- 1School of Civil and Environmental Engineering, Technische Universität Dresden, Dresden, Germany
- 2National Institute of Water and Atmospheric Research, Wellington, New Zealand
- 3Fraunhofer Institute for Energy Economics and Energy System Technology, Kassel, Germany
- 4Oxenu LLP, Oxford, United Kingdom
- 5Institute for the Oceans and Fisheries, University of British Columbia, Vancouver, BC, Canada
- 6National Institute of Water and Atmospheric Research, Hamilton, New Zealand
- 7Department of Physics, University of Auckland, Auckland, New Zealand
A cost-benefit analysis (CBA) is described for a novel wave energy converters (WEC) design based on a marine hybrid bio-structure—a combination of macroalgae, shellfish or other species on a built frame. The Bio-Oscillator design utilises a hard “skeleton” (e.g., carbon fibre, wood) on which biological organisms (e.g., shellfish, large macroalgae) are grown. As waves pass by, the load generated by the oscillating drag and inertia is transferred through mooring lines to power takeoff technology. This novel approach essentially reverses the typical marine engineering view that “bio-fouling is bad” and instead leverages off the added-drag of biological growth on structures. The approach results in a structure that is largely biodegradable, naturally self-replicating and synergistic with the background environment, self-de-risking in terms of failure impact and can leverage off its own form to enhance energy capture beyond a conventional design. This reduces impact while connecting with conventional marine industries such as aquaculture. A CBA examines the economic pros and cons of this approach, focusing on installation and material costs, along with benefits from synergistic production. The analysis suggests that in addition to typical wave energy obstacles (e.g., cable length, capture width, and power take off) the benefits (biodegradability, harvestability, and carbon reduction) of replacing much of the mass of the structure with living biological material can be included.
Introduction
Sustainable, low carbon emission energy production remains the great challenge for our species. With limited success in reduction of CO2 gases to date, a significant transformation in how the energy sector operates is required, if we are to avoid the more serious effects of the changing climate driven by greenhouse gas emissions (Gielen et al., 2019). Efforts to reduce the dependency on fossil fuels have seen an increased use of renewable energy over the last decades. This has been aided by policy development and legislation such as the EU’s Green Deal, that (at least pre-Covid 19) set ambitious targets for its member states’ energy supply (European Commission, 2019).
While most technologies across the renewables sector have seen a significant growth in scale and economic viability over the last decades, marine renewable energy (other than off-shore wind) has generally lagged and presently plays little role in most countries’ strategies for energy independence (Gielen et al., 2019). Despite the evidence of technological viability, ocean power has increased by less than 1 TWh globally—a trajectory well short of the sustainable development scenario of 15 TWh by 2030 (IEA, 2019).
Wave energy conversion, a form of marine renewable energy, uses ocean surface gravity waves to produce renewable energy (Sheng, 2019). Up to 2 TW of wave energy is thought to be accessible globally (Gunn and Stock-Williams, 2012), so that by 2050 ocean energy could, for example, provide upward of 10% of the United Kingdom energy demand (Stegman et al., 2017). Despite this surfeit of resource, the technology has not yet matured to the point where off-the-shelf wave energy converters (WEC) are available (Sheng, 2019). There are a number of reasons why this is the case, with a leading issue being the inability of the technology to reliably survive in a hostile environment. Designers put great effort into minimizing the risk of their converters being damaged during storms, while at the same time remaining economically viable and capturing as much energy as possible. The key is to find this balance and mitigate the impact of storm-damage, while at the same time using cost-effective materials.
One solution is the merging of wave power plants with other marine infrastructure, such as wind power or aquaculture farms. This opens avenues for scaling, maintenance, and multiple revenue streams; thus expanding application to areas where energy generation by itself would not be profitable. The similarities between wave energy device motion and that of floating aquaculture structures (Stevens et al., 2008) leads us to examine the hypothesis that, other than power take-off, a WEC—the Bio-Oscillator—can primarily be built from biological organisms (e.g., macroalgae, shellfish or a combination of both). The hybrid Bio-Oscillator approach can be thought of as modification of a floating aquaculture structure re-arranged to primarily enable sustainable and cost-efficient energy extraction from ocean waves.
While it is appealing to geographically generalize the concept from the outset, the approach needs to merge a high energy wave regime, suitable local species and existing coastal industries against the background of favourable socio-economic parameters (e.g., Bertram et al., 2020). Here we focus on the New Zealand situation as a case study site because its geographical, ecological, and socio-economic characteristics provide some useful variation with which to contextualize the suggested concept.
We examine the hypothesis described above with a cost-benefit analysis (CBA) to explore key themes through high-level questions: (1) Does the CBA suggest that a hybrid approach, such as the Bio-Oscillator, is viable? (2) Are there broader benefits from the nature of the approach? (3) What are the potentially socially sensitive components of such an approach? (4) Finally, while the present ideas focus on a regional application, how generalizable is the approach globally?
Bio-Oscillator Concept
As a significant portion of the mass in WEC devices is essentially passive (Young, 2011), the potential exists to take advantage of this. A number of WEC designs mimic organisms that thrive in wave-swept coastal environments, such as large macroalgae (Finnigan and Caska, 2006). The Bio-Oscillator concept takes this a step further and uses the organisms, grown on a manufactured “skeleton” that acts as a substrate, as part of the structure (Figure 1). The skeleton must be some rigid, buoyant framework that acts as a substrate for biological organisms attached to it which then adds the drag, buoyancy, and mass necessary to capture the wave energy.
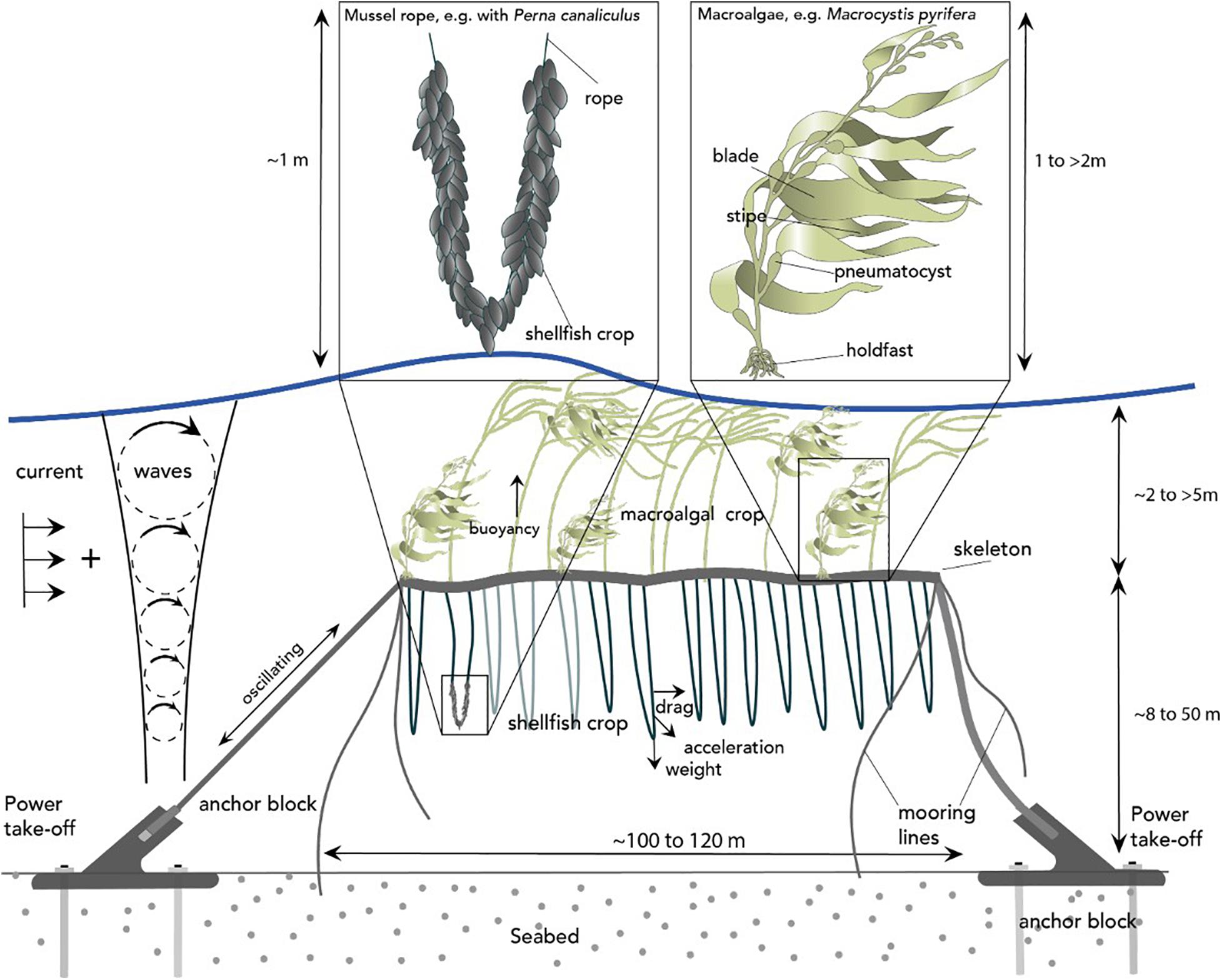
Figure 1. Schematic of initial concept showing the skeleton, anchors, and biological options in a wave-current field.
Additionally, the approach seeks to reduce the impact of severe storms by only exposing biodegradable, renewable, biological elements to extreme energies, placing more sensitive and valuable components such as power take-off in greater depths or within the skeleton with a smaller probability of being damaged. During storms, the biological attachment points, such as holdfasts for kelps or byssal threads for shellfish, function as preferential breaking points within the WEC. As they break off, the load exerted on the remaining parts is reduced, and with it is a reduction in the risk of the WEC skeleton and non-biodegradable debris getting washed away into the wider environment.
The approach utilizes the biological adaptation of the crop to enhance the operational characteristics as well as economic attractiveness of the device. For example, large bladed/fronded macroalgae add drag (and possibly some buoyancy) and heavy lines of shellfish crop add mass and drag. This volume and surface area of this material along with its flexibility will influence the nature of the wave-current interaction by affecting the phase-shift between forcing and response (Stevens et al., 2001). In order to understand this motion it is convenient to view the concept as being like a long-line shellfish farm with power take-off integrated within the moorings or the skeleton (Figure 1). This is different to biomimicry concepts that seek to emulate biological form and function with steel or other materials (i.e., the opposite of that described here—e.g., Finnigan and Caska, 2006) or integrate a wave generator onto an aquaculture installation in a way that the devices function independently. In the present initial concept the power take-off system of the Bio-Oscillator is positioned either on the seabed or within the skeleton (Figure 1).
Aquaculture husbandry techniques are required as bivalves and macroalgae are grown on the skeleton, which can be detached from the mooring section and harvested regularly. The first part of the husbandry would occur in a sheltered environment with the newly seeded skeleton modules until the species growing on the skeleton reach a size and mass suitable for energy capture. The skeleton is then moved offshore and attached to the mooring system. This replaces the skeleton previously offshore which is then taken inshore to harvest the fully-grown biological material and re-start the seeding on the skeleton.
