- 1Molecular Ecology Laboratory, College of Science and Engineering, Flinders University, Bedford Park, SA, Australia
- 2Cetacean Ecology, Behaviour, and Evolution Laboratory, College of Science and Engineering, Flinders University, Bedford Park, SA, Australia
- 3Cetacean Ecology Research Group, School of Natural and Computational Sciences, College of Science, Massey University, Auckland, New Zealand
- 4Department of Biological Sciences, Macquarie University, Sydney, NSW, Australia
- 5National Institute of Water & Atmospheric Research Ltd (NIWA), Wellington, New Zealand
- 6Laboratorio de Ecología Molecular de Vertebrados Acuáticos, Departamento de Ciencias Biológicas, Universidad de los Andes, Bogotá, Colombia
An understanding of population structure and connectivity at multiple spatial scales is required to assist wildlife conservation and management. This is particularly critical for widely distributed and highly mobile marine mammals subject to fisheries by-catch. Here, we present a population genomic assessment of a near-top predator, the common dolphin (Delphinus delphis), which is incidentally caught in multiple fisheries across the Australasian region. The study was carried out using 14,799 ddRAD sequenced genome-wide markers genotyped for 478 individuals sampled at multiple spatial scales across Australasia. A complex hierarchical metapopulation structure was identified, with three highly distinct and genetically diverse regional populations at large spatial scales (>1,500 km). The populations inhabit the southern coast of Australia, the eastern coast of Australia, New Zealand, and Tasmania, with the latter also showing a considerable level of admixture to Australia's east coast. Each of these regional populations contained two to four nested local populations (i.e., subpopulations) at finer spatial scales, with most of the gene flow occurring within distances of 50 to 400 km. Estimates of contemporary migration rates between adjacent subpopulations ranged from 6 to 25%. Overall, our findings identified complex common dolphin population structure and connectivity across state and international jurisdictions, including migration and gene flow across the Tasman Sea. The results indicate that inter-jurisdictional collaboration is required to implement conservation management strategies and mitigate fisheries interactions of common dolphins across multiple spatial scales in the Australasian region.
Introduction
Genetic connectivity and the delineation of populations, including their boundaries, are fundamental issues in conservation biology, because such information can advise on the scale of which to conserve and manage wildlife species (Leslie and Morin, 2016; Taylor et al., 2017; Dunn et al., 2019; Pierre, 2019; Sousa et al., 2019; Taft et al., 2020; Tulloch et al., 2020). Studies using molecular markers can inform on the number and distribution of populations, their genetic diversity, their resilience to environmental change, as well as their vulnerability to anthropogenic impacts and disease outbreaks (DiBattista et al., 2017; Holland et al., 2017; Bradburd et al., 2018; Batley et al., 2019; Breed et al., 2019; Grummer et al., 2019; Jasper et al., 2019; Perry and Lee, 2019; Leitwein et al., 2020). However, incorporating genetic data into conservation policy and management remains a challenge, and enhanced collaboration between conservation geneticists and wildlife managers is needed (Funk et al., 2012; Hendricks et al., 2018; Gardner et al., 2020; Holderegger et al., 2020; Taft et al., 2020).
Studies of population structure and dynamics emerged with the theories of island biogeography and metapopulation dynamics (MacArthur and Wilson, 1967; Levins, 1969; Hanski, 1998), and have evolved since then into characterizing connectivity of species among habitat patches in heterogeneous environments under different spatial and temporal scales (Waples and Gaggiotti, 2006; Compton et al., 2007; Manel et al., 2019). In marine environments, there is still limited information about how geographic barriers and spatial scales impact on population genetic structure (Riginos et al., 2016). Population structure and the dispersal of marine species may be associated with a range of factors such as spatial distance, oceanographic features (e.g., currents, upwellings, environmental gradients) and ecological traits (e.g., feeding ecology and life history), making it difficult to disentangle these factors (Selkoe et al., 2016; Bernatchez et al., 2018), and establishing policies for conservation and management.
The movement of marine species with active dispersal, such as delphinids, can occur at any life stage. Despite this, dolphins can exhibit population genetic structure at relatively small spatial scales (e.g., Hoelzel, 1998; Natoli et al., 2006; Möller et al., 2007; Quérouil et al., 2007) and are often subdivided into local populations (e.g., Natoli et al., 2005; Hoelzel et al., 2007; Mendez et al., 2008; Möller, 2011; Caballero et al., 2012; Nykanen et al., 2018; Parra et al., 2018; Pratt et al., 2018). When these populations are interconnected, but exhibit specific ecological and/or behavioral traits in a geographic area, a complex metapopulation system may arise (Riginos et al., 2016; Selkoe et al., 2016; Perry and Lee, 2019).
At large scales, the dispersal and population structure of dolphins is influenced by oceanographic or environmental variables such as depth, currents, upwellings, salinity gradients, sea surface temperatures, and primary productivity (Fullard et al., 2000; Natoli et al., 2005; Quérouil et al., 2007; Mirimin et al., 2009; Möller et al., 2011; Amaral et al., 2012a; Bilgmann et al., 2014; Fruet et al., 2014; Gaspari et al., 2015a; Pratt et al., 2018). At smaller scales, localized site fidelity, complex social behavior, and feeding specializations may result in adaptations to local environments, which leads to further population subdivision (Hoelzel et al., 2007; Möller et al., 2007; Ansmann et al., 2012; Fruet et al., 2014; Cammen et al., 2016; Foote et al., 2016; Zanardo et al., 2017; Pratt et al., 2018).
Common dolphins (Delphinus delphis) have a high dispersal potential and inhabit coastal and pelagic environments in temperate and subtropical waters of both southern and northern hemispheres (Natoli et al., 2008; Whitehead et al., 2008; Möller, 2011). In Australasia, common dolphin distribution ranges from embayment and gulf waters, to coastal and shelf waters of Australia and New Zealand (Möller et al., 2011; Bilgmann et al., 2014; Stockin et al., 2014; Mason et al., 2016; Zanardo et al., 2016; Dwyer et al., 2020; Peters and Stockin, 2021). At its most extreme, common dolphins in some semi-enclosed, relatively shallow embayments, show moderate to high site fidelity, such as in Port Phillip Bay (Victoria, Australia), Gulf St Vincent (South Australia) (Filby et al., 2010; Mason et al., 2016), and in the Hauraki Gulf (New Zealand) (Stockin et al., 2008; Hupman, 2016; Hupman et al., 2018; Pawley et al., 2018).
Differences in prey abundance, distribution and diversity can lead to feeding specializations in common dolphins (Neumann and Orams, 2003), which may shape their population structure at fine and medium spatial scales (Möller et al., 2007, 2011; Tezanos-Pinto et al., 2009). Movements of common dolphins are known to generally associated with the movement of their prey, which includes schooling fish such as jack mackerel (Trachurus declivis, T. symmetricus. Murphyi, and T. novaezelandiae), blue mackerel (Scomber australasicus), sardines (Sardinops sagax), southern calamari (Sepioteuthis australis), and anchovies (Engraulis australis) (e.g., Meynier et al., 2008; Goldsworthy et al., 2019a). In turn, most of these prey species are heavily targeted by fisheries, both for human consumption and to feed fish held in aquaculture farms, making common dolphins particularly susceptible to interactions with fisheries and to incidental mortalities (Kemper et al., 2003; Bilgmann et al., 2008; Stockin et al., 2009b).
Indeed, common dolphins in Australasia suffer mortalities as by-catch in multiple fisheries (Hamer et al., 2008; Thompson et al., 2013; Abraham et al., 2017; Tulloch et al., 2020). In Australia, common dolphins are incidentally by-caught in purse-seine, trawl, and gillnet fisheries (e.g., Hamer et al., 2008; AFMA, 2019b), with ~380 mortalities recorded in purse-seine nets in 2004-2005 (Hamer et al., 2008), and more than 100 mortalities during 2011-2019 in gillnets (AFMA, 2019a, 2020). In New Zealand, common dolphins are mainly threatened by trawl and surface long-line fisheries (Abraham et al., 2017; Pierre, 2019), with at least 200 captures occurring from 2002 to 2017 in the trawl fishery (MPI, 2019). While mitigation of common dolphin by-catch in these countries has led to a general reduction in mortalities over time (Rowe, 2007; Ward and Grammer, 2018; Goldsworthy et al., 2019b), by-catch incidents have continued and occasionally spike in numbers (Abraham et al., 2017; Goldsworthy et al., 2019b). Notably, the cumulative impacts of dolphin-fishery interactions are currently unknown (Mackay et al., 2016), and by-catch is still managed separately by each fishery and based on fishing management zones, not based on dolphins' stock structure. These issues are exacerbated by limited information about dolphin abundance in Australasia, and how many dolphins can be caught without compromising the long-term viability of the populations. These estimates of potential biological removal (PBR), have been estimated based on aerial surveys and fisheries surveys. For South Australia, an aerial assessment done over 40,000 km2 led to an estimation of 21,733 common dolphins (CV = 0.25; 95% CI = 13,809–34,203) (Parra et al., in review), while in New Zealand an estimation for the Northern Island was of 18,145 common dolphins (CV = 0.33, 95% CI = 9,669–33,726) (Abraham et al., 2017).
In Australasia, common dolphins are known from previous studies to exhibit a degree of population genetic structure (Bilgmann et al., 2007b, 2014; Möller et al., 2011; Amaral et al., 2012a; Zanardo et al., 2016). These studies utilized traditional genetic markers such as mitochondrial DNA (mtDNA) and microsatellites and have identified population genetic differentiation at broad spatial scales (>1,500 km) between common dolphins of the Pacific and Indian Oceans (Amaral et al., 2012a; Bilgmann et al., 2014), as well as over finer spatial scales (<1,000 km; in southern (Bilgmann et al., 2014) and eastern Australia (Möller et al., 2011), and New Zealand (Stockin et al., 2014). However, studies based on a few molecular markers may not be accurate for determining spatial population structure (e.g., Teske et al., 2018; Rajora, 2019). The use of thousands of genome-wide markers circumvents this issue by providing powerful data to clarify spatially complex population structure (Frankham et al., 2010; Funk et al., 2012; Cammen et al., 2016; Teske et al., 2018; Manel et al., 2019).
Here, we assess the population genomic structure of common dolphins using a multi-scale approach across its distribution in Australasia. Our primary aims are to elucidate patterns of genomic diversity, population structure, and connectivity using a novel and powerful genome-wide dataset for common dolphins based on single nucleotide polymorphisms (SNPs). We complement this population genomic assessment with analyses of novel and previously published mtDNA sequences. Our study combines broad and fine-scale approaches to resolve structure and connectivity and provides detailed information to enhance the conservation management of common dolphins in Australasia.
Methods
Study Area and Sampling
The study area encompasses two major oceanic regions, the southern Indian Ocean (Australia's southern coast) and the south-western Pacific Ocean (Australia's eastern coast, Tasmania and New Zealand). Skin samples were collected from live animals (i.e., biopsied) and carcasses (i.e., stranded and by-caught animals) over 17 years (2000-2017) at 16 localities across the species range in Australia and New Zealand (Figure 1). Samples from live individuals were obtained using a hand held biopsy pole (Bilgmann et al., 2007a) or a remote biopsy system (PAXARMS) (Krutzen et al., 2002). A total of 510 samples were analyzed for population genomics, including 310 biopsy samples and 200 stranding and by-catch samples, with the GPS location allocated to where an individual was found/caught (Figure 1).
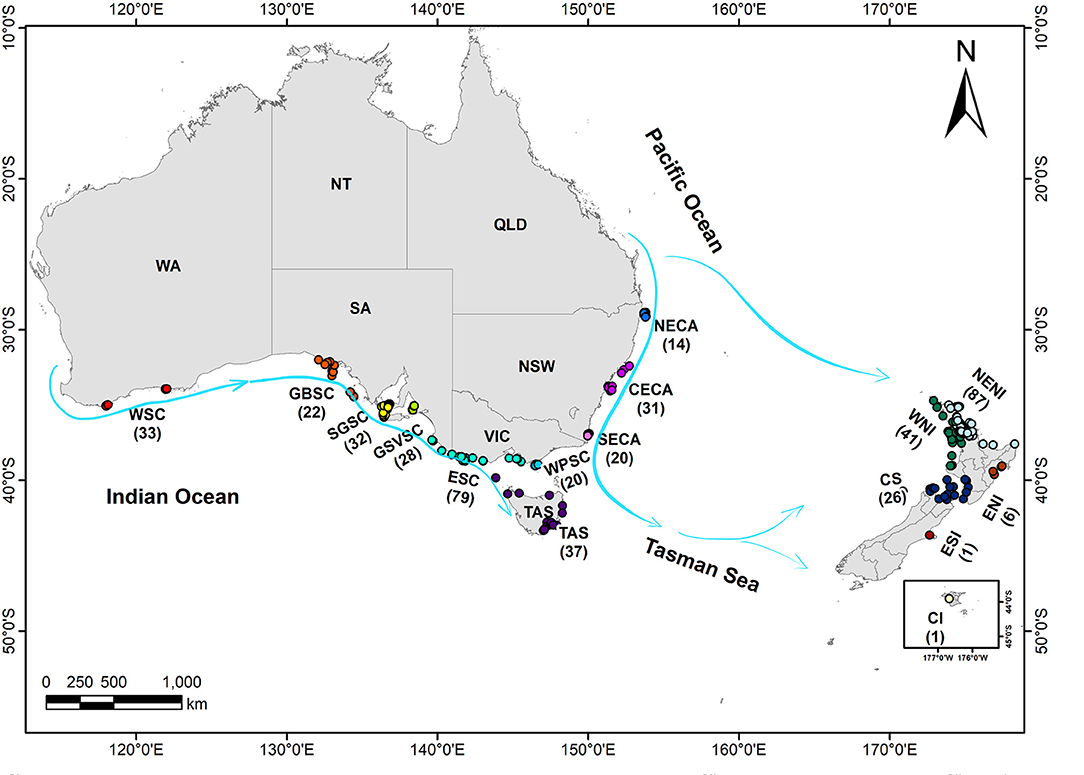
Figure 1. Study area in the Australasian region showing the geolocations for 478 common dolphin (Delphinus delphis) samples used for the genome-wide analyses. Blue lines correspond to main current systems in Australasia. WSC, *West, Southern coast of Australia; GBSC, Great Australian Bight, Southern coast of Australia; SGSC, shelf waters in Spencer Gulf, Southern coast of Australia; GSVSC, Gulf St Vincent, Southern coast of Australia; ESC, East, Southern coast of Australia; WPSC, Wilson Promontory, Southern coast of Australia; NECA, North, Eastern coast of Australia; CECA, Central, Eastern coast of Australia; SECA, South, Eastern coast of Australia; CS, Cook Strait, New Zealand; WNI, West North Island, New Zealand; NENI, North East Island, New Zealand; ENI, East, North Island, New Zealand; CI, Chatman Island, New Zealand; ESI, East, South Island, New Zealand.
DNA Extraction and Sex Determination
Genomic DNA was extracted from samples using a modified salting out protocol (Sunnucks and Hales, 1996). Extraction quality was then assessed using a NanoDrop-2000 (Thermo scientific) spectrophotometer, quantity estimated by a Qubit 2.0 fluorometer (Life Technologies), and integrity evaluated with agarose gels. If degradation was observed, DNA fragments >5 Kbp were selected using AMPure XP magnetic beads (Beckman Coulter Genomics), using a ratio of 0.8:1 (beads: DNA). The sex was genetically determined by the amplification of fragments of the ZFX and ZFY genes for all DNA samples, following Banks et al. (1995).
