- Oregon Institute of Marine Biology, University of Oregon, Charleston, OR, United States
Tidal currents flowing over benthic relief (e.g., banks, shelf break) can produce large internal waves. These waves propagate away from their origin and are capable of crossing the continental shelf and seas. Studies of shoreward transport of larval invertebrates and fish by these internal waves unintentionally tested whether they can capture, concentrate and transport floating plastic. Plastic surface drifters deployed in front of sets of internal wave convergences were often captured (>90% captured) and transported kilometers by the waves. There are, however, few investigations into how internal tidal waves may affect the fate and distribution of floating plastic waste. A number of areas of future research are suggested: (1) How much floating plastic is found in internal wave convergences? (2) How buoyant must floating plastic be to be captured by internal waves? (3) Why did only some sets of internal waves cause concentration and transport of surface material? (4) Do concentration and transport of floating plastic vary over the spring/neap tidal cycle? (5) Do seasonal changes in the depth of the pycnocline alter the transport of floating plastic by internal waves? (6) Plastic debris deposited on shore may not be evenly distributed, but may be more abundant landward of sites on the shelf break that more readily generate large internal waves. (7) Internal waves that travel long distances (10–100 s of km) have the potential to accumulate large amounts of plastic debris. (8) At locations where internal waves cross the continental shelf, how far offshore does transport commence?
Introduction
Buoyant material and organisms, e.g., floating seaweed, woody debris, plastic, buoyant, or hydrophobic pollutants and surface oriented zooplankton can become concentrated in convergence zones, areas in a body of water where surface currents converge. Surface convergence zones are generated by diversity of mechanisms, which have the capacity to concentrate floating plastic. An extensive review of this topic is presented in van Sebille et al. (2020), hence, I will only briefly review the topic here. (1) Wind blowing on water produces Langmuir circulation (Leibovich, 1983). In Langmuir circulation, the surface of the water (fresh or salt) is covered with clockwise and counter clockwise rotating helical currents oriented in the direction in which the wind is blowing. Where a pair of helical currents meet, a convergence zone is produced, which is capable of concentrating floating material or surface oriented animals (Hamner and Schneider, 1986). (2) At the mouth of estuaries during falling tides, a plume of lower salinity water is pushed out to sea. The plume pushes against the surrounding ocean water and where the two water masses meet a convergence zone is frequently present (Bowman and Esaias, 1978). These convergence zones are often ephemeral changing with the tides and seasonally with variation in runoff from land. (3) During active wind driven upwelling, a front often forms between the recently upwelled water and the lower density water pushed offshore by Ekman transport (Bowman and Esaias, 1978). When upwelling favorable winds weaken or reverse direction, the lower density water propagates back toward shore as a gravity current (Shanks et al., 2000). The convergence zone moves back toward shore as part of the hydrodynamics of the gravity current and this moving convergence zone has been observed to concentrate and transport surface oriented zooplankton (Shanks et al., 2000). (4) A similar convergent front, a tidal mixing front, is often formed in shallow seas (Pingree et al., 1974). Here turbulence from the wind and tidal currents completely mix the water column on the shallow side of the front. The front is thus the zone of separation between a mixed water column on one side and a stratified water column on the other: The location of the front changes with the spring to neap tidal cycle. (5) Where water masses with differing physical characteristics (i.e., salinity and temperature) meet, a convergent front often is observed. These types of fronts likely do not generate directional transport of the material concentrated in the convergence (Bowman and Esaias, 1978). Across a range of spatial scales, flow in the ocean is turbulent. (6) At larger scales (10 s of meters to kilometers) the swirling flow (eddies) can generate convergence zones known as Lagragian coherent structures (LCS) (Reniers et al., 2010). Eddies at this scale tend to move with the current in which they are imbedded and change and evolve with time, hence, the associated LCS convergence zones, while capable of capturing floating material including plastics (Gove et al., 2019), are ephemeral.
The tides, by generating large internal waves, are the source of one of the most common types of convergence zones. Tidal currents flowing over the continental shelf break or over relief (e.g., banks and reefs) generate large, meters to tens of meters in amplitude, non-linear internal waves (solitons) (Kao et al., 1985; Hibiya, 1990). Tidally generated internal waves (note to save space I will often write just internal waves), also known as the internal tide, are present on all continental shelves, around many islands and within many seas (see the Internal Wave Atlas)1. Tidally generated internal waves are generated at each change of the tide, hence, they are ubiquitous and common (Helfrich and Melville, 2006; Woodson, 2018). Surface currents over these internal waves produce convergence zones, which move with the internal waves. In the following I present a description of tidally generated internal waves, their oceanography, and data demonstrating that the convergence zones over large internal waves have the capacity to capture, concentrate and transport floating plastic debris, i.e., the internal wave convergences have the capacity to accumulate dispersed plastic and carry it along with the moving convergence.
Tidally generated internal waves and the internal tides are an active area of research in physical oceanography with an extensive literature. The purpose of the article presented here is not to review this literature, but to review experiments that tested if floating plastic could be caught and transported by tidally generated internal wave convergences. For an introduction into the oceanography of tidally generated internal waves there is an entire issue of Oceanography (the journal of the Oceanography Society, Vol 25, #2, June 2012) devoted to the subject and the web site Internal Wave Atlas (see text footnote 1) has numerous images of internal waves from around the world and articles on internal waves. In addition, there have been reviews written on the topic (Helfrich and Melville, 2006; Woodson, 2018).
When tidal currents flow over large benthic relief (e.g., the shelf break or banks and reefs), an internal lee wave is formed, that is, an underwater wave is formed on the downstream side of the relief. When tidal currents change direction (e.g., ebb to flood) the lee wave propagates away from its point of origin and evolves from a single lee wave into a set or train of many waves. The number of waves within a set varies with the density structure of the water column (Brunt-Vasalla frequency). Waves generated at the continental shelf break propagate across the shelf toward the coast (Howell and Brown, 1985) while those generated over banks and reefs can travel for hundreds of kilometers across seas (Apel et al., 1985). The surface manifestations of tidally generated internal waves are visible from space. The surface currents over the waves and the bands of smooth water (slicks) often associated with the convergences zones cause variations in sea surface roughness, which are visible in both visible and radar images taken from space (Apel et al., 1975). Wave sets are formed at the change of the tide each day and propagate at around 20–60 cm/s over the continental shelf (slower in shallower water) and at 1–2 m/s in deeper water. When sets propagate across a sea or wide shelf, multiple sets can be seen in satellite images, each set formed at the change of a tide (Figure 1, see also the Internal Wave Atlas (see text footnote 1). In deep water internal waves can travel for days before they encounter a shoreline and break. Waves formed at the continental shelf break and propagating toward shore can cross the entire shelf and, hence, their duration is dependent on the width of the shelf but is on the order of hours to days.
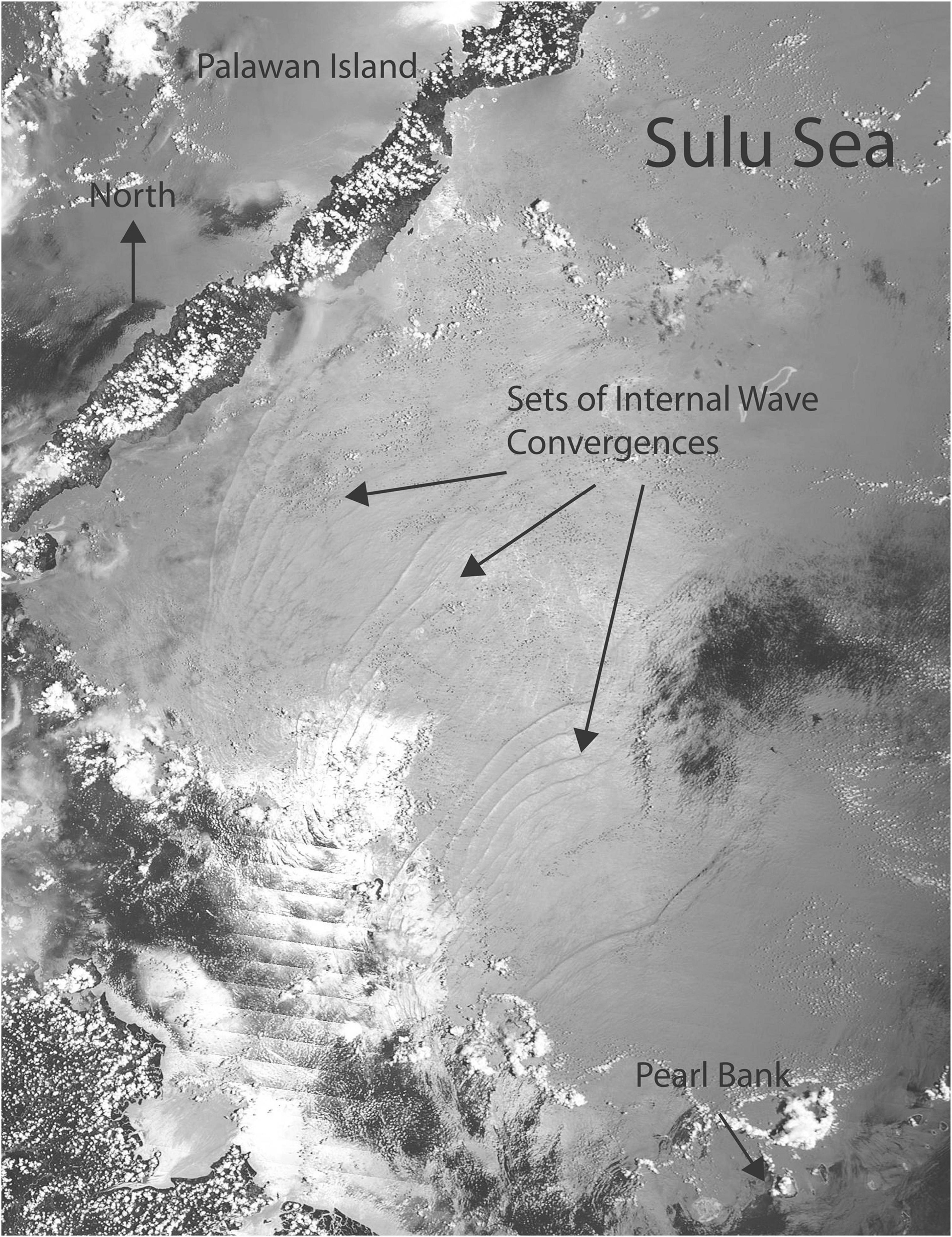
Figure 1. Satellite image of internal wave convergences in the Sulu Sea. The sets of internal waves were formed by the interaction of tidal currents with the Pearl Bank and adjacent reefs (5.85 N, 119.85 E). From this point of origin the waves propagate across the Sulu Sea (about 450 km) and, ultimately, run ashore on Palawan Island. Each set of waves was formed during a tidal change, hence, the set of waves closest to Palawan Island had been propagating across the Sulu Sea for about 2 days. Image is from the Internal Wave Atlas (http://internalwaveatlas.com/Atlas2_PDF/IWAtlas2_Pg393_SuluSea2.pdf) and is a compilation of MODIS Bands 1, 3, and 4. The vertical axis of the image is approximately 500 km.