The longitudinal alignment of the Bio-Oscillator is parallel to the wave propagation direction, which makes the width that is exposed to the wave power relatively small. At the same time, the longitudinal extent potentially provides enough drag and added mass from the biological organisms, which are attached to the skeleton that forms the substrate (n.b. some aquaculture terminology refers to the “backbone”—we use a different term for clarity as there are some unique aspects to this application). Waves set the device into an oscillating motion, which is then captured by the power take-off units that either is mounted on anchor blocks or within the structure. From there, a submarine cable transfers the generated electricity to shore. Mooring lines help to keep the structure in place for an optimal alignment with the most common wave direction. This study does not primarily focus on the technical aspects of the power take-off as it is well-established and while each application will have a particular configuration there are distinct families of power take-off approaches (e.g., Gaspar et al., 2016; Henriques et al., 2016; Jusoh et al., 2019). This understanding includes new approaches to multi-tether designs (Sergiienko et al., 2018), which can drive electric or hydraulic generators. Similarly, the aquaculture benefits are described elsewhere (Naylor and Burke, 2005). Here we concentrate on the economic, biophysical, and socio-economic dimensions of the hybrid concept.
Methods
The present assessment synthesizes four aspects of the Bio-Oscillator concept, each with a focused to maximize the level of relevance to the hybrid nature of the approach. First, the impact of location selection is examined as a balance of available wave energy and operational requirements. Second, following this, material factors are examined including the skeleton material and this is applied to three configurations (i) heaving, (ii) floating oscillating wave surge converter (OWSC), and (iii) fixed OWSC devices. Thirdly, biological organisms are examined by looking at species “services.” Fourth, and finally, cost sensitivity to two structural design configurations are considered, contrasting a longline and a raft set-up. These are both “heaving: designs and are configurations commonly used for mussel farming (Stevens et al., 2008).”
Cost-benefit analyses are performed to assess how the number of deployed devices, and their location, influences the installation costs. It needs to be emphasized that the costs described here are not absolute and only allow for relative comparisons to be made, as the market normally fluctuates significantly as do regional differences in cost structures. A detailed hydrodynamic assessment of wave structure interaction is beyond the present scope as this would require details around location and power take off that are premature at this point in the technology readiness level trajectory.
Locations
A number of sites around Aotearoa New Zealand were selected as areas of possible deployment including near Auckland, Taranaki, Greymouth, and Tiwai Point (Figure 2 and Table 1). Aspects examined include the wave energy regime, local energy demand, infrastructure, coastal industries, and presence/absence of marine protected areas (MPAs). Estimates of energy at the sites were provided by a Wave Model (WAM) hindcast (Gorman et al., 2003) implemented over a domain covering the south-west Pacific and Southern Oceans. The mode quantifies the generation and propagation of deep-water waves toward the New Zealand coast over a 20-year period (1979–1998). From this analysis we chose the onshore energy flux EOF (in W/m, see Table 2 for notation) at 30 m depth to be the critical quantity as this suits the scale of the envisioned longline structure of the Bio-Oscillator. These data are available as archived outputs from the WAM hindcast and eight sites were examined (Table 1 and Figure 2). Two locations represent the Auckland west coast and were selected to examine alongshore variability including distance to grid adjacent to the nation’s largest urban power usage, as well as a large steel processing plant. Two sites were chosen around Taranaki as this is where there is a present energy industry focus and the locations incorporate varying wave conditions and grid connection distances. Two other sites are situated on the South Island’s west and south coasts. The Taranaki region has two additional control points to investigate the effect of wave power density when going inshore (T-shallow) or offshore (T-deep) from the standard 30 m deployment depth.
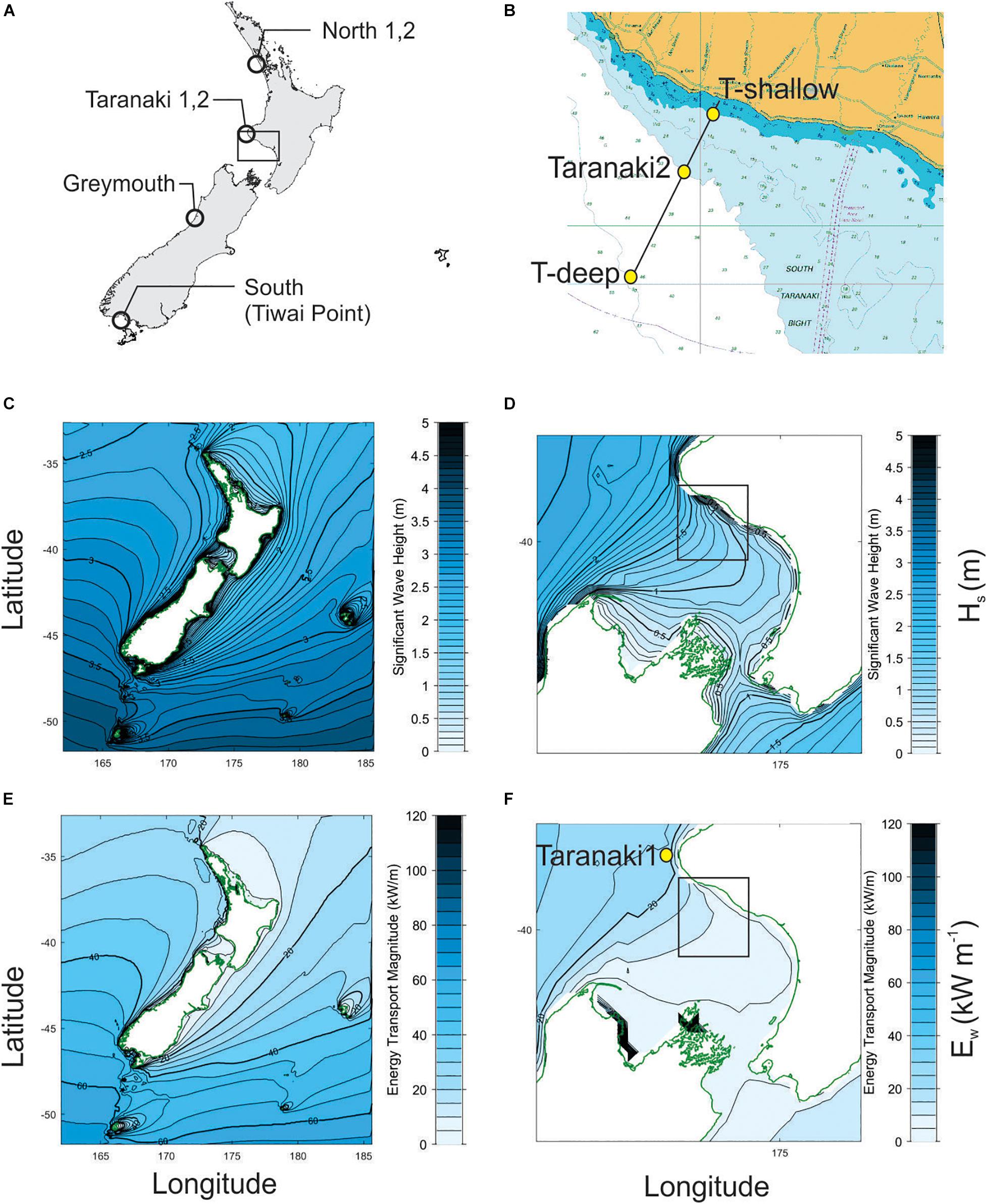
Figure 2. Regional setting and resources showing (A) New Zealand location diagram, (B) case study region inset from panel (A) with test locations (Taranaki-2 and deeper and shallower equivalents), and hindcast simulated 1957–2002, on a ∼10 km resolution NZ domain, using ERA40 winds showing (C,D) mean HS and (E,F) wave energy flux Ew. Panels (D,F) show the region in the location diagram (B).
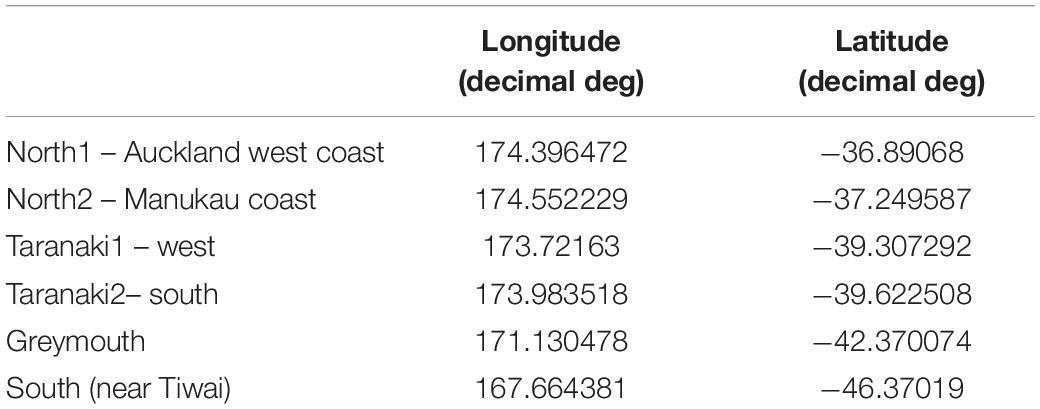
Table 1. Locations used in the analysis (see Figure 2).
Three distances were estimated using georeferenced maps in order to take plausible pathways to approach harbours and electricity grid points. These distances included (i) from deployment site to shore which is approximately the length of the submarine cable (LC–S), (ii) from the shore cable termination to an onshore grid connection location (LC–O), and (iii) from the deployment site to a suitable port (LP). Furthermore, to determine how the length of the submarine cable changes when getting closer to shore (10 m depth), or further offshore (50 m depth), the distances between the 30 m isobath and 10 and 50 m are measured. In order to keep the calculations as simple as possible, the differences in distance to port from 10 to 50 m water depth-sites to the 30 m depth sites are not considered. The installation costs contain submarine CC–S and onshore CC–O cable costs as well as the vessel hire cost. The prices for the submarine cable (LC–S = 433 EUR m–1) and onshore grid connection (57 EUR m–1) are given in Dalton et al. (2010) and Kim et al. (2012). Costs for the vessel deployment (CD, separate to cable costs) are approximated, based on an estimate of a modest approximate vessel day-rate, using the relationship 34,430 ++ distance to port LP × 0.29 m–1 [EUR].
Skeleton Material
A key element of the device is the material used for the skeleton, as this will potentially be a large component of material cost as well as being crucial for survival. A range of materials were evaluated regarding financial and environmental costs to provide an overall estimate of “longline costs” CL. Material costs per device are calculated with the value of 15.80 EUR per meter of longline (Buck et al., 2010). This price includes mooring construction, connecting pieces for the whole longline device, the complete buoyancy and collectors.
There are some specific environmental conditions for ocean material applications when compared to land-based applications. The need for reduced maintenance is also a motivation as the accessibility for repairs is restricted and the capital for doing so is intensive (Bleck and Moeller, 2017). Furthermore, it is important to identify suitable materials to enable efficient power take-off and moorings that should have a low susceptibility to biofouling. This contrasts with the material of the skeleton itself which should either function as a substrate for macroalgae or it would need to be wrapped by material that fulfils that purpose and enables a harvest-friendly attachment for crop lines. The skeleton itself must have some buoyancy (Figure 1). Where possible, it is assumed that this buoyancy is in-built using the skeleton material. This will not be possible for some materials (e.g., natural materials like flax) and so some additional flotation will be required. This is assumed part of the skeleton cost.
