- 1Laboratory of Environmental Pollution and Integrative Omics, Guanxi Key Laboratory of Tumor Immunology and Microenvironmental Regulation, Guilin Medical University, Guilin, China
- 2Department of Chemistry, City University of Hong Kong, Kowloon, Hong Kong
- 3State Key Laboratory of Marine Pollution, City University of Hong Kong, Kowloon, Hong Kong
- 4Division of Newborn Medicine, Children’s Hospital Boston, Boston, MA, United States
- 5Department of Pediatrics, Harvard Medical School, Boston, MA, United States
- 6Faculty of Agriculture, Kyushu University, Fukuoka, Japan
- 7School of Life Sciences, Hong Kong Bioinformatics Centre, The Chinese University of Hong Kong, Hong Kong, China
- 8School of Biological Sciences, The University of Hong Kong, Hong Kong, Hong Kong
- 9Department of Science and Environmental Studies, The Education University of Hong Kong, Tai Po, Hong Kong
Hypoxia is a serious issue that affects the marine environment, with a growing number of hypoxic “dead zones” occurring each year. Reports have indicated that hypoxia is detrimental to the reproductive function and sexual development of fish via the disruption of endocrine signaling in organs involved in the hypothalamus–pituitary–gonad axis, including the brain. While we previously reported that hypoxia induces transcriptome-wide alterations in the brain of marine medaka (Oryzias melastigma), whether these effects were reflected at the protein level remains unclear. Therefore, the present study used high-throughput proteomic sequencing along with bioinformatics analysis to assess the short-term and multi-generational effects of hypoxia on the brain proteome of O. melastigma. We identified 36,567 peptides and 7,599 proteins (1% false discovery rate in brain samples), with functions involved in cellular and metabolic processes such as signaling and reproductive processes as well as energy production and conversion. Furthermore, we determined that hypoxia resulted in the significant differential expressions of 33 upregulated and 69 downregulated proteins in the short-term exposure group and 24 upregulated and 52 downregulated proteins in the multi-generational exposure group. Pathway enrichment analysis of the deregulated proteins indicated that hypoxia could impair brain function by altering arachidonic acid metabolism, tight junctions, and adrenergic signaling under short-term hypoxic exposure and by altering p53 and PI3K–Akt signaling under multi-generational hypoxic exposure, which may lead to the onset of neurodegenerative disorders including Alzheimer’s disease and amyotrophic lateral sclerosis. Ingenuity pathway analysis of the deregulated proteins showed that hypoxia affected common signaling pathways in the brain (e.g., integrin, paxillin, and epithelial adherens junction signaling) under both short-term and multi-generational exposures. Hypoxia also deregulated pathways specific to short-term exposure (including integrin-linked kinase, calcium, and integrin signaling) and multi-generational exposure (including sphingosine-1-phosphate signaling, endocannabinoid neuronal synapse pathway, and endoplasmic reticulum stress pathway). Overall, our results provide additional insights into the mechanisms of hypoxia disrupting neuronal function at the protein level in marine medaka.
Introduction
Hypoxia, at levels of dissolved oxygen (DO) <2.0 mg L–1, is becoming increasingly prevalent in aquatic environments. Notably, the global number of hypoxic “dead zones” has been increasing exponentially since the 1960s (Diaz and Rosenberg, 2008; Galic et al., 2018). The occurrence of aquatic hypoxia is partly exacerbated by water pollution in the form of excess nutrients from agricultural and urban waste, which leads to eutrophication (Conley et al., 2009; Jenny et al., 2016). Decreased oxygen levels generally cause adverse effects on the physiology and behavior of marine biota (Pollock et al., 2007; Galic et al., 2018). For example, hypoxia reduced the swimming speed in fish such as the Atlantic cod and dogfish (Metcalfe and Butler, 1984; Schurmann and Steffensen, 1994). Hypoxia also delayed development and growth in zebrafish embryos (Kajimura et al., 2005) and reduced the spawning, fertilization, and hatching success rates in common carp (Wu et al., 2003).
Hypoxia and its induced protein, hypoxia-inducible factor 1 (HIF-1), have been linked with several neurodegenerative diseases (Zhang et al., 2011). For example, amyotrophic lateral sclerosis (ALS) is characterized by damaged motor neurons, and hypoxia may cause ALS pathogenesis by inducing motor neuron death through impairment of HIF-1-regulated vascular endothelial growth factor (VEGF) signaling (Skene and Cleveland, 2001). While VEGF regulates blood supply and angiogenesis and is normally upregulated in response to hypoxia, ALS-like symptoms develop upon loss of the hypoxia response element in the VEGF promoter, and people with impaired VEGF activity have higher susceptibility to ALS when working in environments with a high probability of hypoxic exposure (Oosthuyse et al., 2001; Skene and Cleveland, 2001; Vanacore et al., 2010). Another example is Alzheimer’s disease, which is caused by neuron damage due to the aggregation of amyloid β (Aβ) peptides (Peers et al., 2009). Since hypoxia has been shown to increase the amyloid precursor protein (APP) levels, which are then converted to Aβ peptides (Peers et al., 2009; Zhang et al., 2011), it may be a driver of Alzheimer’s disease. Hypoxia was also reported to influence the progression of Parkinson’s disease, which is characterized by the loss of dopaminergic neurons (Zhang et al., 2011). For adult rat models of Parkinson’s disease, exposure to intermittent hypoxic episodes was found to partially restore dopamine synthesis in the right hemisphere of the brain and increase serum antioxidant activity; therefore hypoxic conditioning may delay neurodegeneration in Parkinson’s disease (Belikova et al., 2012).
Notably, multi-generational exposure to mild hypoxia can induce antioxidant responses in various neuronal cell types in the brain to promote survival and protection against oxidative stresses, such as through increased PI3K–Akt signaling and erythropoietin expression in astrocytes, increased MAPK signaling, and brain-derived neurotrophic factor expression in cortical neurons (Terraneo and Samaja, 2017; Terraneo et al., 2017). Cerebral blood and oxygen circulation is also affected by multi-generational hypoxia through increased angiogenesis and capillary density as well as elevated cerebral blood flow associated with increased nitric oxide (NO) synthesis and metabolism (Xu and LaManna, 2006; Terraneo and Samaja, 2017). In addition to antioxidant responses, multi-generational hypoxia simultaneously increases reactive oxygen species (ROS) production in the brain through the mitochondrial electron transport chain, thereby resulting in redox imbalance (Terraneo and Samaja, 2017; Terraneo et al., 2017). An overall increase in the ROS levels leads to brain damage, with neuron apoptosis occurring through the release of mitochondrial apoptotic proteins into the cytosol (Turrens, 2003; Coimbra-Costa et al., 2017).
The brain forms part of the hypothalamus–pituitary–gonad (HPG) axis, which is responsible for the regulation and production of hormones involved in reproductive function (Thomas and Rahman, 2009). Hypoxia has been reported to affect the hormone levels, reproductive development, and sex differentiation in fish (Wu et al., 2003; Yu and Wu, 2006). Moreover, hypoxia alters the proteomic profile of Oryzias melastigma testes in a transgenerational manner (Wang et al., 2016). We previously demonstrated that the transcriptomic changes in the brains of O. melastigma upon hypoxia exposure were related to brain function and development, including synaptic transmission and potassium ion transport (Lai et al., 2016). However, it remains unknown whether changes at the transcriptome level are reflected at the protein level. Therefore, in the present study, we identified changes to the brain proteome of O. melastigma in response to short-term and multi-generational hypoxia exposure.
Materials and Methods
Fish Maintenance and Hypoxia Exposure
Approval for the experimental setup involving fish was obtained from the Committee on the Use of Live Animals in Teaching and Research (CULATR, no. 2714-12) at the University of Hong Kong. O. melastigma were kept at 5.8 mg O2 L–1, 28 ± 2°C, pH 7.2, in a daily 14:10-h light/dark cycle, as previously described (Wang et al., 2016), until the start of the hypoxic/normoxic exposure experiment. For short-term exposure, sexually mature male O. melastigma were exposed to normoxic (5.8 ± 0.2 mg O2 L–1) or hypoxic (1.5 ± 0.2 mg O2 L–1) conditions, as described in Lai et al. (2016), for a period of 1 month. For multi-generational exposure, sexually mature male medaka fish were continuously exposed to either normoxic or hypoxic conditions for three generations. The progeny of every generation mated and continued to be exposed to hypoxia or normoxia, and the mate pairs (both males and females) were also kept in either normoxic or hypoxic conditions. The desired DO levels were achieved by continuously passing a constant ratio of premixed air and nitrogen through a 4-cm-wide gas-stripping column into a 300-L reservoir tank. A DO meter (YSI model 580) was used to monitor the DO levels twice per day. The temperature, salinity, pH, and ammonia levels were also kept constant and monitored daily throughout the exposure period.
Protein Extraction From Brain Tissues for iTRAQ
Total protein was extracted from the brain tissues of nine fish from each treatment group. Three brain tissues were pooled together into a single biological replicate, and three replicates were used for iTRAQ (isobaric tags for relative and absolute quantitation) analysis. Proteins were extracted using lysis buffer 3 [8 M urea, 1 mM phenylmethylsulfonyl fluoride (PMSF), 40 mM triethylammonium bicarbonate (TEAB)/Tris–HCl, 2 mM EDTA, and 10 mM dithiothreitol (DTT), pH 8.5] and two 5-mm magnetic beads. Proteins were released from the mixture using a TissueLyser (2 min, 50 Hz). The mixture was centrifuged at 25,000 × g, 4°C, for 20 min. The supernatant was transferred to a new tube, reduced using DTT (10 mM) at 56°C for 1 h, and then alkylated in the dark with iodoacetamide (IAM, 55 mM) at 25°C for 45 min. The supernatant was centrifuged at 25,000 × g, 4°C, for 20 min, and then the supernatant containing the proteins was collected. The Bradford assay was used to quantify the total protein concentration of the supernatant.