Mitochondrial DNA Control Region (mtDNA CR) Sequencing and Data Analysis
A fragment of the mtDNA CR of ~450 base pairs was amplified by the polymerase chain reaction (PCR) with primers DLP1.5 and DLP5, as modified by Möller and Beheregaray (2001), and sequenced in an Applied Biosystems 3730XL Sequencer. MtDNA CR sequences were then trimmed and aligned using Geneious v.6.0.4 (Kearse et al., 2012). ARLEQUIN v3.5.2.2 (Excoffier and Lischer, 2010) was used to estimate nucleotide and haplotype diversities overall and for each locality. To assess genetic differentiation between localities, pairwise ΦST (Weir and Cockerham, 1984) was estimated with significance assessed using 10,000 permutations False Discovery Rate (FDR = 10%) and corrected for multiple tests by the B-Y method (Benjamini and Yekutieli, 2001). Heatmap plots of pairwise ΦST values were then constructed in the language R (R Development Core Team, 2018), with the package ggplot2 (Wickham, 2016). A haplotype network was built in PopART 1.7, using the ancestral parsimony and 95% cut-off (Clement et al., 2002). The latter was carried out to assess evolutionary relationships of the inferred maternal lineages (i.e., mtDNA CR haplotypes). Together with estimates of nucleotide diversity, this provides an indication of long-term evolutionary divergence (or similarity) of common dolphin lineages. A total of 197 samples were sequenced and retained for analysis after filtering out poor quality peaks, and trimming to 440 base pairs to match the sequence fragments of Möller et al. (2011) (N = 63), Stockin et al. (2014) (N = 24), and Bilgmann et al. (2014) (N = 110). Altogether a total of 394 individual sequences were available for analyses based on mtDNA CR.
Genomic Library Preparation and ddRAD Sequencing
Double digest restriction-site associated DNA (ddRAD) libraries were prepared following Peterson et al. (2012), with modifications. Each sample was digested with two restriction enzymes SbfI and MseI, and then ligated with one of 96 individual barcodes designed in-house. Samples were then pooled into a multiplex of 12 individuals. Libraries were size selected for 250–800 bp fragments with a Pippin prep electrophoresis gel (Sage Science). The samples were amplified by PCR, and after this removal of PCR byproducts was done using AMPure XP magnetic beads (ratio of 0.8:1). Real-time PCR was used to determine the DNA concentration to accurately pool eight libraries of 12 samples together in equal concentrations, creating one multiplex library of 96 uniquely barcoded samples sent for sequencing. The multiplex libraries were then single-end, 100 bp sequenced using multiple lanes in an Illumina HiSeq 2500 at the South Australian Health & Medical Research Institute (SAHMRI).
Sequences and SNP Filtering
Sequence quality checks were performed on the raw reads, followed by demultiplexing, trimming of barcodes and RAD tags (only one error allowed) and sorting into individual samples using process_radtags with STACKS v1.48 (Catchen et al., 2013). Next, filtered sequences were processed to generate a final SNP dataset using the dDocent2.2.19 pipeline (Puritz et al., 2014). The resulted variant calling file (VCF), with sequence variation across all samples (raw SNP catalog), was then filtered using VCFtools (Danecek et al., 2011) (for details see Supplementary Material). To further assess the quality of the SNP dataset and to exclude exogenous sequences, the quality-filtered reads were mapped against two genomes from closely related dolphin species: the Tursiops truncatus genome (Tur_tru_Illumina_phased_v1, GenBank Assembly ID: GCA_003435595.3) and the southern Australian bottlenose dolphin (SABD), Tursiops aduncus genome (Batley et al., unpublished). This was done using Bowtie2, following suggested standard procedures from Langmead and Salzberg (2012), allowing no mismatches in seed alignment and up to 20 consecutive seed fails. A linkage disequilibrium (LD) filter was implemented to obtain a dataset with the most likely number of independent markers (for details see Supplementary Material).
Detecting Neutral SNPs
SNPs putatively under selection were identified and removed from the dataset so that population structure analyses were based on markers conforming to neutral expectations (Luikart et al., 2003). This was done using an outlier test in BayeScan v2.1 (Foll and Gaggiotti, 2008) run with 100,000 iterations and prior odds of 10,000. Loci with a false discovery rate <10% were considered as not behaving as neutral and removed from the population analyses.
Relatedness Estimates for Excluding Potential Duplicate Samples
Relatedness between pairs of individuals was estimated using the triadic likelihood estimator (TrioML) in Coancestry v1.0.1.9 (Wang, 2011) to exclude potential re-sampled individuals (set at r > 0.7) from the population analyses. This method estimates pairwise relatedness (r) by using a third individual as a control, thus decreasing the chance of genes identical in state being mistakenly inferred as identical by descent (Wang, 2007).
Genomic Data Analyses
Genomic Diversity, Population Structure, and Genomic Differentiation
Genomic diversity was estimated for each locality sample as expected heterozygosity (HE), observed heterozygosity (HO), the inbreeding coefficient (FIS), and percentage of polymorphic loci (P) using Genodive 2.0b27 (Meirmans and Van Tienderen, 2004). Population genetic structure was assessed using a Principal Component Analysis (PCA) and Discriminant Analysis of Principal Components (DAPC) with the R package Adegenet (Jombart and Ahmed, 2011) using an annealing simulation of 50,000 steps, and an optimal number of PCs to be retained, as suggested in Adegenet (Jombart and Collins, 2015). Both PCA and DAPC are model-free approaches for investigating population structure. The Bayesian information criterion (BIC) and Akaike information criterion (AIC) were then used to determine the best-supported number of clusters in the dataset, using the snapclust.chooseK function in the R package Adegenet (Beugin et al., 2018). Bayesian clustering was used to infer population stratification based on estimated individual ancestries using Admixture v1.3.0 (Alexander et al., 2009). This was done by performing a maximum likelihood estimates, using the ancestry portion and the population allele frequency to assign the most likely number of K (e.g., populations) in the dataset, testing for K1-16, and to model the probability of observed genotypes. The maximum likelihood of K and the fast-sequential quadratic algorithm were subsequently used as a cross validation with 10 replicates for each K value, using K1-8 (Alexander et al., 2009; Alexander and Lange, 2011). Genetic differentiation among localities was estimated as pairwise FST (Weir and Cockerham, 1984) using Genodive 2.0b27. Significance levels were assessed using 10,000 permutations (FDR = 10%), and then corrected by the B-Y method. Heatmap plots of FST were constructed with the R package ggplot2. A Mantel test (Mantel, 1967) was used to test for isolation by distance (IBD) using the shortest waterway distance matrix calculated in ArcMap v10.4 (Esri Inc., Redlands, CA) and a linearized pairwise FST matrix (FST /1- FST) as genetic distance. Scatterplots were then generated with the R package Adegenet.
Contemporary Migration Rates and First-Generation Migrants
Contemporary migration rates were estimated with BayesAss v3.0.4 (Wilson and Rannala, 2003) using the putatively neutral, unlinked SNPs. The method applies a Bayesian Markov chain Monte Carlo approach to estimate asymmetrical rates of recent migration (m), which represents the proportion of each population having migrant ancestry over the last generations. Common dolphins exhibit a long generation time ~15 years, with interbirth intervals from 1 to 3 years (Taylor et al., 2007; Möller, 2011). The analysis was run with 10 million iterations and 1 million iterations as burn-in, and mixing parameters (allele frequencies, inbreeding coefficients, and migration rates) were adjusted to achieve recommended acceptance rates (Wilson and Rannala, 2003). Convergence was then inspected by plotting the cumulative log likelihoods of the iterations using TRACER 1.7 (Rambaut et al., 2018), with three runs used to verify consistency across runs.
First generation migrants were identified by performing a population assignment test in GeneClass2 (Piry et al., 2004), using the criteria of Rannala and Mountain (1997). This uses the multilocus genotypes and 1,000 simulations to provide a probability of an individual belonging to a population (Paetkau et al., 2004). An exclusion rate of 0.01 was applied. Only 800 SNPs were used due to the limitations of the software which only allowed successful runs to this maximum number of SNPs. The SNP subset was chosen using a random generator in R studio, and extracted from the full dataset of filtered putatively neutral SNPs.
Results
Diversity and Differentiation Based on mtDNA CR
In the 440 bp of the mtDNA CR sequences of 394 Australasian common dolphins, three indels and 94 substitutions were observed. This resulted in 173 unique mtDNA haplotypes, 66 of which were not previously described for common dolphins in this region (Möller et al., 2011; Bilgmann et al., 2014; Stockin et al., 2014). Most haplotypes were represented by only one individual in the dataset. The overall haplotype diversity was high (h = 0.860), while the nucleotide diversity was low (π = 0.0160) (Supplementary Table 4). The haplotype diversity observed was similar to that previously reported for the eastern and southern Australia and for New Zealand (e.g., Möller et al., 2011; Bilgmann et al., 2014; Stockin et al., 2014). Fixation indices based on mtDNA CR indicated low to moderate differentiation between samples from the Pacific and the Indian Ocean (0.029–0.620) (Supplementary Figure 2, Supplementary Table 7). The haplotype network indicated very shallow phylogeographic structure (Supplementary Figure 3).
Genome-Wide SNP Data and Filtering for Putatively Neutral Loci
A total of 1,601,109,786 raw sequence reads were obtained, and a raw SNP catalog of 339,932 SNPs (Supplementary Table 1). The alignment rates with the Tursiops genomes were very high, attesting to the high quality of the SNP dataset: 97% aligned with the T. truncatus genome and 99% with the SABD T. aduncus genome (retaining 26,199 SNPs, Supplementary Table 1). Filtering with stringent criteria resulted in a high-resolution dataset of 17,875 SNPs (Supplementary Table 1). The outlier test detected 3,076 SNPs likely not behaving as neutral, and these were excluded from the total dataset. This final dataset included 14,799 unlinked, putatively neutral SNPs (Supplementary Table 1, Supplementary Figure 1) that were used for the population structure analyses and to estimate migration rates.
Exclusion of Duplicate Samples
Thirty-two sample pairs were estimated as likely originating from duplicate individuals (r ≥ 0.7), including 24 pairs of biopsies and eight pairs of stranding or by-caught individuals. One sample from each of the pairs was excluded, resulting in a final dataset of 478 individuals (Figure 1).
Genomic Diversity, Population Structure, and Genomic Differentiation
Genome-wide diversity was relatively high for all localities (Table 1) and there was no indication of population-level inbreeding (Table 1). When analyzing all samples combined, PCA and DAPC analyses suggested three distinct regional populations: (1) southern coast of Australia; (2) eastern coast of Australia; and (3) New Zealand and Tasmania, although the latter shows a degree of admixture to Australia's eastern coast (Supplementary Figures 5, 6). Admixture analysis suggested a hierarchical metapopulation structure in Australasia, with moderate levels of admixture within the regional populations (Figure 2, Supplementary Figure 7). At a metapopulation level, three clusters (K = 3), corresponding to geographical regional populations, were considered most likely (Figure 2). The membership probability of an individual belonging to a population varied according to the geographic position of the locality, with individuals from localities close to the interface between the Indian and Pacific Oceans (i.e., Wilsons Promontory in Victoria and southern localities in New South Wales, Australia), and between Tasmania and Australia's eastern coast being more admixed (Supplementary Figure 7A).
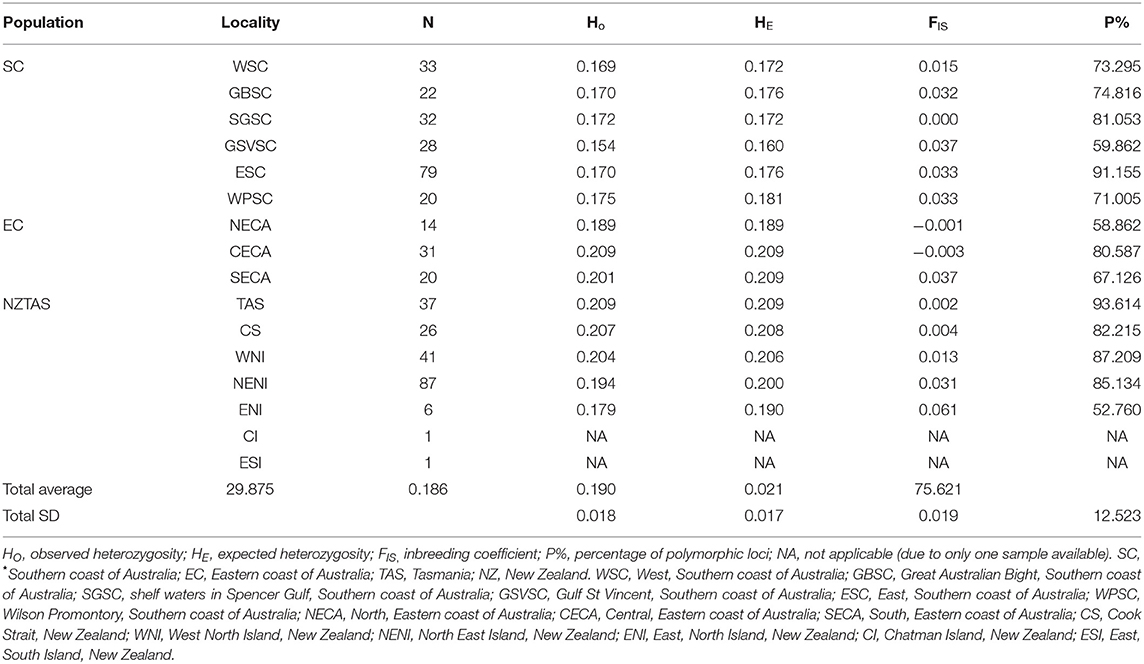
Table 1. Measures of genomic diversity based on 14,799 SNPs for Australasian common dolphins (Delphinus delphis) by locality*.
