated under the same conditions, which is not always possible. Extraction, which directly impacts the product properties, is one of the main commercial constraints in the production of fuels, food and feed, and high-value products like polysaccharides and pigments. Extraction is a highly specific process which has to be adopted to obtain the desired products (Rizwan et al., 2018). Recently, it has been suggested that “milking” of secondary metabolites from marine organisms can significantly reduce the time and cost of this process (Hejazi and Wijffels, 2004; Kim et al., 2016). “Milking” is a term used to describe a non-destructive regenerative extraction of target metabolites from marine organisms while keeping the cells metabolically active just like producing milk from cows. Unlike conventional biorefinery, which requires a time-consuming cultivation step and intensively energy-consuming harvesting and cell disruption steps, the milking process does not require the reculturing of cells from the exponential stage of the cultivation process nor harvesting and cell disruption (Hejazi and Wijffels, 2004). Milking has been demonstrated in two microalgal species; Dunaliella salina for β-carotene and Botryococcus brauni for hydrocarbon (Hejazi et al., 2004; Moheimani et al., 2014). These microalgae have a weak or no cell wall and release storage compounds to the cultivation medium under stress (exocytosis), which remain the prerequisite for the success of milking.
Generally, crude extracts consist of a complex mixture of natural products that require several fractionation and purification steps to obtain pure compounds. The separation method used depends on the physical or chemical properties of the individual natural product and the amount needed. Chromatography, especially column chromatography, is the main method used to obtain pure natural products from a complex mixture. Several chromatographic options can be used depending on the polarity, size or ionic strength of the compounds of interest. Ideally, the process would involve a one-step purification and a scale-up system that allows working with the amounts of crude extract that are at the same time relevant for the industrial level (Ebada et al., 2008; Zhang et al., 2018).
Suitable and standardized scalable extraction and purification methods are not yet established. New separation techniques are necessary, capable of treating dilute solutions or solutions containing only minute amounts of target molecules in the presence of vast amounts of accompanying compounds in both small and large-scale processes, even in the presence of particulate matter. In addition to standard separation procedures, applications of magnetic (nano)particles in batch systems, magnetically stabilized fluidized beds or magnetically modified two-phase systems can be used successfully for the separation of natural products (Safarik and Safarikova, 2004). In most cases, magnetic carriers bearing an immobilized affinity or hydrophobic ligand or ion-exchange groups, or magnetic biopolymer particles having affinity to the isolated molecule are mixed with a sample containing target compound(s). Following an incubation period when the target compound(s) bind to the magnetic particles, the whole magnetic complex is easily and rapidly removed from the sample using an appropriate magnetic separator. After washing out the contaminants, the isolated target compound(s) can be eluted and used for further work. All the steps of the purification procedure can take place in one single vessel. The separation process can be performed directly in crude samples containing suspended solid material (Safarik and Safarikova, 2004). Magnetic techniques based on the application of magnetic nano- and microparticles have been successfully used for the preconcentration, detection and determination of different types of xenobiotics, viruses, microbial pathogens and protozoan parasites in water samples (Safarik et al., 2012). Currently, magnetic solid-phase extraction (Safarikova and Safarik, 1999) is one of the most often used preconcentration procedure used in (bio)analytical chemistry.
The structure elucidation of secondary metabolites is of critical importance, especially when developing products for the pharmaceutical sector. Marine natural products have unique complex chemical scaffolds, and their structures are classically elucidated by the combination of HR-MS, 1D and 2D NMR data analysis. Computer techniques are being developed to aid structure elucidation such as Computer-Assisted Structure Elucidation (Burns et al., 2019) but are still in their infancy. To determine the absolute configuration of metabolites, single-crystal X-ray diffraction, NMR advanced Mosher’s methods (for alcohols), Marfey’s method (for aminoacids) are often used (Bhushan and Brückner, 2011; Cimmino et al., 2017). Computational approaches such as density functional theory (DFT) coupled with (i) NMR, (ii) chiroptical methods e.g., electronical circular dichroism (ECD), vibrational circular dichroism (VCD) spectroscopy and (iii) optical rotation are being successfully employed in identification of relative and absolute configuration of marine natural products (Merten et al., 2015; Batista et al., 2018; Menna et al., 2019; Wang and Hamann, 2019; Fan et al., 2020; Marcarino et al., 2020; Zhu and Sun, 2020). The elucidation of biosynthetic pathways from genomic sequence data is also used to support the absolute configuration assignment (Hu et al., 2018; Kim et al., 2020).
Screening for biological activity of marine extracts or purified compounds is an important next step in determining their applicability for a specific purpose. It is typically done using in vitro, ex vivo or in vivo approaches. The bioassay-guided identification and purification of novel compounds is a common practice (Strömstedt et al., 2014; White et al., 2019). First, the selection of the extraction protocol may affect the possibility of testing for biological activity, as not all solvents that are used for extractions are biocompatible. Second, an array of selected bioassays can be performed to discover novel bioactive compounds, encompassing for example antimicrobial, anticancer, antiviral, antiparasitic, anti-inflammatory, nematotoxic or entomotoxic activities. These bioassays are usually very specific, fast and often in a high-throughput format. They test for individual bioactivity, such as inhibition of a specific enzyme, or inhibition of bacterial growth using only one bacterial species. Since the amount of the extracted material is usually limited, a limited array of bioassays can be performed on a given extract/compound. Therefore, the choice of bioassays and their quality determine the potential of the extract/compound for future applicability. In contrast to the use of dereplication to avoid the rediscovery of compounds from different extracts/bioresources, the use of different bioassays for the same extract is encouraged to avoid missed opportunities for alternative bioactivities. Namely, there is a myriad of bioactive compounds in any extract and it is becoming accepted that any compound can execute multiple bioactivities. Repurposing of compounds with known bioactivity for a different one is increasingly becoming an attractive proposition because it involves the use of de-risked compounds, with potentially lower overall development costs and shorter development timelines (Pushpakom et al., 2019). Importantly, as in any application, the compounds in original or transformed form inevitably end up in the environment, an important bioassay to include in the screening process is the (eco)toxicity assessment (Walker, 2008; Yan et al., 2019). No product safety risks can be taken, especially considering the tragic case with the sedative drug thalidomide (Thalidomid®) prescribed to relieve pregnancy nausea, in which one of the stereoisomers had the desired bioactivity and the other caused birth defects in thousands of children. This tragedy marked a turning point in product testing, resulting in the development of systematic toxicity testing (Kim and Scialli, 2011). Hence, in the last decade in silico methods have been developing to assess both bioactivity and toxicity of known bioactive compounds and their synthetic analogs or novel compounds with known chemical structures (Yang et al., 2018; Liu et al., 2019; Brzuzan et al., 2020). In addition, the virtual screening technique called target fishing (Singh N. et al., 2020) can help recognizing the molecular target of the discovered marine natural products.
Screening and preclinical validation have been essentially performed in rodents, mostly because, among the model organisms used in pharmaceutical and biomedical research, they have a large repository of genetic/molecular tools available and are genetically similar to humans. However, ethical and economic concerns associated with low throughput (Giacomotto and Ségalat, 2010), have potentiated the search for alternative models that may be used as the first line of screening. This decreases the burden on mammalian models, that still need to be used as a final step of validation prior to clinical trials. In vitro and ex vivo screenings using cell lines and tissues representative of both healthy and pathological human conditions, are currently used to identify biological activities of marine-derived extracts (Laizé et al., 2014; Kolanti et al., 2016; Yuan et al., 2017; Carson et al., 2018b; Martínez Andrade et al., 2018; Sun et al., 2018). Nevertheless, the results obtained, even if promising, still need to be validated in vivo. Zebrafish has become a model of increasing interest for human disorders and it allows both low-throughput screening using adults, and high-throughput screening using larvae, thus fitting the requirements to develop vertebrate whole-animal assays (Lee et al., 2017). The increasing number of molecular tools and the development of mutants and transgenic fish (Hwang et al., 2013; Cornet et al., 2018), capable of highlighting almost any given tissue (Chen et al., 2017), allowing in vivo follow-up of treatments through fluorescence microscopy is also an asset, associated to their easy maintenance, short life cycle, large progeny and translucent larvae.
The long-term socio-economic sustainability of production is an important aspect of marine biotechnology. It relies on the stakeholders’ capabilities to manage the resource sustainably and to provide the social, economic, and regulatory conditions to ensure a living income. Therefore, wild harvesting is not desirable as it could endanger the species itself or its associated species and impact the whole marine ecosystem. The quality and quantity of the product should not be dependent on the seasonal fluctuations and abundance of biomass or its target compound. From an economic and social perspective, commercial/industrial activities preferably maintain regular volumes of production throughout the year. The translation of research laboratory discoveries into commercial items that entail obtaining and maintaining the supply levels and safety requirements are nowadays recognized as the major hurdles in bringing marine natural-product-based molecules to market (Taylor, 2015; Newman and Cragg, 2020). With regards to sustainability and zero-waste principle, an important consideration when growing marine biomass and isolating bioactive compounds is cascading, circular economy and zero waste/waste utilization. The biorefinery should be designed typically in a cascading approach to exploit all bioactive and not just the dominant or more economic compounds. The biological leftovers from a cascading process could ultimately be valorized as a feed or low end as biomass for production of bioenergy (Tedesco and Stokes, 2017; Álvarez-Viñas et al., 2019).
After the target product is known, the best production pathway is identified (Chubokov et al., 2016). There are two general production upscaling methods (Figure 3). One is the culture of organisms in tanks, ponds or bioreactors or to a certain extent at sea infrastructures for seaweed, sponges and corals. Alternatively, the naturally harvested biomass is directly used or molecules of interest are synthesized. When the organisms that naturally produce the compounds of interest are used (Figure 3, left), the desired properties for scaled-up products are a short production cycle and a high yield per unit (Li S. et al., 2019). One of the possible approaches for product harvesting is to trap the secondary metabolites secreted by the invertebrate or associated microorganisms to the surrounding water. This strategy has the advantage of preserving the producer organism with no stress or injury. The proof of concept was described recently for a device that traps the secreted metabolites with Amberlite XAD-16 (Vlachou et al., 2018). Based on this concept, the group patented and built Somartex® (self operating marine trapping extractor) for testing in different marine locations13. Alternatively, compounds of interest can be produced with plasmid-based expression systems in well-established prokaryotic and eukaryotic hosts, such as Bacillus subtilis, Escherichia coli, Saccharomyces cerevisiae (Kroll et al., 2010; Mao et al., 2017; Miao et al., 2020), the yeast genus Pichia as well as cell systems. These recombinant expression systems have dramatically increased the opportunities for biotechnology and commercialization of products. The selection of the expression plasmids to be used depends on the combination of important factors, such as the selection of replicons, promoters (either homologous or heterologous ones), selection markers and cloning sites (Rosano and Ceccarelli, 2014). Systems biology and CRISPR/Cas systems are used to improve bioprocess performance (Tripathi and Shrivastava, 2019). Appropriate growth media and growth conditions (e.g., batch, fed-batch, and continuous or perfusion modes and optimization of physico-chemical parameters in bioreactors) have to be adapted next, followed by the establishment of effective purification strategies (Tripathi and Shrivastava, 2019; Duzenli and Okay, 2020). Finally, product yield optimization is achieved by fine-tuning the metabolic pathways. This promising process is not yet fully implemented in practice due to the combinatorial complexity of optimizing metabolic pathways and the potential cellular toxicity resulting in the overexpression of key pathways (Chubokov et al., 2016).
Instead of culturing the whole organisms, another possible focus is to establish cell lines using marine invertebrates (Figure 3, right). So far, this has not been successfully implemented. Nevertheless, some research groups are working on the improvement of invertebrate cell culture and recently a substantial increase in both the rate and number of cell divisions in sponge cells has been reported by optimization of the nutrient medium (Conkling et al., 2019). A final approach for addressing the supply issue, especially for macroorganism-derived marine natural products is de novo chemical synthesis or laboratory polymerization of natural monomers (Kristufek et al., 2017; John et al., 2019). This method can be very costly with many steps and low yields due to the chemical complexity of the compounds that often have many functional groups and chiral centers. An example is halichondrin B, a potent cytotoxic macrolide isolated from the sponge Halichondria okadai with a staggering 32 stereocentres (Hirata and Uemura, 1986; Ledford, 2010). Its structurally truncated analog, eribulin, has 19 stereocentres and is an FDA-approved drug for the treatment of metastatic breast cancer. However, its supply still consists of a 62-step chemical synthesis (Ledford, 2010). When an entirely chemical production is required, strategies will very much depend on the kind of structure to be synthesized, but in some particular cases, as the synthesis of peptides or their derivatives, solid-phase approaches have proved to be the method of choice for their efficient production (Martín et al., 2014). In addition, biosynthetic steps merged with chemical synthesis steps offer a simplified option for the total synthesis of some natural products (Kirschning and Hahn, 2012). Enzymatic synthesis mimicking natural biosynthesis is also used to produce natural products (Greunke et al., 2017).
Marine biotechnology is considered to be one of the main pillars of bioeconomy. The main use case scenarios are discussed below, following the order of the marine biotechnology products pyramid value, presenting industrial sectors and products with substitution potential (Figure 4). A short, non-exhaustive overview presenting some of the European companies that specialize in delivering marine biotechnology products is included in Table 3.
Figure 4. Marine biotechnology products pyramid values. Adapted from Day et al. (2016). The price/quantity ratio is depicted by the areas of the triangles.
Table 3. A non-exhaustive overview of the European companies offering marine biotechnology products.
The development of marine natural products is typically connected with enormous financial investments to sustain experimentation costs (Giugni and Giugni, 2010), especially in the medical sector. Intellectual property, primarily in the form of patents, facilitates the commercialization potential (Starling-Windhof et al., 2020). The growing number of marine-based drugs that are entering clinical trials is paired by a cumulative increase in the number of published patents (Mandhare et al., 2019). When successfully passing all the clinical trials phases of the drug discovery pharmaceutical pipeline, a natural product takes up to 15–20 years before entering the market (Taylor, 2015).
In terms of bioenergy, microalgae have been widely evaluated for the production of biodiesel, especially due to their chemical composition and global abundance (Chisti, 2007; Schenk et al., 2008; Singh and Dhar, 2011). Marine microalgae can contain high levels of lipids and their doubling rates exceed most terrestrial plants, making them suitable to produce biofuel. Together with a large liquid fuel market and the need to break the dependence on fossil fuels, renewable fuel generated from algae was a celebrated prospect. One of the approaches was to apply electro-extraction of valuable biofuel compounds from microalgae, avoiding the use of solvents and other chemicals (Brennan and Owende, 2010; Goettel et al., 2013). However, while the technology is well-known and technically feasible, producing biodiesel from microalgae is not economically feasible with the current technology (Williams and Laurens, 2010; Quinn and Davis, 2015; Abdo et al., 2016; Novoveska et al., 2018). The main reason for this is that microalgae cannot be grown at sufficiently dense cultures due to their inherent demand for light. As the culture grows denser, the microalgae become photon deprived due to self-shading, which limits the maximum achievable density of the culture. In addition, harvesting costs are high as the biomass must be separated from large volumes of water. Currently, the research on genetic modification of marine microalga Nannochloropsis and Phaeodactylum tricornutum for biofuel production is still ongoing to overcome these obstacles (Du et al., 2018; Nymark et al., 2019).
Seaweed aquaculture can contribute to a sustainable supply of biomass for profitable biofuel production especially in the form of biogas and bioethanol, with the advantage of not competing with food crops for land or freshwater resources (Borines et al., 2011; Fernand et al., 2017). However, biogas and bioethanol production should focus on the use of residual and overabundant marine biomass to avoid competition with the biomass requirements of the seaweed biopolymers industry. The simultaneous production of combustible biomethane and disposal of undesirable marine biomass in a synergistic waste management system is a concept with environmental and resource-conserving advantages (Barbot et al., 2016). In addition to the direct use of biomass for biofuel, seaweed can be used in renewable energy systems as an alternative to solid electrolytes used in dye-sensitized photovoltaic cells. Research conducted by Bella et al. (2015) on derived products from algae using green chemistry and multivariate-based preparation methods and smart activation via spontaneous sublimation, provides a concrete starting point for third generation solar cells. These findings are also supported by Anand and Suresh (2015) who suggested that the exploration of vast marine pigment resources for their potential use as sensitizers in solar cells could provide low-cost and environmentally friendly alternatives to expensive ruthenium metal complex.
A large portion of human food and animal feed may need to be sourced from sustainable marine origins to address future food supply challenges, i.e., an increasing human population and a decline in terrestrial food resources (Olsen, 2011).
Whole marine organisms can be a source for innovative or new foods, especially those that are adapted from diets, incorporating seaweed or jellyfish, that are common in specific geographical areas. Seaweeds have traditionally been used in Asia and Northern Europe as food ingredients, and are recently becoming more popular throughout European cuisine, although they are still considered an unusual foodstuff (Barbier et al., 2020). There is a current trend of consumers adopting organic, local and “natural” foods from clean environments, which should increase acceptance and popularity of marine sourced foods/food ingredients. Jellyfish have been an important food source in Chinese cuisine for over 1,000 years (Hsieh et al., 2001). By adapting the harvesting (using local species), preprocessing (omitting the use of alum salts) and preparation techniques, jellyfish are more recently being introduced as a food source in western-style cuisine (also as part of scientific projects, supported by the European Commission; two of which are PULMO14 and GoJelly15). Therefore, opportunities exist to develop food-friendly processing protocols. However, strict regulatory mechanisms [e.g., Novel Food Regulation (EU) No 2015/2283] are another important bottleneck in the delivery of novel food (ingredients) from marine organisms. A novel food is defined as food that was not consumed to a significant degree within the EU countries before 15 May 1997, when the first Regulation on novel foods came into force [Regulation (EC) No 258/97; 2015/2283]. Furthermore, there are some safety considerations due to the bioaccumulation of toxic substances, which may present a risk of chronic poisoning, thus favoring culturing rather than harvesting, where possible. Seaweeds can accumulate heavy metals (arsenic, cadmium, mercury, and lead) when present in their surrounding environment. Therefore, in the EU, the identification and registration of potential toxic substances are covered by the REACH Regulation [Regulation (EC) No 1907/2006] and the allowed threshold values for some heavy metals in seaweed which are used as dietary supplements are covered within the Commission Regulation 1881/2006.
In addition to whole organisms, compounds from fish, shellfish, micro- and macroalgae, bacteria and fungi are used in the food and feed industries as natural preservatives, pigments, stabilizers, gelling agents, functional food ingredients, nutraceuticals, dietary supplements and prebiotics (Boziaris, 2014). Nutrition products with bioactive ingredients are envisioned to have medical benefits such as anticancer, antiinflammatory, antioxidant, antiosteoporotic, antimicrobial, antidiabetic, hypocholesterolemic, and adipogenesis inhibition. Marine-derived polymers are also used as gelling, stabilizing, thickening, flocculent, and binding agents. Examples include polyunsaturated and ω-3 fatty acids, astaxanthin, chitin, chitosan, chitooligosaccharides, chondroitin sulfate and glucosamine from shark cartilage and crustacean by-products, and spirulin from cyanobacteria (Boziaris, 2014; Harnedy and FitzGerald, 2015; Suleria et al., 2016). Moreover, the food and beverage industries use cold-active enzymes derived from marine organisms in the processing of heat-sensitive ingredients/products. The use of such enzymes avoids heat-induced changes to the nutritional and organoleptic properties of foods (Nikolaivits et al., 2017). The efficient release of the bioactive compounds for application in the food and feed industries can be aided by marine-derived enzymes having unique catalytic specificity and the ability to operate under extreme conditions. If any compounds are considered novel foods, there may be additional requirements, e.g., the supply of a scientific dossier concerning their quality and safety, which may require human intervention studies to validate their efficacy and safety.
A scheme of the process of using marine organisms as a source of food or feed is shown in Figure 5. To efficiently use marine organisms as a source of food and feed, the process of identification, isolation and nutritional value analysis is used to select species that could be used to produce food and feed. The next step represents the establishment of cultivation systems and feeding experiments that generate sufficient quantities of food/feed, using a comparative analysis with a control group that uses classic food/feed sources to compare the overall quality and palatability with the existing products.
Figure 5. The potential for using marine organisms in food and feed industries and for bioremediation processes.
Fish meal is characterized by high protein contents, digestible energy and balanced composition of essential amino acids, minerals, and vitamins (Kaushik et al., 2004), while fish oil contains long-chain PUFAs and are mostly used in animal feed formulations to raise aquaculture and terrestrial livestock. Although vast improvements have been made both in feed conversion efficiency and in utilizing waste streams (discards, offal), this situation is not sustainable (Guttormsen, 2002). The fish feed industry is therefore looking for sources of oils to replace fish oils and microalgae are a promising source, not only for essential lipids, but also for essential amino acids (Dineshbabu et al., 2019; Tibbetts et al., 2020). In addition, live feeds (in contrast to formulated feeds) are indispensable for the rearing of larvae of most marine fish species (Nielsen et al., 2017). Live feeds are usually based on Artemia or rotifers, while copepods are also becoming more common as live feeds, having a superior lipid composition, compared to other live feed organisms (Nielsen et al., 2017). To produce live feed organisms, the production of their prey organisms, typically microalgae, is necessary, and the microalgae have to be optimized for their content of essential lipids and amino acids for feeding zooplankton organisms (Vu et al., 2016).
Marine phytobiomass is currently being explored for different purposes in the agricultural sector (i.e., fertilizers, heat production in biogas plants, feeds) with large quantities available along the coastlines (Mossbauer et al., 2012). Biofertilizers are products containing living microorganisms or natural substances that can improve chemical and biological soil properties, stimulating plant growth, and restoring soil fertility (Ronga et al., 2019). Historically, seagrasses and seaweeds were used as organic soil amendments in coastal areas to increase soil fertility and harvest yields (Acksel et al., 2017; Michalak and Chojnacka, 2018; Franzén et al., 2019). Marine biomass can also be used for crop protection. As an example, Posidonia oceanica was found to reduce weed pressure after application (Grassi et al., 2015) and the extraction of venom from jellyfish, namely Rhopilema esculentum and Nemopilema nomurai, can be used as a natural insecticide (Yu et al., 2005; Yu H. et al., 2015). The chemical properties of marine biomass applied to land might also affect the microbial decomposition leading to improved soil structures and increased carbon sequestration rates with positive feedbacks to land restoration and the climate. The long-term application of marine biomass to soil formed humus-rich top-soils. The tannins in the biomass influenced the residence time of organic matter (Kagiya et al., 2019). Accordingly, the exploration of marine biomass and their extracts have the potential to push the agriculture sector toward new market chains and more sustainable production. But the short-term availability of nutrients is slower in comparison with other conventional fertilizers. In response to this, controlled composting processes were developed to increase nutrient use efficiency. They can however cause environmental trade-offs due to increased risk of N2O and NH3 releases during the compost production (Han et al., 2014). In addition, marine biomass can exceed the legal thresholds of undesired substances due to their ability to accumulate anthropogenic chemicals (Malea et al., 2018; Franzén et al., 2019). Thus, the use of marine biomass directly as organic fertilizer in crop production requires additional research and innovation, particularly to provide biomass with a homogenous structural and chemical quality. In any case, the establishment of either resilient production chains for processing beach wrack biomass (Emadodin et al., 2020) or a predictable yield of farmed seagrass and seaweed are necessary to ensure that the direct biomass use for agriculture will become economically resilient in the future (Chiaiese et al., 2018; Philis et al., 2018).
Plant biostimulants are nowadays used to enhance the nutrition efficiency, abiotic stress tolerance, crop yield and quality traits (du Jardin, 2015; Popko et al., 2018). Biostimulants are extracts derived from organic material that can stimulate the growth and development of several crops under both optimal and stress conditions. Biostimulants are heterogenic, representing a composite of polysaccharides, minerals, vitamins, oils, fats, acids, pigments, and hormones (El Boukhari et al., 2020). Protein hydrolyzates have been reported to exert stimulatory effects on plants (Colla et al., 2017). Extracts made by seaweeds, microalgae and cyanobacteria have been identified to contain phytohormones (as auxins, cytokinins, gibberellins) and plant growth regulators (as abscisic acid, jasmonic acid, polyamines, ethylene) which are known to play key roles in plant growth, development and defense (Sharma et al., 2014). Moreover, high protein content in some cyanobacteria with specific amino acid profiles are sought to provide amino acids for key phytohormones (Sharma et al., 2014; García-Gonzalez and Sommerfeld, 2016; Chiaiese et al., 2018; Mógor et al., 2018). Microalgal extracts are therefore increasingly used as biostimulants and biofertilizers in agriculture. Finally, chitosan can be used as a coating for fertilizers, pesticides, herbicides, nematocides, and insecticides for their controlled release to soil and chitosan films are used to coat seeds and leaves to prevent microbial infection (Sudha et al., 2014).
Marine biotechnology offers a broad range of bioremediation applications (either using whole cells or their metabolites, Figure 5). Marine invertebrates have been used in antifouling management and control, particularly sponges (Stowe et al., 2011; Ganapiriya et al., 2012). Bacteria from the phyla Proteobacteria, Actinobacteria, Cyanobacteria, Bacteroidetes, and Firmicutes can be used as an alternative process for the degradation of aromatic pollutants, such as polycyclic aromatic hydrocarbons (Nikolaivits et al., 2017). These bacterial communities have a great biodegradation potential for various types of hydrocarbons, aromatics and carbohydrates in oil-polluted sediments and petroleum spills (Atlas and Hazen, 2011; Acosta-González and Marqués, 2016). Hydrocarbon-degrading bacterial taxa have been uncovered on plastic marine debris using HTS, which supports the theory of potential plastic degradation in the ocean by consortia of microbial taxa (Zettler et al., 2013). Microorganisms synthesize enzymes that can degrade plastics, e.g., lipases, alkane hydroxylases, laccases, and others. However, the interactions between plastic and microbial consortia need to be further investigated to provide realistic mitigation measures (Urbanek et al., 2018). Marine fungi represent potential bioremediation agents: fungi that produce lignin-degrading enzymes are used in decolorization of highly colored effluents from paper, pulp mills, textile and dye-making industries (D’Souza et al., 2006), they also have strong oil degradative capabilities (Simister et al., 2015; Bovio et al., 2016) or are used in the bioremoval of copper and zinc from sediments (Cecchi et al., 2019). Both micro- and macroalgae have been shown to remove nutrients, heavy metals, some pharmaceutical compounds and even capture free floating microplastics from surrounding waters (Sundbæk et al., 2018; Mondal et al., 2019; Bulgariu and Bulgariu, 2020). Additionally, seaweed farms provide habitat and shelter for a variety of marine organisms. Due to their high detoxification efficiency, low cost and demand for nutrients, seaweeds and seagrasses could be used as biosorbents to remove pollutants from wastewater (Pennesi et al., 2015).
Microalgae can also bioaccumulate heavy metals. Therefore, they are used in bioremediation of effluents from industries with toxic heavy metals (Dwivedi, 2012; Rath, 2012; Kumar et al., 2015; Zeraatkar et al., 2016). Among them, Chlorella, Scenedesmus, Tetraselmis, and Arthrospira are reported to bio-sequester toxic heavy metals with high uptake capacity (Pérez-Rama et al., 2002; Aksu and Dönmez, 2006; Gokhale et al., 2008; Şeker et al., 2008; Mirghaffari et al., 2015). Microalgae remove heavy metals through adsorption or absorption mechanisms (Brembu et al., 2011). Roy et al. (1993) reported that the sorption of heavy metals is a two-step process. The first step is rapid surface adsorption by cell wall polysaccharides and other functional groups such as carboxyl, hydroxyl, sulfate, and other charged groups, which differ in affinity and specificity for various organic and inorganic compounds (Crist et al., 1981; Volesky, 1990; Bulgariu and Gavrilescu, 2015). The second step is a slow process that requires energy for the uptake of heavy metals into the cell interior (Wang et al., 2010). Like other organisms, microalgae synthesize metal-binding peptides, namely the cysteine rich metallothionein, which neutralize the toxic effects caused by heavy metals (Cobbett and Goldsbrough, 2002; Perales-Vela et al., 2006).
In addition to heavy metal biosorption, microalgae have bioremediation potential of emerging contaminants, primarily synthetic organic chemicals (Sutherland and Ralph, 2019). Emerging contaminants typically fall into several broad categories, including pharmaceuticals, personal care products, illicit drugs, artificial sweeteners, pesticides, plasticizers, and flame retardants (Petrie et al., 2015; Norvill et al., 2016; Tran and Gin, 2017). Reported rates for adsorption of emerging contaminants have been variable, with adsorption rates reported to be from 0 to 100% (Peng et al., 2014; de Wilt et al., 2016; Guo et al., 2016; Ali et al., 2018; Gojkovic et al., 2019).
Macroalgae are dominant primary producers in coastal areas and open seas, but a substantial part of this biomass is transported to deep sea and sediment, where the macroalgal carbon is sequestered from the atmosphere (Krause-Jensen and Duarte, 2016; Ortega et al., 2019). Micro- and macroalgae have recently been getting attention due to their potential for C-capture which is important to mitigate climate change (Matsunaga et al., 2005; Vaz-Pinto et al., 2014; Singh and Dhar, 2019), and can be further used for value-added products using the biorefinery approach. Further, they are of strategic importance to provide bio-based fertilizers from marine organisms as the compost may increase the carbon storage within the soil, thus reducing carbon dioxide emissions (Radziemska et al., 2019). Moreover, when seaweeds are used as a feed additive, the methane emission from cattle is reduced. Ruminants and mainly cattle are considered as a major culprit in the emission of greenhouse gas, mainly in the form of enteric methane (Herrero and Thornton, 2013) with a high impact on climate change. This causes high pressure on the livestock sector forced to take mitigating actions to reduce the production of greenhouse gasses, but on the other hand, it needs to increase production efficiency because of the ever-increasing population and the globally changing consumption patterns. Ruminant production is currently the largest anthropogenic contributor to the global methane budget (Dangal et al., 2017). To address this environmental challenge, the potential of the marine red seaweed Asparagopsis taxiformis added as a feed amendment to reduce enteric methane emission was evaluated. This resulted in methane reduction of 98% without inhibiting the fermentation process or live-weight-gain on beef cattle (Kinley et al., 2020; Roque et al., 2019). At this stage, a few – mainly tropical – seaweed species have been evaluated via in vitro studies for their methane reducing capacity (Machado et al., 2014).
Among metazoans, sponges have been investigated as a natural bioremediation solution (Fu et al., 2007; Stabili et al., 2008), due to their capacity, as active filter-feeders, to primarily feed on the ultraplankton fraction (less than five microns particle size) of the particulate matter (Pile, 1999), along with dissolved organic matter (de Goeij et al., 2008a,b) in the surrounding seawater. Additionally, sponges – or their microsymbionts – show the capacity to accumulate metallic trace elements, as well as various organic pollutants (Bauvais et al., 2015; Gentric et al., 2015), rendering them prominent candidate bioindicators or bioremediators.
Marine compounds can be incorporated into skincare and make-up products. Their drug-like benefits produce pharmaceutical hybrids in which the bioactive ingredients are added to the topical or oral cosmetics to produce a cosmeceutical with enhanced properties. There are examples of products that are already on the market derived mostly from microorganisms (bacteria, microalgae, fungi), but also from macroalgae, fish and corals (Martins et al., 2014; Corinaldesi et al., 2017; Brunt and Burgess, 2018). Examples of substances of interest for cosmetic applications are (i) mycosporine-like amino acids (MAAs), produced by marine organisms under high UV stress (cyanobacteria, micro- and macroalgae). These compounds absorb UV radiation between 310 and 360 nm and are considered as photoprotective and antiageing agents (de la Coba et al., 2009); (ii) exopolysaccharides, produced by several microorganisms that increase the moisture content of the skin (Satpute et al., 2010); (iii) carotenoid and polyphenolic compounds that can act as antioxidants and also have anti-inflammatory properties (Sachindra et al., 2007; Lopes et al., 2016; Mourelle et al., 2017); and (iv) enzymes and peptides that may act as anti-aging agents by protecting collagen stores (Chen et al., 2011). Potassium alginate and fucoidan from brown algae, aluminum silicate from sea mud, chitin from crustaceans, shell “powder” from oysters, and carrageenan from red algae are some examples of less differentiated but widely used marine active ingredients. Marine jellyfish and fish-derived collagen (developed also within the GoJelly project14) and gelatin are also excellent functional ingredients for the cosmetic industry. Importantly, many of the marine skincare products that are already in the market are not pure compounds but treated extract or enriched mixtures (Martins et al., 2014).
Examples of cosmeceuticals used for hydrating, moisturizing, anti-wrinkle and anti-aging that use algal extracts are, among others: Biotherm® by Blue Therapy, La Mer®, Elemis®, OceanBasis®, Guam algae® by Lacote, and La Prairie®. Microalgae extracts are used in Dermochlorella DG® by CODIF Research & Nature, XCELL-30® by Greensea, Alguronic Acid® by Algenist and Alguard® by Frutarom. Fungal extracts are used in Eyedeline and Brighlette by Lipotec. Resilience® by Estée Lauder uses pseudopterosin typetricyclic diterpene glycosides isolated from soft corals. They show wound-healing, anti-inflammatory and analgesic properties to prevent irritation caused by exposure to the sun or chemicals. Other interesting and potent marine-derived cosmetic ingredients are Hyadisine®, Antarticine®, and Hyafini®, derived from extremophilic marine microorganisms (Martins et al., 2014). Marine polymers are increasingly used in cosmetic products, e.g., SeaCode® by Lipotec with a mixture of extracellular glycoproteins and other glucidic exopolymers produced by fermentation of a Pseudoalteromonas sp. from Antarctic waters for soothing and reducing irritation of sensitive skin against chemicals, and with hydrating, anti-wrinkle and expression lines attenuator properties. Despite these marketed examples, the marine environment remains an undervalorized resource for cosmetic discovery.