Protein Digestion and Peptide Labeling
The solution containing 100 μg protein in 8 M urea was diluted four times using TEAB (100 mM). The proteins were digested with Trypsin Gold (Promega, Madison, WI, United States) at a 40:1 protein/trypsin ratio at 37°C overnight. The digested peptides were desalted using a Strata-X C18 column (Phenomenex) and then vacuum-dried. The peptides were reconstituted in 30 μl of TEAB (0.5 M) and vortexed. The iTRAQ Reagent 8Plex Kit was used for iTRAQ labeling of the peptides following the manufacturer’s instructions. The peptides were then pooled and passed through a Strata-X C18 column (Phenomenex) for desalting and afterward vacuum-dried.
Peptide Fractionation
The peptides were dissolved in 2 ml of buffer A [95% H2O, 5% acetonitrile (ACN), pH adjusted to 9.8 with ammonia] and then loaded onto a high-pH RP column (particle size, 5 μm; Phenomenex). The peptides were passed through the column using a Shimadzu LC-20AB HPLC Pump system at a flow rate of 1 ml min–1 using the following gradient of buffer B (5% H2O, 95% ACN, pH adjusted to 9.8 with ammonia): 5% for 10 min, then 5–35% for 40 min, followed by 35–95% for 1 min and then 95% for 3 min. The gradient of buffer B was then decreased to 5% within 1 min and then maintained at 5% for 10 min. The peptides were eluted in fractions separated at 1-min intervals while monitoring absorbance at 214 nm. A total of 20 fractions were collected and vacuum-dried.
High-Performance Liquid Chromatography and Mass Spectrometry Detection
For each peptide fraction, buffer A [0.1% formic acid (FA) and 2% ACN in H2O] was added and the reconstituted fraction was centrifuged for 10 min at 20,000 × g. The supernatant was then loaded using the autosampler onto the C18 trap column of the LC-20AD nano-HPLC instrument (Shimadzu, Kyoto, Japan) at a rate of 5 μl min–1 for 8 min. The fraction was passed through the trap column and the peptide separation was performed on an self-packed 75-μm-wide analytical C18 column at a rate of 300 nl min–1 using a buffer B (0.1% FA and 2% H2O in ACN) gradient going from 8 to 35% in 35 min, then up to 60% in 5 min. The gradient was kept at 80% for 5 min and then decreased to 5% within 1 min, and the column was then equilibrated for 10 min. Mass spectrometry was done using the TripleTOF 5600 System (SCIEX, Framingham, MA, United States) fitted with a Nanospray III source (SCIEX, Framingham, MA, United States), with a pulled-quartz tip emitter (New Objectives, Woburn, MA, United States), with the setting of the ion spray voltage at 2,300 V, curtain gas and nebulizer gas of 30 and 15, respectively, and the interface heater temperature set at 150°C. Data were collected using the Analyst 1.6 software (AB SCIEX, Concord, ON, United States) on high-sensitivity mode. With a MS1 accumulation time of 250 ms, the mass range for scanning set at 350–1,500 Da, and filtering for MS scans exceeding 150 counts per second with a charge state between 2 + and 5 + and dynamic exclusion of half the peak width (12 s), up to 30 product ion scans were selected for collision-induced dissociation (CID). The collision energy was adjusted for precursor ions for CID, and a Q2 transmission window at 100% for 100 Da was used for iTRAQ data collection.
Bioinformatics Analysis and Protein Quantification
Using tandem mass spectrometry (MS/MS) data in MGF format as input, the peptides labeled with isobaric tags were quantified using IQuant software (Wen et al., 2014). The Mascot Percolator software package was used to rescore the peptide-spectrum matches (PSMs). PSMs were pre-filtered at a false discovery rate (FDR) of 1%, and then used for protein set assembly. Protein-level FDRs for the identified set of proteins were estimated using the picked protein FDR strategy (Savitski et al., 2015), and the identified proteins at a protein-level FDR < 0.01 were used for pathway, Clusters of Orthologous Groups (COG)/Eukaryotic Orthologous Group (KOG), and Gene Ontology (GO) analyses. Differentially expressed proteins (DEPs) were identified based on the filtering criteria of relative fold change > 1.2 and q-value < 0.05. The list of DEPs were used for additional GO, Kyoto Encyclopedia of Genes and Genomes (KEGG) pathway, and COG/KOG analysis.
Results
Protein Identification in the Brain of O. melastigma
By using the iTRAQ technology, a total of 1,235,781 spectra were generated, and 36,567 peptides and 7,599 proteins were identified at a FDR cutoff < 0.01 in the brain samples (Table 1). Our results demonstrated that 3,229 of the identified proteins originated from a single peptide (Figure 1A) and over 20% of the proteins had a molecular mass over 100 kDa (Figure 1B and Supplementary Table 1).
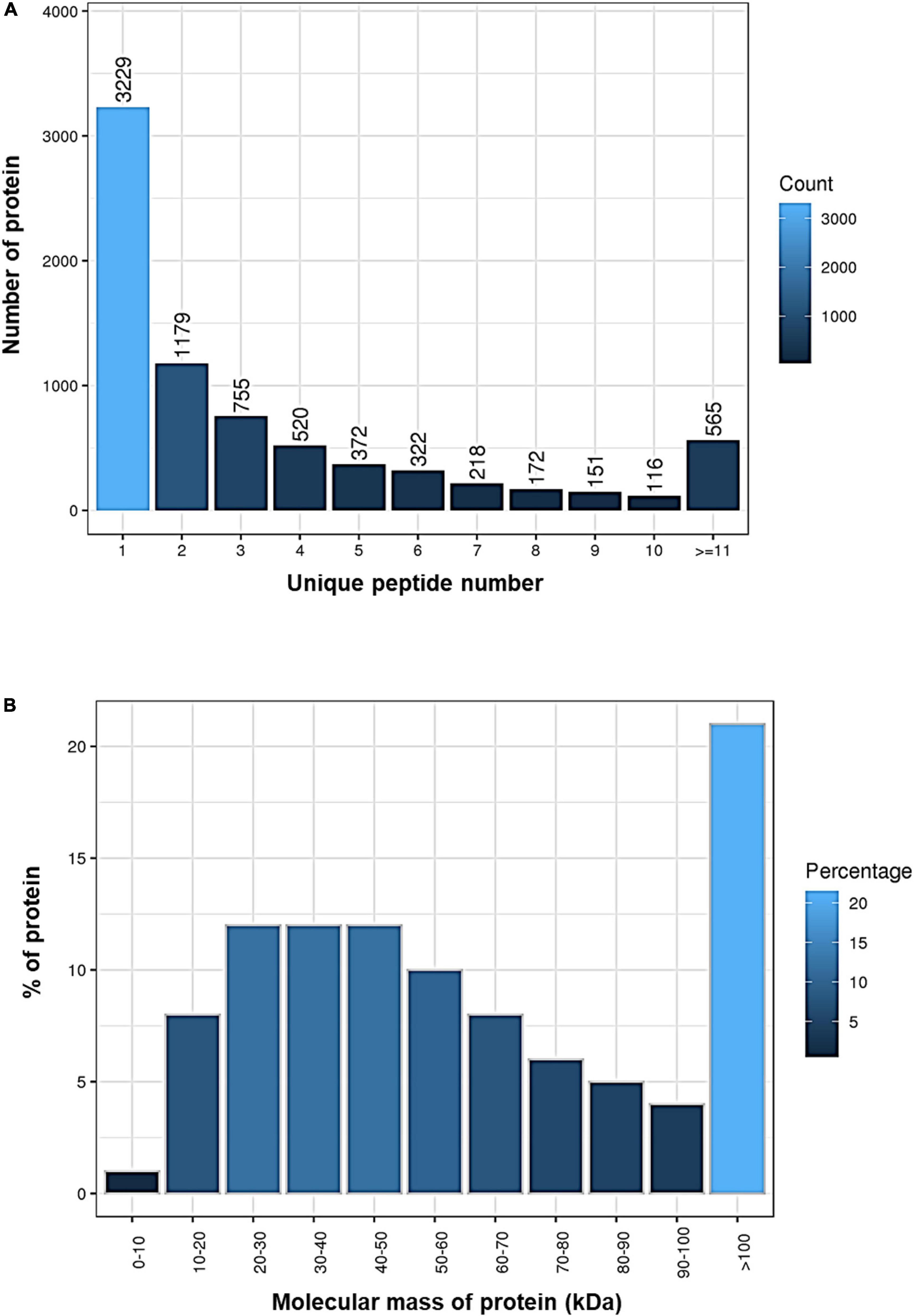
Figure 1. Identification of proteins in the brain of Oryzias melastigma using isobaric tags for relative and absolute quantitation (iTRAQ) technology. (A) Number of proteins with different unique peptide numbers. (B) Percentages of proteins with different molecular masses.
Classification of Identified Proteins in the Brain of O. melastigma
The proteins identified in the brain of O. melastigma were subjected to GO and KOG annotation to determine their functional roles in the brain. In GO annotation, the molecular functions, cellular components, and biological processes of the proteins were classified (Figure 2A). Our results indicated that the majority of these proteins were involved in binding and catalytic activity and were also responsible for structural molecule activity and signal transducer activity (Figure 2A). Many of the proteins were localized to the cell membrane, organelle, macromolecular complex, as well as the synapse (Figure 2A). In the analysis of biological processes, our results identified enrichment in terms of cellular and metabolic processes. Furthermore, these proteins also served roles in response to stimuli, developmental process, signaling, cell proliferation, and reproductive process (Figure 2A). Then, KOG annotation was conducted by matching with similar protein sequences of multiple reference genomes for functional and evolutionary genome analysis to cover three categories: (1) metabolism, (2) information storage and processing, and (3) cellular processing and signaling (Figure 2B). We identified the involvement of these proteins in the transport and metabolism of a large number of molecules, including lipids, inorganic ions, amino acids, carbohydrates, and coenzymes. Additionally, energy production and conversion were also highlighted (Figure 2B).