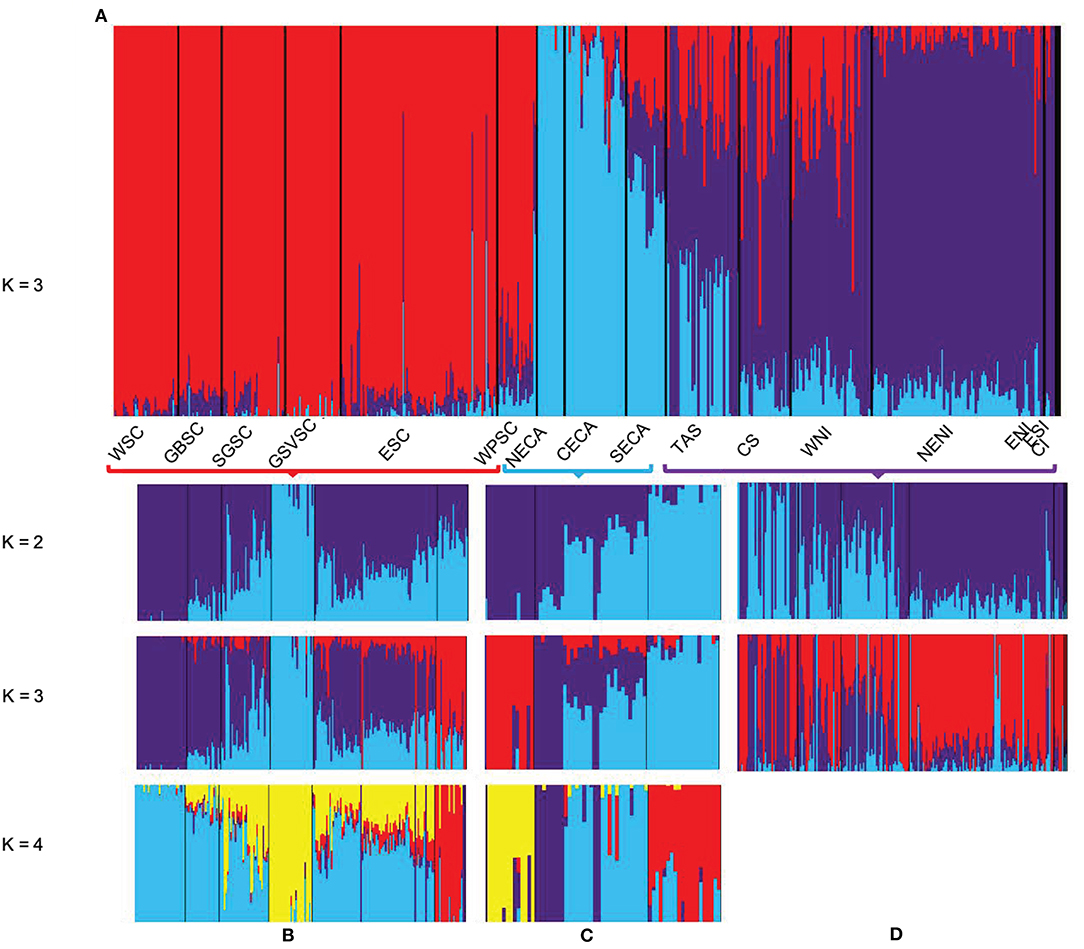
Figure 2. Population genomic structure analysis using Admixture based on 14,799 SNPs for Australasian common dolphins (Delphinus delphis) (labeled by locality*). The results depict levels of admixture for each individual sample and grouping into two and/or three genomic clusters. Each sample (labeled by geographic sample groups in the x axis) is represented by one vertical line and is color-coded by the membership probability to (A) one of the three regional populations in Australia and New Zealand or (B–D) one of the potential local populations of the: (B) Southern coast of Australia; (C) Eastern coast of Australia; or (D) New Zealand and Tasmania. *Acronyms used as in Figure 1.
Further subdivision was disclosed within each regional population, when analyzing the three datasets separately, with two additional clusters (K = 2) within each region (Figure 2, Supplementary Figures 7B–D) best supported, but three and four clusters also highly supported for eastern and southern Australia (Figure 2, Supplementary Figures 7B–D), respectively. In the southern coast of Australia, the strongest separation was disclosed between individuals from Gulf St Vincent and the other localities, followed by Wilsons Promontory compare to the west coast individuals. In the case of Australia's eastern coast, the northern localities were most distinct from the central-south localities, with a greater proportion of admixed individuals in the central localities, possibly representing a further sub-population. For New Zealand and Tasmania, differentiation was disclosed mainly between localities in the east coast and west coast of New Zealand, with Tasmanian individuals considerable admixed. However, most of the Tasmanian common dolphins showed higher probability of assignment to the New Zealand population (~57%) based on Admixture's Q-values (>0.8), and DAPC results (Supplementary Figures 6, 7), and were therefore considered primarily part of the New Zealand regional population.
Fixation indices indicated moderate genetic differentiation between the southern coast of Australia (SCA), eastern coast of Australia (ECA), and the New Zealand/Tasmania (NZT) regional populations (SCA vs. ECA = 0.060–0.213; SCA vs. NZT = 0.045–0.142; ECA vs. NZT = 0.018–0.142) (Figure 3A, Supplementary Table 6). In contrast, low genetic differentiation was observed between common dolphin subpopulations (Figures 3B–D). In the southern coast of Australia, the highest differences of FST were between Gulf St Vincent, Wilsons Promontory, and west southern coast vs. the other localities; whereas for the eastern coast of Australia, the northern localities showed the highest differentiation compared to the southern localities, followed by the central localities vs. the northern and southern localities. For New Zealand/Tasmania, the differentiation occurred between West coast of New Zealand/Tasmania vs. the localities from the east coast of New Zealand (Figure 3, Supplementary Figure 8).
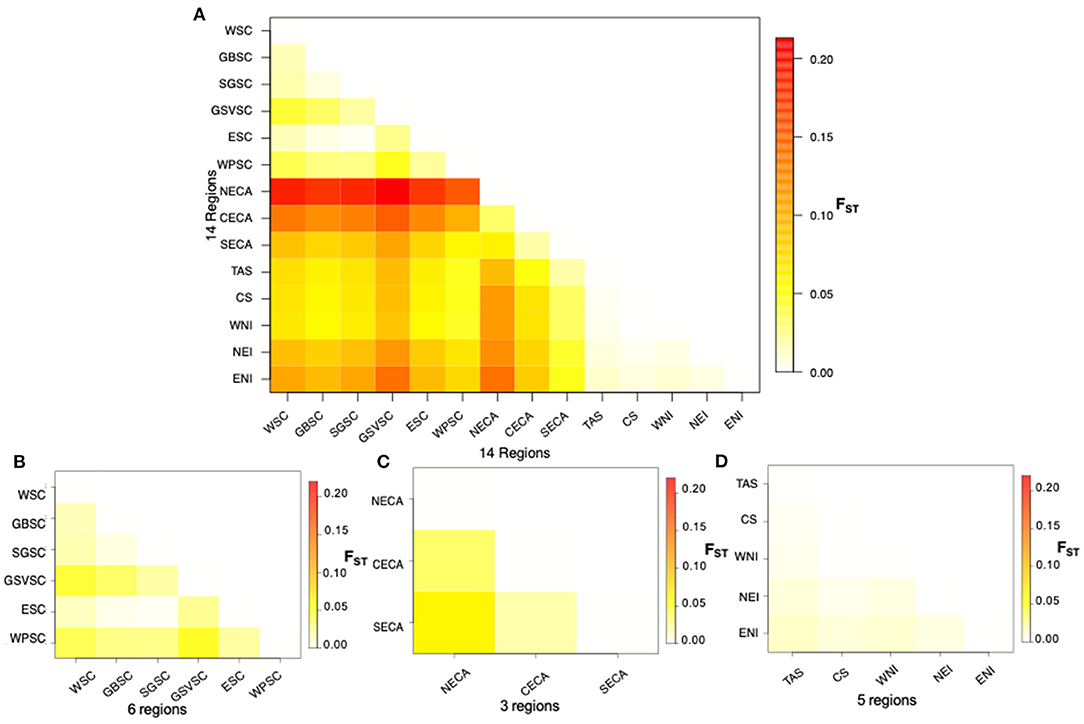
Figure 3. Heatmap of pairwise FST values between localities* based on 14,799 SNPs for Australasian common dolphins (Delphinus delphis); (A) Australia and New Zealand; (B) Southern coast of Australia; (C) Eastern coast of Australia; and (D) New Zealand and Tasmania. *Acronyms used as in Figure 1.
A significant signal of IBD was observed at the metapopulation level (r2 = 0.084, p = 0.003) (Figure 4A), and for the populations from the southern and eastern Australia (r2 = 0.346, p = 0.001; r2 = 0.742, p = 0.03, respectively). In contrast, there was no evidence of IBD in the New Zealand and Tasmania regional population (r2 = 0.005, p = 0.615) (Figures 4B–D).
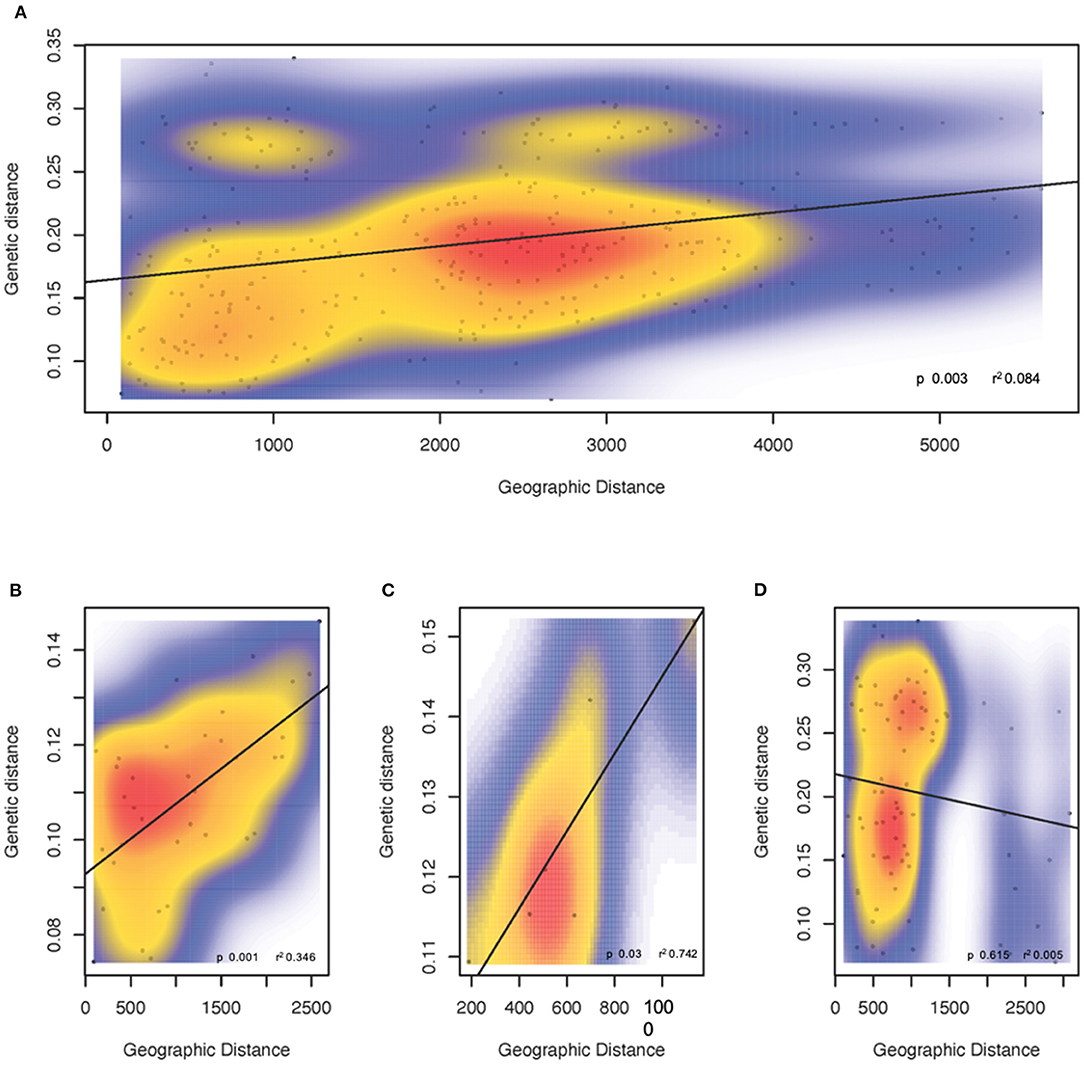
Figure 4. Isolation by distance between FST values and the shortest waterway distance based on 14,799 SNPs for Australasian common dolphins (Delphinus delphis). (A) Australia and New Zealand metapopulation; and regional populations, (B) Southern coast of Australia, (C) Eastern coast of Australia, and (D) New Zealand and Tasmania.
Contemporary Migration Rates and First-Generation Migrants
Estimates of contemporary migration rates based on BayesAss, that provide inferred rates of the portion of recent immigration over the last generations, indicated asymmetric migration between population pairs (Supplementary Table 2, Figure 5). There was relatively low estimates of migration (2–9%) between pairs of the three main regional populations, and moderate estimates of migration (6–25%) between pairs of the two subpopulations (Figure 5). First-generation migrants were detected in GeneClass between the three main regional populations, with 14 individuals rejected (p <0.01) from the population they were sampled in Supplementary Table 3. These individuals were retained in all the population analyses to provide a representative picture of the metapopulation dynamics (Supplementary Table 3).
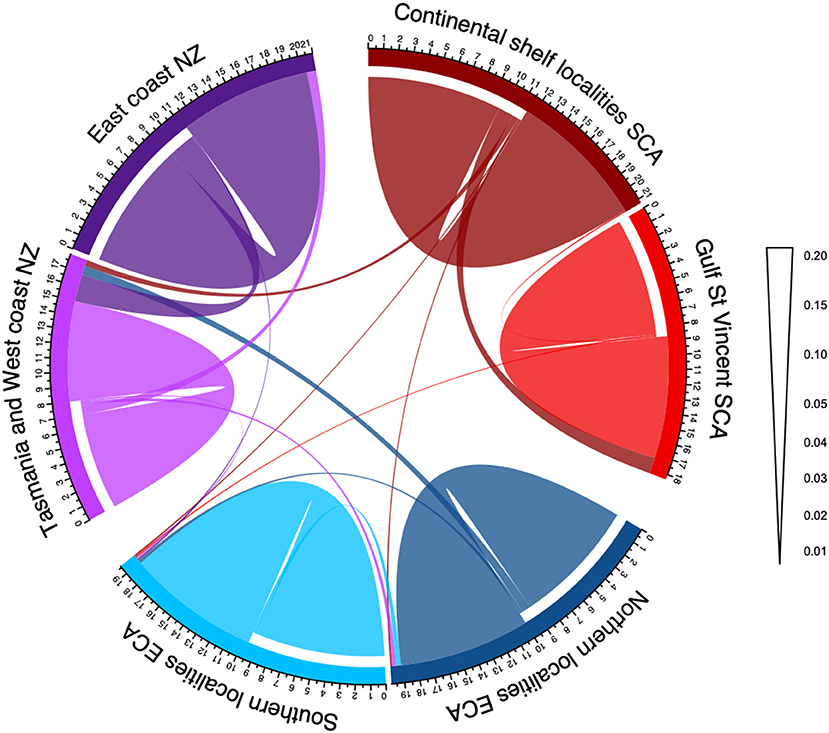
Figure 5. Circos plot of inferred contemporary migration rates (per last generations) between local populations of Australasian common dolphins (Delphinus delphis) based on 14,799 SNPs. Plot corresponds to the migration directionally (full values from BayesAss are provided in Supplementary Table 2). Width of the curves indicates the amount of migration according to the scale going from one subpopulation into another one. Scale bar is in units of the proportion of migrations. Ticks represent the gross number of migrants per 100s. Migration rate < 0.01 is not shown. SCA, *Southern coast of Australia; ECA, Eastern coast of Australia; NZ, New Zealand.
Discussion
The delineation of populations and their respective geographic boundaries, as well as estimation of the degree of connectivity between populations are crucial for the conservation management of small cetaceans (Rosel et al., 2017; Taylor et al., 2017; Dunn et al., 2019; Pierre, 2019; Sousa et al., 2019; Taft et al., 2020). Integrating genomic technology for answering these questions can inform about the dolphin populations and scale at which anthropogenic activities may impact upon them (e.g., Leslie and Morin, 2016). Genomic analyses also provide baseline information for design of further studies and the monitoring of populations; for example, the area at which to estimate population abundance and trends, data on parameters for modeling population persistence, and for estimating sustainable by-catch rates (Waples and Gaggiotti, 2006; Allendorf et al., 2010; Frankham et al., 2010; Grummer et al., 2019; Manel et al., 2019).
