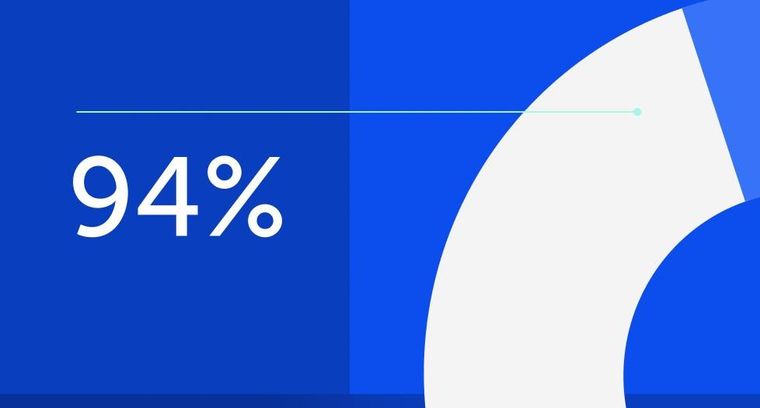
94% of researchers rate our articles as excellent or good
Learn more about the work of our research integrity team to safeguard the quality of each article we publish.
Find out more
ORIGINAL RESEARCH article
Front. Mar. Sci., 22 June 2021
Sec. Coral Reef Research
Volume 8 - 2021 | https://doi.org/10.3389/fmars.2021.615795
This article is part of the Research TopicVariance Matters: Individual Differences and Their Consequences for Natural Selection Within and Among Coral HolobiontsView all 10 articles
The Florida Keys reef tract (FKRT) has a unique geological history wherein Holocene sea-level rise and bathymetry interacted, resulting in a reef-building system with notable spatial differences in reef development. Overprinted on this geologic history, recent global and local stressors have led to degraded reefs dominated by fleshy algae, soft corals, and sponges. Here, we assessed how coral physiology (calcification rate, tissue thickness, reproduction, symbiosis, and bleaching) varies seasonally (winter vs. summer) and geographically using 40 colonies of the mustard hill coral Porites astreoides from four sites across 350 km along the FKRT from 2015 to 2017. The study coincided with a high-temperature event in late summer 2015 that caused heterogeneous levels of coral bleaching across sites. Bleaching severity differed by site, with bleaching response more aligned with heat stress retroactively calculated from local degree heating weeks than those predicted by satellites. Despite differences in temperature profiles and bleaching severity, all colonies hosted Symbiodiniaceae of the same genus (formerly Clade A and subtypes). Overall, P. astreoides at Dry Tortugas National Park, the consistently coolest site, had the highest calcification rates, symbiont cell densities, and reproductive potential (all colonies were reproductive, with most planula larvae per polyp). Corals at Dry Tortugas and Fowey Rocks Light demonstrated strong seasonality in net calcification (higher in summer) and did not express visual or partial-mortality responses from the bleaching event; in contrast, colonies in the middle and southern part of the upper keys, Sombrero Key and Crocker Reef, demonstrated similar reduced fitness from bleaching, but differential recovery trajectories following the heat stress. Identifying reefs, such as Dry Tortugas and possibly Fowey Rocks Light that may serve as heat-stress refugia, is important in selecting candidate sites for adaptive reef-management strategies, such as selective propagation and assisted gene flow, to increase coral-species adaptation to ocean warming.
Coral reefs have declined in live-coral cover by 50–80% over the last four decades from the culminating impacts of local and global stressors (Gardner et al., 2005; De’ath et al., 2012; Hughes et al., 2018), putting at risk the vast ecological goods and services that coral reefs provide such as coastal protection, fisheries, and tourism (Moberg and Folke, 1999; Pratchett et al., 2014; Storlazzi et al., 2019). Ocean warming is identified as the greatest threat to coral reefs (Intergovernmental Panel on Climate Change (IPCC), 2018), particularly because of recent severe and repeated marine heatwaves resulting in widespread coral bleaching (Eakin et al., 2019), exacerbated disease outbreaks (Randall and van Woesik, 2015; Precht et al., 2016), and increasingly severe storm events (Gardner et al., 2005; Webster et al., 2005), with little reprieve between disturbances (Hughes et al., 2003, 2018). Coral reef communities in the Caribbean and Florida Keys have already shifted from three-dimensional, reef-building structures to flattened reefs dominated by soft-bodied benthic dwellers (Alvarez-Filip et al., 2009; Norström et al., 2009; Ruzicka et al., 2013; Lenz et al., 2015). These structurally degraded ecosystems leave coastlines, islands, and their communities vulnerable to erosion and inundation, especially as hurricanes (Webster et al., 2005), sea-level rise, and wave stress intensify (Storlazzi et al., 2019). Identifying sites where corals maintain biological performance that supports reef building, coral-population recovery, and ecological resilience is critical as local and global stressors continue to impact coral reef ecosystems (Beyer et al., 2018; Guest et al., 2018; Darling et al., 2019).
Exceptional reefs that stand out against the current backdrop of degradation can greatly influence how sites are prioritized in conservation and management strategies (Beyer et al., 2018; Guest et al., 2018; Hoegh-Guldberg et al., 2018). Some sites and regions have been described as “reefs of hope” (McClanahan et al., 2009), “bright spots” (Cinner et al., 2016), and “reef oases” (Guest et al., 2018). These reefs were identified based on percent coral cover, reef biodiversity, or fish assemblages as being influenced by factors including location, socioeconomic status, and environmental and biological traits (Cinner et al., 2016; Beyer et al., 2018; Guest et al., 2018; Darling et al., 2019). Although metrics on percent coral cover and reef assemblages are readily available to compare sites, there is a general lack of information on the critical biological processes that promote coral recovery and resilience. Identifying locations where in situ coral physiology outperforms that at other sites could further help prioritize reef management, protection, and restoration strategies (Beyer et al., 2018; Guest et al., 2018; Darling et al., 2019).
The Florida Keys reef tract (FKRT) consists of a unique, subtropical and largely submerged spur-and-groove and patch-reef system that stretches 350 km approximately 6–10 km from shores of the Florida Keys islands (Stephenson and Stephenson, 1950). Based on geological characteristics, the reef system is traditionally divided into subregions including the upper, middle, and lower Keys, and the Marquesas/Dry Tortugas (Murdoch and Aronson, 1999; Lidz et al., 2006; Toth et al., 2017, 2018) featuring strong spatial and temporal variability in reef development (Lidz and Shinn, 1991; Precht and Aronson, 2004; Lidz et al., 2006; Toth et al., 2018). Mean percent coral cover throughout the FKRT is now less than 5% (Ruzicka et al., 2013) and current spatial patterns in coral net calcification rates along the FKRT correlate with average Holocene reef thickness with the greatest suppression in reef development between the middle and lower Keys (Kuffner et al., 2013; Manzello et al., 2015; Toth et al., 2018). The suppression of reef development in the middle Keys may be driven by unfavorable environmental conditions of water traveling out of tidal passes, connecting the offshore reefs to Florida Bay and bringing large fluctuations in temperature, salinity, turbidity, and nutrients; this theory is known as the “inimical waters hypothesis” (Schlager, 1981; Neumann and Macintyre, 1985; Hallock and Schlager, 1986; Ginsburg and Shinn, 1994; Smith, 2002; Precht and Miller, 2007).
In recent decades, in situ sea temperatures throughout the FKRT have increased, with mean maximum daily temperatures exceeding 30.5°C at least once every year since 1994 (Kuffner et al., 2015; Manzello, 2015). As thermal stress along the FKRT persists on a near-annual basis, it is important to understand the in situ response of coral physiology (i.e., calcification, coral-algal symbiosis, reproduction, and survivorship); in particular, how traits vary in response to differences in water-residence time, bathymetry, proximity to tidal passes, and other physical or environmental gradients along the FKRT. Given the environmental history of the FKRT and recent thermal-stress events, the goal of our 2-year study was to quantify differences in coral response to temperature stress that may be driven by reef location. Using four, previously established sites where coral-calcification assessment has occurred since 2009 (Kuffner et al., 2013, 2019), we assessed coral colony-level traits of 40 Porites astreoides colonies followed from May 2015 to May 2017 across the length of the FKRT from Miami to the Dry Tortugas. We compared calcification rates (e.g., Kuffner et al., 2013; Manzello et al., 2015), tissue thickness, reproductive potential (Chornesky and Peters, 1987; Serrano et al., 2016), and Symbiodiniaceae concentration, identity, and diversity (Kenkel et al., 2013; Hauff et al., 2016; Cunning et al., 2017) as proxies for coral fitness that reveal information about resistance and resilience against heat stress. Few studies have simultaneously measured these essential physiological traits in the field to determine possible trade-offs as corals experience the natural spatial and temporal variability of environmental conditions along the FKRT (Manzello et al., 2018). We provide evidence based on our colony-level traits that corals at specific sites (Biscayne and Dry Tortugas National Parks) maintained physiological function despite the severe thermal anomaly while corals in the middle Keys and southern part of the upper Keys were more vulnerable, further supporting the inimical waters hypothesis.
Four sites spanning the ∼350-km Florida Keys reef tract (FKRT) (Figure 1A) were established for the calcification-assessment network by the United States Geological Survey (USGS) Coral Reef Ecosystems Studies (CREST) project in 2009 (Kuffner et al., 2013). Sites were chosen because of historical oceanographic data collected by the Sustained Ecological Research Related to Management of the Florida Keys Seascape (SEAKEYS) program (Ogden et al., 1994), and meteorological data from the National Data Buoy Center (NDBC) C-MAN stations (Kuffner et al., 2013). The study sites are located at Pulaski Shoal Light (Pulaski, 24.694°N 82.773°W) in Dry Tortugas National Park, Sombrero Key Light (Sombrero, 24.627°N 81.109°W) and Crocker Reef (Crocker, 24.563°N 80.527°W) in the Florida Keys National Marine Sanctuary (FKNMS), and Fowey Rocks Light (Fowey, 25.591°N 80.096°W) in Biscayne National Park (Figure 1A and Supplementary Table 1). All sites are in 3–5 m water depth on the outer reef in low to moderate relief reef-crest habitat. Fowey, Crocker, and Sombrero are 5–10 km off the coast of the Florida Keys island chain, and Pulaski is about 100 km west of the city of Key West, FL.