As internal waves move into shoaling water, they can break forming an internal bore. These are capable of transported water and associated zooplankton within the bore (Pineda, 1995; Leichter et al., 1998), however, as these are subsurface currents, they likely do not transport buoyant plastic debris, which would have a surface distribution. The topic of this review is the concentration and transport of floating plastic debris.
Currents over the waves generate surface convergence zones that travel with the wave (Ewing, 1950; da Silve et al., 1998). Initially, researchers described these internal waves as linear waves similar to simple surface waves (Sverdrup et al., 1942). Linear waves should not cause substantial transport (i.e., horizontal movement) of material caught in the convergence zone, as the material would remain in the convergence only briefly (Ewing, 1950). Later research demonstrated that the waves were in fact non-linear waves or solitons (Osborne and Burch, 1980) and this form of wave can trap and transport material within the convergence moving with the wave (Shanks, 1983; Lamb, 1997). Over an internal wave of depression (i.e., the wave dips downward, Figure 2), surface flow is from the trailing edge of the wave toward the leading edge (Figure 3) where the flow turns downward forming a convergence zone at the leading edge of the wave. This convergence zone moves with the wave. As is typical with convergence zones, floating material can be transporting by the surface current into the convergence zone where, under the right conditions (discussed below), they can become trapped and transported along with the set of internal waves and their associated surface convergence zones.
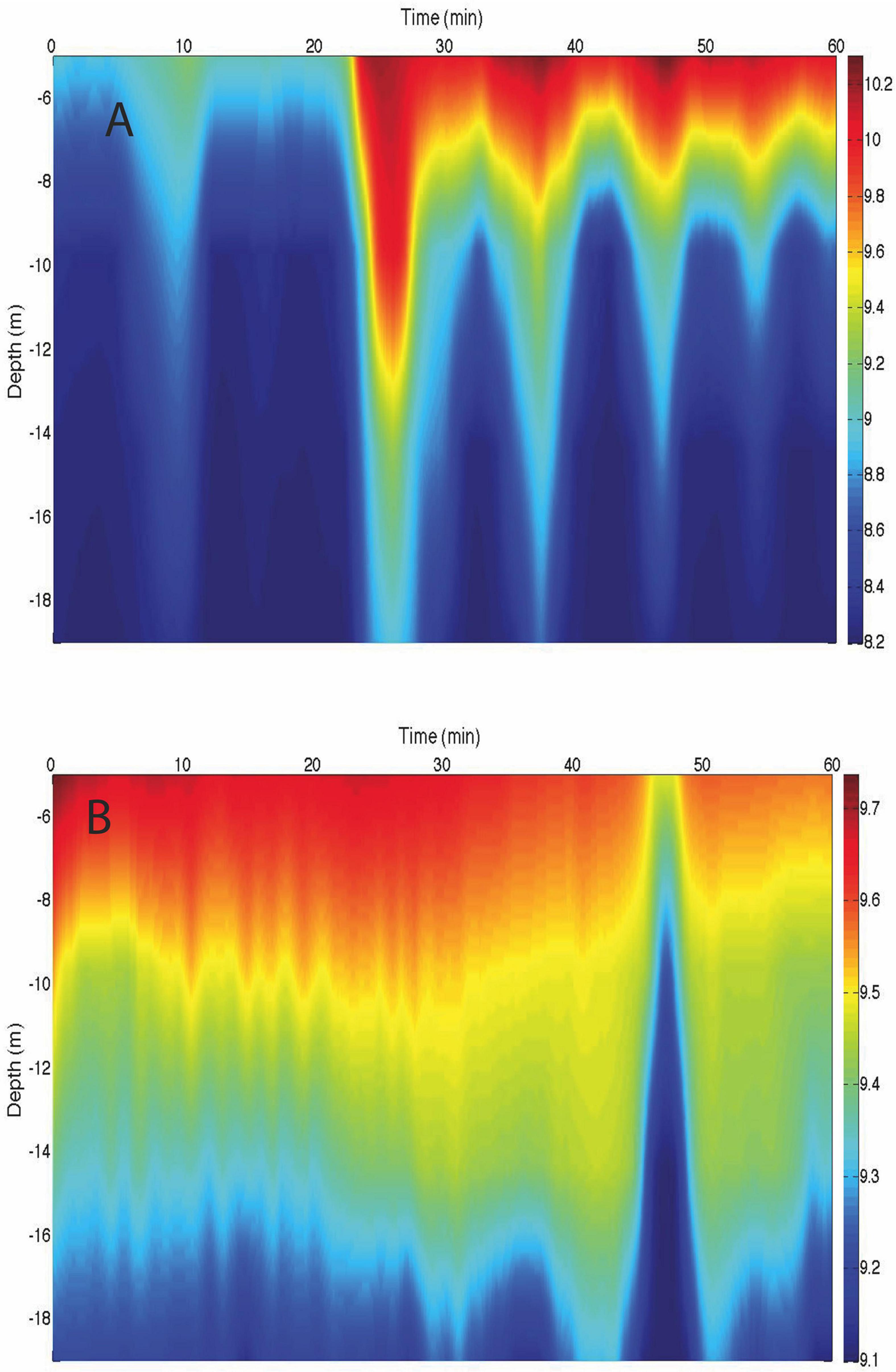
Figure 2. (A) Internal waves of depression. (B) An internal wave of elevation is present at about 48 min. To the right of each figure the scale bars are temperature in °C. Depth is in meters. The data were collected with a chain of thermistors on a mooring placed off Coos Bay, Oregon (43.36 N, 124.37 W). Images are from L. Rasmuson (personnel communication).
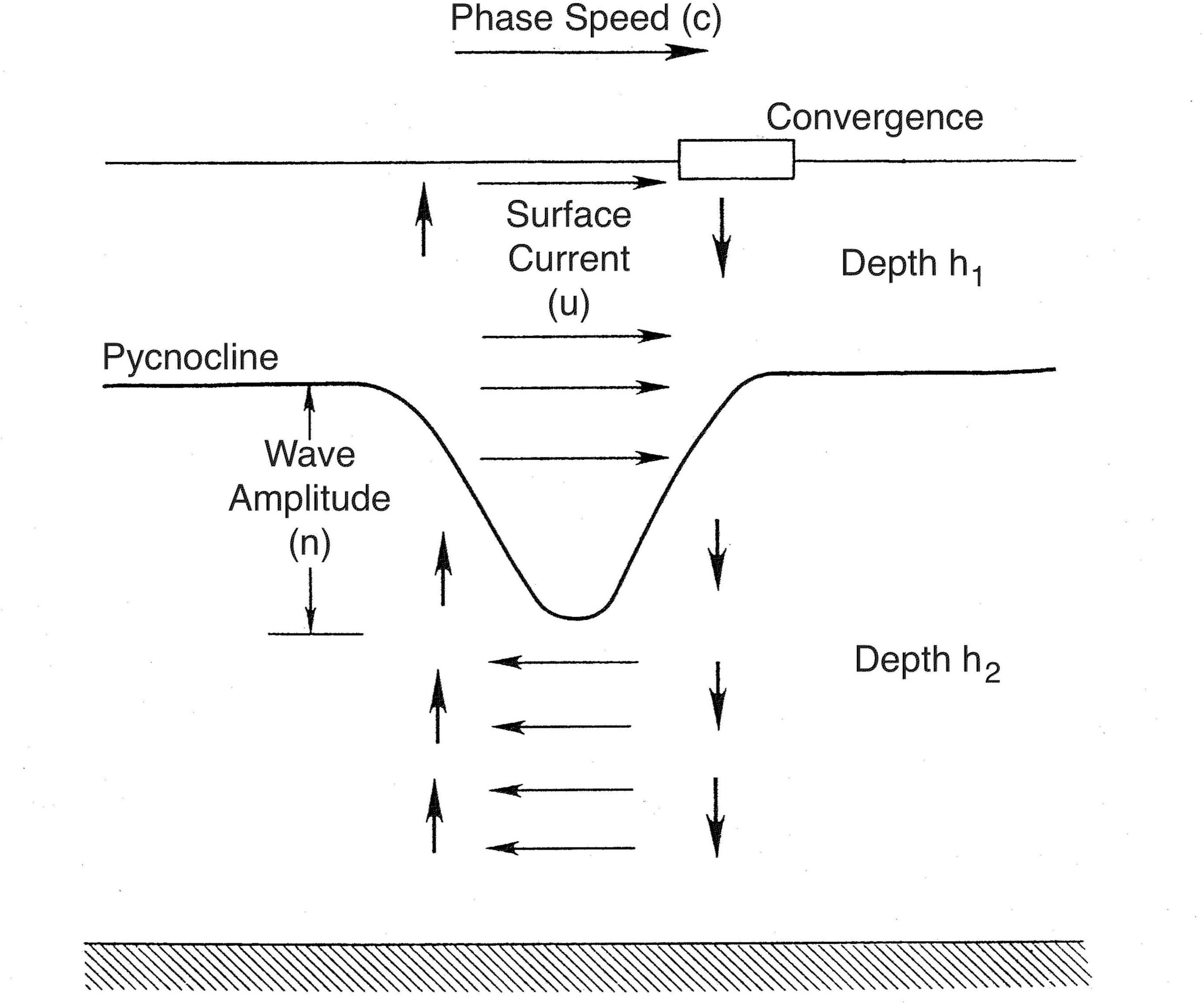
Figure 3. Non-linear internal wave or soliton as a wave of depression on a pycnocline. Arrows indicate the direction of currents associated with the internal wave. Depths h1 and h2 are the distance from the surface to the undisturbed pycnocline and from there to the bottom, respectively. (c) and (u) are the phase speed of the internal wave and surface current speed above the soliton, respectively. (n) is the amplitude of the internal wave. Theoretically, transport within the convergence zone of floating debris or larvae/zooplankton swimming at the surface should occur when u ≥ c. Figure modified from that in Shanks (Shanks, 1995).