This study revealed a hierarchical metapopulation structure for Australasian common dolphins, with high levels of genome-wide diversity and negligible inbreeding among them. At a broad scale, the southern Indian Ocean was represented by a single regional population inhabiting the southern coast of Australia. The south-west Pacific Ocean was represented by two regional populations, one along the eastern coast of Australia and the other in New Zealand/Tasmania, which suggests substantial connectivity across the Tasman Sea. Further subdivision was disclosed at finer scales, with evidence for at least two subpopulations within each regional population, but perhaps more. The varying levels of population connectivity identified across inter-state and international jurisdictions, have substantial implications for the conservation and management of common dolphins, which are subject to interactions and mortalities in multiple fisheries in the region.
Population Structure of Australasian Common Dolphins
Studies investigating genetic partitioning at fine spatial scales in the sea usually provide evidence for distinct subpopulations, whereas metapopulations are generally disclosed when broader spatial scales are explored (Pitt and Kingsford, 2000; Dawson et al., 2014; Calò et al., 2016; Almany et al., 2017; Jasper et al., 2019). Nonetheless, sampling effort often makes it difficult to assess marine systems over broad scales, and as a consequence metapopulations may remain largely undisclosed (Manel et al., 2019). We assessed the population structure of common dolphins over a broad geographical area and revealed a hierarchical metapopulation structure across Australasia. Metapopulations have also been described for other dolphin species, such as spinner dolphins (Stenella longirostris) among Pacific Islands (Oremus et al., 2007), Hector's dolphins (Cephalorhynchus hectori hectori) between the west and east coasts of New Zealand (Heimeier et al., 2018), bottlenose dolphins (Tursiops truncatus) in the North Atlantic Ocean and Mediterranean Sea (Louis et al., 2014; Gaspari et al., 2015b), and Indo-Pacific bottlenose dolphins (Tursiops cf. australis) in southern Australia (Pratt et al., 2018). Although our sampling took place over 17 years, we believe there was little impact on the population genetic structure disclosed given than common dolphins exhibit a long generation time (~15 years; Taylor et al., 2007). In addition, we found similar patterns of genomic diversity for sites sampled in multiple years, and similar levels of genomic differentiation between sites sampled in same and different years (data not shown).
At a broad scale, Australasian common dolphins showed moderate genomic differentiation at the level of the two ocean basins investigated, the Indian and Pacific Oceans. The mtDNA dataset provided enough resolution to distinguish historical population structure between the two oceans basins, as previously demonstrated in other genetic studies of common dolphins (e.g. Amaral et al., 2012a,b), bottlenose dolphins (Tursiops spp.) (e.g., Tezanos-Pinto et al., 2009; Charlton-Robb et al., 2011), and killer whales (Orcinus orca) (e.g., Reeves et al., in review). This regional distinction was also clear based on analyses of the SNP dataset. In Australia, we found pronounced genomic divergence between common dolphins of the southern coast, the eastern coast, and Tasmania. This split was evident along the Wilsons Promontory, which was once a land-bridge (the Bassian Isthmus, ~14,000 ybp), which connected mainland Australia with Tasmania (Waters, 2008; Condie et al., 2011). The Wilsons Promontory region has been described as a prominent biogeographic boundary for many marine species (e.g., invertebrates, algae, small pelagic fish), accounting for many of the genetic discontinuities observed today along these coastlines (York et al., 2008; Teske et al., 2015, 2017; Costello et al., 2017).
An oceanographic perspective can also assist the interpretation of the pattern of regional genomic differentiation inferred in this study. The East Australian current (EAC) flows from the western boundary current into a southward direction, bringing warm and productive waters along the coast. The EAC is dominated by anticyclonic eddies, that creates three different water masses, and gradients of oceanographic variables along the coast, represented by northern, central and southern areas (Suthers et al., 2011). It then becomes weaker as it enters Tasmania and diverges eastward into New Zealand via the Tasman Front, where upwelling occurs mainly on the west coast (York et al., 2008; Flynn et al., 2018). These may impact on common dolphin movements along the eastern Australian region, leading to the pattern of local differentiation (Möller et al., 2011) and Tasman Sea regional separation.
The warm Leeuwin current runs south from western Australia into the southern coast of Australia. It then becomes the Great Australian Bight current, and later the Zeehan current, which extends from western Victoria into western Tasmanian waters, excluding the Bass Strait where currents in shallow waters tend to follow an eastward direction (York et al., 2008; Kämpf, 2015). Likewise, these currents may impact on common dolphin movements along the southern Australian region, leading to the proposed differentiation at finer-scales. The Zeehan current is weaker, and during the summer it is replaced by the cold Flinders current that enters from the west coast of Tasmania into the southern coast of Australia, bringing productive waters toward the continental shelf and leading to upwelling events (York et al., 2008; Lynch et al., 2014; Kämpf, 2015; Flynn et al., 2018). These complex oceanographic features cause variations in primary and secondary productivities along the two Australian coasts and in Tasmania, and may act as contemporary barriers that maintain historical divisions between marine organisms (Waters, 2008; Condie et al., 2011; Teske et al., 2017). While common dolphins have a high dispersal capability, their distributions are known to associate closely with that of their prey movements (e.g., Bilgmann et al., 2008; Meynier et al., 2008; Natoli et al., 2008; Zanardo et al., 2016; Peters et al., 2020), which may coincide with areas of high primary productivity along the two Australian coasts, as well as in New Zealand and Tasmania.
Previous population genetic studies of common dolphins based on microsatellite DNA markers suggested five subpopulations along the southern coast of Australia, including Tasmania (Bilgmann et al., 2014), three in the eastern coast of Australia (Möller et al., 2011), and three in New Zealand (Stockin et al., 2014), with the last two studies not including samples from Tasmania. At finer spatial scales, the genetic differences disclosed in our study suggested further subdivision within the identified Australasian regional populations. Two additional sub-populations were disclosed for New Zealand/Tasmania, two to four in southern Australia, and two to three in eastern Australia. These could potentially be explained by geological and oceanographic features (as mentioned above) reflecting on the contemporary population and feeding ecology of common dolphins. In the Australasian region, common dolphins are mainly found along continental shelf waters between the 20 and 200 m isobaths (Stockin et al., 2008, 2014; Möller et al., 2011; Meissner et al., 2015; Bilgmann et al., 2018; Peters and Stockin, 2021). By contrast, in the Gulf St Vincent, common dolphins are present in relatively shallow, protected waters (~20 m) of the inner gulf (Supplementary Figure 4), with seasonal circulation year-round and may represent a resident population (Filby et al., 2010; Kämpf and Bell, 2014). The geological formation during the Cenozoic, established the Gulf St Vincent and Spencer Gulf as inverse estuaries (Bourman et al., 2016). These unique formations provide highly productive ecosystems, offering shelter for common dolphin prey species, such as sardines (S. sagax) and anchovies (E. australis) (Filby et al., 2010; Kämpf and Bell, 2014). These characteristics may have impacted on site fidelity of dolphins to this area and over time leading to genetic differentiation of Gulf St Vincent animals to those outside the gulf.
In the case of the eastern Australia, the northern localities are oceanographically and biologically differentiated due to the presence of a distinct water mass (Keane and Neira, 2008; Suthers et al., 2011). This could affect the distribution of common dolphins if they feed upon particular fish assemblages (Möller et al., 2011), and in turn lead to restricted movement and genetic differentiation between subpopulations. Common dolphins along the continental shelf of southern and eastern Australia presented a strong signal of IBD, a finding consistent with other common dolphin studies carried out across different ocean basins (Amaral et al., 2012a; Bilgmann et al., 2014). With the use of genomic markers, the pattern of IBD was also disclosed in this study at smaller spatial scales.
In the New Zealand and Tasmania regional population, genetic subdivision was found between the west and east coasts of New Zealand. This pattern between west and east coast subdivision has also been reported for Hector's dolphins that inhabit the southern island of New Zealand (Heimeier et al., 2018). Common dolphins from the west coast of New Zealand and Tasmania appear to comprise a subpopulation exhibiting moderate gene flow to dolphins on New Zealand's east coast and, to a lesser extent, with populations in Australia's eastern coast. The latter could also be due to historical factors. After the last glacial cycle (12,000–120,000 ybp) (Ashe and Wilson, 2019), subpopulation differentiation of dolphins across the Tasman Sea could have occurred due to habitat preferences and changes in prey availability. In New Zealand, differences in major currents, such as the east and the west Auckland current in the North Island, and the D'Urville current in the Cook Strait (Ayers and Waters, 2005; Ross et al., 2009; Chiswell et al., 2015), may influence fish distribution (Papa et al., 2020), and could have also led to restrictions on dolphin movement, and subsequent genetic differentiation.
Contemporary Migration Within and Between Ocean Basins
In an idealized population, individuals that are closer to each other, are genetically more similar than individuals that are further apart (Hanski, 1998). This may lead to a pattern of IBD, which was a common finding of the study across both broad and fine spatial scales. Migration of individuals between populations can also be shaped by intrinsic and extrinsic factors, promoting genetic discontinuities across heterogeneous environments (Armansin et al., 2019; Grummer et al., 2019; Rajora, 2019). For example, individuals may be unable or unlikely to disperse across physical or environmental barriers, and thus gene flow between populations may become compromised (Armansin et al., 2019). The estimated migration rates were relatively small between ocean basins (i.e., Pacific and Indian Oceans) and the regional populations (i.e., Southern Australia, Eastern Australia, and New Zealand/Tasmania, as described above) (<6%), separated by strong oceanographic discontinuities (i.e., unique biogeographic region in the Bassian Isthmus (Waters et al., 2010; Teske et al., 2017) (Figure 5). By contrast, estimated migration rates between subpopulations within ocean basins and between more homogeneous environments were higher (<18%). The migration rates and the number of first-generation migrants identified support the idea that genetic connectivity mainly occurs between subpopulations nested within regional populations. This suggests that if an extinction event was to occur, by either natural or anthropogenic causes, a subpopulation's home range will more likely be recolonized by individuals from within that region (e.g., Sandoval-Castillo et al., 2018; Riginos et al., 2019; Waters et al., 2020). However, if such events are strong enough to prevent gene flow and change the availability of prey resources, the subpopulation could decline without replacement. These types of events have been recorded for common dolphin populations from the Mediterranean Sea, which suffered dramatic declines due to combined impacts from by-catch mortalities, reduction of prey availability, and habitat degradation (Genov et al., 2020).
It appears that, in addition to spatial distance, heterogeneous marine environments found across the distribution of Australasian common dolphins have led to low and moderate connectivity between and within regional populations, respectively. In southern Australia, gene flow is restricted from continental shelf waters to Gulf St Vincent (~5%). This protected environment allows common dolphin prey species to be locally available throughout the year (Filby et al., 2013; Ward et al., 2017; Goldsworthy et al., 2019a). The year-round availability of food resources could influence the feeding behavior of the common dolphins, maximizing their energy efficiency due to a lesser need for long-range movements, and perhaps increase reproductive success and lifetime fitness. All of these could lead to high site fidelity and residency by common dolphins to Gulf St Vincent, which while rare for the species, has been suggested for other semi-enclosed embayments in Australia, such as Port Philip Bay (Mason et al., 2016), and the Hauraki Gulf in New Zealand (Stockin et al., 2008, 2009a; Hupman, 2016; Peters et al., 2020). These characteristics, which may have led to genetic divergence of the Gulf St Vincent dolphins, also make them particularly at risk of decline due to interactions with fisheries in Gulf St Vincent and Investigator Strait (Hamer et al., 2008; Goldsworthy et al., 2019b).
In eastern Australia, stronger differentiation of common dolphins from the northern and southern localities translated in the lowest estimated migration rates (~3%) between any two subpopulations in Australasia. In this area, the EAC creates eddies, which act as barriers for eggs and larval fish (Condie et al., 2011; Suthers et al., 2011). Thus, given the close association of common dolphins' distribution to that of their prey, these circulation patterns and differences in water masses could potentially act as oceanographic barriers for dolphin movements and gene flow (Möller et al., 2011).
Within the New Zealand and Tasmania population, moderate migration rates were estimated between the two subpopulations (~18%). Migration here was strongly asymmetric, occurring mostly from the east into the west coast of New Zealand. Along the east coast of New Zealand there are several habitats (e.g., Hauraki Gulf), which have been proposed as breeding and calving areas for groups of common dolphins (Stockin et al., 2008; Dwyer et al., 2020). In this regional population, New Zealand's east coast appears to be acting as a genetic source, while the west coast, which presents higher rates of dolphin mortality due to fisheries (Thompson et al., 2013; Abraham et al., 2017), may be acting as a sink, similar to source-sink dynamics observed in other marine species (e.g., Benestan et al., 2016; DiBattista et al., 2017; Lal et al., 2017; Manel et al., 2019). Interestingly, Tasmania in Australia and the west coast of New Zealand displayed high connectivity over more than 1,000 km across the Tasman Sea, and genetic signatures show that individuals from western New Zealand are also found in Tasmanian waters. However, to the best of our knowledge, movements of common dolphins over long distances (~1,000 km) have only been document across the Mediterranean Sea through photo-ID (Genov et al., 2012). Similar patterns of connectivity across the Tasman Sea have been disclosed for other marine species, such as teleosts and invertebrates (e.g., Hippocampus abdominalis, Hoplosthethus atlanticus, Nerita melanogastrus) (Cumming et al., 2016; Flynn et al., 2018; Ashe and Wilson, 2019; Gardner et.al. unpublished). Historically, dispersal of marine species across the Tasman Sea seems to have occurred during the last glacial cycle (12,000–120,00 ybp), leading to the colonization of multiple areas by marine species in both countries (Ashe and Wilson, 2019). However, contemporary genetic connectivity seems to have been retained by oceanographic currents (Flynn et al., 2018). Further sampling and assessment of common dolphins from Tasmania may clarify whether this represents a contact area, and if they should be considered as a separate or combined unit for management with New Zealand. The latter would involve cross jurisdictional cooperation between policy makers.
Implications for Conservation and Management of Australasian Common Dolphins
Common dolphins in Australasia are mainly threatened due to by-catch in commercial fisheries (Abraham et al., 2017; Tulloch et al., 2020), and potentially by anthropogenic-associated competition for food resources. In Australia, incidental by-catch has occurred mainly in trawl fisheries and purse-seine fisheries that catch mackerel (T. declivis, T.s. murphyi, T. novaezelandiae, and S. australasicus) and sardines (S. sagax), as well as in gillnet fisheries targeting gummy sharks (Mustelus sp.) (Australian Goverment, 2019b). In New Zealand the observed incidental by-catch of common dolphins occurs mainly in mid-water trawl fisheries that catch the same mackerel species (Thompson et al., 2013; Abraham et al., 2017). However, by-catch within recreational set nets has also been documented as a threat to these dolphins (Stockin et al., 2009b). These incidental catches are known to have resulted in mortalities of hundreds of common dolphins in at least two of these fisheries (Abraham et al., 2017; Goldsworthy et al., 2019b). High mortalities were disclosed in 2004-2005 in the South Australian Sardine Fishery (SASF) (Hamer et al., 2008), likely exceeding the potential biological removal of individuals for one of the population's segment (Parra et al., in review). For New Zealand, an increase of common dolphin mortalities was reported between 2002 and 2003 in the trawl fishery (Thompson et al., 2013; MPI, 2019). After these periods, codes of practices were implemented in both fisheries, leading to a reduction in mortality rates (Hamer et al., 2008; Goldsworthy et al., 2019b; Pierre, 2019). More recently the Small Pelagic and Gillnet fisheries of Australia also implemented a by-catch trigger limit of six dolphins per operator, leading to a temporally exclusion of the vessel for 6 months from the fishing management zone if that occurs (Mackay et al., 2016; AFMA, 2019a,b). A recent study in New Zealand also reported that common dolphins are still one of the main marine mammal species accidentally caught by commercial fisheries (Abraham et al., 2017), although use of mitigation measures in the jack mackerel trawl fisheries has reduced mortalities to negligible levels in 2016-18 (Fisheries New Zealand, 2020). In Australia's SASF, an upsurge in mortalities has been recorded between 2018-19, with discrepancy in the data recorded by fishermen and independent observers (Goldsworthy et al., 2019b). These issues suggest that information about common dolphin population structure, connectivity and abundance are critical to evaluate the risk of by-catch to particular dolphin populations, and to establish strategies to mitigate the combined interactions and mortalities within the multiple fisheries.