Figure 1. (A) Map of sites established by the U.S. Geological Survey for the calcification assessment network along the Florida Key Reef Tract, with Pulaski Shoal Light (green) in Dry Tortugas National Park, Sombrero Key Light (orange) in the middle Keys, Crocker Reef (yellow) in the southern upper Keys, and Fowey Rocks Light (blue) in the northern upper Keys in Biscayne National Park. (B) Underwater photograph (image by I.B. Kuffner) of one of the 40 calcification stations with a Poritesastreoides colony fastened to the top. The cement block is 19 × 19 × 19 cm for scale. (C) Mean daily temperature (top, left axis) at each site with a red dashed line indicating the 29.6_C maximum monthly mean in the Florida Keys (Manzello et al., 2015) and degree heating weeks calculated using climatology specific to each respective site (bottom, right axis).
The mustard hill coral, Porites astreoides (de Lamarck, 1816), was chosen for this study because of its abundance throughout the FKRT, year-round reproductive strategy with peak larval release in late April and May (Chornesky and Peters, 1987), and its potential as a bioindicator of thermal stress (Kenkel et al., 2013). P. astreoides is a fast-growing “weedy” species common throughout the Caribbean in shallow and deep reefs with higher relative abundance compared to other coral species (Green et al., 2008; Darling et al., 2012). Colonies in shallow reefs are commonly dominated by Symbiodinium spp. (formerly Clade A; LaJeunesse et al., 2018), particularly “A4/A4a” (Thornhill et al., 2006; Kenkel et al., 2013; Serrano et al., 2016; Cunning et al., 2018).
At the previously established sites (Kuffner et al., 2019), concrete cinder-block stations (19 × 19 × 19 cm, n = 10 per site), placed 2–4 m apart from one another and fastened to the benthos with two 15 cm stainless-steel threaded rods drilled and epoxied into the substratum (Morrison et al., 2013), were used to monitor coral survival and growth for 2 years. Each block was designed to hold a single coral colony that was epoxied to a polyvinyl chloride (PVC) disc that can be reversibly bolted to the top of the cinder block using a wingnut (Figure 1B). In April 2015, 10 P. astreoides colonies of the yellow/green color morph measuring 4–6 cm in diameter were collected from each site at 3 to 5 m water depth within ∼200 m using a hammer and chisel to remove colonies from the substratum. A total of 40 colonies were used in the study. Care was taken to collect colonies no closer than 20 m apart to minimize the chance of collecting genetic clones (Baums et al., 2019). Corals were epoxied onto the PVC discs, weighed, measured, photographed and deployed to the cinder-block stations.
Seawater temperature was recorded every 15 min (n = 96 per day) with HOBO Water Temp Prov2 temperature loggers (Onset, Pocasset, MA, United States ± 0.2°C) attached to two of the cinder-block stations at each site. All temperature data are publicly available (Kuffner, 2016). Mean daily temperature, mean monthly maximum, and mean monthly minimum were used to characterize sites. Degree heating weeks (DHW) for each site during this study were calculated using in situ temperature data and were also compared to the National Oceanic and Atmospheric Administration (NOAA) Coral Reef Watch Satellite Regional Stations in the Florida Keys based on local climatology (Wyatt et al., 2020). To characterize the light environments at each site, monthly mean (± SD) photosynthetically active radiation (PAR) data were collected from satellite-derived water-quality data products for the Florida Keys made publicly available through the Optical Oceanography Laboratory1 using the virtual buoys closest to the sites (Pulaski = FK01, 24.693N 82.773°W; Sombrero = FK07, 24.628°N 81.112°W; Crocker = FK08, 24.816°N 80.676°W; Fowey = FK11, 25.591°N 80.096°W). Daily precipitation was used as a proxy for salinity and was acquired from the NOAA Tropical Rainfall Measuring Mission from the Environmental Research Division’s Data Access Program (ERDDAP) that corresponded closest to the sites and summarized by month2.
Every 6 months during the 2-year study (Supplementary Table 1), the 40 colonies of P. astreoides were retrieved from the field to measure seasonal changes in coral condition and calcification rates. Colonies were brought to shore in coolers or 5-gallon buckets filled with ambient seawater, buoyant weighed (Jokiel et al., 1978), measured with calipers, photographed, and returned to the study site within 6 hrs. The difference in buoyant weight for each colony at each time point was converted to dry mass using 1.02 g cm–3 as seawater density and 2.93 g cm–3 as aragonite density of the coral skeleton (Jokiel et al., 1978). The rate of coral calcification was calculated using the number of days between each measured interval and normalized to the initial planar-area footprint of the coral colony (using formula for the area of an ellipse; Kuffner et al., 2013). Photographs of each colony were captured on land and were white-balance corrected with the same white, gridded-photography background in each image to assess colony pigmentation over time based on a condition score of (1): no bleaching, (2): paling to normal, (3): 1–50% bleached, (4): 51–99% bleached, (5): 100% bleached, and (6): dead (Baird et al., 2018).
At the end of the study in April 2017, coral colonies were sampled to compare coral-tissue thickness, reproduction (i.e., gametogenesis and planulation), symbiont cell densities, and the composition of Symbiodiniaceae taxa across sites. Using a masonry-tile saw, two 4-mm-wide sagittal sections were taken from the center axis of the colony with the saw wetted with ambient seawater. Slices from each colony were photographed and archived. An additional 4 cm × 2 cm section was taken from the center of the colony and processed as described in Chornesky and Peters (1987) for histology to measure tissue thickness (Loya et al., 2001) and reproductive potential. If the center of the colony had died, a section was taken from the colony side. The tissue sample was placed in buffered zinc formalin fixative (Z-fix, Anatech Ltd.) diluted in filtered seawater (1:4) (Padilla-Gamiño et al., 2014) for at least 48 h and then transferred into 70% ethanol (diluted in distilled water) until processed by Histotech Ltd. (Cleveland, OH, United States) where samples were decalcified in diluted HCl and EDTA. The 4–6 μm thick sagittal sections along the tissue and cross sections of the top, middle, and bottom thirds were mounted on slides and stained with hematoxylin and eosin (Chornesky and Peters, 1987). One of the slices was used to examine tissue thickness using microscopic images and the FIJI package of the opensource image software ImageJ (Schneider et al., 2012). Each slice mounted on a microscopic slide consisted of approximately 5–20 polyps. The number of oocytes, spermatocytes, and planula larvae per polyp were counted in ten haphazardly chosen polyps of each colony.
For Symbiodiniaceae, symbiont cell densities were first measured by taking a 4 cm × 2 cm section from the third sagittal slice and removing the tissue from the skeleton using an airbrush filled with filtered seawater (0.1 μm). The tissue slurry was homogenized, and 1 mL was aliquoted into a buffered zinc formalin fixative to later count symbiont cell densities, with n = 10 samples per colony, using a hemocytometer (n = 10 per site, n = 8 at Sombrero). Cell densities were normalized to skeletal surface area (cm2) determined by the aluminum foil wrapping technique (Marsh, 1970). Second, a sterilized razor was used to remove approximately 5 mm2 of coral tissue and placed in 1% sodium dodecyl sulfate DNA buffer and shipped to the Hawai‘i Institute of Marine Biology (HIMB) where genomic DNA was extracted and isolated using a cetyl trimethylammonium bromide protocol3. The amplification of the ribosomal Internal Transcribed Spacer 2 (ITS2) region, a multicopy genetic marker, was used to identify Symbiodiniaceae diversity with next-generation amplicon sequencing (NGS) (LaJeunesse, 2001; Thornhill et al., 2007, 2014). DNA samples were sent to the HIMB Genetics Core Facility for library preparation using “itsD” and “its2rev2” primers (Stat et al., 2009) and sequenced on an Illumina MiSeq platform with 2 × 300 paired-end read chemistry. The paired forward and reverse reads from each sample (provided in fastq.gz files outputted from the Illumina sequencing) were submitted directly to SymPortal4. The SymPortal analytical framework is designed to resolve genetically differentiated taxa of Symbiodiniaceae (formerly Symbiodinium spp. LaJeunesse et al., 2018) using NGS data of the ITS2 amplicon and its high intragenomic diversity by identifying defining intragenomic ITS2 sequence variants (DIVs) as specific profile types (Hume et al., 2019).
Environmental parameters including temperature, light, and precipitation were compared across the four FKRT calcification network sites using a linear mixed-effects model with lmer in package lme4 (Bates et al., 2015), site as a fixed effect, and date as a random effect. For the biological response variables measured over time (calcification rates, tissue loss and colony-condition scores), univariate analyses were conducted to assess interactive effects of time interval (Summer 2015, Winter 2016, Summer 2016, and Winter 2017) and site (Pulaski, Sombrero, Crocker, and Fowey). Specifically, calcification rates and colony condition scores were analyzed with linear mixed effect models in lme4 with site and time interval as fixed effects and coral colony as a random effect. ANOVA tables for all environmental and biological metrics were generated using Type II sum of squares Satterthwaite approximation of degrees of freedom using lmerTest (Kuznetsova et al., 2017). Percent tissue loss was analyzed using glmmTMB for a zero-inflated mixed model with the glmmTMB package (Brooks et al., 2017) with the same fixed and random effects. Anova in the package car (Fox et al., 2013) was used to generate a Type II Wald Chi-square test of the zero-inflated mixed model. Post hoc Tukey tests were conducted using the package emmeans (Lenth, 2018). Assumptions of analysis of variance were confirmed of all models using graphical inspection of the residuals with the DHARMa package (Hartig, 2017) and the simulateResiduals command.
The coral-level biological responses (tissue loss, tissue thickness, fecundity, spermatocytes, symbiont cell density, calcification rate, and condition score) for P. astreoides were analyzed using a permutational multivariate analysis of variance (PERMANOVA) with 999 model permutations using adonis2 in the vegan package (Oksanen et al., 2007). First, the three response variables were assessed by site and time interval as main effects and second, all response variables measured in the final time interval. Principal components analysis (PCA) was used to visualize the multivariate relationship of the response variables by sites for each time interval separately with the factoextra package (Kassambara and Mundt, 2017).