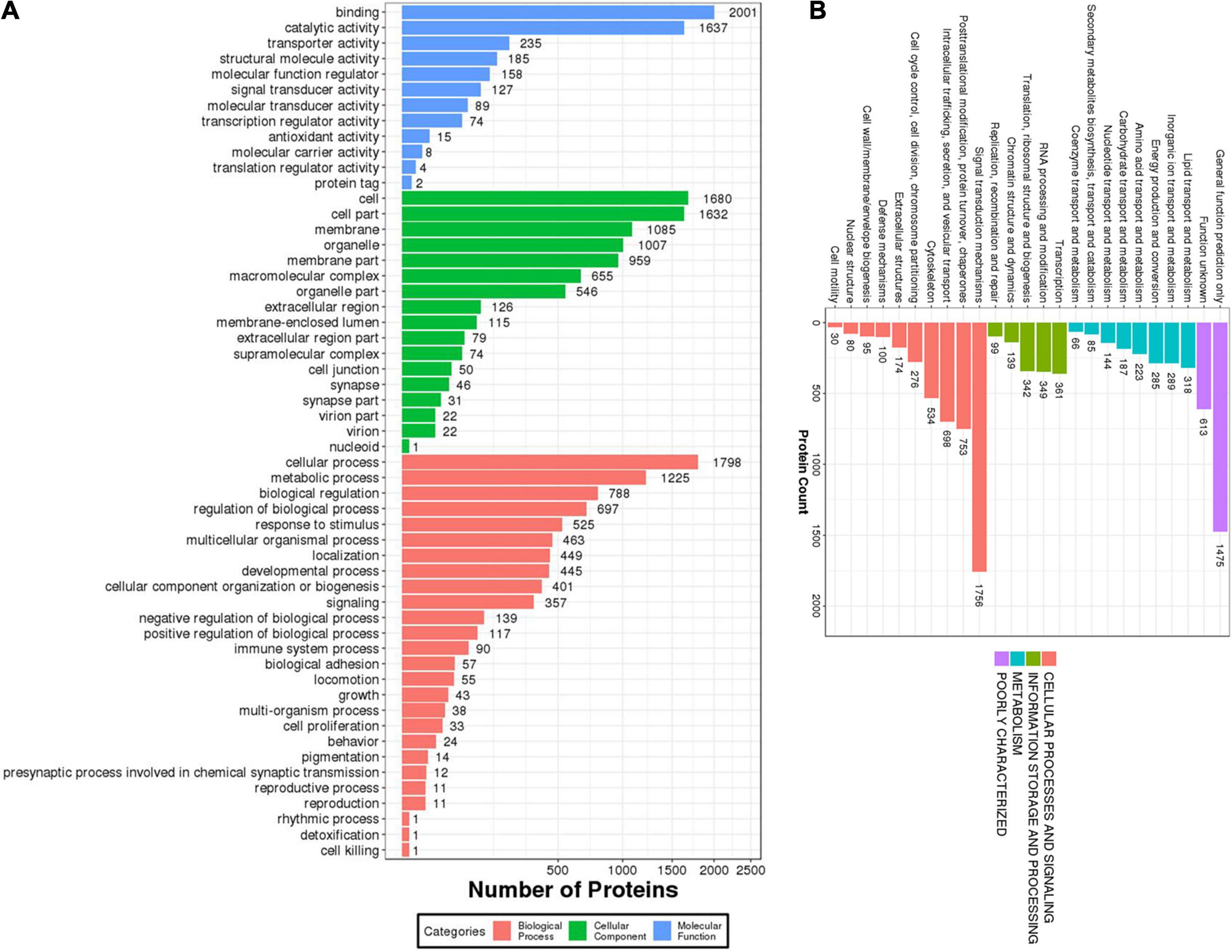
Figure 2. Functional characterization of the identified proteins in the brain of Oryzias melastigma. (A) Gene Ontology (GO) annotation of the biological processes, cellular components, and molecular functions mediated by the identified proteins. (B) Eukaryotic Orthologous Group (KOG) annotation of the cellular processes and signaling, information storage and processing, and metabolism mediated by the identified proteins.
Effect of Hypoxia on Differential Protein Expression
A comparative proteomic analysis was conducted to determine the differential protein expression in hypoxic brains of O. melastigma. Our results indicate that short-term hypoxia exposure could lead to the deregulated expression of 33 upregulated and 69 downregulated proteins in the F0 generation (Figure 3A and Table 2). In the multi-generational exposure group, we observed the upregulation of 24 proteins and the downregulation of 52 proteins relative to the corresponding normoxic group (Figure 3B and Table 3). Then, we compared the identified differentially expressed proteins with our previous report of transcriptome data (Lai et al., 2016). We found five and seven common deregulated genes–proteins in short-term and multi-generational hypoxia exposures, respectively (Table 4).

Table 2. Differentially expressed proteins in the brain of Oryzias melastigma following short-term hypoxia exposure.
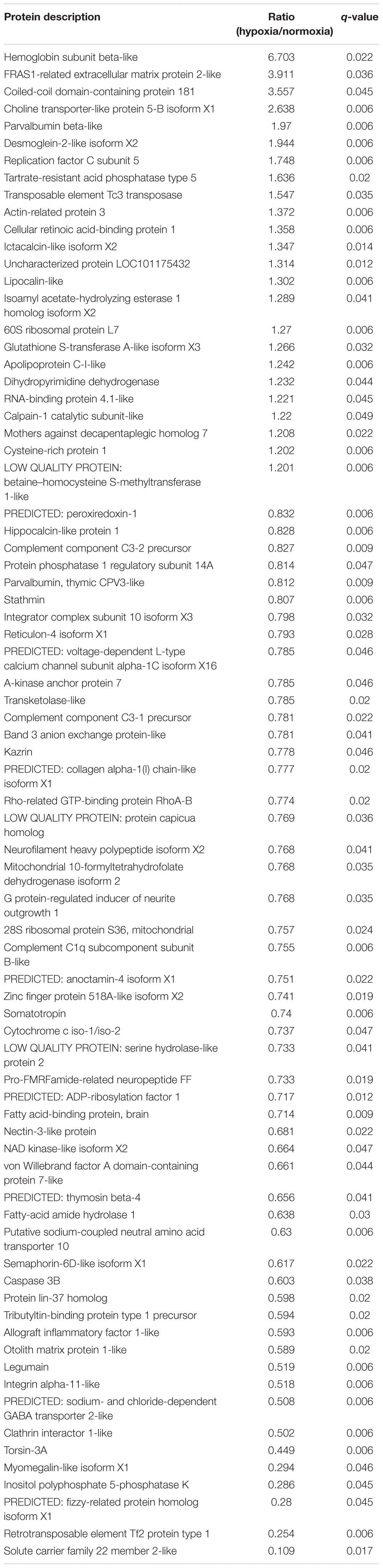
Table 3. Differentially expressed proteins in the brain of Oryzias melastigma following long-term hypoxia exposure.
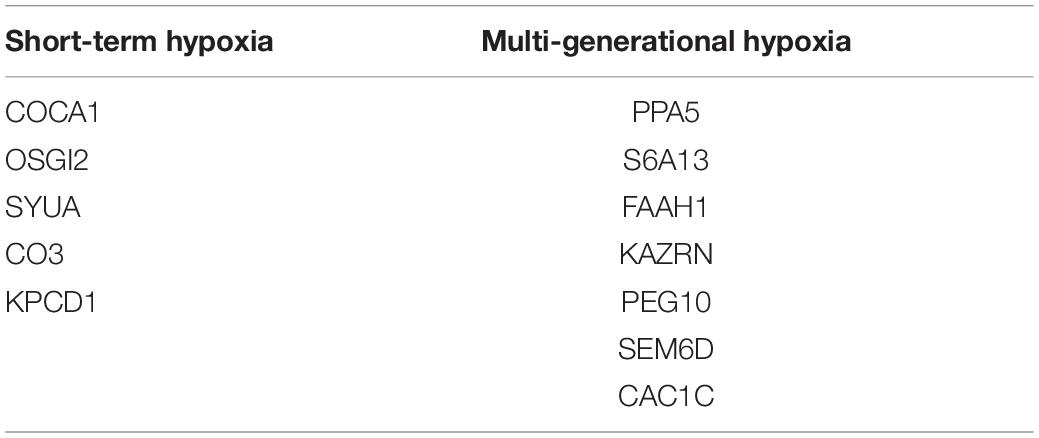
Table 4. Common deregulated genes and proteins after short-term and multi-generational hypoxia exposures.
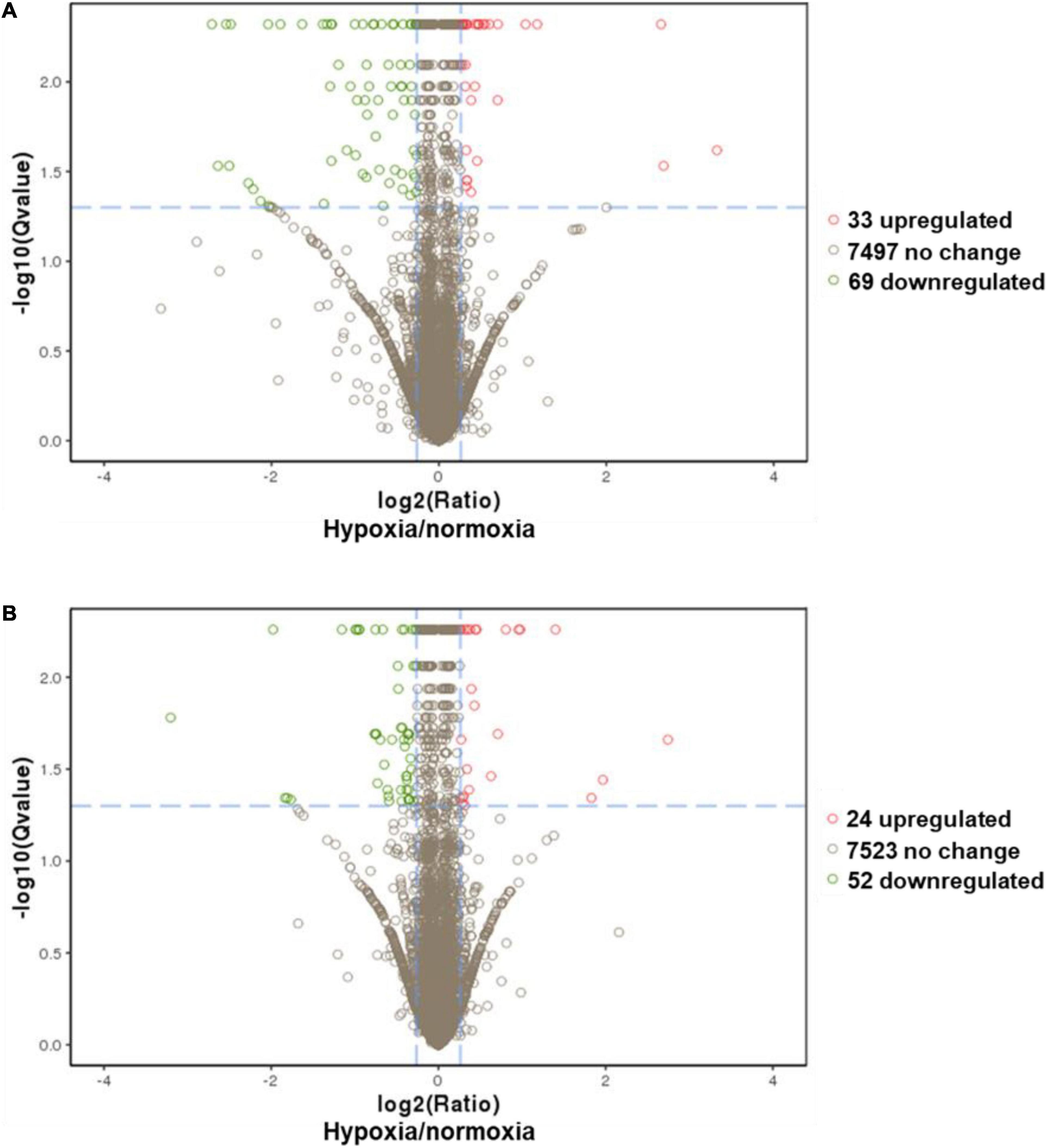
Figure 3. Differential expressions of proteins in the brain of Oryzias melastigma caused by hypoxia. (A) Short-term hypoxia exposure causes the deregulation of protein expression. (B) Multi-generational hypoxia exposure causes the deregulation of protein expression. The x-axis represents the relative expression of proteins (hypoxia/normoxia). The y-axis represents the -log(q-value). The cutoffs for the determination of differentially expressed proteins are -log(q-value) >1.3 and hypoxia to normoxia ratio > 1.2. Green bubbles represent downregulated proteins and red bubbles represent upregulated proteins under hypoxia exposure.