Biomolecules and biomaterials from marine sources have useful characteristics such as increased salt tolerance, pressure tolerance, cold adaptivity, and heat tolerance. They may have novel physical, chemical/stereochemical as well as original biochemical properties (Trincone, 2011). Although there are wide possibilities for use of marine based products for the development of bio-inspired materials in medicine (such as replacement heart valves, bone implants or hips and joints, Khrunyk et al., 2020), there are still several challenges that must be solved. For instance, the isolation and purification of the biopolymers play an important role in targeted drug delivery and control of the sustained drug release concentrations. Moreover, the reproducibility of materials composition maintaining the same properties from the same species, regions and even seasons, is another challenge that needs to be solved. Algae, jellyfish, sponges, ascidians, mussels, crustaceans, have been reported as commercially important, new, and potential biomaterial resources. Their polysaccharides, enzymes, peptides, lipids, pigments, bioceramics, biominerals, and toxins can be used in the biomedical field. Applications of these materials include hard and soft tissue engineering, bio-adhesives, dental biomaterials, and drug and cell delivery systems. Bioactive ceramic materials are developed from corals, shells and sea urchins, using them as sources for hydroxyapatite synthesis, which is the main inorganic material in bone structure (Palaveniene et al., 2018; Pawelec and Planell, 2019; Haugen et al., 2019). The structural organization of marine organisms, particularly sponges, has inspired many technical solutions in fabrications of biomaterials, architecture and aerodynamics (Macha et al., 2019). Several sponge taxa are known to produce inorganic skeletal elements (spicules) composed of amorphous hydrated silica, through an enzymatic process (Voigt et al., 2017). This process, along with properties of the sponge-produced siliceous structures has led to several mimicking attempts targeting biomaterials for mainly biomedical (Müller et al., 2006, 2007; Schröder et al., 2007; Barros et al., 2016) or optical applications (Müller et al., 2009). Silica-based materials are used in many high-tech products including microelectronics and optoelectronics. Further, the silica-forming enzymes, silicateins, from both demosponges (marine and freshwater sponges) and hexactinellid sponges can be used for the production of highly ordered inorganic–organic composite materials with defined optical, electrical, and mechanical properties (Schröder et al., 2009). Diatoms are valuable in terms of their waste as they produce nanostructured and mesoporous biosilica shells (frustules) with a highly ordered hierarchical architecture. These unique, morphological, chemical, and mechanical properties make the biosilicate of diatoms a very attractive nanomaterial for a wide range of applications (Pletikapić et al., 2012). Diatom frustules have good mechanical properties, low density and a high surface area, hence they can be used as fillers to improve the mechanical properties of polymers (Lamastra et al., 2017). Some studies have shown that solid frustules increase the modulus and yield strength of the epoxy matrix (Taşdemirci et al., 2008; Gültürk et al., 2013). The application of various wastes as additives for composite production has attracted great interest to the scientific community due to its beneficial environmental effects: (i) waste material management, (ii) production of biodegradable products, and (iii) cost.
Recent developments of electronic devices that possess functionalities of biological synapses are used to advance in mapping the functions of the human brain. In this perspective, biocompatible artificial synapses based on seaweed matrix biopolymer (ι-carrageenan) with silver dynamics added, has the potential for constructing neuromorphic systems, using an environmentally benign material (Kim and Lee, 2018). Oyster shells, chitin from the exoskeleton of crustaceans and collagen extracted from cartilaginous or bony fish skin byproducts can be used to produce biomedical scaffolds (Gheysari et al., 2020). Biopolymers, such as sodium alginate, carrageenan and fucoidan derived from marine algae, chitosan obtained by deacetylation of the chitin extracted from the exoskeleton of crabs and shrimps, and porous silica shell of marine diatoms are used for the development of drug and cell delivery systems, hydrogels and bioactive coatings and also scaffolds for next generation of tissue engineering products (Figure 6, Kim, 2013; Posocco et al., 2015; Cardoso et al., 2016; Perale and Hilborn, 2016; Roman et al., 2019), and nanotoxicity studies (Ciglenečki-Jušić and Svetličić, 2015). The potential of the isolated algal plasma membrane is also underexplored. The plasma membrane is highly permeable for anionic fluorescent dyes, thus a method for sealing reconstructed plasma membranes would increase the attractiveness of these transport vehicles toward the development of a new generation of drug delivery systems (Ivošević DeNardis et al., 2020). Collagen can also be extracted from jellyfish to produce medical devices and biomaterials (such as scaffolds and hydrogels) useful for wound healing and regenerative medicine. This is the so-called next generation collagen by Jellagen PTY Ltd16, already on the market as biomaterial for 2- and 3-D cell cultures and as a universal collagen scaffold. Fish-derived gelatin is another alternative to commonly used gelatin in medical devices, usually sourced from bovines or porcine hides and skins (Gelatin Manufacturers Institute of America [GMIA], 2019). Indeed, marine-animal sourcing of gelatin can ensure a higher standard of safety as it does not carry the intrinsic risks of disease transmissions typical of farming animals (e.g., the Creutzfeldt Jakob disease risk, ISO 22442). These novel applications that represent a realistic alternative to currently used and sourced materials are foreseen in the new European regulatory framework for the medical devices industry, the Medical Devices Regulation [Regulation (EU) 2017/745].
The global study by Geyer et al. (2017) showed that in 2015 only 9% of world-wide plastics were recycled, while 12% was incinerated and 79% was disposed to landfills and natural environment. This can represent an opportunity to use macroalgae and microalgae as a potential feedstock to produce sustainable, recyclable/and or compostable plastic while offsetting our carbon footprint. Algal biomass can be used for packaging in a variety of ways: firstly, raw seaweed fronds are pre-treated and used as single-use disposable plates (e.g., serving fish on a pre-treated, dried and shaped blade of seaweed). Secondly, several compounds can be extracted from algae to serve as precursors to produce films, lining for packaging or packaging material itself (bioplastics). Currently, the most common algae-based precursors for bioplastics are poly-lactic acid (PLA), polyhydroxyalkanoates (PHAs), starch, cellulose, proteins, lipids and other polysaccharides such as alginates and carrageenans (Zhang C. et al., 2019). PLA is currently the compound with the most commercial interest. The monomer of PLA is a lactic acid which is derived from carbohydrates during fermentation and then polymerized into PLA. Many macroalgal and microalgal species are carbohydrate rich and therefore suitable as a feedstock for PLA production. However, due to the slow degradation of PLA, there is an active ongoing search for other polymers (Jem and Tan, 2020). In addition, macroalgae can serve as a substrate to cultivate marine bacteria capable of synthesizing PHAs, which are a biodegradable plastic alternative (Ghosh et al., 2019). Several species of cyanobacteria and microalgae have also been recorded to produce PHAs (Costa et al., 2019).
The increasing standard of life inherently represents a growing demand for pharmaceuticals, nutraceuticals and cosmeceuticals. Marine organisms, such as algae, sponges, mollusks (including cone snails), cyanobacteria, actinobacteria, fungi, tunicates and fish biosynthesize metabolites with significant biological activities for therapeutic and industrial applications, with anticancer, anti-inflammatory, anti- and pro-osteogenic, anti-obesity, antimicrobial, antiviral, and anticoagulant activities (Majik et al., 2014; Surget et al., 2017; Carson et al., 2018a,b; Jin Q. et al., 2018; Kumar, 2019; Mayer et al., 2020). To date, seventeen clinically approved drugs of marine origin include: cytarabine (Cytosar-U®), nelarabine (Arranon®), fludarabine phosphate (Fludara®), trabectedin (Yondelis®), eribulin mesylate (Halaven®), brentuximab vedotin (Adcetris®), plitidepsin (Aplidin®), polatuzumab vedotin (PolivyTM), enfortumab vedotin-ejfv (PADCEVTM), and more recently, lurbinectedin (Zepzelca®) and belantamab mafodotin (BLENREP®) for cancer treatment, ziconotide (Prialt®) for severe chronic pain, ω-3-acid ethyl esters (Lovaza®), eicosapentaenoic acid ethyl ester (Vascepa®), and ω-3-carboxylic esters (Epanova®) for hyper-triglyceridemia treatment, and ι-carrageenan (carragelose) and vidarabine (Vira-A®; US discontinued17) for antiviral treatment (Martins et al., 2014; Jimenez et al., 2020). These lists have prospects of increasing soon as dozens of marine natural products are currently under clinical trials.
Compounds originating from marine organisms are also used in nutraceuticals, healthcare and well-being. Carotenoids are pigments that serve as antioxidants with many health benefits including prevention or slowdown of some chronic diseases, cellular damage and aging. Specifically, carotenoids have the potential to reduce the risk of inflammation, heart disease, cancer (Griffiths et al., 2016), type 2 diabetes (Sluijs et al., 2015), obesity (Gammone and D’Orazio, 2015) and even some neurodegenerative diseases (Cho et al., 2018). Phlorotannins are bioactive compounds from brown seaweeds with potential use in food, pharmaceutical and cosmeceutical industries (Li et al., 2011). The cyanobacteria from the Baltic Sea, both the bloom-forming species such as Nodularia spumigena (Fewer et al., 2013; Niedermeyer et al., 2014) and the cyanobacterial species that are rarely reported in the sea (Mazur-Marzec et al., 2015), produce metabolites of pharmaceutical potential. Dolastatin 10, produced by the cyanobacterium Symploca sp. was of great interest as an anticancer drug due to its potent in vitro anticancer activity. However, it was dropped from Phase II clinical trials due to its toxic side-effects and the lack of significant clinical activity (Perez et al., 2005; Tidgewell et al., 2010). Many analogs of dolastatin were synthesized and tested through the years and their use as payload in antibody-drug conjugates (ADC) allowed their successful use in the clinics, as seen with brentuximab vedotin (Adcetris®), now used as a treatment for different types of lymphomas (Tan, 2013; Jager and Hutchings, 2015). Nostoc sphaeroides and other cyanobacteria have been used for the treatment of diarrhea, hepatitis and hypertension. N. sphaeroides is also used as an important ingredient of medicines (Barsanti and Gualtieri, 2014). N. ellipsosporum produces a bioactive compound called cyanovirin, a low-molecular-weight protein with potent activity against various human immunodeficiency viruses type 1 (HIV-1), HIV-2 and simian immunodeficiency viruses (Boyd et al., 1997; Dey et al., 2000; Lotfi et al., 2018). The extracts of the growth media and cell extracts of unicellular microalgae Chlamydomonas pyrenoidosa and Chlorella vulgaris possess antibacterial activity against both Gram-negative and Gram-positive bacteria (Hussein et al., 2018). Similarly, extracts of diatoms, green algae and dinoflagellates have antifungal activities (Dewi et al., 2018). Genomes of higher eukaryotes encode hundreds of kinases, many of which having important roles in controlling the molecular machinery of cell proliferation, survival and motility. Recent studies of the arctic marine hydrozoan Thuiaria breitfussi revealed a family of bioactive breitfussins, molecules that act as cell specific kinase inhibitors (Hansen et al., 2019). Two of the breitfussins were shown to selectively inhibit the survival of several cancer cell lines. The highest inhibition was verified for the triple negative breast cancer cell line MDA-MB-468. These results open a very promising avenue for the development of selective kinase inhibitors for use in cancer therapy. It is also worth noticing that the compounds were isolated from a sessile marine organism, which may hint on where to find potential cell active compounds (i.e., defense related molecules). Recently, fermented Pacific oyster extracts (Ihn et al., 2019) have proven to be efficient in inhibiting osteoclastogenesis in rodents, thus being proposed as a possible treatment for another highly prevalent human pathology, osteoporosis, which has greatly increased in the human population with the increase in life expectancy.
Among marine organisms, sponges have been considered as a “drug treasure” during the past 70 years, due to the huge diversity of their secondary metabolites with an equal variety of biotechnological properties (Schröder et al., 2003; Müller et al., 2004; Perdicaris et al., 2013). In the early 1950s, pharmaceutical interest for marine sponges started by the investigation of the Caribbean sponge Tectitethya crypta (=Cryptotethya crypta de Laubenfels, 1949) and extraction of the nucleosides: spongouridine (3-β-D-arabofuranosyluracil), spongothymidine (3-β-D-arabofuranosylthymine) and spongosine (9-β-D-ribofuranosyl-2-methoxyadenine) (Bergmann and Feeney, 1950, 1951; Bergmann and Burke, 1956). These unique nucleosides were the basis for the synthesis of the antiviral drug ara-A, as well as the first marine-derived anticancer agent, ara-C, currently used in the routine treatment of patients with leukemia and lymphoma (Proksch et al., 2002). Besides the above-mentioned bioactivities, sponges produce many immunosuppressive, neurosuppressive and muscle relaxant compounds (Anjum et al., 2016). Numerous bioactive substances, important in public health disease treatment and control, have been isolated from marine sponges. The most promising drugs are those for treatment of malaria, manzamines, with antiplasmodial (Yousaf et al., 2002) and even immunomodulating activity (Ang et al., 2001), as well as activities against atherosclerosis (Stead et al., 2000), cardiovascular diseases (Barrese and Taglialatela, 2013) and against the novel corona virus 2019-nCoV (Vijayaraj et al., 2020). A group of particular interest is the microtubule-stabilizing drugs, potent macrolide secondary metabolites isolated from New Zealand marine sponge Mycale hentscheli: peloruside A, mycalamide A and pateamine as well as zampanolide from the Tongan sponge Cacospongia mycofijiensis (Miller et al., 2010). Peloruside A stabilizes microtubules and thus possesses promising activities against cancer, neurodegenerative and autoimmune diseases (Kanakkanthara et al., 2016). A recently identified microbiome of sponge host Mycale hentscheli shows remarkable chemical diversity and biosynthetic potential of multiple symbionts, including microtubule-inhibiting and eukaryotic translation-inhibiting bioactive compounds (Rust et al., 2020).
Jellyfish extracted molecules (proteins, peptides, mucins) have antioxidant, wound healing and antimicrobial properties (Merquiol et al., 2019; Nudelman et al., 2019). The most famous jellyfish-derived compound is the green fluorescent protein (GFP), one of the most important tools in molecular biology research, serving as a molecular marker alongside other that found important applications in wider scientific research, and was awarded the Nobel Prize in Chemistry in 2008 to Osamu Shimomura, Martin Chalfie, and Roger Tsien.
The potential of marine biotechnology may significantly contribute to achieving 14 out of 17 United Nations (UN) Sustainable Development Goals (SDGs)18. First and foremost, the discovery of biodiversity and the sustainable use of marine bioresources contributes to SDG14 (Life below water). The new solutions and processes developed, such as new bioremediation methods or alternative fertilizers contribute to SDGs 6 (Clean water and sanitation), 13 (Climate action) and 15 (Life on land). Importantly, alternative food, feed and fertilizer sources contribute to SDG2 (Zero hunger). The use of marine algal biomass as an alternative energy source contributes to SDG 7 (Affordable and clean energy). The valorization of waste, also food by-products can contribute to the decrease of urban pollution (SDG11, Sustainable cities and communities). The development of new products in nutraceutical, cosmeceutical and medical industries contributes to SDG3 (Good health and well-being). Promotion of resource efficiency and technological development contribute to SDG12 (Responsible consumption and production) and 9 (Industry, innovation and infrastructure); the establishment of partnerships between governments, industry, civil society and the scientific sector contributes to SDG17 (Partnerships for the goals). Indirectly, successful biotechnological development can lead to new products, new industries and new job openings. With a proactive interregional collaboration, good practice examples can be taken by regions that are lagging in terms of regional development and job security, which contributes to SDGs 1 (No poverty), 10 (Reduced inequalities), and 8 (Decent work and economic growth).
Table 4 briefly outlines the strategies and funding mechanisms in the EU that directly or indirectly tackle marine biotechnology. They can also serve as a tool for streamlining current efforts and consolidating future directions. Depending on the funding scheme these tools can act at European-, regional-, national-, and bilateral-scale. The European Technology Platform for Sustainable Chemistry (SusChem) has issued a new Strategic Innovation and Research Agenda in 2019 with a vision where sustainable chemistry and biotechnology provide solutions for future generations. The SusChem priorities align well with the field of marine biotechnology as the priorities are (i) advanced materials and advanced processes for circular economy and resource efficiency, and (ii) low carbon economy toward mitigating climate change and protecting environmental and human health. The European Green Deal [COM(2019), 640] is an EU growth strategy to transition into a prosperous society with a resource-efficient and competitive economy. National funding mechanisms can sometimes provide partial financing through research programs and national projects. At the European scale, Horizon2020 and its successor, Horizon Europe framework programs are the ones directly funded by the EU budget. The marine biotechnology sector significantly benefits from the Horizon funding mechanism, intending to directly address societal challenges and promote the development of innovative societies through international cooperation and collaboration of academic and industrial partners. Some financing opportunities have limited country participation depending on the governmental organizations (national ministries) that typically endorse the respective national participation. Therefore, the establishment of collaborations between scientific institutions and the policy making sector is of extreme strategic importance. An example is the Action ERA-NET COFUND on Blue Bioeconomy – unlocking the potential of aquatic bioresources, which is currently running with limited participation from 16 European countries. Another source of funding at the European level stems from the European Regional Development Fund. Marine biotechnology is not uniformly represented as a strategic priority at the regional and transregional levels. The Interreg Baltic Sea Region, for example, has marine biotechnology at the core of blue growth. Marine biotechnology is also encompassed in the Interreg Mediterranean and Atlantic transnational collaborations. Another funding source is the European Maritime and Fisheries Fund. This is implemented at the national scale, through co-financing operational programs. Moreover, marine biotechnology is a part of the maritime economy, a high-potential economic sector. The co-funding programs come along with the national ones that are financed by the Public Investments Programs. Among the Joint Programming Initiatives (JPIs), JPI-Oceans has a priority in marine biotechnology, with limited country participation. To a lesser extent LIFE programs, funded by Environment Directorate-General, could also indirectly be used to better manage marine biodiversity.
Table 4. A list of European strategies and funding mechanisms that directly or indirectly include marine biotechnology.
During the whole development process, the policy framework imposes guidelines throughout the whole technology readiness level scale. The scientific and technological breakthroughs must also be addressed to local, national and international policies that protect and promote the ocean health and functioning aspects of ecosystems. In the field of marine biotechnology, the Convention on Biological Diversity (CBD) and the Nagoya Protocol on Access to Genetic Resources and the Fair and Equitable Sharing of Benefits Arising from their Utilization as well as the United Nations Convention on the Law of the Sea (UNCLOS) are of particular relevance (Lallier et al., 2014). Nagoya protocol has clear implications for scientists working on genetic resources, including those doing biotechnology research on marine organisms, as well as any user of genetic resources along the biodiscovery pipeline (Broggiato et al., 2018). Bioprospecting, a term that defines screening for new organisms and their compounds with biotechnological value, is controlled at different levels. In Exclusive economic zones, these resources are under the sovereignty of the coastal country, which requires special permits to sample habitats of interest. In many cases, bioprospecting in the waters of a third country is allowed only if the country provides its access and use of the genetic resources either for commercial interest or for academic research, even if the material taken is of negligible intrinsic value. The “gold mine” syndrome (according to which each crude sample contains a hidden treasure) hampers the ability of partners to agree on an a priori chain value (Querellou, 2010). The exclusive access to these potential economic benefits can only be obtained through patents associated with the use of “marine genetic resources” for an inventive purpose or process. Another challenge is the access and benefit sharing of resources collected in areas beyond national jurisdiction (Collins et al., 2019). The ocean is a common good and negotiations are necessary to find the solution for the fair and equitable benefit sharing from the utilization of marine genetic resources (Rabone et al., 2019).
Overall, there are currently many legal and practical challenges along the pathway for the commercialization of products derived from marine organisms as legislation is not progressing at the same rate as technology. Serious burdens that delay the market entry of products are the safety assessments and compliances for marine biotechnology products. Another clear practical challenge involves the potential spatial conflicts, in other words, the impacts of different existing marine uses such as tourism or maritime commerce, with the exploration and use of marine biota. The recent increase in interest in marine spatial planning over the past two decades has opened opportunities to overcome conflicts and to proactively determine simultaneous and integrated uses. The above mentioned Maritime Spatial Planning Directive (2014/89/EU) addresses this facet of marine uses. However, of note is the progress made by many non-EU countries who are also taking initiative to develop plans for their coastal and exclusive economic zones (Collie et al., 2013; Portman, 2016; Smythe and McCann, 2018).
Marine exploitation needs increased governance practices as well as ethical practices. Hence, policy involvement is necessary while developing products and processes from marine sources and this may be ensured through the Responsible Research and Innovation concept (see more in section “Communication and stakeholder engagement in development finalization”). This new perspective of conducting science which has to be transdisciplinary, tackling the complex interactions between nature, society and governance is nowadays called the social-ecological systems (Folke et al., 2005; Rozzi et al., 2015; Nakicenovic et al., 2016). This mode of governance takes into consideration different dimensions: economic (cost-efficiency), political, social, legal (European and national legislation) and scientific (e.g., environmental issues) ones. The marine biotechnology sector is thus complex, multi-dimensional and is facing uncertainties. Therefore, the adoption of governance adapted to social-ecological systems considering different aspects of this sector is challenging but necessary.
Transdisciplinary collaborations, such as marine biotechnology, seek to produce knowledge through integration and collaboration to address societal challenges (Misra and Lotrecchiano, 2018). These complex collaborations are high-risk, high-impact and are needed to establish modern, innovative societies (Rotter et al., 2020b). On one hand, this demands the creation of teams with varying expertise, including scientific organizations, industrial actors, policy makers and the civil society, i.e., the quadruple helix (Rotter et al., 2020b and Figure 4 therein). On the other hand, these collaborations need to establish efficient communication channels to share infrastructure, experts, expertise and data, which is endorsed by the open access policy of scientific information. These collaborations are endorsed by the Responsible Research and Innovation framework (RRI). RRI aims at an interactive process where societal actors, researchers and innovators actively cooperate to co-define, co-design and co-construct solutions, services and products that are socially acceptable, sustainable and resolve important societal issues (Theodotou Schneider, 2019). This ensures the uptake of the results in science, industry, policy and society. This is an increasingly popular narrative in the European policy making sector, oriented toward publicly funded research that should be inclusive, sustainable and involve policy makers and the general public (von Schomberg, 2013; Jakobsen et al., 2019). If the public perception of a certain product is not positive, the rest of the development may be put on hold. In fact, low public product awareness and acceptance is an important barrier for product commercialization. This is especially the case for commodity products, for which consumer appreciation is decisive, in contract to high end products like medical drugs and industrially valorizable compounds. One solution is the financing of transdisciplinary collaborative networks (such as the European COST Action Ocean4Biotech, Rotter et al., 2020a), where representatives from transdisciplinary communities can co-create knowledge to develop solutions that are efficient, safe, of general public interest and legally feasible.
There are three features within the RRI framework (Owen et al., 2012): (i) science is made for society defining societal challenges, setting targets and impact; (ii) science is made with society and innovations should be constantly iterated by monitoring the economic, social, environmental impact and including the general public and the policy making sector; and (iii) science is responsible and should include the principle of openness, ethics, and financial responsibility toward financers, technology or product users. As marine ecosystems become important in the quest for sustainable development, it is important to ensure that citizens will understand the cause-effect of societal actions and inactions and how human and ocean health are tightly connected. At the same time, citizens, scientists, policy makers and industry are responsible for the ocean’s health to ensure sustainable and long-lasting marine resources. Thus, the RRI roadmap (Theodotou Schneider, 2019) facilitates building trust among these diverse stakeholders, which is the prerequisite for systemic innovation. True and active involvement will increase our understanding of marine bio-based information, knowledge and upcoming marine bio-based products, and catalyze the increase of ocean literacy toward ocean health and sustainable marine biotechnology. Applying the RRI Roadmap will enable a faster industry uptake, where currently there is a lack of coordination and cooperation along the value chain as well as lack of knowledge and insufficient information exchange (Marine Board, 2010). Moreover, these barriers, together with the lack of effective training in the art of science communication, demand a structural change in the academic curricula, especially when training future marine biotechnologists. International marine biotechnology education programs (such as “A Blue Biotechnology Master for a Blue Career”19) are a way to transferring knowledge from the scientific community to the industrial sector for blue biotechnology business development by employing high skilled graduates.
Oceans harbor a vast variety of organisms that offer biological and chemical diversity with metabolic abilities unrivaled in terrestrial systems. Still, many of the known organisms and bioactive compounds have not been exploited to their full commercial and possibly also functional potential. Despite this, it is clear that marine contribution can address the societal challenges, especially health and wellbeing, demographic change (the increase of the global population and the need for sustainable food sources), climate change and resource efficiency (Hurst et al., 2016). Hence, in 2016, a marine biotechnology strategic research and innovation roadmap was presented, uncovering five thematic areas: enabling infrastructure, exploration of the environment, biomass production and processing, product innovation and policy support (Hurst et al., 2016). The year 2021 marks the start of the 10-year period that should enable the achievement of long-term goals and focus especially on the collaborative and financial efforts to advance in the development of all value chains as presented in Figure 4. Marine biotechnology is also embedded in various European territories through smart specialization strategies. These are policy approaches that are tailored based on the specific requirements in various European territories with the aim a smart, sustainable and inclusive Europe that will shift toward and resource-efficient blooming economy (Doussineau et al., 2020). Marine biotechnology in Europe is still in its early stages, evidenced by a high number of publications (globally, around a third of marine biotechnology publications are European), a low number of patents (globally, 13% patents filed in connection with new marine molecules are European) and a high knowledge fragmentation (most of the 12,500 blue biotechnology entreprises employ 10 people or less) (Doussineau et al., 2020). National initiatives have been supported in Europe, for instance in Norway and Ireland with the construction of a National Marine Biodiscovery Laboratory in Ireland20 (Gabrielsen, 2012; Bekkby et al., 2013). Regional initiatives in marine biotechnology, such as in the Germany’s northernmost state Schleswig Holstein has led to the establishment of the GEOMAR Centre for Marine Biotechnology, formerly KiWiZ in Kiel. Moreover, the continuous financial support is important for the regional development of the field, evidenced by past and current network initiatives (e.g., Submariner network21 in the Baltic sea basin, BLUEMED initiative22 and B-Blue23 in the Mediterranean Sea basin and Ocean4Biotech24 that centralizes all European expertise and beyond). Overall, we propose that regional national and transnational initiatives should be fostered to set up long-term inventories of marine biomaterial open to the public with a strong emphasis on taxonomy and geographic information (Leal et al., 2016). These initiatives stimulate the representatives from research, industry and policy sectors to jointly collaborate with the aim of transforming the results of scientific research work and technological breakthroughs into industrial, economic and commercial successes.
AR designed the article concept and drafted the manuscript. All authors contributed in writing, read, edited, and approved of the final version of the manuscript.
MB owns the private company Institue for Science & ethics. AM-G is employed by the private company AquaBioTech Limited. GP is founder and employee of Industrie Biomediche Insubri SA. CR is employed by private Norwegian Institute, Møreforsking AS. XTS owns the private company XPRO Consulting Limited. FB declares institutional research funds were received from Acerta, ADC Therapeutics, Bayer AG, Cellestia, CTI Life Sciences, EMD Serono, Helsinn, ImmunoGen, Menarini Ricerche, NEOMED Therapeutics 1, Nordic Nanovector ASA, Oncology Therapeutic Development, and PIQUR Therapeutics AG; consultancy fees from Helsinn and Menarini; that expert statements were provided to HTG; and travel grants from Amgen, Astra Zeneca, Jazz Pharmaceuticals, and PIQUR Therapeutics AG.
The remaining authors declare that the research was conducted in the absence of any commercial or financial relationships that could be construed as a potential conflict of interest.
AR, KK, and TR: the publication is part of a project that has received funding from the European Union Horizon 2020 Research and Innovation Programme under grant agreement no. 774499 – GoJelly project. AR and KK: this research was funded by the Slovenian Research Agency (research core funding P1-0245 and P1-0237). AR: this publication has been produced with financial assistance of the Interreg MED Programme, co-financed by the European Regional Development Fund (Project No. 7032, internal ref. 8MED20_4.1_SP_001) – B-Blue project. AB: acknowledges the support from the Research Council of Norway through the grant 267474 from the HAVBRUK2 program. MLC: acknowledges the Portuguese Foundation for Science and Technology (UIDB/04326/2020), the European Maritime and Fisheries Fund (MAR2020 OSTEOMAR/16-02-01-FMP-0057 and ALGASOLE/16.02.01-FMP-0058), the European Regional Development Fund (Atlantic Area BLUEHUMAN/EAPA/151/2016 and INTERREG V-A Spain-Portugal ALGARED+), and the European Commision (H2020-MSCA-ITN BIOMEDAQU/766347). MFC: wishes to acknowledge the funding from CEEC program supported by FCT/MCTES (CEECIND/02968/2017); ACTINODEEPSEA project (POCI-01-0145-FEDER-031045) co-financed by COMPETE 2020, Portugal 2020, ERDF and FCT; Strategic Funding UIDB/04423/2020 and UIDP/04423/2020 through national funds provided by FCT and ERDF. MC: financial support from the Programme of the Institute of Oceanology, PAS (grant no. II.3) and National Science Centre in Poland (project number NCN 2016/21/B/NZ9/02304). MCu: acknowledges the funding from the Ministerio de Ciencia e Innovación of Spain (SAF2009-0839 and RTA 2015-00010-C03-02) and INTERREG-MAC2/1.1b/279 (AHIDAGRO). AD-M: acknowledge financial support from INTERREG-MAC/1.1b/042 (BIOTRANSFER2) and Agustín de Betancourt Programme (Cabildo de Tenerife and Universidad de La Laguna). AD: work has been supported by the ERDF Activity 1.1.1.2 “Post-doctoral Research Aid” of the Specific Aid Objective 1.1.1, Operational Programme “Growth and Employment” (No. 1.1.1.2/VIAA/1/16/048). RJF: funding for this research was provided under the Marine Research Programme 2014–2020, through the Marine Institute of Ireland under grant PBA/MB/16/01 “A National Marine Biodiscovery Laboratory of Ireland (NMBLI)” and through the Food Institutional Research Measure, administered by the Department of Agriculture, Food, and the Marine, Ireland under grant issue 17/F/260 (MaraBioActive). SG: this work was supported by the Applied Molecular Biosciences Unit-UCIBIO which is financed by national funds from FCT/MCTES (UID/Multi/04378/2019). SG thanks financial support provided by FCT/MCTES through grant IF/00700/2014 and OceanTresaures project PTDC/QUIQUI/119116/2010. NID: wishes to acknowledge the funding from the Croatian Science Foundation Project CELLSTRESS (IP-2018-01-5840). MMa and TD: we wish to acknowledge funding from the General Secretariat for Research and Technology (GSRT) and the Hellenic Foundation for Research and Innovation (HFRI) under grant no. 239 (SPINAQUA project). AM-G: acknowledges the financial contribution from the project BYTHOS funded by the European Union’s Interreg V-A Italia-Malta Programme under project code C1-1.1-9. HM-M: financial support from National Science Centre in Poland 2016/21/B/NZ9/02304 and 2017/25/B/NZ9/00202. MMe: this work has been supported by the French Government, through the UCAJEDI Investments in the Future project managed by the National Research Agency (ANR) with the reference number ANR-15-IDEX-01. MMe: thanks the Canceropôle Provence-Alpes-Côte d’Azur, and the Provence-Alpes-Côte d’Azur Region for the financial support provided to the MetaboCell project. DO: supported by the Doctorate Study program in Ecology and Environmental Sciences, Marine Research Institute, Klaipėda University, Lithuania. CR: we gratefully acknowledge the Research Council of Norway, the Møre and Romsdal County Council and Møreforsking AS for their financial contributions through the PROMAC (244244; www.promac.no), the Norwegian Seaweed Biorefinery Platform (294946; http://seaweedplatform.no/), and the Blå-Grønn (55031) projects. ER: this work benefited from financial support from the PACA Canceropôle, the National Cancer Institute, the PACA Regional Council and the French Government, managed by the National Research Agency as part of the Université Côte d’AzurJEDI Investissement d’Avenir project (ANR-15-IDEX-01). JS: work was supported by the Slovenian Research Agency (P4-0127 and J4-1771). IS: financial support from Ministry of Education, Youth and Sports of the Czech Republic (project CZ.02.1.01/0.0/0.0/17_048/0007323). XT: the tool “RRI Roadmap” was developed as part of the European Horizon 2020 project MARINA “Marine Knowledge Sharing Platform for Federating Responsible Research and Innovation Communities” under the European Union’s Horizon 2020 Research and Innovation Programme under Grant Agreement No. 710566 (2016–2019). OT: his contribution is carried out with the support of the Marine Institute and is funded under the Marine Research Programme by the Irish Government (Grant-Aid Agreement No. PBA/MB/16/01).
This publication is based upon work from COST Action CA18238 (Ocean4Biotech), supported by COST (European Cooperation in Science and Technology) program.