Of the environmental data available for each site, only in situ temperature revealed site-level differences (Figure 1C). Mean daily temperature measured throughout the study significantly differed across sites (Figure 1C; P < 0.001) except for mean daily temperatures between the Crocker and Sombrero reef sites in the middle and lower Keys, respectively (P = 0.247). There were season-specific differences in mean temperature across site (P < 0.001), except in Summer 2015 between Pulaski and Crocker (Tukey Post hoc, P = 0.998) and between Sombrero and both Crocker and Fowey (Tukey Post hoc, P ≥ 0.468). During the height of the coral bleaching event in August 2015 (Manzello et al., 2018), the maximum mean daily temperatures reached 32.3°C at Fowey, 32.1°C at Crocker and Sombrero, and 31.7°C at Pulaski (Figure 1C), exceeding the 30.5°C bleaching threshold in the Florida Keys (Manzello, 2015). These values are above the maximum monthly mean from NOAA Coral Reef Watch (2020) Version 3.0 Daily Global 5-km Satellite Virtual Station for each site which is 29.53°C at Fowey (2.7-km from site), 29.63°C at Crocker (1.7-km from site), 29.71°C at Sombrero (1.7 km from site), and 29.59°C at Pulaski (2-km from site). In situ temperature measured at Pulaski in Dry Tortugas National Park was generally cooler than the other sites, and DHW reached 2.6 in September 2015 and 0 in 2016 while Sombrero reached 3.6 and 0.9 DWH in 2015 and 2016, respectively (Figure 1C). Precipitation (mm), as a proxy for salinity, was similar across the four sites (P ≥ 0.099) with mean ± SE seasonal precipitation for all sites ranging from 258.7 ± 30.9 mm at minimum in Winter 2017 to 801.2 ± 103.8 mm at maximum in Summer 2016. Mean ± SE of PAR estimated at the seafloor did not differ by site (P = 0.999) but differed by season, with winter having lower values of 40.6 ± 1.6 mol m–2 d–1 than the summer values of 55.5 ± 1.3 mol m–2 d–1 (P < 0.001).
From 2015 to 2017, the mean ± SE net calcification rate of P. astreoides across all sites was 3.1 ± 0.2 mg cm–2 d–1 (n = 40, all data are available in Kuffner et al., 2021). Net calcification rates varied significantly by site and time period, and sites responded differently across time periods (Supplementary Table 2). If only corals considered to be “live” (retaining > 50% live tissue) are included, mean net calcification rates across the 2-year study were 4.8 ± 1.2 mg cm–2 d–1 (n = 10) at Pulaski Shoal and 2.9 ± 0.8 mg cm–2 d–1 (n = 21) at the other Florida Keys sites. The highest rate of net calcification measured during any one time period at any site was 9.4 mg cm–2 d–1 (n = 10) at Pulaski Shoal. Mean net calcification rates of colonies at Pulaski and Fowey were 56 and 47% higher in the summer than winter, respectively (Figure 2A, P < 0.001). In the middle Keys, mean net calcification rates were lowest at Sombrero, 1.7 ± 0.4 mg cm–2 d–1, and continued to decline over time. In the southern end of the upper Keys at Crocker, mean net calcification rate was 2.4 mg cm–2 d–1, but in summer 2016 was 49% higher than the other time periods (Figure 2A; P = 0.006).
Figure 2. Coral traits measured after the winter and summer seasons between April 2015 and 2017 for 40 colonies of the coral Porites astreoides from four sites on the Florida Keys Reef Tract. (A) Mean net calcification rates normalized to planar-footprint area (± SE), (B) accumulative percent-planar tissue loss (± SE), and (C) condition scores at the end of each 6-month time period based on a scale from 1 to 6, with six indicating the poorest condition based upon paling, bleaching, and mortality (Baird et al., 2018).
Mean percent live-tissue loss by the end of the 2-year study was low for corals at Pulaski and Fowey Rocks with 0.04 and 13%, respectively, and higher at Sombrero and Crocker reefs, with 47 and 13%, respectively (n = 40; Figure 2B). There was a significant interaction of site and time interval (P < 0.001). Sombrero had colonies that underwent a threefold increase in tissue loss from the initial to final time point of the study (Tukey Post hoc P < 0.001), including one dead colony and four other colonies with over 90% tissue loss. Percent tissue loss remained steadier at Crocker (Tukey Post hoc P ≥ 0.868) and higher than at Pulaski and Fowey.
The coral condition scores based on visual paling, bleaching and mortality had a significant interactive effect with site and time interval (n = 40; P < 0.001), demonstrating that Pulaski and Fowey had consistently healthy colonies throughout the study. Corals at Crocker were highly stressed in Summer 2015 and recovered, while corals at Sombrero accumulated stress over time in response to the high temperature anomalies (Figure 2C).
Two-dimensional PCA plots explained 83.9–92.1% of the variation and highlight differences in overall coral condition among sites at each time interval (Figures 3A–D). The high (poor) condition score at Crocker in Summer 2015 isolated this site from the rest. The consistently higher calcification rates at Pulaski across seasons distanced corals at that site from those at Sombrero.
Figure 3. Principal components analysis (PCA) of dimension 1 and 2 with percent variation explained in parentheses consisting of the three traits measured for corals on the Florida Key Reef Tract at each site at each time interval: (A) Summer 2015, (B) Winter 2016, (C) Summer 2016, and (D) Winter 2017. 95% confidence ellipses are placed around the mean of each site.
Tissue thickness varied by site (n = 3–10 per site; P < 0.001; Supplementary Figure 1A) and was >50% lower at Sombrero than colonies from all other sites (Tukey Post hoc, P ≥ 0.075), while tissue thickness of colonies from Pulaski, Crocker, and Fowey did not differ (Tukey Post hoc, P ≥ 0.075). Symbiont cell densities in colonies from Pulaski had a 2 to 3-fold higher concentration, 6.08 × 106 cells cm–2, than the other three sites (n = 10 per site except Sombrero which had n = 3; Supplementary Figure 1B; Tukey Post hoc, P ≤ 0.015).
For reproduction, oocytes, spermatocytes, and planula larvae were present in all colonies from Pulaski; Sombrero only had three reproductive colonies (Supplementary Figure 2). Mean oocytes, spermatocytes, and larvae differed by sites (Supplementary Table 3; P ≤ 0.039). Colonies at Sombrero had 85% less oocytes per polyp than at both Pulaski and Fowey (Supplementary Figure 1C; P ≤ 0.025). The number of spermatocytes per polyp marginally differed between Pulaski and Crocker (Tukey Post hoc P = 0.065). Pulaski had 35% more larvae per polyp than Sombrero, Crocker, and Fowey (Tukey Post hoc P < 0.001).
Using the SymPortal pipeline, we were able to determine different relative abundance of defining-intragenomic variants (DIVs) by site with colonies from Pulaski consisting of only three DIVs, whereas the other sites had five (n = 7 per site). Symbiodinium spp. (formerly Clade A, LaJeunesse et al., 2018) was ubiquitous in all colonies sampled at all four sites. Seven Symbiodiniaceae ITS2 type profiles were generated from SymPortal, with three being unique to the colonies sampled at Pulaski, while five occurred throughout corals at Sombrero, Crocker, and Fowey (Supplementary Figure 3).
Taken together, the suite of holobiont response variables (calcification rate, condition score, tissue loss, tissue thickness, oocyte and larval density, spermatocyte density, and symbiont cell density) demonstrated clear overall differences by site (PERMANOVA P = 0.001). There was no significant effect of symbiont ITS2-profile type DIV (P = 0.720), nor of the interaction between site and symbiont type (P = 0.081; Supplementary Table 3). The site-level differences were evident with calcification, symbiont cell densities, and reproductive potential highest for Pulaski in Dry Tortugas National Park and in the poor status of colonies at Sombrero in the middle Keys showing high (poor) coral condition scores and high tissue loss. This multivariate representation of the overall coral response can be visualized in the PCA plot, showing that the analyses accounted for 53% of the variation associated with the PC1 axis and 20% on PC2 (Figure 4 and Supplementary Table 2). Separation by site was most apparent between Sombrero and Pulaski. Figure 4 illustrates that symbiont cell density, fecundity, and calcification rates respond together and high levels of those variables were most associated with Pulaski. Similarly, high levels of tissue loss and poor coral-condition score responded together with the inverse of tissue thickness (Figure 4).
Figure 4. Principal components analysis (PCA) of dimensions 1 and 2 explaining 83.4% of the variation when incorporating traits in corals destructively sampled at the end of the study: fecundity (oocytes and planula larvae per polyp), spermaries per polyp, Symbiodiniaceae cell densities, and tissue thickness with the three non-destructive traits measured and bolded (calcification rates, tissue loss, and condition score). Corals are from 4 sites on the Florida Key Reef Tract; 95% confidence ellipses are placed around the mean for each site.
Against a backdrop of historical and modern changes in reef condition along the Florida Keys reef tract, Dry Tortugas National Park represents an exceptional location, demonstrated by thicker and longer duration of Holocene reef development (Toth et al., 2018) and higher rates of net coral calcification (Kuffner et al., 2013, 2020). Our study, based on quantitative comparisons of coral performance and proxies for fitness including colony-level calcification, coral-algal symbiosis, and reproduction, provided even more evidence supporting the exceptionality of the Dry Tortugas area. Understanding the capacity for corals to continue high physiological performance, even in extreme, fluctuating environments with prolonged temperature anomalies, can determine resilient from susceptible coral reefs and specific coral species under anthropogenic climate change (Loya et al., 2001; Fabricius et al., 2011; Grottoli et al., 2014). As thermal stress intensifies, it is important to identify coral traits that can serve as proxies for fitness and determine potential trade-offs among these traits, specifically regarding in situ coral-algal symbiosis and reproduction concurrent with calcification along the FKRT (Kuffner et al., 2013; Manzello et al., 2015; Toth et al., 2018).