The required structural characteristics make the selection of materials a primary design consideration (see Table 3). Eight materials out of the groups of composites, natural materials, and steel are assessed including glass-reinforced composites, flax, palm, ramie, wood, carbon steel, mild steel, and stainless steel. In order to base the subsequent analysis on comparable values, the properties density, yield strength (Y), and length (LL) of the device are combined. An assumed effect of action for a heave force distribution of 270 kN m–1 (Babarit et al., 2012; see Supplementary Information) is multiplied by the device dimensions (LL, W) to provide an estimated weight of each structure per m of wave front.
Due to the low cost, high strength, lightweight, and excellent corrosion resistance of the material (Kootsookos and Mouritz, 2004), marine craft, and offshore oil drilling platforms are typical areas of application for glass-reinforced composites. While metals are commonly used in currently deployed WECs, stainless steel is mostly used only for small elements, because of high costs and potentially excessively high densities for small scale floating applications. Although the production of high-strength and ultra-high-strength steels (e.g., carbon steel) is possible, it is common practice to work with normal-strength steels regarding fatigue strength, compliance with buckling and weldability (Bleck and Moeller, 2017). Less conventional natural materials like wood, flax, palm (talipot), ramie, and wool fibre reduce the environmental costs of the device.
The material costs (CM)were retrieved in Euro from an industry-standard database (CES, 2016) and included in the Supplementary Information. A factor of fNZ = 1.2 is applied to the prices to account for generally higher materials and building costs in New Zealand. All environmental impact properties of the materials only represent the primary production, and not the processing or recycling and end-of-life values. They are neglected because of uncertainties of how the material would be processed to produce the skeleton’s parts. Furthermore, compared to the primary production, the quantified environmental impact of processing and end-of-life is significantly lower. The embodied energy is the energy required to generate one kg of refined product from its source material. The quoted numbers are based on published literature and life-cycle inventory databases (CES, 2016). The source of data for the CO2 and water footprints is obtained in the same way. The carbon footprint is the CO2-equivalent for greenhouse gases that are released into the atmosphere and water usage of primary production is obtained by direct measurements of factory inputs and outputs.
Biological Organisms
A literature survey was conducted to examine biophysical characteristics of regionally available species considering aspects such as organism size and morphology (Stevens et al., 2007; Nelson, 2020). In addition, less well-reported quantities such as buoyancy and strength were considered. The biology is represented in terms of mass, drag and cost to deploy as well as required husbandry techniques.
Structural Configuration
The capture-width ratio (CWR) represents the hydrodynamic efficiency of a device and thus influences the cost per unit energy. Designs like overtopping devices and oscillating water column devices are not considered sensible for an adaptation to the Bio-oscillator concept because drag or added mass do not benefit the energy production. Instead, we focus on perturbations of the category of a wave activated body, which is generally deemed most appropriate for the Bio-Oscillator. The two configuration options examined are the (1) heaving raft and (2) heaving long-line designs. We then extend this, based on Babarit’s (2015) database for CWRs of WECs, an evaluation is made regarding the adaptation of the three device variations (but not overtopping devices and oscillating water column) to the Bio-Oscillator concept. The values for CWR and characteristic dimensions for the categories are taken from Babarit (2015) which incoporates the wave-structure interaction dynamics. Because material costs for the skeleton scale with the deployment numbers and only weakly link to the installation costs, they are examined separately.
The power output P in kW is calculated with P = CWR.EOF (EOF = onshore energy flux, see Table 2). It is assumed that the Bio-Oscillator functions as a combination of a heaving device and an attenuator like the Pelamis, which Babarit (2015) describes as a variant of a heaving device with an average CWR of 16%. The longline has a capture width of 0.4 m while the raft configuration has a characteristic dimension B = 2(AW/π)1/2, with AW being the devices’ maximum horizontal cross-sectional area, in this case the side length of the raft with 7 m, which results in a characteristic dimension of 3 m for the raft design.
Cost Benefit Model
A numerical simulator was developed (using Python, see Supplementary Information) to analyse the costs and benefits of the Bio-Oscillator for two design options (longline and raft) at three farm scales (10-100-500 devices) with three different wave energy fluxes each (10, 30, 60 kW/m) contextualised by possible locations. As decisions regarding design component aspects, such as power take-off, cable interconnection between devices, and costs for seeds and spat for the husbandry, these dimensions are not included in the evaluation as it was assumed these would be similar no matter what the design was. The CBA focuses on sensitivity to particular questions (e.g., best location, best material, and best design) rather than a multivariate “best overall scheme” as there are too many unknowns at this stage. It is stressed again that the sensitivity of cost and energy production are considered primarily in a relative sense, so the absolute values are only indicative of variations in true cost.
The analysis (see notation in Table 2 and Supplementary Information for full description) considers the material costs of the devices as well as expenditure incurred through deployment and cable installations. The power output is calculated based on the dimension of the device, potential onshore energy flux and the CWR. In this way, the cost (EUR) and Benefit (power output, W) are functions of location (i.e., length of cable and wave energy flux), skeleton material, biology, device configuration, and overall number of devices in an array. The longline/raft price ratio is taken from Sallih and þórðarson (2005) with a factor of Rf = 1.10—the raft being 10% more expensive when looking at the same quantity of seed and length of rope. The power take-off appliance costs CPTO are assumed to be 20% of the initial costs of the device (Dalton et al., 2010).
• Cost Sensitivity to location (EUR) = LC–S × 433 EUR/m + LC–O × 56 EUR/m + 34,430 EUR + LP × 0.287 EUR/m.
• Cost Sensitivity to farm design (EUR) = CC–S + CC–O + CD + fNZ × (Rf × CL × LL) + CPTO.
• Energy produced Power output (kW) = f[CWR, EOF, W(longline, raft)].
• Cost Sensitivity to skeleton material (EUR) = (ρ/Y) × LL × EOF × W × Cw.
Results
Location and Farm Scale
Aotearoa New Zealand is centred within the Roaring Forties and Furious Fifties, and so sustains a substantial wave energy resource, especially along the west coast (Gorman et al., 2003; Bertram et al., 2020). In general, wave energy dissipates moving onshore, primarily due to bottom friction (Hsiao and Shemdin, 1978), thus deployment of any WEC further offshore has a higher available onshore energy flux at the cost of more infrastructure and greater exposure. Deploying the Bio-Oscillator further offshore is more sensible for some sites, compared to others, depending on the horizontal distance between the isobath of the different depths. Due to the significant costs for submarine cables, distance to shore has the potential to significantly influence the outcome of the analysis. The resulting analysis shows at which sites a deployment in greater depth, and therefore with a higher energy flux, is financially more beneficial (Figure 3).
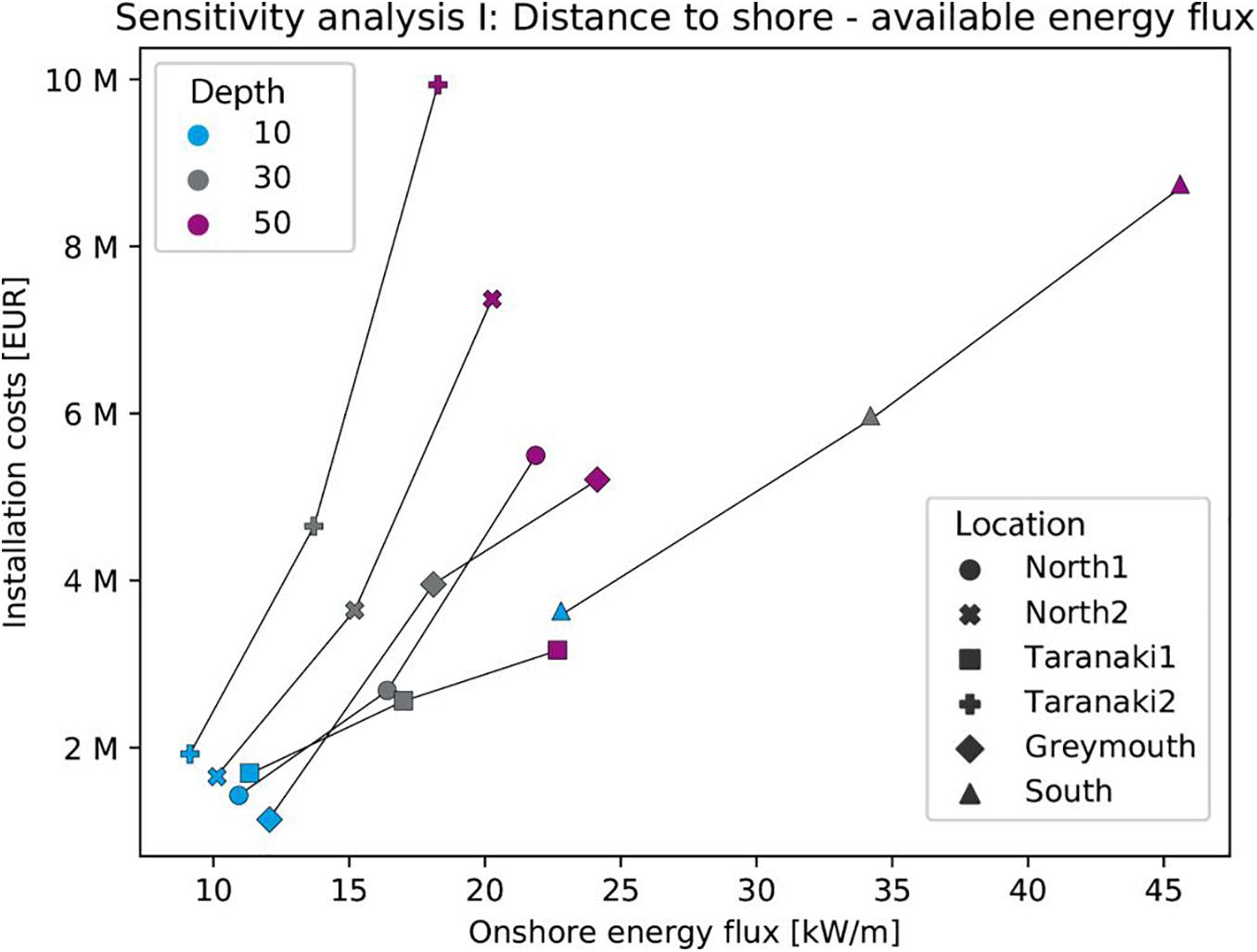
Figure 3. Relative installation costs [EUR] for cables (submarine and onshore) and deployment with vessel, depending on measured distances from six chosen locations. Available onshore energy flux in 30 m depth taken from WAM (Gorman et al., 2003) linear assumption for onshore energy flux at 10 and 50 m deep sites. Lines connect three depths of each location. A less steeper gradient implies a more favourable onshore energy flux—installation costs ratio.