The Supplementary Material for this article can be found online at: https://www.frontiersin.org/articles/10.3389/fmars.2021.629629/full#supplementary-material
Supplementary Figure 1 | Identification of key compounds in Microcystis aeruginosa PCC 7806extracts. 1 – Aeruginosin 684; 2 – M + 2H 392 & MH + 783; 3 – Linear MC-LR; 4 – M + 2H 615; 5 – MC-LR & DMLR (20%); 6 – Cyanopeptolin A; 7 – Aerucyclamide D; 8 – Cyanopeptolin 963A (–H2O); 9 – Aerucyclamide A; 10 – Aerucyclamide C; 11 – Aerucyclamide B. Other traces of cyanopeptolins, 895, B & C are also present.
Aasen, I. M., Ertesvåg, H., Heggeset, T. M. B., Liu, B., Brautaset, T., Vadstein, O., et al. (2016). Thraustochytrids as production organisms for docosahexaenoic acid (DHA), squalene, and carotenoids. Appl. Microbiol. Biotechnol. 100, 4309–4321. doi: 10.1007/s00253-016-7498-4
Abdelmohsen, U. R., Grkovic, T., Balasubramanian, S., Kamel, M. S., and Quinn, R. J. (2015). Elicitation of secondary metabolism in actinomycetes. Biotechnol. Adv. 33, 798–811. doi: 10.1016/j.biotechadv.2015.06.003
Abdel-Wahab, M. A., Dayarathne, M. C., Suetrong, S., Guo, S. Y., Alias, S. A., Bahkali, A., et al. (2017). New saprobic marine fungi and a new combination. Bot. Mar. 60, 469–488.
Abdo, S. M., Wahba, S. Z., Ali, G. H., El-Enin, S. A. A., El-Khatib, K. M., El-Galad, M. I., et al. (2016). Preliminary economic assessment of biofuel production from microalgae. Renew. Sust. Energ. Rev. 55, 1147–1153.
Abdul Wahab, M. A., de Nys, R., and Whalan, S. (2012). Closing the lifecycle for the sustainable aquaculture of the bath sponge Coscinoderma matthewsi. Aquaculture 324–325, 281–289. doi: 10.1016/j.aquaculture.2011.11.006
Abinaya, M., and Gayathri, M. (2019). Biodegradable collagen from Scomberomorus lineolatus skin for wound healing dressings and its application on antibiofilm properties. J. Cell. Biochem. 120, 15572–15584. doi: 10.1002/jcb.28824
Achamlale, S., Rezzonico, B., and Grignon-Dubois, M. (2009). Rosmarinic acid from beach waste: isolation and HPLC quantification in Zostera detritus from Arcachon lagoon. Food Chem. 113, 878–883. doi: 10.1016/j.foodchem.2008.07.040
Acksel, A., Kappenberg, A., Kühn, P., and Leinweber, P. (2017). Human activity formed deep, dark topsoils around the Baltic Sea. Geoderma Reg. 10, 93–101. doi: 10.1016/j.geodrs.2017.05.005
Acosta-González, A., and Marqués, S. (2016). Bacterial diversity in oil-polluted marine coastal sediments. Curr. Opin. Biotech. 38, 24–32. doi: 10.1016/j.copbio.2015.12.010
Adnan, M., Alshammari, E., Patel, M., Ashraf, S. A., Khan, S., and Hadi, S. (2018). Significance and potential of marine microbial natural bioactive compounds against biofilms/biofouling: necessity for green chemistry. PeerJ 6:e5049. doi: 10.7717/peerj.5049
Adpressa, D. A., and Loesgen, S. (2016). Bioprospecting chemical diversity and bioactivity in a marine derived Aspergillus terreus. Chem. Biodivers. 13, 253–259. doi: 10.1002/cbdv.201500310
Aksu, Z., and Dönmez, G. (2006). Binary biosorption of cadmium (II) and nickel (II) onto dried Chlorella vulgaris: co-ion effect on mono-component isotherm parameters. Process Biochem. 41, 860–868. doi: 10.1016/j.procbio.2005.10.025
Albarano, L., Esposito, R., Ruocco, N., and Costantini, M. (2020). Genome mining as new challenge in natural products discovery. Mar. Drugs 18:199. doi: 10.3390/md18040199
Al-Belushi, K. I. A., Stead, S. M., and Burgess, J. G. (2015). The development of marine biotechnology in Oman: potential for capacity building through open innovation. Mar. Policy 57, 147–157. doi: 10.1016/j.marpol.2015.03.001
Ali, M. E., El-Aty, A. M. A., Badawy, M. I., and Ali, R. K. (2018). Removal of pharmaceutical pollutants from synthetic wastewater using chemically modified biomass of green alga Scenedesmus obliquus. Ecotoxicol. Environ. Saf. 151, 144–152. doi: 10.1016/j.ecoenv.2018.01.012
Alipanah, L., Winge, P., Rohloff, J., Najafi, J., Brembu, T., and Bones, A. M. (2018). Molecular adaptations to phosphorus deprivation and the comparison with nitrogen deprivation responses in the diatom Phaeodactylum tricornutum. PLoS One 13:e0193335. doi: 10.1371/journal.pone.0193335
Álvarez-Viñas, M., Flórez-Fernández, N., Dolores Torres, M., and Domínguez, H. (2019). Successful approaches for a red seaweed biorefinery. Mar. Drugs 17:620. doi: 10.3390/md17110620
Alvarez-Yela, A. C., Mosquera-Rendón, J., Noreña, P. A., Cristancho, M., and López-Alvarez, D. (2019). Microbial diversity exploration of marine hosts at Serrana bank, a coral atoll of the seaflower biosphere reserve. Front. Mar. Sci. 6:338. doi: 10.3389/fmars.2019.00338
Amara, I., Miled, W., Slama, R. B., and Ladhari, N. (2018). Antifouling processes and toxicity effects of antifouling paints on marine environment. A review. Environ. Toxicol. Pharmacol. 57, 115–130. doi: 10.1016/j.etap.2017.12.001
Amend, A., Burgaud, G., Cunliffe, M., Edgcomb, V. P., Ettinger, C. L., Gutiérrez, M. H., et al. (2019). Fungi in the marine environment: open questions and unsolved problems. mBio 10:e01189-18. doi: 10.1128/mBio.01189-18
Anand, M., and Suresh, S. (2015). Marine seaweed Sargassum wightii extract as a low-cost sensitizer for ZnO photoanode based dye-sensitized solar cell. Adv. Nat. Sci. Nanosci. 6:035008. doi: 10.1088/2043-6262/6/3/035008
Andronopoulou, E., and Vorgias, C. E. (2004). Multiple components and induction mechanism of the chitinolytic system of the hyperthermophilic archaeon Thermococcus chitonophagus. Appl. Microbiol. Biot. 65, 694–702. doi: 10.1007/s00253-004-1640-4
Ang, K., Holmes, M., and Kara, U. (2001). Immunemediated parasite clearance in mice infected with Plasmodium berghei following treatment with manzamine A. Parasitol. Res. 87, 715–721. doi: 10.1007/s004360000366
Angelova, R., Baldikova, E., Pospiskova, K., Maderova, Z., Safarikova, M., and Safarik, I. (2016). Magnetically modified Sargassum horneri biomass as an adsorbent for organic dye removal. J. Clean. Prod. 137, 189–194. doi: 10.1016/j.jclepro.2016.07.068
Angly, F. E., Felts, B., Breitbart, M., Salamon, P., Edwards, R. A., and Carlson, C. (2006). The marine viromes of four oceanic regions. PLoS Biol. 4:e368. doi: 10.1371/journal.pbio.0040368
Anjum, K., Abbas, S. Q., Shah, S. A., Akhter, N., Batool, S., and Hassan, S. S. (2016). Marine sponges as a drug treasure. Biomol. Ther. 24, 347–362. doi: 10.4062/biomolther.2016.067
Arora, N., Patel, A., Mehtani, J., Pruthi, P. A., Pruthi, V., and Poluri, K. M. (2019). Co-culturing of oleaginous microalgae and yeast: paradigm shift towards enhanced lipid productivity. Environ. Sci. Pollut. Res. 26, 16952–16973. doi: 10.1007/s11356-019-05138-6
Atlas, R. M., and Hazen, T. C. (2011). Oil biodegradation and bioremediation: a tale of the two worst spills in U.S. history. Environ. Sci. Technol. 45, 6709–6715. doi: 10.1021/es2013227
Aune, G. J., Furuta, T., and Pommier, Y. (2002). Ecteinascidin 743: a novel anticancer drug with a unique mechanism of action. Anticancer Drugs 13, 545–555. doi: 10.1097/00001813-200207000-00001
Azumi, M., Ogawa, K., Fujita, T., Takeshita, M., Yoshida, R., Furumai, T., et al. (2008). Bacilosarcins A and B, novel bioactive isocoumarins with unusual heterocyclic cores from the marine-derived bacterium Bacillus subtilis. Tetrahedron 64, 6420–6425. doi: 10.1016/j.tet.2008.04.076
Bacon, C. W., and White, J. F. (2016). Functions, mechanisms and regulation of endophytic and epiphytic microbial communities of plants. Symbiosis 68, 87–98. doi: 10.1007/s13199-015-0350-2
Bakus, G. J., Targett, N. M., and Schulte, B. (1986). Chemical ecology of marine organisms: an overview. J. Chem. Ecol. 12, 951–987. doi: 10.1007/bf01638991
Baldi, F., Ivošević, N., Minacci, A., Pepi, M., Fani, R., Svetličić, V., et al. (1999). Adhesion of Acinetobacter venetianus to diesel fuel droplets studied by in situ electrochemical and molecular probes. Appl. Environ. Microbiol. 65, 2041–2048. doi: 10.1128/aem.65.5.2041-2048.1999
Barbier, M., Araújo, R., Rebours, C., Jacquemin, B., Holdt, S. L., and Charrier, B. (2020). Development and objectives of the PHYCOMORPH European guidelines for the sustainable aquaculture of seaweeds (PEGASUS). Bot. Mar. 63:1. doi: 10.1515/bot-2019-0051
Barbot, Y. N., Al-Ghaili, H., and Benz, R. (2016). A review on the valorization of macroalgal wastes for biomethane production. Mar. Drugs 14, 120. doi: 10.3390/md14060120
Barcelos, M. C. S., Vespermann, K. A. C., Pelissari, F. M., and Molina, G. (2020). Current status of biotechnological production and applications of microbial exopolysaccharides. Crit. Rev. Food Sci. Nutr. 60, 1475–1495. doi: 10.1080/10408398.2019.1575791
Barnay-Verdier, S., Dall’osso, D., Joli, N., Olivré, J., Priouzeau, F., Zamoum, T., et al. (2013). Establishment of primary cell culture from the temperate symbiotic cnidarian, Anemonia viridis. Cytotechnology 65, 697–704. doi: 10.1007/s10616-013-9566-2
Barrese, V., and Taglialatela, M. (2013). New advances in beta-blocker therapy in heart failure. Front. Physiol. 4:323. doi: 10.3389/fphys.2013.00323
Barros, A. A., Aroso, I. M., Silva, T. H., Mano, J. F., Duarte, A. R. C., and Reis, R. L. (2016). In vitro bioactivity studies of ceramic structures isolated from marine sponges. Biomed. Mater. 11:045004. doi: 10.1088/1748-6041/11/4/045004
Barsanti, L., and Gualtieri, P. (2014). Algae: Anatomy, Biochemistry, and Biotechnology. Boca Raton, FL: CRC Press.
Bartelme, R. P., McLellan, S. L., and Newton, R. J. (2017). Freshwater recirculating aquaculture system operations drive biofilter bacterial community shifts around a stable nitrifying consortium of ammonia-oxidizing Archaea and comammox Nitrospira. Front. Microbiol. 8:101. doi: 10.3389/fmicb.2017.00101
Baslow, M. H. (1969). Marine Pharmacology; A Study of Toxins and Other Biologically Active Substances of Marine Origin. Baltimore: Williams & Wilkins Co.
Batista, A. N. L., dos Santos, F. M., Batista, J. M., and Cass, Q. B. (2018). Enantiomeric mixtures in natural product chemistry: separation and absolute configuration assignment. Molecules 32:492. doi: 10.3390/molecules23020492
Battin, T. J., Kaplan, L., Newbold, J. D., and Hansen, C. M. E. (2003). Contributions of microbial biofilms to ecosystem processes in stream mesocosm. Nature 426, 239–4332.
Battin, T. J., Sloan, W. T., Kjelleberg, S., Daims, H., Head, I. M., Curtis, T. P., et al. (2007). Microbial landscape: new paths to biofilm research. Nature 5, 76–81. doi: 10.1038/nrmicro1556
Bauermeister, A., Pereira, F., Grilo, I. R., Godinho, C. C., Paulino, M., Almeida, V., et al. (2019). Intra-clade metabolomic profiling of MAR4 Streptomyces from the Macaronesia Atlantic region reveals a source of anti-biofilm metabolites. Environ. Microbiol. 21, 1099–1112. doi: 10.1111/1462-2920.14529
Bauermeister, A., Velasco-Alzate, K., Dias, T., Macedo, H., Ferreira, E. G., Jimenez, P. C., et al. (2018). Metabolomic fingerprinting of Salinispora from Atlantic Oceanic islands. Front. Microbiol. 9:3021. doi: 10.3389/fmicb.2018.03021
Bauvais, C., Zirah, S., Piette, L., Chaspoul, F., Domart-Coulon, I., Chapon, V., et al. (2015). Sponging up metals: bacteria associated with the marine sponge Spongia officinalis. Mar. Environ. Res. 104, 20–30. doi: 10.1016/j.marenvres.2014.12.005
Bekkby, T., Moy, F. E., Olsen, H., Rinde, E., Bodvin, T., Bøe, R., et al. (2013). “The Norwegian programme for mapping of marine habitats – providing knowledge and maps for ICZMP,” in Global Challenges in Integrated Coastal Zone Management, Vol. II, eds E. Moksness, E. Dahl, and J. Støttrup (Oxford: John Wiley and Sons), 21–30.
Bella, F., Mobarak, N. N., Jumaah, F. N., and Ahmad, A. (2015). From seaweeds to biopolymeric electrolytes for third generation solar cells: an intriguing approach. Electrochim. Acta 151, 306–311. doi: 10.1016/j.electacta.2014.11.058
Benito-González, I., López-Rubio, A., Martínez-Abad, A., Ballester, A. R., Falcó, I., González-Candelas, L., et al. (2019). In-depth characterization of bioactive extracts from Posidonia oceanica waste biomass. Mar. Drugs 17:409. doi: 10.3390/md17070409
Bentzon-Tilia, M., Sonnenschein, E. C., and Gram, L. (2016). Monitoring and managing microbes in aquaculture – towards a sustainable industry. Microb. Biotechnol. 9, 576–584. doi: 10.1111/1751-7915.12392
Bergmann, W., and Burke, D. C. (1956). Contributions to the study of marine products. XL. The nucleosides of sponges. IV. Spongosine. J. Org. Chem. 21, 226–228. doi: 10.1021/jo01108a020
Bergmann, W., and Feeney, R. J. (1950). The isolation of a new thymine pentoside from sponges. J. Am. Chem. Soc. 72, 2809–2810. doi: 10.1021/ja01162a543
Bergmann, W., and Feeney, R. J. (1951). Contributions to the study of marine products. XXXII. The nucleosides of sponges. I. J. Org. Chem. 16, 981–987. doi: 10.1021/jo01146a023
Berrue, F., Ibrahim, A., Boland, P., and Kerr, R. G. (2009). Newly isolated marine Bacillus pumilus (SP21): a source of novel lipoamides and other antimicrobial agents. Pure Appl. Chem. 81, 1027–1031. doi: 10.1351/pac-con-08-09-25
Bertrand, S., Bohni, N., Schnee, S., Schumpp, O., Gindro, K., and Wolfender, J. L. (2014). Metabolite induction via microorganism co-culture: a potential way to enhance chemical diversity for drug discovery. Biotechnol. Adv. 32, 1180–1204. doi: 10.1016/j.biotechadv.2014.03.001
Bhushan, R., and Brückner, H. (2011). Use of Marfey’s reagent and analogs for chiral amino acid analysis: assessment and applications to natural products and biological systems. J. Chromatogr. B 879, 3148–3161. doi: 10.1016/j.jchromb.2011.05.058
Bibi, F., Naseer, M. I., Hassan, A. M., Yasir, M., Khalaf Al-Ghamdi, A. A., and Azhar, E. I. (2018). Diversity and antagonistic potential of bacteria isolated from marine grass Halodule uninervis. 3 Biotech 8:48. doi: 10.1007/s13205-017-1066-1
Blackall, L. L., Wilson, B., and Van Oppen, M. J. H. (2015). Coral-the world’s most diverse symbiotic ecosystem. Mol. Ecol. 24, 5330–5347. doi: 10.1111/mec.13400
Blunt, J., Munro, M., and Upjohn, M. (2012). “The role of databases in marine natural products research,” in Handbook of Marine Natural Products, eds E. Fattorusso, W. H. Gerwick, and O. Taglialatela-Scafati (New York, NY: Springer), 389–421. doi: 10.1007/978-90-481-3834-0_6
Blunt, J. W., Carrol, A. R., Copp, B. R., Davis, R. A., Keyzers, R. A., and Prinsep, M. R. (2018). Marine natural products. Nat. Prod. Rep. 35, 8–53.
Bode, H. B., Bethe, B., Höfs, R., and Zeeck, A. (2002). Big effects from small changes: possible ways to explore nature’s chemical diversity. Chembiochem 3, 619–627. doi: 10.1002/1439-7633(20020703)3:7<619::aid-cbic619>3.0.co;2-9
Bohutskyi, P., Liu, K., Nasr, L. K., Byers, N., Rosenberg, J. N., Oyler, G. A., et al. (2015). Bioprospecting of microalgae for integrated biomass production and phytoremediation of unsterilized wastewater and anaerobic digestion centrate. Appl. Microbiol. Biotechnol. 99, 6139–6154. doi: 10.1007/s00253-015-6603-4
Bojko, B., Onat, B., Boyaci, E., Psillakis, E., Dailianis, T., and Pawliszyn, J. (2019). Application of in situ solid-phase microextraction on mediterranean sponges for untargeted metabolomics screening and environmental monitoring. Front. Mar. Sci. 6:632. doi: 10.3389/fmars.2019.00632
Bolaky, B. (2020). Operationalising blue economy in Africa: the case of South-West Indian Ocean. ORF Issue Brief No. 398. New Delhi: Observer Research Foundation.
Bollinger, A., Thies, S., Katzke, N., and Jaeger, K. E. (2018). The biotechnological potential of marine bacteria in the novel lineage of Pseudomonas pertucinogena. Microb. Biotechnol. 13, 19–31. doi: 10.1111/1751-7915.13288
Bonugli-Santos, R. C., dos Santos Vasconcelos, M. R., Passarini, M. R. Z., Vieira, G. A. L., Lopes, V. C. P., Mainardi, P. H., et al. (2015). Marine-derived fungi: diversity of enzymes and biotechnological applications. Front. Microbiol. 6:269. doi: 10.3389/fmicb.2015.00269
Borines, M. G., McHenry, M. P., and de Leon, R. L. (2011). Integrated macroalgae production for sustainable bioethanol, aquaculture and agriculture in Pacific island nations. Biofuel Bioprod. Bior. 5, 599–608. doi: 10.1002/bbb.310
Bork, P., Bowler, C., de Vargas, C., Gorsky, G., Karsenti, E., and Wincker, P. (2015). Tara Oceans studies plankton at planetary scale. Science 348:873. doi: 10.1126/science.aac5605
Bouaïcha, N., Miles, C. O., Beach, D. G., Labidi, Z., Djabri, A., Benayache, N. Y., et al. (2019). Structural diversity, characterization and toxicology of microcystins. Toxins 11:714. doi: 10.3390/toxins11120714
Boudouresque, C. F., Pergent, G., Pergent-Martini, C., Ruitton, S., Thibaut, T., and Verlaque, M. (2016). The necromass of the Posidonia oceanica seagrass meadow: fate, role, ecosystem services and vulnerability. Hydrobiologia 781, 25–42. doi: 10.1007/s10750-015-2333-y
Bovio, E., Fauchon, M., Toueix, Y., Mehiri, M., Varese, G. C., and Hellio, C. (2019a). The sponge-associated fungus Eurotium chevalieri MUT 2316 and its bioactive molecules: potential applications in the field of antifouling. Mar. Biotechnol. 21, 743–752. doi: 10.1007/s10126-019-09920-y
Bovio, E., Garzoli, L., Poli, A., Luganini, A., Villa, P., Musumeci, R., et al. (2019b). Marine fungi from the sponge Grantia compressa: biodiversity, chemodiversity, and biotechnological potential. Mar. Drugs 17:220. doi: 10.3390/md17040220
Bovio, E., Garzoli, L., Poli, A., Prigione, V., Firsova, D., McCormack, G. P., et al. (2018). The culturable mycobiota associated with three Atlantic sponges, including two new species: Thelebolus balaustiformis and T. spongiae. FUSE 1, 141–167. doi: 10.3114/fuse.2018.01.07
Bovio, E., Gnavi, G., Prigione, V., Spina, F., Denaro, R., Yakimov, M., et al. (2017). The culturable mycobiota of a Mediterranean marine site after an oil spill: isolation, identification and potential application in bioremediation. Sci. Total Environ. 576, 310–318. doi: 10.1016/j.scitotenv.2016.10.064
Bovio, E., Gnavi, G., Spina, F., Prigione, V., and Varese, G. C. (2016). Marine fungi from a crude-oil polluted site in Mediterranean Sea: diversity and selection of potential bioremediation agents. Biol. Mar. Mediterr. 23, 317–318.
Bowels, R. D., Hunt, A. E., Bremer, G. B., Duchars, M. G., and Eaton, R. A. (1999). Long-chain n-3 polyunsaturated fatty acid production by members of the marine protistan group the thraustochytrids: screening of isolates and optimisation of docosahexaenoic acid production. J. Biotechnol. 70, 193–202. doi: 10.1016/s0168-1656(99)00072-3
Bowman, J. P. (2007). Bioactive compound synthetic capacity and ecological significance of marine bacterial genus pseudoalteromonas. Mar. Drugs 5, 220–241. doi: 10.3390/md504220
Boyd, M. R., Gustafson, K. R., McMahon, J. B., Shoemaker, R. H., O’Keefe, B. R., Mori, T., et al. (1997). Discovery of cyanovirin-N, a novel human immunodeficiency virus-inactivating protein that binds viral surface envelope glycoprotein gp120: potential applications to microbicide development. Antimicrob. Agents Chemother. 41, 1521–1530. doi: 10.1128/aac.41.7.1521
Boziaris, I. S. (2014). Food ingredients from the marine environment. Marine biotechnology meets food science and technology. Front. Mar. Sci. 1:66. doi: 10.3389/fmars.2014.00066
Brailo, M., Schreier, H. J., McDonald, R., Maršić-Lučić, J., Gavrilović, A., Pećarević, M., et al. (2019). Bacterial community analysis of marine recirculating aquaculture system bioreactors for complete nitrogen removal established from a commercial inoculum. Aquaculture 503, 198–206. doi: 10.1016/j.aquaculture.2018.12.078
Brembu, T., Jørstad, M., Winge, P., Valle, K. C., and Bones, A. M. (2011). Genome-wide profiling of responses to cadmium in the diatom Phaeodactylum tricornutum. Environ. Sci. Technol. 45, 7640–7647. doi: 10.1021/es2002259
Brembu, T., Chauton, M., Winge, P., Bones, A. M., and Vadstein, O. (2017a). Hallmarks of the dynamic responses to silicon in Thalassiosira pseudonana - Identification, characterization and classification of signature genes and their corresponding motifs. Sci. Rep. 7:4865.
Brembu, T., Mühlroth, A., Alipanah, L., and Bones, A. M. (2017b). The effects of phosphorus limitation on carbon metabolism in diatoms. Philos. Trans. R. Soc. B 372:20160406. doi: 10.1098/rstb.2016.0406
Brennan, L., and Owende, P. (2010). Biofuels from microalgae - a review of technologies for production, processing, and extractions of biofuels and co-products. Renew. Sust. Energ. Rev. 14, 557–577. doi: 10.1016/j.rser.2009.10.009
Broggiato, A., Lallier, L. A., Sirakaya, A., and Vanagt, T. (2018). “Utilisation of marine genetic resources (GRS): the access and benefit-sharing legal framework,” in Grand Challenges in Marine Biotechnology, eds P. H. Rampelotto and A. Trincone (New York, NY: Springer), 579–599. doi: 10.1007/978-3-319-69075-9_15
Bruns, A., Nübel, U., Cypionka, H., and Overmann, J. (2003). Effect of signal compounds and incubation conditions on the culturability of freshwater bacterioplankton. Appl. Environ. Microbiol. 69, 1980–1989. doi: 10.1128/aem.69.4.1980-1989.2003
Brunt, E. G., and Burgess, J. G. (2018). The promise of marine molecules as cosmetic active ingredients. Int. J. Cosmet. Sci. 40, 1–15. doi: 10.1111/ics.12435
Brussaard, C. P. D., Wilhelm, S. W., Thingstad, F., Weinbauer, M. G., Bratbak, G., Heldal, M., et al. (2008). Global-scale processes with a nanoscale drive: the role of marine viruses. ISME J. 2, 575–578. doi: 10.1038/ismej.2008.31
Brzuzan, P., Mazur-Marzec, H., Florczyk, M., Stefaniak, F., Fidor, A., Konkel, R., et al. (2020). Luciferase reporter assay for small-molecule inhibitors of MIR92b-3p function: screening cyanopeptolins produced by Nostoc from the Baltic Sea. Toxicol. in vitro 68:104951. doi: 10.1016/j.tiv.2020.104951
Buijs, Y., Bech, P. K., Vazquez-Albacete, D., Bentzon-Tilia, M., Sonnenschein, E. C., Gram, L., et al. (2019). Marine Proteobacteria as a source of natural products: advances in molecular tools and strategies. Nat. Prod. Rep. 36, 1333–1350. doi: 10.1039/c9np00020h
Bulgariu, L., and Bulgariu, D. (2020). “Bioremediation of toxic heavy metals using marine algae biomass,” in Green Materials for Wastewater Treatment. Environmental Chemistry for a Sustainable World, eds M. Naushad and E. Lichtfouse (New York, NY: Springer), 69–98. doi: 10.1007/978-3-030-17724-9_4
Bulgariu, L., and Gavrilescu, M. (2015). “Bioremediation of heavy metals by microalgae,” in Handbook of Marine Microalgae, ed. S. K. Kim (Cambridge, MA: Academic Press), 457–469. doi: 10.1016/b978-0-12-800776-1.00030-3
Burns, D. C., Mazzola, E. P., and Reynolds, W. F. (2019). The role of computer-assisted structure elucidation (CASE) programs in the structure elucidation of complex natural products. Nat. Prod. Rep. 36:919. doi: 10.1039/C9NP00007K
Canganella, F., Bianconi, G., Kato, C., and Gonzalez, J. (2006). “Microbial ecology of submerged marine caves and holes characterized by high levels of hydrogen sulphide,” in Life in Extreme Environments, eds R. Amils, C. Ellis-Evans, and H. Hinghofer-Szalkay (Dordrecht: Springer), 61–70. doi: 10.1007/s11157-006-9103-2
Cardoso, M. J., Costa, R. R., and Mano, J. F. (2016). Marine origin polysaccharides in drug delivery systems. Mar. Drugs 14, 34. doi: 10.3390/md14020034
Carroll, A. R., Copp, B. R., Davis, R. A., Keyzers, R. A., and Prinsep, M. R. (2019). Marine natural products. Nat. Prod. Rep. 36, 122–173.
Carroll, A. R., Copp, B. R., Davis, R. A., Keyzers, R. A., and Prinsep, M. R. (2020). Marine natural products. Nat. Prod. Rep. 37, 175–223.
Carson, M. A., Nelson, J., Cancela, M. L., Laizé, V., Gavaia, P. J., Rae, M., et al. (2018a). Red algal extracts from Plocamium lyngbyanum and Ceramium secundatum stimulate osteogenic activities in vitro and bone growth in zebrafish larvae. Sci. Rep. 8:7725. doi: 10.1038/s41598-018-26024-0
Carson, M. A., Nelson, J., Cancela, M. L., Laizé, V., Gavaia, P. J., Rae, M., et al. (2018b). Screening for osteogenic activity in extracts from Irish marine organisms: the potential of Ceramium pallidum. PLoS One 13:e0207303. doi: 10.1371/journal.pone.0207303
Cartuche, L., Reyes-Batlle, M., Sifaoui, I., Arberas-Jiménez, I., Piñero, J. E., Fernández, J. J., et al. (2019). Antiamoebic activities of indolocarbazole metabolites isolated from Streptomyces sanyensis cultures. Mar. Drugs 17:588. doi: 10.3390/md17100588
Cartuche, L., Sifaoui, I., López-Arencibia, A., Bethencourt-Estrella, C. J., San Nicolás-Hernández, D., Lorenzo-Morales, J., et al. (2020). Antikinetoplastid activity of indolocarbazoles from Streptomyces sanyensis. Biomolecules 10:657. doi: 10.3390/biom10040657
Cecchi, G., Cutroneo, L., Di Piazza, S., Vagge, G., Capello, M., and Zotti, M. (2019). From waste to resource: mycoremediation of contaminated marine sediments in the SEDITERRA project. J. Soil. Sediment. 20, 2653–2663. doi: 10.1007/s11368-019-02527-9
Chen, C. L., Liou, S. F., Chen, S. J., and Shih, M. F. (2011). Protective effects of Chlorella-derived peptide on UVB-induced production of MMP-1 and degradation of procollagen genes in human skin fibroblasts. Regul. Toxicol. Pharmacol. 60, 112–119. doi: 10.1016/j.yrtph.2011.03.001
Chen, G., Wang, G. Y., Li, X., Waters, B., and Davies, J. (2000). Enhanced production of microbial metabolites in the presence of dimethyl sulfoxide. J. Antibiot. 53, 1145–1153. doi: 10.7164/antibiotics.53.1145
Chen, J. R., Lai, Y. H., Tsai, J. J., and Hsiao, C. D. (2017). Live fluorescent staining platform for drug-screening and mechanism-analysis in zebrafish for bone mineralization. Molecules 22:2068. doi: 10.3390/molecules22122068
Chen, L., and Qian, P. Y. (2017). Review on molecular mechanisms of antifouling compounds: an update since 2012. Mar. Drugs 15, 264–284. doi: 10.3390/md15090264
Chen, L., Wei, Y., Shi, M., Li, Z., and Zhang, S. H. (2019). An archaeal chitinase with a secondary capacity for catalyzing cellulose and its biotechnological applications in shell and straw degradation. Front. Microbiol. 10:1253. doi: 10.3389/fmicb.2019.01253
Chen, Q. Y., Liu, Y., Cai, W., and Luesch, H. (2014). Improved total synthesis and biological evaluation of potent apratoxin S4 based anticancer agents with differential stability and further enhanced activity. J. Med. Chem. 57, 3011–3029. doi: 10.1021/jm4019965
Chen, Y. H., Lu, M. C., Chang, Y. C., Hwang, T. L., Wang, W. H., Weng, C. F., et al. (2012). Pseudoalteromone A: a novel bioactive ubiquinone from a marine bacterium Pseudoalteromonas sp. CGH2XX (Pseudoalteromonadaceae). Tetrahedron Lett. 53, 1675–1677. doi: 10.1016/j.tetlet.2012.01.104
Cheng, Y. B., Jensen, P. R., and Fenical, W. (2013). Cytotoxic and antimicrobial napyradiomycins from two marine-derived, MAR 4 Streptomyces strains. Eur. J. Org. Chem. 18:201300349. doi: 10.1002/ejoc.201300349
Chiaiese, P., Corrado, G., Colla, G., Kyriacou, M., and Rouphael, Y. (2018). Renewable sources of plant biostimulation: microalgae as a sustainable means to improve crop performance. Front. Plant Sci. 9:1782. doi: 10.3389/fpls.2018.01782
Chianese, G., Esposito, F. P., Parrot, D., Ingham, C., de Pascale, D., and Tasdemir, D. (2018). Linear aminolipids with moderate antimicrobial activity from the Antarctic Gram-negative bacterium Aequorivita sp. Mar. Drugs 16, 187. doi: 10.3390/md16060187
Cho, K. S., Shin, M., Kim, S., and Lee, S. B. (2018). Recent advances in studies on the therapeutic potential of dietary carotenoids in neurodegenerative diseases. Oxid. Med. Cell. Longev. 2018:4120458. doi: 10.1155/2018/4120458
Chokshi, K., Pancha, I., Ghosh, A., and Mishra, S. (2017). Salinity induced oxidative stress alters the physiological responses and improves the biofuel potential of green microalgae Acutodesmus dimorphus. Bioresour. Technol. 244, 1376–1383. doi: 10.1016/j.biortech.2017.05.003
Chubokov, V., Mukhopadhyay, A., Petzold, C. J., Deasling, J. D., and García Martín, H. (2016). Synthetic and systems biology for microbial production of commodity chemicals. NPJ Syst. Biol. Appl. 2:16009. doi: 10.1038/npjsba.2016.9
Cicatiello, P., Gravagnuolo, A. M., Gnavi, G., Varese, G. C., and Giardina, P. (2016). Marine fungi as source of new hydrophobins. Int. J. Biol. Macromol. 92, 1229–1233. doi: 10.1016/j.ijbiomac.2016.08.037
Ciglenečki-Jušić, I., and Svetličić, V. (2015). “Nanoparticles and marine environment: an overview,” in Nanotechnology to Aid Chemical and Biological Defense, ed. T. A. Camesano (Dordrecht: Springer), 95–111. doi: 10.1007/978-94-017-7218-1_7
Cimmino, A., Masi, M., Evidente, M., Superchi, S., and Evidente, A. (2017). Application of Mosher’s method for absolute configuration assignment to bioactive plants and fungi metabolites. J. Pharm. Biomed. Anal. 144, 59–89. doi: 10.1016/j.jpba.2017.02.037
Claustre, H., Sciandra, A., and Vaulot, D. (2008). Introduction to the special section bio-optical and biogeochemical conditions in the South East Pacific in late 2004: the BIOSOPE program. Biogeosciences Discuss. 5, 605–640.