My research has focused on the concentration and transport of surface associated zooplankton, particularly larval invertebrates and fish, by these moving convergences (Shanks, 1983, 1988). As far as I can tell, the concentration of floating plastic debris by tidally generated internal waves has been little investigated (Gove et al., 2019) and no one has investigated the transport of plastic trapped by an internal wave convergence. The research on larval transport, while not designed to investigate the transport of plastic debris, unintentionally tested this hypothesis; large tidally generated internal waves can concentrate and transport both surface-associated larvae and buoyant plastic debris.
Here I review and interpret the results from published biological studies of larval transport by internal waves in light of the problem of plastic debris in the ocean (Shanks, 1983, 1987, 1988, 1995). This paper does not present new unpublished research; in fact, the data are nearly 40 years old. Why present these old data now in this forum? The papers on larval transport by internal waves clearly demonstrated that concentration and transport of larvae can occur, in the process the research also tested the hypothesis that floating plastic could also be concentrated and transported. This work was done decades before plastic debris in the ocean became a research topic; hence, the importance of the work to the study of the fate of plastic in the ocean was not mentioned. I imagine very few if any of the people working on plastic pollution in the ocean are familiar with these papers, yet tidally generated internal waves are ubiquitous, present on all shore, are generated daily, and, as the research I will describe demonstrated, can efficiently concentrate and transport floating plastic debris. In the discussion, I present additional descriptions of the physical oceanography of the internal tides and associated internal waves, place this discussion within the context of plastic concentration and transport, and suggest a number of areas for future research on plastic debris and internal waves. As there are very few investigations of tidally generated internal waves and their effect on floating plastic debris this area of study is pretty much wide open. I conclude with a brief discussion of the possibility that pollutants (e.g., pesticides, oil, heavy metals etc.) might also be concentrated within internal wave convergences and, perhaps, transported.
Methods
Detailed descriptions of the methods used in studies of larval transport by internal waves were presented in the publications cited above and are briefly described here.
As large tidally generated internal waves propagate away from their point of origin, surface convergences are formed over large internal waves of depression (i.e., the wave is oriented downward) (Figure 3). The convergence zones over the internal waves are always present, but the visible surface expression of the convergence, slicks (i.e., bands of smooth glassy water), is disrupted at wind speeds high enough to generate white caps (moderate breeze, ∼6 m/s). At wind speeds between a light breeze and the formation of white caps, the convergence zones appear as wide bands (10 s of meters wide) of smooth water oriented parallel to the bottom topography (the waves are refracted by the bottom) with along shore lengths on the order of hundreds of meters to kilometers. The propagating internal waves evolve into a set of waves with each convergence zone delineated by a slick. The spacing between slicks is on the order of hundreds of meters over the continental shelf and kilometers in deep water. Over the continental shelf, the entire set of convergence zones and underlying waves propagate steadily at around 20–60 cm/s; waves propagate more slowly in shallower water, which causes the refraction of the waves. Often floating algal debris delineates the slicks. In the introduction I presented a fairly extensive list of hydrodynamics processes that will produce convergence zones. None of these different convergence zones have the characteristics of those generated by a set of tidally generated internal waves, slicks with the above described characteristics are uniquely produced by tidally generated internal waves and it is this set of characteristics that is used by physical oceanographers to identify the surface expression of internal waves in satellite images (see the Internal Wave Atlas see text footnote 1).
The above list of characteristics clearly differentiates internal wave convergences from all other types of convergences. For example, Langmuir circulation produces numerous convergence zones, convergence and divergences zones cover the ocean under the wind, discrete sets of convergences are not formed. The associated Langmuir slicks are generally much smaller and shorter in length on the order of a few meters or less in width and 10–100 s of meters in length. In other words, they are about 10 times smaller in both width and length. They are oriented roughly in the wind direction and their orientation is not affected by bottom topography. They do not propagate in any regular direction as do tidally generated internal waves (shoreward propagation) but are moved by tidal and ocean currents. Generally if the wind is blowing hard enough to create Langmuir circulation, the wind is likely to be blowing hard enough to obliterate the surface slicks associated with internal wave convergences.
The previously published observations on internal waves and larval transport were made on a number of days in the ocean off Southern California in the Southern California Bight, in enclosed waters around the San Juan Archipelago in Washington state (Salish Sea), and in the Atlantic Ocean off Beaufort, North Carolina (mid-Atlantic Bight) (Figure 4). These sampling locations were widely distributed geographically and represent a range of tidal regimes and coastal morphology. Sampling for larvae associated with internal wave convergences depended on visual identification of slicks and, hence, the work was limited to periods of light winds when slicks were clearly visible (see above). A small boat (<5 m) was driven seaward perpendicular to shore until a set of slicks with the above characteristics was observed. To test if the convergence zones were causing the concentration and transport of surface material, a line of small surface drifters was set in front of and perpendicular to the leading slick in the set (Figure 5). The line was several hundred meters to a kilometer long and consisted of 50 or so floats. A variety of float designs were used. In the initial study, the floats were Styrofoam coffee cups weighted with sand so that just the rim of the cup floated right at the surface of the water (i.e., little windage). The bulk of the coffee cup was underwater and acted as a sail pushed by the currents at the ocean surface. In later studies, the float consisted of small doughnut shaped foam net float with a wooden dowel forced through the center of the float and a small weight attached to the end of the dowel such that the dowel stood upright. The float was painted a highly visible color. In these floats, a portion of the dowel was exposed to the wind, but the remainder of the dowel, the net float, and the weight were all underwater and acted as a sail pushed by the surface currents. For the sake of this review, one can view the surface floats as model floating plastic debris and their use, unintentionally, tested the hypothesis that the convergences over tidally generated internal waves can concentrate and transport floating plastic. By transport I mean that the plastic floats traveled along with the convergence zone as the underlying internal wave propagated toward shore.
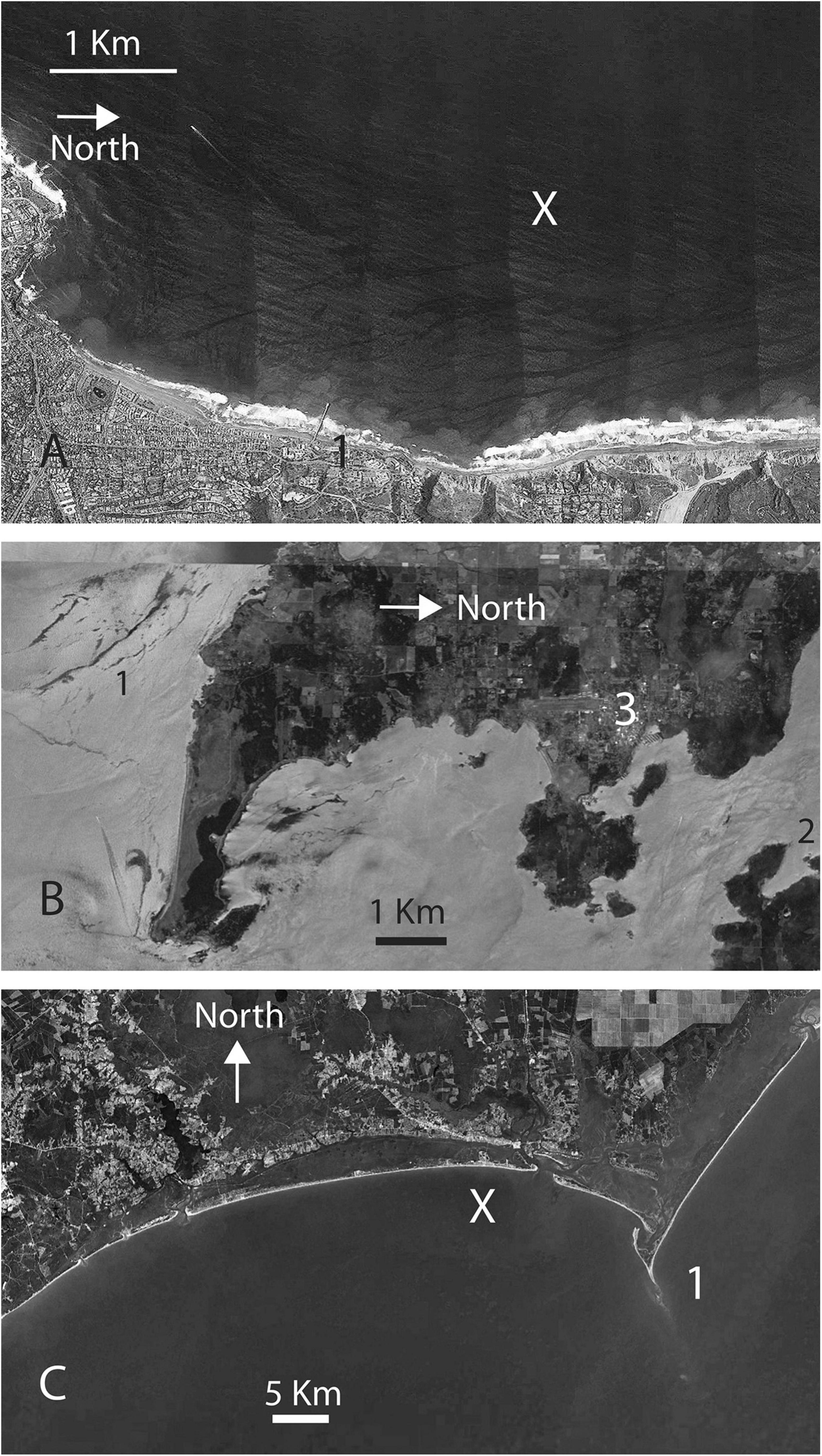
Figure 4. Areas where internal wave convergences were sampled. (A) La Jolla, California. The pier at “1” is located at the Scripps Institution of Oceanography, University of California, San Diego (32.87 N, 117.25 W). Internal wave convergences were sampled within about 3 km of X. The slightly darker streaks landward and to the right of X are internal wave convergences. (B) Sample sites within the San Juan Archipelago, Washington State. B1) This sample site was offshore Cattle Point. The linear dark smudges in the picture are internal wave convergences. B2) Indicates Park Bay where we sampled internal wave convergences and ran an initial experiment on the capacity of internal waves to differentially transport material to shore. The town of Friday Harbor is indicated by 3 (48.53 N, 123.02 W) (C) Cape Lookout Bight with Cape Lookout indicated by the “1” (34.59 N, 76.54 W). A sand bars extends seaward from the Cape most of the way across the shelf. To the south and outside the image area is Cape Fear, which also has an attached sand bar, which extends across the shelf. Sampling was carried out within about 3 km of X. Images are visible light aerial photographs from Google Earth.