In the Australasian region, fisheries that threaten common dolphins operate under different jurisdictions based on geographic delimitation and stock delineation for each prey targeted (Abraham and Thompson, 2015a,b,c; Patterson et al., 2019). The delineation of fish stocks represents different management zones (e.g., Supplementary Table 5) and often it does not consider the population structure of the targeted fish species (Papa et al., 2020). In addition, these management zones are not only used to manage the targeted species, but also to manage interactions and mortalities of by-caught species, such as common dolphins (Abraham and Thompson, 2015a,b,c; Mackay et al., 2016; AFMA, 2019a,b; Goldsworthy et al., 2019b; Patterson et al., 2019). Our findings suggest that regional populations and subpopulations of Australasian common dolphins are currently allocated across and within different fishing management zones. This suggests that the use of the management zones as presently implemented (e.g., Supplementary Table 5) could differentially impact populations of common dolphins.
Marine species that present connectivity over large spatial scales, such as common dolphins, need planning and implementation of conservation and management strategies over broad spatial scales that can guarantee the long-term persistence of populations (Rosel et al., 2017; Taylor et al., 2017; Dunn et al., 2019; Grummer et al., 2019; Manel et al., 2019; Sousa et al., 2019; Taft et al., 2020; Tulloch et al., 2020). Some of the dolphin subpopulations identified here are potentially at higher-risk of negative impacts from the fisheries. For example, the Gulf St Vincent subpopulation, which is possibly resident and relatively small (Filby et al., 2010), suffers by-catch induced mortalities by the SASF (Goldsworthy et al., 2019b), and these could potentially impact their long-term viability. In the West coast of New Zealand-Tasmania subpopulation, interactions with common dolphins and other top predators occur mainly with the mid-water trawl fisheries (Kemper et al., 2003; Thompson et al., 2013; Hamilton and Baker, 2019). Both of these fisheries have implemented codes of practice to reduce the number of entanglements and mortalities, including not setting nets when a cetacean sighting occurs, reporting cetacean interactions, modifying the fishery's gear (FAO, 1995; Rowe, 2007; Hamer et al., 2008; Goldsworthy et al., 2019b), and/or implementing an annual assessment with independent observers (Hamer et al., 2008; Goldsworthy et al., 2019b). Nevertheless, the management zones used to mitigate common dolphin interactions with these fisheries (Supplementary Table 5) are not in concordance with the population genomic structure of common dolphin disclosed here (Figure 2).
In Australasia, small cetacean populations have been generally managed in zones or units that do not reflect their genetic structure (e.g., Möller et al., 2001, 2011; Krutzen and Sherwin, 2004; Möller and Beheregaray, 2004; Bilgmann et al., 2007b, 2008, 2014, 2018; Wiszniewski et al., 2009; Amaral et al., 2012a; Stockin et al., 2014; Zanardo et al., 2016, 2017; Pratt et al., 2018). These could potentially make dolphin populations more vulnerable to decline due to anthropogenic impacts, as exemplified for common dolphins in the Mediterranean Sea (e.g., Natoli et al., 2008; Moura et al., 2013; Genov et al., 2020). These challenges highlight the need of using genetics and genomics markers as a tool for delineating population and estimating connectivity for biological meaningful management zones to be implemented (Funk et al., 2012; Leslie and Morin, 2016; Rosel et al., 2017; Taylor et al., 2017; Dunn et al., 2019). In particular, genetic and genomic analyses provide an opportunity to identify populations or subpopulations that require prioritization or additional conservation policies.
In this study, we found that Australasian common dolphins present a complex hierarchical metapopulation, with nested subpopulations within regional populations. The estimated contemporary migration rates between most of these subpopulations suggest that they are not entirely genetically or demographically independent, and therefore common dolphins in the Australasian region should be managed on both meso-scale (regional population level) and fine-scale (subpopulation level). Each population and subpopulation are relevant to conserve for the maintenance of the complex metapopulation system. Subpopulations with potentially high-risk of anthropogenic impacts due to fisheries may act as sink population as previously reported for other marine species with sink-source dynamics (e.g., Benestan et al., 2016; Lal et al., 2017; Manel et al., 2019; Rajora, 2019). However, the levels of contemporary migration suggest that the subpopulations identified cannot be managed entirely separately. Thus, we suggest that assessment and management of by-catch interactions and mortalities of common dolphins needs to be considered across multiple fisheries, management zones, and jurisdictions for adequate conservation management to occur. For example, interactions and mortalities in the West coast of New Zealand need to be perhaps considered in conjunction with impacts on common dolphins in Tasmania, as they (provisionally) appear to belong to the same subpopulation, albeit with moderate connectivity to other southeastern Australian localities. The equivalent level of management should be applied within southern Australian states (southern Western Australia, South Australia, Victoria) and within eastern Australia states (New South Wales and southern Queensland). Therefore, potential management zones for mitigation and assessments of common dolphin by-catch in Australasia need to be based on populations' boundaries and connectivity, and through collaboration between inter-state and international jurisdictions.
Our findings integrating genetics and genomics markers provide reliable estimates of population structure and connectivity at broad and fine spatial scales for common dolphins of Australasia. Future risk assessments of by-catch and potential biological removal will require an application of the population structure and connectivity information presented here. Genomic analyses are also essential in additional studies addressing adaptation in marine ecosystems, in which selection can potentially further clarify substructure within regional populations (e.g., Shafer et al., 2015; Bernatchez et al., 2018; Hendricks et al., 2018; Sandoval-Castillo et al., 2018; Xuereb et al., 2018; Rajora, 2019). This is a topical issue that needs to be considered in future conservation policies of marine ecosystems given the increase in anthropogenic impacts and ongoing changes of Earth's climate.
Data Availability Statement
The datasets used for this study can be found in the online repository Figshare at: https://figshare.com/s/083be1832e010f249797, https://figshare.com/s/cff21a1394ad83c55f83, and https://figshare.com/s/683409860163e2d08e75
Ethics Statement
The animal study was reviewed and approved by under the ethics approval of the Flinders University Macquarie University, and Massey University animal ethics committees, and under research permits from Flinders University and South Australia Health Service Animal Welfare Committee (E326); DENR,SA (E25889); DEC,WA (SF008961); DSE,VIC (FF383247); DECCW, NSW (A2126); DEWHA (2008-001), SA State water a Ministerial Exemption PIRSA (9902404); KS and EB New Zealand Department of Conservation (RNW/HO/2008/03); GT-P New Zealand Department of Conservation (DOCDM-13171418); GT-P and KS New Zealand (AEC/17 14/25).
Author Contributions
Conceptualization and first manuscript were developed by AB, LM, and LB. Samples were provided by KS, KB, LM, NZ, GT-P, EB, KH, and GP. Genetic data and analyses was performed by AB and JS-C. All authors have made contributions to the manuscript.
Funding
Ministry for Primary Industries of New Zealand (Project PRO201708A) and the National Institute of Water and Atmospheric Research (KH, KS, LM, and LB). The Australian Marine Mammal Centre (AMMC) Grants Program, Australian Antarctic Division (AAD) (project number 2010/3, LM, GP, KB, and LB). Ph.D. scholarship from the Mexican National Council for Science and Technology to AB, and Flinders University.
Conflict of Interest
The authors declare that the research was conducted in the absence of any commercial or financial relationships that could be construed as a potential conflict of interest.
Acknowledgments
AB is grateful for the support from CONACYT (the Mexican National Council for Science and Technology) Scholarship (Grant No. 440689) and sponsorship from Flinders University, as well as proof reading of the manuscript by Orlando Lam-Gordillo. We are thankful for all the samples provided and the work of many volunteers in the field. Biopsy samples were available through CEBEL/MELFU at Flinders University and the CERG at Massey University. We are thankful to the Massey University, University of Auckland, the National Institute of Water and Atmospheric Research and the Ministry of Primary Industries of New Zealand, the Tasmanian Museum and Art Gallery, Rosemary Gales, and Kris Carlyon and members of the Department of Primary Industries, Parks, Water and Environment of Tasmania, for providing tissues samples of stranding and by-catch individuals of common dolphins. Also, we thank the Ministry for Primary Industries, New Zealand, the Australian Marine Mammal Centre (AMMC) Grants Program and the Australian Antarctic Division (AAD) for funding part of this work. We thank the editor and the reviewers whose comments improved this manuscript. Finally but not least we are really thankful for Iwi collaborative partnership that permitted the genetic analyses of NZ cetacean samples. We acknowledge the biological whakapapa this research represents to mana whenua.
Supplementary Material
The Supplementary Material for this article can be found online at: https://www.frontiersin.org/articles/10.3389/fmars.2021.616673/full#supplementary-material
References
Abraham, E. R., Neubauer, P., Berkenbusch, K., and Richard, Y. (2017). Assessment of the Risk to New Zealand Marine Mammals From Commercial Fisheries. New Zealand Aquatic Environment and Biodiversity Report No. 189: Ministry of Primary Industries.
Abraham, E. R., and Thompson, F. N. (2015a). Captures of Common Dolphin in Setnet Fisheries, in the New Zealand Exclusive Economic Zone, During the 2017–18 Fishing Year [Online]. Available online at: psc.dragonfly.co.nz/2019v1/released/common/dolphin/setnet/all/vessels/eez/2017/18/ (accessed September 24, 2020).
Abraham, E. R., and Thompson, F. N. (2015b). Captures of Common Dolphin in Surface Longline Fisheries, in the New Zealand Exclusive Economic Zone, During the 2017–18 Fishing Year [Online]. Available online at: psc.dragonfly.co.nz/2019v1/released/common/dolphin/surface/longline/all/vessels/eez/2017/18/ (accessed September 24, 2020).
Abraham, E. R., and Thompson, F. N. (2015c). Captures of Common Dolphin in Trawl Fisheries, in the New Zealand Exclusive Economic Zone, During the 2017–18 Fishing Year [Online]. Available online at: psc.dragonfly.co.nz/2019v1/released/common/dolphin/trawl/all/vessels/eez/2017/18/ (accessed September 24, 2020).
AFMA (2019a). Gillnet Dolphin Mitigation Strategy. Minimising Dolphin Interactions With Gillnets in the Southern and Eastern Scalefish and Shark Fishery. Australian Fisheries Management Authority (AFMA). Available online at: afma.gov.au/sites/default/files/gillnet_dolphin_mitigation_strategy_updated_aug_2019_accessible.pdf
AFMA (2019b). Small Pelagic Fishery Dolphin Strategy. Minimising Dolphin Interactions in the Small Pelagic Fishery. Australian Fisheries Management Authority (AFMA). Available online at afma.gov.au/sites/default/files/spf_dolphin_strategy_2019_updated_accessible.pdf
AFMA (2020). Protected Species Interactions Quarterly Reports: 2012-2019. Australian Fisheries Management Authority (AFMA). Available online at: afma.gov.au/sustainability/environment/protected/species/management/protected/species/interaction/reports
Alexander, D. H., and Lange, K. (2011). Enhancements to the ADMIXTURE algorithm for individual ancestry estimation. BMC Bioinformatics 12:246. doi: 10.1186/1471-2105-12-246
Alexander, D. H., Novembre, J., and Lange, K. (2009). Fast model-based estimation of ancestry in unrelated individuals. Genome Res. 19, 1655–1664. doi: 10.1101/gr.094052.109
Allendorf, F. W., Hohenlohe, P. A., and Luikart, G. (2010). Genomics and the future of conservation genetics. Nat. Rev. Genet. 11, 697–709. doi: 10.1038/nrg2844
Almany, G. R., Planes, S., Thorrold, S. R., Berumen, M. L., Bode, M., Saenz-Agudelo, P., et al. (2017). Larval fish dispersal in a coral-reef seascape. Nat. Ecol. Evol. 1:148. doi: 10.1038/s41559-017-0148
Amaral, A. R., Beheregaray, L. B., Bilgmann, K., Boutov, D., Freitas, L., Robertson, K. M., et al. (2012a). Seascape genetics of a globally distributed, highly mobile marine mammal: the short-beaked common dolphin (Genus Delphinus). PLoS ONE 7:e31482. doi: 10.1371/journal.pone.0031482
Amaral, A. R., Beheregaray, L. B., Bilgmann, K., Freitas, L., Robertson, K. M., Sequeira, M., et al. (2012b). Influences of past climatic changes on historical population structure and demography of a cosmopolitan marine predator, the common dolphin (genus Delphinus). Mol. Ecol. 21, 4854–4871. doi: 10.1111/j.1365-294X.2012.05728.x
Ansmann, I. C., Parra, G. J., Lanyon, J. M., and Seddon, J. M. (2012). Fine-scale genetic population structure in a mobile marine mammal: inshore bottlenose dolphins in Moreton Bay, Australia. Mol. Ecol. 21, 4472–4485. doi: 10.1111/j.1365-294X.2012.05722.x
Armansin, N. C., Stow, A. J., Cantor, M., Leu, S. T., Klarevas-Irby, J. A., Chariton, A. A., et al. (2019). Social barriers in ecological landscapes: the social resistance hypothesis. Trends Ecol. Evol. 35, 137–148. doi: 10.1016/j.tree.2019.10.001
Ashe, J. L., and Wilson, A. B. (2019). Navigating the southern seas with small fins: genetic connectivity of seahorses (Hippocampus abdominalis) across the Tasman Sea. J. Biogeogr. 47, 207–219. doi: 10.1111/jbi.13733
Ayers, K. L., and Waters, J. M. (2005). Marine biogeographic disjunction in central New Zealand. Mar. Biol. 147, 1045–1052. doi: 10.1007/s00227-005-1632-7
Banks, J., Levine, K., Syvanen, M., Theis, J., and Gilson, A. (1995). DNA Tissue Gender Typing. Standard Operating Procedure of the Wildlife Forensic Laboratory of the California Department of Fish and Game. Sacramento, CA: California Department of Fish and Game.