Our study demonstrated site-specific differences in P. astreoides bleaching and recovery response during and after a high-temperature stress event, suggesting that either environmental setting, coral genetics, or both elicited a gradient in “sensitive” to “resistant” responses across the Florida Keys reef tract. During and after the 2015 bleaching event in the Florida Keys (Gintert et al., 2018; Manzello et al., 2018), P. astreoides at Pulaski in Dry Tortugas N. P. consistently outperformed colonies at the other three sites, based on high net-calcification rates and symbiont cell densities, low levels of tissue loss, and high reproductive potential. Our results lend additional evidence supporting the hope that “oases” reef sites may still exist (Guest et al., 2018), allowing some corals to persist and continue thriving under the increased thermal stress impacting the FKRT. We documented that critical biological processes, including calcification, symbiosis, and reproduction, were maintained at certain sites while failing at others, suggesting that some populations can persist even when major regional disturbances like the 2015 bleaching event continue to occur.
While P. astreoides has been referred to as a “winner” species in the Caribbean because of its weedy life history (Darling et al., 2012), potential resistance to stony coral tissue loss disease (Aeby et al., 2019), and increasing relative abundance (Green et al., 2008), our results indicate caution in that even such weedy winners are vulnerable and that site-specific responses can inform ecological limits of a resistant species (Grottoli et al., 2014; Manzello et al., 2015). For instance, even though Symbiodinium spp. hosted by shallow-dwelling P. astreoides colonies are mildly tolerant of ultraviolet and high-temperature stress (Loram et al., 2007; Reynolds et al., 2008), P. astreoides is still sensitive to rapid temperature fluctuations and susceptible to bleaching (Edmunds et al., 2005; Gleason et al., 2006; Grottoli et al., 2014; Manzello et al., 2015). The prevalence of P. astreoides throughout the Caribbean and FKRT is likely due to its high-output reproductive strategy and mode as an asexual, hermaphroditic and gonochoric brooder, releasing larvae for multiple months (Chornesky and Peters, 1987; McGuire, 1998; Green et al., 2008; Schlöder and Guzman, 2008; Edmunds, 2010; Yakob and Mumby, 2013; Serrano et al., 2016). This reproductive ability supports high success in recruitment and population recovery following disturbance (Edmunds, 2010), and a high level of larval dispersal is suggested by the lack of population structure in shallow coral reefs of FKRT (Serrano et al., 2016; Gallery et al., in press). However, it is important not to take for granted the reproductive savviness of this species and consider its potential limitations, as many previously thriving species are declining in the FKRT and Caribbean, such as Acropora palmata, because of disease susceptibility and reproductive failure (Baums et al., 2005; Williams et al., 2008; Randall and Szmant, 2009).
Coral calcification, or the net accumulation of calcium-carbonate skeleton, is an integrated measure of coral performance responsive to environmental changes over geological and ecological time scales (Kuffner et al., 2013; Toth et al., 2018). Here, we demonstrated that the corals at our middle Keys and southern upper Keys sites had consistently lower net calcification rates that reflected no seasonal influence, whereas corals in the Dry Tortugas and at the northern upper Keys had overall higher calcification that was seasonal (greater in summer). A study by Manzello et al. (2015) showed similar geographic results, with lower rates of calcification observed in the middle Keys for P. astreoides and Montastraea cavernosa. On a geologic timescale, there is a similar geographic pattern observed in Holocene reef development, with the thickest reefs at either end of the FKRT and the middle Keys suppressed (Toth et al., 2018). Thus, evidence continues to mount in support of Ginsburg and Shinn’s (1964) inimical-waters hypothesis explaining these spatial patterns in coral calcification and reef development. Pinpointing what environmental variables are most important in driving rates of calcification and reef development remains elusive. Temperature (Shinn, 1966; Jokiel and Coles, 1977, 1990), salinity (Coles and Jokiel, 1978; Lirman and Manzello, 2009), carbonate mineral saturation states (Langdon et al., 2000; Albright et al., 2016), light attenuation (Falkowski et al., 1984), and flow (Dennison and Barnes, 1988) can greatly influence coral growth. Our in situ temperature data do suggest that temperature variability may be an important difference among the sites in determining bleaching vulnerability, as Sombrero Reef experienced the highest and the lowest mean daily temperatures. As reef managers update marine-spatial planning and plan extensive reef-restoration activities, it is important to develop finer scale capabilities in the collection of remotely sensed and in situ environmental data (Ogden et al., 1994) so that we can better understand and characterize the conditions that are conducive to high coral-calcification rates and positive reef accretion.
Most reef-monitoring programs that track the long-term health and status of reefs focus primarily on the percent cover of live corals, algae, and other benthic organisms (Edmunds, 2002; Brown et al., 2004; Ruzicka et al., 2013). In our study, we measured several physiological variables critical to biological processes (i.e., calcification, symbiosis, and reproduction), and these measurements allowed us to make clear distinctions in coral condition among sites. Using a dual approach, we measured traits both non-destructively over time (using buoyant weight and photographic image analysis) and destructively at the end of the study to assess tissue thickness, reproductive potential, and maintenance of the coral-algal symbiosis. We discovered that responses were generally correlated, indicating a lack of trade-offs among processes such as calcification and larval production. Through the 2-year study we identified Sombrero and Pulaski as the worst and best locations for P. astreoides, respectively, with Crocker and Fowey as moderate sites. It should be noted that even though there was a loss and thinning of tissue at Sombrero with few Symbiodiniaceae cells and nominal to no oocytes or larvae present, spermaries were still functional and producing spermatocytes in the remaining tissue. Work on other spawning marine invertebrates (e.g., oysters, urchins, and ascidians) has demonstrated reproductive plasticity in response to environmental changes, particularly continued functioning of male-gamete production after egg production and traits were altered; the production of male gametes is considered less energetically demanding and may serve as a last-ditch effort for genetic persistence (Levitan and Petersen, 1995; Crean and Marshall, 2008). Ward (1995) observed substantial energetic costs in reproduction, lipid storage, and calcification in the brooding coral Pocillopora damicornis; colonies that released larvae required more lipids for developing larvae and calcified more slowly than colonies that only released sperm. For P. astreoides colonies from Dry Tortugas, we found no evidence of trade-offs, as the colonies maintained high calcification rates and produced the most larvae and gametes of both sexes, as well as having higher symbiont cell densities compared with corals at the other sites. Determining why coral condition was so universally better in Dry Tortugas compared to the other Keys reef tract sites will require further testing. Given the importance of heterotrophic feeding in determining lipids stores and the resilience they impart during bleaching events (Grottoli et al., 2006; Solomon et al., 2020), testing the hypothesis that corals in Dry Tortugas are receiving nutritional subsidies (more or higher quality plankton) from upwelling events could reveal important insights regarding the mechanism behind the enhanced growth rates we observed.
Symbiodiniaceae species and community composition greatly influence the strength of coral-algal symbiosis under elevated temperatures (Baskett et al., 2009; Putnam et al., 2012; Cunning et al., 2015; Parkinson et al., 2015), including survivorship and maintenance of calcification (Stat et al., 2008). For instance, symbiosis with Cladocopium spp. and Durusdinium spp. (formerly Clade C and D, respectively) is commonly identified as being thermally tolerant but it is also associated with reduced coral-calcification rates (Stat and Gates, 2011; Pettay et al., 2015). Further, symbiont communities or shuffling of symbionts housed within the coral may be shaped by environmental conditions (Jones et al., 2008; LaJeunesse et al., 2010; Cunning et al., 2015). Our study demonstrated that the association of Symbiodinium spp. (A4 and other subtypes) with Porites astreoides was ubiquitous (A4 and other subtypes), which is consistent with previous studies (Thornhill et al., 2006; Kenkel et al., 2013; Serrano et al., 2016). Symbiodinium spp. are considered tolerant of high ultraviolet and thermal conditions; however, knowledge of the physiological functioning of subtypes (e.g., A4a, A4e) or the combination of subtypes (i.e., A4-A4e-A4a) remains limited. Since symbiont communities were similar across our sites, we do not have any evidence to support that site differences in bleaching response resulted from differences in zooxanthellae populations. According to Grottoli et al. (2014), the inflexibility of shallow-dwelling P. astreoides under repeated warming events can result in accumulated stress, turning former Caribbean coral “winner” species into a “loser.” Accumulated stress from back-to-back marine heatwaves (2014 was also a bleaching year in the Florida Keys Precht et al., 2016) paired with the inflexibility of P. astreoides with respect to symbiont shuffling and lack of upregulated feeding under temperature stress could explain the observed poor performance of colonies located at Sombrero Reef. Porites astreoides has demonstrated high adaptive plasticity between inshore and offshore colonies (Kenkel et al., 2013; Kenkel and Matz, 2016); therefore, a common garden or reciprocal transplant experiment would be required to address if local adaptation or acclimatization of corals along the FKRT contributes to differential susceptibility to heat stress (Sanford and Kelly, 2011; Torda et al., 2017).
The SEAKEYS program served as an invaluable monitoring resource that characterized water-column parameters at several offshore navigational structures across the FKRT subregions (Ogden et al., 1994); however, with the discontinuation of this program in 2009 we relied on our own in situ temperature loggers, virtual buoys, and remote satellites to contextualize the environment at each site. Understanding the species-specific responses of corals and reef-building processes to environmental and biological drivers is important and would benefit from more attentive monitoring for effective management and restoration. With the intensity and frequency of marine heatwaves increasing, repeated bleaching events are becoming a new normal. This study is a snapshot within a 2-year window with limited ability to fully contextualize environmental conditioning, selection, and position along a performance curve at each study site. With recent events, there have been conflicting outcomes demonstrating how colonies are able to retain (Ritson-Williams and Gates, 2020); strengthen (Brown et al., 2002; Putnam, 2021), or weaken (Grottoli et al., 2014; Gintert et al., 2018) resistance to bleaching. Through increased monitoring and identification of key environmental parameters beyond temperature, higher-resolution maps of potential reef refugia could be developed (Guest et al., 2018; Lyons et al., 2020). For example, modeling the extent and dynamics of inimical waters impacting coral performance within reefs of the middle and upper Keys as warming continues could better explain the differential responses to coral bleaching within and among species as observed by Gintert et al. (2018). Further understanding of the biological drivers such as local adaptation, gene expression, nutritional provisioning, and symbiosis can be further examined to explain differences in coral performance and reef-building potential in the FKRT subregions. While outlier sites exist along and within subregions of the FKRT (Kuffner et al., 2013, 2020; Ruzicka et al., 2013; Gintert et al., 2018; Toth et al., 2018), it remains unknown why these sites are relatively more “exceptional,” and a better understanding of biophysical settings could help differentiate the mechanisms by which they can escape, resist, or recover from disturbance events (Guest et al., 2018).