Unusually, the hindcast results for the Taranaki-2 locations found no discernable reduction in energy moving onshore (Figure 2). This is likely a local effect due to refraction on this coastline and demonstrates that any such project needs local-scale observations and modelling. While such a targeted numerical analysis was beyond the present project scope, considering location Taranaki-2, it is apparent that a deployment in deeper water (T-deep, Figure 2) increases the installation costs disproportionately to the rise in available energy flux, compared for example to the locations Greymouth or Tiwai. This is due to the relatively long distances of the submarine cable at the Taranaki site. Data in Figure 3 that are in the upper left (lower right) quadrant have relatively low (high) onshore energy flux at relatively high (low) costs. The area in between those two can be considered as having reasonable, but not outstanding, performance. Overall, the less steep the gradient is between the points in Figure 3 that connect all three depths of one location, the better the ratio of onshore energy flux to installation cost.
The CBA determined how the number of deployed devices, their location and the primary capture system design (longline and raft), influences the costs of the respective approach. The lines in Figure 4 connect one type of device (e.g., 100 longline devices—colour cyan) with differing onshore energy fluxes. The results display the relationship between the financial costs and power output. As is to be expected, depending on the number of the devices and configurations type (longline/raft), the EUR kW–1 value is high for the longline setup with a low number of devices. Economically more sensible EUR kW–1 values are achieved with a raft system and deployment of a higher number of devices (Table 4).
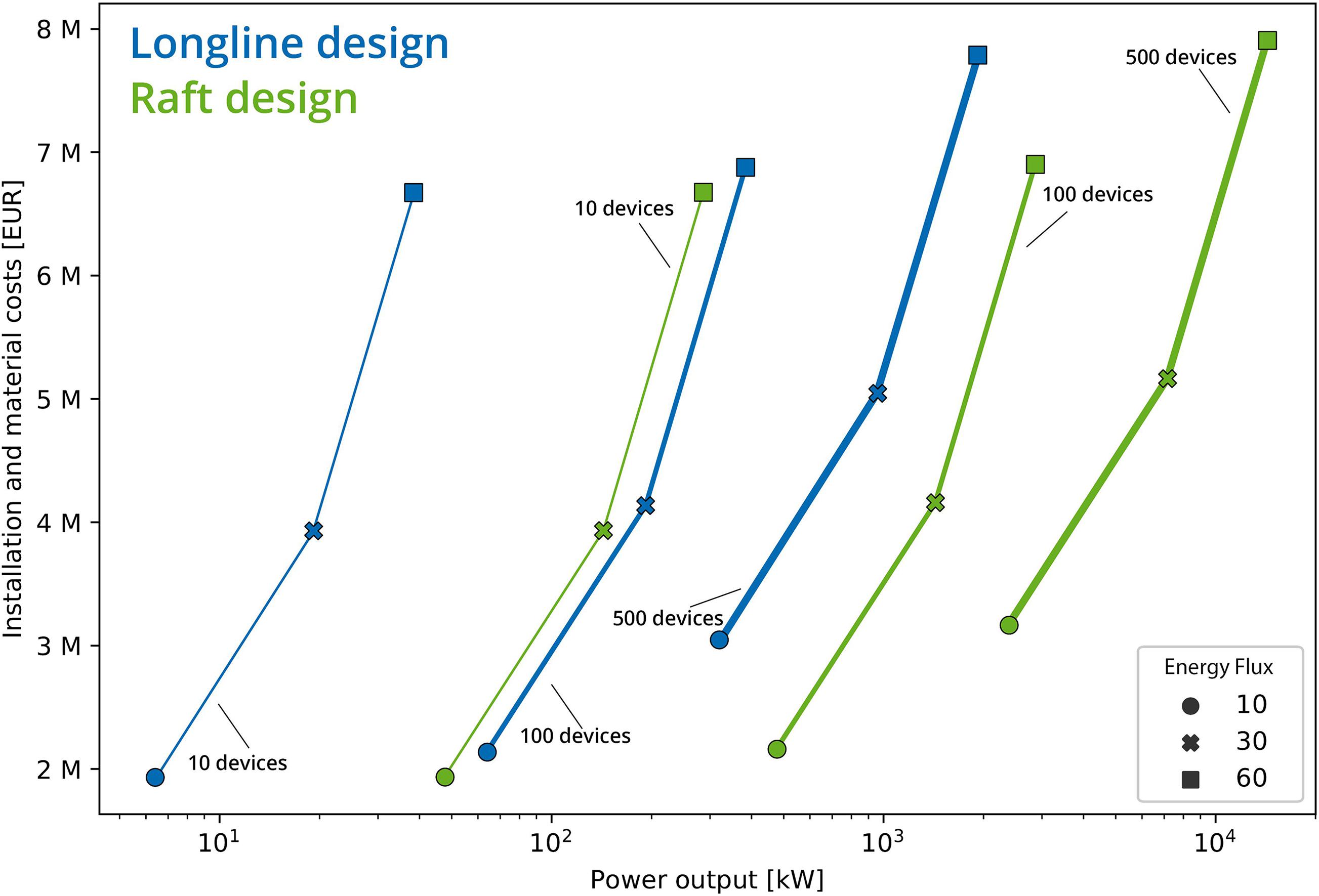
Figure 4. Installation costs per kilowatt values, depending on chosen primary capture system design (longline or raft) and number of installed devices (10, 100, or 500) represented through colours. Symbols show sensitivity of results for different energy flux assumptions (10, 30, and 60 kW/m). Installation costs are based on mean values of cable distances from the sensitivity analysis + assumption for deployment with vessel of 46,000 EUR. Power output categories are based on Babarit (2015).
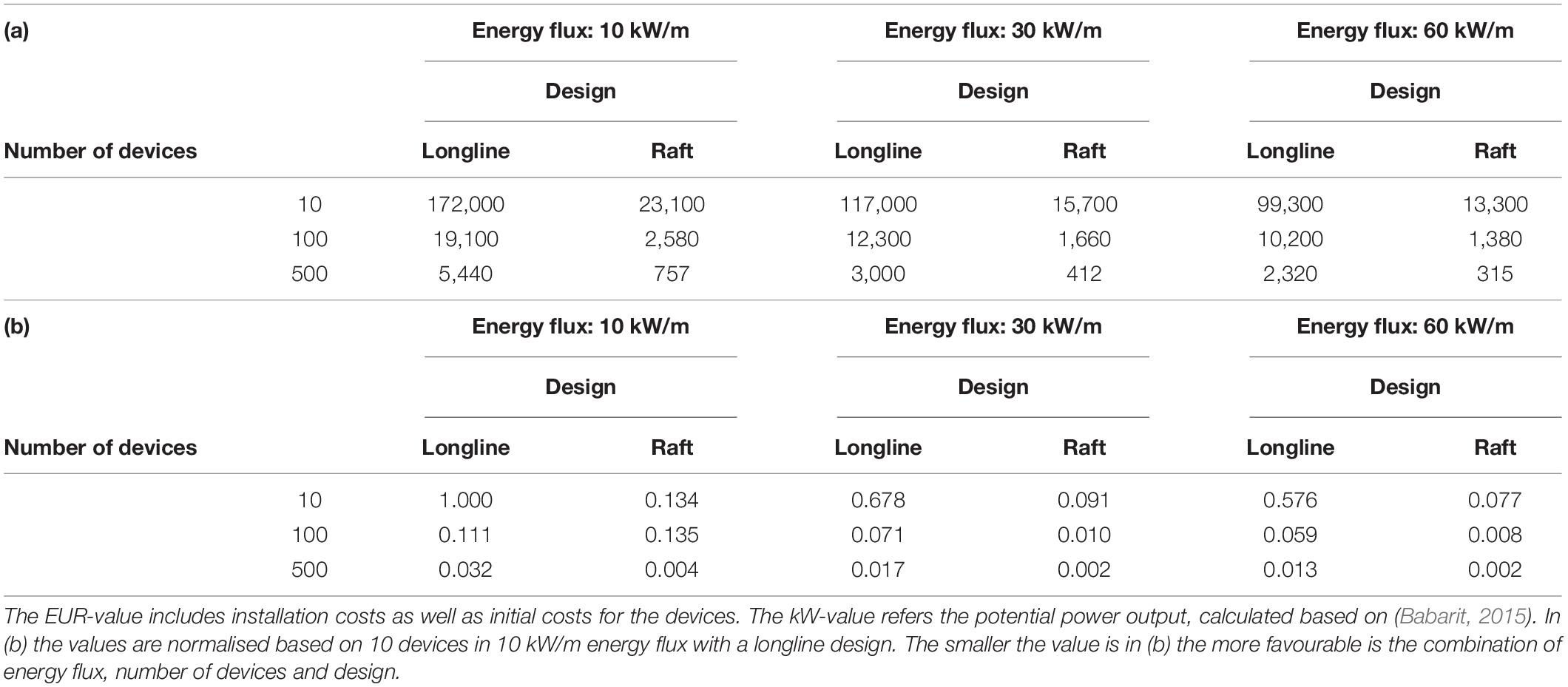
Table 4. The displayed EUR/kW values depend on energy flux (10, 30, 60 kW/m), number of devices (10-100-500) and chosen design (longline or raft).
While the initial costs for the raft as a primary capture design are around 10% higher than the costs for the longline design, the range of added costs—including material costs, cable installation and deployment—differs only marginally between the designs from 0.04 (10 devices, furthest offshore) to 3.75% (500 devices closest to shore). This is due to the installation costs constituting the most significant part of the total costs. Overall, the power output with the raft design is calculated to be 13.4% higher than the output of the longline system. The mean of the CWR of the heaving device group in Babarit (2015) is 16%. So with both of them being a heaving device with an assumed efficiency (CWR) of 16% but the wider capture width of the raft [3 m calculated with B = 2 × (7 m/π)1/2], assuming the raft to have a maximum characteristic dimension of 7 m, makes the raft more efficient because we assume the longline to only have a capture width of 0.4 m. The wider capture width of the rafts results in a higher efficiency. Therefore, the results suggest that the raft set-up is the favourable design for the application of this concept.
Skeleton Material
The combined environmental and financial costs of eight materials that are representative for the three groups, composites, natural materials and steel, show that the embodied energy for the primary production of natural materials reaches around 10% of the values for steel and glass fibre (Figure 5 and Table 5). Similar orders of magnitude are apparent with the CO2 footprint of the primary production. Compared to the manufactured material, the water usage of the natural materials is high. That is due to the values being calculated from life-cycle water consumption, including irrigation water requirements. The group of natural materials therefore needs approximately 11–15 times more water than the primary production of glass fibre and steel. Decisions based on local values will likely provide guidance for comparison of economic and environmental costs. For example, even while palm is inexpensive for the yield strength it provides, steel proves to be less expensive for the same yield. However, the extra weight of steel means that it has higher overall cost. In addition to the eight materials, costs were also calculated for a traditional configuration with only steel and no biostructure by assuming options of 2× and 5× mild steel weight to the Bio-Oscillator configuration (Figure 5 and Table 5).