Cobbett, C., and Goldsbrough, P. (2002). Phytochelatins and metallothioneins: roles in heavy metal detoxification and homeostasis. Annu. Rev Plant Biol. 53, 159–182. doi: 10.1146/annurev.arplant.53.100301.135154
Cockroft, N. T., Cheng, X., and Fuchs, J. R. (2019). STarFish: a stacked ensemble target fishing approach and its application to natural products. J. Chem. Inf. Model. 59, 4906–4920. doi: 10.1021/acs.jcim.9b00489
Coffey, B., Mills, S., Coffey, A., McAuliffe, O., and Ross, R. P. (2010). Phage and their lysins as biocontrol agents for food safety applications. Annu. Rev. Food. Sci. Technol. 1, 449–468. doi: 10.1146/annurev.food.102308.124046
Colla, G., Hoagland, L., Ruzzi, M., Cardarelli, M., Bonini, P., Canaguier, R., et al. (2017). Biostimulant action of protein hydrolysates: unraveling their effects on plant physiology and microbiome. Front. Plant Sci. 8:2202. doi: 10.3389/fpls.2017.02202
Collie, J. S., Beck, M. W., Craig, B., Essington, T. E., Fluharty, D., Rice, J., et al. (2013). Marine spatial planning in practice. Estuar. Coast. Shelf Sci. 117, 1–11.
Collins, J. (2019). Report of the Workshop “Marine Genetic Resources in Areas Beyond National Jurisdiction: Bridging Policy, Law, Science and Research and Development” 21-22 May 2019. Brussels: EU.
Collins, J. E., Harden-Davies, H., Jaspars, M., Thiele, T., Vanagt, T., and Huys, I. (2019). Inclusive innovation: enhancing global participation in and benefit sharing linked to the utilization of marine genetic resources from areas beyond national jurisdiction. Mar. Policy 109:103696. doi: 10.1016/j.marpol.2019.103696
Colonia, B. S. O., de Melo Pereira, G. V., and Soccol, C. R. (2020). Omega-3 microbial oils from marine thraustochytrids as a sustainable and technological solution: a review and patent landscape. Trends Food Sci. Tech. 99, 244–256. doi: 10.1016/j.tifs.2020.03.007
Comeau, A. M., Vincent, W. F., Bernier, L., and Lovejoy, C. (2016). Novel chytrid lineages dominate fungal sequences in diverse marine and freshwater habitats. Sci. Rep. 6:30120. doi: 10.1038/srep30120
Conkling, M., Hesp, K., Munroe, S., Sandoval, K., Martens, D. E., Sipkema, D., et al. (2019). Breakthrough in marine invertebrate cell culture: sponge cells divide rapidly in improved nutrient medium. Sci. Rep. 9:17321. doi: 10.1038/s41598-019-53643-y
Corey, E. J., Gin, D. Y., and Kania, R. S. (1996). Enantioselective total synthesis of ecteinascidin 743. J. Am. Chem. Soc. 118, 9202–9203. doi: 10.1021/ja962480t
Corinaldesi, C., Barone, G., Marcellini, F., Dell’Anno, A., and Danovaro, R. (2017). Marine microbial-derived molecules and their potential use in cosmeceutical and cosmetic products. Mar. Drugs 15:118. doi: 10.3390/md15040118
Cornet, C., Di Donato, V., and Terriente, J. (2018). Combining zebrafish and CRISPR/Cas9: toward a more efficient drug discovery pipeline. Front. Pharmacol. 9:703. doi: 10.3389/fphar.2018.00703
Costa, O. Y. A., Raaijmakers, J. M., and Kuramae, E. E. (2018). Microbial extracellular polymeric substances: ecological function and impact on soil aggregation. Front. Microbiol. 9:1636. doi: 10.3389/fmicb.2018.01636
Costa, S. S., Miranda, A. L., de Morais, M. G., Costa, J. A. V., and Druzian, J. I. (2019). Microalgae as source of polyhydroxyalkanoates (PHAs) — a review. Int. J. Biol. Macromol. 131, 536–547. doi: 10.1016/j.ijbiomac.2019.03.099
Costello, M. J., and Chaudhary, C. (2017). Marine biodiversity, biogeography, deep-sea gradients, and conservation. Curr. Biol. 27, R511–R527.
Crist, R. H., Oberholser, K., Shank, N., and Nguyen, M. (1981). Nature of bonding between metallic ions and algal cell walls. Environ. Sci. Technol. 15, 1212–1217. doi: 10.1021/es00092a010
Cruz, S., Gomes, S. E., Borralho, P. M., Rodrigues, C. M. P., Gaudêncio, S. P., and Pereira, F. (2019). In silico HCT116 human colon cancer cell-based models en route to the discovery of lead-like anticancer drugs. Biomolecules 8:56. doi: 10.3390/biom8030056
Cueto, M., Jensen, P. R., Kauffman, C., Fenical, W., Lobkovsky, E., and Clardy, J. (2001). Pestalone, a new antibiotic produced by a marine fungus in response to bacterial challenge. J. Nat. Prod. 64, 1444–1446. doi: 10.1021/np0102713
Cuevas, C., and Francesch, A. (2009). Development of Yondelis§(trabectedin, ET-743). A semisynthetic process solves the supply problems. Nat. Prod. Rep. 26, 322–337. doi: 10.1039/b808331m
Cuevas, C., Pérez, M., Martín, M. J., Chicharro, J. L., Fernández-Rivas, C., Flores, M., et al. (2000). Synthesis of Ecteinascidin ET-743 and phthalascidin Pt-650 from cyanosafracin B. Org. Lett. 2, 2545–2548. doi: 10.1021/ol0062502
Culot, A., Grosset, N., and Gautier, M. (2019). Overcoming the challenges of phage therapy for industrial aquaculture: a review. Aquaculture 513:734423. doi: 10.1016/j.aquaculture.2019.734423
Custódio, L., Laukaityte, S., Engelen, A. H., Rodrigues, M. J., Pereira, H., Vizetto-Duarte, C., et al. (2016). A comparative evaluation of biological activities and bioactive compounds of the seagrasses Zostera marina and Zostera noltei from southern Portugal. Nat. Prod. Res. 30, 724–728. doi: 10.1080/14786419.2015.1040791
Dangal, S. R. S., Tian, H., Zhang, B., Pan, S., Lu, C., and Yang, J. (2017). Methane emission from global livestock sector during 1890–2014: magnitude, trends and spatiotemporal patterns. Glob. Chang. Biol. 23, 4147–4161. doi: 10.1111/gcb.13709
Daranas, A. H., Norte, M., and Fernández, J. J. (2001). Toxic marine microalgae. Toxicon 39, 1101–1132. doi: 10.1016/s0041-0101(00)00255-5
Day, J., Hughes, A., Greenhill, L., and Stanley, M. S. (2016). Blue Biotechnology. Commonwealth Blue Economy Report Series, No. 5. London: Commonwealth Secretariat.
de Carvalho, C. C. C. R. (2018). Marine biofilms: a successful microbial strategy with economic implications. Front. Mar. Sci. 5:126. doi: 10.3389/fmars.2018.00126
de Felício, R., Pavão, G. B., de Oliveira, A. L. L., Erbert, C., Conti, R., Pupo, M. T., et al. (2015). Antibacterial, antifungal and cytotoxic activities exhibited by endophytic fungi from the Brazilian marine red alga Bostrychia tenella (Ceramiales). Rev. bras. Farmacogn. 25, 641–650. doi: 10.1016/j.bjp.2015.08.003
de Goeij, J. M., Moodley, L., Houtekamer, M., Carballeira, N. M., and van Duyl, F. C. (2008a). Tracing 13C-enriched dissolved particulate organic carbon in the bacteria-containing coral reef sponge Halisarca caerulea: evidence for DOM feeding. Limnol. Oceanogr. 53, 1376–1386. doi: 10.4319/lo.2008.53.4.1376
de Goeij, J. M., van den Berg, H., van Oostveen, M. M., Epping, E. H. G., and van Duy, F. C. (2008b). Major bulk dissolved organic carbon (DOC) removal by encrusting coral reef cavity sponges. Mar. Ecol. Prog. Ser. 357, 139–151. doi: 10.3354/meps07403
de la Calle, F. (2017). Marine microbiome as source of natural products. Microb. Biotechnol. 10, 1293–1296. doi: 10.1111/1751-7915.12882
de la Coba, F., Aguilera, J., de Gálvez, M. V., Álvarez, M., Gallego, E., Figueroa, F. L., et al. (2009). Prevention of the ultraviolet effects on clinical and histopathological changes, as well as the heat shock protein-70 expression in mouse skin by topical application of algal UV-absorbing compounds. J. Dermatol. Sci. 55, 161–169. doi: 10.1016/j.jdermsci.2009.06.004
de los Santos, E. L. C. (2019). NeuRiPP: neural network identification of RiPP precursor peptides. Sci. Rep. 9:13406. doi: 10.1038/s41598-019-49764-z
de Morais, M. G., Vaz Bda, D. S., de Morais, E. G., and Costa, J. A. (2015). Biologically active metabolites synthesized by microalgae. BioMed Res. Int. 2015:835761. doi: 10.1155/2015/835761
De Philippis, R., and Vincenzini, M. (2003). Outermost polysaccharidic investments of cyanobacteria: nature, significance and possible applications. Recent Res. Dev. Microbiol. 7, 13–22.
de Vargas, C., Audic, S., Henry, N., Decelle, J., Mahé, F., Logares, R., et al. (2015). Eukaryotic plankton diversity in the sunlit ocean. Science 348:6237. doi: 10.1126/science.1261605
de Vera, C. R., Díaz Crespín, G., Hernández Daranas, A., Montalvão Looga, S., Lillsunde, K. E., Tammela, P., et al. (2018). Marine microalgae: promising source for new bioactive compounds. Mar. Drugs 16, 317. doi: 10.3390/md16090317
de Wilt, A., Butkovskyi, A., Tuantet, K., Leal, L. H., Fernandes, T. V., Langenhoff, A., et al. (2016). Micropollutant removal in an algal treatment system fed with source separated wastewater streams. J. Hazard. Mater. 304, 84–92. doi: 10.1016/j.jhazmat.2015.10.033
Defeo, O., McLachlan, A., Schoeman, D. S., Schlacher, T. A., Dugan, J., Jones, A., et al. (2009). Threats to sandy beach ecosystems: a review. Estuar. Coast. Shelf S. 81, 1–12.
Demay, J., Bernard, C., Reinhardt, A., and Marie, B. (2019). Natural products from Cyanobacteria: focus on beneficial activities. Mar. Drugs 17:320. doi: 10.3390/md17060320
Devadatha, B., Sarma, V. V., Jeewon, R., Wanasinghe, D. N., Hyde, K. D., and Jones, E. B. G. (2018). Thyridariella, a novel marine fungal genus from India: morphological characterization and phylogeny inferred from multigene DNA sequence analyses. Mycol. Prog. 17, 791–804. doi: 10.1007/s11557-018-1387-4
Dewi, I. C., Falaise, C., Hellio, C., Bourgougnon, N., and Mouget, J. L. (2018). “Anticancer, antiviral, antibacterial and antifungal properties in microalgae,” in Microalgae in Health and Disease Prevention, eds I. A. Levine and J. Fleurence (Amsterdam: Elsevier), 235–261. doi: 10.1016/b978-0-12-811405-6.00012-8
Dey, B., Lerner, D. L., Lusso, P., Boyd, M. R., Elder, J. H., and Berger, E. A. (2000). Human immunodeficiency virus type 1 gp120 interaction with CD4 and Coreceptor and inhibition of diverse enveloped viruses. J.Virol. 74, 4562–4569. doi: 10.1128/.74.10.4562-4569.2000
Di Donato, P., Buono, A., Poli, A., Finore, I., Abbamondi, G. R., Nicolaus, B., et al. (2019). Exploring marine environments for the identification of extremophiles and their enzymes for sustainable and green bioprocesses. Sustainability 11:149. doi: 10.3390/su11010149
Di Pippo, F., Ellwood, N. T. W., Gismondi, A., Bruno, L., Rossi, F., Magni, P., et al. (2013). Characterization of exopolysaccharides produced by seven biofilm-forming cyanobacterial strains for biotechnological applications. J. Appl. Phycol. 25, 1697–1708. doi: 10.1007/s10811-013-0028-1
Dias, T., Gaudêncio, S. P., and Pereira, F. (2019). A computer-driven approach to discover natural product leads for methicillin-resistant Staphylococcus aureus infection therapy. Mar. Drugs 17:16. doi: 10.3390/md17010016
Diaz-Ferguson, E. E., and Moyer, G. R. (2014). History, applications, methodological issues and perspectives for the use of environmental DNA (eDNA) in marine and freshwater environments. Rev. Biol. Trop. 62, 1273–1284. doi: 10.15517/rbt.v62i4.13231
Dineshbabu, G., Goswami, G., Kumar, R., Sinha, A., and Das, D. (2019). Microalgae-nutritious, sustainable aqua- and animal feed source. J. Funct. Foods 62:103545. doi: 10.1016/j.jff.2019.103545
Dinsdale, E. A., Edwards, R. A., Hall, D., Angly, F., BreitBart, M., Brulc, J. M., et al. (2008). Functional metagenomic profiling of nine biomes. Nature 452, 629–632. doi: 10.1038/nature06810
Dong, X., and Strous, M. (2019). An integrated pipeline for annotation and visualization of metagenomic contigs. Front. Genet. 10:999. doi: 10.3389/fgene.2019.00999
Doussineau, M., Haarich, S., Gnamus, A., Gomez, J., and Holstein, F. (2020). Smart Specialisation and Blue biotechnology in Europe, EUR 30521 EN. Luxembourg: Publications Office of the European Union.
D’Souza, D. T., Tiwari, R., Sah, A. K., and Raghukumar, C. (2006). Enhanced production of laccase by a marine fungus during treatment of colored effluents and synthetic dyes. Enzyme Microb. Technol. 38, 504–511. doi: 10.1016/j.enzmictec.2005.07.005
Du, Z., Alvaro, J., Hyden, B., Zienkiewicz, K., Benning, N., Zienkiewicz, A., et al. (2018). Enhancing oil production and harvest by combining the marine alga Nannochloropsis oceanica and the oleaginous fungus Mortierella elongata. Biotechnol. Biofuels 11:174. doi: 10.1186/s13068-018-1172-2
du Jardin, P. (2015). Plant biostimulants: definition, concept, main categories and regulation. Sci. Hortic. 196, 3–14. doi: 10.1016/j.scienta.2015.09.021
Duarte, C. M. (2015). Seafaring in the 21st century: the Malaspina 2010 circumnavigation expedition. Limnol. Oceanogr. Bull. 24, 11–14. doi: 10.1002/lob.10008
Duckworth, A. R. (2009). Farming sponges to supply bioactive metabolites and bath sponges: a review. Mar. Biotechnol. 11, 669–679. doi: 10.1007/s10126-009-9213-2
Duckworth, A. R., and Battershill, C. (2003). Sponge aquaculture for the production of biologically active metabolites: the influence of farming protocols and environment. Aquaculture 221, 311–329. doi: 10.1016/s0044-8486(03)00070-x
Dull, J. L., Singh, A. K., Hoare, M., and Ayer, S. W. (1994). Conditions for the production of jadomycin B by Streptomyces venezuelae ISP5230: effects of heat shock, ethanol treatment and phage infection. J. Ind. Microbiol. 13, 120–125. doi: 10.1007/bf01584109
Duzenli, O. F., and Okay, S. (2020). Promoter engineering for the recombinant protein production in prokaryotic systems. AIMS Bioeng. 7, 62–81. doi: 10.3934/bioeng.2020007
Dwivedi, S. (2012). Bioremediation of heavy metal by algae: current and future perspective. J. Adv. Lab. Res. Biol. 3, 195–199.
Ebada, S. S., Edrada, R. A., Lin, W., and Proksch, P. (2008). Methods for isolation, purification and structural elucidation of bioactive secondary metabolites from marine invertebrates. Nat. Protoc. 3, 1820–1831. doi: 10.1038/nprot.2008.182
Egan, S., Holmström, C., and Kjelleberg, S. (2001). Pseudoalteromonas ulvae sp. nov., a bacterium with antifouling activities isolated from the surface of a marine alga. Int. J. Syst. Evol. Microbiol. 51, 1499–1504. doi: 10.1099/00207713-51-4-1499
Egan, S., James, S., Holmström, C., and Kjelleberg, S. (2002). Correlation between pigmentation and antifouling compounds produced by Pseudoalteromonas tunicata. Environ. Microbiol. 4, 433–442. doi: 10.1046/j.1462-2920.2002.00322.x
Egorova, K., and Antranikian, G. (2005). Industrial relevance of thermophilic Archaea. Curr. Opin. Microbiol. 8, 649–655. doi: 10.1016/j.mib.2005.10.015
Eguía, E., and Trueba, A. (2007). Application of marine biotechnology in the production of natural biocides for testing on environmentally innocuous antifouling coatings. J. Coat. Technol. Res. 4, 191–202. doi: 10.1007/s11998-007-9022-3
El Boukhari, M. E. M., Barakate, M., Bouhia, Y., and Lyamlouli, K. (2020). Trends in seaweed extract based biostimulants: manufacturing process and beneficial effect on soil-plant systems. Plants 9:359. doi: 10.3390/plants9030359
Emadodin, I., Reinsch, T., Rotter, A., Orlando-Bonaca, M., Taube, F., and Javidpour, J. (2020). A perspective on the potential of using marine organic fertilizers for the sustainable management of coastal ecosystem services. Environ. Sustainab. 3, 105–115. doi: 10.1007/s42398-020-00097-y
Enke, H., Lorenzen, W., Jahns, S., Enke, D., Niedermeyer, T., and Moschny, J. (2020). Modified Microcystins and Nodularins. U.S. patent application publication No US2020/0087348 A1. Washington, DC: U.S. Patent and Trademark Office.
Erdoǧan, A., Demirel, Z., Eroǧlu, A. E., and Dalay, M. C. (2016). Carotenoid profile in Prochlorococcus sp. and enrichment of lutein using different nitrogen sources. J. Appl. Phycol. 28, 3251–3257. doi: 10.1007/s10811-016-0861-0
Essien, S. O., Young, B., and Baroutian, S. (2020). Recent advances in subcritical water and supercritical carbon dioxideextraction of bioactive compounds from plant materials. Trends Food Sci. Technol. 97, 156–169. doi: 10.1016/j.tifs.2020.01.014
Estes, S. K., Austin, M. C., Mandelare, P. E., Paig-Tran, E. W. M., Loesgen, S., and Strother, J. A. (2019). The microbiota of marine fishes produce neuroactive secondary metabolites. Integr. Comp. Biol. 59, E309–E309.
Esteves, A. I., Hardoim, C. C., Xavier, J. R., Gonçalves, J. M., and Costa, R. (2013). Molecular richness and biotechnological potential of bacteria cultured from Irciniidae sponges in the north-east Atlantic. FEMS Microbiol. Ecol. 85, 519–536. doi: 10.1111/1574-6941.12140
Eswara Rao, T., Imchen, M., and Kumavath, R. (2017). Marine enzymes: production and applications for human health. Adv. Food Nutr. Res. 80, 149–163.
European Commission (2019). Roadmap for the Blue Bioeconomy. Luxembourg: Publications office of the European Union.
European Commission (2020). The EU Blue Economy Report. 2020. Luxembourg: Publications office of the European Union.
Fan, B., Dewapriya, P., Li, F., Grauso, L., Blümel, M., Mangoni, A., et al. (2020). Pyrenosetin D, a new pentacyclic decalinoyltetramic acid derivative from the seaweed endophytic fungus Pyrenochaetopsis sp. FVE-087. Mar. Drugs 18:281. doi: 10.3390/md18060281
Fan, B., Parrot, D., Blümel, M., Labes, A., and Tasdemir, D. (2019). Influence of OSMAC-based cultivation in metabolome and anticancer activity of fungi associated with the brown alga Fucus vesiculosus. Mar. Drugs 17:67. doi: 10.3390/md17010067
FAO (2020). The State of World Fisheries and Aquaculture 2020 - Sustainability in Action. Rome: Food and Agriculture Organization of the United Nations.
Faulkner, D. J. (2000). Highlights of marine natural products chemistry (1972–1999). Nat. Prod. Rep. 17, 1–6. doi: 10.1039/A909113K
Fazi, S., Baldassarre, L., Cassin, D., Quero, G. M., Pizzetti, I., Cibic, T., et al. (2020). Prokaryotic community composition and distribution in coastal sediments following a Po river flood event (northern Adriatic Sea, Italy). Estuar. Coast. Shelf Sci. 233:106547. doi: 10.1016/j.ecss.2019.106547
Fazi, S., Bandla, A., Pizzetti, I., and Swarup, S. (2016). Microbial biofilms as one of the key elements in modulating ecohydrological processes in both natural and urban water corridors. Ecohydrol. Hydrobiol. 16, 33–38. doi: 10.1016/j.ecohyd.2015.08.002
Fehér, D., Barlow, R., McAtee, J., and Hemscheidt, T. K. (2010). Highly brominated antimicrobial metabolites from a marine Pseudoalteromonas sp. J. Nat. Prod. 73, 1963–1966. doi: 10.1021/np100506z
Feling, R. H., Buchanan, G. O., Mincer, T. J., Kauffman, C. A., Jensen, P. R., and Fenical, W. (2003). Salinosporamide A: a highly cytotoxic proteasome inhibitor from a novel microbial source, a marine bacterium of the new genus “Salinospora“. Angew. Chem. Int. Ed. 42, 355–357. doi: 10.1002/anie.200390115
Fenical, W., Jensen, P. R., Palladino, M. A., Lam, K. S., Lloyd, G. K., and Potts, B. C. (2009). Discovery and development of the anticancer agent salinosporamide A (NPI-0052). Bioorg. Med. Chem. 17, 2175–2180. doi: 10.1016/j.bmc.2008.10.075
Ferdouse, F., Løvstad Holdt, S., Smith, R., Murúa, P., and Yang, Z. (2018). The Global Status of Seaweed Production, Trade and Utilization. FAO GLOBEFISH RESEARCH PROGRAMME VOL. 124. Rome: Food and Agriculture Organization of the United Nations.
Fernand, F., Israel, A., Skjermo, J., Wichard, T., Timmermans, K. R., and Goldberg, A. (2017). Offshore macroalgae biomass for bioenergy production: environmental aspects, technological achievements and challenges. Renew. Sust. Energ. Rev. 75, 35–45. doi: 10.1016/j.rser.2016.10.046
Fernandes, P. (2014). Marine enzymes and food industry: insight on existing and potential interactions. Front. Mar. Sci. 1:46. doi: 10.3389/fmars.2014.00046
Fernández-Ruiz, I., Coutinho, F. H., and Rodriguez-Valera, F. (2018). Thousands of novel endolysins discovered in uncultured phage genomes. Front. Microbiol. 9:1033. doi: 10.3389/fmicb.2018.01033
Ferraro, V., Cruz, I. B., Ferreira Jorge, R., Malcata, F. X., Pintado, M. E., and Castro, P. M. (2010). Valorisation of natural extracts from marine source focused on marine by-products: a review. Food Res. Int. 43, 2221–2233. doi: 10.1016/j.foodres.2010.07.034
Ferro, L., Gojkovic, Z., Muñoz, R., and Funk, C. (2019). Growth performance and nutrient removal of a Chlorella vulgaris-Rhizobium sp. co-culture during mixotrophic feed-batch cultivation in synthetic wastewater. Algal Res. 44:101690. doi: 10.1016/j.algal.2019.101690
Fewer, D. P., Jokela, J., Paukku, E., Österholm, J., Wahlsten, M., Permi, P., et al. (2013). New structural variants of aeruginosin produced by the toxic bloom forming cyanobacterium Nodularia spumigena. PLoS One 8:e73618. doi: 10.1371/journal.pone.0073618
Field, C. B., Behrenfeld, M. J., Randerson, J. T., and Falkowski, P. (1998). Primary production of the biosphere: integrating terrestrial and oceanic components. Science 281, 237–240. doi: 10.1126/science.281.5374.237
Fitridge, I., Dempster, T., Guenther, J., and de Nys, R. (2012). The impact and control of biofouling in marine aquaculture: a review. Biofouling 28, 649–669. doi: 10.1080/08927014.2012.700478
Flewelling, A. J., Currie, J., Gray, C. A., and Johnson, J. A. (2015). Endophytes from marine macro-algae: promising sources of novel natural products. Curr. Sci. India 109, 88–111.
Folke, C., Hahn, T., Olsson, P., and Norberg, J. (2005). Adaptive governance of social-ecological systems. Annu. Rev. Environ. Resour. 30, 441–473.
Formighieri, C., Franck, F., and Bassi, R. (2012). Regulation of the pigment optical density of an algal cell: filling the gap between photosynthetic productivity in the laboratory and in mass culture. J. Biotechnol. 162, 115–123. doi: 10.1016/j.jbiotec.2012.02.021
Franzén, D., Infantes, E., and Gröndahl, F. (2019). Beach-cast as biofertiliser in the Baltic Sea region-potential limitations due to cadmium-content. Ocean Coast. Manag. 169, 20–26. doi: 10.1016/j.ocecoaman.2018.11.015
Fu, W., Wu, Y., Sun, L., and Zhang, W. (2007). Efficient bioremediation of total organic carbon (TOC) in integrated aquaculture system by marine sponge Hymeniacidon perleve. Biotechnol. Bioeng. 97, 1387–1397. doi: 10.1002/bit.21352
Gabrielsen, K. L. (2012). Marbank – A biobank of arctic marine organisms. Planta Med. 78:L18. doi: 10.1055/s-0032-1321352
Galasso, C., Corinaldesi, C., and Sansone, C. (2017). Carotenoids from marine organisms: biological functions and industrial applications. Antioxidants 6:96. doi: 10.3390/antiox6040096
Gallardo-Rodríguez, J., Sánchez-Mirón, A., García-Camacho, F., López-Rosales, L., Chisti, Y., and Molina-Grima, E. (2012). Bioactives from microalgal dinoflagellates. Biotechnol. Adv. 30, 1673–1684. doi: 10.1016/j.biotechadv.2012.07.005
Gammone, M. A., and D’Orazio, N. (2015). Anti-obesity activity of the marine carotenoid fucoxanthin. Mar. Drugs 13, 2196–2214. doi: 10.3390/md13042196
Ganapiriya, V., Maharajan, A., and Kumarasamy, P. (2012). Antifouling effect of bioactive compounds from marine sponge Acanthella elongata and different species of bacterial film on larval attachment of Balanus amphitrite (Cirripedia, Crustacea). Braz. Arch. Biol. Technol. 55, 395–402. doi: 10.1590/s1516-89132012000300010
García-Gonzalez, J., and Sommerfeld, M. (2016). Biofertilizer and biostimulant properties of microalga Acutodesmus dimorphus. J. Appl. Phycol. 28, 1051–1061. doi: 10.1007/s10811-015-0625-2
Gargan, L. M., Morato, T., Pham, C. K., Finarelli, J. A., Carlsson, J. E. L., and Carlsson, J. (2017). Development of a sensitive detection method to survey pelagic biodiversity using eDNA and quantitative PCR: a case study of devil ray at seamounts. Mar. Biol. 164:112. doi: 10.1007/s00227-017-3141-x
Garzoli, L., Gnavi, G., Tamma, F., Tosi, S., Varese, G. C., and Picco, A. M. (2015). Sink or swim: updated knowledge on marine fungi associated with wood substrates in the Mediterranean Sea and hints about their potential to remediate hydrocarbons. Prog. Oceanogr. 137, 140–148. doi: 10.1016/j.pocean.2015.05.028
Garzoli, L., Poli, A., Prigione, V., Gnavi, G., and Varese, G. C. (2018). Peacock’s tail with a fungal cocktail: first assessment of the mycobiota associated with the brown alga Padina pavonica. Fungal Ecol. 35, 87–97. doi: 10.1016/j.funeco.2018.05.005
Gaudêncio, S. P., and Pereira, F. (2015). Dereplication: racing to speed up the natural products discovery process. Nat. Prod. Rep. 32, 779–810. doi: 10.1039/c4np00134f
Gautam, K., Tripathi, J. K., Pareek, A., and Sharma, D. K. (2019). Growth and secretome analysis of possible synergistic interaction between green algae and cyanobacteria. J. Biosci. Bioeng. 127, 213–221. doi: 10.1016/j.jbiosc.2018.07.005
Gavahian, M., Chu, Y. H., and Sastry, S. (2018). Extraction from food and natural products by moderate electric field: mechanisms, benefits, and potential industrial applications. Compr. Rev. Food Sci. Food Saf. 17, 1040–1052. doi: 10.1111/1541-4337.12362
Ge, L., Wang, P., and Mou, H. (2011). Study on saccharification techniques of seaweed wastes for the transformation of ethanol. Renew. Energy 36, 84–89. doi: 10.1016/j.renene.2010.06.001
Gelatin Manufacturers Institute of America [GMIA] (2019). Gelatin Handbook. Available online at: http://www.gelatin-gmia.com/uploads/1/1/8/4/118450438/gmia_gelatin_manual_2019.pdf (accessed August 22, 2020).
Gentric, C., Rehel, K., Dufour, A., and Sauleau, P. (2015). Bioaccumulation of metallic trace elements and organic pollutants in marine sponges from the South Brittany Coast, France. J. Environ. Sci. Heal. A 51, 213–219. doi: 10.1080/10934529.2015.1094327
Gerovasileiou, V., Chintiroglou, C., Vafidis, D., Koutsoubas, D., Sini, M., Dailianis, T., et al. (2015). Census of biodiversity in marine caves of the Eastern Mediterranean Sea. Mediterr. Mar. Sci. 16, 245–265. doi: 10.12681/mms.1069
Gerovasileiou, V., and Voultsiadou, E. (2012). Marine caves of the Mediterranean Sea: a sponge biodiversity reservoir within a biodiversity hotspot. PLoS One 7:e39873. doi: 10.1371/journal.pone.0039873
Gerwick, W. H., Bradley, S., and Moore, B. S. (2012). Lessons from the past and charting the future of marine natural products drug discovery and chemical biology. Chem. Biol. 19, 85–98. doi: 10.1016/j.chembiol.2011.12.014
Gerwick, W. H., and Fenner, A. M. (2013). Drug discovery from marine microbes. Microb. Ecol. 65, 800–806. doi: 10.1007/s00248-012-0169-9
Geyer, R., Jambeck, J. R., and Law, K. L. (2017). Production, use, and fate of all plastics ever made. Sci. Adv. 3:e1700782. doi: 10.1126/sciadv.1700782
Ghaly, A. E., Ramakrishnan, V. V., Brooks, M. S., Budge, S. M., and Dave, D. (2013). Fish processing wastes as a potential source of proteins, amino acids and oils: a critical review. J. Microb. Biochem. Technol. 5, 107–129.