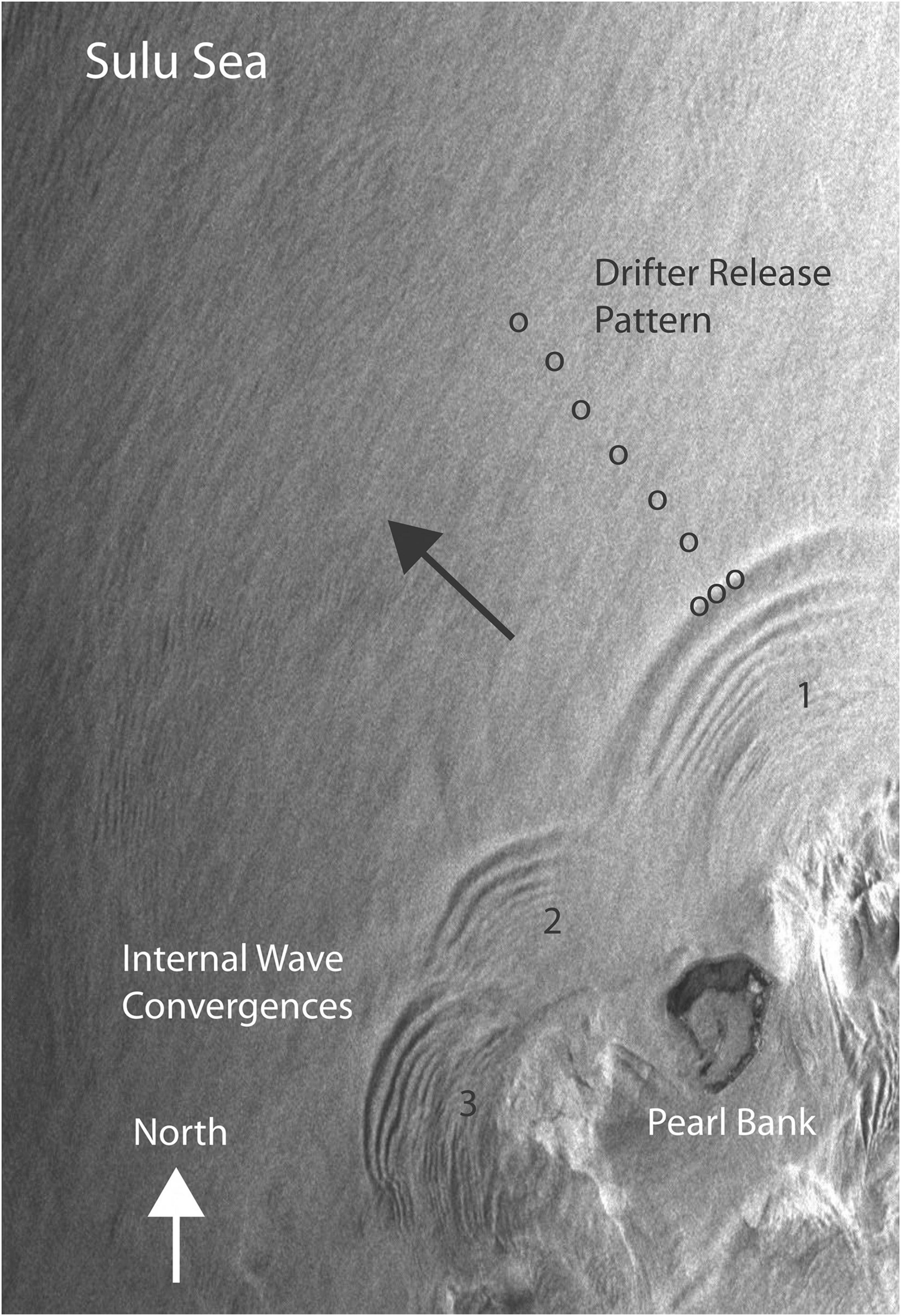
Figure 5. Synthetic aperture radar (SAR, C-band, VV) satellite image of three sets of internal wave convergences (labeled 1–3), which have been formed by the interaction of tidal currents with the Pearl Bank in the Sulu Sea (5.85 N, 119.85 E). The arrow indicates the direction of propagation of the internal waves. This image is used to describe the experimental design of the field sampling. A set of internal wave convergences was first located from a boat. In front of the set of convergences, a line of plastic surface floats was deployed (indicated by the black circles). A neuston net was then used to sample the zooplankton and floating debris within the convergences. After the plankton sampling, the surface drifters were located and note was taken if they were within a convergences or not and if transport by the convergences had occurred, an estimate of the distance to which they were carried was made. Image area is 100 × 100 km and is from https://earth.esa.int/web/guest/missions/esa-operational-eo-missions/ers/instruments/sar/applications/tropical/-/asset_publisher/tZ7pAG6SCnM8/content/oceanic-internal-waves-sulu-sea.
After the line of surface floats was set, zooplankton sampling commenced. Zooplankton was sampled with a neuston net, a floating zooplankton net, that fished the waters within about 30 cm of the surface. The collection of plastic debris from convergence zones described in Ruiz et al. (2020) used a nearly identical technique. Replicate neuston net tows were made in the slicks, between the slicks, and, if the wind remained light for a long enough period, in front of and behind the entire set of slicks. Following the zooplankton sampling, the surface drifters were recovered and we noted whether the drifters were present in slicks or not and estimated how far they had been transported shoreward from their release point. GPS was not available at the time these studies were done so distances were determined by triangulating compass sighting on landmarks on shore.
Samples were preserved with formalin and zooplankton was identified and enumerated using a dissecting microscope. When possible, identifiable buoyant debris (e.g., Sargassum floats, conifer needles, and tar balls) was also counted as an additional test of the hypothesis that floating material could be concentrated and transported by internal wave convergences. This can be viewed as an additional test of the hypothesis that the convergences over tidally generated internal waves can capture, concentrate and transport floating material similar to plastic debris.
Like surface waves, tidally generated internal waves are refracted by bottom topography. Wave refraction could focus waves toward some portions of a coastline and away from other sections. Material transported by the waves could be deposited more densely on sections of a coast where waves are focused. In addition, some areas, due to the morphology of the shelf break or the presence of reefs or banks, are better suited to the production of tidally generated internal waves (Sawyer, 1983; Figures 1, 6). As with wave refraction, sections of shore adjacent to areas that are favorable to the generation of internal waves may experience more deposition of material transported by internal waves. During the sampling in the San Juan Islands we carried out a small-scale test of this hypothesis. Internal waves were generated at the mouth of Park Bay, Orcas Island and propagated into the Bay (Figure 7). We sampled these internal wave slicks on several days and on one date we released surface drifters in front of a set of internal wave convergences and then mapped the path of transport of the drifters and their eventual deposition in the internal zone (Figure 7). We measured the settlement and recruitment of barnacle cyprids (settlement stage of barnacle larvae) by setting out settlement plates at a number of locations in the Park Bay intertidal zone. We then compared the barnacle recruitment at these sites to the density of surface drifters deposited by the internal waves to the sites.