Effects of Hypoxia on Neural Functions
To understand the neurotoxicological effects of hypoxia, the hypoxia-induced DEPs were subjected to GO and pathway enrichment analysis. Through GO enrichment analysis, we determined that short-term (Figure 4A and Supplementary Table 2) and multi-generational (Figure 4B and Supplementary Table 3) hypoxia exposures could lead to significantly different alterations of the biological processes and molecular functions (p < 0.05). For biological processes, short-term hypoxia exposure affected a wide range of lipid metabolic processes such as phospholipids, glycerolipids, glycerophospholipids, and phosphatidylinositols. Additionally, phosphate-containing compound metabolic processes, including phosphorus and organophosphate, were interrupted by short-term hypoxia exposure (Figure 4A and Supplementary Table 2). Interestingly, multi-generational hypoxia exposure led to the alteration of the different acid biosynthetic processes such as carboxylic acids, organic acids, and cellular amino acids. Additionally, multi-generational hypoxia exposure could trigger a response to toxic substances and result in cell death and apoptosis. More importantly, multi-generational hypoxia exposure can dysregulate the immune system (Figure 4B and Supplementary Table 3). Through pathway enrichment analysis, we determined that short-term hypoxia exposure altered the arachidonic acid metabolism and tight junctions in the brain tissue (p < 0.05) (Figures 4C,D). Furthermore, this could lead to hypertrophic cardiomyopathy through the dysregulation of adrenergic signaling and to Alzheimer’s disease (p < 0.05) (Figures 4C,D). On the other hand, multi-generational hypoxia exposure could lead to a larger number of alterations in the brain of O. melastigma (p < 0.05). This could trigger the development of neuronal diseases such as Alzheimer’s disease and amyotrophic lateral sclerosis (p < 0.05) (Figures 4E,F). Additionally, multi-generational exposure can also lead to tuberculosis, atherosclerosis, and various cancers, which possibly occur through the dysregulation of certain signaling pathways, such as the p53 signaling pathway and the PI3K–Akt signaling pathway (p < 0.05) (Figures 4E,F). Taken together, our results suggest that short-term and multi-generational exposures could lead to different neural responses in the brains of fish.
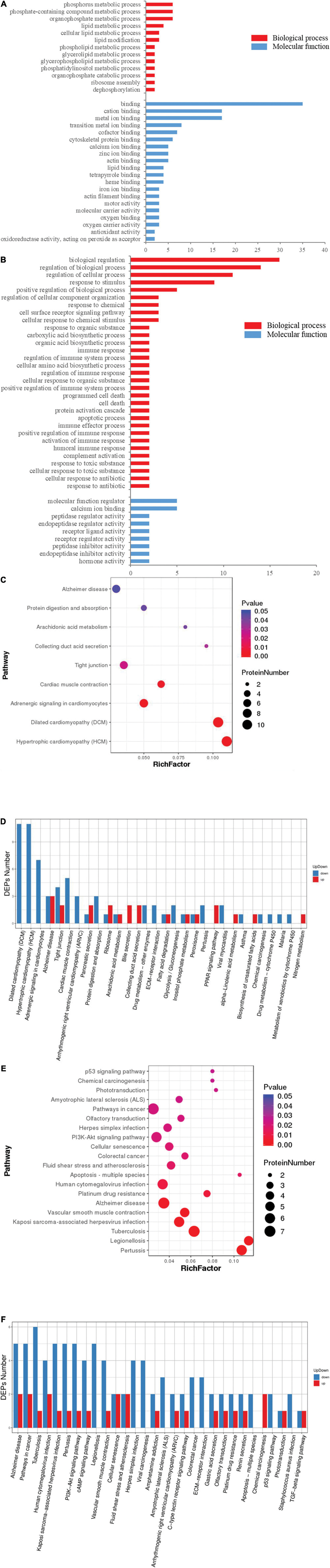
Figure 4. Functional distortion in the brain of Oryzias melastigma caused by short-term and multi-generational hypoxia exposures. Gene ontology analysis of differentially expressed proteins obtained from (A) short-term hypoxia exposure and (B) multi-generational hypoxia exposure. Red bars represent biological processes and blue bars represent molecular functions. Pathway enrichment analyses including (C) rich factor analysis and (D) Koto Encyclopedia of Genes and Genomes (KEGG) pathway analysis were used to determine alterations of the pathways caused by short-term hypoxia exposure. The effects of multi-generational hypoxia exposure are shown using (E) rich factor analysis and (F) KEGG pathway analysis.
Mechanisms Underlying the Neurotoxicological Effect of Hypoxia
To elucidate the possible molecular mechanisms behind the effects of hypoxia on brain toxicology in fish, ingenuity pathway analysis (IPA) was conducted. The canonical pathway analysis in IPA highlighted both similar and different responses of the brain to short-term and multi-generational hypoxia exposures. The results indicate that both short-term and multi-generational hypoxia exposures could lead to the alteration of integrin signaling, paxillin signaling, Parkinson’s signaling, epithelial adherens junction signaling, and D-myo-inositol (1,4,5)-trisphosphate degradation and metabolism (Table 5). However, only integrin subunit alpha 11 (ITGA11) and inositol polyphosphate-5-phosphatase J (INPP5J) were found to be commonly deregulated under these two hypoxia treatments. Furthermore, certain pathways that serve important roles in brain function were observed to be unique to either short-term or multi-generational hypoxia exposure. Under short-term hypoxia exposure, ILK signaling, tight junction signaling, calcium signaling, integrin signaling, RhoA signaling, CXCR4 signaling, thrombin signaling, mTOR signaling, calcium transport I, protein kinase A signaling, and PAK signaling were associated with neurological diseases or functions and were highlighted in the analysis. These signaling pathways were controlled by a cluster of proteins including Actn3, MYH6, MYL2, MYL4, PPP2R3A, TNNT3, TTN, PRKD1, PRKD1, RPS27, and ANXA5 (Table 6 and Supplementary Table 4). Under multi-generational hypoxia exposure, another group of pathways including anandamide degradation, GABA receptor signaling, the sumoylation pathway, pentose phosphate pathway, sphingosine-1-phosphate signaling, endocannabinoid neuronal synapse pathway, and endoplasmic reticulum stress pathway were altered. The DEPs involved in these pathways included FAAH, CACNA1C, SLC6A13, RFC5, RHOA, TKT, and CASP3 (Table 7 and Supplementary Table 5). These data suggest that short-term and multi-generational hypoxia exposures could lead to both common and unique mechanisms of neurotoxicity in marine fish.

Table 5. Common deregulated canonical pathways in the brain of Oryzias melastigma caused by short-term and multi-generational hypoxia exposures.
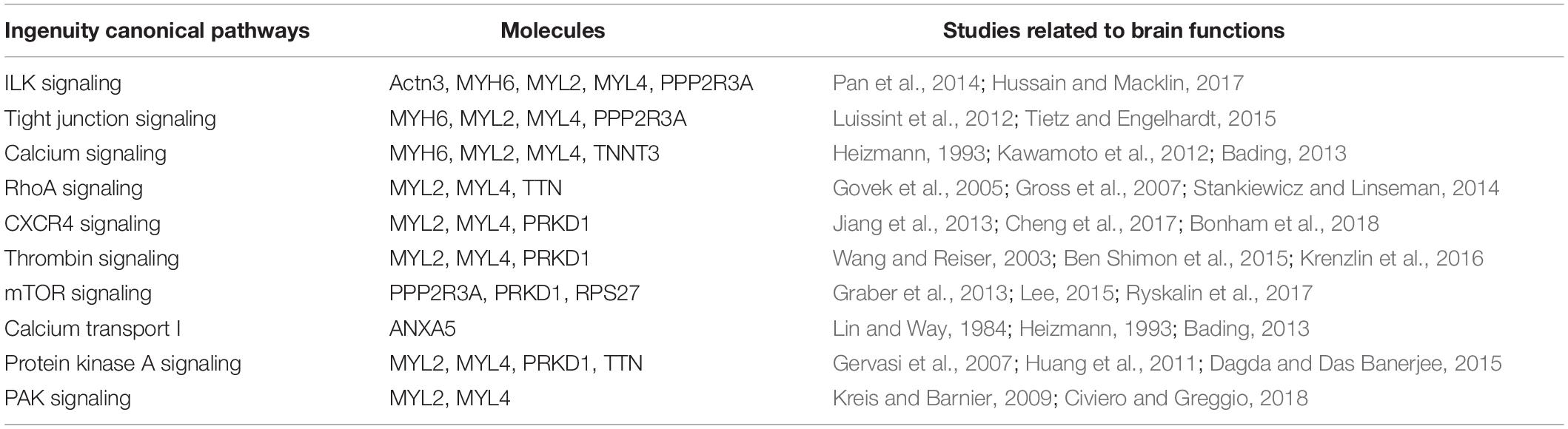
Table 6. Unique deregulated canonical pathways in the brain of Oryzias melastigma caused by short-term hypoxia exposure.