Gheysari, H., Mohandes, F., Mazaheri, M., Dolatyar, B., Askari, M., and Simchi, A. (2020). Extraction of hydroxyapatite nanostructures from marine wastes for the fabrication of biopolymer-based porous scaffolds. Mar. Drugs 18:26. doi: 10.3390/md18010026
Ghosh, S., Gnaim, R., Greiserman, S., Fadeev, L., Gozin, M., and Golberg, A. (2019). Macroalgal biomass subcritical hydrolysates for the production of polyhydroxyalkanoate (PHA) by Haloferax mediterranei. Bioresour. Technol. 271, 166–173. doi: 10.1016/j.biortech.2018.09.108
Ghuneim, L. A. J., Jones, D. L., Golyshin, P. N., and Golyshina, O. V. (2018). Nano-sized and filterable bacteriaand archaea: biodiversity and function. Front. Microbiol. 9:1971. doi: 10.3389/fmicb.2018.01971
Giacomotto, J., and Ségalat, L. (2010). High-Throughput screening and small animal models, where are we? Br. J. Pharmacol. 160, 204–216. doi: 10.1111/j.1476-5381.2010.00725.x
Giani, M., Garbayo, I., Vílchez, C., and Martínez-Espinosa, R. M. (2019). Haloarchaeal carotenoids: healthy novel compounds from extreme environments. Mar. Drugs 17:524. doi: 10.3390/md17090524
Girão, M., Ribeiro, I., Ribeiro, T., Azevedo, I. C., Pereira, F., Urbatzka, R., et al. (2019). Actinobacteria isolated from Laminaria ochroleuca: a source of new bioactive compounds. Front. Microbiol. 10:683. doi: 10.3389/fmicb.2019.00683
Giugni, D., and Giugni, V. (2010). Intellectual Property: a powerful tool to develop biotech research. Microb. Biotechnol. 3, 493–506. doi: 10.1111/j.1751-7915.2010.00172.x
Glöckner, F. O., and Joint, I. (2010). Marine microbial genomics in Europe: current status and perspectives. Microb. Biotechnol. 3, 523–530. doi: 10.1111/j.1751-7915.2010.00169.x
Gnavi, G., Garzoli, L., Poli, A., Prigione, V., Burgaud, G., and Varese, G. C. (2017). The culturable mycobiota of Flabellia petiolata: first survey of marine fungi associated to a Mediterranean green alga. PLoS One 12:e0175941. doi: 10.1371/journal.pone.0175941
Goettel, M., Eing, C., Gusbeth, C., and Frey, W. (2013). Pulsed electric field assisted extraction of intracellular valuables from microalgae. Algal Res. 2, 401–408. doi: 10.1016/j.algal.2013.07.004
Gojkovic, Z., Lindberg, R. H., Tysklind, M., and Funk, C. (2019). Northern green algae have the capacity to remove active pharmaceutical ingredients. Ecotoxicol. Environ. Saf. 170, 644–656. doi: 10.1016/j.ecoenv.2018.12.032
Gokhale, S., Jyoti, K., and Lele, S. (2008). Kinetic and equilibrium modeling of chromium (VI) biosorption on fresh and spent Spirulina platensis/Chlorella vulgaris biomass. Bioresour. Technol. 99, 3600–3608. doi: 10.1016/j.biortech.2007.07.039
Golubic, S., Seong-Joo, L., and Browne, K. M. (2000). “Cyanobacteria: architects of sedimentary structures,” in Microbial Sediments, eds R. E. Riding and S. M. Awramik (Berlin: Springer), 57–67. doi: 10.1007/978-3-662-04036-2_8
González-Menéndez, V., Crespo, G., de Pedro, N., Diaz, C., Martín, J., Serrano, R., et al. (2018). Fungal endophytes from arid areas of Andalusia: high potential sources for antifungal and antitumoral agents. Sci. Rep. 8:9729. doi: 10.1038/s41598-018-28192-5
Gouda, S., Das, G., Sen, S. K., Shin, H. S., and Patra, J. K. (2016). Endophytes: a treasure house of bioactive compounds of medicinal importance. Front. Microbiol. 7:1538. doi: 10.3389/fmicb.2016.01538
Grassi, F., Mastrorilli, M., Mininni, C., Parente, A., Santino, A., Scarcella, M., et al. (2015). Posidonia residues can be used as organic mulch and soil amendment for lettuce and tomato production. Agron. Sustain. Dev. 35, 679–689. doi: 10.1007/s13593-014-0268-8
Greunke, C., Glöckle, A., Antosch, J., and Gulder, T. A. M. (2017). Biocatalytic total synthesis of ikarugamycin. Angew. Chem. Int. Ed. 56, 4351–4355. doi: 10.1002/anie.201611063
Griffiths, K., Aggarwal, B., Singh, R., Buttar, H., Wilson, D., and De Meester, F. (2016). Food antioxidants and their anti-inflammatory properties: a potential role in cardiovascular diseases and cancer prevention. Diseases 4:28. doi: 10.3390/diseases4030028
Grignon-Dubois, M., and Rezzonico, B. (2013). The economic potential of beach-cast seagrass – Cymodocea nodosa: a promising renewable source of chicoric acid. Bot. Marina 56, 303–311.
Grob, C., Ulloa, O., Claustre, H., Huot, Y., Alarcon, G., and Marie, D. (2007). Contribution of picoplankton to the total particulate organic carbon concentration in the eastern South Pacific. Biogeosciences 4, 837–852. doi: 10.5194/bg-4-837-2007
Grosso, C., Valentão, P., Ferreres, F., and Andrade, P. B. (2015). Alternative and efficient extraction methods for marine-derived compounds. Mar. Drugs 13, 3182–3230. doi: 10.3390/md13053182
Großkopf, T., and Soyer, O. S. (2014). Synthetic microbial communities. Curr. Opin. Microbiol. 18, 72–77. doi: 10.1016/j.mib.2014.02.002
Guan, C., Parrot, D., Wiese, J., Sönnichsen, F. D., Saha, M., Tasdemir, D., et al. (2017). Identification of rosmarinic acid and sulfated flavonoids as inhibitors of microfouling on the surface of eelgrass Zostera marina. Biofouling 33, 867–880. doi: 10.1080/08927014.2017.1383399
Guillén, J., Martínez Vidal, J., Triviño, A., Soler, G., Fages, E., Torre, L., et al. (2014). Guida Di Buone Prassi Per la Gestione, Rimozione e Trattamento Delle Banquette di Alghe e Piante Marine Lungo le Coste. Progetto Seamatter LIFE11 ENV/ES/000600. El Campello: Instituto de Ecología Litoral.
Gültürk, E. A., Güden, M., and Taşdemirci, A. (2013). Calcined and natural frustules filled epoxy matrices: the effect of volume fraction on the tensile and compression behavior. Compos. B. Eng. 44, 491–500. doi: 10.1016/j.compositesb.2012.03.022
Guo, W. Q., Zheng, H. S., Li, S., Du, J. S., Feng, X. C., Yin, R. L., et al. (2016). Removal of cephalosporin antibiotics 7-ACA from wastewater during the cultivation of lipid-accumulating microalgae. Bioresour. Technol. 221, 284–290. doi: 10.1016/j.biortech.2016.09.036
Gupta, S., and Abu-Ghannam, N. (2011). Recent developments in the application of seaweeds or seaweed extracts as a means for enhancing the safety and quality attributes of foods. Innov. Food Sci. Emerg. Technol. 12, 600–609. doi: 10.1016/j.ifset.2011.07.004
Gustafson, K., Roman, M., and Fenical, W. (1989). The macrolactins, a novel class of antiviral and cytotoxic macrolides from a deep-sea marine bacterium. J. Am. Chem. Soc. 111, 7519–7524. doi: 10.1021/ja00201a036
Gustavsson, J., Cederberg, C., Sonesson, U., van Otterdijk, R., and Meybeck, A. (2011). Global Food Losses and Food Waste – Extent, Causes and Prevention. Study Conducted for the International Congress Save Food! Düsseldorf, Germany, 16–17 May 2011. Rome: FAO.
Guttormsen, A. G. (2002). Input Factor substitutability in salmon aquaculture. Mar. Resour. Econ. 17, 91–102. doi: 10.1086/mre.17.2.42629354
Hamann, M. T., Otto, C. S., Scheuer, P. J., and Dunbar, D. C. (1996). Kahalalides: bioactive peptides from a marine mollusk Elysia rufescens and its algal diet Bryopsis sp.(1). Org. Lett. 61, 6594–6600. doi: 10.1021/jo960877%2B
Han, W., Clarke, W., and Pratt, S. (2014). Composting of waste algae: a review. Waste Manag. 34, 1148–1155. doi: 10.1016/j.wasman.2014.01.019
Hansen, K. Ø, Andersen, J. H., Bayer, A., Pandey, S. K., Lorentzen, M., Jørgensen, K. B., et al. (2019). Kinase chemodiversity from the Artic: the breitfussins. J. Med. Chem. 62, 10167–10181. doi: 10.1021/acs.jmedchem.9b01006
Harada, L. K., Silva, E. C., Campos, W. F., Del Fiol, F. S., Vila, M., Da̧browska, K., et al. (2018). Biotechnological applications of bacteriophages: state of the art. Microbiol. Res. 21, 38–58. doi: 10.1016/j.micres.2018.04.007
Hårdeman, F., and Sjöling, S. (2007). Metagenomic approach for the isolation of a novel low-temperature-active lipase from uncultured bacteria of marine sediment. FEMS Microbiol. Ecol. 59, 524–534. doi: 10.1111/j.1574-6941.2006.00206.x
Harnedy, P. A., and FitzGerald, R. J. (2012). Bioactive peptides from marine processing waste and shellfish: a review. J. Funct. Foods 4, 6–24. doi: 10.1016/j.jff.2011.09.001
Harnedy, P. A., and FitzGerald, R. J. (2015). “Extraction and enrichment of protein from red and green macroalgae,” in Natural Products from Marine Algae, Methods in Molecular Biology, eds D. B. Stengel and S. Connan (New York, NY: Humana Press), 103–108. doi: 10.1007/978-1-4939-2684-8_4
Hartmann, E. M., Durighello, E., Pible, O., Nogales, B., Beltrametti, F., Bosch, R., et al. (2014). Proteomics meets blue biotechnology: a wealth of novelties and opportunities. Mar. Genomics 17, 35–42. doi: 10.1016/j.margen.2014.04.003
Haugen, H. J., Lyngstadaas, S. P., Rossi, F., and Perale, G. (2019). Bone grafts: which is the ideal biomaterial? J. Clin. Periodontol. 46, 92–102. doi: 10.1111/jcpe.13058
Hawrot-Paw, M., Koniuszy, A., Gałczyńska, M., Zaja̧c, G., and Szyszlak-Bargłowicz, J. (2020). Production of microalgal biomass using aquaculture wastewater as growth medium. Water 12:106. doi: 10.3390/w12010106
Heggeset, T. M. B., Ertesvåg, H., Liu, B., Elingsen, T. E., Vadstein, O., and Aasen, I. M. (2019). Lipid and DHA-production in Aurantiochytrium sp. – Responses to nitrogen starvation and oxygen limitation revealed by analyses of production kinetics and global transcriptomes. Sci. Rep. 9:19470. doi: 10.1038/s41598-019-55902-4
Hejazi, M. A., Holwerda, E., and Wijffels, R. H. (2004). Milking microalga Dunaliella salina for β-carotene production in two-phase bioreactors. Biotechnol. Bioeng. 85, 475–481. doi: 10.1002/bit.10914
Hejazi, M. A., and Wijffels, R. H. (2004). Milking of microalgae. Trends Biotechnol. 22, 189–194. doi: 10.1016/j.tibtech.2004.02.009
Hellio, C., Trepos, R., Aguila-Ramírez, R. N., and Hernández-Guerrero, C. J. (2015). “Protocol for assessing antifouling activities of macroalgal extracts,” in Natural Products from Marine Algae, eds D. B. Stengel and S. Connan (New York: Humana Press), 421–435. doi: 10.1007/978-1-4939-2684-8_27
Hernandes Coutinho, F., Silveira, C. B., Gregoracci, G. B., Thompson, C. C., Edwards, R. A., Brussaard, C. P. D., et al. (2017). Marine viruses discovered via metagenomics shed light on viral strategies throughout the oceans. Nat. Commun. 8:15955.
Hernandes Coutinho, F., Bueno Gregoracci, G., Walter, J. M., Carneiro Thompson, C., and Thompson, F. L. (2018). Metagenomics sheds light on the ecology of marine microbes and their viruses. Trends Microbiol. 26, 955–965. doi: 10.1016/j.tim.2018.05.015
Hernandes Coutinho, F., Rosselli, R., and Rodríguez-Valera, F. (2019). Trends of microdiversity reveal depth-dependent evolutionary strategies of viruses in the Mediterranean. mSystems 4:e00554-19. doi: 10.1128/mSystems.00554-19
Hernández-López, E. L., Gasperin, J., Bernáldez-Sarabia, J., Licea-Navarro, A. F., Guerrero, A., and Lizárraga-Partida, M. L. (2019). Detection of Alcanivorax spp., Cycloclasticus spp., and methanomicrobiales in water column and sediment samples in the Gulf of Mexico by qPCR. Environ. Sci. Pollut Res. 26, 35131–35139. doi: 10.1007/s11356-019-06551-7
Herrero, M., and Thornton, P. K. (2013). Livestock and global change: emerging issues for sustainable food systems. Proc. Natl. Acad. Sci. U.S.A. 110, 20878–20881. doi: 10.1073/pnas.1321844111
Hirata, Y., and Uemura, D. (1986). Halichondrins - antitumor polyether macrolides from a marine sponge. Pure Appl. Chem. 58, 701–710. doi: 10.1351/pac198658050701
Holmström, C., Egan, S., Franks, A., McCloy, S., and Kjelleberg, S. (2002). Antifouling activities expressed by marine surface associated Pseudoalteromonas species. FEMS Microbiol. Ecol. 41, 47–58. doi: 10.1016/s0168-6496(02)00239-8
Hong, Z. S., Kim, E. J., Jin, Y. C., Lee, J. S., Choi, Y. J., and Lee, H. G. (2015). Effects of supplementing brown seaweed by-products in the diet of Holstein cows during transition on ruminal fermentation, growth performance and endocrine responses. Asian Australas. J. Anim. Sci. 28, 1296–1302. doi: 10.5713/ajas.15.0235
Horiuchi, A., Aslam, M., Kanai, T., and Atomi, H. (2016). A structurally novel chitinase from the chitin-degrading hyperthermophilic archaeon Thermococcus chitonophagus. Appl. Environ. Microbiol. 82, 3554–3562. doi: 10.1128/aem.00319-16
Hossain, M. P., Rabeta, M. S., and Husnul Azan, T. (2019). Medicinal and therapeutic properties of cephalopod ink: a short review. Food Res. 3, 188–198. doi: 10.26656/fr.2017.3(3).201
Hou, J., and Cui, H. L. (2018). In vitro antioxidant, antihemolytic, and anticancer activity of the carotenoids from halophilic Archaea. Curr. Microbiol. 75, 266–271. doi: 10.1007/s00284-017-1374-z
Hsieh, Y.-H., Leong, F. M., and Rudloe, J. (2001). Jellyfish as food. Hydrobiologia 451, 11–17. doi: 10.1007/978-94-010-0722-1_2
Hu, Y., Wang, M., Wu, C., Tan, Y., Li, J., Hao, X., et al. (2018). Identification and proposed relative and absolute configurations of niphimycins C–E from the marine-derived Streptomyces sp. IMB7-145 by genomic analysis. J. Nat. Prod. 81, 178–187. doi: 10.1021/acs.jnatprod.7b00859
Huang, I. S., and Zimba, P. V. (2019). Cyanobacterial bioactive metabolites-A review of their chemistry and biology. Harmful Algae 83, 42–94. doi: 10.1016/j.hal.2018.11.008
Hudson, G. A., and Mitchell, D. A. (2018). RiPP antibiotics: biosynthesis and engineering potential. Curr. Opin. Microbiol. 45, 61–69. doi: 10.1016/j.mib.2018.02.010
Hug, L. A., Baker, B. J., Anantharaman, K., Brown, C. T., Probst, A. J., Castelle, C. J., et al. (2016). A new view of the tree of life. Nat. Microbiol. 1:16048. doi: 10.1038/nmicrobiol.2016.48
Hughes, C. C., MacMillan, J. B., Gaudêncio, S. P., Fenical, W., and Clair, J. L. (2009a). Ammosamides A and B target myosin. Angew. Chem. Int. Ed. 48, 728–732. doi: 10.1002/anie.200804107
Hughes, C. C., MacMillan, J. B., Gaudêncio, S. P., Jensen, P. R., and Fenical, W. (2009b). The ammosamides: structures of cell cycle modulators from a marine-derived Streptomyces sp. Angew. Chem. Int. Ed. 48, 725–727. doi: 10.1002/anie.200804890
Hurst, D., Børresen, T., Almesjö, L., De Raedemaecker, F., and Bergseth, S. (2016). Marine Biotechnology Strategic Research and Innovation Roadmap: Insights to the Future Direction of European Marine Biotechnology. Oostende: Marine Biotechnology ERA-NET.
Hussein, H. J., Naji, S. S., and Sahi, N. (2018). Antibacterial properties of the Chlorella vulgaris isolated from polluted water in Iraq. Int. J. Pharm. Sci. Res. 10, 2457–2460.
Hwang, W. Y., Fu, Y., Reyon, D., Maeder, M. L., Tsai, S. Q., Sander, J. D., et al. (2013). Efficient genome editing in zebrafish using a CRISPR-Cas system. Nat. Biotechnol. 31, 227–229.
Ihn, H. J., Kim, J. A., Lim, S., Nam, S. H., Hwang, S. H., Lim, J., et al. (2019). Fermented oyster extract prevents ovariectomy-induced bone loss and suppresses osteoclastogenesis. Nutrients 11:e1392. doi: 10.3390/nu11061392
Ilan, M., Contini, H., Carmeli, S., and Rinkevich, B. (1996). Progress towards cell cultures from a marine sponge that produces bioactive compounds. J. Mar. Biotechnol. 4, 145–149.
Imhoff, J. F. (2016). Natural products from marine fungi—still an underrepresented resource. Mar. Drugs 14:19. doi: 10.3390/md14010019
Ishibashi, Y., Aoki, K., Okino, N., Hayashi, M., and Ito, M. (2019). A thraustochytrid-specific lipase/phospholipase with unique positional specificity contributes to microbial competition and fatty acid acquisition from the environment. Sci. Rep. 9:16357. doi: 10.1038/s41598-019-52854-7
Ishika, T., Laird, D. W., Bahri, P. A., and Moheimani, N. R. (2019). Co-cultivation and stepwise cultivation of Chaetoceros muelleri and Amphora sp. for fucoxanthin production under gradual salinity increase. J. Appl. Phycol. 31, 1535–1544. doi: 10.1007/s10811-018-1718-5
Ivošević DeNardis, N., Pletikapić, G., Frkanec, R., Horvat, L., and Vernier, P. T. (2020). From algal cells to autofluorescent ghost plasma membrane vesicles. Bioelectrochemistry 134:107524. doi: 10.1016/j.bioelechem.2020.107524
Jager, U., and Hutchings, M. (2015). Antibody–drug conjugates in relapsed/refractory CD30-positive lymphomas. Eur. Oncol. Haematol. 11, 123–129. doi: 10.17925/eoh.2015.11.02.123
Jakobsen, S. E., Fløysand, A., and Overton, J. (2019). Expanding the field of Responsible Research and Innovation (RRI) – from responsible research to responsible innovation. Eur. Plann. Stud. 27, 2329–2343. doi: 10.1080/09654313.2019.1667617
Jang, K. H., Nam, S. J., Locke, J. B., Kauffman, C. A., Beatty, D. S., Paul, L. A., et al. (2013). Anthracimycin, a potent anthrax antibiotic from a marine-derived actinomycete. Angew. Chem. Int. Ed. 52, 7822–7824. doi: 10.1002/anie.201302749
Janssen, E. M. L. (2019). Cyanobacterial peptides beyond microcystins – A review on co-occurrence, toxicity, and challenges for risk assessment. Water Res. 151, 488–499. doi: 10.1016/j.watres.2018.12.048
Jem, K. J., and Tan, B. (2020). The development and challenges of poly (lactic acid) and poly (glycolic acid). Adv. Ind. Eng. Polym. Res. 3, 60–70. doi: 10.1016/j.aiepr.2020.01.002
Jeunen, G. J., Knapp, M., Spencer, H. G., Lamare, M. D., Taylor, H. R., Stat, M., et al. (2019). Environmental DNA (eDNA) metabarcoding reveals strong discrimination among diverse marine habitats connected by water movement. Mol. Ecol. Resour. 19, 426–438. doi: 10.1111/1755-0998.12982
Jimenez, P. C., Wilke, D. V., Branco, P. C., Bauermeister, A., Rezende-Teixeira, P., Gaudencio, S. P., et al. (2020). Enriching cancer pharmacology with drugs of marine origin. Br. J. Pharmacol. 177, 3–27. doi: 10.1111/bph.14876
Jin, J., Yang, X., Liu, T., Xiao, H., Wang, G., Zhou, M., et al. (2018). Fluostatins M–Q featuring a 6-5-6-6 ring skeleton and high oxidized A-rings from marine Streptomyces sp. PKU-MA00045. Mar. Drugs 16:87. doi: 10.3390/md16030087
Jin, Q., Yu, H., and Li, P. (2018). The evaluation and utilization of marine-derived bioactive compounds with anti-obesity effect. Curr. Med. Chem. 25, 861–878. doi: 10.2174/0929867324666170602082620
John, G., Nagarajan, S., Kumar Vemula, P., Silverman, J. R., and Pillai, C. K. S. (2019). Natural monomers: a mine for functional and sustainable materials – Occurrence, chemical modification and polymerization. Prog. Polym. Sci. 92, 158–209. doi: 10.1016/j.progpolymsci.2019.02.008
Joint, I., Mühling, M., and Querellou, J. (2010). Culturing marine bacteria - an essential prerequisite for biodiscovery. Microb. Biotechnol. 3, 564–575. doi: 10.1111/j.1751-7915.2010.00188.x
Jones, E. B. G., Suetrong, S., Sakayaroj, J., Bahkali, A. H., Abdel-Wahab, M. A., Boekhout, T., et al. (2015). Classification of marine Ascomycota, Basidiomycota, Blastocladiomycota and Chytridiomycota. Fungal Divers. 73, 1–72. doi: 10.1007/s13225-015-0339-4
Jones, M. R., Pinto, E., Torres, M. A., Dörr, F., Mazur-Marzec, H., Szubert, K., et al. (2020). Comprehensive database of secondary metabolites from cyanobacteria. bioRxiv [Preprint]. doi: 10.1101/2020.04.16.038703
Jones, R. M., Cartier, E. G., McIntosh, M. J., Bulaj, G., Farrar, V. E., and Olivera, B. M. (2001). Composition and therapeutic utility of conotoxins from genus Conus. Patent status 1996-2000. Expert Opin. Ther. Pat. 11, 603–623. doi: 10.1517/13543776.11.4.603
Joshi, S., Eshwar, S., and Jain, V. (2019). “Marine polysaccharides: biomedical and tissue engineering applications,” in Marine-Derived Biomaterials for Tissue Engineering Applications, eds A. H. Choi and B. Ben-Nissan (Singapore: Springer), 443–487. doi: 10.1007/978-981-13-8855-2_19
Kaeberlein, T., Lewis, K., and Epstein, S. S. (2002). Isolating “uncultivable” microorganisms in pure culture in a simulated natural environment. Science 296, 1127–1129. doi: 10.1126/science.1070633
Kagiya, N., Reinsch, T., Taube, F., Salminen, J.-P., Kluß, C., Hasler, M., et al. (2019). Turnover rates of roots vary considerably across temperate forage species. Soil Biol. Biochem. 139:107614. doi: 10.1016/j.soilbio.2019.107614
Kanakkanthara, A., Northcote, P. T., Miller, J. H., and Peloruside, A. (2016). A lead non-taxoid-sitemicrotubule-stabilizing agent with potential activity against cancer, neuro-degeneration, and autoimmune disease. Nat. Prod. Rep. 33, 549–561. doi: 10.1039/c5np00146c
Kaushik, S. J., Coves, D., Dutto, G., and Blanc, D. (2004). Almost total replacement of fish meal by plant protein sources in the diet of a marine teleost, the European seabass, Dicentrarchus labrax. Aquaculture 230, 391–404. doi: 10.1016/s0044-8486(03)00422-8
Kelly, R. P., Closek, C. J., O’Donnell, J. L., Kralj, J. E., Shelton, A. O., and Samhouri, J. F. (2017). Genetic and manual survey methods yield different and complementary views of an ecosystem. Front. Mar. Sci. 3:283. doi: 10.3389/fmars.2016.00283
Keuter, S., Rahav, E., Herut, B., and Rinkevich, B. (2015). Distribution patterns of bacterioplankton in the oligotrophic south-eastern Mediterranean Sea. FEMS Microbiol. Ecol. 91:fiv070. doi: 10.1093/femsec/fiv070
Keuter, S., and Rinkevich, B. (2016). Spatial homogeneity of bacterial and archaeal communities in the deep eastern Mediterranean Sea surface sediments. Int. Microbiol. 19, 109–119.
Khrunyk, Y., Lach, S., Petrenko, I., and Ehrlich, H. (2020). Progress in modern marine biomaterials research. Mar. Drugs 18:589. doi: 10.3390/md18120589
Kildgaard, S., Subko, K., Phillips, E., Goidts, V., de la Cruz, M., Díaz, C., et al. (2017). A dereplication and bioguided discovery approach to reveal new compounds from a marine-derived fungus Stilbella fimetaria. Mar. Drugs 15:253. doi: 10.3390/md15080253
Kim, D. Y., Vijayan, D., Praveenkumar, R., Han, J. I., Lee, K., Park, J. Y., et al. (2016). Cell-wall disruption and lipid/astaxanthin extraction from microalgae: Chlorella and Haematococcus. Bioresour. Technol. 199, 300–310. doi: 10.1016/j.biortech.2015.08.107
Kim, J. H., and Scialli, A. R. (2011). Thalidomide: the tragedy of birth defects and the effective treatment of disease. Toxicol. Sci. 122, 1–6. doi: 10.1093/toxsci/kfr088
Kim, M. C., Winter, J. M., Cullum, R., Li, Z., and Fenical, W. (2020). Complementary genomic, bioinformatics, and chemical approaches facilitate the absolute structure assignment of ionostatin, a linear polyketide from a rare marine-derived actinomycete. ACS Chem. Biol. 15, 2507–2515. doi: 10.1021/acschembio.0c00526
Kim, M. K., and Lee, J. S. (2018). Short-term plasticity and long-term potentiation in artificial biosynapses with diffusive dynamics. ACS Nano 12, 1680–1687. doi: 10.1021/acsnano.7b08331
Kim, S. K. (2013). Marine Biomaterials: Characterization, Isolation and Applications. Boca Raton: CRC Press.
Kim, S. K., and Mendis, E. (2006). Bioactive compounds from marine processing by-products—a review. Food Res. Int. 39, 383–393.
Kini, S., Divyashree, M., Mani, K. M., and Mamatha, S. B. (2020). “Algae and cyanobacteria as a source of novel bioactive compounds for biomedical applications,” in Advances in Cyanobacterial Biology, eds P. Kumar Singh, A. Kumar, V. Kumar Singh, and A. Kumar Shrivastava (Cambridge, MA: Academic Press), 173–194. doi: 10.1016/b978-0-12-819311-2.00012-7
Kinley, R. D., Martinez-Fernandez, G., Matthews, M. K., de Nys, R., Magnusson, M., and Tomkins, N. W. (2020). Mitigating the carbon footprint and improving productivity of ruminant livestock agriculture using a red seaweed. J. Clean. Prod. 259:120836. doi: 10.1016/j.jclepro.2020.120836
Kirschning, A., and Hahn, F. (2012). Merging chemical synthesis and biosynthesis: a new chapter in the total synthesis of natural products and natural product libraries. Angew. Chem. Int. Ed. 51, 4012–4022. doi: 10.1002/anie.201107386
Klavins, M., Bisters, V., and Burlakovs, J. (2019). Small scale gasification application and perspectives in circular economy. Env. Clim. Tech. 22, 42–54. doi: 10.2478/rtuect-2018-0003
Kloosterman, A. M., Medema, M. H., and van Wezel, G. P. (2021). Omics-based strategies to discover novel classes of RiPP natural products. Curr. Opin. Microbiol. 69, 60–67. doi: 10.1016/j.copbio.2020.12.008
Kolanti, E., Chandra Veerla, S., Kumar Khajuria, D., and Roy Mahapatra, D. (2016). Optical diagnostics of osteoblast cells and osteogenic drug screening. Photon. Ther. Diagn. XII Proc. 9689:96894I. doi: 10.1117/12.2217943
Kouzuma, A., and Watanabe, K. (2014). Microbial ecology pushes frontiers in biotechnology. Microbes Environ. 29, 1–3. doi: 10.1264/jsme2.ME2901rh
Kratky, L., and Zamazal, P. (2020). Economic feasibility and sensitivity analysis of fish waste processing biorefinery. J. Clean. Prod. 243:118677. doi: 10.1016/j.jclepro.2019.118677
Krause-Jensen, D., and Duarte, C. M. (2016). Substantial role of macroalgae in marine carbon sequestration. Nat. Geosci. 9, 737–742. doi: 10.1038/ngeo2790
Kristufek, S. L., Wacker, K. T., Tsao, Y. Y. T., Su, L., and Wooley, K. L. (2017). Monomer design strategies to create natural product-based polymer materials. Nat. Prod. Rep. 34, 433–459. doi: 10.1039/c6np00112b
Kroll, J., Klinter, S., Schneider, C., Voß, I., and Steinbüchel, A. (2010). Plasmid addiction systems: perspectives and applications in biotechnology. Microb. Biotechnol. 3, 634–657. doi: 10.1111/j.1751-7915.2010.00170.x
Kroth, P. G., Bones, A. M., Daboussi, F., Ferrante, M. I., Jaubert, M., Kolot, M., et al. (2018). Genome editing in diatoms: achievements and goals. Plant Cell Rep. 37, 1401–1408. doi: 10.1007/s00299-018-2334-1
Krüger, A., Schäfers, C., Schröder, C., and Antranikian, G. (2018). Towards a sustainable biobased industry–highlighting the impact of extremophiles. New Biotechnol. 40, 144–153. doi: 10.1016/j.nbt.2017.05.002
Kumar, K. S., Dahms, H. U., Won, E. J., Lee, J. S., and Shin, K. H. (2015). Microalgae–a promising tool for heavy metal remediation. Ecotoxicol. Environ. Saf. 113, 329–352. doi: 10.1016/j.ecoenv.2014.12.019
Kumar, M. S. (2019). Peptides and peptidomimetics as potential antiobesity agents: overview of current status. Front. Nutr. 6:11. doi: 10.3389/fnut.2019.00011
Kumar, P. S., Saravanan, A., Jayasree, R., and Jeevanantham, S. (2020). “18 - Food industry waste biorefineries,” in Refining Biomass Residues for Sustainable Energy and Bioproducts, eds R. P. Kumar, E. Gnansounou, J. K. Raman, and G. Baskar (Cambridge, MA: Academic Press), 407–426. doi: 10.1016/b978-0-12-818996-2.00018-1
Kumari, B., Tiwari, B. K., Hossain, M. B., Brunton, N. P., and Rai, D. K. (2018). Recent advances on application of ultrasound and pulsed electric field technologies in the extraction of bioactives from agro-industrial by-products. Food Bioprocess. Technol. 11, 223–241. doi: 10.1007/s11947-017-1961-9
Kwon, H. C., Kauffman, C. A., Jensen, P. R., and Fenical, W. (2006). Marinomycins A-D, antitumor-antibiotics of a new structure class from a marine actinomycete of the recently discovered genus “Marinispora”. J. A Chem. Soc. 128, 1622–1632. doi: 10.1021/ja0558948
La Salle, J., Williams, K. J., and Moritz, C. (2016). Biodiversity analysis in the digital era. Philos. Trans. R. Soc. B 371:337. doi: 10.1098/rstb.2015.0337
Laizé, V., Gavaia, P. J., and Cancela, M. L. (2014). Fish: a suitable system to model human bone disorders and discover drugs with osteogenic or osteotoxic activities. Drug Discov. Today Dis. Models 13, 29–37. doi: 10.1016/j.ddmod.2014.08.001
Lallier, L. E., McMeel, O., Greiber, T., Vanagt, T., Dobson, A. D. W., and Jaspars, M. (2014). Access to and use of marine genetic resources: understanding the legal framework. Nat. Prod. Rep. 31, 612–616. doi: 10.1039/c3np70123a
Lam, K. N., Cheng, J., Engel, K., Neufeld, J. D., and Charles, T. C. (2015). Current and future resources for functional metagenomics. Front. Microbiol. 6:1196. doi: 10.3389/fmicb.2015.01196
Lamastra, F. R., Mori, S., Cherubini, V., Scarselli, M., and Nanni, F. (2017). A new green methodology for surface modification of diatomite filler in elastomers. Mater. Chem. Phys. 194, 253–260. doi: 10.1016/j.matchemphys.2017.03.050
Lamb, J. B., Van De Water, J. A., Bourne, D. G., Altier, C., Hein, M. Y., Fiorenza, E. A., et al. (2017). Seagrass ecosystems reduce exposure to bacterial pathogens of humans, fishes, and invertebrates. Science 355, 731–733. doi: 10.1126/science.aal1956
Laohakunjit, N., Selamassakul, O., and Kerdchoechuen, O. (2014). Seafood-like flavour obtained from the enzymatic hydrolysis of the protein by-products of seaweed (Gracilaria sp.). Food Chem. 158, 162–170. doi: 10.1016/j.foodchem.2014.02.101
Lautru, S., Deeth, R. J., Bailey, L. M., and Challis, G. L. (2005). Discovery of a new peptide natural product by Streptomyces coelicolor genome mining. Nat. Chem. Biol. 1, 265–269. doi: 10.1038/nchembio731
Leal, M. C., Calado, R., Sheridan, C., Alimonti, A., and Osinga, R. (2013). Coral aquaculture to support drug discovery. Trends Biotechnol. 31, 555–561. doi: 10.1016/j.tibtech.2013.06.004
Leal, M. C., Hilário, A., Munro, M. H. G., Blunt, J. W., and Calado, R. (2016). Natural products discovery needs improved taxonomic and geographic information. Nat. Prod. Rep. 33, 747–750. doi: 10.1039/c5np00130g
Leal, M. C., Puga, J., Serôdio, J., Gomes, N. C. M., and Calado, R. (2012). Trends in the discovery of new marine natural products from invertebrates over the last two decades – where and what are we bioprospecting? PLoS One 7:e30580. doi: 10.1371/journal.pone.0030580
Ledford, H. (2010). Complex synthesis yields breast-cancer therapy. Nature 468, 608–609. doi: 10.1038/468608a
Lee, H., and Suh, J. W. (2016). Anti-tuberculosis lead molecules from natural products targeting Mycobacterium tuberculosis ClpC1. J. Ind. Microbiol. Biotechnol. 43, 205–212. doi: 10.1007/s10295-015-1709-3
Lee, K. Y., Jang, G. H., Byun, C. H., Jeun, M., Searson, P. C., and Lee, K. H. (2017). Zebrafish models for functional and toxicological screening of nanoscale drug delivery systems: promoting preclinical applications. Biosci. Rep. 37:BSR20170199.