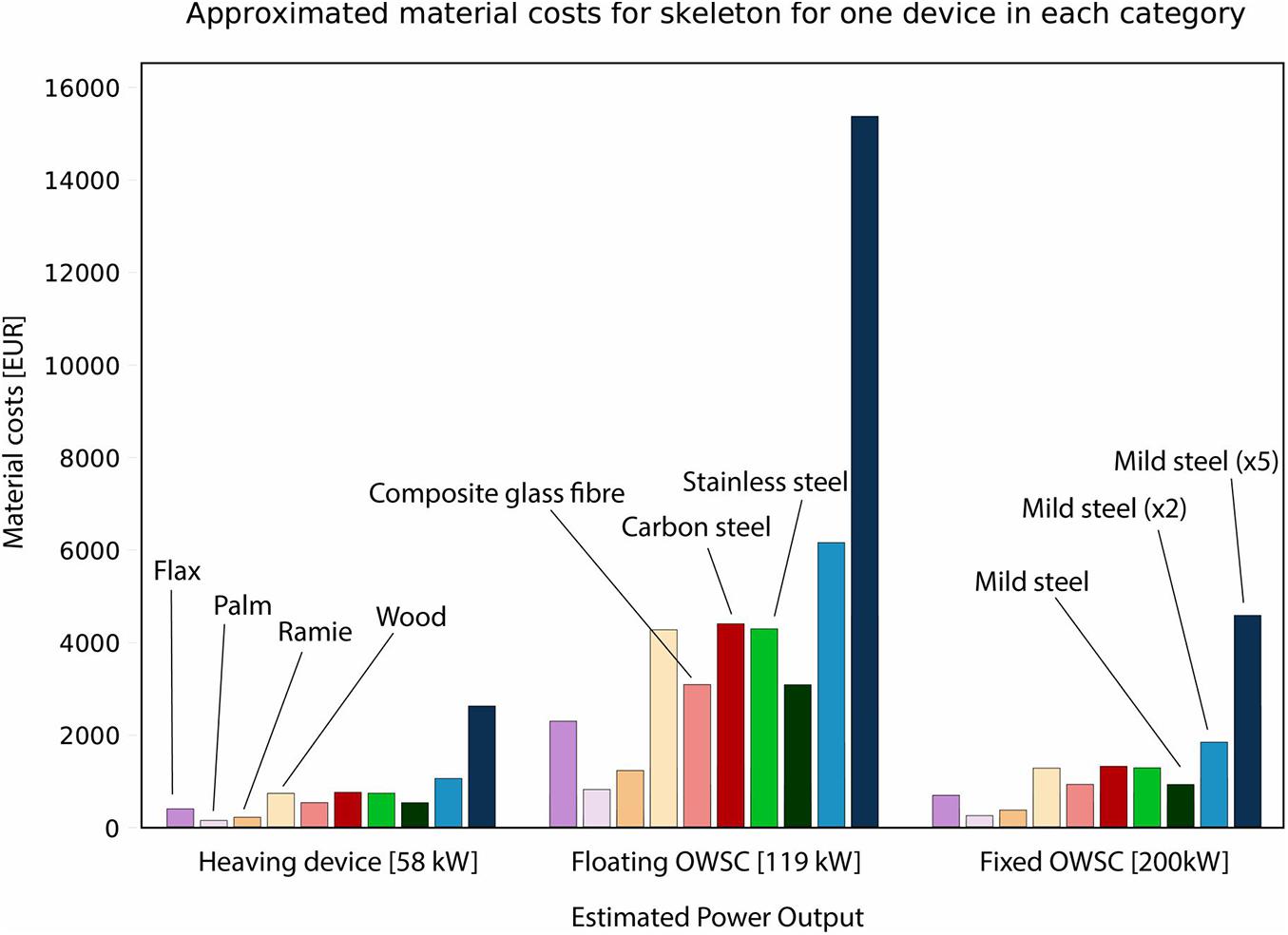
Figure 5. Material costs of skeleton for eight materials + mild steel versions with twice and five times the estimated amount (supposed to give an idea for costs of a comparable structure not using the Bio-Oscillator approach). From left to right the categories: heaving device, floating OWSC, fixed OWSC (OWSC = oscillating wave surge converter) with their power output calculated based on 30 kW/m energy flux. Average values of capture width ratios of device categories and equation to calculate the power output are taken from Babarit (2015). Values for the differing forces acting on individual devices were taken from Babarit et al. (2012) and depend on the category (heave force for heaving device, surge force for fixed and floating OWSC). This enables calculation of the necessary strength and amount of material and therefore costs.
Biological Organisms
At the selected locations, workable species options within the marine fauna and flora include macroalgae Macrocystis pyrifera (giant kelp) or Durvillaea Antarctica (bull kelp), and shellfish, such as Perna canaliculus (New Zealand mussel) or Pecten novaezelandiae (New Zealand scallop), Ostrea lutaria (dredge oyster), and Crassostrea gigas (Pacific Oyster). These all potentially lend themselves as crops to be harvested by existing coastal industries. Notably, M. pyrifera possesses gas-filled pneumatocysts that make it positively buoyant (Utter and Denny, 1996; Nelson, 2020). Similarly, D. Antarctica maintains a gas-filled honeycomb structure, offering also some positive buoyancy (Stevens et al., 2002). Conversely, shellfish crop grows in heavy, relatively inflexible lines (e.g., Stevens et al., 2007), which can provide a counterbalance.
Structural Configuration
Installation costs consider the expenditures for the deployment with the vessel with a set fee of 46,000 EUR. Averages of cable costs from the previous sensitivity analysis are used to estimate costs for the cables with three different energy fluxes (Figure 4). The mean value for cables in 10, 30, and 50 m depth provides the costs for installation assumed to be 10, 30, and 60 kW/m, respectively.
The analysis comparing material costs for the different device categories, i.e., heaving device, fixed and floating OWSC, shows that the potential power output does not directly correlate with the skeleton prices (Figure 5). This is a consequence of the different devices having different wave-structure interaction mechanics. For example, material costs for the assumed fixed OWSC skeleton device only reach 30% of the floating OWSC design, while the power output of the fixed OWSC device is 168% higher compared with the floating OWSC’s power output. The heaving device skeleton in the envisioned configuration costs 57% of the fixed OWSC equivalent, but also only produces ∼30% of the energy. Babarit (2015) groups existing devices and deriving mean values. The fixed OWSC have a higher efficiency but lower characteristic dimension therefore less material cost. The floating OWSC has a lower efficiency but higher mean characteristic dimensions and therefore higher material costs. These numbers only give magnitudes of costs and power outputs, since the efficiency estimates are based on Babarit (2015) for selected category of device. Nevertheless this survey suggests that, while the fixed OWSC category of device provides the best cost-benefit ratio, a heaving device has the lowest initial costs. Even though the selection of a suitable primary capture system can be based on cost of the device, the expenditures for the installation (such as submarine cables) are significantly higher than the initial costs for the device itself.
The costs for the skeleton in each category (Figure 5 and Table 5) are influenced by the characteristic dimension of the device category, as well as the effect of action that is crucial for the device category (either the heave or surge force). Depending on the category, different forces act on the device. Therefore, with 270 kN m–1, the effect of action for heaving devices is higher than the surge force (210 kN m–1) that presumably acts on fixed and floating OWSCs (Babarit et al., 2012). While approximate and design-dependent it does provide an indication of the sensitivity of result to device design.
Discussion
Viability of the Hybrid Approach
It is difficult to imagine a scenario where increased material usage does not involve extra costs. One of the few scenarios is that which we examine here where the mass is self-replicating. The simplest way to compare a traditional device configuration, with only steel and no biostructure, is to assume the biology is replaced with steel. The example here where we consider 2× and 5× scaling of mild steel weight to the Bio-Oscillator configuration shows a substantial increase of environmental costs for the skeleton (Table 5) and also increased direct prices (Figure 5). Mindful that the sensitivity of cost and energy production in the present CBA are considered primarily in a relative sense, this initial examination suggests there is merit in a biophysical hybrid approach to marine energy structures.
The capital expenditures that are considered in this CBA suggest the raft system approach is only between 0 and 4% more expensive than the longline setup but has a reasonable higher theoretically calculated energy output (13.4%). The economically most sensible solution based on the EUR kW–1 values is therefore a raft setup with a higher number of devices installed as an array (Table 3). These benefits would likely be extended by the integrated maricultural dimension of the bio-oscillator approach.
Being geographically distant from any major economies, New Zealand is not a typical market for most raw materials due to large shipping distances and high manufacturing prices. Considering that the evaluation of materials’ suitability for the Bio-Oscillator is conducted with values from the database CES EduPack (CES, 2016), whose numbers are often global averages, their country-specific accuracy may vary significantly. Notably, the price and carbon footprint for material production strongly depend on local processes. In addition, the overall CBA results inform further context-specific investigations in other settings, where the local conditions are favourable for certain materials, benefitting the financial and potential environmental costs associated to the respective deployment. In different settings with an equally high potential for wave energy, such as United Kingdom waters (Stegman et al., 2017), the financial costs for raw materials are likely to be significantly lower than reported here due to the proximity to much of the raw material production.
The costs incurred by laying the offshore cables are likely to constitute a significant part of the manufacturing, deployment and maintenance costs of a WEC (Kim et al., 2012). Therefore, the overall costs are largely dependent on the distances that must be bridged for the grid connection, which we assess in a sensitivity analysis of deployment depth and available onshore energy flux. Deployments of WECs always need to strike a balance between energy flux and installation costs, which do both correlate with depth and distance to shore, to some extent. While the correlation is initially apparent, it does not universally apply, as our analysis indicates. For example, the WAM hindcast indicates the onshore energy density at 30 m is higher than in 50 m of water depth for the Taranaki-2 sites. This is likely due to refraction resulting in values for energy flux that are not necessarily decreasing in a unidirectional fashion toward shore. Comparing location Taranaki-2 to Greymouth or Tiwai (Table 4), it is apparent that a deployment in deeper water increases the installation costs disproportionately. While the installation costs for the 50 m deep sites of Greymouth and Tiwai are 130–150% of their 30 m deployment cost, the further offshore site of Taranaki-2 (deep) reaches 210% of the installation costs compared to its 30 m deep site. Since the rise in available onshore energy flux is percentage-wise the same for all three locations (and in absolute numbers, lower for the Taranki-2 site), a deep-water deployment is not sensible there. Other factors, such as seabed conditions and water depth do significantly influence the difficulty of the installation of the submarine cable and hence cost but are not considered in the present analysis.
The type of power take-off used, while not the focus here, will ultimately be integrated into the design pathway. The wide range of options possible (Jusoh et al., 2019), especially through hydraulic transmission, allows significant choice with regard to biological-physical structural configuration. A number of options are included in Figure 6 showing selected configuration options showing (a) equivalent to Figure 1, (b) suspended shellfish, (c) moored kelp, (d) “Pelamis equivalent,” (e) heave buoy, and (f) hinged flap approaches. The most critical step being whether the take-off infrastructure is placed on the seabed (e.g., Gaspar et al., 2016) or integrated into the floating structure (e.g., Liu et al., 2017). The floating option is more easily accessed for maintenance but more vulnerable to storm damage. The raft design described here would be more suited to the floating hydraulic power take-off described in Liu et al. (2017) as the larger scale and more options for counter-rotation between large structural elements favour this approach. The seabed infrastructure approach should be suited to most situations but will be challenged in locations with strong uni-directional currents.