Batley, K. C., Sandoval-Castillo, J., Kemper, C. M., Attard, C. R. M., Zanardo, N., Tomo, I., et al. (2019). Genome-wide association study of an unusual dolphin mortality event reveals candidate genes for susceptibility and resistance to cetacean morbillivirus. Evol. Appl. 12, 718–732. doi: 10.1111/eva.12747
Benestan, L., Quinn, B. K., Maaroufi, H., Laporte, M., Clark, F. K., Greenwood, S. J., et al. (2016). Seascape genomics provides evidence for thermal adaptation and current-mediated population structure in American lobster (Homarus americanus). Mol. Ecol. 25, 5073–5092. doi: 10.1111/mec.13811
Benjamini, Y., and Yekutieli, D. (2001). The control of the false discovery rate in multiple testing under dependency. Ann. Statist. 29, 1165–1188. doi: 10.1214/aos/1013699998
Bernatchez, S., Xuereb, A., Laporte, M., Benestan, L., Steeves, R., Laflamme, M., et al. (2018). Seascape genomics of eastern oyster (Crassostrea virginica) along the Atlantic coast of Canada. Evol. Appl. 12, 587–609. doi: 10.1111/eva.12741
Beugin, M. P., Gayet, T., Pontier, D., Devillard, S., and Jombart, T. (2018). A fast likelihood solution to the genetic clustering problem. Methods Ecol. Evol. 9, 1006–1016. doi: 10.1111/2041-210X.12968
Bilgmann, K., Griffiths, O. J., Allen, S. J., and Möller, L. M. (2007a). A biopsy pole system for bow-riding dolphins: sampling success, behavioral responses, and test for sampling bias. Mar. Mamm. Sci. 23, 218–225. doi: 10.1111/j.1748-7692.2006.00099.x
Bilgmann, K., Möller, L. M., Harcourt, R. G., Gales, R., and Beheregaray, L. B. (2008). Common dolphins subject to fisheries impacts in Southern Australia are genetically differentiated: implications for conservation. Anim. Conserv. 11, 518–528. doi: 10.1111/j.1469-1795.2008.00213.x
Bilgmann, K., Möller, L. M., Harcourt, R. G., Gibbs, S. E., and Beheregaray, L. (2007b). Genetic differentiation in bottlenose dolphins from South Australia: association with local oceanography and coastal geography. Mar. Ecol. Prog. Ser. 341, 265–276. doi: 10.3354/meps341265
Bilgmann, K., Parra, G. J., and Möller, L. M. (2018). Occurrence, distribution and abundance of cetaceans off the western Eyre Peninsula in the Great Australian Bight. Deep Sea Res. Part II Top. Stud. Oceanogr. 157–158, 134–145. doi: 10.1016/j.dsr2.2017.11.006
Bilgmann, K., Parra, G. J., Zanardo, N., Beheregaray, L. B., and Möller, L. M. (2014). Multiple management units of short-beaked common dolphins subject to fisheries bycatch off southern and southeastern Australia. Mar. Ecol. Prog. Ser. 500, 265–279. doi: 10.3354/meps10649
Bourman, R. P., Murray-Wallace, C. V., and Harvey, N. (2016). Coastal Landscapes of South Australia. Adelaide, SA: University of Adelaide Press.
Bradburd, G. S., Coop, G. M., and Ralph, P. L. (2018). Inferring continuous and discrete population genetic structure across space. Genetics 210, 33–52. doi: 10.1534/genetics.118.301333
Breed, M. F., Harrison, P. A., Blyth, C., Byrne, M., Gaget, V., Gellie, N. J. C., et al. (2019). The potential of genomics for restoring ecosystems and biodiversity. Nat. Rev. Genet. 20, 615–628. doi: 10.1038/s41576-019-0152-0
Caballero, S., Islas-Villanueva, V., Tezanos-Pinto, G., Duchene, S., Delgado-Estrella, A., Sanchez-Okrucky, R., et al. (2012). Phylogeography, genetic diversity and population structure of common bottlenose dolphins in the Wider Caribbean inferred from analyses of mitochondrial DNA control region sequences and microsatellite loci: conservation and management implications. Anim. Conserv. 15, 95–112. doi: 10.1111/j.1469-1795.2011.00493.x
Calò, A., Muñoz, I., Pérez-Ruzafa, Á., Vergara-Chen, C., and García-Charton, J. A. (2016). Spatial genetic structure in the saddled sea bream (Oblada melanura [Linnaeus, 1758]) suggests multi-scaled patterns of connectivity between protected and unprotected areas in the Western Mediterranean Sea. Fish. Res. 176, 30–38. doi: 10.1016/j.fishres.2015.12.001
Cammen, K. M., Andrews, K. R., Carroll, E. L., Foote, A. D., Humble, E., Khudyakov, J. I., et al. (2016). Genomic methods take the plunge: recent advances in high-throughput sequencing of marine mammals. J. Hered. 107, 481–495. doi: 10.1093/jhered/esw044
Catchen, J., Hohenlohe, P. A., Bassham, S., Amores, A., and Cresko, W. A. (2013). Stacks: an analysis tool set for population genomics. Mol. Ecol. 22, 3124–3140. doi: 10.1111/mec.12354
Charlton-Robb, K., Gershwin, L. A., Thompson, R., Austin, J., Owen, K., and McKechnie, S. (2011). A new dolphin species, the Burrunan Dolphin Tursiops australis sp. nov., endemic to southern Australian coastal waters. PLoS ONE 6:e24047. doi: 10.1371/journal.pone.0024047
Chiswell, S. M., Bostock, H. C., Sutton, P. J. H., and Williams, M. J. M. (2015). Physical oceanography of the deep seas around New Zealand: a review. N. Z. J. Mar. Freswater Res. 49, 286–317. doi: 10.1080/00288330.2014.992918
Clement, M., Snell, Q., Walke, P., Posada, D., and Crandall, K. (2002). “TCS: estimating gene genealogies,” in Proceedings 16th International Parallel and Distributed Processing Symposium (Ft. Lauderdale, FL), 7. doi: 10.1109/IPDPS.2002.1016585
Compton, B. W., McGarigal, K., Cushman, S. A., and Gamble, L. R. (2007). A resistant-kernel model of connectivity for amphibians that breed in vernal pools. Conserv. Biol. 21, 788–799. doi: 10.1111/j.1523-1739.2007.00674.x
Condie, S. A., Mansbridge, J. V., and Cahill, M. L. (2011). Contrasting local retention and cross-shore transports of the East Australian Current and the Leeuwin Current and their relative influences on the life histories of small pelagic fishes. Deep Sea Res. Part II Top. Stud. Oceanogr. 58, 606–615. doi: 10.1016/j.dsr2.2010.06.003
Costello, M. J., Tsai, P., Wong, P. S., Cheung, A. K. L., Basher, Z., and Chaudhary, C. (2017). Marine biogeographic realms and species endemicity. Nat. Commun. 8:1057. doi: 10.1038/s41467-017-01121-2
Cumming, R. A., Nikula, R., Spencer, H. G., and Waters, J. M. (2016). Trans-Tasman genetic connectivity in the intertidal air-breathing slug Onchidella nigricans. Mar. Ecol. Prog. Ser. 562, 93–100. doi: 10.3354/meps11926
Danecek, P., Auton, A., Abecasis, G., Albers, C. A., Banks, E., DePristo, M. A., et al. (2011). The variant call format and VCFtools. Bioinformatics 27, 2156–2158. doi: 10.1093/bioinformatics/btr330
Dawson, M. N., Cieciel, K., Decker, M. B., Hays, G. C., Lucas, C. H., and Pitt, K. A. (2014). Population-level perspectives on global change: genetic and demographic analyses indicate various scales, timing, and causes of scyphozoan jellyfish blooms. Biol. Invas. 17, 851–867. doi: 10.1007/s10530-014-0732-z
DiBattista, J. D., Travers, M. J., Moore, G. I., Evans, R. D., Newman, S. J., Feng, M., et al. (2017). Seascape genomics reveals fine-scale patterns of dispersal for a reef fish along the ecologically divergent coast of Northwestern Australia. Mol. Ecol. 26, 6206–6223. doi: 10.1111/mec.14352
Dunn, D. C., Harrison, A. L., Curtice, C., DeLand, S., Donnelly, B., Fujioka, E., et al. (2019). The importance of migratory connectivity for global ocean policy. Proc. Biol. Sci. 286:20191472. doi: 10.1098/rspb.2019.1472
Dwyer, S. L., Pawley, M. D. M., Clement, D. M., and Stockin, K. A. (2020). Modelling habitat use suggests static spatial exclusion zones are a non-optimal management tool for a highly mobile marine mammal. Mar. Biol. 167:62. doi: 10.1007/s00227-020-3664-4
Excoffier, L., and Lischer, H. E. (2010). Arlequin suite ver 3.5: a new series of programs to perform population genetics analyses under Linux and Windows. Mol. Ecol. Resour. 10, 564–567. doi: 10.1111/j.1755-0998.2010.02847.x
FAO (1995). Code of Conduct for Responsible Fisheries. Rome: Food and Agriculture Organization of the United Nations (FAO).
Filby, N. E., Bossley, M., Sanderson, K. J., Martinez, E., and Stockin, K. A. (2010). Distribution and population demographics of common dolphins (Delphinus delphis) in the Gulf St. Vincent, South Australia. Aquat. Mamm. 36, 33–45. doi: 10.1578/am.36.1.2010.33
Filby, N. E., Bossley, M., and Stockin, K. A. (2013). Behaviour of free-ranging short-beaked common dolphins (Delphinus delphis) in Gulf St Vincent, South Australia. Aust. J. Zool. 61, 291–300. doi: 10.1071/zo12033
Fisheries New Zealand (2020). Aquatic Environment and Biodiversity Annual Review 2019–20. Compiled by the Aquatic Environment Team, Fisheries Science and Information, Fisheries New Zealand, Wellington New Zealand, 765. Available online at: www.mpi.govt.nz/dmsdocument/40980-aquatic-environment-and-biodiversity-annual-review-201920
Flynn, A. J., Kloser, R. J., and Sutton, C. (2018). Micronekton assemblages and bioregional setting of the Great Australian Bight: a temperate northern boundary current system. Deep Sea Res. Part II Top. Stud. Oceanogr. 157–158, 58–77. doi: 10.1016/j.dsr2.2018.08.006
Foll, M., and Gaggiotti, O. (2008). A genome-scan method to identify selected loci appropriate for both dominant and codominant markers: a Bayesian perspective. Genetics 180, 977–993. doi: 10.1534/genetics.108.092221
Foote, A. D., Vijay, N., Avila-Arcos, M. C., Baird, R. W., Durban, J. W., Fumagalli, M., et al. (2016). Genome-culture coevolution promotes rapid divergence of killer whale ecotypes. Nat. Commun. 7:11693. doi: 10.1038/ncomms11693
Frankham, R., Ballou, J. D., and Briscoe, D. A. (2010). Introduction to Conservation Genetics, 2nd ed. (Cambridge, UK; New York, NY: Cambridge University Press).
Fruet, P., Secchi, E. R., Daura-Jorge, F., Vermeulen, E., Flores, P. A. C., Simões-Lopes, P. C., et al. (2014). Remarkably low genetic diversity and strong population structure in common bottlenose dolphins (Tursiops truncatus) from coastal waters of the Southwestern Atlantic Ocean. Conserv. Genet. 15, 879–895. doi: 10.1007/s10592-014-0586-z)
Fullard, K. J., Early, G., Heide-Jorgensen, M. P., Bloch, D., Rosing-Asvid, S. A., and Amos, W. (2000). Population structure of long-finned pilot whales in the North Atlantic: a correlation with sea surface temperature? Mol. Ecol. 9, 949–958. doi: 10.1046/j.1365-294x.2000.00957.x
Funk, W. C., McKay, J. K., Hohenlohe, P. A., and Allendorf, F. W. (2012). Harnessing genomics for delineating conservation units. Trends Ecol. Evol. 27, 489–496. doi: 10.1016/j.tree.2012.05.012
Gardner, B., Hoban, S., and Luikart, G. (2020). IUCN Red List and the value of integrating genetics. Conserv. Genet. 21, 795–801. doi: 10.1007/s10592-020-01301-6
Gaspari, S., Holcer, D., Mackelworth, P., Fortuna, C., Frantzis, A., Genov, T., et al. (2015a). Population genetic structure of common bottlenose dolphins (Tursiops truncatus) in the Adriatic Sea and contiguous regions: implications for international conservation. Aquatic Conserv. Mar. Freshw. Ecosyst. 25, 212–222. doi: 10.1002/aqc.2415
Gaspari, S., Scheinin, A., Holcer, D., Fortuna, C., Natali, C., Genov, T., et al. (2015b). Drivers of Population Structure of the Bottlenose Dolphin (Tursiops truncatus) in the Eastern Mediterranean Sea. Evol. Biol. 42, 177–190. doi: 10.1007/s11692-015-9309-8
Genov, T., Bearzi, G., Bonizzoni, S., and Tempesta, M. (2012). Long-distance movement of a lone short-beaked common dolphin Delphinus delphis in the central Mediterranean Sea. Mar. Biodivers. Rec. 5:E9. doi: 10.1017/S1755267211001163
Genov, T., Kotnjek, P., and Centrih, T. (2020). Occurrence of common dolphins (Delphinus delphis) in the Gulf of Trieste and the northern Adriatic Sea. Aquat. Conserv. Mar. Freshw. Ecosyst. 1–7. doi: 10.1002/aqc.3407
Goldsworthy, S. D., Bailleul, F., NurseyBray, M., Mackay, A., Oxley, A., Reinhold, S.-L., et al. (2019a). Assessment of the Impacts of Seal Populations on the Seafood Industry in South Australia. Adelaide, SA: South Australian Research and Development Institute (Aquatic Sciences).
Goldsworthy, S. D., Ferguson, G. J., and Ward, T. M. (2019b). Assessment of Dolphin Interactions, Effectiveness of Code of Practice and Fishing Behaviour in the South Australian Sardine Fishery: 2018-19. Report to PIRSA Fisheries and Aquaculture. South Australian Research and Development Institute (Aquatic Sciences). F2010/000726-10. SARDI Research Report Series No. 1041, 36.
Grummer, J. A., Beheregaray, L. B., Bernatchez, L., Hand, B. K., Luikart, G., Narum, S. R., et al. (2019). Aquatic landscape genomics and environmental effects on genetic variation. Trends Ecol. Evol. 34, 641–654. doi: 10.1016/j.tree.2019.02.013
Hamer, D. J., Ward, T. M., and McGarvey, R. (2008). Measurement, management and mitigation of operational interactions between the South Australian Sardine Fishery and short-beaked common dolphins (Delphinus delphis). Biol. Conserv. 141, 2865–2878. doi: 10.1016/j.biocon.2008.08.024
Hamilton, S., and Baker, G. B. (2019). Technical mitigation to reduce marine mammal bycatch and entanglement in commercial fishing gear: lessons learnt and future directions. Rev. Fish Biol. 29, 223–247. doi: 10.1007/s11160-019-09550-6
Heimeier, D., Alexander, A., Hamner, R. M., Pichler, F., and Baker, C. S. (2018). The influence of selection on MHC DQA and DQB haplotypes in the endemic New Zealand hector's and maui dolphins. J. Hered. 109, 744–756. doi: 10.1093/jhered/esy050
Hendricks, S., Anderson, E. C., Antao, T., Bernatchez, L., Forester, B. R., Garner, B., et al. (2018). Recent advances in conservation and population genomics data analysis. Evol. Appl. 11, 1197–1211. doi: 10.1111/eva.12659
Hoelzel, A. R. (1998). Genetic structure of cetacean populations in sympatry, parapatry, and mixed assemblages: implications for conservation policy. J. Hered. 89, 451–458. doi: 10.1093/jhered/89.5.451
Hoelzel, A. R., Hey, J., Dahlheim, M. E., Nicholson, C., Burkanov, V., and Black, N. (2007). Evolution of population structure in a highly social top predator, the killer whale. Mol. Biol. Evol. 24, 1407–1415. doi: 10.1093/molbev/msm063
Holderegger, R., Schmidt, B. R., Grünig, C., Meier, R., Csencsics, D., Gassner, M., et al. (2020). Ready-to-use workflows for the implementation of genetic tools in conservation management. Conserv. Genet. Resour. 12, 691–700. doi: 10.1007/s12686-020-01165-5
Holland, L. P., Jenkins, T. L., and Stevens, J. R. (2017). Contrasting patterns of population structure and gene flow facilitate exploration of connectivity in two widely distributed temperate octocorals. J. Hered. 119, 35–48. doi: 10.1038/hdy.2017.14
Hupman, K. (2016). Photo-identification and its application to gregarious delphinids: Common dolphins (Delphinus sp.) in the Hauraki Gulf, New Zealand (Doctor of Philosophy). Massey University, Albany, New Zeland.