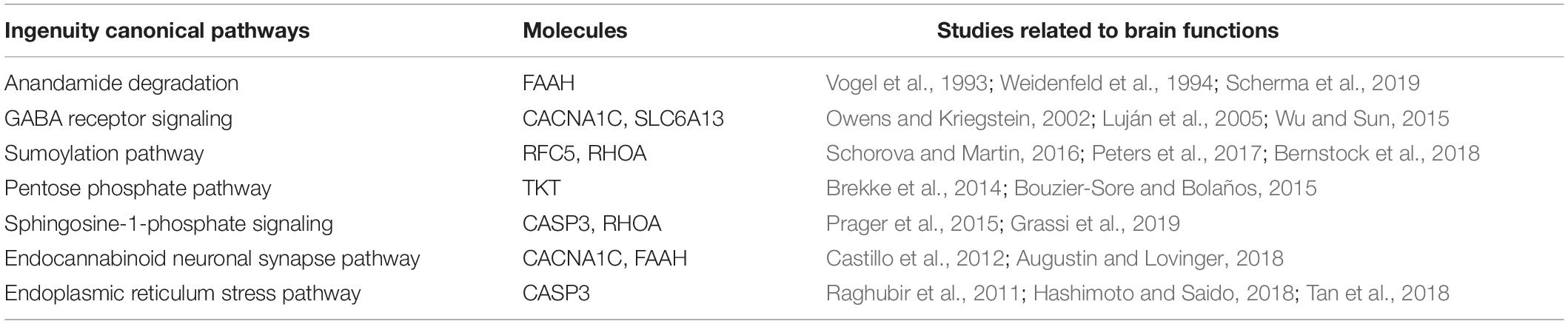
Table 7. Unique deregulated canonical pathways in the brain of Oryzias melastigma caused by multi-generational hypoxia exposure.
Discussion
Hypoxia is a pressing global problem facing marine ecosystems, and it is believed that this problem cannot be solved in the near future due to global warming. Studies have demonstrated that hypoxia is an endocrine disruptor, affecting both male and female reproductive systems and interfering with the hormonal reproductive hormonal system through the HPG axis. As the core of the HPG axis, the brain was reported to be interrupted by hypoxia. We have previously shown that hypoxic stress induced transcriptomic changes in the brain of O. melastigma. However, studies of the hypoxic responses of proteomes in other organs and tissues of fish remain limited. For example, in the heart of goldfish, hypoxia deregulated five enzymes involved in glycolysis and gluconeogenesis, including fructose-1,6-bisphosphate aldolase (Imbrogno et al., 2019). Presently, we attempted to elucidate the proteomic changes of fish brains after short-term and multi-generational hypoxia exposures.
In the first part of our study, we identified proteins in the brain of O. melastigma for functional analysis, which demonstrated that these proteins contribute to important brain functions. These functions include signal transducer activity, which is important for the functioning of neurotransmitters and hormones in regulating neuronal functions (Cooper, 2000). One possible outcome is the onset of chronic neurodegenerative diseases. For example, Alzheimer’s disease was highlighted in our results. More importantly, we identified some proteins that were associated with reproductive processes, including WBP2 N-terminal like, double-strand break repair protein, male-enhanced antigen 1, spindlin, and synaptonemal complex protein 1. Of the identified proteins, WBP2 N-terminal like was reported to serve an important role in spermatozoa and oocyte activation (Hamilton et al., 2018; Gonzalez-Castro et al., 2019). The other identified reproductive protein, double-strand break repair protein, is responsible for generating either non-crossovers or crossovers during the process of homologous recombination in meiosis (Gray and Cohen, 2016). We also identified the male-enhanced antigen 1 (mea1) in the brain of medaka. Notably, the mea1 protein was reported to be expressed specifically in elongated spermatids and residual bodies, which suggests a functional role in spermiogenesis (Ohinata et al., 2002). Spindlin, a maternal effect factor associated with the meiotic spindle during the meiosis of oocytes (Zhang et al., 2015), is responsible for the oocyte-to-embryo transition in carp fish (Sun et al., 2010). Synaptonemal complex protein 1 (SYP-1), which is localized to the short arms of chromosomes, serves a role in recruiting the chromosomal passenger complex and phosphorylated histone H3 to the short arm of chromosomes during meiosis I, thereby promoting the disjunction of chromosomes in meiosis I (Sato-Carlton et al., 2017).
After elucidating the functions of the identified proteins, we investigated the adverse effects of both short-term and multi-generational hypoxia exposures. Upon comparing the results, we observed some common deregulation of the cell signaling pathways, including integrin signaling, paxillin signaling, and Parkinson’s signaling, that are related to neural functions and diseases caused by both short-term and multi-generational hypoxia exposures. Integrins are a class of transmembrane receptors involved in cell adhesion to the extracellular matrix (ECM) (Giancotti, 1999) and are expressed in various brain sections, including the hippocampus and cortex (Pinkstaff et al., 1999). As receptors, integrins play a crucial role in the pathophysiology of many brain diseases via signal transmissions from the ECM to the cell. Notably, aberrations in integrin signaling within the brain may impair the synaptic activity in brain disorders such as schizophrenia and epilepsy (Wu and Reddy, 2012). Paxillin is an adaptor protein for focal adhesions that coordinates the integrin-mediated rearrangements of the cytoskeleton (Rashid et al., 2017). In human cancer research, paxillin was identified as an oncogene that promotes cell migration and invasion in gliomas (Chen et al., 2016). Additionally, hypoxia could induce the invasive potential of glioblastomas through the HIF-2α–EPHB2–paxillin axis, thereby suggesting a possible interaction between hypoxia and paxillin signaling (Qiu et al., 2019). Parkinson’s disease is a progressive neurodegenerative disorder (Beitz, 2014). Recently, it has been reported that oxidative stress may play a role in Parkinson’s disease (Islam, 2016). Moreover, numerous studies on signaling in Parkinson’s disease models have demonstrated a link between hypoxia-inducible factors and Parkinson’s disease (Kim et al., 2020; Zhang et al., 2020). Therefore, the results of our study further suggest hypoxia as a possible cause of Parkinson’s disease.
Apart from the common effects, both short-term and multi-generational hypoxia exposures could lead to unique neural alterations. Notably, the metabolisms of various classes of lipids (e.g., phospholipids, glycerophospholipids, and phosphatidylinositols) were found to be affected by short-term hypoxia exposure. Phospholipids (glycerophospholipids and sphingolipids) are major structural components of the plasma membrane of various cell types of the brain, including neurons and glial cells (Adibhatla and Hatcher, 2007; Kosicek and Hecimovic, 2013). Neural membrane function can be affected by their glycerophospholipid composition (Farooqui et al., 2000). Imbalances of phospholipid metabolism have previously been reported in cases of Alzheimer’s disease (Kosicek and Hecimovic, 2013). Furthermore, short-term hypoxia exposure also affected arachidonic acid metabolism in the fish brain. Adequate levels of arachidonic acid, a polyunsaturated fatty acid, are required for normal cerebral functions and structures, including synaptic signaling and neurotransmitter release (Ojeda et al., 1989; Kaufmann et al., 1996; Contreras and Rapoport, 2002). Altered brain arachidonic acid metabolism has previously been associated with several neurological, neurodegenerative, and brain diseases, including schizophrenia and Parkinson’s and Alzheimer’s disease (Müller et al., 2002; Teismann et al., 2003; Esposito et al., 2008). Unlike the responses to short-term hypoxia exposure, multi-generational hypoxia exposure could lead to the alteration of the carboxylic acid metabolism in the fish brain. Carboxylic acids are a class of organic acids characterized by a carboxyl (-COOH) functional group. Various carboxylic acids have been reported to play important roles in neural functions. For example, 5-methoxyindole-2-carboxylic acid can mitigate brain damage from stroke (Sumien et al., 2019), while losartan carboxylic acid, the potent AT1 blocker metabolite of losartan, improved motor coordination and enabled central nervous system protection from an increase in locomotor activity (Subudhi et al., 2018). Apart from the deregulation of organic acid metabolism, long-term hypoxia exposure could also alter certain cell signaling pathways in the brain, such as PI3K–Akt signaling and p53 signaling. PI3K–Akt is an important signaling pathway in cell proliferation and cell cycle control (Yu and Cui, 2016). It has been reported that aberrations in PI3K–Akt signaling may be involved in the pathogenesis of Alzheimer’s disease (Gabbouj et al., 2019) and brain malformation (Jansen et al., 2015). Notably, an Alzheimer’s disease mouse model demonstrated that the activation of PI3K–Akt signaling could mitigate neurodegeneration and memory impairment (Ali et al., 2017). For example, the transcription factor p53, a mediator of cellular response to stress, is required for normal brain development (Contreras et al., 2018). More importantly, p53 is the key mediator of autophagy and apoptosis, processes that are known to be dysregulated in ALS and Parkinson’s and Alzheimer’s diseases (Ghavami et al., 2014). Interestingly, some cancer studies reported that the p53 and Akt pathways were linked to the modulation of apoptosis under hypoxic conditions (Leszczynska et al., 2015; Yun and Glazer, 2015). In line with the above results, ALS, which is characterized by the deterioration of motor neurons (McCombe et al., 2020), was also highlighted in our analysis. Therefore, multi-generational hypoxia exposure could result in behavioral disorders in fish via neurodegeneration. Due to the harmful effects of this potential interaction, future studies should be conducted to address this issue.