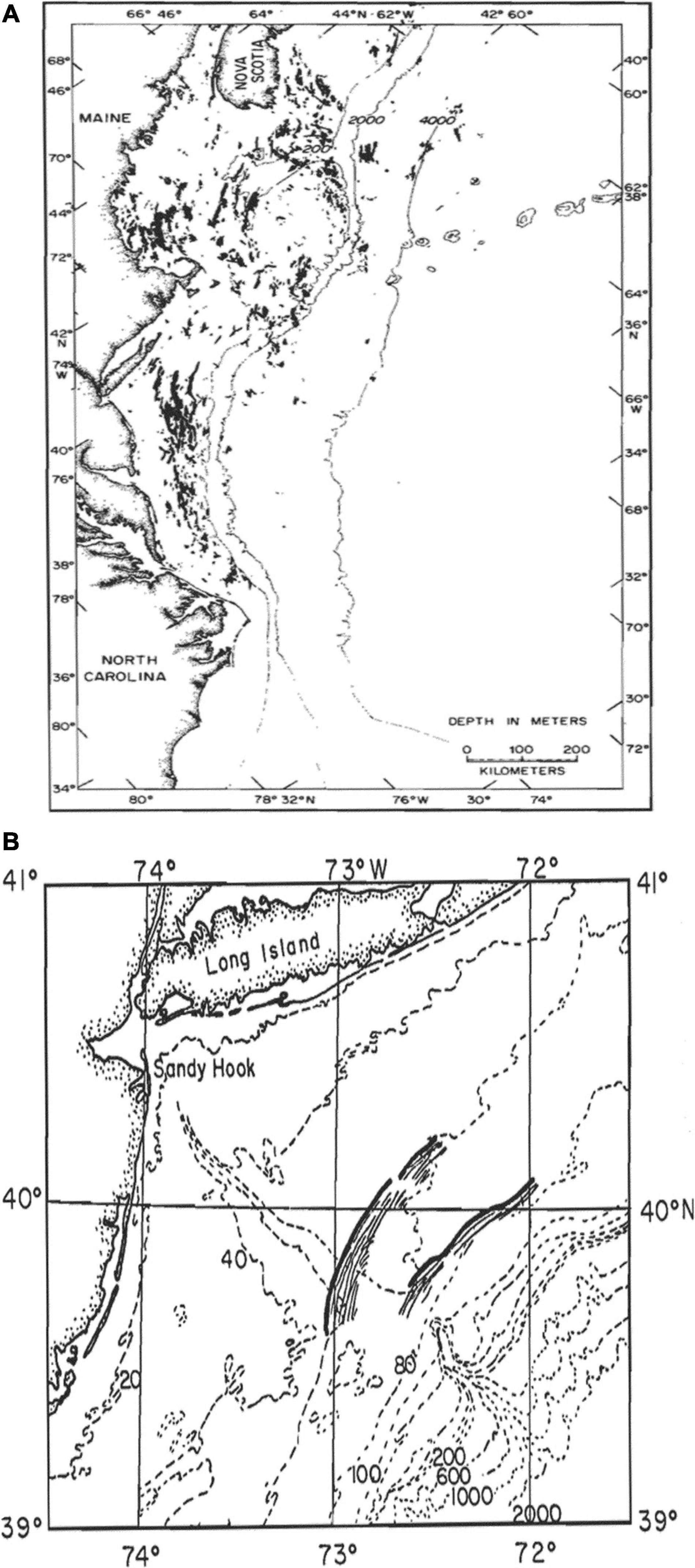
Figure 6. Large tidally generated internal waves are not produced equally along a continental shelf. Waves tend to be more regularly and strongly produced along sections of the shelf break that are steeper. Images are modified from Sawyer (1983) and are part of an analysis based on Landsat images of internal wave production along the Atlantic coast of North America. (A) This image is a composite, produced from multiple Landsat images. The black blotches indicate the location of sets of internal wave convergences in the images. Notice that visible internal wave convergences are not evenly distributed. For example, there are none off the coast of Maine. (B) A close up image offshore of New York City and Long Island. The parallel lines indicate internal wave convergences. Each set of waves was produced on a different tidal exchange. Notice there are no sets to the north or south, the production of the internal waves was not evenly distributed along the shelf.
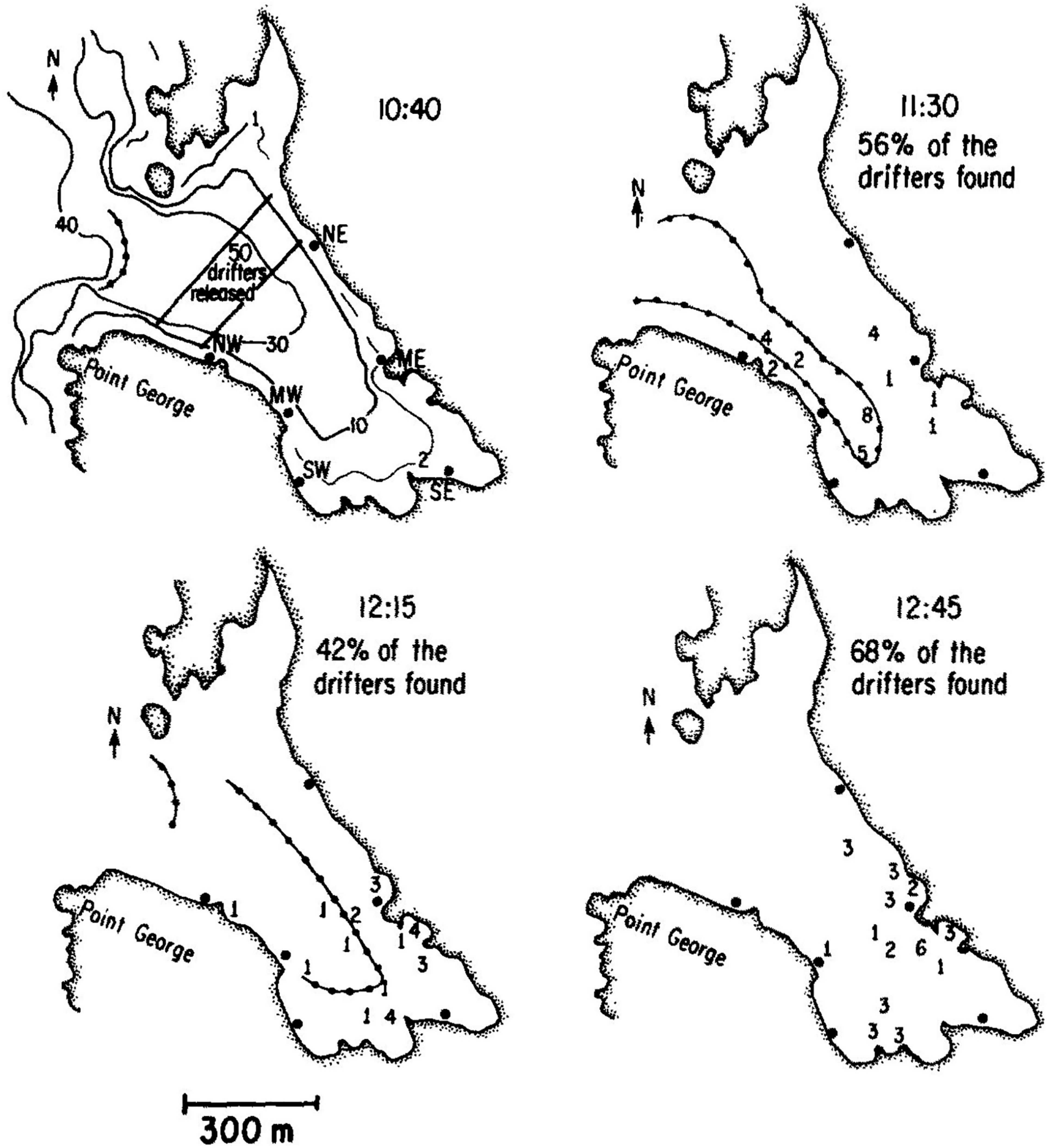
Figure 7. The initial experiment at Park Bay, San Juan Archipelago Washington state to test if transport by internal wave convergences would differentially transport and deposit surface drifters in the intertidal zone (Shanks and Wright, 1987). In the 10:40 image, depth contours are in meters. The letters around the shore indicate the locations where we sampled barnacle cyprids settlement in the intertidal zone. Zooplankton sampling indicated that barnacle cyprids were captured, concentrated and transported by internal wave convergences. At 10:40 an internal wave convergence had formed at the mouth of Park Bay (line with dots). 50 plastic surface drifters were set across the Bay in two lines. These were then followed for roughly 2 h as the internal wave convergence propagated into the Bay. The small numbers indicate the number drifters observed at each observation time. Drifters tended to be transported to the areas of the shore around intertidal sample site ME and between SW and SE. Figure modified from that in Shanks and Wright (Shanks and Wright, 1987).
Results
The first test of the hypothesis that convergence zones over tidally generated internal waves could cause the concentration and transport of objects swimming/floating at the surface took place in the Southern California Bight (Shanks, 1983; Figure 4). Sampling occurred on 5 days. On 3 days, the surface drifters were not caught, concentrated or transported by the internal waves and there were not significant differences in the concentrations of the enumerated zooplankton. As the convergence zone slicks moved shoreward, the drifters would enter the landward side of a slick and exit out the seaward side. On the other 2 days the results were dramatically different. As the slicks moved shoreward, each surface drifter (Styrofoam coffee cup) in the line was pulled into the convergence, trapped and transported shoreward. Like a broom sweeping a floor, the internal wave convergence swept up the surface drifters and carried them along in the convergence. After 2–3 h, 90% of the drifters were concentrated in the first two slicks of the sampled sets and the drifters had been transported shoreward 2–3 km from the most seaward end of the line of drifter release. Floating algal debris (primarily surf grass and Macrocystis) was abundant in the convergences and absent in the waters between the slicks. A variety of zooplankton, particularly late or settlement stage larvae invertebrates (e.g., cyprids, crab megalopae) and fish, were an order of magnitude or more concentrated in the slick samples than in the samples collected between the slicks. Tidally generated internal waves, by producing moving convergence zones, were capable of concentrating and transporting buoyant debris, including plastic surface drifters, and larval invertebrates and fish. Transport distances were on the order of kilometers in hours indicating shoreward transport at about 25 cm/s.