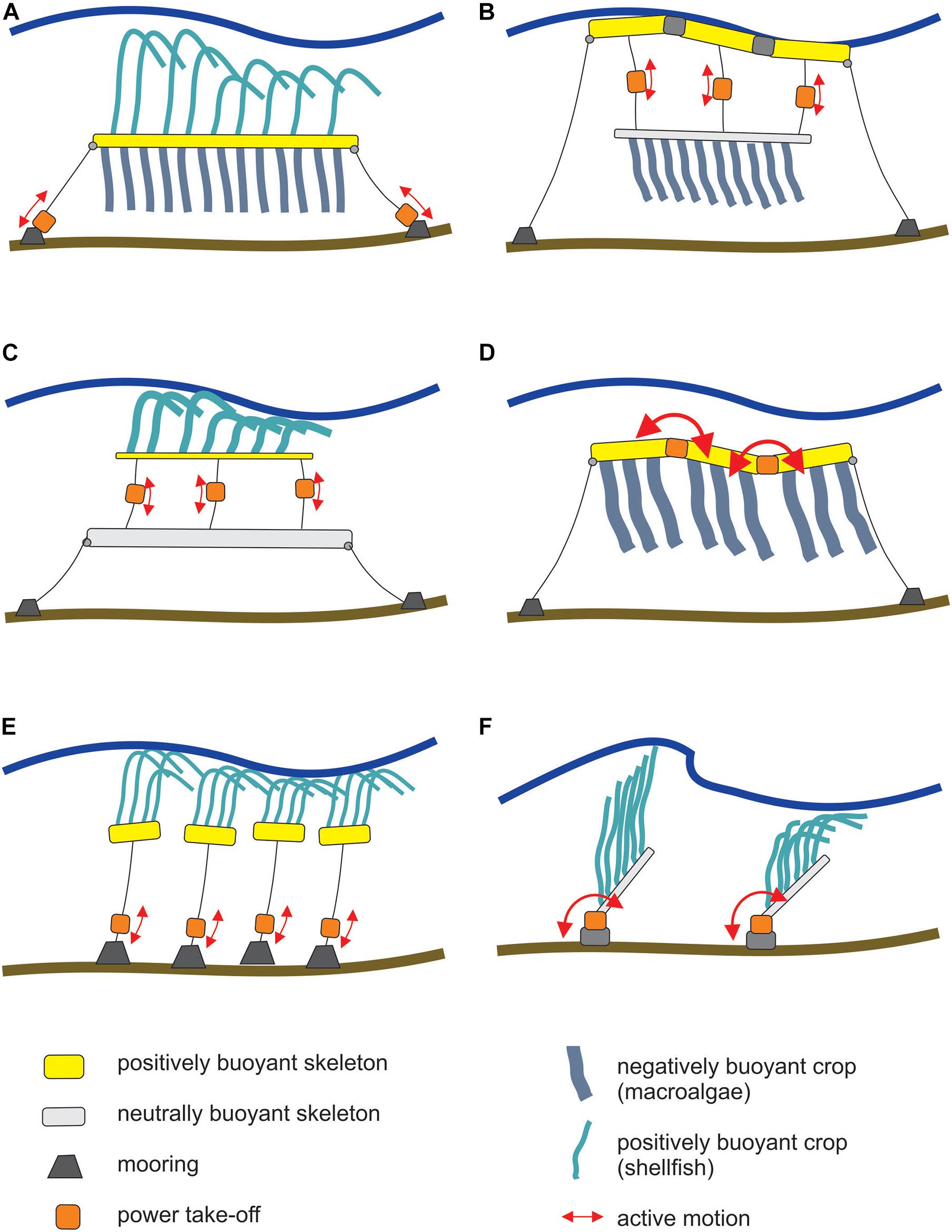
Figure 6. Potential configuration options showing (A) equivalent to Figure 1, (B) suspended shellfish, (C) moored macroalgae, (D) “Pelamis equivalent,” (E) heaving buoy, and (F) hinged flap approaches. As a visual aid, the amount of crop has been de-emphasized relative to the structural elements.
The CBA gives an indication as to which setups are economically more viable in the different sites. However, uncertainty of the device depreciation lifetime, economies of scale, as well as the primary capture system design, significant risk for investors remains. Currently, the life span of the device is, conservatively, assumed to be half that of terrestrial wind turbines, i.e., around 10 years (c.f. Martínez et al., 2009). However, the time dimension is not considered in the CBA due to lacking reference values for lifespans of the rope-float constructions and respective power take-off in a high wave energy, and therefore hostile, environment. The lifespan and thus investment costs are likely to differ between deployments exposed to higher energy densities further offshore and lower energy densities closer to shore. Simultaneously, larger deployments may affect the lifespan and efficiency of maintenance operations of the devices during and after high energy wave events. At the same time, economies of scale are likely to substantially lower production costs and large deployments would increase margins derived from aquaculture.
Local industries that traditionally harvest near-shore aquaculture have technical constraints in regard to distance from their landing site and bathymetry of the area which will narrow down the potential sites for deployment of a hybrid Bio-Oscillator approach. In this regard, future economic feasibility assessments should examine findings that suggest a substantially greater production rate (Cheney et al., 2010; Barillé et al., 2020) for off-shore shellfish aquaculture compared to onshore systems and in that regard may rely on three driving variables, i.e., sea surface temperature, chlorophyll-a concentration as a proxy for food availability, and suspended particulate matter concentration, as described in Barillé et al. (2020).
A key factor influencing how the CBA is viewed is the combined role of risk and impact of failure. The risk will be likely greater moving further offshore and would need to be offset by stronger construction, but typically offset by the ability to harvest more energy. The economics will presumably be further improved by installation of greater numbers of energy extractors, but this will have a compounding effect as one failure can cascade to take out many units. Ultimately, environmental impact due to structural failure is an important but under-reported metric and here, the role played by biology becomes apparent. The impact will be reduced by some amount that scales with the proportion of biology used if failure only introduces locally present organisms into the water and onto local beaches.
Co-benefits
The scale of farm size will be associated with ultimate usage. While substantial benefits might be possible at the utility scale there would also be applications at the smalls scale in support say of coastal infrastructure like navigation markers or aquaculture operations. An economically profitable off-shore energy and food production installation creates positive environmental outcomes as off-shore structures can function as additional habitats for targeted species—the so-called Blue Economy. As the demand for seafood rises worldwide, the lack of more localized production represents a lost opportunity for sustainability and economic growth (Lester et al., 2018). The approach is therefore well-positioned to create the necessary political and societal conditions to obtain suitable investment, regulatory, and political support.
The co-benefit of aquaculture is worth considering further. For example, three wave farms of a scale comparable to a large New Zealand 2.5 km × 0.5 km mussel farm, built using the proposed technology, potentially would have the combined capacity to replace a 250 MW coal/gas generator (i.e., around 150 M EUR yr–1). At the same time, aquaculture of that scale produces a harvest worth around 4 M EUR yr–1. Depending on the cost structure and the synergies around combined operation and maintenance, the aquaculture might form a modest component of the benefit at best. In addition, off-shore aquaculture in a high-energy environment, as proposed with the Bio-Oscillator, is ecologically more desirable compared to in-shore aquaculture, as among others, nutrients and faeces are not deposited to the same extent on the benthos due to greater dilution in open waters, hence reducing disturbance to existing benthic communities (Naylor and Burke, 2005; Black, 2008).
The ability of the present approach to support renewable energy hubs can be examined using the case of the Tiwai Point aluminium refinery. At the time of writing this is a major issue for the New Zealand national energy grid as the refinery is no longer economically viable and closing. The refinery was powered by the large Manapōuri hydroelectricity scheme which accounts for around 15% of the national energy production. This closure superficially points to a surfeit of energy. However, viewed from the point of view of a renewable energy hub there is the option to combine a range of renewable sources and develop low-impact, energy-intensive industries. Such initiatives could include pumped hydro storage, data centre developments, underwriting otherwise uneconomic long-distance transport of electricity to enable closure of fossil-sourced energy production (Gaston, 2020).
Any new, socially constructive, project needs to consider how its activities integrate in a global system dramatically affected by the Covid-19 pandemic. Initial reports (IEA, 2020) suggest that there is a dramatic short-term restructuring of energy usage patterns that largely favours renewable energy consumption. This is flanked by a shift toward more sustainably and locally sourced produce. However, construction of new renewable energy infrastructure is also dramatically affected as supply chains are slowed. Conversely, government stimulus packages have the potential to boost development if they look to renewable rather than fossil fuel resources.
Social Sensitivities
While the economic dimension discussed above is typically a leading priority for investors to assess feasibility of the Bio-Oscillator approach, the administrative constraints, societal sentiment and environmental considerations around large-scale offshore are also important dimensions to be assessed. The social license to operate for marine energy initiatives is well-explored (Whitmarsh and Palmieri, 2009; Kerr et al., 2014; Bonar et al., 2015), but not generally resolved. In the past, offshore renewable energy developments have been opposed due to a range of concerns about their cumulative impacts (Bonar et al., 2015; Copping et al., 2015), including the desire to generally avoid industrial development in the ocean (McLachlan, 2009; Devine-Wright, 2011), effects on marine life (Bailey et al., 2010; Devine-Wright, 2011), potentially adverse impacts on local fisheries (Conway et al., 2010; Devine-Wright, 2011), navigational safety (McLachlan, 2009) marine recreation (Firestone and Kempton, 2007), tourism (Warren et al., 2005; McLachlan, 2009), property values (Warren et al., 2005), and community harmony (Firestone and Kempton, 2007). For example, “Cornwall’s Wave Hub was opposed because some respondents feared a detrimental effect on local surfing conditions and a lack of compensation for displacement of the fishing industry from productive areas.” (Bonar et al., 2015).
A vulnerability of conventional WEC is their structural reliability being impaired by marine debris floating on the surface, including shipping containers or fishing gear, or destroyed due to high energy wave events. Any structural failure will result in man-made marine debris washing up on shores. The Bio-Oscillator mitigates these concerns, because during storms the biological attachment points, such as holdfasts for kelps or byssal threads for shellfish, function as preferential breaking points within the WEC, ideally breaking off during high-energy periods, reducing load on man-made parts and minimizing the risk of the WEC skeleton itself being harmed. Even catastrophic failure will only release the easily recoverable skeleton and its biodegradable growth.
In the N.Z. context, while not globally unique, there is a strong emphasis on involvement and consideration of Māori perspectives and interests based around the principals laid down in the Treaty of Waitangi (Cram et al., 2010). This has both economic and social threads. Economically, most coastal and marine industries have involvement or ownership by Māori commercial entities. Socially, there is a historical context for combining natural products with positive outcomes. For example, Māori historically used local flax for its material properties. By scraping the green flesh away from the flax leaves, a strong fibre called muka was extracted. By pounding, washing, twisting, plaiting and weaving the muka, it functioned as a base material for items used in a seawater environment, such as fishing nets and ropes (Wehi and Clarkson, 2007). These sorts of connections will be found in most coastal settings and help provide a perspective on the Bio-Oscillator approach.