Data Availability Statement
The datasets presented in this study can be found in online repositories. The names of the repository/repositories and accession number(s) can be found below: http://www.ebi.ac.uk/pride/archive/projects/PXD022579.
Ethics Statement
The animal study was reviewed and approved by Committee on the Use of Live Animals in Teaching and Research (CULATR, #2714-12) at the University of Hong Kong.
Author Contributions
KL and RK contributed to the conception, organization, and execution of the research project. SW and NT contributed to the experimental setup and sample preparation. YT, JZ, WT, XL, TC, and DA contributed to the bioinformatic analysis and statistical analysis. RW contributed to manuscript preparation. All authors contributed to the article and approved the submitted version.
Funding
This work was supported by a Basic Research Free Exploration Project Grant (Grant No. R-BTC4801) to DA and an IRF grant of the State Key Laboratory of Marine Pollution of the City University of Hong Kong (Grant No. IRF_2017_0025) to RK.
Conflict of Interest
The authors declare that the research was conducted in the absence of any commercial or financial relationships that could be construed as a potential conflict of interest.
Acknowledgments
KL was supported by the “Hong Kong SAR, Macao SAR and Taiwan Province Talent Young Scientist Program of Guangxi”.
Supplementary Material
The Supplementary Material for this article can be found online at: https://www.frontiersin.org/articles/10.3389/fmars.2021.618489/full#supplementary-material
References
Adibhatla, R. M., and Hatcher, J. F. (2007). Role of lipids in brain injury and diseases. Future Lipidol. 2, 403–422. doi: 10.2217/17460875.2.4.403
Ali, T., Kim, T., Rehman, S. U., Khan, M. S., Amin, F. U., Khan, M., et al. (2017). Natural dietary supplementation of anthocyanins via PI3K/Akt/Nrf2/HO-1 pathways mitigate oxidative stress, neurodegeneration, and memory impairment in a mouse model of Alzheimer’s disease. Mol. Neurobiol. 55, 6076–6093. doi: 10.1007/s12035-017-0798-6
Augustin, S. M., and Lovinger, D. M. (2018). Functional relevance of endocannabinoid-dependent synaptic plasticity in the central nervous system. ACS Chem. Neurosci. 9, 2146–2161. doi: 10.1021/acschemneuro.7b00508
Bading, H. (2013). Nuclear calcium signalling in the regulation of brain function. Nat. Rev. Neurosci. 14, 593–608. doi: 10.1038/nrn3531
Belikova, M. V., Kolesnikova, E. E., and Serebrovskaya, T. V. (2012). “Intermittent hypoxia and experimental Parkinson’s disease,” in Intermittent Hypoxia and Human Diseases, eds L. Xi and T. Serebrovskaya (London: Springer), 147–153. doi: 10.1007/978-1-4471-2906-6_12
Ben Shimon, M., Lenz, M., Ikenberg, B., Becker, D., Shavit Stein, E., Chapman, J., et al. (2015). Thrombin regulation of synaptic transmission and plasticity: implications for health and disease. Front. Cell. Neurosci. 9:151. doi: 10.3389/fncel.2015.00151
Bernstock, J. D., Yang, W., Ye, D. G., Shen, Y., Pluchino, S., Lee, Y. J., et al. (2018). SUMOylation in brain ischemia: patterns, targets, and translational implications. J. Cerebr. Blood Flow Metab. 38, 5–16. doi: 10.1177/0271678X17742260
Bonham, L. W., Karch, C. M., Fan, C. C., Tan, C., Geier, E. G., Wang, Y., et al. (2018). CXCR4 involvement in neurodegenerative diseases. Transl. Psychiatry 8:73. doi: 10.1038/s41398-017-0049-7
Bouzier-Sore, A. K., and Bolaños, J. P. (2015). Uncertainties in pentose-phosphate pathway flux assessment underestimate its contribution to neuronal glucose consumption: relevance for neurodegeneration and aging. Front. Aging Neurosci. 7:89. doi: 10.3389/fnagi.2015.00089
Brekke, E. M., Morken, T. S., Widerøe, M., Håberg, A. K., Brubakk, A. M., and Sonnewald, U. (2014). The pentose phosphate pathway and pyruvate carboxylation after neonatal hypoxic-ischemic brain injury. J. Cerebr. Blood Flow Metab. 34, 724–734. doi: 10.1038/jcbfm.2014.8
Castillo, P. E., Younts, T. J., Chávez, A. E., and Hashimotodani, Y. (2012). Endocannabinoid signaling and synaptic function. Neuron 76, 70–81. doi: 10.1016/j.neuron.2012.09.020
Chen, B., Xia, L., Xu, C., Xiao, F., and Wang, Y.-F. (2016). Paxillin functions as an oncogene in human gliomas by promoting cell migration and invasion. OncoTargets Therapy 9, 6935–6943. doi: 10.2147/ott.s114229
Cheng, X., Wang, H., Zhang, X., Zhao, S., Zhou, Z., Mu, X., et al. (2017). The role of SDF-1/CXCR4/CXCR7 in neuronal regeneration after cerebral ischemia. Front. Neurosci. 11:590. doi: 10.3389/fnins.2017.00590
Civiero, L., and Greggio, E. (2018). PAKs in the brain: function and dysfunction. Biochim. Biophys. Acta Mol. Basis Dis. 1864, 444–453. doi: 10.1016/j.bbadis.2017.11.005
Coimbra-Costa, D., Alva, N., Duran, M., Carbonell, T., and Rama, R. (2017). Oxidative stress and apoptosis after acute respiratory hypoxia and reoxygenation in rat brain. Redox Biol. 12, 216–225. doi: 10.1016/j.redox.2017.02.014
Conley, D. J., Paerl, H. W., Howarth, R. W., Boesch, D. F., Seitzinger, S. P., Havens, K. E., et al. (2009). Ecology: controlling eutrophication: nitrogen and phosphorus. Science 323, 1014–1015. doi: 10.1126/science.1167755
Contreras, E. G., Sierralta, J., and Glavic, A. (2018). p53 is required for brain growth but is dispensable for resistance to nutrient restriction during Drosophila larval development. PLoS One 13:e0194344. doi: 10.1371/journal.pone.0194344
Contreras, M. A., and Rapoport, S. I. (2002). Recent studies on interactions between n-3 and n-6 polyunsaturated fatty acids in brain and other tissues. Curr. Opin. Lipidol. 13, 267–272. doi: 10.1097/00041433-200206000-00006
Dagda, R. K., and Das Banerjee, T. (2015). Role of protein kinase A in regulating mitochondrial function and neuronal development: implications to neurodegenerative diseases. Rev. Neurosci. 26, 359–370. doi: 10.1515/revneuro-2014-0085
Diaz, R. J., and Rosenberg, R. (2008). Spreading dead zones and consequences for marine ecosystems. Science 321, 926–929. doi: 10.1126/science.1156401
Esposito, G., Giovacchini, G., Liow, J.-S., Bhattacharjee, A. K., Greenstein, D., Schapiro, M., et al. (2008). Imaging neuroinflammation in Alzheimer’s disease with radiolabeled arachidonic acid and PET. J. Nuclear Med. 49, 1414–1421. doi: 10.2967/jnumed.107.049619
Farooqui, A. A., Horrocks, L. A., and Farooqui, T. (2000). Glycerophospholipids in brain: their metabolism, incorporation into membranes, functions, and involvement in neurological disorders. Chem. Phys. Lipids 106, 1–29. doi: 10.1016/s0009-3084(00)00128-6
Gabbouj, S., Ryhänen, S., Marttinen, M., Wittrahm, R., Takalo, M., Kemppainen, S., et al. (2019). Altered insulin signaling in Alzheimer’s disease brain – special emphasis on PI3K-Akt pathway. Front. Neurosci. 13:629. doi: 10.3389/fnins.2019.00629
Galic, N., Hawkins, T., and Forbes, V. E. (2018). Adverse impacts of hypoxia on aquatic invertebrates: a meta-analysis. Sci. Total Environ. 652, 736–743. doi: 10.1016/j.scitotenv.2018.10.225
Gervasi, N., Hepp, R., Tricoire, L., Zhang, J., Lambolez, B., Paupardin-Tritsch, D., et al. (2007). Dynamics of protein kinase A signaling at the membrane, in the cytosol, and in the nucleus of neurons in mouse brain slices. J. Neurosci. 27, 2744–2750. doi: 10.1523/JNEUROSCI.5352-06.2007
Ghavami, S., Shojaei, S., Yeganeh, B., Ande, S. R., Jangamreddy, J. R., Mehrpour, M., et al. (2014). Autophagy and apoptosis dysfunction in neurodegenerative disorders. Prog. Neurobiol. 112, 24–49. doi: 10.1016/j.pneurobio.2013.10.004
Giancotti, F. G. (1999). Integrin signaling. Science 285, 1028–1033. doi: 10.1126/science.285.5430.1028
Gonzalez-Castro, R. A., Amoroso-Sanches, F., Stokes, J. E., Graham, J. K., and Carnevale, E. M. (2019). Localisation of phospholipase Cζ1 (PLCZ1) and postacrosomal WW-binding protein (WBP2 N-terminal like) on equine spermatozoa and flow cytometry quantification of PLCZ1 and association with cleavage invitro. Reproduct. Fertil. Dev. 31, 1778–1792. doi: 10.1071/rd19217
Govek, E. E., Newey, S. E., and Van Aelst, L. T. (2005). The role of the Rho GTPases in neuronal development. Genes Dev. 19, 1–49. doi: 10.1101/gad.1256405
Graber, T. E., McCamphill, P. K., and Sossin, W. S. (2013). A recollection of mTOR signaling in learning and memory. Learn. Mem. 20, 518–530. doi: 10.1101/lm.027664.112