A second set of samples was collected at locations around the San Juan Islands, Washington state (Shanks and Wright, 1987; Figure 4). The observation methods were similar to those used in Southern California. Some sets of internal wave slicks did not catch and transport surface drifters while others did. When surface drifters were caught, concentrated, and transported by the slicks, we found significantly higher concentrations of a variety of mostly late stage larval invertebrates (e.g., cyprids and megalopae). Conifer needles were enumerated in these samples as an example of naturally occurring buoyant surface debris. Their concentration in the slicks relative to the water between the slicks were 50 to > 1,000 times higher. As in the Southern California Bight, convergences over tidally generated internal waves were capable of concentrating and transporting surface associated animals and buoyant debris and buoyant plastic.
The internal waves generated at the mouth of Park Bay deposited drifters at higher densities along some portions of the shore within the Bay than at other locations (Shanks and Wright, 1987; Figure 7). Barnacle cyprids were transported by the internal waves and their settlement and recruitment to juveniles on plates set in the Park Bay intertidal zone was significantly higher where the internal waves had deposited plastic surface drifters at higher densities. This limited and small scale experimental test supports the hypothesis that internal waves have the capacity to differentially transport floating plastic debris and surface associated animals to shore causing alongshore variation in the density of floating plastic debris at the shore and larval recruitment in the intertidal zone.
Sampling of internal wave convergences took place in the Atlantic Ocean off Beaufort, North Carolina (Figure 4). Cape Lookout and Cape Fear are located to the north and south of the study area (Shanks, 1987, 1988). Extending seaward from the Capes are narrow sand banks that cross much of the continental shelf. In this sample location, we observed internal wave convergences that were oriented perpendicular and parallel to shore. The slicks oriented perpendicular to shore were likely formed as tidal currents flowed over the sand banks associated with the Capes. These convergence zones did not capture the surface drifters and there were not significant differences in the concentrations of zooplankton or floating debris in the slicks vs. the water between the slicks. The slicks oriented parallel to shore were likely due to internal waves generated at the shelf break. These slicks did capture, concentrate and transport the plastic surface drifters and surface associated zooplankton and floating debris were found at significantly higher concentrations in the neuston samples from the slicks.
On one sample date the winds remained light for a long enough time that replicate samples were collected within slicks, between the slicks, in front of the entire set of internal wave slicks and behind the entire set of slicks. If the internal wave convergences were concentrating and transporting surface associated material one should see high concentrations within the convergence zone slicks and significantly lower concentrations of the enumerated material behind than in front of the set as material from in front of the set would have been caught up into the convergence zones as the waves progressed shoreward. In this set of observations, 96% of the released surface drifters were caught by the first two slicks in the set (5 slicks in the set) and transported several kilometers shoreward over the several hours of sampling; shoreward transport was at a speed of about 25 cm/s. We enumerated a variety of larval invertebrates and fish and many of these taxa were highly concentrated within the slicks and concentrations in the waters behind the set of slicks was significantly lower than in front of the set; all results consistent with the hypothesis that the internal wave convergences were capturing, concentrating and transporting the larvae shoreward.
A few days prior to these observations a spill of liquid asphalt occurred within the estuary at the shipping terminal about 5 km from the sample site (Figure 4). The asphalt spill was swept out to sea by tidal currents. Small tar balls were common in the neuston samples and we enumerated these (Shanks, 1987). We also enumerated the floats from Sargassum seaweed. Consistent with the hypothesis that internal wave convergences could cause concentration and transport of surface associated material, both tar balls and Sargassum floats were more abundant in front of the set of internal wave convergences than behind and were highly concentrated within the sampled slicks.
Knowing the decrease in concentration between the waters in front of the set of internal wave slicks relative to that behind the set coupled with the concentration in the slicks, we could estimate the amount of water the slicks had to have “fished” to accumulate their load of larvae, Sargassum floats and tar balls. The calculations suggest that the load of Sargassum floats may have been captured and transported as the slicks propagated shoreward across 20–30 km of water. The tar balls in the slicks may have been captured as the waves swept about 8 km of water. Similar calculations were made using the data from the enumerated larvae. Transport distances were similar, 10 to several 10 s of km. Some of the enumerated larvae, however, were abundant in the slicks, but absent from the waters in front and behind the set of internal waves; these larvae may have been transported into the sample area from some distant source.
Discussion
We sampled in and around the convergence zones (slicks) over tidally generated internal waves in three distinctly different geographic and hydrodynamic settings. In each location we found some slicks associated with internal tidal waves that did not capture, concentrate or transport surface drifters or surface associated debris or organisms. Other slicks associated with internal wave convergences at these same locations, however, did capture and transport surface drifters with >90% of the released plastic surface drifters caught and transported by the first couple of slicks within a set of slicks. These slicks also contained high concentrations of surface associated organisms, primarily late stage larvae of invertebrates and fish, and buoyant debris such as confer needles, tar balls, and Sargassum floats. On one date we were able to sample in front of a set of slicks, behind the set, the slicks in the set, and the water between slicks. From these data we were able to estimate the distance over which organisms and material had been captured and concentrated by the propagating internal waves; capture and transport distances ranged from at least 8 to > 30 km. While this research was designed to investigate the onshore transport of larvae, the techniques used, particularly the use of plastic surface drifters, conclusively tested whether the convergences over tidally generated internal waves could transport floating plastic; the research clearly demonstrated that they could capture concentration and transport, however, the role these convergences play in the fate of floating plastic debris has been little studied, see, however, Gove et al. (2019) and Miyao and Isobe (2016). In the following I present a series of potential research questions to address gapes in our knowledge.
(Q1) I have been able to find only one paper, which sampled plastic in internal wave convergences (Gove et al., 2019), and, consistent with the observations reported here, they found microplastic particles to be 126 times more concentrated within the sampled internal wave slicks. A second paper (Miyao and Isobe, 2016) used a camera mounted on a balloon to observe floating surface drifters around convergence zones. One convergence was at the boundary between two water masses and the other appeared to be associated with tidally generated internal waves. In both cases the floating surface drifters, what might be considered models of floating plastic debris, were pulled into the surface convergences. A companion paper in this special issue (Ruiz et al., 2020) tested if “naturally” occurring floating plastic debris could be collected and removed from the ocean by “fishing” linear accumulations of plastic. The linear accumulations of plastic debris depicted in figures in this paper look strikingly like internal wave convergences and in the Internal Wave Atlas (see text footnote 1, Bay of Biscay, Figure 7) there is a satellite image of numerous internal wave in the area in which this study took place. This limited set of observations begs the question: we have demonstrated that the convergence over tidally generated internal waves can concentrate and transport plastic surface drifters, but how much floating plastic does one find in internal wave convergences and is this a regular occurrence? If internal waves regularly concentrate plastic debris, could this be exploited; could one “fish” internal wave slicks to remove plastic debris (Ruiz et al., 2020; Cozar et al., 2021)?
(Q2) How buoyant must plastic be to be captured by internal wave convergences? The surface drifters used in the above described studies were quite buoyant, all remained at the surface even in the convergence zone where vertical currents would have been present. Most of the larval invertebrates and fishes transported by internal waves were late stages that swim well, in other words, if they attempted to remain at the surface, their upward swimming would make them behave as if they were very buoyant. One set of samples collected in slicks contained very high concentrations of a small flatworm (2 mm) (Shanks, 1983). These organisms are undoubtedly slow swimmers yet they remained at the surface despite the vertical currents typical of a convergence zone. As with wind driven Langmuir circulation, vertical currents just below the surface are likely very small (Woodcock., 1950), however, near surface vertical currents over large tidally generated internal waves have not been measured. The flatworm example suggests that even weakly buoyant plastic debris may be captured by internal wave convergences, however, wave action may push floating material deep enough to be affected by the faster vertical currents away from the surface. In this way less buoyant plastic debris, e.g., plastic bags, could be carried out of the surface convergence zone and into the water column. None of these ideas have been investigated.
(Q3) Why did only some sets of internal waves cause concentration and transport of surface associated material? This has not been investigated, but there is a theoretical explanation. Surface flow over an internal wave of depression (Figures 2, 3) is toward the leading edge of the wave and in the direction of wave propagation. At the front of the wave, the surface current turns downward creating a convergence zone. Material at the surface is carried in the direction of wave propagation by this surface current. If material is going to be trapped and transported in the slick it must enter the convergence zone, however, if the speed of the surface current is less than the propagation speed of the wave, then material transported in the surface current will never reach the convergence zone (Shanks, 1995). Theory suggests that surface transport should occur when the speed of the converging surface current is equal to or greater than the propagation speed of the wave (Shanks et al., 2000). For this to occur, wave amplitude must be large and the pycnocline upon which the wave is traveling must be relatively shallow. This is a vague description and clearly needs further investigation both theoretical, but, particularly, in the field.
The hydrodynamics of tidally generated internal waves are complex. The waves are formed by tidal currents flowing across submarine topography such as the continental shelf break, a reef or bank. The strength of tidal currents vary with the spring to neap tidal cycle, hence, the forcing factor, tidal currents, varies on a roughly 2-week cycle (Woodson, 2018). Larger waves tend to be generated at the higher current speeds during spring than neap tides. The waves travel on the density structure of the water column and this varies tremendously both seasonally but also over shorter intervals. There is the potential for feedback between larger internal waves and water column density structure. As larger internal waves propagate toward shore and into shoaling water they can become unstable and break forming turbulent internal bores. The turbulence can disrupt the pycnocline and this weakening of the pycnocline can happen over a fortnightly cycle as tidal currents vary in strength (Cairns, 1968).
If the pycnocline is deeper, waves tend to be oriented upward, waves of elevation (Figure 2). These types of waves do not appear to make moving convergences capable of transporting surface material. On a shallow pycnocline the internal waves tend to be oriented downward, waves of depression (Figures 2, 3), and these are capable of generating convergences that capture and transport floating material. Through the fortnightly spring to neap tidal cycle the steady day to day change in the strength of the tidal currents, leads to the generation of internal waves of varying amplitudes and the effect waves of different amplitude have on the density structure of the surface waters means that the transport of material at the surface of the ocean, zooplankton or floating debris, may vary on a fortnightly cycle in a complex way (Woodson, 2018).