The Florida Keys Reef Tract is in a precarious state given that live-coral cover is less than 5% (Ruzicka et al., 2013; Toth et al., 2014) and reef development is net negative in many locations (Perry et al., 2013; Yates et al., 2017; Kuffner et al., 2019). The reignition of successful sexual reproduction and coral recruitment is of utmost importance to the re-establishment of coral populations and reef accretion, for currently, the reefs of the Florida Keys are not in a positive-growth phase and have not been since the mid to late Holocene (Toth et al., 2018). Locating reefs where coral populations historically maintained ecological function, such as maintaining three-dimension structure, biodiversity, and productivity, especially in regions that have undergone warming in the past (Richey et al., 2007; Stathakopoulos and Riegl, 2015), could advance research in determining site selection for reef restoration efforts along the continuum of environmental and physical conditions throughout the Florida Keys. Our results demonstrated that measuring calcification rates in situ is a readily accessible approach that can serve as a proxy for other coral-performance traits to identify successful populations.
Here, we provide evidence that higher calcification rates do not translate to resource partitioning or trade-offs as seen in our P. astreoides colonies living in Dry Tortugas National Park (DTNP). The Pulaski study site within DNTP is remote at the western terminus of the reef system, furthest away from anthropogenic sources of degradation (e.g., coastal development and run-off). DTNP potentially serves as a source for both larval delivery to FKRT sites as the Florida Current could disperse larvae northward (Lee et al., 1992, 1994; Serrano et al., 2016) and larval retention due to the presence of mesoscale eddies that extend down to 100 m depths (Hitchcock et al., 2005; Kourafalou and Kang, 2012). The transport of larvae from healthy populations could be critical in supplying reefs in FKRT impacted by multiple stressors. Recent work by Schoepf et al. (2019) showed that exposure to elevated temperatures does not guarantee stress-resistance, and naïve corals from suitable and cooler conditions were more able to withstand heat stress than counterparts periodically conditioned. Reefs in DTNP are repeatedly bathed in cooler waters and thus may benefit from episodic upwelling (Figure 1C; Ogden et al., 1994; Kuffner, 2016), and depending on pelagic larval duration of coral species, the populations naturally occurring or those potentially restored there in the future could assist in repopulating reefs in FKRT, if those habitats are suitable for recruitment and coral growth.
The datasets presented in this study can be found in online repositories. The names of the repository/repositories and accession number(s) can be found below: https://www.ncbi.nlm.nih.gov/, PRJNA727078. NCBI BioProject: https://www.ncbi.nlm.nih.gov/bioproject/727078 and the two USGS data release [Kuffner et al. (2021), https://doi.org/10.5066/P955KBD3 and Kuffner (2016), https://doi.org/10.5066/F71C1TZK].
IK and EL designed the study and wrote the manuscript. EL analyzed the data. All authors edited and approved the final version of the manuscript, and collected the data.
This study was funded by the National Science Foundation (NSF) Graduate Research Fellowship Program and NSF-USGS Graduate Research Internship Program awarded to EL, and the U.S. Geological Survey (USGS) Coastal-Marine Hazards and Resources Program of the Hazards Mission Area. This paper was also funded in part by a grant/cooperative agreement from the National Oceanic and Atmospheric Administration, Project A/AS-1, which is sponsored by the University of Hawaii Sea Grant College Program, SOEST, under Institutional Grant No. NA18OAR4170076 from NOAA Office of Sea Grant, Department of Commerce. The views expressed herein are those of the author(s) and do not necessarily reflect the views of NOAA or any of its subagencies (UNIHI-SEAGRANT-JC-20-10).
The authors declare that the research was conducted in the absence of any commercial or financial relationships that could be construed as a potential conflict of interest.
We thank M. Donahue, P. Marko, and X. Serrano for their feedback on the manuscript, R. Cunning, A. Eggars, and B. Hume for their assistance with the sequencing and bioinformatics of ITS2, B. Reynolds for boating and diving support, and L. Toth for assistance with the climatology data. The study was conducted under scientific permits from the Florida Keys National Marine Sanctuary (FKNMS-2013-024-A2 and FKNMS-2016-085-A1) and the National Park Service (BISC-2014-SCI-0020, BISC-2016-SCI-0003, DRTO-2015-SCI-0010, and DRTO-2016-SCI-0010), and coral samples are curated under NPS accession numbers BISC−228 and DRTO-274. Any use of trade, firm, or product names is for descriptive purposes only and does not imply endorsement by the U.S. Government.
The Supplementary Material for this article can be found online at: https://www.frontiersin.org/articles/10.3389/fmars.2021.615795/full#supplementary-material
Aeby, G. S., Ushijima, B., Campbell, J. E., Jones, S., Williams, G. J., Meyer, J. L., et al. (2019). Pathogenesis of a tissue loss disease affecting multiple species of corals along the Florida reef tract. Front. Mar. Sci. 6:678. doi: 10.3389/fmars.2019.00678
Albright, R., Caldeira, L., Hosfelt, J., Kwiatkowski, L., Maclaren, J. K., Mason, B. M., et al. (2016). Reversal of ocean acidification enhances net coral reef calcification. Nature 531, 362–365. doi: 10.1038/nature17155
Alvarez-Filip, L., Dulvy, N. K., Gill, J. A., Côté, I. M., and Watkinson, A. R. (2009). Flattening of Caribbean coral reefs: region-wide declines in architectural complexity. Proc. Biol. Sci. 276, 3019–3025. doi: 10.1098/rspb.2009.0339
Baird, A. H., Madin, J. S., Álvarez-Noriega, M., Fontoura, L., Kerry, J. T., Kuo, C. Y., et al. (2018). A decline in bleaching suggests that depth can provide a refuge from global warming in most coral taxa. Mar. Ecol. Prog. Ser. 603, 257–264. doi: 10.3354/meps12732
Baskett, M. L., Gaines, S. D., and Nisbet, R. M. (2009). Symbiont diversity may help coral reefs survive moderate climate change. Ecol. Appl. 19, 3–17. doi: 10.1890/08-0139.1
Bates, D., Mächler, M., Bolker, B., and Walker, S. (2015). Fitting linear mixed-effects models using lme4. J. Stat. Softw. 67, 1–48.
Baums, I. B., Baker, A. C., Davies, S. W., Grottoli, A. G., Kenkel, C. D., Kitchen, S. A., et al. (2019). Considerations for maximizing the adaptive potential of restored coral populations in the western Atlantic. Ecol. Appl. 29:e01978.
Baums, I. B., Miller, M. W., and Hellberg, M. E. (2005). Regionally isolated populations of an imperiled Caribbean coral, Acropora palmata. Mol. Ecol. 14, 1377–1390. doi: 10.1111/j.1365-294X.2005.02489.x
Beyer, H. L., Kennedy, E. V., Beger, M., Chen, C. A., Cinner, J. E., Darling, E. S., et al. (2018). Risk−sensitive planning for conserving coral reefs under rapid climate change. Conserv. Lett. 11:e12587. doi: 10.1111/conl.12587
Brooks, M. E., Kristensen, K., Koen, J., van Benthem, K. J., Magnusson, A., and Berg, C. (2017). glmmTMB balances speed and flexibility among packages for zero-inflated generalized linear mixed modeling. R J. 9, 378–400. doi: 10.32614/rj-2017-066
Brown, B., Dunne, R., and Goodson, M. (2002). Experience shapes the susceptibility of a reef coral to bleaching. Coral Reefs 21, 119–126. doi: 10.1007/s00338-002-0215-z
Brown, E. K., Cox, E., Jokiel, P., Rodgers, K., Smith, W., Tissot, B. N., et al. (2004). Development of benthic sampling methods for the coral reef assessment and monitoring program (CRAMP) in Hawai’i. Pac. Sci. 58, 145–158. doi: 10.1353/psc.2004.0013
Chornesky, E. A., and Peters, E. C. (1987). Sexual reproduction and colony growth in the scleractinian coral Porites astredoides. Biol. Bull. 172, 161–177. doi: 10.2307/1541790
Cinner, J. E., Huchery, C., MacNeil, M. A., Graham, N. A. J., McClanahan, T. R., Maina, J., et al. (2016). Bright spots among the world’s coral reefs. Nature 535, 416–419.
Coles, S. L., and Jokiel, P. L. (1978). Synergistic effects of temperature, salinity and light on the hermatypic coral Montipora verrucosa. Mar. Biol. 49, 187–195. doi: 10.1007/bf00391130
Crean, A. J., and Marshall, D. J. (2008). Gamete plasticity in a broadcast spawning marine invertebrate. Proc. Natl. Acad. Sci. U.S.A. 105, 13508–13513. doi: 10.1073/pnas.0806590105
Cunning, R., Gates, R. D., and Edmunds, P. J. (2017). Using high-throughput sequencing of ITS2 to describe Symbiodinium metacommunities in St. John, US Virgin Islands. PeerJ 5:e3472. doi: 10.7717/peerj.3472
Cunning, R., Silverstein, R. N., and Baker, A. C. (2018). Symbiont shuffling linked to differential photochemical dynamics of Symbiodinium in three Caribbean reef corals. Coral Reefs 37, 145–152. doi: 10.1007/s00338-017-1640-3
Cunning, R., Vaughan, N., Gillette, P., Capo, T. R., Matté, J. L., and Baker, A. C. (2015). Dynamic regulation of partner abundance mediates response of reef coral symbioses to environmental change. Ecology 96, 1411–1420. doi: 10.1890/14-0449.1
Darling, E. S., Alvarez-Filip, L., Oliver, T. A., McClanahan, T. R., and Côté, I. M. (2012). Evaluating life-history strategies of reef corals from species traits. Ecol. Lett. 15, 1378–1386. doi: 10.1111/j.1461-0248.2012.01861.x
Darling, E. S., McClanahan, T. R., Maina, J., Gurney, G. G., Graham, N. A. J., Januchowski-Hartley, F., et al. (2019). Social-environmental drivers inform strategic management of coral reefs in the Anthropocene. Nat. Ecol. Evol. 3, 1341–1350.
de Lamarck, J. B. M. (1816). Histoire Naturelle des Animaux sans Vertèbres, Tome Troisième [In full: Histoire Naturelle des Animaux Sans Vertèbres Présentant les Caractéres Généraux et Particuliers de ces Animaux, Leur Distribution, Leurs Classes, Leurs Familles, Leurs Genres, et la Citation des Principales Espèces qui s’y Rapportent]. Paris: Deterville/Verdière, 586.