Overall, technological advancements and current findings in regard to the economics of offshore aquaculture, the understanding of an inclusive and participatory approach to include stakeholders in the planning and implementation processes, as well as environmental considerations, make a broader and well-informed shift toward off-shore aquaculture seem feasible and reasonable. The hybrid Bio-Oscillator approach is designed to resolve problems of traditional WECs while integrating energy production with aquaculture, therefore creating new habitats that can serve as sanctuaries for intensively fished species, improving the resilience of nearby kelp-beds and through spill-over increase diversity and local fisheries’ catches.
Global Generalization and Future Research
While we have developed the idea in a N.Z.-context, it is worth considering how it translates globally in terms of waves, biology and a changing climate. At the large-scale, NZ has substantial wave energy resources, especially to the south west. This is unlikely to reduce with changing climate. However, there are many exposed coastlines globally with comparable wave metrics—some of which will be changing with climate (Godoi et al., 2016; Zheng et al., 2017).
While the sensitivity analysis favours the heaving device (raft) in terms of cost, power output is greater for the longline OWSC. Local conditions and circumstances, including material costs, topography or the type of envisioned crop, will determine which structural option is economically most favourable. In this regard and most importantly at this point, there is a substantial avenue for further research that assesses the compatibility and most effective ways to attach biological organism to the substrate and best practices for subsequent harvest and reseeding. This will affect the final economic assessment as it is likely that the OWSC enables greater options for biological material and the aquaculture sales and reduced environmental impact that come with this.
There are several factors that should be taken into consideration when selecting the most appropriate species to use within an offshore macroalgae-based system, such as its structural characteristics, productivity, and tolerance to a dynamic offshore environment and potential changes to existing ecosystems. While shellfish are well-equipped to survive dynamic offshore environments, the exact characteristics of the respective habitat, commercial demand, as well as the kind of artificial substrate best suited for their growth, may substantially influence financial feasibility of the device. The results here inform consideration around how the approach might actually be deployed and operated. There is logic to locating bare skeleton structures in a convenient near-shore environment and then installing them into the energy production location where sufficiently mature and at the same time recovering a fully cropped skeleton for harvest (Figure 7). This would result in more efficient use of vessels and more controlled husbandry processes.
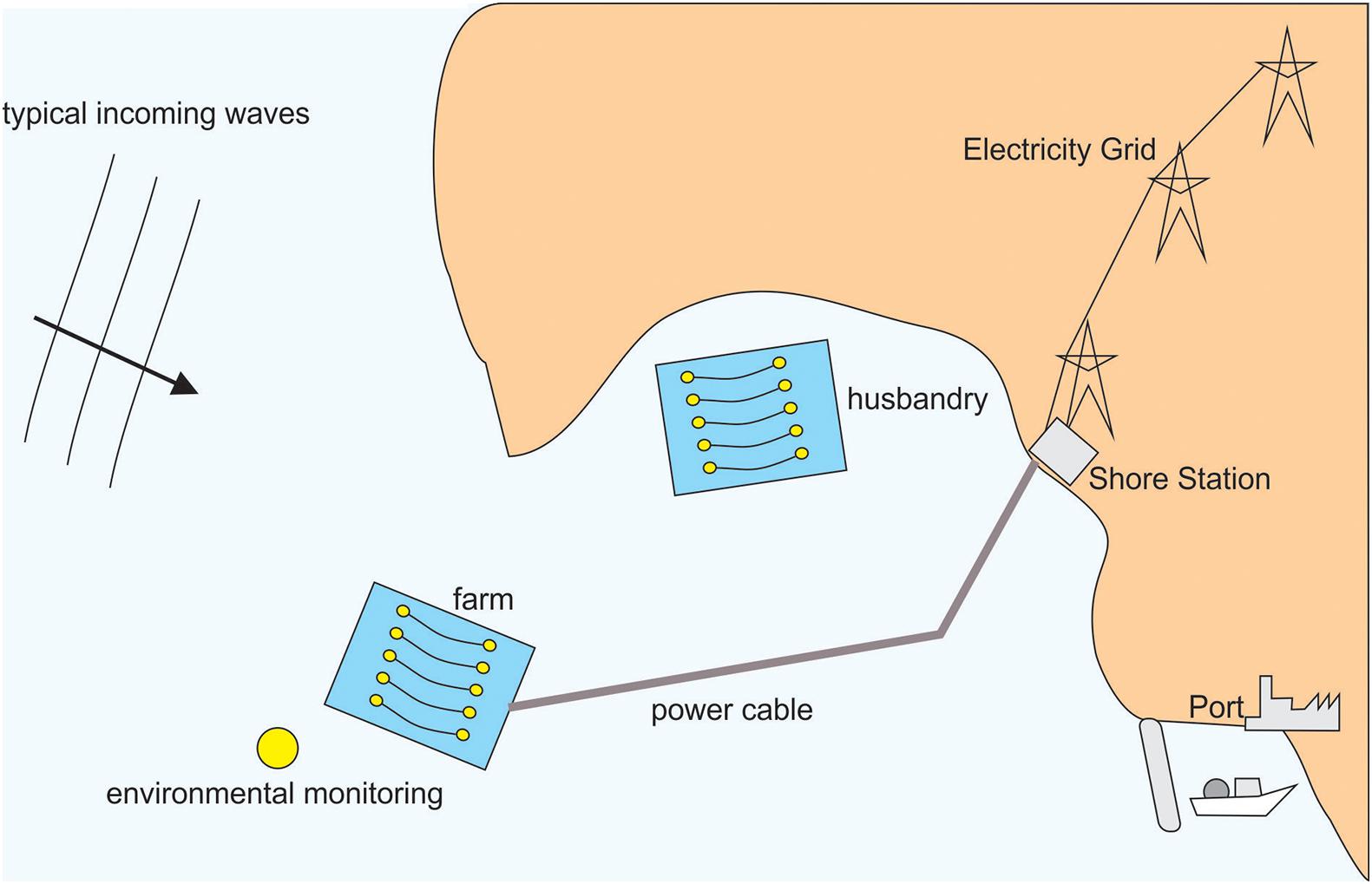
Figure 7. Regional arrangement with the farm and its monitoring best placed to access the typical wave field. In addition, husbandry of the crop can take place somewhere sheltered. All of this then needs to be within reach of both electricity (grid) and marine infrastructure (port).
Future work on the topic needs to consider a number of aspects are missing from the CBA including: the profits derived from aquaculture, the costs stemming from planning and stakeholder consultations, variability in husbandry costs for seeds and spat for the aquaculture, as well as the operation and maintenance expenditures that are necessary to keep the Bio-Oscillator processes running. The biophysical novelty at the heart of the Bio-Oscillator, while conceptually established here, needs to be verified. We recommend several primary threads of work. (i) A more detailed examination of potential adaptation of existing primary capture systems or device categories should support the selection of the most efficient one in terms of costs and benefits which could provide an additional thread to assessments such as Bertram et al. (2020). (ii) Parallel to this, a suitable power take-off should be selected to develop a precise concept for the design of the structure. (iii) The efficiency of the use of biological organisms to add drag and mass can be further investigated, as well as the storm survivability of the device, which incorporates developing effective ways to attach the biological organisms to the chosen substrates. (iv) Based on these designs, scaled testing can be embarked upon.
Data Availability Statement
The original contributions presented in the study are included in the article/Supplementary Material, further inquiries can be directed to the corresponding author/s.
Author Contributions
TH developed the economic analysis. CF developed the socio-economic context and structural configuration designs. VR developed the biological survey. RG produced the wave forcing. CS developed the initial concept and led the project. TH, CF, VR, and CS wrote the manuscript. All authors contributed to the article and approved the submitted version.
Funding
Funding came from the New Zealand Ministry of Business, Innovation and Employment Strategic Science Investment Fund via the National Institute of Water and Atmospheric Research Marine Environment Platform. MER-Consortium and the Erasmus Mundus initiative of the European Union provided some financial support. The 45-year wave hindcast was conducted under the scope of the “Wave and Storm-Surge Projections” (WASP) Project, funded through the New Zealand Ministry of Science and Innovation, contract C01X0806.
Conflict of Interest
CF was employed by the company Oxenu LLP.
The remaining authors declare that the research was conducted in the absence of any commercial or financial relationships that could be construed as a potential conflict of interest.
Acknowledgments
The authors would like to thank Neil Barr, Wendy Nelson, Milla Antilla, Roberta D’Archino, William Quinn, Gareth Gretton, Craig Stewart, Jon Trueblood, and Alex Mignucci for their help in the field, laboratory, analysis, and with wider discussions. In addition, we acknowledge the efforts of two reviewers to help improve the manuscript. TH wishes to acknowledge her home University supervisors Kai-Uwe Graw and Matthias Brockel. This manuscript is dedicated to the memory of John Huckerby who did much to support the field of marine energy in Aotearoa New Zealand.
Supplementary Material
The Supplementary Material for this article can be found online at: https://www.frontiersin.org/articles/10.3389/fmars.2021.628148/full#supplementary-material
References
Babarit, A. (2015). A database of capture width ratio of wave energy converters. Renew. Ener. 80, 610–628. doi: 10.1016/j.renene.2015.02.049
Babarit, A., Hals, J., Muliawan, M. J., Kurniawan, A., Moan, T., and Krokstad, J. (2012). Numerical benchmarking study of a selection of wave energy converters. Renewable Energy 41, 44–63. doi: 10.1016/j.renene.2011.10.002
Bailey, H., Senior, B., Simmons, D., Rusin, J., Picken, G., and Thompson, P. M. (2010). Assessing underwater noise levels during pile-driving at an offshore windfarm and its potential effects on marine mammals. Mar. Pollut. Bull. 60, 888–897. doi: 10.1016/j.marpolbul.2010.01.003
Barillé, L., Le Bris, A., Goulletquer, P., Thomas, Y., Glize, P., Kane, F., et al. (2020). Biological, socio-economic, and administrative opportunities and challenges to moving aquaculture offshore for small French oyster-farming companies. Aquaculture 521:735045. doi: 10.1016/j.aquaculture.2020.735045
Bertram, D. V., Tarighaleslami, A. H., Walmsley, M. R. W., Atkins, M. J., and Glasgow, G. D. E. (2020). A systematic approach for selecting suitable wave energy converters for potential wave energy farm sites. Renew. Sustain. Ener. Rev. 132:110011. doi: 10.1016/j.rser.2020.110011
Black, K. D. (2008). Environmental Aspects of Aquaculture: Aquaculture, Innovation and Social Transformation. Dordrecht: Springer, 97–113.
Bleck, W., and Moeller E. (eds.) (2017). Handbuch Stahl: Auswahl, Verarbeitung, Anwendung. Munich: Carl Hanser Verlag GmbH Co KG.