(Q4) Time series of the daily settlement/recruitment of larvae of taxa that have been demonstrated to be transported toward shore in convergences over internal waves (e.g., Pachygrapsus crassipes megalopae, Callinectes sapidus megalopae, and barnacle cyprids) are often cross correlated with the maximum daily tidal range, i.e., the spring/neap tidal cycle (Shanks, 1983, 1986, 1998, 2009b; Pineda, 1991). Such data have been collected in the Southern California Bight, southern Oregon, and in the Atlantic off North Carolina. Despite the broad geographic distribution of the sample sites, the daily patterns of settler abundance were quite similar. Settler abundance was highly pulsed, with periods of low abundance separated by brief pulses with orders of magnitude higher counts. Peaks in abundance are significantly cross correlated with the spring to neap tidal cycle, but peaks are not located at either the maximum spring tides or minimal tides at the neap nor is the settlement proportional to the daily tidal range. Instead, the pulses tend to occur either several days prior to the spring tide or shortly after the spring tide. The pulsed arrival of larvae at the shore has been interpreted as due to variation in their shoreward transport by internal tidal waves (Shanks, 1983, 1986, 2009a; Pineda, 1991); during brief periods of the month, several days, the internal tides are such that they actively transport larvae to shore. The necessary biological and physical oceanographic sampling needed to test this hypothesis has yet to be made. This pattern of delivery at the shore, however, has only been seen in species that are transported shoreward by internal waves. Given that both surface active larvae and buoyant debris at the surface are transported shoreward in convergence zones over tidally generated internal waves, it seems reasonable to hypothesize that the delivery of floating plastic debris to the shore will have a similar pattern, days of low abundance punctuated by brief pulses, several days, with high abundance. This hypothesis has not been tested.
(Q5) The depth of the pycnocline tends to vary seasonally, deeper during the winter, shallower during the spring, and summer. Internal waves on deeper pycnocline tend to be waves of elevation and these do not appear to cause transport of material at the surface. On a shallower pycnocline waves of depression tend to be formed and these can cause transport. Hence, there may be seasonal variation in the concentration and transport by internal waves, less or no concentration and transport in winter, and concentration transport in spring and summer. From the perspective of transport of floating plastic debris, ignoring wind driven transport of this material, which is clearly important, more onshore transport by internal waves may occur in the spring/summer when pycnoclines are shallower than during the winter when they are deeper. This hypothesis has not been tested, however, Ruiz et al. (2020) observed linear plastic debris lines primarily in the spring consistent with these linear features being caused by internal wave convergences.
(Q6) Satellite images of the US Atlantic continental shelf indicate that internal waves are not evenly produced along the shelf break, but tend to be more readily produced at locations where the shelf break is particularly steep (Sawyer, 1983; Figure 6). This suggests the hypothesis that plastic debris deposited on the shore may not be evenly distributed along that coast, but may be more abundant landward of sites on the shelf break that more readily generate large internal waves. On a very small scale, the sampling in Park Bay tested this hypothesis and the results were consistent with the hypothesis. The hypothesis has not been tested at a larger scale nor have the effects on the distribution of plastic debris been investigated.
(Q7) There are a number of sites in the world where tidal currents flowing over banks or reefs generate large internal waves. For example, at the southern edge of the Sulu Sea, sets of very large internal waves are generated at the Pearl Banks (Apel et al., 1985; Figures 1, 5). These propagate northward across the Sulu Sea and in satellite images multiple sets of internal waves are present each having been generated by a shift in the tidal flow at the Pearl Banks (Figure 1). These sets propagate across the Sulu Sea and ultimately wash ashore on Palawan Island, the waves travel about 450 km. If during this transit, these waves capture and transport floating plastic debris then they could collect floating plastic from hundreds of kilometers of ocean and, when the waves wash ashore on Palawan, the plastic could be deposited on the beach. Potentially large amounts of plastic debris could be transported to Palawan by this mechanism. As the tides flow through the Straits of Gibraltar, internal waves are generated at the sill in the Straits (Brandt et al., 1996). These waves propagate into the Mediterranean Sea. In satellite images, the waves appear to be refracted toward North Africa. If these waves are capturing and transport floating debris, it could be deposited on the shore of North Africa. Tidal currents flowing over banks in the southern Caribbean around the islands of Trinidad and Tobago generate large internal waves that propagate across the entire Caribbean ultimately washing ashore at least as far north as Puerto Rico (≈1,000 km) (Giese et al., 1990). These waves travel over hundreds of kilometers of ocean and could conceivably accumulate a tremendous amount of plastic. Videos posted on YouTube show dense patches of plastic debris in the coastal waters off Honduras2 ,3 (see also Figure 1A in Cozar et al., 2021). The plastic patches were in the shape of long bands. The bands were several 10 s of meters wide and appeared to stretch for kilometers along the coast. The seaward and landward edges of the bands were sharply delineated. In some of the images there appears to be additional bands further offshore. In my work in the Southern California Bight described above, slicks were often delineated by dense accumulations of floating algae (Macrocystis). Obviously, it is not possible to definitively state that the plastic patches in the YouTube videos were caused by internal wave convergences, however, they appear remarkably similar to the internal wave convergences off Southern California with accumulated floating plastic in place of floating seaweed. Internal waves that travel over large distances have the potential to accumulate large amounts of plastic debris and transport it over tens to hundreds of kilometers. This hypothesis has not been tested.
(Q8) Similarly, internal waves formed at the continental shelf break may transport material across the shelf. Woodson (2018) in his review suggests that transport in internal wave convergences may only occur close to shore. However, the sampling off Beaufort, North Carolina (Shanks, 1987, 1988) described above suggested that material (floating debris and larvae) contained in the internal wave slicks could have accumulated during passage of the waves over tens of kilometers of ocean. Sampling of internal wave convergences has only been done within several kilometers of shore, hence, over a continental shelf we do not know at what distance offshore waves might cause shoreward transport of larvae or floating plastic.
Internal wave convergences may also be capable of capturing, concentrating, and, perhaps, transporting other marine pollutants. At low wind speeds convergences over large internal waves are delineated by surface slicks of smooth water. This is caused by two mechanisms. (1) The surface currents over these internal waves, by interacting with the surface wave field, cause variations in sea surface roughness; surface currents in the direction of surface wave propagation cause surface waves to decrease in amplitude, flatten out, and when the internal wave surface currents flow into a wave field the wave amplitude is increased (Apel et al., 1985). This variation in sea surface roughness is apparent in satellite images produced by synthetic aperture radar (see the Internal Wave Atlas see text footnote 1). (2) The other cause for the smooth water in the slick is the dampening of surface capillary waves by the surface film in the slick (Barger et al., 1974).
The ocean’s very surface, the surface microlayer (SML), is composed of a thin (<100 μm) layer composed of lipids primarily derived from phytoplankton and transparent exopolymers (TEP) (Wurl et al., 2016) formed by the abiotic coagulation of polymeric substances released primarily by phytoplankton. Converging currents can sweep up the surface microlayer compressing it (Barger et al., 1974). Within internal wave slicks, the density of the compressed surface film can be five times higher than in the rippled water away from the slick and this compressed surface film dampens the surface capillary waves (Barger et al., 1974). Note that the work by Barger et al. (1974) was done in exactly the same geographic location as the work by Shanks (1983) described above. The alternation in sea surface roughness caused by the compression of the surface film and slick formation is apparent in both visible and synthetic aperture radar images from satellites.
A variety of pollutants are often found concentrated in the SML including oils, pesticides, PCBs, and heavy metals (Wurl and Obbard, 2004). These can be “free” in the water at the surface (oils) or associated or adsorbed onto the TEP. The concentration of these pollutants in slicks (origin or formation mechanism unknown) is significantly higher than in the surface waters without slicks (Wurl and Obbard, 2004). Except for the observations by Shanks (1987) of an oil spill concentrated in internal wave convergences, sampling of internal wave slicks for pollutants in SML does not appear to have been done. Given that the SML is strongly compressed in the slick (Barger et al., 1974), it is likely that associated pollutants will also be compressed and at higher concentrations. Many of the contaminants that have been observed in SML are toxic to the eggs and larvae of fish and invertebrates (Wurl and Obbard, 2004). Larval invertebrates and fish have been observed at high concentrations in internal wave slicks (Shanks, 1983, 1988; Kingsford and Choat, 1986; Shanks and Wright, 1987; Kingsford, 1990; Gove et al., 2019) where they may be exposed to high concentrations of toxic pollutants. Within internal wave slicks, the compression of the SML can cause the surface film to collapse and form into a thick layer of scum (Barger et al., 1974), which, if the film collapses enough, can sink. This is a potential mechanism for the export of surface associated pollutants into the water column. I have described the capture, concentration and shoreward transport of floating plastic (plastic surface drifters) by internal wave convergences. Could something similar be happening with pollutants in the SML, could they be concentrated and transported shoreward?
Author Contributions
The author confirms being the sole contributor of this work and has approved it for publication.
Conflict of Interest
The author declares that the research was conducted in the absence of any commercial or financial relationships that could be construed as a potential conflict of interest.