De’ath, G., Fabricius, K. E., Sweatman, H., and Puotinen, M. (2012). The 27-year decline of coral cover on the Great Barrier Reef and its causes. Proc. Natl. Acad. Sci. U.S.A. 109, 17995–17999. doi: 10.1073/pnas.1208909109
Dennison, W. C., and Barnes, D. J. (1988). Effect of water motion on coral photosynthesis and calcification. J. Exp. Mar. Biol. Ecol. 115, 67–77. doi: 10.1016/0022-0981(88)90190-6
Eakin, C. M., Mark Eakin, C., Sweatman, H. P. A., and Brainard, R. E. (2019). The 2014–2017 global-scale coral bleaching event: insights and impacts. Coral Reefs 38, 539–545. doi: 10.1007/s00338-019-01844-2
Edmunds, P. J. (2002). Long-term dynamics of coral reefs in St. John, US Virgin Islands. Coral Reefs 21, 357–367. doi: 10.1007/s00338-002-0258-1
Edmunds, P. J. (2010). Population biology of Porites astreoides and Diploria strigosa on a shallow Caribbean reef. Mar. Ecol. Prog. Ser. 418, 87–104. doi: 10.3354/meps08823
Edmunds, P. J., Gates, R. D., Leggat, W., Hoegh-Guldberg, O., and Allen-Requa, L. (2005). The effect of temperature on the size and population density of dinoflagellates in larvae of the reef coral Porites astreoides. Invertebr. Biol. 124, 185–193. doi: 10.1111/j.1744-7410.2005.00018.x
Fabricius, K. E., Langdon, C., Uthicke, S., Humphrey, C., Noonan, S., De’ath, G., et al. (2011). Losers and winners in coral reefs acclimatized to elevated carbon dioxide concentrations. Nat. Clim. Change 1, 165–169. doi: 10.1038/nclimate1122
Falkowski, P. G., Dubinsky, Z., Muscatine, L., and Porter, J. W. (1984). Light and the bioenergetics of a symbiotic coral. Bioscience 34, 705–709. doi: 10.2307/1309663
Fox, J., Friendly, M., and Weisberg, S. (2013). Hypothesis tests for multivariate linear models using the car package. R J. 5, 39–52. doi: 10.32614/rj-2013-004
Gallery, D. N., Green, M. L. I., Kuffner, B., Lenz, E. A., and Toth, L. T. (In press). Genetic structure and diversity of the mustard hill coral, Porites astreoides, along the Florida Keys reef tract. Mar. Biodivers.
Gardner, T. A., Côté, I. M., Gill, J. A., Grant, A., and Watkinson, A. R. (2005). Hurricanes and Caribbean coral reefs: impacts, recovery, and role in long-term decline. Ecology 86, 174–184. doi: 10.1890/04-0141
Ginsburg, R. N., and Shinn, E. A. (1964). Distribution of the Reef-Building Community in Florida and the Bahamas. Am. Assoc. Petrol. Geol. Bull. 48:527.
Ginsburg, R. N., and Shinn, E. A. (1994). “Preferential distribution of reefs in the Florida reef tract: the past is the key to the present,” in Colloquium on Global Aspects of Coral Reefs: Health, Hazards, and History, Rosential School of Marine and Atmospheric Science, ed. R. N. Ginsburg (Coral Gables, FL: University of Miami), 21–26.
Gintert, B. E., Manzello, D. P., Enochs, I. C., Kolodziej, G., Carlton, R., Gleason, A. C. R., et al. (2018). Marked annual coral bleaching resilience of an inshore patch reef in the Florida keys: a nugget of hope, aberrance, or last man standing? Coral Reefs 37, 533–547. doi: 10.1007/s00338-018-1678-x
Gleason, D. F., Edmunds, P. J., and Gates, R. D. (2006). Ultraviolet radiation effects on the behavior and recruitment of larvae from the reef coral Porites astreoides. Mar. Biol. 148, 503–512. doi: 10.1007/s00227-005-0098-y
Green, D. H., Edmunds, P. J., and Carpenter, R. C. (2008). Increasing relative abundance of Porites astreoides on Caribbean reefs mediated by an overall decline in coral cover. Mar. Ecol. Prog. Ser. 359, 1–10. doi: 10.3354/meps07454
Grottoli, A., Rodrigues, L., and Palardy, J. (2006). Heterotrophic plasticity and resilience in bleached corals. Nature 440, 1186–1189. doi: 10.1038/nature04565
Grottoli, A. G., Warner, M. E., Levas, S. J., Aschaffenburg, M. D., Schoepf, V., McGinley, M., et al. (2014). The cumulative impact of annual coral bleaching can turn some coral species winners into losers. Glob. Change Biol. 20, 3823–3833. doi: 10.1111/gcb.12658
Guest, J. R., Edmunds, P. J., Gates, R. D., Kuffner, I. B., Andersson, A. J., Barnes, B. B., et al. (2018). A framework for identifying and characterising coral reef “oases” against a backdrop of degradation. J. Appl. Ecol. 55, 2865–2875. doi: 10.1111/1365-2664.13179
Hallock, P., and Schlager, W. (1986). Nutrient excess and the demise of coral reefs and carbonate platforms. PALAIOS 1, 389–398. doi: 10.2307/3514476
Hartig, F. (2017). DHARMa: Residual Diagnostics for Hierarchical (Multi-Level/Mixed) Regression Models. R Package Version 0.2, 4.
Hauff, B., Haslun, J. A., Strychar, K. B., Ostrom, P. H., and Cervino, J. M. (2016). Symbiont diversity of zooxanthellae (Symbiodinium spp.) in Porites astreoides and Montastraea cavernosa from a reciprocal transplant in the lower keys. Int. J. Biol. 8:9. doi: 10.5539/ijb.v8n2p9
Hitchcock, G. L., Lee, T. N., Ortner, P. B., Cummings, S., Kelble, C., and Williams, E. (2005). Property fields in a Tortugas eddy in the southern Straits of Florida. Deep Sea Res. I Oceanogr. Res. Pap. 52, 2195–2213. doi: 10.1016/j.dsr.2005.08.006
Hoegh-Guldberg, O., Kennedy, E. V., Beyer, H. L., McClennen, C., and Possingham, H. P. (2018). Securing a long-term future for coral reefs. Trends Ecol. Evol. 33, 936–944. doi: 10.1016/j.tree.2018.09.006
Hughes, T. P., Baird, A. H., Bellwood, D. R., Card, M., Connolly, S. R., Folke, C., et al. (2003). Climate change, human impacts, and the resilience of coral reefs. Science 301, 929–933. doi: 10.1126/science.1085046
Hughes, T. P., Kerry, J. T., Baird, A. H., Connolly, S. R., Dietzel, A., Eakin, C. M., et al. (2018). Global warming transforms coral reef assemblages. Nature 556, 492–496. doi: 10.1038/s41586-018-0041-2
Hume, B. C. C., Smith, E. G., Ziegler, M., Warrington, H. J. M., Burt, J. A., LaJeunesse, T. C., et al. (2019). SymPortal: a novel analytical framework and platform for coral algal symbiont next-generation sequencing ITS2 profiling. Mol. Ecol. Resour. 19, 1063–1080. doi: 10.1111/1755-0998.13004
Intergovernmental Panel on Climate Change (IPCC) (2018). Global Warming of 1.5°C: An IPCC Special Report on the Impacts of Global Warming of 1.5°C Above Pre-Industrial Levels and Related Global Greenhouse Gas Emission Pathways, in the Context of Strengthening the Global Response to the Threat of Climate Change, Sustainable Development, and Efforts to Eradicate Poverty. Geneva: Intergovernmental Panel on Climate Change.
Jokiel, P. L., and Coles, S. L. (1977). Effects of temperature on the mortality and growth of Hawaiian reef corals. Mar. Biol. 43, 201–208. doi: 10.1007/bf00402312
Jokiel, P. L., and Coles, S. L. (1990). Response of Hawaiian and other Indo-Pacific reef corals to elevated temperature. Coral Reefs 8, 155–162. doi: 10.1007/bf00265006
Jokiel, P. L., Maragos, J. E., and Franzisket, L. (1978). “Coral growth: buoyant weight technique,” in Coral Reefs: Research Methods, eds D. R. Stoddart and R. E. Johannes (Paris: UNESCO).
Jones, A. M., Berkelmans, R., van Oppen, M. J. H., Mieog, J. C., and Sinclair, W. (2008). A community change in the algal endosymbionts of a scleractinian coral following a natural bleaching event: field evidence of acclimatization. Proc. Biol. Sci. 275, 1359–1365. doi: 10.1098/rspb.2008.0069
Kassambara, A., and Mundt, F. (2017). Factoextra: Extract and Visualize the Results of Multivariate Data Analyses. R Package Version 1, Vol. 5, 337–354.