Bonar, P. A., Bryden, I. G., and Borthwick, A. G. (2015). Social and ecological impacts of marine energy development. Renew. Sustain. Energy Rev. 47, 486–495. doi: 10.1016/j.rser.2015.03.068
Buck, B. H., Ebeling, M. W., and Michler-Cieluch, T. (2010). Mussel cultivation as a co-use in offshore wind farms: potential and economic feasibility. Aquacult. Econom. Manag. 14, 255–281. doi: 10.1080/13657305.2010.526018
Cheney, D., Langan, R., Heasman, K., Friedman, B., and Davis, J. (2010). Shellfish culture in the open ocean: lessons learned for offshore expansion. Mar. Technol. Soc. J. 44, 55–67. doi: 10.4031/mtsj.44.3.6
Conway, F., Stevenson, J., Hunter, D., Stefanovich, M., Campbell, H., Covell, Z., et al. (2010). Ocean space, ocean place: the human dimensions of wave energy in Oregon. Oceanography 23, 82–91. doi: 10.5670/oceanog.2010.47
Copping, A., Hanna, L., Van Cleve, B., Blake, K., and Anderson, R. M. (2015). Environmental risk evaluation system—an approach to ranking risk of ocean energy development on coastal and estuarine environments. Estuar. Coasts 38, 287–302. doi: 10.1007/s12237-014-9816-3
Cram, F., Prendergast, T. A., Taupo, K., Phillips, H., and Parsons, M. (2010). “Traditional knowledge and decision-making: maori involvement in aquaculture and biotechnology,” in Proceedings of the Traditional Knowledge Conference (2008) Te Tatau Pounamu: The Greenstone Door Auckland: Te Pae o te Maramatanga, Auckland, 147–157.
Dalton, G. J., Alcorn, R., and Lewis, T. (2010). Case study feasibility analysis of the Pelamis wave energy convertor in Ireland, Portugal and North America. Renew. Ener. 35, 443–455. doi: 10.1016/j.renene.2009.07.003
Devine-Wright, P. (2011). Enhancing local distinctiveness fosters public acceptance of tidal energy: a UK case study. Ener. Policy 39, 83–93. doi: 10.1016/j.enpol.2010.09.012
Finnigan, T., and Caska, A. (2006). “Simulation of a biomimetic ocean wave energy device using blade-element theory,” in Proceedings of the Sixteenth International Offshore and Polar Engineering Conference. International Society of Offshore and Polar Engineers, San Francisco, CA.
Firestone, J., and Kempton, W. (2007). Public opinion about large offshore wind power: underlying factors. Ener. Policy 35, 1584–1598. doi: 10.1016/j.enpol.2006.04.010
Gaspar, J. F., Calvário, M., Kamarlouei, M., and Soares, C. G. (2016). Power take-off concept for wave energy converters based on oil-hydraulic transformer units. Renew. Ener. 86, 1232–1246. doi: 10.1016/j.renene.2015.09.035
Gaston, N. (2020). Five Alternatives to Aluminum at Tiwai. MacDiarmid Institute. Available online at: https://www.macdiarmid.ac.nz/news-and-events/news/in-the-media/five-alternatives-to-aluminium-at-tiwai/ (accessed August 25, 2020).
Gielen, D., Boshell, F., Saygin, D., Bazilian, M. D., Wagner, N., and Gorini, R. (2019). The role of renewable energy in the global energy transformation. Ener. Strat. Rev. 24, 38–50. doi: 10.1016/j.esr.2019.01.006
Godoi, V. A., Bryan, K. R., and Gorman, R. M. (2016). Regional influence of climate patterns on the wave climate of the southwestern Pacific: the New Zealand region. J. Geophys. Res. Oceans 121, 4056–4076. doi: 10.1002/2015jc011572
Gorman, R. M., Bryan, K. R., and Laing, A. K. (2003). Wave hindcast for the New Zealand region: nearshore validation and coastal wave climate. N. Z. J. Mar. Freshw. Res. 37, 567–588. doi: 10.1080/00288330.2003.9517190
Gunn, K., and Stock-Williams, C. (2012). Quantifying the global wave power resource. Renew. Ener. 44, 296–304. doi: 10.1016/j.renene.2012.01.101
Henriques, J. C. C., Gomes, R. P. F., Gato, L. M. C., Falcão, A., Robles, E., and Ceballos, S. (2016). Testing and control of a power take-off system for an oscillating-water-column wave energy converter. Renew. Ener. 85, 714–724. doi: 10.1016/j.renene.2015.07.015
Hsiao, S. V., and Shemdin, O. H. (1978). “Bottom dissipation in finite-depth water waves,” in Proceedings of the 16th Coastal Engineering Conference, Hamburg, 434–448.
IEA (2020). The Covid-19 Crisis and Clean Energy Progress, International Energy Association Report. Available online at: https://www.iea.org/reports/the-covid-19-crisis-and-clean-energy-progress (accessed September 1, 2020).
Jusoh, M. A., Ibrahim, M. Z., Daud, M. Z., Albani, A., and Mohd Yusop, Z. (2019). Hydraulic power take-off concepts for wave energy conversion system: a review. Energies 12:4510. doi: 10.3390/en12234510
Kerr, S., Watts, L., Colton, J., Conway, F., Hull, A., Johnson, K., et al. (2014). Establishing an agenda for social studies research in marine renewable energy. Ener. Policy 67, 694–702. doi: 10.1016/j.enpol.2013.11.063
Kim, C. K., Toft, J. E., Papenfus, M., Verutes, G., Guerry, A. D., Ruckelshaus, M. H., et al. (2012). Catching the right wave: evaluating wave energy resources and potential compatibility with existing marine and coastal uses. PLoS One 7:e47598. doi: 10.1371/journal.pone.0047598
Kootsookos, A., and Mouritz, A. P. (2004). Seawater durability of glass-and carbon-polymer composites. Compos. Sci. Technol. 64, 1503–1511. doi: 10.1016/j.compscitech.2003.10.019
Lester, S. E., Gentry, R. R., Kappel, C. V., White, C., and Gaines, S. D. (2018). Opinion: offshore aquaculture in the United States: untapped potential in need of smart policy. Proc. Natl. Acad. Sci. U.S.A. 115, 7162–7165. doi: 10.1073/pnas.1808737115
Liu, C., Yang, Q., and Bao, G. (2017). Performance investigation of a two-raft-type wave energy converter with hydraulic power take-off unit. Appl. Ocean Res. 62, 139–155. doi: 10.1016/j.apor.2016.12.002
Martínez, E., Sanz, F., Pellegrini, S., Jiménez, E., and Blanco, J. (2009). Life cycle assessment of a multi-megawatt wind turbine. Renew. Ener. 34, 667–673. doi: 10.1016/j.renene.2008.05.020
McLachlan, C. (2009). ‘You don’t do a chemistry experiment in your best china’: symbolic interpretations of place and technology in a wave energy case. Ener. Policy 37, 5342–5350. doi: 10.1016/j.enpol.2009.07.057
Naylor, R., and Burke, M. (2005). Aquaculture and ocean resources: raising tigers of the sea. Ann. Rev. Environ. Resour. 30, 185–218. doi: 10.1146/annurev.energy.30.081804.121034
Sallih, K. B., and þórðarson, J. (2005). Mussel Farming in the State of Sarawak, Malaysia: a Feasibility Study. Reykjavic: The United Nations University, UNU Fisheries Training Programme.
Sergiienko, N. Y., Rafiee, A., Cazzolato, B. S., Ding, B., and Arjomandi, M. (2018). Feasibility study of the three-tether axisymmetric wave energy converter. Ocean Eng. 150, 221–233. doi: 10.1016/j.oceaneng.2017.12.055
Sheng, W. (2019). Wave energy conversion and hydrodynamics modelling technologies: a review. Renew. Sustain. Ener. Rev. 109, 482–498. doi: 10.1016/j.rser.2019.04.030
Stegman, A., De Andres, A., Jeffrey, H., Johanning, L., and Bradley, S. (2017). Exploring marine energy potential in the UK using a whole systems modelling approach. Energies 10:1251. doi: 10.3390/en10091251
Stevens, C. L., Hurd, C. L., and Smith, M. J. (2001). Water motion relative to subtidal kelp fronds. Limnol. Oceanogr. 46, 668–678. doi: 10.4319/lo.2001.46.3.0668
Stevens, C. L., Hurd, C. L., and Smith, M. J. (2002). Field measurement of the dynamics of the bull kelp Durvillaea antarctica (Chamisso) Heriot. J. Exp. Mar. Biol. Ecol. 269, 147–171. doi: 10.1016/s0022-0981(02)00007-2
Stevens, C. L., Plew, D. R., Smith, M. J., and Fredriksson, D. W. (2007). Hydrodynamic forcing of long-line mussel farms: observations. J. Waterw. Port Coastal Ocean Eng. 133, 192–199. doi: 10.1061/(ASCE)0733-950X2007133:3(192)
Stevens, C., Plew, D., Hartstein, N., and Fredriksson, D. (2008). The physics of open-water shellfish aquaculture. Aquacult. Eng. 38, 145–160. doi: 10.1016/j.aquaeng.2008.01.006
Utter, B., and Denny, M. (1996). Wave-induced forces on the giant kelp Macrocystis pyrifera (Agardh): field test of a computational model. J. Exp. Biol. 199, 2645–2654.
Warren, C. R., Lumsden, C., O’Dowd, S., and Birnie, R. V. (2005). ‘Green on green’: public perceptions of wind power in Scotland and Ireland. J. Environ. Plan. Manag. 48, 853–875. doi: 10.1080/09640560500294376
Wehi, P. M., and Clarkson, B. D. (2007). Biological flora of New Zealand 10, Phormium tenax, harakeke, New Zealand flax. N. Z. J. Bot. 45, 521–544. doi: 10.1080/00288250709509737
Whitmarsh, D., and Palmieri, M. G. (2009). Social acceptability of marine aquaculture: The use of survey-based methods for eliciting public and stakeholder preferences. Mar. Policy 33, 452–457. doi: 10.1016/j.marpol.2008.10.003
Young, P. A. (2011). The Power of Many? Linked Wave Energy Point Absorbers. (Master of Science thesis, University of Otago, Dunedin.
Keywords: wave-structure interaction, marine energy converter, aquaculture, macroalgae, renewable energy, blue economy
Citation: Hildebrand T, Fischer C, Relano V, Gorman R and Stevens C (2021) Cost-Benefit Analysis of a Hybrid Biophysical Approach to Wave Energy Extraction: Bio-Oscillator. Front. Mar. Sci. 8:628148. doi: 10.3389/fmars.2021.628148
Received: 11 November 2020; Accepted: 24 March 2021;
Published: 15 April 2021.
Edited by:
Lars Johanning, University of Exeter, United KingdomReviewed by:
Shun-Han Yang, Chalmers University of Technology, SwedenSheng Xu, Universidade de Lisboa, Portugal
Copyright © 2021 Hildebrand, Fischer, Relano, Gorman and Stevens. This is an open-access article distributed under the terms of the Creative Commons Attribution License (CC BY). The use, distribution or reproduction in other forums is permitted, provided the original author(s) and the copyright owner(s) are credited and that the original publication in this journal is cited, in accordance with accepted academic practice. No use, distribution or reproduction is permitted which does not comply with these terms.
*Correspondence: Craig Stevens, Y3JhaWcuc3RldmVuc0BuaXdhLmNvLm56