Leese, F., Altermatt, F., Bouchez, A., Ekrem, T., Hering, D., Meissner, K., et al. (2016). DNAqua-Net: developing new genetic tools for bioassessment and monitoring of aquatic ecosystems in Europe. Res. Ideas Outcomes 2:e11321. doi: 10.3897/rio.2.e11321
Leyland, B., Leu, S., and Boussiba, S. (2017). Are thraustochytrids algae? Fungal Biol. 121, 835–840. doi: 10.1016/j.funbio.2017.07.006
Li, D., Wang, F., Xiao, X., Zeng, X., Gu, Q. Q., and Zhu, W. (2007). A new cytotoxic phenazine derivative from a deep sea bacterium Bacillus sp. Arch. Pharm. Res. 30, 552–555. doi: 10.1007/bf02977647
Li, F., Janussen, D., Peifer, C., Pérez-Victoria, I., and Tasdemir, D. (2018). Targeted isolation of tsitsikammamines from the Antarctic deep-sea sponge Latrunculia biformis by molecular networking and anticancer activity. Mar. Drugs 16:268. doi: 10.3390/md16080268
Li, H., Zhong, Y., Lu, Q., Zhang, X., Wang, Q., Liu, H., et al. (2019). Co-cultivation of Rhodotorula glutinis and Chlorella pyrenoidosa to improve nutrient removal and protein content by their synergistic relationship. RSC Adv. 9, 14331–14342. doi: 10.1039/c9ra01884k
Li, P., Harding, S. E., and Liu, Z. (2001). Cyanobacterial exopolysaccharides: their nature and potential biotechnological applications. Biotechnol. Genet. Eng. Rev. 18, 375–404. doi: 10.1080/02648725.2001.10648020
Li, S., Ji, L., Shi, Q., Wu, H., and Fan, J. (2019). Advances in the production of bioactive substances from marine unicellular microalgae Porphyridium spp. Bioresour. Technol. 292:122048. doi: 10.1016/j.biortech.2019.122048
Li, Y. X., Wijesekara, I., Li, Y., and Kim, S. K. (2011). Phlorotannins as bioactive agents from brown algae. Process Biochem. 46, 2219–2224. doi: 10.1016/j.procbio.2011.09.015
Liarzi, O., and Ezra, D. (2014). “Endophyte-mediated biocontrol of herbaceous and non-herbaceous plants,” in Advances in Endophytic Research, eds V. Verma, C. Vijay, and C. Gange Alan (New Delhi: Springer), 335–369. doi: 10.1007/978-81-322-1575-2_18
Liu, L., Yang, H., Cai, Y., Cao, Q., Sun, L., Wang, Z., et al. (2019). In silico prediction of chemical aquatic toxicity for marine crustaceans via machine learning. Toxicol. Res. 8, 341–352. doi: 10.1039/c8tx00331a
Liu, Y., Singh, P., Liang, Y., Li, J., Xie, N., Song, Z., et al. (2017). Abundance and molecular diversity of thraustochytrids in coastal waters of southern China. FEMS Microbiol. Ecol. 93:fix070. doi: 10.1093/femsec/fix070
Lloyd, K. G., Steen, A. D., Ladau, J., Yin, J., and Crosby, L. (2018). Phylogenetically novel uncultured microbial cells dominate earth microbiomes. mSystems 3;e00055-18. doi: 10.1128/mSystems.00055-18
Lopes, A., Rodrigues, M. J., Pereira, C., Oliveira, M., Barreira, L., Varela, J., et al. (2016). Natural products from extreme marine environments: searching for potential industrial uses within extremophile plants. Ind. Crops Prod. 94, 299–307. doi: 10.1016/j.indcrop.2016.08.040
Lotfi, H., Sheervalilou, R., and Zarghami, N. (2018). An update of the recombinant protein expression systems of Cyanovirin-N and challenges of preclinical development. Bioimpacts 8, 139–151. doi: 10.15171/bi.2018.16
Loureiro, C., Medema, M. H., van der Oost, J., and Sipkema, D. (2018). Exploration and exploitation of the environment for novel specialized metabolites. Curr. Opin. Biotechnol. 50, 206–213. doi: 10.1016/j.copbio.2018.01.017
Lovejoy, C., Bowman, J. P., and Hallegraeff, G. M. (1998). Algicidal effects of a novel marine Pseudoalteromonas isolate (Class Proteobacteria, Gamma Subdivision) on harmful algal bloom species of the genera Chattonella, Gymnodinium, and Heterosigma. Appl. Environ. Microbiol. 64, 2806–2813. doi: 10.1128/aem.64.8.2806-2813.1998
Lupini, G., Proia, L., Di Maio, M., Amalfitano, S., and Fazi, S. (2011). CARD–FISH and confocal laser scanner microscopy to assess successional changes of the bacterial community in freshwater biofilms. J. Microbiol. Methods 86, 248–251. doi: 10.1016/j.mimet.2011.05.011
Macha, I. J., Ben-Nissan, B., and Müller, W. (2019). “Marine-based biomaterials for tissue engineering applications,” in Marine-Derived Biomaterials for Tissue Engineering Applications, eds A. H. Choi and B. Ben-Nissan (Singapore: Springer), 99–111. doi: 10.1007/978-981-13-8855-2_5
Machado, H., Sonnenschein, E. C., Melchiorsen, J., and Gram, L. (2015). Genome mining reveals unlocked bioactive potential of marine Gram-negative bacteria. BMC Genomics 16:158. doi: 10.1186/s12864-015-1365-z
Machado, L., Magnusson, M., Paul, N. A., de Nys, R., and Tomkins, N. (2014). Effects of marine and freshwater macroalgae on in vitro total gas and methane production. PLoS One 9:e85289. doi: 10.1371/journal.pone.0085289
Majik, M. S., Tilvi, S., and Parvatkar, P. T. (2014). Recent development on the synthesis of Varitriol, an antitumour agent from marine derived fungus Emericella variecolor. Curr. Org. Synth. 11, 268–287. doi: 10.2174/1570179410666131124134200
Malea, P., Kevrekidis, T., Chatzipanagiotou, K. R., and Mogias, A. (2018). Cadmium uptake kinetics in parts of the seagrass Cymodocea nodosa at high exposure concentrations. J. Biol. Res. Thessalon. 25:5. doi: 10.1186/s40709-018-0076-4
Mandalakis, M., Gavriilidou, A., Polymenakou, P. N., Christakis, C. A., Nomikou, P., Medvecký, M., et al. (2019). Microbial strains isolated from CO2-venting Kolumbo submarine volcano show enhanced co-tolerance to acidity and antibiotics. Mar. Environ. Res. 144, 102–110. doi: 10.1016/j.marenvres.2019.01.002
Mandelare, P. E., Adpressa, D. A., Kaweesa, E. N., Zakharov, L. N., and Loesgen, S. (2018). Coculture of two developmental stages of a marine-derived Aspergillus alliaceus results in the production of the cytotoxic bianthrone allianthrone A. J. Nat. Prod. 81, 1014–1022. doi: 10.1021/acs.jnatprod.8b00024
Mandhare, A., Banerjee, P., and Shinde, P. (2019). Marine natural products as source of new drugs: a patent review (2015–2018). Expert Opin. Ther. Pat. 29, 283–309. doi: 10.1080/13543776.2019.1598972
Manomi, S., Job, N., and Philip, R. (2015). Bioactive potential of endophytic fungi from macro-algae. Int. J. Res. Mar. Sci. 4, 27–32.
Mao, X., Liu, Z., Sun, J., and Lee, S. Y. (2017). Metabolic engineering for the microbial production of marine bioactive compounds. Biotechnol. Adv. 35, 1004–1021. doi: 10.1016/j.biotechadv.2017.03.001
Marcarino, M. O., Zanardi, M. M., Cicetti, S., and Sarotti, A. M. (2020). NMR calculations with quantum methods: development of new tools for structural elucidation and beyond. Acc. Chem. Res. 53, 1922–1932. doi: 10.1021/acs.accounts.0c00365
Marchese, P., Garzoli, L., Gnavi, G., O’Connell, E., Bouraoui, A., Mehiri, M., et al. (2020). Diversity and bioactivity of fungi associated with the marine sea cucumber Holothuria poli: disclosing the strains potential for biomedical applications. J. Appl. Microbiol. 129, 612–625. doi: 10.1111/jam.14659
Margulis, L. (1991). “Symbiogenesis and Symbionticism,” in Symbiosis as a Source of Evolutionary Innovation: Speciation and Morphogenesis, eds L. Margulis and R. Fester (Cambridge, MA: MIT Press), 1–14.
Marine Board (2010). Marine Biotechnology: A New Vision and Strategy for Europe. Position Paper 15, Belgium. Available online at: http://www.marinebiotech.eu/sites/marinebiotech.eu/files/public/library/MBT%20publications/2010%20ESF%20Position%20Paper.pdf (accessed December 18, 2019).
Markou, G., and Nerantzis, E. (2013). Microalgae for high-value compounds and biofuels production: a review with focus on cultivation under stress conditions. Biotechnol. Adv. 31, 1532–1542. doi: 10.1016/j.biotechadv.2013.07.011
Martín, M. J., Rodríguez-Acebes, R., García-Ramos, Y., Martínez, V., Murcia, C., Digón, I., et al. (2014). Stellatolides, a new cyclodepsipeptide family from the sponge Ecionemia acervus: isolation, solid-phase total synthesis, and full structural assignment of stellatolide A. J. Am. Chem. Soc. 136, 6754–6762. doi: 10.1021/ja502744a
Martinez, E. J., and Corey, E. J. (2000). A new, more efficient, and effective process for the synthesis of a key pentacyclic intermediate for production of ecteinascidin and phthalascidin antitumor agents. Org. Lett. 2, 993–996. doi: 10.1021/ol0056729
Martínez Andrade, K. A., Lauritano, C., Romano, G., and Ianora, A. (2018). Marine microalgae with anti-cancer properties. Mar. Drugs 16:165. doi: 10.3390/md16050165
Martins, A., Vieira, H., Gaspar, H., and Santos, S. (2014). Marketed marine natural products in the pharmaceutical and cosmeceutical industries: tips for success. Mar. Drugs 12, 1066–1101. doi: 10.3390/md12021066
Martins, S. E., Fillmann, G., Lillicrap, A., and Thomas, K. V. (2018). Review: ecotoxicity of organic and organo-metallic antifouling co-biocides and implications for environmental hazard and risk assessments in aquatic ecosystems. Biofouling 34, 34–52. doi: 10.1080/08927014.2017.1404036
Mathan, S., Subramanian, V., Nagamony, S., and Ganapathy, K. (2013). Isolation of endophytic fungi from marine algae and its bioactivity. Int. J. Res. Pharm. Sci. 4, 45–49.
Matos, J., Gomes, A., Cardoso, C., Afonso, C., Campos, A. M., Gomes, R., et al. (2020). Commercial Red Seaweed in Portugal (Gelidium sesquipedale and Pterocladiella capillacea, Florideophyceae): going beyond a single-purpose product approach by valorizing bioactivity. Thalassas 36, 213–224. doi: 10.1007/s41208-019-00181-z
Matsunaga, T., Takeyama, H., Miyashita, H., and Yokouchi, H. (2005). Marine microalgae. Adv. Biochem. Engin. Biotechnol. 96, 165–188.
Mattey, M., and Spencer, J. (2008). Bacteriophage therapy - cooked goose or Phoenix rising? Curr. Opin. Biotechnol. 19, 608–612. doi: 10.1016/j.copbio.2008.09.001
Mayer, A. M. S., Guerrero, A. J., Rodríguez, A. D., Taglialatela-Scafati, O., Nakamura, F., and Fusetani, N. (2020). Marine pharmacology in 2014-2015: marine compounds with antibacterial, antidiabetic, antifungal, anti-inflammatory, antiprotozoal, antituberculosis, antiviral, and anthelmintic activities; Affecting the immune and nervous systems, and other miscellaneous mechanisms of action. Mar. Drugs 18:5. doi: 10.3390/md18010005
Mazur, L. P., Cechinel, M. A. P., de Souza, S. M. A. G. U., Boaventura, R. A. R., and Vilar, V. J. P. (2018). Brown marine macroalgae as natural cation exchangers for toxic metal removal from industrial wastewaters: a review. J. Environ. Manage. 223, 215–253. doi: 10.1016/j.jenvman.2018.05.086
Mazur-Marzec, H., Błaszczyk, A., Felczykowska, A., Hohlfeld, N., Kobos, J., Toruńska-Sitarz, A., et al. (2015). Baltic cyanobacteria – a source of biologically active compounds. Eur. J. Phycol. 50, 343–360. doi: 10.1080/09670262.2015.1062563
McAlpine, J. B., Chen, S. N., Kutateladze, A., MacMillan, J. B., Appendino, G., Barison, A., et al. (2019). The value of universally available raw NMR data for transparency, reproducibility, and integrity in natural product research. Nat. Prod. Rep. 36, 35–107.
McCauley, E. P., Piña, I. C., Thompson, A. D., Bashir, K., Weinberg, M., Kurz, S. L., et al. (2020). Highlights of marine natural products having parallel scaffolds found from marine-derived bacteria, sponges, and tunicates. J. Antibiot. 73, 504–525. doi: 10.1038/s41429-020-0330-5
Mehbub, M. F., Lei, J., Franco, C., and Zhang, W. (2014). Marine sponge derived natural products between 2001 and 2010: trends and opportunities for discovery of bioactives. Mar. Drugs 12, 4539–4577. doi: 10.3390/md12084539
Mehle, A., Dugan, V. G., Taubenberger, J. K., and Doudna, J. A. (2012). Reassortment and mutation of the avian influenza virus polymerase PA subunit overcome species barriers. J. Virol. 86, 1750–1757. doi: 10.1128/jvi.06203-11
Melis, A. (2009). Solar energy conversion efficiencies in photosynthesis: minimizing the chlorophyll antennae to maximize efficiency. Plant Sci. 177, 272–280. doi: 10.1016/j.plantsci.2009.06.005
Menna, M., Imperatore, C., Mangoni, A., Della Sala, G., and Tagliatela-Scafati, O. (2019). Challenges in the configuration assignment of natural products. A case-selective perspective. Nat. Prod. Rep. 36, 476–489. doi: 10.1039/c8np00053k
Merquiol, L., Romano, G., Ianora, A., and D’Ambra, I. (2019). Biotechnological applications of Scyphomedusae. Mar. Drugs 17:604. doi: 10.3390/md17110604
Merten, C., Smyrniotopoulos, V., and Tasdemir, D. (2015). Assignment of absolute configurations of highly flexible linear diterpenes from the brown alga Bifurcaria bifurcata by VCD spectroscopy. Chem. Commun. 51, 16217–16220. doi: 10.1039/c5cc05659d
Miao, C. C., Han, L. L., Lu, Y. B., and Feng, H. (2020). Construction of a High-Expression System in Bacillus through Transcriptomic Profiling and Promoter Engineering. Microorganisms 8:1030. doi: 10.3390/microorganisms8071030
Michalak, I., and Chojnacka, K. (2018). Algae Biomass: Characteristics and Applications. Berlin: Springer.
Miller, J. H., Singh, A. J., and Northcote, P. T. (2010). Microtubule-stabilizing drugs from marine sponges: focus on peloruside A and zampanolide. Mar. Drugs 8, 1059–1079. doi: 10.3390/md8041059
Mincer, T. J., Jensen, P. R., Kauffman, C. A., and Fenical, W. (2002). Widespread and persistent populations of a major new marine actinomycete taxon in ocean sediments. Appl. Environ. Microbiol. 68, 5005–5011. doi: 10.1128/aem.68.10.5005-5011.2002
Mirghaffari, N., Moeini, E., and Farhadian, O. (2015). Biosorption of Cd and Pb ions from aqueous solutions by biomass of the green microalga, Scenedesmus quadricauda. J. Appl. Phycol. 27, 311–320. doi: 10.1007/s10811-014-0345-z
Misra, S., and Lotrecchiano, G. R. (2018). Transdisciplinary communication: introduction to the special series. Inform. Sci. J. 21, 14–50.
Mo, C., Douek, J., and Rinkevich, B. (2002). Development of a PCR strategy for thraustochytrids identification based on 18S-rDNA sequence. Mar. Biol. 140, 883–889. doi: 10.1007/s00227-002-0778-9
Mo, C., and Rinkevich, B. (2001). A simple, reliable and fast protocol for thraustochytrids DNA extraction. Mar. Biotechnol. 3, 100–102. doi: 10.1007/s101260000069
Mógor, ÁF., Ördög, V., Lima, G. P. P., Molnár, Z., and Mógor, G. (2018). Biostimulant properties of cyanobacterial hydrolysate related to polyamines. J. Appl. Phycol. 30, 453–460. doi: 10.1007/s10811-017-1242-z
Moheimani, N. R., Matsuura, H., Watanabe, M. M., and Borowitzka, M. A. (2014). Non-destructive hydrocarbon extraction from Botryococcus braunii BOT-22 (race B). J. Appl. Phycol. 26, 1453–1463. doi: 10.1007/s10811-013-0179-0
Molina-Miras, A., Morales-Amador, A., de Vera, C. R., López-Rosales, L., Sánchez-Mirón, A., Souto, M. L., et al. (2018). A pilot-scale bioprocess to produce amphidinols from the marine microalga Amphidinium carterae: isolation of a novel analogue. Algal Res. 31, 87–98. doi: 10.1016/j.algal.2018.01.010
Molinski, T. F., Dalisay, D. S., Lievens, S. L., and Saludes, J. P. (2009). Drug development from marine natural products. Nat. Rev. Drug Discov. 8, 69–85. doi: 10.1038/nrd2487
Mondal, M., Halder, G., Oinam, G., Indrama, T., and Tiwari, O. N. (2019). “Chapter 17 - bioremediation of organic and inorganic pollutants using microalgae,” in New and Future Developments in Microbial Biotechnology and Bioengineering, ed. V. Gupta (Amsterdam: Elsevier), 223–235. doi: 10.1016/b978-0-444-63504-4.00017-7
Mondol, M. A. M., Shin, H. J., and Islam, M. T. (2013). diversity of secondary metabolites from marine Bacillus species: chemistry and biological activity. Mar. Drugs 11, 2846–2872. doi: 10.3390/md11082846
Morabito, C., Bournaud, C., Maës, C., Schuler, M., Cigliano, R. A., Dellero, Y., et al. (2019). The lipid metabolism in thraustochytrids. Prog. Lipid Res. 76:101007. doi: 10.1016/j.plipres.2019.101007
Moreno-Garcia, L., Adjallé, K., Barnabé, S., and Raghavan, V. S. G. (2017). Microalgae biomass production for a biorefinery system: recent advances and the way towards sustainability. Renew. Sust. Energ. Rev. 76, 493–506. doi: 10.1016/j.rser.2017.03.024
Mossbauer, M., Haller, I., Dahlke, S., and Schernewski, G. (2012). Management of stranded eelgrass and macroalgae along the German Baltic coastline. Ocean Coast. Manag. 57, 1–9. doi: 10.1016/j.ocecoaman.2011.10.012
Moumbock, A. F. A., Gao, M., Qaseem, A., Li, J., Kirchner, P. A., Ndingkokhar, B., et al. (2021). StreptomeDB 3.0: an updated compendium of streptomycetes natural products. Nucleic Acids Res. 8:49. doi: 10.1093/nar/gkaa868
Mourelle, M. L., Gómez, C. P., and Legido, J. L. (2017). The potential use of marine microalgae and Cyanobacteria in cosmetics and thalassotherapy. Cosmetics 4:46. doi: 10.3390/cosmetics4040046
Mousing, E. A., Richardson, K., Bendtsen, J., Cetinić, I., and Perry, M. J. (2016). Evidence of small-scale spatial structuring of phytoplankton alpha-and beta-diversity in the open ocean. J. Ecol. 104, 1682–1695. doi: 10.1111/1365-2745.12634
Mozetič, P., Cangini, M., Francé, J., Bastianini, M., Bernardi Aubry, F., Bužančić, M., et al. (2019). Phytoplankton diversity in adriatic ports: lessons from the port baseline survey for the management of harmful algal species. Mar. Pol. Bull. 147, 117–132. doi: 10.1016/j.marpolbul.2017.12.029
Mühlroth, A., Li, K., Røkke, G., Winge, P., Olsen, Y., Hohmann-Marriott, M. F., et al. (2013). Pathways of lipid metabolism in marine algae, co-expression network, bottlenecks and candidate genes for enhanced production of DHA and EPA in species of Chromista. Mar. Drugs 11, 4662–4697. doi: 10.3390/md11114662
Mühlroth, A., Winge, P., El Assimi, A., Jouhet, J., Maréchal, E., Hohmann-Marriott, M. F., et al. (2017). Mechanisms of phosphorus acquisition and lipid class remodeling under P limitation in a marine microalga. Plant Physiol. 175, 1543–1559. doi: 10.1104/pp.17.00621
Müller, W. E. G., Boreiko, A., Wang, X., Krasko, A., Geurtsen, W., Custódio, M. R., et al. (2007). Morphogenetic activity of silica and bio-silica on the expression of genes controlling biomineralization using SaOS-2 cells. Calcif. Tissue Int. 81, 382–393. doi: 10.1007/s00223-007-9075-4
Müller, W. E. G., Geurtsen, W. K., and Schröder, H. C. (2006). Biosilica-Adhesive Protein Nanocomposite Materials: Synthesis and Application in Dentistry. US Patent Application No. US60/839,601. Washington, DC: U.S. Patent and Trademark Office.
Müller, W. E. G., Grebenjuk, V. A., Le Pennec, G., Schröder, H. C., Brümmer, F., Hentschel, U., et al. (2004). Sustainable production of bioactive compounds by sponges - cell culture and gene cluster approach: a review. Mar. Biotechnol. 6, 105–117.
Müller, W. E. G., Wang, X., Cui, F. Z., Jochum, K. P., Tremel, W., Bill, J., et al. (2009). Sponge spicules as blueprints for the biofabrication of inorganic-organic composites and biomaterials. Appl. Microbiol. Biotechnol. 83, 397–413. doi: 10.1007/s00253-009-2014-8
Nakicenovic, N., Rockström, J., Gaffney, O., and Zimm, C. (2016). “Global commons in the anthropocene: world development on a stable and resilient planet,” in Proceedings of the IIASA Working Paper, (Laxenburg: IIASA).
Nam, S. J., Gaudêncio, S. P., Kauffman, C. A., Jensen, P. R., Kondratyuk, T. P., Marler, L. E., et al. (2010). Fijiolides A and B, inhibitors of TNF-α-induced NFκB activation, from a marine-derived sediment bacterium of the genus Nocardiopsis. J. Nat. Prod. 73, 1080–1086. doi: 10.1021/np100087c
Nelson, D. R., Mengistu, S., Ranum, P., Celio, G., Mashek, M., Mashek, D., et al. (2013). New lipid-producing, cold-tolerant yellow-green alga isolated from the rocky mountains of Colorado. Biotechnol. Prog. 29, 853–861. doi: 10.1002/btpr.1755
Nerva, L., Ciuffo, M., Vallino, M., Margaria, P., Varese, G. C., Gnavi, G., et al. (2016). Multiple approaches for the detection and characterization of viral and plasmid symbionts from a collection of marine fungi. Virus Res. 219, 22–38. doi: 10.1016/j.virusres.2015.10.028
Nerva, L., Chitarra, W., Siciliano, I., Gaiotti, F., Ciuffo, M., Forgia, M., et al. (2019a). Mycoviruses mediate mycotoxin regulation in Aspergillus ochraceus. Environ. Microbiol. 21, 1957–1968. doi: 10.1111/1462-2920.14436
Nerva, L., Forgia, M., Ciuffo, M., Chitarra, W., Chiapello, M., Vallino, M., et al. (2019b). The mycovirome of a fungal collection from the sea cucumber Holothuria polii. Virus Res. 273:197737. doi: 10.1016/j.virusres.2019.197737
Nerva, L., Silvestri, A., Ciuffo, M., Palmano, S., Varese, G. C., and Turina, M. (2017). Transmission of Penicillium aurantiogriseum partiti-like virus 1 to a new fungal host (Cryphonectria parasitica) confers higher resistance to salinity and reveals adaptive genomic changes. Environ. Microbiol. 19, 4480–4492. doi: 10.1111/1462-2920.13894
Newman, D. J., and Cragg, G. M. (2020). Natural products as sources of new drugs over the nearly four decades from 01/1981 to 09/2019. J. Nat. Prod. 83, 770–803. doi: 10.1021/acs.jnatprod.9b01285
Nichols, D., Cahoon, N., Trakhtenberg, E. M., Pham, L., Mehta, A., and Belanger, A. (2010). Use of ichip for high-throughput in situ cultivation of “uncultivable” microbial species. Appl. Environ. Microbiol. 76, 2445–2450. doi: 10.1128/aem.01754-09
Nicoletti, R., and Andolfi, A. (2018). “The marine-derived filamentous fungi in biotechnology,” in Grand Challenges in Marine Biotechnology, eds P. H. Rampelotto and A. Trincone (New York, NY: Springer), 157–189. doi: 10.1007/978-3-319-69075-9_4
Niedermeyer, T. H. J., Daily, A., Swiatecka-Hagenbruch, M., and Moscow, J. A. (2014). Selectivity and potency of microcystin congeners against OATP1B1 and OATP1B3 expressing cancer cells. PLoS One 9:e91476. doi: 10.1371/journal.pone.0091476
Nielsen, R., Nielsen, M., Abate, T. G., Hansen, B. W., Jepsen, P. M., Nielsen, S. L., et al. (2017). The importance of live-feed traps - farming marine fish species. Aquac. Res. 48, 2623–2641. doi: 10.1111/are.13281
Nikolaivits, E., Dimarogona, M., Fokialakis, N., and Topakas, E. (2017). Marine-derived biocatalysts: importance, accessing, and application in aromatic pollutant bioremediation. Front. Microbiol. 8:265. doi: 10.3389/fmicb.2017.00265
Nisticò, R. (2017). Aquatic-derived biomaterials for a sustainable future: a European opportunity. Resources 6:65. doi: 10.3390/resources6040065
Noda, J., Mühlroth, A., Bučinská, L., Dean, J., Bones, A. M., and Sobotka, R. (2016). Tools for biotechnological studies of the freshwater alga Nannochloropsis limnetica: antibiotic resistance and protoplast production. J. Appl. Phycol. 29, 853–863. doi: 10.1007/s10811-016-1001-6
Nordic Council of Ministers (2018). A Common Path: Iceland’s Presidency. Copenhagen: Nordic Council of Ministers.
Norvill, Z. N., Shilton, A., and Guieysse, B. (2016). Emerging contaminant degradation and removal in algal wastewater treatment ponds: identifying the research gaps. J. Hazard. Mater. 313, 291–309. doi: 10.1016/j.jhazmat.2016.03.085
Novoveska, L., Brown, M., and Liner, B. (2018). Rise and fall of the algae biofuel industry. World Water 41, 14–27.
Novoveská, L., Ross, M., Stanley, M., Pradelles, R., Wasiolek, V., and Sassi, J. F. (2019). Microalgal carotenoids: a review of production, current markets, regulations, and future direction. Mar. Drugs 17:640. doi: 10.3390/md17110640
Novoveská, L., Zapata, A. M., Zabolotney, J. B., Attwood, M. C., and Sundstrom, E. R. (2016). Optimizing polyculture cultivation and wastewater treatment in large-scale offshore photobioreactors. Algal Res. 18, 86–94. doi: 10.1016/j.algal.2016.05.033
Nudelman, R., Alhmoud, H., Delalat, B., Fleicher, S., Fine, E., Guliakhmedova, T., et al. (2019). Jellyfish-based smart wound dressing devices containing in situ synthesized antibacterial nanoparticles. Adv. Funct. Mater. 29:1902783. doi: 10.1002/adfm.201902783
Nymark, M., Sharma, A. K., Grønbech Hafskjold, M. C., Sparstad, T., Bones, A. M., and Winge, P. (2017). CRISPR/Cas9 gene editing in the marine diatom Phaeodactylum tricornutum. Biol. Protoc. 7:e2442. doi: 10.21769/BioProtoc.2442
Nymark, M., Sharma, A. K., Sparstad, T., Bones, A. M., and Winge, P. (2016). A CRISPR/Cas9 system adapted for gene editing in marine algae. Sci. Rep. 6:24951. doi: 10.1038/srep24951
Nymark, M., Volpe, C., Grønbech Hafskjold, M. C., Kirst, H., Serif, M., Vadstein, O., et al. (2019). Loss of ALBINO3b insertase results in a truncated light-harvesting antenna in diatoms. Plant Physiol. 181, 1257–1276. doi: 10.1104/pp.19.00868
Ogram, A., Sayler, G. S., and Barkay, T. (1987). The extraction and purification of microbial DNA from sediments. J. Microbiol. Methods 7, 57–66. doi: 10.1016/0167-7012(87)90025-x
Olivera, B. M., Gray, W. R., Zeikus, R., McIntosh, J. M., Varga, J., Rivier, J., et al. (1985). Peptide neurotoxins from fish-hunting cone snails. Science 230, 1338–1343. doi: 10.1126/science.4071055
Olsen, Y. (2011). Resources for fish feed in future mariculture. Aquac. Environ. Interact. 1, 187–200. doi: 10.3354/aei00019
Oppong-Danquah, E., Parrot, D., Blümel, M., Labes, A., and Tasdemir, D. (2018). Molecular networking-based metabolome and bioactivity analyses of marine-adapted fungi co-cultivated with phytopathogens. Front. Microbiol. 9:2072. doi: 10.3389/fmicb.2018.02072
Orlando-Bonaca, M., and Rotter, A. (2018). Any signs of replacement of canopy-forming algae by turf-forming algae in the northern Adriatic Sea? Ecol. Ind. 87, 272–284. doi: 10.1016/j.ecolind.2017.12.059
Ortega, A., Geraldi, N. R., Alam, I., Kamau, A. A., Acinas, S. G., Logares, R., et al. (2019). Important contribution of macroalgae to oceanic carbon sequestration. Nat. Geosci. 12, 748–754. doi: 10.1038/s41561-019-0421-8
Overlingė, D., Kataržytė, M., Vaičiūtė, D., Gyraite, G., Gečaitė, I., Jonikaitė, E., et al. (2020). Are there concerns regarding cHAB in coastal bathing waters affected by freshwater-brackish continuum? Mar. Pollut. Bull. 159:111500. doi: 10.1016/j.marpolbul.2020.111500
Owen, R., Macnaghten, P., and Stilgoe, J. (2012). Responsible research and innovation: from science in society to science for society, with society. Sci. Public Policy 39, 751–760. doi: 10.1093/scipol/scs093
Palaveniene, A., Harkavenko, V., Kharchenko, V., Daugela, P., Pranskunas, M., Juodzbalys, G., et al. (2018). Cuttlebone as a marine-derived material for preparing bone grafts. Mar. Biotechnol. 20, 363–374. doi: 10.1007/s10126-018-9816-6
Pan, R., Bai, X., Chen, J., Zhang, H., and Hong Wang, H. (2019). Exploring structural diversity of microbe secondary metabolites using OSMAC strategy: a literature review. Front. Microbiol. 10:294. doi: 10.3389/fmicb.2019.00294
Pang, K. L., Overy, D. P., Jones, E. B. G., da Luz Calado, M., Burgaud, G., Walker, A. K., et al. (2016). ‘Marine fungi’ and ‘marine-derived fungi’ in natural product chemistry research: toward a new consensual definition. Fungal Biol. Rev. 30, 163–175. doi: 10.1016/j.fbr.2016.08.001
Panno, L., Bruno, M., Voyron, S., Anastasi, A., Gnavi, G., Miserere, L., et al. (2013). Diversity, ecological role and potential biotechnological applications of marine fungi associated to the seagrass Posidonia oceanica. N. Biotechnol. 30, 685–694. doi: 10.1016/j.nbt.2013.01.010
Papazian, S., Parrot, D., Burýšková, B., Weinberger, F., and Tasdemir, D. (2019). Surface chemical defence of the eelgrass Zostera marina against microbial foulers. Sci. Rep. 9:3323. doi: 10.1038/s41598-019-39212-3
Parrot, D., Blümel, M., Utermann, C., Chianese, G., Krause, S., Kovalev, A., et al. (2019). Mapping the surface microbiome and metabolome of brown seaweed Fucus vesiculosus by amplicon sequencing, integrated metabolomics and imaging techniques. Sci. Rep. 9:1061. doi: 10.1038/s41598-018-37914-8
Parsons, R. J., Breitbart, M., Lomas, M. W., and Carlson, C. A. (2012). Ocean time-series reveals recurring seasonal patterns of virioplankton dynamics in the northwestern Sargasso Sea. ISME J. 6, 273–284. doi: 10.1038/ismej.2011.101
Pascelli, C., Laffy, P. W., Kupresanin, M., Ravasi, T., and Webster, N. S. (2018). Morphological characterization of virus-like particles in coral reef sponges. PeerJ 6:e5625. doi: 10.7717/peerj.5625
Pathirana, C., Jensen, P. R., and Fenical, W. (1992). Marinone and debromomarinone, antibiotic sesquiterpenoid napthoquinones of a new structure class from a marine bacterium. Tetrahedron. Lett. 33, 7663–7666. doi: 10.1016/0040-4039(93)88010-g
Paulsen, S. S., Strube, M. L., Bech, P. K., Gram, L., and Sonnenschein, E. C. (2019). Marine chitinolytic Pseudoalteromonas represents an untapped reservoir of bioactive potential. mSystems 4:e00060-19. doi: 10.1128/mSystems.00060-19
Pawelec, K. M., and Planell, J. A. (2019). Bone Repair Biomaterials, 2nd Edn. Cambridge, MA: Woodhead Publishing.