Kenkel, C. D., Goodbody-Gringley, G., Caillaud, D., Davies, S. W., Bartels, E., and Matz, M. V. (2013). Evidence for a host role in thermotolerance divergence between populations of the mustard hill coral (Porites astreoides) from different reef environments. Mol. Ecol. 22, 4335–4348. doi: 10.1111/mec.12391
Kenkel, C. D., and Matz, M. V. (2016). Gene expression plasticity as a mechanism of coral adaptation to a variable environment. Nat. Ecol. Evol. 1, 0014. doi: 10.1038/s41559-016-0014
Kourafalou, V. H., and Kang, H. (2012). Florida current meandering and evolution of cyclonic eddies along the Florida reef tract: are they interconnected? J. Geophys. Res. 117:C05028. doi: 10.1029/2011JC007383
Kuffner, I. B. (2016). Underwater Temperature on Off-Shore Coral Reefs of the Florida Keys, U.S.A. (ver. 6.0, February 2021): U.S. Geological Survey Data Release. St. Petersburg, FL: U.S. Geological Survey. doi: 10.5066/F71C1TZK
Kuffner, I. B., Hickey, T. D., and Morrison, J. M. (2013). Calcification rates of the massive coral Siderastrea siderea and crustose coralline algae along the Florida Keys (USA) outer-reef tract. Coral Reefs 32, 987–997. doi: 10.1007/s00338-013-1047-8
Kuffner, I. B., Lenz, E. A., Bartlett, L. A., Stathakopoulos, A., and Morrison, J. M. (2021). Experimental Coral-Growth and Physiological Data and Time-Series Imagery Data for Porites astreoides in the Florida Keys, U.S.A.: U.S. Geological Survey Data Release. doi: 10.5066/P955KBD3
Kuffner, I. B., Lidz, B. H., Harold Hudson, J., and Anderson, J. S. (2015). A century of ocean warming on Florida Keys coral reefs: historic in situ observations. Estuaries Coasts 38, 1085–1096. doi: 10.1007/s12237-014-9875-5
Kuffner, I. B., Stathakopoulos, A., Toth, L. T., and Bartlett, L. A. (2020). Reestablishing a stepping-stone population of the threatened elkhorn coral Acropora palmata to aid regional recovery. Endanger. Species Res. 43, 461–473. doi: 10.3354/esr01083
Kuffner, I. B., Toth, L. T., Harold Hudson, J., Goodwin, W. B., Stathakopoulos, A., Bartlett, L. A., et al. (2019). Improving estimates of coral reef construction and erosion with in situ measurements. Limnol. Oceanogr. 64, 2283–2294. doi: 10.1002/lno.11184
Kuznetsova, A., Brockhoff, P. B., and Christensen, R. H. B. (2017). lmerTest package: tests in linear mixed effects models. J. Stat. Softw. 82, 1–26.
LaJeunesse, T. C. (2001). Investigating the biodiversity, ecology, and phylogeny of endosymbiotic dinoflagellates in the genus Symbiodinium using the ITS region: in search of a “species” level marker. J. Phychol. 37, 866–880. doi: 10.1046/j.1529-8817.2001.01031.x
LaJeunesse, T. C., Parkinson, J. E., Gabrielson, P. W., Jeong, H. J., Reimer, J. D., Voolstra, C. R., et al. (2018). Systematic revision of Symbiodiniaceae highlights the antiquity and diversity of coral endosymbionts. Curr. Biol. 28, 2570–2580.e6.
LaJeunesse, T. C., Smith, R., Walther, M., Pinzón, J., Pettay, D. T., McGinley, M., et al. (2010). Host-symbiont recombination versus natural selection in the response of coral-dinoflagellate symbioses to environmental disturbance. Proc. Biol. Sci. 277, 2925–2934. doi: 10.1098/rspb.2010.0385
Langdon, C., Takahashi, T., Sweeney, C., Chipman, D., Goddard, J., Marubini, F., et al. (2000). Effect of calcium carbonate saturation state on the calcification rate of an experimental coral reef. Glob. Biogeochem. Cycles 14, 639–654. doi: 10.1029/1999gb001195
Lee, T. N., Clarke, M. E., Williams, E., Szmant, A. F., and Berger, T. (1994). Evolution of the Tortugas gyre and its influence on recruitment in the Florida Keys. Bull. Mar. Sci. 54, 621–646.
Lee, T. N., Rooth, C., Williams, E., McGowan, M., Szmant, A. F., and Clarke, M. E. (1992). Influence of Florida current, gyres and wind-driven circulation on transport of larvae and recruitment in the Florida Keys coral reefs. Cont. Shelf Res. 12, 971–1002. doi: 10.1016/0278-4343(92)90055-o
Lenz, E. A., Bramanti, L., Lasker, H. R., and Edmunds, P. J. (2015). Long-term variation of octocoral populations in St. John, US Virgin Islands. Coral Reefs 34, 1099–1109. doi: 10.1007/s00338-015-1315-x
Levitan, D. R., and Petersen, C. (1995). Sperm limitation in the sea. Trends Ecol. Evol. 10, 228–231. doi: 10.1016/s0169-5347(00)89071-0
Lidz, B. H., Reich, C. D., Peterson, R. L., and Shinn, E. A. (2006). New maps, new information: coral reefs of the Florida Keys. J. Coast. Res. 222, 260–282. doi: 10.2112/05a-0023.1
Lidz, B. H., and Shinn, E. A. (1991). Paleoshorelines, reefs, and a rising sea: South Florida, U.S.A. J. Coast. Res. 7, 203–229.
Lirman, D., and Manzello, D. (2009). Patterns of resistance and resilience of the stress-tolerant coral Siderastrea radians (Pallas) to sub-optimal salinity and sediment burial. J. Exp. Mar. Biol. Ecol. 369, 72–77. doi: 10.1016/j.jembe.2008.10.024
Loram, J. E., Trapido-Rosenthal, H. G., and Douglas, A. E. (2007). Functional significance of genetically different symbiotic algae Symbiodinium in a coral reef symbiosis. Mol. Ecol. 16, 4849–4857. doi: 10.1111/j.1365-294x.2007.03491.x
Loya, Y., Sakai, K., Yamazato, K., Nakano, Y., Sambali, H., and van Woesik, R. (2001). Coral bleaching: the winners and the losers. Ecol. Lett. 4, 122–131. doi: 10.1046/j.1461-0248.2001.00203.x
Lyons, M. B., Roelfsema, C. M., Kennedy, E. V., Kovacs, E. M., Borrego-Acevedo, R., Markey, K., et al. (2020). Mapping the world’s coral reefs using a global multiscale earth observation framework. Rem. Sens. Ecol. Conserv. 6, 556–568.
Manzello, D. P., Enochs, I. C., Kolodziej, G., and Carlton, R. (2015). Coral growth patterns of Montastraea cavernosa and Porites astreoides in the Florida Keys: the importance of thermal stress and inimical waters. J. Exp. Mar. Biol. Ecol. 471, 198–207. doi: 10.1016/j.jembe.2015.06.010
Manzello, D. P., Enochs, I. C., Kolodziej, G., Carlton, R., and Valentino, L. (2018). Resilience in carbonate production despite three coral bleaching events in 5 years on an inshore patch reef in the Florida Keys. Mar. Biol. 165, 99.
Marsh, J. A. (1970). Primary productivity of reef-building calcareous red algae. Ecology 51, 255–263. doi: 10.2307/1933661
McClanahan, T. R., Cinner, J. E., Graham, N. A. J., Daw, T. M., Maina, J., Stead, S. M., et al. (2009). Identifying reefs of hope and hopeful actions: contextualizing environmental, ecological, and social parameters to respond effectively to climate change. Conserv. Biol. 23, 662–671. doi: 10.1111/j.1523-1739.2008.01154.x
McGuire, M. P. (1998). Timing of larval release by Porites astreoides in the northern Florida Keys. Coral Reefs 17, 369–375. doi: 10.1007/s003380050141
Moberg, F., and Folke, C. (1999). Ecological goods and services of coral reef ecosystems. Ecol. Econ. 29, 215–233. doi: 10.1016/s0921-8009(99)00009-9
Morrison, J. M., Kuffner, I. B., and Hickey, T. D. (2013). Methods for Monitoring Corals and Crustose Coralline Algae to Quantify in-situ Calcification Rates. U.S. Geological Survey Open-File Report 2013-1159. Reston, VA: U.S. Geological Survey, 11.
Murdoch, T. J. T., and Aronson, R. B. (1999). Scale-dependent spatial variability of coral assemblages along the Florida Reef Tract. Coral Reefs 18, 341–351. doi: 10.1007/s003380050210
Neumann, A. C., and Macintyre, I. (1985). “Reef response to sea level rise: keep-up, catch-up or give-up,” in Proceedings of the 5th International Coral Reef Congress, Vol. 3, Tahiti, 105–110.
NOAA Coral Reef Watch (2020). Updated Daily. NOAA Coral Reef Watch Version 3.1 Daily Global 5-km Satellite Virtual Station Time Series Data for Florida Keys, Jan. 1, 2015-Mar. 31, 2017. College Park, MD: NOAA Coral Reef Watch.
Norström, A. V., Nyström, M., Lokrantz, J., and Folke, C. (2009). Alternative states on coral reefs: beyond coral–macroalgal phase shifts. Mar. Ecol. Prog. Ser. 376, 295–306. doi: 10.3354/meps07815
Ogden, J. C., Porter, J. W., Smith, N. P., Szmant, A. M., Jaap, W. C., and Forcucci, D. (1994). A long-term interdisciplinary study of the Florida Keys seascape. Bull. Mar. Sci. 54, 1059–1071.
Oksanen, J., Kindt, R., Legendre, P., O’Hara, B., Henry, M., Stevens, H., et al. (2007). The Vegan Package. Community Ecology Package, Vol. 10, 631–637.
Padilla-Gamiño, J. L., Hédouin, L., Waller, R. G., Smith, D., Truong, W., and Gates, R. D. (2014). Sedimentation and the reproductive biology of the Hawaiian reef-building coral Montipora capitata. Biol. Bull. 226, 8–18. doi: 10.1086/bblv226n1p8
Parkinson, J. E., Banaszak, A. T., Altman, N. S., LaJeunesse, T. C., and Baums, I. B. (2015). Intraspecific diversity among partners drives functional variation in coral symbioses. Sci. Rep. 5:15667.
Perry, C. T., Murphy, G. N., Kench, P. S., Smithers, S. G., Edinger, E. N., Steneck, R. S., et al. (2013). Caribbean-wide decline in carbonate production threatens coral reef growth. Nat. Comm. 4:1402.