Footnotes
- ^ www.internalwaveatlas.com
- ^ https://www.youtube.com/watch?v=P752pV41m9g
- ^ https://www.youtube.com/watch?v=dYBZK7sz25s
References
Apel, J. R., Byrne, H. M., Proni, J. R., and Charnell, R. L. (1975). Observations of oceanic internal and surface waves from the earth resources technology satellite. J. Geophys. Res. 80, 865–881. doi: 10.1029/jc080i006p00865
Apel, J. R., Holbrook, J. R., Liu, A. K., and Tsai, J. J. (1985). The Sulu sea internal soliton experiment. J. Phys. Oceanogr. 15, 1625–1651. doi: 10.1175/1520-0485(1985)015<1625:tssise>2.0.co;2
Barger, W. R., Daniel, W. H., and Garrett, W. D. (1974). Surface chemical properties of banded sea slicks. Deep Sea Res. 21, 83–89. doi: 10.1016/0011-7471(74)90022-9
Brandt, P., Alpers, W., and Backhaus, J. O. (1996). Study of the generation and propagation of internal waves in the Strait of Gibraltar using a numberical model and synthetic aperture radar images of the European ERS 1 satellite. J. Geophys. Res. Oceans 101, 14237–14253. doi: 10.1029/96jc00540
Cairns, J. L. (1968). Thermocline strength fluctuations in coastal waters. J. Geophys. Res. 73, 2591–2595. doi: 10.1029/jb073i008p02591
Cozar, A., Aliani, S., Basurko, O. C., Arias, M., Isobe, A., Topouzelis, K., et al. (2021). Marine litter windrows: a strategic target to understand and manage the ocean plastic pollution. Front. Mar. Sci. 8:571796.
da Silve, J. C. B., Ermakov, S. A., Robinson, I. S., Jeans, D. R. G., and Kijashko, S. V. (1998). Role of surface films in ERS SAR signatures of internal waves on the shelf. J. Geophys. Res. 103, 8009–8031. doi: 10.1029/97jc02725
Giese, G. S., Chapman, D. C., Black, P. G., and Fornshell, J. A. (1990). Causation of large-amplitude coastal seiches on the caribbean coast of puerto rico. Am. Meterol. Soc. 20, 1449–1458. doi: 10.1175/1520-0485(1990)020<1449:colacs>2.0.co;2
Gove, J. M., Whitney, J. L., McManus, M. A., Lecky, J., Carvalho, F. C., and Lynch, J. M. (2019). Prey-size plastics are invading larval fish nurseries. Proc. Natl. Acad. Sci. USA 116, 24143–24149. doi: 10.1073/pnas.1907496116
Hamner, W. M., and Schneider, D. (1986). Regularly spaced rows of medusae in the bering sea: role of langmuir circulation. Limnol. Oceanogr. 31, 171–177. doi: 10.4319/lo.1986.31.1.0171
Helfrich, K. R., and Melville, W. K. (2006). Long nonlinear internal waves. Annu. Rev. Fluid Mech. 38, 395–425.
Hibiya, T. (1990). Study of internal wave generation by tide-topography interaction. J. Oceanogr. Soc. Japan 46, 21–32. doi: 10.1007/bf02334221
Howell, T. L., and Brown, W. S. (1985). Nonlinear internal waves on the california continental shelf. J. Geophys. Res. 90, 7256–7264. doi: 10.1029/jc090ic04p07256
Kao, T. W., Pan, F.-S., and Renouard, D. (1985). Internal solitons on the pycnocline: generation, propagation, and shoaling and breaking over a slope. J. Fluid Mech. 159, 19–53. doi: 10.1017/s0022112085003081
Kingsford, M. J. (1990). Linear oceanographic features: a focus for research on recruitment processes. Aust. J. Ecol. 15, 391–401. doi: 10.1111/j.1442-9993.1990.tb01465.x
Kingsford, M. J., and Choat, J. H. (1986). The influence of surface slicks on the distribution and onshore movement of small fish. Mar. Biol. 91, 161–171. doi: 10.1007/bf00569432
Lamb, G. (1997). Particle Transport by nonbreaking, solitary internal waves. J. Geophys. Res. 102, 641–18660.
Leibovich, S. (1983). The form and dynamics of Langmuir circulations. Annu. Rev. Fluid Mech. 15, 391–427. doi: 10.1146/annurev.fl.15.010183.002135
Leichter, J. J., Shellenbarger, G., Genovese, S. J., and Wing, S. R. (1998). Breaking internal waves on a Florida (USA) coral reef: a plankton pump at work? Mar. Ecol. Progr. Ser. 166, 83–97. doi: 10.3354/meps166083
Miyao, Y., and Isobe, A. (2016). A combined balloon photography and buoy-tracking experiment for mapping surface current in coastal waters. J. Atmos. Oceanic Technol. 33, 1237–1250. doi: 10.1175/jtech-d-15-0113.1
Osborne, A. R., and Burch, T. L. (1980). Internal solitons in the Andaman sea. Science 208, 451–460. doi: 10.1126/science.208.4443.451
Pineda, J. (1991). Predictable upwelling and the shoreward transport of planktonic larvae by internal tidal bores. Science 253, 548–551. doi: 10.1126/science.253.5019.548
Pineda, J. (1995). An internal tidal bore regime at nearshore stations along western U.S.A.: predictable upwelling within the lunar cycle. Cont. Shelf Res. 15, 1023–1041. doi: 10.1016/0278-4343(95)80007-z
Pingree, R. D., Forster, G. R., and Morrison, G. K. (1974). Turbulent convergent tidal fronts. J. Mar. Biol. Assoc. U. K. 54, 469–479. doi: 10.1017/s0025315400058653
Reniers, A. J. H. M., MacMahan, J. H., Beron-Vera, F. J., and Olascoaga, M. J. (2010). Rip-current pulses tied to Lagrangian coherent structures. Geophys. Res. Lett. 37. doi: 10.1029/2009GL041443
Ruiz, I., Basurko, O. C., Rubio, A., Delpey, M., Granado, I., Declerck, A., et al. (2020). Litter windrows in the Sout-East coast of the Bay of Biscay: an ocean process enabling effective active fishin for litter. Front. Mar. Sci. 7:308.
Shanks, A. L. (1983). Surface slicks associated with tidally forced internal waves may transport pelagic larvae of benthic invertebrates and fishes shoreward. Mar. Ecol. Progr. Ser. 13, 311–315. doi: 10.3354/meps013311
Shanks, A. L. (1986). Tidal periodicity in the daily settlement of intertidal barnacle larvae and an hypothesized mechanism for the cross-shelf transport of cyprids. Biol. Bull. 170, 429–440. doi: 10.2307/1541852
Shanks, A. L. (1987). The onshore transport of an oil spill by internal waves. Science 235, 1198–1200. doi: 10.1126/science.235.4793.1198
Shanks, A. L. (1988). Further support fo the hypothesis that internal waves can transport larvae of invertebrates and fish onshore. Fish. Bull. 86, 703–714.
Shanks, A. L. (1995). Oriented swimming by megalopae of several eastern North Pacific crab species and its potential role in their onshore migration. J. Exp. Mar. Biol. Ecol. 186, 1–16. doi: 10.1016/0022-0981(94)00144-3
Shanks, A. L. (1998). Abundance of post-larval Callinectes sapidus, Penaeus spp., Uca spp., and Labinia spp. collected at an outer coastal site and their cross-shelf transport. Mar. Ecol. Prog. Ser. 168, 57–69. doi: 10.3354/meps168057
Shanks, A. L. (2009a). Barnacle settlement vs. recruitment as indicators of larval delivery: effects of post-settlement mortality and recruit density. Mar. Ecol. Prog. Ser. 385, 205–216. doi: 10.3354/meps08105
Shanks, A. L. (2009b). Barnacle settlement vs. recruitment as indicators of larval delivery: time series analysis and hypothesized delivery mechanisms. Mar. Ecol. Prog. Ser. 385, 217–226. doi: 10.3354/meps08002
Shanks, A. L., and Wright, W. G. (1987). Internal-wave-mediated shoreward transport of cyprids, megalopae, and gammarids and correlated longhsore differences in the settling rate of intertidal barnacles. J. Exp. Mar. Biol. Ecol. 114, 1–13. doi: 10.1016/0022-0981(87)90135-3
Shanks, A. L., Largier, J., Brink, L., Brubaker, J., and Hooff, R. (2000). Demonstration of the onshore transport of larval invertebrates by the shoreward movement of an upwelling front. Limnol. Oceanogr. 45, 230–236. doi: 10.4319/lo.2000.45.1.0230
Sverdrup, H. U., Johnson, M. W., and Fleming, R. H. (1942). The Oceans Their Physics, Chemistry, and General Biology. Englewood Cliffs: Prentice-Hall, Inc.
van Sebille, E., Aliani, S., Law, K. L., Maximenko, N., Alsina, J. M., and Bagaev, A. (2020). The physical oceanography of the transport of floating marine debris. Environ. Res. Lett. 15:023003.
Woodson, C. B. (2018). The fate and impact of internal waves in nearshore ecosystems. Annu. Rev. Mar. Sci. 10, 421–441. doi: 10.1146/annurev-marine-121916-063619
Wurl, O., and Obbard, J. P. (2004). A review of pollutants in the sea-surface microlayer (SML): a unique habitat for marine organisms. Mar. Pollut. Bull. 48, 1016–1030. doi: 10.1016/j.marpolbul.2004.03.016
Keywords: internal tides, internal waves, windrows, convergence zone, slick, plastic, flotsam
Citation: Shanks AL (2021) Observational Evidence and Open Questions on the Role of Internal Tidal Waves on the Concentration and Transport of Floating Plastic Debris. Front. Mar. Sci. 8:621062. doi: 10.3389/fmars.2021.621062
Received: 24 October 2020; Accepted: 05 May 2021;
Published: 01 June 2021.
Edited by:
Andrés Cózar, University of Cadiz, SpainReviewed by:
Stefano Aliani, National Research Council (CNR), ItalyAnna Rubio, Technology Center Expert in Marine and Food Innovation (AZTI), Spain
Luisa Galgani, University of Siena, Italy
Copyright © 2021 Shanks. This is an open-access article distributed under the terms of the Creative Commons Attribution License (CC BY). The use, distribution or reproduction in other forums is permitted, provided the original author(s) and the copyright owner(s) are credited and that the original publication in this journal is cited, in accordance with accepted academic practice. No use, distribution or reproduction is permitted which does not comply with these terms.
*Correspondence: Alan L. Shanks, YXNoYW5rc0B1b3JlZ29uLmVkdQ==