Pawlowski, J., Kahlert, M., Kelly-Quinn, M., Altermatt, F., Apothéloz-Perret-Gentil, L., Beja, P., et al. (2018). The future of biotic indices in the ecogenomic era: integrating eDNA metabarcoding in biological assessment of aquatic ecosystems. Sci. Total Environ. 63, 1295–1310.
Paz, G., Yudkovsky, Y., Shaish, L., Stern, N., Lubinevski, H., Mienis, H. K., et al. (2018). Initiating DNA barcoding of Eastern Mediterranean deep-sea biota. Mediterr. Mar. Sci. 1, 416–429.
Peng, F. Q., Ying, G. G., Yang, B., Liu, S., Lai, H. J., Liu, Y. S., et al. (2014). Biotransformation of progesterone and norgestrel by two freshwater microalgae (Scenedesmus obliquus and Chlorella pyrenoidosa): transformation kinetics and products identification. Chemosphere 95, 581–588. doi: 10.1016/j.chemosphere.2013.10.013
Peng, L., Lan, C. Q., and Zhang, Z. (2016). Cultivation of freshwater green alga Neochloris oleoabundans in non-sterile media co-inoculated with protozoa. The Canadian Journal of Chemical Engineering 94, 439–445. doi: 10.1002/cjce.22407
Pennesi, C., Rindi, F., Totti, C., and Beolchini, F. (2015). “Marine macrophytes: biosorbents,” in Springer Handbook of Marine Biotechnology, ed. S. K. Kim (Berlin: Springer), 597–610. doi: 10.1007/978-3-642-53971-8_24
Perale, G., and Hilborn, J. (2016). Bioresorbable Polymers for Biomedical Applications. Cambridge: Woodhead Publishing.
Perales-Vela, H. V., Pena-Castro, J. M., and Canizares-Villanueva, R. O. (2006). Heavy metal detoxification in eukaryotic microalgae. Chemosphere 64, 1–10. doi: 10.1016/j.chemosphere.2005.11.024
Perdicaris, S., Vlachogianni, T., and Valavanidis, A. (2013). Bioactive Natural substances from marine sponges: new developments and prospects for future pharmaceuticals. Nat. Prod. Chem. Res. 3:114. doi: 10.4172/2329-6836.1000114
Pereira, F., Almeida, J. R., Paulino, M., Grilo, I. R., Macedo, H., Cunha, I., et al. (2020). Antifouling napyradiomycins from marine-derived actinomycetes Streptomyces aculeolatus. Mar. Drugs 18:63. doi: 10.3390/md18010063
Pereira, F., Latino, D. A. R. S., and Gaudêncio, S. P. (2014). A chemoinformatics approach to the discovery of lead-like molecules from marine and microbial sources en route to antitumor and antibiotic drugs. Mar. Drugs 12, 757–778. doi: 10.3390/md12020757
Pereira, F., Latino, D. A. R. S., and Gaudêncio, S. P. (2015). QSAR-assisted virtual screening of lead-like molecules from marine and microbial natural sources for antitumor and antibiotic drug discovery. Molecules 20, 4848–4873. doi: 10.3390/molecules20034848
Pereira, R. C., and Costa-Lotufo, L. V. (2012). Bioprospecting for bioactives from seaweeds: potential, obstacles and alternatives. Rev. Bras. Farmacogn. Braz. J. Pharmacogn. 22, 894–905. doi: 10.1590/s0102-695x2012005000077
Pereira, S., Zille, A., Micheletti, E., Moradas-Ferreira, P., De Philippis, R., and Tamagnini, P. (2009). Complexity of cyanobacterial exopolysaccharides: composition, structures, inducing factors and putative genes involved in their biosynthesis and assembly. FEMS Microbiol. Rev. 33, 917–941. doi: 10.1111/j.1574-6976.2009.00183.x
Perez, E. A., Hillman, D. W., Fishkin, P. A., Krook, J. E., Tan, W. W., Kuriakose, A., et al. (2005). Phase II trial of dolastatin-10 in patients with advanced breast cancer. Invest. New Drugs 23, 257–261. doi: 10.1007/s10637-005-6735-y
Pérez-López, P., Ternon, E., González-García, S., Genta-Jouve, G., Feijoo, G., Thomas, O. P., et al. (2014). Environmental solutions for the sustainable production of bioactive natural products from the marine sponge Crambe crambe. Sci. Total Environ. 475, 71–82. doi: 10.1016/j.scitotenv.2013.12.068
Pérez-Rama, M., Alonso, J. A., López, C. H., and Vaamonde, E. T. (2002). Cadmium removal by living cells of the marine microalga Tetraselmis suecica. Bioresour. Technol. 84, 265–270. doi: 10.1016/s0960-8524(02)00045-7
Pérez-Victoria, I., Martín, J., and Reyes, F. (2016). Combined LC/UV/MS and NMR strategies for the dereplication of marine natural products. Planta Med. 82, 857–871. doi: 10.1055/s-0042-101763
Petersen, L. E., Marner, M., Labes, A., and Tasdemir, D. (2019). Rapid metabolome and bioactivity profiling of fungi associated with the leaf and rhizosphere of the Baltic seagrass Zostera marina. Mar. Drugs 17:419. doi: 10.3390/md17070419
Petrie, B., Barden, R., and Kasprzyk-Hordern, B. (2015). A review on emerging contaminants in wastewaters and the environment: current knowledge, understudied areas and recommendations for future monitoring. Water Res. 72, 3–27. doi: 10.1016/j.watres.2014.08.053
Pettit, R. K. (2011). Small-molecule elicitation of microbial secondary metabolites. Microb. Biotechnol. 4, 471–478. doi: 10.1111/j.1751-7915.2010.00196.x
Philis, G., Gracey, E. O., Gansel, L. C., Fet, A. M., and Rebours, C. (2018). Comparing the primary energy and phosphorus consumption of soybean and seaweed-based aquafeed proteins – a material and substance flow analysis. J. Clean. Prod. 200, 1142–1153. doi: 10.1016/j.jclepro.2018.07.247
Pile, A. J. (1999). Resource partitioning by Caribbean coral reef sponges: is there enough food for everyone? Mem. Queensl. Mus. 44, 457–461.
Pitocchi, R., Cicatiello, P., Birolo, L., Piscitelli, A., Bovio, E., Varese, G. C., et al. (2020). Cerato-platanins from marine fungi as effective protein biosurfactants and bioemulsifiers. Int. J. Mol. Sci. 21:2913. doi: 10.3390/ijms21082913
Pizzetti, I., Gobet, A., Fuchs, B. M., Amman, R., and Fazi, S. (2011). Abundance and diversity of Planctomycetes in a Tyrrhenian coastal system of central Italy. Aquat. Microb. Ecol. 65, 129–141. doi: 10.3354/ame01535
Pletikapić, G., Berquand, A., Mišić Radić, T., and Svetličić, V. (2012). Quantitative nanomechanical mapping of marine diatom in seawater by peak force tapping atomic force microscopy. J. Phycol. 48, 174–185. doi: 10.1111/j.1529-8817.2011.01093.x
Poli, A., Bovio, E., Ranieri, L., Varese, G. C., and Prigione, V. (2020). News from the sea: a new genus and seven new species in the Pleosporalean families Roussoellaceae and Thyridariaceae. Diversity 12:144. doi: 10.3390/d12040144
Poli, A., Finore, I., Romano, I., Gioiello, A., Lama, L., and Nicolaus, B. (2017). Microbial diversity in extreme marine habitats and their biomolecules. Microorganisms 5:25. doi: 10.3390/microorganisms5020025
Poli, A., Vizzini, A., Prigione, V., and Varese, G. C. (2018). Basidiomycota isolated from the Mediterranean Sea–Phylogeny and putative ecological roles. Fungal Ecol. 36, 51–62. doi: 10.1016/j.funeco.2018.09.002
Polymenakou, P., Mandalakis, M., Dailianis, T., Dimitriadis, C., Medvecky, M., Magoulas, A., et al. (2018). Preliminary assessment of methanogenic microbial communities in marine caves of Zakynthos Island (Ionian Sea, Greece). Mediterr. Mar. Sci. 19, 284–289. doi: 10.12681/mms.14374
Polymenakou, P. N., Christakis, C. A., Mandalakis, M., and Oulas, A. (2015). Pyrosequencing analysis of microbial communities reveals dominant cosmopolitan phylotypes in deep-sea sediments of the eastern Mediterranean Sea. Res Microbiol. 166, 448–457. doi: 10.1016/j.resmic.2015.03.005
Polymenakou, P. N., Lampadariou, N., Mandalakis, M., and Tselepides, A. (2009). Phylogenetic diversity of sediment bacteria from the southern Cretan margin, Eastern Mediterranean Sea. Syst. Appl. Microbiol. 32, 17–26. doi: 10.1016/j.syapm.2008.09.006
Popko, M., Michalak, I., Wilk, R., Gramza, M., Chojnacka, K., and Górecki, H. (2018). Effect of the new plant growth biostimulants based on amino acids on yield and grain quality of winter wheat. Molecules 23:470. doi: 10.3390/molecules23020470
Porter, D. (1990). “Phylum Labyrinthulomycota,” in Handbook of Protoctista, eds L. Margulis, J. O. Corliss, M. Melkonian, and D. J. Chapman (Boston: Jones and Bartlett), 388–398.
Portman, M. E. (2016). Environmental Planning for Oceans and Coasts: Methods, Tools, Technologies. Switzerland: Springer.
Posocco, B., Dreussi, E., de Santa, J., Toffoli, G., Abrami, M., Musiani, F., et al. (2015). Polysaccharides for the delivery of antitumor drugs. Materials 8, 2569–2615. doi: 10.3390/ma8052569
Potts, B. C., Albitar, M. X., Anderson, K. C., Baritaki, S., Berkers, C., Bonavida, B., et al. (2011). Marizomib, a proteasome inhibitor for all seasons: preclinical profile and a framework for clinical trials. Curr. Cancer Drug Targets 11, 254–284. doi: 10.2174/156800911794519716
Prabha, S. P., Nagappan, S., Rathna, R., Viveka, R., and Nakkeeran, E. (2020). “21 - Blue biotechnology: a vision for future marine biorefineries,” in Refining Biomass Residues for Sustainable Energy and Bioproducts, eds R. P. Kumar, E. Gnansounou, J. K. Raman, and G. Baskar (Cambridge, MA: Academic Press), 463–480. doi: 10.1016/b978-0-12-818996-2.00021-1
Prieto-Davó, A., Dias, T., Gomes, S. E., Rodrigues, S., Parera-Valadezl, Y., Borralho, P. M., et al. (2016). The Madeira Archipelago as a significant source of marine-derived actinomycete diversity with anticancer and antimicrobial potential. Front. Microbiol. 7:1594. doi: 10.3389/fmicb.2016.01594
Proksch, P., Edrada, R. A., and Ebel, R. (2002). Drugs from the seas - current status and microbiological implications. Appl. Microbiol. Biotechnol. 59, 125–134. doi: 10.1007/s00253-002-1006-8
Pushpakom, S., Iorio, F., Eyers, P. A., Escott, K. J., Hopper, S., Wells, A., et al. (2019). Drug repurposing: progress, challenges and recommendations. Nat. Rev. Drug Discov. 18, 41–58. doi: 10.1038/nrd.2018.168
Qi, S. H., and Ma, X. (2017). Antifouling compounds from marine invertebrates. Mar. Drugs 15:263. doi: 10.3390/md15090263
Querellou, J. (2010). Marine biotechnology: a new vision and strategy for Europe. Mar. Board ESF Position Paper 15, 64–68.
Quinn, J. C., and Davis, R. (2015). The potentials and challenges of algae based biofuels: a review of the techno-economic, life cycle, and resource assessment modeling. Bioresour. Technol. 184, 2444–2452.
Rabinowitz, C., Douek, J., Weisz, E., Shabtay, A., and Rinkevich, B. (2006). Isolation and characterization of four novel thraustochytrid strains from a colonial tunicate. Indian J. Mar. Sci. 35, 341–350.
Rabone, M., Harden-Davies, H., Collins, J. E., Zajderman, S., Appeltans, W., Droege, G., et al. (2019). Access to marine genetic resources (MGR): raising awareness of best-practice through a new agreement for Biodiversity Beyond National Jurisdiction (BBNJ). Front. Mar. Sci. 6:520. doi: 10.3389/fmars.2019.00520
Radziemska, M., Vaverková, M. D., Adamcová, D., Brtnický, M., and Mazur, Z. (2019). Valorization of fish waste compost as a fertilizer for agricultural use. Waste Biomass Valori. 10, 2537–2545. doi: 10.1007/s12649-018-0288-8
Raghukumar, S. (2002). Ecology of the marine protists, the Labyrinthulomycetes (Thraustochytrids and Labyrinthulids). Eur. J. Protistol. 38, 127–145. doi: 10.1078/0932-4739-00832
Rajasekar, T., Balaji, S., Kumaran, S., Deivasigamani, B., and Pugzhavendhan, S. R. (2012). Isolation and characterization of marine fungal metabolites against clinical pathogens. Asian Pac. J. Trop. Dis. 2, S387–S392.
Rämä, T., Hassett, B. T., and Bubnova, E. (2017). Arctic marine fungi: from filaments and flagella to operational taxonomic units and beyond. Bot. Mar. 60, 433–452.
Rath, B. (2012). Microalgal bioremediation: current practices and perspectives. J. Biochem. Technol. 3, 299–304.
Rayner, R., Jolly, C., and Gouldman, C. (2019). Ocean observing and the blue economy. Front. Mar. Sci. 6:330. doi: 10.3389/fmars.2019.00330
Reen, F. J., Romano, S., Dobson, A. D. W., and O’Gara, F. (2015). The Sound of silence: activating silent biosynthetic gene clusters in marine microorganisms. Mar. Drugs 13, 4754–4783. doi: 10.3390/md13084754
Rego, D., Redondo, L. M., Geraldes, V., Costa, L., Navalho, J., and Pereira, M. T. (2015). Control of predators in industrial scale microalgae cultures with pulsed electric fields. Bioelectrochemistry 103, 60–64. doi: 10.1016/j.bioelechem.2014.08.004
Reich, M., and Labes, A. (2017). How to boost marine fungal research: a first step towards a multidisciplinary approach by combining molecular fungal ecology and natural products chemistry. Mar. Genomics 36, 57–75. doi: 10.1016/j.margen.2017.09.007
Renner, M. K., Shen, Y. C., Cheng, X. C., Jensen, P. R., Frankmoelle, W., Kauffman, C. A., et al. (1999). Cyclomarins A-C, new antiinflammatory cyclic peptides produced by a marine bacterium (Streptomyces sp.). J. Amer. Chem. Soc. 121, 11273–11276. doi: 10.1021/ja992482o
Reyes-Garcés, N., Gionfriddo, E., Gómez-Ríos, G. A., Alam, M. N., Boyacl, E., Bojko, B., et al. (2018). Advances in solid phase microextraction and perspective on future directions. Anal. Chem. 90, 302–360. doi: 10.1021/acs.analchem.7b04502
Rinehart, K. L., Holt, T. G., Fregeau, N. L., Stroh, J. G., Keifer, P. A., Sun, F., et al. (1990). Ecteinascidins 729, 743, 745, 759A, 759B, and 770: potent antitumor agents from the Caribbean tunicate Ecteinascidia turbinata. J. Org. Chem. 55, 4512–4515. doi: 10.1021/jo00302a007
Rinkevich, B. (1999). Cell cultures from marine invertebrates: obstacles, new approaches and recent improvements. J. Biotechnol. 70, 133–153. doi: 10.1016/s0168-1656(99)00067-x
Rinkevich, B. (2005). Marine invertebrate cell culture: new millennium trends. Mar. Biotechnol. 7, 429–439. doi: 10.1007/s10126-004-0108-y
Rinkevich, B. (2011). Cell cultures from marine invertebrates: new insights for capturing endless stemness. Mar. Biotechnol. 13, 345–354. doi: 10.1007/s10126-010-9354-3
Rinkevich, B., and Rabinowitz, C. (1993). In vitro culture of blood cells from the colonial protochordate Botryllus schlosseri. In Vitro Cell. Dev. Biol. 29A, 79–85. doi: 10.1007/bf02634375
Rizwan, M., Mujtaba, G., Memon, S. A., Lee, K., and Rashid, N. (2018). Exploring the potential of microalgae for new biotechnology applications and beyond: a review. Renew. Sust. Energ. Rev. 92, 394–404. doi: 10.1016/j.rser.2018.04.034
Roca, C., Lehmann, M., Torres, C. A. V., Baptista, S., Gaudencio, S. P., Freitas, F., et al. (2016). Exopolysaccharide production by a marine Pseudoalteromonas sp. strain isolated from Madeira Archipelago ocean sediments. New Biotechnol. 33, 460–466. doi: 10.1016/j.nbt.2016.02.005
Rocha, J., Peixe, L., Gomes, N. C. M., and Calado, R. (2011). Cnidarians as a source of new marine bioactive compounds—an overview of the last decade and future steps for bioprospecting. Mar. Drugs 9, 1860–1886. doi: 10.3390/md9101860
Roman, D. L., Roman, M., Som, C., Schmutz, M., Hernandez, E., Wick, P., et al. (2019). Computational assessment of the pharmacological profiles of degradation products of chitosan. Front. Bioeng. Biotechnol. 7:214. doi: 10.3389/fbioe.2019.00214
Romano, S., Jackson, S. A., Patry, S., and Dobson, A. D. W. (2018). Extending the “One Strain Many Compounds” (OSMAC) principle to marine microorganisms. Mar. Drugs 16:244. doi: 10.3390/md16070244
Ronga, D., Biazzi, E., Parati, K., Carminati, D., Carminati, E., and Tava, A. (2019). Microalgal biostimulants and biofertilisers in crop productions. Agronomy 9:192. doi: 10.3390/agronomy9040192
Roque, B. M., Brooke, C. G., Ladau, J., Polley, T., Marsh, L. J., Najafi, N., et al. (2019). Effect of the macroalgae Asparagopsis taxiformis on methane production and rumen microbiome assemblage. Anim. Microb. 1:3. doi: 10.1186/s42523-019-0004-4
Rosano, G. L., and Ceccarelli, E. A. (2014). Recombinant protein expression in Escherichia coli: advances and challenges. Front. Microbiol. 5:172. doi: 10.3389/fmicb.2014.00172
Rotter, A., Bacu, A., Barbier, M., Bertoni, F., Bones, A. M., Cancela, M. L., et al. (2020a). A new network for the advancement of marine biotechnology in Europe and beyond. Front. Mar. Sci. 7:278. doi: 10.3389/fmars.2020.00278
Rotter, A., Klun, K., Francé, J., Mozetič, P., and Orlando-Bonaca, M. (2020b). Non-indigenous species in the Mediterranean Sea: turning from pest to source by developing the 8Rs model, a new paradigm in pollution mitigation. Front. Mar. Sci. 7:178. doi: 10.3389/fmars.2020.00178
Roy, D., Greenlaw, P. N., and Shane, B. S. (1993). Adsorption of heavy metals by green algae and ground rice hulls. J. Environ. Sci. Health A 28, 37–50. doi: 10.1080/10934529309375861
Rozzi, R., Stuart Chapin, F. I. I. I., Baird Callicott, J., Pickett, S. T. A., Power, M. E., Armesto, J. J., et al. (2015). “Introduction: linking ecology and ethics for an interregional and intercultural earth stewardship,” in Earth Stewardship, eds R. Rozzi, F. S. Chapin, III J. B. Callicott, S. Pickett, M. E. Power, J. J. Armesto, et al. (Switzerland: Springer), 1–14. doi: 10.1007/978-3-319-12133-8_1
Rust, M., Helfrich, E. J. N., Freeman, M. F., Nanudorn, P., Field, C. M., Rückertd, C., et al. (2020). A multiproducer microbiome generates chemical diversity in the marine sponge Mycale hentscheli. PNAS 117, 9508–9518. doi: 10.1073/pnas.1919245117
Rustad, T., Storrø, I., and Slizyte, R. (2011). Possibilities for the utilisation of marine by-products. Int. J. Food Sci. Technol. 46, 2001–2014. doi: 10.1111/j.1365-2621.2011.02736.x
Sachindra, N. M., Sato, E., Maeda, H., Hosokawa, M., Niwano, Y., Kohno, M., et al. (2007). Radical scavenging and singlet oxygen quenching activity of marine carotenoid fucoxanthin and its metabolites. J. Agric. Food Chem. 55, 8516–8522. doi: 10.1021/jf071848a
Safarik, I., Ashoura, N., Maderova, Z., Pospiskova, K., Baldikova, E., and Safarikova, M. (2016). Magnetically modified Posidonia oceanica biomass as an adsorbent for organic dyes removal. Mediterr. Mar. Sci. 17, 351–358. doi: 10.12681/mms.1549
Safarik, I., Horska, K., Pospiskova, K., and Safarikova, M. (2012). Magnetic techniques for the detection and determination of xenobiotics and cells in water. Anal. Bioanal. Chem. 404, 1257–1273. doi: 10.1007/s00216-012-6056-x
Safarik, I., Prochazkova, J., Baldikova, E., and Pospiskova, K. (2020). “Magnetically responsive algae and seagrass derivatives for pollutant removal,” in Wastes: Solutions, Treatments and Opportunities III, eds C. Vilarinho, F. Castro, M. Goncalves, and A. L. Fernando (Boca Raton, FL: CRC Press), 131–136. doi: 10.1201/9780429289798-21
Safarik, I., and Safarikova, M. (2004). Magnetic techniques for the isolation and purification of proteins and peptides. BioMagn. Res. Technol. 2:7. doi: 10.1186/1477-044X-2-7
Safarikova, M., and Safarik, I. (1999). Magnetic solid-phase extraction. J. Magn. Magn. Mater. 194, 108–112.
Salazar, R., Cortés-Funes, H., Casado, E., Pardo, B., López-Martín, A., Cuadra, C., et al. (2013). Phase I study of weekly kahalalide F as prolonged infusion in patients with advanced solid tumors. Cancer Chemother. Pharmacol. 72, 75–83. doi: 10.1007/s00280-013-2170-5
Salehiziri, M., Amalfitano, S., Gallipoli, A., Gianico, A., Rad, H. A., Braguglia, C. M., et al. (2020). Investigating the influences of quorum quenching and nutrient conditions on activated sludge flocs at a short-time scale. Chemosphere 248:125917. doi: 10.1016/j.chemosphere.2020.125917
Sana, B. (2015). “Marine microbial enzymes: current status and future prospects,” in Springer Handbook of Marine Biotechnology, ed. S. K. Kim (Berlin: Springer), 905–917. doi: 10.1007/978-3-642-53971-8_38
Satpute, S. K., Banat, I. M., Dhakephalkar, P. K., Banpurkar, A. G., and Chopade, B. A. (2010). Biosurfactants, bioemulsifiers and exopolysaccharides from marine microorganisms. Biotechnol. Adv. 28, 436–450. doi: 10.1016/j.biotechadv.2010.02.006
Sayre, R. (2010). Microalgae: the potential for carbon capture. Bioscience 60, 722–727. doi: 10.1525/bio.2010.60.9.9
Schenk, P. M., Thomas-Hall, S. R., Stephens, E., Marx, U. C., Mussgnug, J. H., Posten, C., et al. (2008). Second generation biofuels: high-efficiency microalgae for biodiesel production. Bioenergy Res. 1, 20–43. doi: 10.1007/s12155-008-9008-8
Schröder, H. C., Brandt, D., Schloßmacher, U., Wang, X., Tahir, M. N., Tremel, W., et al. (2007). Enzymatic production of biosilica glass using enzymes from sponges: basic aspects and application in nanobiotechnology (material sciences and medicine). Naturwissenschaften 94, 339–359. doi: 10.1007/s00114-006-0192-0
Schröder, H. C., Brümmer, F., Fattorusso, E., Aiello, A., Menna, M., de Rosa, S., et al. (2003). Sustainable production of bioactive compounds from sponges: primmorphs as bioreactors. Prog. Mol. Subcell. Biol. 37, 163–197. doi: 10.1007/978-3-642-55519-0_7
Schröder, H. C., Schloßmacher, U., Boreiko, A., Natalio, F., Baranowska, M., Brandt, D., et al. (2009). “Silicatein: nanobiotechnological and biomedical applications,” in Biosilica in Evolution, Morphogenesis, and Nanobiotechnology. Progress in Molecular and Subcellular Biology, Vol. 47, eds W. E. G. Müller and M. A. Grachev (Berlin: Springer), 252–273.
Schultz, M. P. (2007). Effects of coating roughness and biofouling on ship resistance and powering. Biofouling 23, 331–341. doi: 10.1080/08927010701461974
Şeker, A., Shahwan, T., Eroǧlu, A. E., Yılmaz, S., Demirel, Z., and Dalay, M. C. (2008). Equilibrium, thermodynamic and kinetic studies for the biosorption of aqueous lead (II), cadmium (II) and nickel (II) ions on Spirulina platensis. J. Hazard. Mater. 154, 973–980. doi: 10.1016/j.jhazmat.2007.11.007
Selman, M., Dankar, S. K., Forbes, N. E., Jia, J. J., and Brown, E. G. (2012). Adaptive mutation in influenza A virus non-structural gene is linked to host switching and induces a novel protein by alternative splicing. Emerg. Microbes Infect. 1:e42. doi: 10.1038/emi.2012.38
Senevirathne, M., and Kim, S. K. (2012). Development of bioactive peptides from fish proteins and their health promoting ability. Adv. Food Nutr. Res. 65, 235–248. doi: 10.1016/b978-0-12-416003-3.00015-9
Serif, M., Dubois, G., Finoux, A. L., Teste, M. A., Jallet, D., and Daboussi, F. (2018). One-step generation of multiple gene knock-outs in the diatom Phaeodactylum tricornutum by DNA-free genome editing. Nat. Commun. 9:3924. doi: 10.1038/s41467-018-06378-9
Seymour, M. (2019). Rapid progression and future of environmental DNA research. Commun. Biol. 2:80. doi: 10.1038/s42003-019-0330-9
Shah, M. M. R., Liang, Y., Cheng, J. J., and Daroch, M. (2016). Astaxanthin-producing green microalga Haematococcus pluvialis: from single cell to high value commercial products. Front. Plant Sci. 7:531. doi: 10.3389/fpls.2016.00531
Shalaby, M., Agwa, M., Saeed, H., Khedr, S. M., Morsy, O., and El-Demellawy, M. A. (2019). Fish scale collagen preparation, characterization and its application in wound healing. J. Polym. Environ. 28, 166–178. doi: 10.1007/s10924-019-01594-w
Shankar, S., Wang, L. F., and Rhim, J. W. (2019). Effect of melanin nanoparticles on the mechanical, water vapor barrier, and antioxidant properties of gelatin-based films for food packaging application. Food Packag. Shelf Life 21:100363. doi: 10.1016/j.fpsl.2019.100363
Sharma, A. K., Mühlroth, A., Jouhet, J., Maréchal, E., Alipanah, L., Kissen, R., et al. (2020). The Myb-like transcription factor phosphorus starvation response (PtPSR) controls conditional P acquisition and remodeling in marine microalgae. New Phytol. 225, 2380–2395. doi: 10.1111/nph.16248
Sharma, A. K., Nymark, M., Sparstad, T., Bones, A. M., and Winge, P. (2018). Transgene-free genome editing in marine algae by bacterial conjugation–comparison with biolistic CRISPR/Cas9 transformation. Sci. Rep. 8:14401. doi: 10.1038/s41598-018-32342-0
Sharma, H. S. S., Fleming, C., Selby, C., Rao, J. R., and Martin, T. (2014). Plant biostimulants: a review on the processing of macroalgae and use of extracts for crop management to reduce abiotic and biotic stresses. J. Appl. Phycol. 26, 465–490. doi: 10.1007/s10811-013-0101-9
Shi, Y., Tyson, G. W., and DeLong, E. F. (2009). Metatranscriptomics reveals unique microbial small RNAs in the oceans’s water column. Nature 459, 266–269. doi: 10.1038/nature08055
Shilabin, A. G., and Hamann, M. T. (2011). In vitro and in vivo evaluation of select kahalalide F analogs with antitumor and antifungal activities. Bioorgan. Med. Chem. 19, 6628–6632. doi: 10.1016/j.bmc.2011.06.050
Shirodkar, P. V., Muraleedharan, U. D., and Raghukumar, S. (2017). Production of extracellular polysaccharases by the marine protists, Thraustochytrids, with special reference to alpha-amylase activity. Int. J. Pharma Bio Sci. 8, 453–462.
Shokralla, S., Spall, J. L., Gibson, J. F., and Hajibabaei, M. (2012). Next-generation sequencing technologies for environmental DNA research. Mol. Ecol. 21, 1794–1805. doi: 10.1111/j.1365-294x.2012.05538.x
Silber, J., Kramer, A., Labes, A., and Tasdemir, D. (2016). From discovery to production: biotechnology of marine fungi for the production of new antibiotics. Mar. Drugs 14:137. doi: 10.3390/md14070137
Silva, T., Salomon, P. S., Hamerski, L., Walter, J., Menezes, R. B., Siqueira, J. E., et al. (2018). Inhibitory effect of microalgae and cyanobacteria extracts on influenza virus replication and neuraminidase activity. PeerJ 6:e5716. doi: 10.7717/peerj.5716
Simister, R. L., Poutasse, C. M., Thurston, A. M., Reeve, J. L., Baker, M. C., and White, H. K. (2015). Degradation of oil by fungi isolated from Gulf of Mexico beaches. Mar. Pollut. Bull. 100, 327–333. doi: 10.1016/j.marpolbul.2015.08.029
Simon, J.-C., Marchesi, J. C., Mougel, C., and Selosse, M. A. (2019). Host-microbiota interactions: from holobiont theory to analysis. Microbiome 7:5. doi: 10.1186/s40168-019-0619-4
Singh, J., and Dhar, D. W. (2019). Overview of carbon capture technology: microalgal biorefinery concept and state-of-the-art. Front. Mar. Sci. 6:29. doi: 10.3389/fmars.2019.00029
Singh, N., Chaput, L., and Villoutreix, B. O. (2020). Virtual screening web servers: designing chemical probes and drug candidates in the cyberspace. Brief Bioinform. 18:bbaa034. doi: 10.1093/bib/bbaa034
Singh, N. K., and Dhar, D. W. (2011). Microalgae as second generation biofuel. A review. Agron. Sustain. Dev. 31, 605–629. doi: 10.1007/s13593-011-0018-0
Singh, S., Dhanjal, D. S., Bhardwaj, S., Thotapalli, S., Kumar, V., Datta, S., et al. (2020). An insight in bacteriophage based biosensors with focus on their detection methods and recent advancements. Environ. Technol. Innov. 20:101081. doi: 10.1016/j.eti.2020.101081
Sipkema, D., Osinga, R., Schatton, W., Mendola, D., Tramper, J., and Wijffels, R. H. (2005). Large-scale production of pharmaceuticals by marine sponges: sea, cell, or synthesis? Biotechnol. Bioeng. 90, 201–222. doi: 10.1002/bit.20404
Skjånes, K., Knutsen, G., Källqvist, T., and Lindblad, P. (2008). H2 production from marine and freshwater species of green algae during sulfur starvation and considerations for bioreactor design. Int. J. Hydrogen Energy 33, 511–521. doi: 10.1016/j.ijhydene.2007.09.040
Skjånes, K., Rebours, C., and Lindblad, P. (2013). Potential for green microalgae to produce hydrogen, pharmaceuticals and other high value products in a combined process. Crit. Rev. Biotechnol. 33, 172–215. doi: 10.3109/07388551.2012.681625
Slattery, S. S., Diamond, A., Wang, H., Therrien, J. A., Lant, J. T., Jazey, T., et al. (2018). An expanded plasmid-based genetic toolbox enables Cas9 genome editing and stable maintenance of synthetic pathways in Phaeodactylum tricornutum. ACS Synth. Biol. 7, 328–338. doi: 10.1021/acssynbio.7b00191
Sluijs, I., Cadier, E., Beulens, J. W., van der, A. D. L., and Spijkerman, A. M. (2015). Dietary intake of carotenoids and risk of type 2 diabetes. Nutr. Metab. Cardiovasc. Dis. 25, 376–381.
Smerilli, A., Orefice, I., Corato, F., Gavalás Olea, A., Ruban, A. V., and Brunet, C. (2017). Photoprotective and antioxidant responses to light spectrum and intensity variations in the coastal diatom Skeletonema marinoi. Environ. Microbiol. 19, 611–627. doi: 10.1111/1462-2920.13545
Smiline Girija, A. S., Hariprasad, G., Vijayashree Priyadharsini, J., Pandi Suba, K., Raghuraman, R., and Gnanavendhan, S. G. (2008). Antimicrobial potential of Loligo duvauceli ink against the common clinical bacterial and yeast isolates. Biomedicine 28, 213–215.