Grassi, S., Mauri, L., Prioni, S., Cabitta, L., Sonnino, S., Prinetti, A., et al. (2019). Sphingosine 1-phosphate receptors and metabolic enzymes as druggable targets for brain diseases. Front. Pharmacol. 10:807. doi: 10.3389/fphar.2019.00807
Gray, S., and Cohen, P. E. (2016). Control of meiotic crossovers: from double-strand break formation to designation. Annu. Rev. Genet. 50, 175–210. doi: 10.1146/annurev-genet-120215-035111
Gross, R. E., Mei, Q., Gutekunst, C.-A., and Torre, E. (2007). The pivotal role of RhoA GTPase in the molecular signaling of axon growth inhibition after CNS injury and targeted therapeutic strategies. Cell Transplant. 16, 245–262. doi: 10.3727/000000007783464740
Hamilton, L. E., Suzuki, J., Acteau, G., Shi, M., Xu, W., Meinsohn, M.-C., et al. (2018). WBP2 shares a common location in mouse spermatozoa with WBP2NL/PAWP and like its descendent is a candidate mouse oocyte activating factor. Biol. Reproduct. 99, 1171–1183. doi: 10.1093/biolre/ioy156
Hashimoto, S., and Saido, T. C. (2018). Critical review: involvement of endoplasmic reticulum stress in the aetiology of Alzheimer’s disease. Open Biol. 8:180024. doi: 10.1098/rsob.180024
Huang, W., Zhou, Z., Asrar, S., Henkelman, M., Xie, W., and Jia, Z. (2011). p21-Activated kinases 1 and 3 control brain size through coordinating neuronal complexity and synaptic properties. Mol. Cell. Biol. 31, 388–403. doi: 10.1128/MCB.00969-10
Hussain, R., and Macklin, W. B. (2017). Integrin-linked kinase (ILK) deletion disrupts oligodendrocyte development by altering cell cycle. J. Neurosci. 37, 397–412. doi: 10.1523/JNEUROSCI.2113-16.2016
Imbrogno, S., Aiello, D., Filice, M., Leo, S., Mazza, R., Cerra, M. C., et al. (2019). MS-based proteomic analysis of cardiac response to hypoxia in the goldfish (Carassius auratus). Sci. Rep. 9:18953. doi: 10.1038/s41598-019-55497-w
Islam, M. T. (2016). Oxidative stress and mitochondrial dysfunction-linked neurodegenerative disorders. Neurol. Res. 39, 73–82. doi: 10.1080/01616412.2016.1251711
Jansen, L. A., Mirzaa, G. M., Ishak, G. E., O’Roak, B. J., Hiatt, J. B., Roden, W. H., et al. (2015). PI3K/AKT pathway mutations cause a spectrum of brain malformations from megalencephaly to focal cortical dysplasia. Brain 138, 1613–1628. doi: 10.1093/brain/awv045
Jenny, J.-P., Francus, P., Normandeau, A., Lapointe, F., Perga, M.-E., Ojala, A., et al. (2016). Global spread of hypoxia in freshwater ecosystems during the last three centuries is caused by rising local human pressure. Glob. Change Biol. 22, 1481–1489. doi: 10.1111/gcb.13193
Jiang, Z., Zhou, W., Guan, S., Wang, J., and Liang, Y. (2013). Contribution of SDF-1a/CXCR4 signaling to brain development and glioma progression. Neurosignals 21, 240–258. doi: 10.1159/000339091
Kajimura, S., Aida, K., and Duan, C. (2005). Insulin-like growth factor-binding protein-1 (IGFBP-1) mediates hypoxia-induced embryonic growth and developmental retardation. Proc. Natl. Acad. Sci. U.S.A. 102, 1240–1245. doi: 10.1073/pnas.0407443102
Kaufmann, W. E., Worley, P. F., Pegg, J., Bremer, M., and Isakson, P. (1996). COX-2, a synaptically induced enzyme, is expressed by excitatory neurons at postsynaptic sites in rat cerebral cortex. Proc. Natl. Acad. Sci. U.S.A. 93, 2317–2321. doi: 10.1073/pnas.93.6.2317
Kawamoto, E. M., Vivar, C., and Camandola, S. (2012). Physiology and pathology of calcium signaling in the brain. Front. Pharmacol. 3:61. doi: 10.3389/fphar.2012.00061
Kim, S., Lee, M., and Choi, Y. K. (2020). The role of a neurovascular signaling pathway involving hypoxia-inducible factor and notch in the function of the central nervous system. Biomolecules Ther. 28, 45–57. doi: 10.4062/biomolther.2019.119
Kosicek, M., and Hecimovic, S. (2013). Phospholipids and Alzheimer’s disease: alterations, mechanisms and potential biomarkers. Int. J. Mol. Sci. 14, 1310–1322. doi: 10.3390/ijms14011310
Kreis, P., and Barnier, J.-V. (2009). PAK signalling in neuronal physiology. Cell. Signal. 21, 384–393. doi: 10.1016/j.cellsig.2008.11.001
Krenzlin, H., Lorenz, V., Danckwardt, S., Kempski, O., and Alessandri, B. (2016). The importance of thrombin in cerebral injury and disease. Int. J. Mol. Sci. 17:84. doi: 10.3390/ijms17010084
Lai, K.-P., Li, J.-W., Tse, A. C.-K., Wang, S. Y., Chan, T.-F., and Wu, R. S.-S. (2016). Differential responses of female and male brains to hypoxia in the marine medaka Oryzias melastigma. Aquat. Toxicol. 172, 36–43. doi: 10.1016/j.aquatox.2015.12.016
Lee, D. Y. (2015). Roles of mTOR signaling in brain development. Exp. Neurobiol. 24, 177–185. doi: 10.5607/en.2015.24.3.177
Leszczynska, K. B., Foskolou, I. P., Abraham, A. G., Anbalagan, S., Tellier, C., Haider, S., et al. (2015). Hypoxia-induced p53 modulates both apoptosis and radiosensitivity via AKT. J. Clin. Invest. 125, 2385–2398. doi: 10.1172/JCI80402
Lin, S.-C. C., and Way, E. L. (1984). Calcium transport in and out of brain nerve endings in vitro—the role of synaptosomal plasma membrane Ca2+-ATPase in Ca2+-extrusion. Brain Res. 298, 225–234. doi: 10.1016/0006-8993(84)91422-7
Luissint, A. C., Artus, C., Glacial, F., Ganeshamoorthy, K., and Couraud, P. O. (2012). Tight junctions at the blood brain barrier: physiological architecture and disease-associated dysregulation. Fluids Barr. CNS 9:23. doi: 10.1186/2045-8118-9-23
Luján, R., Shigemoto, R., and López-Bendito, G. (2005). Glutamate and GABA receptor signalling in the developing brain. Neuroscience 130, 567–580. doi: 10.1016/j.neuroscience.2004.09.042
McCombe, P. A., Lee, J. D., Woodruff, T. M., and Henderson, R. D. (2020). The peripheral immune system and amyotrophic lateral sclerosis. Front. Neurol. 11:279. doi: 10.3389/fneur.2020.00279
Metcalfe, J. D., and Butler, P. J. (1984). Changes in activity and ventilation in response to hypoxia in unrestrained, unoperated dogfish (Scyliorhinus canicula L.). J. Exp. Biol. 180, 153–162.
Müller, N., Riedel, M., Scheppach, C., Brandstätter, B., Sokullu, S., Krampe, K., et al. (2002). Beneficial antipsychotic effects of celecoxib add-on therapy compared to risperidone alone in schizophrenia. Am. J. Psychiatry 159, 1029–1034. doi: 10.1176/appi.ajp.159.6.1029
Ohinata, Y., Sutou, S., Kondo, M., Takahashi, T., and Mitsui, Y. (2002). Male-enhanced antigen-1 gene flanked by two overlapping genes is expressed in late spermatogenesis1. Biol. Reprod. 67, 1824–1831. doi: 10.1095/biolreprod.101.002550
Ojeda, S. R., Urbanski, H. F., Junier, M.-P., and Capdevila, J. (1989). The role of arachidonic acid and its metabolites in the release of neuropeptides. Ann. N. Y. Acad. Sci. 559, 192–207. doi: 10.1111/j.1749-6632.1989.tb22609.x
Oosthuyse, B., Moons, L., Storkebaum, E., Beck, H., Nuyens, D., Brusselmans, K., et al. (2001). Deletion of the hypoxia-response element in the vascular endothelial growth factor promoter causes motor neuron degeneration. Nat. Genet. 28, 131–138. doi: 10.1038/88842
Owens, D. F., and Kriegstein, A. R. (2002). Is there more to gaba than synaptic inhibition? Nat. Rev. Neurosci. 3, 715–727. doi: 10.1038/nrn919
Pan, L., North, H. A., Sahni, V., Jeong, S. J., Mcguire, T. L., Berns, E. J., et al. (2014). β1-Integrin and integrin linked kinase regulate astrocytic differentiation of neural stem cells. PLoS One 9:e104335. doi: 10.1371/journal.pone.0104335
Peers, C., Dallas, M. L., Boycott, H. E., Scragg, J. L., Pearson, H. A., and Boyle, J. P. (2009). Hypoxia and neurodegeneration. Ann. N. Y. Acad. Sci. 1177, 169–177. doi: 10.1111/j.1749-6632.2009.05026.x
Peters, M., Wielsch, B., and Boltze, J. (2017). The role of SUMOylation in cerebral hypoxia and ischemia. Neurochem. Int. 107, 66–77. doi: 10.1016/j.neuint.2017.03.011
Pinkstaff, J. K., Detterich, J., Lynch, G., and Gall, C. (1999). Integrin subunit gene expression is regionally differentiated in adult brain. J. Neurosci. 19, 1541–1556. doi: 10.1523/jneurosci.19-05-01541.1999
Pollock, M. S., Clarke, L. M. J., and Dubé, M. G. (2007). The effects of hypoxia on fishes: from ecological relevance to physiological effects. Environ. Rev. 15, 1–14. doi: 10.1139/a06-006
Prager, B., Spampinato, S. F., and Ransohoff, R. M. (2015). Sphingosine 1-phosphate signaling at the blood–brain barrier. Trends Mol. Med. 21, 354–363. doi: 10.1016/j.molmed.2015.03.006
Qiu, W., Song, S., Chen, W., Zhang, J., Yang, H., and Chen, Y. (2019). Hypoxia-induced EPHB2 promotes invasive potential of glioblastoma. Int. J. Clin. Exp. Pathol. 12, 539–548.