Hupman, K., Stockin, K. A., Pollock, K., Pawley, M. D. M., Dwyer, S. L., Lea, C., et al. (2018). Challenges of implementing Mark-recapture studies on poorly marked gregarious delphinids. PLoS ONE 13:e0198167. doi: 10.1371/journal.pone.0198167
Jasper, M., Schmidt, T. L., Ahmad, N. W., Sinkins, S. P., and Hoffmann, A. A. (2019). A genomic approach to inferring kinship reveals limited intergenerational dispersal in the yellow fever mosquito. Mol. Ecol. Resour. 19, 1254–1264. doi: 10.1111/1755-0998.13043
Jombart, T., and Ahmed, I. (2011). adegenet 1.3-1: new tools for the analysis of genome-wide SNP data. Bioinformatics 27, 3070–3071. doi: 10.1093/bioinformatics/btr521
Jombart, T., and Collins, C. (2015). A Tutorial for Discriminant Analysis of Principal Components (DAPC) Using Adegenet 2.0.0. Available online at: adegenet.r-forge.r-project.org/files/tutorial-dapc.pdf
Kämpf, J. (2015). Phytoplankton blooms on the western shelf of Tasmania: evidence of a highly productive ecosystem. Ocean Sci. 11, 1–11. doi: 10.5194/os-11-1-2015
Kämpf, J., and Bell, D. (2014). “The murray/coorong estuary: meeting of the waters?,” in Estuaries of Australia in 2050 and Beyond, ed E. Wolanski (Dordrecht; Heidelberg; New York, NY; London: Springer), 31–47.
Keane, J. P., and Neira, F. J. (2008). Larval fish assemblages along the south-eastern Australian shelf: linking mesoscale non-depth-discriminate structure and water masses. Fish. Oceanogr. 17, 263–280. doi: 10.1111/j.1365-2419.2008.00478.x
Kearse, M., Moir, R., Wilson, A., Stones-Havas, S., Cheung, M., Sturrock, S., et al. (2012). Geneious Basic: an integrated and extendable desktop software platform for the organization and analysis of sequence data. Bioinformatics 28, 1647–1649. doi: 10.1093/bioinformatics/bts199
Kemper, C., Pemberton, D., Cawthorn, M., Heinrich, S., Mann, J., Würsig, B., et al. (2003). “Aquaculture and marine mammals: co-existence or conflict?,” in Marine Mammals: Fisheries, Tourism and Management Issues, eds N. Gales, M. Hindell and R. Kirkwood (Melbourne, VIC: CSIRO Publishing),208–225.
Krutzen, M., Barre, L., Möller, L. M., Heithaus, M. R., Simms, C., and Sherwin, W. B. (2002). A Biopsy System for small ceteceans: darting success and wound healing in Tursiops spp. Mar. Mamm. Sci. 18, 863–878. doi: 10.1111/j.1748-7692.2002.tb01078.x
Krutzen, M., and Sherwin, W. B. (2004). Population structure in an inshore cetacean revealed by microsatellite and mtDNA analysis: Bottlenose dolphins (Tursiops Sp.) in Shark Bay Western Australia. Mar. Mamm. Sci. 20, 28–47. doi: 10.1111/j.1748-7692.2004.tb01139.x
Lal, M. M., Southgate, P. C., Jerry, D. R., Bosserelle, C., and Zenger, K. R. (2017). Swept away: ocean currents and seascape features influence genetic structure across the 18,000 Km Indo-Pacific distribution of a marine invertebrate, the black-lip pearl oyster Pinctada margaritifera. BMC Genomics 18:66. doi: 10.1186/s12864-016-3410-y
Langmead, B., and Salzberg, S. L. (2012). Fast gapped-read alignment with Bowtie 2. Nat. Methods 9:357. doi: 10.1038/nmeth.1923
Leitwein, M., Duranton, M., Rougemont, Q., Gagnaire, P. A., and Bernatchez, L. (2020). Using haplotype information for conservation genomics. Trends Ecol. Evol. 35, 245–258. doi: 10.1016/j.tree.2019.10.012
Leslie, M. S., and Morin, P. A. (2016). Using genome-wide SNPs to detect structure in high-diversity and low-divergence populations of severely impacted Eastern Tropical Pacific Spinner (Stenella longirostris) and Pantropical Spotted Dolphins (S. attenuata). Front. Mar. Sci. 3:253. doi: 10.3389/fmars.2016.00253
Levins, R. (1969). Some demographic and genetic consequences of environmental heterogeneity for biological control. Bull. Entomol. Soc. Am. 15, 237–240. doi: 10.1093/besa/15.3.237
Louis, M., Viricel, A., Lucas, T., Peltier, H., Alfonsi, E., Berrow, S., et al. (2014). Habitat-driven population structure of bottlenose dolphins, Tursiops truncatus, in the North-East Atlantic. Mol. Ecol. 23, 857–874. doi: 10.1111/mec.12653
Luikart, G., England, P. R., Tallmon, D., Jordan, S., and Taberlet, P. (2003). The power and promise of population genomics: from genotyping to genome typing. Nat. Rev. Genet. 4, 981–994. doi: 10.1038/nrg1226
Lynch, T. P., Morello, E. B., Evans, K., Richardson, A. J., Rochester, W., Steinberg, C. R., et al. (2014). IMOS National Reference Stations: a continental-wide physical, chemical and biological coastal observing system. PLoS ONE 9:e113652. doi: 10.1371/journal.pone.0113652
MacArthur, R. H., and Wilson, E. O. (1967). The Theory of Island Biogeography. Princeton, NJ: Princeton University Press
Mackay, A. I., Goldsworthy, S. D., and Harrison, P. (2016). Critical Knowledge Gaps: Estimating Potential Maximum Cumulative Anthropogenic Mortality Limits of Key Marine Mammal Species to Inform Management in the Small Pelagic Fishery Area. Adelaide, SA: S.A.R.a.D.I.A. Sciences.
Manel, S., Loiseau, N., Andrello, M., Fietz, K., Goni, R., Forcada, A., et al. (2019). Long-distance benefits of marine reserves: myth or reality? Trends. Ecol. Evol. 34, 342–354. doi: 10.1016/j.tree.2019.01.002
Mantel, N. (1967). The detection of disease clustering and a generalized regression approach. Cancer Res. 27, 209–220.
Mason, S., Salgado Kent, C., Donnelly, D., Weir, J., and Bilgmann, K. (2016). Atypical residency of short-beaked common dolphins (Delphinus delphis) to a shallow, urbanized embayment in south-eastern Australia. R. Soc. Open Sci. 3:160478. doi: 10.1098/rsos.160478
Meirmans, P. G., and Van Tienderen, P. H. (2004). GENOTYPE and GENODIVE: two programs for the analysis of genetic diversity of asexual organisms. Mol. Ecol. Not. 4, 792–794. doi: 10.1111/j.1471-8286.2004.00770.x
Meissner, A. M., Christiansen, F., Martinez, E., Pawley, M. D., Orams, M. B., and Stockin, K. A. (2015). Behavioural effects of tourism on oceanic common dolphins, Delphinus sp., in New Zealand: the effects of Markov analysis variations and current tour operator compliance with regulations. PLoS ONE 10:e0116962. doi: 10.1371/journal.pone.0116962
Mendez, M., Rosenbaum, H. C., and Bordino, P. (2008). Conservation genetics of the franciscana dolphin in Northern Argentina: population structure, by-catch impacts, and management implications. Conserv. Genet. 9, 419–435. doi: 10.1007/s10592-007-9354-7
Meynier, L., Stockin, K. A., Bando, M. K. H., and Duignan, P. J. (2008). Stomach contents of common dolphin (Delphinus sp.) from New Zealand waters. N. Z. J. Mar. Freshw. Res. 42, 257–268. doi: 10.1080/00288330809509952
Mirimin, L., Westgate, A., Rogan, E., Rosel, P. E., Read, A., Coughlan, J., et al. (2009). Population structure of short-beaked common dolphins (Delphinus delphis) in the North Atlantic Ocean as revealed by mitochondrial and nuclear genetic markers. Mar. Biol. 156, 821–834. doi: 10.1007/s00227-008-1120-y
Möller, L., and Beheregaray, L. (2001). Coastal Bottlenose dolphins from Southeastern Australia are Tursiops Aduncus according to sequences of the mitochondrial DNA control region. Mar. Mamm. Sci. 17, 249–263. doi: 10.1111/j.1748-7692.2001.tb01269.x
Möller, L. M. (2011). Sociogenetic structure, kin associations and bonding in delphinids. Mol. Ecol. 21, 745–764. doi: 10.1111/j.1365-294X.2011.05405.x
Möller, L. M., and Beheregaray, L. B. (2004). Genetic evidence for sex-biased dispersal in resident bottlenose dolphins (Tursiops aduncus). Mol. Ecol. 13, 1607–1612. doi: 10.1111/j.1365-294X.2004.02137.x
Möller, L. M., Beheregaray, L. B., Harcourt, R. G., and Krutzen, M. (2001). Alliance membership and kinship in wild male bottlenose dolphins (Tursiops aduncus) of southeastern Australia. Proc. Biol. Sci. 268, 1941–1947. doi: 10.1098/rspb.2001.1756
Möller, L. M., Valdez, F. P., Allen, S., Bilgmann, K., Corrigan, S., and Beheregaray, L. B. (2011). Fine-scale genetic structure in short-beaked common dolphins (Delphinus delphis) along the East Australian Current. Mar. Biol. 158, 113–126. doi: 10.1007/s00227-010-1546-x
Möller, L. M., Wiszniewski, J., Allen, S., and Beheregaray, L. (2007). Habitat type promotes rapid and extremely localised genetic differentiation in dolphins. Mar. Freshw. Res. 58, 640–648. doi: 10.1071/MF06218
Moura, A. E., Natoli, A., Rogan, E., and Hoelzel, A. R. (2013). Atypical panmixia in a European dolphin species (Delphinus delphis): implications for the evolution of diversity across oceanic boundaries. J. Evol. Biol. 26, 63–75. doi: 10.1111/jeb.12032
MPI (2019). Aquatic Environment and Biodiversity Annual Review 2018. Wellington: Ministry for Primary Industries. Compiled by the Fisheries Science Team, Ministry for Primary Industries.
Natoli, A., Birkun, A., Aguilar, A., Lopez, A., and Hoelzel, A. R. (2005). Habitat structure and the dispersal of male and female bottlenose dolphins (Tursiops truncatus). Proc. Biol. Sci. 272, 1217–1226. doi: 10.1098/rspb.2005.3076
Natoli, A., Canadas, A., Peddemors, V. M., Aguilar, A., Vaquero, C., Fernandez-Piqueras, P., et al. (2006). Phylogeography and alpha taxonomy of the common dolphin (Delphinus sp.). J. Evol. Biol. 19, 943–954. doi: 10.1111/j.1420-9101.2005.01033.x
Natoli, A., Cañadas, A., Vaquero, C., Politi, E., Fernandez-Navarro, P., and Hoelzel, A. R. (2008). Conservation genetics of the short-beaked common dolphin (Delphinus delphis) in the Mediterranean Sea and in the eastern North Atlantic Ocean. Conserv. Biol. 9, 1479–1487. doi: 10.1007/s10592-007-9481-1
Neumann, D. R., and Orams, M. B. (2003). Feeding behaviours of short-beaked common dolphins, Delphinus delphis, in New Zealand. Aquat. Mamm. 29, 137–149. doi: 10.1578/016754203101023997
Nykanen, M., Dillane, E., Englund, A., Foote, A. D., Ingram, S. N., Louis, M., et al. (2018). Quantifying dispersal between marine protected areas by a highly mobile species, the bottlenose dolphin, Tursiops truncatus. Ecol. Evol. 8, 9241–9258. doi: 10.1002/ece3.4343
Oremus, M., Poole, M. D., and Baker, C. S. (2007). Isolation and interchange among insular spinner dolphin communities in the South Pacific revealed by individual identification and genetic diversity. Mar. Ecol. Prog. Ser. 336, 275–289. doi: 10.3354/meps336275
Paetkau, D., Slade, R., Burden, M., and Estoup, A. (2004). Genetic assignment methods for the direct, real-time estimation of migration rate: a simulation-based exploration of accuracy and power. Mol. Ecol. 13, 55–65. doi: 10.1046/j.1365-294X.2003.02008.x
Papa, Y., Oosting, T., Valenza-Troubat, N., Wellenreuther, M., and Ritchie, P. A. (2020). Genetic stock structure of New Zealand fish and the use of genomics in fisheries management: an overview and outlook. N. Z. J. Zool. 1–31. doi: 10.1080/03014223.2020.1788612
Parra, G. J., Bilgman, K., Peter, K. J., and Möller, L. M. (in review). Abundance potential biological removal of common dolphins subject to fishery-impacts in South Australian Waters. Front. Mar. Sci.
Parra, G. J., Cagnazzi, D., Jedensjö, M., Ackermann, C., Frere, C., Seddon, J., et al. (2018). Low genetic diversity, limited gene flow and widespread genetic bottleneck effects in a threatened dolphin species, the Australian humpback dolphin. Biol. Conserv. 220, 192–200. doi: 10.1016/j.biocon.2017.12.028
Patterson, H., Williams, A., Woodhams, J., and Curtotti, R. (2019). Fishery Status Reports 2019. Canberra, ACT: Australian Bureau of Agricultural and Resource Economics and Sciences (ABARES).