Smythe, T. C., and McCann, J. (2018). Lessons learned in marine governance: case studies of marine spatial planning practice in the U.S. Mar. Policy 94, 227–237. doi: 10.1016/j.marpol.2018.04.019
Soliev, A. B., Hosokawa, K., and Enomoto, K. (2011). Bioactive pigments from marine bacteria: applications and physiological roles. Evid. Based Complem. Altern. Med. 2011:670349. doi: 10.1155/2011/670349
Son, M., Yu, J., and Kim, K. H. (2015). Five questions about mycoviruses. PLoS Pathog. 11:e1005172. doi: 10.1371/journal.ppat.1005172
Souza de Oliveira, L., Bueno Gregoracci, G., Gueiros Zacarias Silva, G., Tavares Salgado, L., Amado Filho, G., Alves-Ferreira, M., et al. (2012). Transcriptomic analysis of the red seaweed Laurencia dendroidea (Florideophyceae, Rhodophyta) and its microbiome. BMC Genomics 13:487. doi: 10.1186/1471-2164-13-487
Spoof, L., and Catherine, A. (2017). “Tables of microcystins and nodularin,” in Handbook of cyanobacteria Monitoring and Cyanotoxin Analysis, First Edition, eds J. Meriluoto, L. Spoof, and G. A. Codd (Hoboken, NY: Wiley), 526–537.
Stabili, L., Licciano, M., Longo, C., Corriero, G., and Mercurio, M. (2008). Evaluation of microbiological accumulation capability of the commercial sponge Spongia officinalis var. adriatica (Schmidt) (Porifera, Demospongiae). Water Res. 42, 2499–2506. doi: 10.1016/j.watres.2008.02.008
Starling-Windhof, A., Srinivasan, A., Tomeo, T., Clark, A. M., and Mattei, P. (2020). “Trends in the intellectual property (IP) landscape of drug delivery systems: 30 years of growth and evolution,” in Nanopharmaceuticals Volume 1: Expectations and Realities of Multifunctional Drug Delivery, ed. R. Shegokar (Amsterdam: Elsevier), 201–230. doi: 10.1016/b978-0-12-817778-5.00010-5
Stead, P., Hiscox, S., Robinson, P. S., Pike, N. B., Sidebottom, P. J., Roberts, A. D., et al. (2000). Eryloside F, a novel penasterol disaccharide possessing potent thrombin receptor antagonist activity. Bioorg. Med. Chem. Lett. 10, 661–664. doi: 10.1016/s0960-894x(00)00063-9
Steinert, G., Stauffer, C. H., Aas-Valleriani, N., Borchert, E., Bhushan, A., Campbell, A., et al. (2018). “BluePharmTrain: biology and biotechnology of marine sponges,” in Grand Challenges in Marine Biotechnology, eds P. Rampelotto and A. Trincone (Cham: Springer), 505–553. doi: 10.1007/978-3-319-69075-9_13
Sterling, T., and Irwin, J. J. (2015). ZINC 15 – ligand discovery for everyone. J. Chem. Inf. Model. 55, 2324–2337. doi: 10.1021/acs.jcim.5b00559
Stévant, P., Rebours, C., and Chapman, A. (2017). Seaweed aquaculture in Norway: recent industrial developments and future perspectives. Aquac. Int. 25, 1373–1390. doi: 10.1007/s10499-017-0120-7
Stincone, P., and Brandelli, A. (2020). Marine bacteria as source of antimicrobial compounds. Crit. Rev. Biotechnol. 40, 306–319. doi: 10.1080/07388551.2019.1710457
Stowe, S. D., Richards, J. J., Tucker, A. T., Thompson, R., Melander, C., and Cavanagh, J. (2011). Anti-biofilm compounds derived from marine sponges. Mar. Drugs 9, 2010–2035. doi: 10.3390/md9102010
Straub, C. T., Counts, J. A., Nguyen, D. M. N., Wu, C. H., Zeldes, B. M., Crosby, J. R., et al. (2018). Biotechnology of extremely thermophilic archaea. FEMS Microbiol. Rev. 42, 543–578.
Strömstedt, A. A., Felth, J., and Bohlin, L. (2014). Bioassays in natural product research - strategies and methods in the search for anti-inflammatory and antimicrobial activity. Phytochem. Anal. 25, 13–28. doi: 10.1002/pca.2468
Subramani, R., and Aalbersberg, W. (2013). Culturable rare Actinomycetes: diversity, isolation and marine natural product discovery. Appl. Microbiol. Biotechnol. 97, 9291–9321. doi: 10.1007/s00253-013-5229-7
Subramani, R., and Sipkema, D. (2019). Marine rare Actinomycetes: a promising source of structurally diverse and unique novel natural products. Mar. Drugs 17, 249. doi: 10.3390/md17050249
Sudha, P. N., Aisverya, S., Nithya, R., and Vijayalakshmi, K. (2014). Industrial applications of marine carbohydrates. Adv. Food Nutr. Res. 73, 145–181. doi: 10.1016/b978-0-12-800268-1.00008-1
Suganya, T., Varman, M., Masjuki, H. H., and Renganathan, S. (2016). Macroalgae and microalgae as a potential source for commercial applications along with biofuels production: a biorefinery approach. Renew. Sust. Energ. Rev. 55, 909–941. doi: 10.1016/j.rser.2015.11.026
Suleria, H. A. R., Masci, P., Gobe, G., and Osborne, S. (2016). Current and potential uses of bioactive molecules from marine processing waste. J. Sci. Food Agric. 96, 1064–1067. doi: 10.1002/jsfa.7444
Sun, P., Cheng, K. W., He, Y., Liu, B., Mao, X., and Chen, F. (2018). Screening and identification of inhibitors of advanced glycation endproduct formation from microalgal extracts. Food Funct. 9, 1683–1691. doi: 10.1039/c7fo01840a
Sunagawa, S., Coelho, L. P., Chaffron, S., Kultima, J. R., Labadie, K., Salazar, G., et al. (2015). Structure and function of the global ocean microbiome. Science 348:6237. doi: 10.1126/science.1261359
Sundbæk, K. B., Würtzner Koch, I. D., Villaro, C. G., Rasmussen, N. S., Holdt, S. L., and Hartmann, N. B. (2018). Sorption of fluorescent polystyrene microplastic particles to edible seaweed Fucus vesiculosus. J. Appl. Phycol. 30, 2923–2927. doi: 10.1007/s10811-018-1472-8
Surget, G., Roberto, V. P., Le Lann, K., Mira, S., Guérard, F., Laizé, V., et al. (2017). Marine green macroalgae: a source of natural compounds with mineralogenic and antioxidant activities. J. Appl. Phycol. 29, 575–584. doi: 10.1007/s10811-016-0968-3
Sutherland, D. L., and Ralph, P. J. (2019). Microalgal bioremediation of emerging contaminants-Opportunities and challenges. Water Res. 164:114921. doi: 10.1016/j.watres.2019.114921
Takahashi, J. A., Teles, A. P. C., Bracarense, A. D. A. P., and Gomes, D. C. (2013). Classical and epigenetic approaches to metabolite diversification in filamentous fungi. Phytochem. Rev. 12, 773–789. doi: 10.1007/s11101-013-9305-5
Tan, L. T. (2013). Pharmaceutical agents from filamentous marine cyanobacteria. Drug Discov. Today 18, 863–871. doi: 10.1016/j.drudis.2013.05.010
Taşdemirci, A., Yüksel, S., Karsu, D., Gültürk, E., Hall, I. W., and Güden, M. (2008). Diatom frustule-filled epoxy: experimental and numerical study of the quasi-static and high strain rate compression behavior. Mater. Sci. Eng. A 480, 373–382. doi: 10.1016/j.msea.2007.07.037
Taylor, D. (2015). “The pharmaceutical industry and the future of drug development,” in Pharmaceuticals in the Environment, eds R. E. Hester and R. M. Harrison (London: Royal Society of Chemistry), 1–33. doi: 10.1039/9781782622345-00001
Tedesco, S., and Stokes, J. (2017). Valorisation to biogas of macroalgal waste streams: a circular approach to bioproducts and bioenergy in Ireland. Chem. Pap. 71, 721–728. doi: 10.1007/s11696-016-0005-7
Teixeira, T. R., Santos, G. S. D., Armstrong, L., Colepicolo, P., and Debonsi, H. M. (2019). Antitumor potential of seaweed derived-endophytic fungi. Antibiotics 8:205. doi: 10.3390/antibiotics8040205
Theodotou Schneider, X. (2019). Responsible Research and Innovation Roadmap. RRI Tool from the MARINA Horizon 2020 project. Available online at: https://www.researchgate.net/publication/339630196_The_Responsible_Research_and_Innovation_RRI_Roadmap#fullTextFileContent (accessed November 13, 2020).
Thompson, C. C., Kruger, R. H., and Thompson, F. L. (2017). Unlocking marine biotechnology in the developing world. Trends Biotechnol. 35, 1119–1121. doi: 10.1016/j.tibtech.2017.08.005
Thompson, F., Krüger, R., Thompson, C. C., Berlinck, R. G. S., Coutinho, R., Landell, M. F., et al. (2018). Marine biotechnology in Brazil: recent developments and its potential for innovation. Front. Mar. Sci. 5:236. doi: 10.3389/fmars.2018.00236
Thomsen, P. F., Kielgast, J., Iversen, L. L., Møller, P. R., Rasmussen, M., and Willerslev, E. (2012). Detection of a diverse marine fish fauna using environmental DNA from seawater samples. PLoS One 7:e41732. doi: 10.1371/journal.pone.0041732
Tibbetts, S. M., Patelakis, S. J. J., Whitney-Lalonde, C. G., Garrison, L. L., Wall, C. L., and MacQuarrie, S. P. (2020). Nutrient composition and protein quality of microalgae meals produced from the marine prymnesiophyte Pavlova sp. 459 mass-cultivated in enclosed photobioreactors for potential use in salmonid aquafeeds. J. Appl. Phycol. 32, 299–318. doi: 10.1007/s10811-019-01942-2
Tidgewell, K., Clark, B. R., and Gerwick, W. H. (2010). “The natural products chemistry of cyanobacteria,” in Comprehensive Natural Products II, eds H. W. Liu and L. Mander (Amsterdam: Elsevier), 141–188. doi: 10.1016/b978-008045382-8.00041-1
Timmermans, M. L., Paudel, Y. P., and Ross, A. C. (2017). Investigating the biosynthesis of natural products from marine Proteobacteria: a survey of molecules and strategies. Mar. Drugs 15:235. doi: 10.3390/md15080235
Tisthammer, K. H., Cobian, G. M., and Amend, A. S. (2016). Global biogeography of marine fungi is shaped by the environment. Fungal Ecol. 19, 39–46. doi: 10.1016/j.funeco.2015.09.003
Townsed, M., Davies, K., Hanley, N., Hewitt, J. E., Lundquist, C. J., and Lohrer, A. M. (2018). The challenge of implementing the marine ecosystem service concept. Front. Mar. Sci. 5:359. doi: 10.3389/fmars.2018.00359
Toyama, T., Hanaoka, T., Yamada, K., Suzuki, K., Tanaka, Y., Morikawa, M., et al. (2019). Enhanced production of biomass and lipids by Euglena gracilis via co-culturing with a microalga growth-promoting bacterium, Emticicia sp. EG3. Biotechnol. Biofuels 12:205. doi: 10.1186/s13068-019-1544-2
Tran, N. H., and Gin, K. Y. H. (2017). Occurrence and removal of pharmaceuticals, hormones, personal care products, and endocrine disrupters in a full-scale water reclamation plant. Sci. Total Environ. 599, 1503–1516. doi: 10.1016/j.scitotenv.2017.05.097
Trepos, R., Pinori, E., Jonsson, P. R., Berglin, M., Svenson, J., Coutinho, R., et al. (2014). Innovative approaches for the development of new copper-free marine antifouling paints. J. Ocean Technol. 9, 7–18.
Trincone, A. (2011). Marine biocatalysts: enzymatic features and applications. Mar. Drugs 9, 478–499. doi: 10.3390/md9040478
Tripathi, N. K., and Shrivastava, A. (2019). Recent developments in bioprocessing of recombinant proteins: expression hosts and process development. Front. Bioeng. Biotechnol. 7:420. doi: 10.3389/fbioe.2019.00420
Trivella, D. B. B., and de Felicio, R. (2018). The tripod for bacterial natural product discovery: genome mining, silent pathway induction, and mass spectrometry-based molecular networking. mSystems 3:e00160-17. doi: 10.1128/mSystems.00160-17
Urbanek, A. K., Rymowicz, W., and Mirończuk, A. M. (2018). Degradation of plastics and plastic-degrading bacteria in cold marine habitats. Appl. Microbiol. Biotechnol. 102, 7669–7678. doi: 10.1007/s00253-018-9195-y
Vallet, M., Vanbellingen, Q. P., Fu, T., Le Caer, J. P., Della-Negra, S., Touboul, D., et al. (2017). An integrative approach to decipher the chemical antagonism between the competing endophytes Paraconiothyrium variabile and Bacillus subtilis. J. Nat. Prod. 80, 2863–2873. doi: 10.1021/acs.jnatprod.6b01185
Van den Burg, S. W., Aguilar-Manjarrez, J., Jenness, J., and Torrie, M. (2019). Assessment of the geographical potential for co-use of marine space, based on operational boundaries for blue growth sectors. Mar. Policy 100, 43–57. doi: 10.1016/j.marpol.2018.10.050
van der Hooft, J. J. J., Mohimani, H., Bauermeister, A., Dorrestein, P. C., Duncan, K. R., and Medema, M. H. (2020). Linking genomics and metabolomics to chart specialized metabolic diversity. Chem. Soc. Rev. 49, 3297–3314. doi: 10.1039/d0cs00162g
van Oppen, M. J. H., Leong, J. A., and Gates, R. D. (2009). Coral-virus interactions: a double-edged sword? Symbiosis 47, 1–8. doi: 10.1007/bf03179964
van Santen, J. A., Jacob, G., Leen Singh, A., Aniebok, V., Balunas, M. J., Bunsko, D., et al. (2019). The natural products atlas: an open access knowledge base for microbial natural products discovery. ACS Cent. Sci. 5, 1824–1833.
Varshney, P., Mikulic, P., Vonshak, A., Beardall, J., and Wangikar, P. P. (2015). Extremophilic micro-algae and their potential contribution in biotechnology. Bioresour. Technol. 184, 363–372. doi: 10.1016/j.biortech.2014.11.040
Vatsos, I. N., and Rebours, C. (2015). Seaweed extracts as antimicrobial agents in aquaculture. J. Appl. Phycol. 27, 2017–2035. doi: 10.1007/s10811-014-0506-0
Vaz-Pinto, F., Rodil, I. F., Mineur, F., Olabarria, C., and Arenas, F. (2014). Marine algae: biodiversity, taxonomy, environmental assessment, and biotechnology,” in Marine Algae Biodiversity, Taxonomy, Environmental Assessment, and Biotechnology, eds L. Pereira and J. Magalhaes Neto (Boca Raton, FL: CRC Press), 178–194.
Vedachalam, N., Ravindran, M., and Atmanand, M. A. (2019). Technology developments for the strategic Indian blue economy. Mar. Georesources Geotechnol. 37, 828–844. doi: 10.1080/1064119x.2018.1501625
Venter, J. C., Remington, K., Heidelberg, J. F., Halpern, A. L., Rusch, D., Eisen, J. A., et al. (2004). Environmental genome shotgun sequencing of the Sargasso Sea. Science 304, 66–74. doi: 10.1126/science.1093857
Ventura, P., Toullec, G., Fricano, C., Chapron, L., Meunier, V., Röttinger, E., et al. (2018). Cnidarian primary cell culture as a tool to investigate the effect of thermal stress at the cellular level. Mar. Biotechnol. 20, 144–154.
Vignesh, G., and Barik, D. (2019). “Toxic waste from biodiesel production industries and its utilization,” in Energy From Toxic Organic Waste for Heat and Power Generation, ed. D. Barik (Sawston: Woodhead Publishing), 69–82. doi: 10.1016/b978-0-08-102528-4.00006-7
Vijayaraj, R., Altaff, K., Rosita, A. S., Ramadevi, S., and Revathy, J. (2020). Bioactive compounds from marine resources against novel corona virus (2019-nCoV): in silico study for corona viral drug. Nat. Prod. Res. [Epub ahead of print]. doi: 10.1080/14786419.2020.1791115
Vilas-Boas, C., Sousa, E., Pinto, M., and Correia-da-Silva, M. (2017). An antifouling model from the sea: a review of 25 years of zosteric acid studies. Biofouling 33, 927–942. doi: 10.1080/08927014.2017.1391951
Vlachou, P., Le Goff, G., Alonso, C., Álvarez, P. A., Gallard, J. F., Fokialakis, N., et al. (2018). Innovative approach to sustainable marine invertebrate chemistry and a scale-up technology for open marine ecosystems. Mar. Drugs 16, 0152.
Voigt, O., Adamska, M., Adamski, M., Kittelmann, A., Wencker, L., and Wörheide, G. (2017). Spicule formation in calcareous sponges: coordinated expression of biomineralization genes and spicule-type specific genes. Sci. Rep. 7:45658. doi: 10.1038/srep45658
Volesky, B. (1990). “Biosorption and biosorbents,” in Biosorption of Heavy Metals, ed. B. Volesky (Boca Raton, FL: CRC press), 3–6.
von Schomberg, R. (2013). “A vision of responsible innovation,” in Responsible Innovation, eds R. Owen, M. Heintz, and J. Bessant (London: John Wiley).
Vu, M. T. T., Douette, C., Rayner, T. A., Thoisen, C., Nielsen, S. L., and Hansen, B. W. (2016). Optimization of photosynthesis, growth, and biochemical composition of the microalga Rhodomonas salina-an established diet for live feed copepods in aquaculture. J. Appl. Phycol. 28, 1485–1500. doi: 10.1007/s10811-015-0722-2
Wade, W. (2002). Unculturable bacteria-the uncharacterized organisms that cause oral infections. J. R. Soc. Med. 95, 81–83. doi: 10.1258/jrsm.95.2.81
Walker, C. H. (2008). Ecotoxicity testing: science, politics and ethics. Altern. Lab. Anim. 36, 103–112. doi: 10.1177/026119290803600111
Wang, G., Wang, X., Liu, X., and Li, Q. (2012). “Diversity and biogeochemical function of planktonic fungi in the ocean,” in Biology of Marine Fungi, ed. C. Raghukumar (Berlin: Springer), 71–88. doi: 10.1007/978-3-642-23342-5_4
Wang, L., Min, M., Li, Y., Chen, P., Chen, Y., Liu, Y., et al. (2010). Cultivation of green algae Chlorella sp. in different wastewaters from municipal wastewater treatment plant. Appl. Biochem. Biotechnol. 162, 1174–1186. doi: 10.1007/s12010-009-8866-7
Wang, M., Carver, J. J., Phelan, V. V., Sanchez, L. M., Garg, N., Peng, Y., et al. (2016). Sharing and community curation of mass spectrometry data with global natural products social molecular networking. Nat. Biotechnol. 34, 828–837.
Wang, Q., Jin, W., Zhou, X., Guo, S., Gao, S. H., Chen, C., et al. (2019). Growth enhancement of biodiesel-promising microalga Chlorella pyrenoidosa in municipal wastewater by polyphosphate-accumulating organisms. J. Clean. Prod. 240:118148. doi: 10.1016/j.jclepro.2019.118148
Wang, X., and Hamann, M. T. (2019). Integrated X-Ray, NMR, ECD, and computational approaches to assign the stereochemistry of nortriterpenoids. Nat. Prod. Commun. 14:1934578X1984931. doi: 10.1177/1934578X19849311
Webster, N. S., and Taylor, M. W. (2011). Marine sponges and their microbial symbionts: love and other relationships. Environ. Microbiol. 14, 335–346. doi: 10.1111/j.1462-2920.2011.02460.x
Weigand, A., Beermann, A. J., Čiampor, F., Costa, F. O., Csabai, Z., Duarte, S., et al. (2019). DNA barcode reference libraries for the monitoring of aquatic biota in Europe: gap-analysis and recommendations for future work. Sci. Total Environ. 678, 499–524. doi: 10.1016/j.scitotenv.2019.04.247
Weinberger, F., Paalme, T., and Wikström, S. A. (2019). Seaweed resources of the Baltic Sea, Kattegat and German and Danish North Sea coasts. Bot. Mar. 63:19. doi: 10.1515/bot-2019-0019
Welker, M., and van Döhren, H. (2006). Cyanobacterial peptides - Nature’s own combinatorial biosynthesis. FEMS Microbiol. Rev. 30, 530–563. doi: 10.1111/j.1574-6976.2006.00022.x
White, J. D., Kim, T. S., and Nambu, M. (1997). Absolute configuration and total synthesis of (+)-Curacin A, an antiproliferative agent from the cyanobacterium Lyngbya majuscule. J. Am. Chem. Soc. 119, 103–111. doi: 10.1021/ja9629874
White, J. R., Abodeely, M., Ahmed, S., Debauve, G., Johnson, E., Meyer, D. M., et al. (2019). Best practices in bioassay development to support registration of biopharmaceuticals. BioTechniques 67, 126–137. doi: 10.2144/btn-2019-0031
Wijffels, R. H. (2008). Potential of sponges and microalgae for marine biotechnology. Trends Biotechnol. 26, 26–31. doi: 10.1016/j.tibtech.2007.10.002
Wiley, P., Harris, L., Reinsch, S., Tozzi, S., Embaye, S., Clark, K., et al. (2013). Microalgae cultivation using offshore membrane enclosures for growing algae (omega). J. Sustain. Bioenergy Syst. 3, 18–32. doi: 10.4236/jsbs.2013.31003
Wilk, R., and Chojnacka, K. (2015). “Upstream processing in the technology of algal extracts: biomass harvesting and preparation for extraction process,” in Marine Algae Extracts: Processes, Products, and Applications, 2 Volume Set, eds S.-K. Kim and K. Chojnacka (Weinheim: Wiley), 145–159. doi: 10.1002/9783527679577.ch8
Wilkins, L. G. E., Leray, M., O’Dea, A., Yuen, B., Peixoto, R. S., Pereira, et al. (2019). Host-associated microbiomes drive structure and function of marine ecosystems. PLoS Biol. 17:e3000533. doi: 10.1371/journal.pbio.3000533
Williams, P. G. (2009). Panning for chemical gold: marine bacteria as a source of new therapeutics. Trends Biotechnol. 27, 45–52. doi: 10.1016/j.tibtech.2008.10.005
Williams, P. J. L. B., and Laurens, L. M. L. (2010). Microalgae as biodiesel & biomass feedstocks: review & analysis of the biochemistry, energetics & economics. Energy Environ. Sci. 3, 554–590.
Wolfender, J. L., Nuzillard, J. M., van der Hooft, J. J. J., Renault, J. H., and Bertrand, S. (2019). Accelerating metabolite identification in natural product research: toward an ideal combination of liquid chromatography–high-resolution tandem mass spectrometry and NMR profiling, in silico databases, and chemometrics. Anal. Chem. 91, 704–742. doi: 10.1021/acs.analchem.8b05112
WoRMS Editorial Board (2020). World Register of Marine Species. Available online at: http://www.marinespecies.org at VLIZ (accessed April 04, 2020).
Xie, Y., Sen, B., and Wang, G. (2017). Mining terpenoids production and biosynthetic pathway in thraustochytrids. Bioresour. Technol. 244, 1269–1280. doi: 10.1016/j.biortech.2017.05.002
Xu, W., Gong, L. F., Pang, K. L., and Luo, Z. H. (2018). Fungal diversity in deep-sea sediments of a hydrothermal vent system in the Southwest Indian Ridge. Deep Sea Res. Pt I 131, 16–26. doi: 10.1016/j.dsr.2017.11.001
Yan, Z. G., Zheng, X., Gao, F., Fan, J. T., Wang, S. P., and Yang, L. X. (2019). A framework for ecotoxicity testing in the 21st century: Ecotox21. Appl. Sci. 9:428. doi: 10.3390/app9030428
Yang, H., Sun, L., Li, W., Liu, G., and Tang, Y. (2018). In silico prediction of chemical toxicity for drug design using machine learning methods and structural alerts. Front. Chem. 6:30. doi: 10.3389/fchem.2018.00030
Yarden, O. (2014). Fungal association with sessile marine invertebrates. Front. Microbiol. 5:228. doi: 10.3389/fmicb.2014.00228
Yatsunami, R., Ando, A., Yang, Y., Takaichi, S., Kohno, M., Matsumura, Y., et al. (2014). Identification of carotenoids from the extremely halophilic archaeon Haloarcula japonica. Front. Microbiol. 5:100. doi: 10.3389/fmicb.2014.00100
Yebra, D. M., Kiil, S., and Dam-Johansen, K. (2004). Antifouling technology-past, present and future steps towards efficient and environmentally friendly antifouling coatings. Prog. Org. Coat. 50, 75–104. doi: 10.1016/j.porgcoat.2003.06.001
Yokosaka, S., Izawa, A., Sakai, C., Sakurada, E., Morita, Y., and Nishio, Y. (2018). Synthesis and evaluation of novel dolastatin 10 derivatives for versatile conjugations. Bioorg. Med. Chem. 26, 1643–1652. doi: 10.1016/j.bmc.2018.02.011
Yousaf, M., El Sayed, K. A., Rao, K. V., Lim, C. W., Hu, J. F., Kelly, M., et al. (2002). 12,34-Oxamanzamines, novel biocatalytic and natural products from manzamine producing Indo-Pacific sponges. Tetrahedron 58, 7397–7402. doi: 10.1016/s0040-4020(02)00825-6
Yu, H., Liu, X., Dong, X., Li, C., Xing, R., Liu, S., et al. (2005). Insecticidal activity of proteinous venom from tentacle of jellyfish Rhopilema esculentum Kishinouye. Bioorg. Med. Chem. Lett. 15, 4949–4952. doi: 10.1016/j.bmcl.2005.08.015
Yu, H., Li, R., Chen, X., Yue, Y., Xing, R., Liu, S., et al. (2015). Effect of venom from the jellyfish Nemopilema nomurai on the silkworm Bombyx mori L. Toxins 7, 3876–3886. doi: 10.3390/toxins7103876
Yu, X., Chen, L., and Zhang, W. (2015). Chemicals to enhance microalgal growth and accumulation of high-value bioproducts. Front. Microbiol. 6:56. doi: 10.3389/fmicb.2015.00056
Yuan, X. Y., Wang, M., Lei, S., Yang, Q. X., and Liu, Y. Q. (2017). Rapid screening of active components with an osteoclastic inhibitory effect in Herba epimedii using quantitative pattern-activity relationships based on joint-action models. Molecules 22:1767. doi: 10.3390/molecules22101767
Zeraatkar, A. K., Ahmadzadeh, H., Talebi, A. F., Moheimani, N. R., and McHenry, M. P. (2016). Potential use of algae for heavy metal bioremediation, a critical review. J. Environ. Manage. 181, 817–831. doi: 10.1016/j.jenvman.2016.06.059
Zerikly, M., and Challis, G. L. (2009). Strategies for the discovery of new natural products by genome mining. Chembiochem 10, 625–633. doi: 10.1002/cbic.200800389
Zettler, E. R., Mincer, T. J., and Amaral-Zettler, L. A. (2013). Life in the “plastisphere”: microbial communities on plastic marine debris. Environ. Sci. Technol. 47, 7137–7146. doi: 10.1021/es401288x
Zhang, C., Show, P. L., and Ho, S. H. (2019). Progress and perspective on algal plastics – a critical review. Bioresour. Technol. 289:121700. doi: 10.1016/j.biortech.2019.121700
Zhang, J., Kerayama, K., Sumita, M., Yoshizoe, K., Ito, K., Kikuchi, J., et al. (2020a). NMR-TS: de novo molecule identification from NMR spectra. Sci. Technol. Adv. Mater. 21, 552–561. doi: 10.1080/14686996.2020.1793382
Zhang, J., Wen, C., Zhang, H., Duan, Y., and Ma, H. (2020b). Recent advances in the extraction of bioactive compounds with subcritical water: a review. Trends Food Sci. Tech. 95, 183–195. doi: 10.1016/j.tifs.2019.11.018
Zhang, Q. W., Kin, L. G., and Ye, W. C. (2018). Techniques for extraction and isolation of natural products: a comprehensive review. Chin. Med. 13:20. doi: 10.1186/s13020-018-0177-x
Zhang, W., Ding, W., Li, Y.-X., Tam, C., Bougouffa, S., Wang, R., et al. (2019). Marine biofilms constitute a bank of hidden microbial diversity and functional potential. Nat. Commun. 10:517. doi: 10.1038/s41467-019-08463-z
Zhong, J. P., Wang, G., Shang, J. H., Pan, J. Q., Li, K., Huang, Y., et al. (2009). Protective effects of squid ink extract towards hemopoietic injuries induced by cyclophosphamine. Mar. Drugs 7, 9–18. doi: 10.3390/md7010009
Zhu, H., Sandiford, S. K., and van Wezel, G. P. (2014). Triggers and cues that activate antibiotic production by actinomycetes. J. Ind. Microbiol. Biotechnol. 41, 371–386. doi: 10.1007/s10295-013-1309-z
Zhu, S., and Sun, M. (2020). Electronic circular dichroism and raman optical activity: principle and applications. Appl. Spectrosc. Rev. [Epub ahead of print].
Zidorn, C. (2016). Secondary metabolites of seagrasses (Alismatales and Potamogetonales; Alismatidae): chemical diversity, bioactivity, and ecological function. Phytochemistry 124, 5–28. doi: 10.1016/j.phytochem.2016.02.004
Zivanovic, Y., Armengaud, J., Lagorce, A., Leplat, C., Guérin, P., Dutertre, M., et al. (2009). Genome analysis and genome-wide proteomics of Thermococcus gammatolerans, the most radioresistant organism known amongst the Archaea. Genome Biol. 10:R70. doi: 10.1186/gb-2009-10-6-r70
Zullaikah, S., Utomo, A. T., Yasmin, M., Ki Ong, L., and Ju, Y. H. (2019). “Ecofuel conversion technology of inedible lipid feedstocks to renewable fuel,” in Advances in Eco-Fuels for a Sustainable Environment, ed. K. Azad (Sawston: Woodhead Publishing), 237–276. doi: 10.1016/b978-0-08-102728-8.00009-7
Keywords: bioprospecting, blue growth, marine biodiversity, marine natural products, sustainability, ethics, responsible research and innovation (RRI), marine bioeconomy
Citation: Rotter A, Barbier M, Bertoni F, Bones AM, Cancela ML, Carlsson J, Carvalho MF, Cegłowska M, Chirivella-Martorell J, Conk Dalay M, Cueto M, Dailianis T, Deniz I, Díaz-Marrero AR, Drakulovic D, Dubnika A, Edwards C, Einarsson H, Erdoǧan A, Eroldoǧan OT, Ezra D, Fazi S, FitzGerald RJ, Gargan LM, Gaudêncio SP, Gligora Udovič M, Ivošević DeNardis N, Jónsdóttir R, Kataržytė M, Klun K, Kotta J, Ktari L, Ljubešić Z, Lukić Bilela L, Mandalakis M, Massa-Gallucci A, Matijošytė I, Mazur-Marzec H, Mehiri M, Nielsen SL, Novoveská L, Overlingė D, Perale G, Ramasamy P, Rebours C, Reinsch T, Reyes F, Rinkevich B, Robbens J, Röttinger E, Rudovica V, Sabotič J, Safarik I, Talve S, Tasdemir D, Theodotou Schneider X, Thomas OP, Toruńska-Sitarz A, Varese GC and Vasquez MI (2021) The Essentials of Marine Biotechnology. Front. Mar. Sci. 8:629629. doi: 10.3389/fmars.2021.629629
Received: 15 November 2020; Accepted: 08 February 2021;
Published: 16 March 2021.
Edited by:
Maria Angeles Esteban, University of Murcia, SpainReviewed by:
Fabiano Thompson, Federal University of Rio de Janeiro, BrazilCopyright © 2021 Rotter, Barbier, Bertoni, Bones, Cancela, Carlsson, Carvalho, Cegłowska, Chirivella-Martorell, Conk Dalay, Cueto, Dailianis, Deniz, Díaz-Marrero, Drakulovic, Dubnika, Edwards, Einarsson, Erdoǧan, Eroldoǧan, Ezra, Fazi, FitzGerald, Gargan, Gaudêncio, Gligora Udovič, Ivošević DeNardis, Jónsdóttir, Kataržytė, Klun, Kotta, Ktari, Ljubešić, Lukić Bilela, Mandalakis, Massa-Gallucci, Matijošytė, Mazur-Marzec, Mehiri, Nielsen, Novoveská, Overlingė, Perale, Ramasamy, Rebours, Reinsch, Reyes, Rinkevich, Robbens, Röttinger, Rudovica, Sabotič, Safarik, Talve, Tasdemir, Theodotou Schneider, Thomas, Toruńska-Sitarz, Varese and Vasquez. This is an open-access article distributed under the terms of the Creative Commons Attribution License (CC BY). The use, distribution or reproduction in other forums is permitted, provided the original author(s) and the copyright owner(s) are credited and that the original publication in this journal is cited, in accordance with accepted academic practice. No use, distribution or reproduction is permitted which does not comply with these terms.
*Correspondence: Ana Rotter, YW5hLnJvdHRlckBuaWIuc2k=
Disclaimer: All claims expressed in this article are solely those of the authors and do not necessarily represent those of their affiliated organizations, or those of the publisher, the editors and the reviewers. Any product that may be evaluated in this article or claim that may be made by its manufacturer is not guaranteed or endorsed by the publisher.
Research integrity at Frontiers
Learn more about the work of our research integrity team to safeguard the quality of each article we publish.