Raghubir, R., Nakka, V. P., and Mehta, S. L. (2011). Endoplasmic reticulum stress in brain damage. Unfolded Protein Response Cell. Stress Part A 489, 259–275. doi: 10.1016/b978-0-12-385116-1.00015-7
Rashid, M., Belmont, J., Carpenter, D., Turner, C. E., and Olson, E. C. (2017). Neural-specific deletion of the focal adhesion adaptor protein paxillin slows migration speed and delays cortical layer formation. Development 144, 4002–4014. doi: 10.1242/dev.147934
Ryskalin, L., Lazzeri, G., Flaibani, M., Biagioni, F., Gambardella, S., Frati, A., et al. (2017). mTOR-dependent cell proliferation in the brain. BioMed Res. Int. 2017:7082696. doi: 10.1155/2017/7082696
Sato-Carlton, A., Nakamura-Tabuchi, C., Chartrand, S. K., Uchino, T., and Carlton, P. M. (2017). Phosphorylation of the synaptonemal complex protein SYP-1 promotes meiotic chromosome segregation. J. Cell Biol. 217, 555–570. doi: 10.1083/jcb.201707161
Savitski, M. M., Wilhelm, M., Hahne, H., Kuster, B., and Bantscheff, M. (2015). A scalable approach for protein false discovery rate estimation in large proteomic data sets. Mol. Cell. Proteom. 14, 2394–2404. doi: 10.1074/mcp.m114.046995
Scherma, M., Masia, P., Satta, V., Fratta, W., Fadda, P., and Tanda, G. (2019). Brain activity of anandamide: a rewarding bliss? Acta Pharmacol. Sin. 40, 309–323. doi: 10.1038/s41401-018-0075-x
Schorova, L., and Martin, S. (2016). Sumoylation in synaptic function and dysfunction. Front. Syn. Neurosci. 8:9. doi: 10.3389/fnsyn.2016.00009
Schurmann, H., and Steffensen, J. F. (1994). Spontaneous swimming activity of Atlantic cod Gadus morhua exposed to graded hypoxia at three temperatures. J. Exp. Biol. 197, 129–142. doi: 10.1242/jeb.197.1.129
Skene, J. P., and Cleveland, D. W. (2001). Hypoxia and lou gehrig. Nat. Genet. 28, 107–108. doi: 10.1038/88805
Stankiewicz, T. R., and Linseman, D. A. (2014). Rho family GTPases: key players in neuronal development, neuronal survival, and neurodegeneration. Front. Cell. Neurosci. 8:314. doi: 10.3389/fncel.2014.00314
Subudhi, B. B., Sahu, P. K., Singh, V. K., and Prusty, S. (2018). Conjugation to ascorbic acid enhances brain availability of losartan carboxylic acid and protects against parkinsonism in rats. AAPS J. 20:110. doi: 10.1208/s12248-018-0270-1
Sumien, N., Huang, R., Chen, Z., Vann, P. H., Wong, J. M., Li, W., et al. (2019). Effects of dietary 5-methoxyindole-2-carboxylic acid on brain functional recovery after ischemic stroke. Behav. Brain Res. 378:112278. doi: 10.1016/j.bbr.2019.112278
Sun, M., Li, Z., and Gui, J.-F. (2010). Dynamic distribution of spindlin in nucleoli, nucleoplasm and spindle from primary oocytes to mature eggs and its critical function for oocyte-to-embryo transition in gibel carp. J. Exp. Zool. Part A Ecol. Genet. Physiol. 313A, 461–473. doi: 10.1002/jez.618
Tan, H. P., Guo, Q., Hua, G., Chen, J. X., and Liang, J. C. (2018). Inhibition of endoplasmic reticulum stress alleviates secondary injury after traumatic brain injury. Neural Regenerat. Res. 13, 827–836. doi: 10.4103/1673-5374.232477
Teismann, P., Tieu, K., Choi, D.-K., Wu, D.-C., Naini, A., Hunot, S., et al. (2003). Cyclooxygenase-2 is instrumental in Parkinson’s disease neurodegeneration. Proc. Natl. Acad. Sci. U.S.A. 100, 5473–5478. doi: 10.1073/pnas.0837397100
Terraneo, L., Paroni, R., Bianciardi, P., Giallongo, T., Carelli, S., Gorio, A., et al. (2017). Brain adaptation to hypoxia and hyperoxia in mice. Redox Biol. 11, 12–20. doi: 10.1016/j.redox.2016.10.018
Terraneo, L., and Samaja, M. (2017). Comparative response of brain to chronic hypoxia and hyperoxia. Int. J. Mol. Sci. 18:1914. doi: 10.3390/ijms18091914
Thomas, P., and Rahman, M. S. (2009). Biomarkers of hypoxia exposure and reproductive function in Atlantic croaker: a review with some preliminary findings from the northern Gulf of Mexico hypoxic zone. J. Exp. Mar. Biol. Ecol. 381, S38–S50.
Tietz, S., and Engelhardt, B. (2015). Brain barriers: crosstalk between complex tight junctions and adherens junctions. J. Cell Biol. 209, 493–506. doi: 10.1083/jcb.201412147
Turrens, J. F. (2003). Mitochondrial formation of reactive oxygen species. J. Physiol. 552, 335–344. doi: 10.1113/jphysiol.2003.049478
Vanacore, N., Cocco, P., Fadda, D., and Dosemeci, M. (2010). Job strain, hypoxia and risk of amyotrophic lateral sclerosis: results from a death certificate study. Amyotrophic Lateral Sclerosis 11, 430–434. doi: 10.3109/17482961003605796
Vogel, Z., Barg, J., Levy, R., Saya, D., Heldman, E., and Mechoulam, R. (1993). Anandamide, a brain endogenous compound, interacts specifically with cannabinoid receptors and inhibits adenylate cyclase. J. Neurochem. 61, 352–355. doi: 10.1111/j.1471-4159.1993.tb03576.x
Wang, H., and Reiser, G. (2003). Thrombin signaling in the brain: the role of protease-activated receptors. Biol. Chem. 384, 193–202. doi: 10.1515/bc.2003.021
Wang, S. Y., Lau, K., Lai, K.-P., Zhang, J.-W., Tse, A. C.-K., Li, J.-W., et al. (2016). Hypoxia causes transgenerational impairments in reproduction of fish. Nat. Commun. 7:12114. doi: 10.1038/ncomms12114
Weidenfeld, J., Feldman, S., and Mechoulam, R. (1994). Effect of the brain constituent anandamide, a cannabinoid receptor agonist, on the hypothalamo-pituitary-adrenal axis in the rat. Neuroendocrinology 59, 110–112. doi: 10.1159/000126646
Wen, B., Zhou, R., Feng, Q., Wang, Q., Wang, J., and Liu, S. (2014). IQuant: an automated pipeline for quantitative proteomics based upon isobaric tags. Proteomics 14, 2280–2285. doi: 10.1002/pmic.201300361
Wu, C., and Sun, D. (2015). GABA receptors in brain development, function, and injury. Metab. Brain Dis. 30, 367–379. doi: 10.1007/s11011-014-9560-1
Wu, R. S. S., Zhou, B. S., Randall, D. J., Woo, N. Y. S., and Lam, P. K. S. (2003). Aquatic hypoxia is an endocrine disruptor and impairs fish reproduction. Environ. Sci. Technol. 37, 1137–1141. doi: 10.1021/es0258327
Wu, X., and Reddy, D. S. (2012). Integrins as receptor targets for neurological disorders. Pharmacol. Ther. 134, 68–81. doi: 10.1016/j.pharmthera.2011.12.008
Xu, K., and LaManna, J. C. (2006). Chronic hypoxia and the cerebral circulation. J. Appl. Physiol. 100, 725–730. doi: 10.1152/japplphysiol.00940.2005
Yu, J. S. L., and Cui, W. (2016). Proliferation, survival and metabolism: the role of PI3K/AKT/mTOR signalling in pluripotency and cell fate determination. Development 143, 3050–3060. doi: 10.1242/dev.137075
Yu, R. M. K., and Wu, R. S. S. (2006). Hypoxia affects sex differentiation and development, leading to a male-dominated population in zebrafish (Danio rerio). Environ. Sci. Technol. 40, 3118–3122. doi: 10.1021/es0522579
Yun, Z., and Glazer, P. M. (2015). Tumor suppressor p53 stole the AKT in hypoxia. J. Clin. Investig. 125, 2264–2266. doi: 10.1172/jci82058
Zhang, G., Chen, L., Liu, J., Jin, Y., Lin, Z., Du, S., et al. (2020). HIF-1α/microRNA-128-3p axis protects hippocampal neurons from apoptosis via the Axin1-mediated Wnt/β-catenin signaling pathway in Parkinson’s disease models. Aging 12, 4067–4081. doi: 10.18632/aging.102636
Zhang, J., Sun, M., Zhou, L., Li, Z., Liu, Z., Li, X.-Y., et al. (2015). Meiosis completion and various sperm responses lead to unisexual and sexual reproduction modes in one clone of polyploid Carassius gibelio. Sci. Rep. 5:10898. doi: 10.1038/srep10898
Keywords: hypoxia, brain, proteomic, fish, stress
Citation: Lai KP, Tam N, Wang SY, Tse WKF, Lin X, Chan TF, Tong Y, Zhang JW, Au DWT, Wu RSS and Kong RYC (2021) Proteomic Response of the Brain to Hypoxic Stress in Marine Medaka Fish (Oryzias melastigma). Front. Mar. Sci. 8:618489. doi: 10.3389/fmars.2021.618489
Received: 17 October 2020; Accepted: 19 April 2021;
Published: 28 May 2021.
Edited by:
Andrew Stanley Mount, Clemson University, United StatesReviewed by:
William Jarrett Biggers, Wilkes University, United StatesZhenghong Zuo, Xiamen University, China
Copyright © 2021 Lai, Tam, Wang, Tse, Lin, Chan, Tong, Zhang, Au, Wu and Kong. This is an open-access article distributed under the terms of the Creative Commons Attribution License (CC BY). The use, distribution or reproduction in other forums is permitted, provided the original author(s) and the copyright owner(s) are credited and that the original publication in this journal is cited, in accordance with accepted academic practice. No use, distribution or reproduction is permitted which does not comply with these terms.
*Correspondence: Keng Po Lai, a2VuZ3BsYWlAY2l0eXUuZWR1Lmhr; Richard Yuen Chong Kong, Ymhya29uZ0BjaXR5dS5lZHUuaGs=