Pawley, M. D. M., Hupman, K. E., Stockin, K. A., and Gilman, A. (2018). Examining the viability of dorsal fin pigmentation for individual identification of poorly-marked delphinids. Sci. Rep. 8:12593. doi: 10.1038/s41598-018-30842-7
Perry, G. L. W., and Lee, F. (2019). How does temporal variation in habitat connectivity influence metapopulation dynamics? Oikos. 128, 1277–1286. doi: 10.1111/oik.06052
Peters, K. J., Bury, S. J., Betty, E. L., Parra, G. J., Tezanos-Pinto, G., and Stockin, K. A. (2020). Foraging ecology of the common dolphin Delphinus delphis revealed by stable isotope analysis. Mar Ecol Prog Ser 652:173–86. doi: 10.3354/meps13482
Peters, K. J., and Stockin, K. A. (2021). Cetacean sighting records in the New Caledonia Basin, Tasman Sea, New Zealand. N. Z. J. Mar. Freshw. Res. 1–15. doi: 10.1080/00288330.2020.1867201
Peterson, B. K., Weber, J. N., Kay, E. H., Fisher, H. S., and Hoekstra, H. E. (2012). Double digest RADseq: an inexpensive method for de novo SNP discovery and genotyping in model and non-model species. PLoS ONE 7:e37135. doi: 10.1371/journal.pone.0037135
Pierre, J. (2019). Associated Species Marine Mammals. Ministry for Primary Industries and Department of Conservations. Available online at: openseas.org.nz/wp/content/uploads/2019/05/SDRMarineMammals2019.pdf
Piry, S., Alapetite, A., Cornuet, J. M., Paetkau, D., Baudouin, L., and Estoup, A. (2004). GENECLASS2: a software for genetic assignment and first-generation migrant detection. J. Hered. 95, 536–539. doi: 10.1093/jhered/esh074
Pitt, K. A., and Kingsford, M. J. (2000). Geographic separation of stocks of the edible jellyfish Catostylus mosaicus (Rhizostomeae) in New South Wales, Australia. Mar. Ecol. Prog. Ser. 196, 143–155. doi: 10.3354/meps196143
Pratt, E. A. L., Beheregaray, L. B., Bilgmann, K., Zanardo, N., Diaz-Aguirre, F., and Möller, L. M. (2018). Hierarchical metapopulation structure in a highly mobile marine predator: the southern Australian coastal bottlenose dolphin (Tursiops cf. australis). Conserv. Genet. 19, 637–654. doi: 10.1007/s10592-017-1043-6
Puritz, J. B., Hollenbeck, C. M., and Gold, J. R. (2014). dDocent: a RADseq, variant-calling pipeline designed for population genomics of non-model organisms. PeerJ 2:e431. doi: 10.7717/peerj.431
Quérouil, S., Silva, M. A., Freitas, L., Prieto, R., Magalhães, S., Dinis, A., et al. (2007). High gene flow in oceanic bottlenose dolphins (Tursiops truncatus) of the North Atlantic. Conserv. Genet. 8, 1405–1419. doi: 10.1007/s10592-007-9291-5
R Development Core Team (2018). R: A Language and Environment for Statistical Computing. Vienna: R Foundation for Statistical Computing.
Rambaut, A., Drummond, A. J., Xie, D., Baele, G., and Suchard, M. A. (2018). Posterior Summarization in Bayesian Phylogenetics Using Tracer 1.7. Syst. Biol. 67, 901–904. doi: 10.1093/sysbio/syy032
Rannala, B., and Mountain, J. (1997). Detecting immigration by using multilocus genotypes. Proc. Natl. Acad. Sci. U.S.A. 94, 9197–9201.
Riginos, C., Crandall, E. D., Liggins, L., Bongaerts, P., and Treml, E. A. (2016). Navigating the currents of seascape genomics: how spatial analyses can augment population genomic studies. Curr. Zool. 62, 581–601. doi: 10.1093/cz/zow067
Riginos, C., Hock, K., Matias, A. M., Mumby, P. J., Oppen, M. J. H., and Lukoschek, V. (2019). Asymmetric dispersal is a critical element of concordance between biophysical dispersal models and spatial genetic structure in Great Barrier Reef corals. Divers. Distrib. 25, 1684–1696. doi: 10.1111/ddi.12969
Rosel, P. E., Taylor, B. L., Hancock-Hanser, B. L., Morin, P. A., Archer, F. I., Lang, A. R., et al. (2017). A review of molecular genetic markers and analytical approaches that have been used for delimiting marine mammal subspecies and species. Mar. Mamm. Sci. 33, 56–75. doi: 10.1111/mms.12412
Ross, P. M., Hogg, I. D., Pilditch, C. A., and Lundquist, C. J. (2009). Phylogeography of New Zealand's coastal benthos. N. Z. J. Mar. Freshw. Res. 43, 1009–1027. doi: 10.1080/00288330.2009.9626525
Rowe, S. J. (2007). A Review of Methodologies for Mitigating Incidental Catch of Protected Marine Mammals. Wellington: Science and Technical Publishing Department of Conservation.
Sandoval-Castillo, J., Robinson, N. A., Hart, A. M., Strain, L. W. S., and Beheregaray, L. B. (2018). Seascape genomics reveals adaptive divergence in a connected and commercially important mollusc, the greenlip abalone (Haliotis laevigata), along a longitudinal environmental gradient. Mol. Ecol. 27, 1603–1620. doi: 10.1111/mec.14526
Selkoe, K. A., D'Aloia, C. C., Crandall, E. D., Iacchei, M., Liggins, L., Puritz, J. B., et al. (2016). A decade of seascape genetics: contributions to basic and applied marine connectivity. Mar. Ecol. Prog. Ser. 554, 1–19. doi: 10.3354/meps11792
Shafer, A. B., Wolf, J. B., Alves, P. C., Bergstrom, L., Bruford, M. W., Brannstrom, I., et al. (2015). Genomics and the challenging translation into conservation practice. Trends Ecol. Evol. 30, 78–87. doi: 10.1016/j.tree.2014.11.009
Sousa, A., Alves, F., Dinis, A., Bentz, J., Cruz, M. J., and Nunes, J. P. (2019). How vulnerable are cetaceans to climate change? Developing and testing a new index. Ecol. Indic. 98, 9–18. doi: 10.1016/j.ecolind.2018.10.046
Stockin, K. A., Amaral, A. R., Latimer, J., Lambert, D. M., and Natoli, A. (2014). Population genetic structure and taxonomy of the common dolphin (Delphinus sp.) at its southernmost range limit: New Zealand waters. Mar. Mamm. Sci. 30, 44–63. doi: 10.1111/mms.12027
Stockin, K. A., Binedell, V., Wiseman, N., Brunton, D. H., and Orams, M. B. (2009a). Behavior of free-ranging common dolphins (Delphinus sp.) in the Hauraki Gulf, New Zealand. Mar. Mamm. Sci. 25, 283–301. doi: 10.1111/j.1748-7692.2008.00262.x
Stockin, K. A., Duignan, P. J., Alley, M., Roe, W. D., Meynier, L., and Fettermann, T. (2009b). Causes of mortality in stranded Common Dolphin (Delphinus sp.) from New Zealand waters between 1998 and 2008. Pac. Conserv. Biol. 15, 217–227. doi: 10.1071/PC090217
Stockin, K. A., Pierce, G. J., Binedell, V., Wiseman, N., and Orams, M. B. (2008). Factors Affecting the Occurrence and Demographics of Common Dolphins (Delphinus sp.) in the Hauraki Gulf, New Zealand. Aquat. Mamm. 34, 200–211. doi: 10.1578/am.34.2.2008.200
Sunnucks, P., and Hales, D. F. (1996). Numerous transposed sequences of mitochondrial cytochrome oxidase I-II in aphids of the Genus Sitobion (Hemiptera: Aphididae). Mol. Biol. Evol. 13, 510–524.
Suthers, I. M., Young, J. W., Baird, M. E., Roughan, M., Everett, J. D., Brassington, G. B., et al. (2011). The strengthening East Australian Current, its eddies and biological effects — an introduction and overview. Deep Sea Res. Part II Top. Stud. Oceanogr. 58, 538–546. doi: 10.1016/j.dsr2.2010.09.029
Taft, H. R., McCoskey, D. N., Miller, J. M., Pearson, S. K., Coleman, M. A., Fletcher, N. K., et al. (2020). Research–management partnerships: an opportunity to integrate genetics in conservation actions. Conserv. Sci. Pract. 2:e218. doi: 10.1111/csp2.218
Taylor, B. L., Archer, F. I., Martien, K. K., Rosel, P. E., Hancock-Hanser, B. L., Lang, A. R., et al. (2017). Guidelines and quantitative standards to improve consistency in cetacean subspecies and species delimitation relying on molecular genetic data. Mar. Mamm. Sci. 33, 132–155. doi: 10.1111/mms.12411
Taylor, B. L., Chivers, S. J., Larese, J., and Perrin, W. F. (2007). Generation Length and Percent Mature Estimates for IUCN Assessments of Cetaceans. La Jolla, CA: Southwest Fisheries Science Center; Southwest Fisheries Science Center Administrative Report, 24.
Teske, P. R., Golla, T. R., Sandoval-Castillo, J., Emami-Khoyi, A., van der Lingen, C. D., von der Heyden, S., et al. (2018). Mitochondrial DNA is unsuitable to test for isolation by distance. Sci. Rep. 8:8448. doi: 10.1038/s41598-018-25138-9
Teske, P. R., Sandoval-Castillo, J., van Sebille, E., Waters, J., and Beheregaray, L. B. (2015). On-shelf larval retention limits population connectivity in a coastal broadcast spawner. Mar. Ecol. Prog. Ser. 532, 1–12. doi: 10.3354/meps11362
Teske, P. R., Sandoval-Castillo, J., Waters, J., and Beheregaray, L. B. (2017). An overview of Australia's temperate marine phylogeography, with new evidence from high-dispersal gastropods. J. Biogeogr. 44, 217–229. doi: 10.1111/jbi.12783
Tezanos-Pinto, G., Baker, C. S., Russell, K., Martien, K., Baird, R. W., Hutt, A., et al. (2009). A worldwide perspective on the population structure and genetic diversity of bottlenose dolphins (Tursiops truncatus) in New Zealand. J. Hered. 100, 11–24. doi: 10.1093/jhered/esn039
Thompson, F. N., Abraham, E. R., and Berkenbusch, K. (2013). Common dolphin (Delphinus delphis) bycatch in New Zealand commercial trawl fisheries. PLoS ONE 8:e64438. doi: 10.1371/journal.pone.0064438
Tulloch, V., Pirotta, V., Grech, A., Crocetti, S., Double, M., How, J., et al. (2020). Long-term trends and a risk analysis of cetacean entanglements and bycatch in fisheries gear in Australian waters. Biodivers. Conserv. 29, 251–282. doi: 10.1007/s10531-019-01881-x
Wang, J. (2007). Triadic IBD coefficients and applications to estimating pairwise relatedness. Genet. Res. 89, 135–153. doi: 10.1017/S0016672307008798
Wang, J. (2011). COANCESTRY: a program for simulating, estimating and analysing relatedness and inbreeding coefficients. Mol. Ecol. Resour. 11, 141–145. doi: 10.1111/j.1755-0998.2010.02885.x
Waples, R. S., and Gaggiotti, O. (2006). What is a population? An empirical evaluation of some genetic methods for identifying the number of gene pools and their degree of connectivity. Mol. Ecol. 15, 1419–1439. doi: 10.1111/j.1365-294X.2006.02890.x
Ward, T. M., and Grammer, G. L. (2018). Commonwealth Small Pelagic Fishery: Fishery Assessment Report 2017. Report to the Australian Fisheries Management Authority. South Australian Research and Development Institute (Aquatic Sciences). SARDI Publication No. F2010/000270-9. SARDI Research Report Series No. 982, 114.
Ward, T. M., Smart, J., and Ivey, A. (2017). Stock assessment of Australian Sardine (Sardinops sagax) Off South Australia 2017. Report to PIRSA Fisheries and Aquaculture. South Australian Research and Development Institute (Aquatic Sciences). SARDI Publication No. F2007/000765-6. SARDI Research Report Series No. 971, 107.
Waters, J. M. (2008). Marine biogeographical disjunction in temperate Australia: historical landbridge, contemporary currents, or both? Divers. Distrib. 14, 692–700. doi: 10.1111/j.1472-4642.2008.00481.x
Waters, J. M., Emerson, B. C., Arribas, P., and McCulloch, G. A. (2020). Dispersal reduction: causes, genomic mechanisms, and evolutionary consequences. Trends Ecol. Evol. 35, 512–522. doi: 10.1016/j.tree.2020.01.012
Waters, J. M., Wernberg, T., Connell, S. D., Thomsen, M. S., Zuccarello, G. C., Kraft, G. T., et al. (2010). Australia's marine biogeography revisited: back to the future? Austral Ecol. 35, 988–992. doi: 10.1111/j.1442-9993.2010.02114.x
Weir, B. S., and Cockerham, C. C. (1984). Estimating F-statistics for the analysis of population structure. Evolution 38, 1358–1370
Whitehead, H., McGill, B., and Worm, B. (2008). Diversity of deep-water cetaceans in relation to temperature: implications for ocean warming. Ecol. Lett. 11, 1198–1207. doi: 10.1111/j.1461-0248.2008.01234.x
Wilson, G. A., and Rannala, B. (2003). Bayesian inference of recent migration rates using multilocus genotypes. Genetics 163, 1177–1191.
Wiszniewski, J., Beheregaray, L. B., Allen, S. J., and Möller, L. M. (2009). Environmental and social influences on the genetic structure of bottlenose dolphins (Tursiops aduncus) in Southeastern Australia. Conserv. Genet. 11, 1405–1419. doi: 10.1007/s10592-009-9968-z
Xuereb, A., Benestan, L., Normandeau, E., Daigle, R. M., Curtis, J. M. R., Bernatchez, L., et al. (2018). Asymmetric oceanographic processes mediate connectivity and population genetic structure, as revealed by RADseq, in a highly dispersive marine invertebrate (Parastichopus californicus). Mol. Ecol. 27, 2347–2364. doi: 10.1111/mec.14589
York, K. L., Blacket, M. J., and Appleton, B. R. (2008). The Bassian Isthmus and the major ocean currents of southeast Australia influence the phylogeography and population structure of a southern Australian intertidal barnacle Catomerus polymerus (Darwin). Mol. Ecol. 17, 1948–1961. doi: 10.1111/j.1365-294X.2008.03735.x
Zanardo, N., Bilgmann, K., Parra, G. J., and Möller, L. M. (2016). Socio-genetic structure of short-beaked common dolphins in southern Australia. J. Zool. 299, 89–97. doi: 10.1111/jzo.12330
Keywords: delphinids, fisheries genomics, isolation-by-distance, migration, gene flow, metapopulation, conservation genomics
Citation: Barceló A, Sandoval-Castillo J, Stockin KA, Bilgmann K, Attard CRM, Zanardo N, Parra GJ, Hupman K, Reeves IM, Betty EL, Tezanos-Pinto G, Beheregaray LB and Möller LM (2021) A Matter of Scale: Population Genomic Structure and Connectivity of Fisheries At-Risk Common Dolphins (Delphinus delphis) From Australasia. Front. Mar. Sci. 8:616673. doi: 10.3389/fmars.2021.616673
Received: 12 October 2020; Accepted: 14 January 2021;
Published: 16 February 2021.
Edited by:
Michael Paul Jensen, Southwest Fisheries Science Center (NOAA), United StatesReviewed by:
Phillip A. Morin, Southwest Fisheries Science Center (NOAA), United StatesCarlos Carreras, University of Barcelona, Spain
Copyright © 2021 Barceló, Sandoval-Castillo, Stockin, Bilgmann, Attard, Zanardo, Parra, Hupman, Reeves, Betty, Tezanos-Pinto, Beheregaray and Möller. This is an open-access article distributed under the terms of the Creative Commons Attribution License (CC BY). The use, distribution or reproduction in other forums is permitted, provided the original author(s) and the copyright owner(s) are credited and that the original publication in this journal is cited, in accordance with accepted academic practice. No use, distribution or reproduction is permitted which does not comply with these terms.
*Correspondence: Andrea Barceló, YW5kcmVhLmJhcmNlbG9AZmxpbmRlcnMuZWR1LmF1
†ORCID: Catherine R. M. Attard orcid.org/0000-0003-1157-570X