Pettay, D. T., Wham, D. C., Smith, R. T., Iglesias-Prieto, R., and LaJeunesse, T. C. (2015). Microbial invasion of the Caribbean by an Indo-Pacific coral zooxanthellae. Proc. Natl. Acad. Sci. U.S.A. 112, 7513–7518. doi: 10.1073/pnas.1502283112
Pratchett, M. S., Hoey, A. S., and Wilson, S. K. (2014). Reef degradation and the loss of critical goods and services provided by coral reef fishes. Curr. Opin. Environ. Sustain. 7, 37–43. doi: 10.1016/j.cosust.2013.11.022
Precht, W. F., and Aronson, R. B. (2004). Climate flickers and range shifts of reef corals. Front. Ecol. Environ. 2, 307–314. doi: 10.1890/1540-9295(2004)002[0307:CFARSO]2.0.CO;2
Precht, W. F., Gintert, B. E., Robbart, M. L., Fura, R., and van Woesik, R. (2016). Unprecedented disease-related coral mortality in Southeastern Florida. Sci. Rep. 6:31374.
Precht, W. F., and Miller, S. L. (2007). “Ecological shifts along the Florida reef tract: the past as a key to the future,” in Geological Approaches to Coral Reef Ecology, ed. R. B. Aronson (New York, NY: Springer), 237–312. doi: 10.1007/978-0-387-33537-7_9
Putnam, H. M. (2021). Avenues of reef-building coral acclimatization in response to rapid environmental change. J. Exp. Biol. 224 (Suppl. 1):jeb239319. doi: 10.1242/jeb.239319
Putnam, H. M., Stat, M., Pochon, X., and Gates, R. D. (2012). Endosymbiotic flexibility associates with environmental sensitivity in scleractinian corals. Proc. Biol. Sci. 279, 4352–4361. doi: 10.1098/rspb.2012.1454
Randall, C. J., and Szmant, A. M. (2009). Elevated temperature affects development, survivorship, and settlement of the elkhorn coral, Acropora palmata (Lamarck 1816). Biol. Bull. 217, 269–282. doi: 10.1086/bblv217n3p269
Randall, C. J., and van Woesik, R. (2015). Contemporary white-band disease in Caribbean corals driven by climate change. Nat. Clim. Change 5, 375–379. doi: 10.1038/nclimate2530
Reynolds, J. M., Bruns, B. U., Fitt, W. K., and Schmidt, G. W. (2008). Enhanced photoprotection pathways in symbiotic dinoflagellates of shallow-water corals and other cnidarians. Proc. Natl. Acad. Sci. U.S.A. 105, 13674–13678. doi: 10.1073/pnas.0805187105
Richey, J. N., Poore, R. Z., Flower, B. P., and Quinn, T. M. (2007). 1400 yr multiproxy record of climate variability from the northern Gulf of Mexico. Geology 35, 423–426. doi: 10.1130/g23507a.1
Ritson-Williams, R., and Gates, R. (2020). Coral community resilience to successive years of bleaching in Kāne’ohe Bay, Hawai’i. Coral Reefs 39:1944. doi: 10.1007/s00338-020-01944-4
Ruzicka, R. R., Colella, M. A., Porter, J. W., Morrison, J. M., Kidney, J. A., Brinkhuis, V., et al. (2013). Temporal changes in benthic assemblages on Florida Keys reefs 11 years after the 1997/1998 El Niño. Mar. Ecol. Prog. Ser. 489, 125–141.
Sanford, E., and Kelly, M. W. (2011). Local adaptation in marine invertebrates. Ann. Rev. Mar. Sci. 3, 509–535. doi: 10.1146/annurev-marine-120709-142756
Schlager, W. (1981). The paradox of drowned reefs and carbonate platforms. Geol. Soc. Am. Bull. 92, 197–211. doi: 10.1130/0016-7606(1981)92<197:tpodra>2.0.co;2
Schlöder, C., and Guzman, H. M. (2008). Reproductive patterns of the Carribbean coral Porites furcate (Anthozoa, Scleractinia, Poritidae) in Panama. Bull. Mar. Sci. 82, 107–117.
Schneider, C. A., Rasband, W. S., and Eliceiri, K. W. (2012). NIH image to ImageJ: 25 years of image analysis. Nat. Methods 9, 671–675. doi: 10.1038/nmeth.2089
Schoepf, V., Carrion, S. A., Pfeifer, S. M., Naugle, M., Dugal, L., Bruyn, J., et al. (2019). Stress-resistant corals may not acclimatize to ocean warming but maintain heat tolerance under cooler temperatures. Nat. Commun. 10:4031.
Serrano, X. M., Baums, I. B., Smith, T. B., Jones, R. J., Shearer, T. L., and Baker, A. C. (2016). Long distance dispersal and vertical gene flow in the Caribbean brooding coral Porites astreoides. Sci. Rep. 6:21619.
Smith, N. P. (2002). Tidal, low-frequency and long-term mean transport through two channels in the Florida Keys. Cont. Shelf Res. 22, 1643–1650. doi: 10.1016/s0278-4343(02)00027-4
Solomon, S. L., Grottoli, A. G., Warner, M. E., Levas, S., Schoepf, V., and Munoz-Garcia, A. (2020). Lipid class composition of annually bleached Caribbean corals. Mar. Biol. 167:7.
Stat, M., and Gates, R. D. (2011). Clade D Symbiodiniumin scleractinian corals: a “nugget” of hope, a selfish opportunist, an ominous sign, or all of the above? J. Mar. Biol. 2011:730715.
Stat, M., Morris, E., and Gates, R. D. (2008). Functional diversity in coral-dinoflagellate symbiosis. Proc. Natl. Acad. Sci. U.S.A. 105, 9256–9261. doi: 10.1073/pnas.0801328105
Stat, M., Pochon, X., Cowie, R. O. M., and Gates, R. D. (2009). Specificity in communities of Symbiodinium in corals from Johnston Atoll. Mar. Ecol. Prog. Ser. 386, 83–96. doi: 10.3354/meps08080
Stathakopoulos, A., and Riegl, B. M. (2015). Accretion history of mid-Holocene coral reefs from the Southeast Florida continental reef tract, USA. Coral Reefs 34, 173–187. doi: 10.1007/s00338-014-1233-3
Stephenson, T. A., and Stephenson, A. (1950). Life between tide-marks in North America: the Florida Keys. J. Ecol. 38, 354–402. doi: 10.2307/2256451
Storlazzi, C. D., Reguero, B. G., Cole, A. D., Lowe, E., Shope, J. B., Gibbs, A. E., et al. (2019). Rigorously Valuing the Role of U.S. Coral Reefs in Coastal Hazard Risk Reduction. Open-File Report. Reston, VA: U.S. Geological Survey.
Thornhill, D. J., Fitt, W. K., and Schmidt, G. W. (2006). Highly stable symbioses among western Atlantic brooding corals. Coral Reefs 25, 515–519. doi: 10.1007/s00338-006-0157-y
Thornhill, D. J., Lajeunesse, T. C., and Santos, S. R. (2007). Measuring rDNA diversity in eukaryotic microbial systems: how intragenomic variation, pseudogenes, and PCR artifacts confound biodiversity estimates. Mol. Ecol. 16, 5326–5340. doi: 10.1111/j.1365-294x.2007.03576.x
Thornhill, D. J., Lewis, A. M., Wham, D. C., and LaJeunesse, T. C. (2014). Host-specialist lineages dominate the adaptive radiation of reef coral endosymbionts. Evolution 68, 352–367. doi: 10.1111/evo.12270
Torda, G., Donelson, J. M., Aranda, M., Barshis, D. J., Bay, L., and Berumen, M. L. (2017). Rapid adaptive responses to climate change in corals. Nat. Clim. Change 7, 627–636. doi: 10.1038/nclimate3374
Toth, L. T., Cheng, H., Edwards, R. L., Ashe, E., and Richey, J. N. (2017). Millenial-scale variability in the local radiocarbon reservoir age of south Florida during the Holocene. Quat. Geochronol. 42, 130–143. doi: 10.1016/j.quageo.2017.07.005
Toth, L. T., Kuffner, I. B., Stathakopoulos, A., and Shinn, E. A. (2018). A 3,000-year lag between the geological and ecological shutdown of Florida’s coral reefs. Glob. Change Biol. 24, 5471–5483. doi: 10.1111/gcb.14389
Toth, L. T., van Woesik, R., Murdoch, T. J. T., Smith, S. R., Ogden, J. C., Precht, W. F., et al. (2014). Do no-take reserves benefit Florida’s corals? 14 years of change and stasis in the Florida Keys National Marine Sanctuary. Coral Reefs 33, 565–577. doi: 10.1007/s00338-014-1158-x
Ward, S. (1995). Two patterns of energy allocation for growth, reproduction and lipid storage in the scleractinian coral Pocillopora damicornis. Coral Reefs 14, 87–90. doi: 10.1007/bf00303428
Webster, P. J., Holland, G. J., Curry, J. A., and Change, H. R. (2005). Changes in tropical cyclone number, duration, and intensity in a warming environment. Science 309, 1844–1846. doi: 10.1126/science.1116448
Williams, D. E., Miller, M. W., and Kramer, K. L. (2008). Recruitment failure in Florida Keys Acropora palmata, a threatened Caribbean coral. Coral Reefs 27, 697–705. doi: 10.1007/s00338-008-0386-3
Wyatt, A. S., Leichter, J. J., Toth, L. T., Miyajima, T., Aronson, R. B., and Nagata, T. (2020). Heat accumulation on coral reefs mitigated by internal waves. Nat. Geosci. 13, 28–34. doi: 10.1038/s41561-019-0486-4
Yakob, L., and Mumby, P. (2013). Life histories offer a clue to the future of infectious disease on coral reefs. ANZIAM J. 54, 64–73. doi: 10.21914/anziamj.v54i0.5862
Keywords: scleractinia, in situ calcification rates, reproduction, coral-algal symbiosis, coral reef degradation, buoyant weight
Citation: Lenz EA, Bartlett LA, Stathakopoulos A and Kuffner IB (2021) Physiological Differences in Bleaching Response of the Coral Porites astreoides Along the Florida Keys Reef Tract During High-Temperature Stress. Front. Mar. Sci. 8:615795. doi: 10.3389/fmars.2021.615795
Received: 09 October 2020; Accepted: 10 May 2021;
Published: 22 June 2021.
Edited by:
John Everett Parkinson, University of South Florida, United StatesReviewed by:
Dustin Kemp, University of Alabama at Birmingham, United StatesCopyright © 2021 Lenz, Bartlett, Stathakopoulos and Kuffner. This is an open-access article distributed under the terms of the Creative Commons Attribution License (CC BY). The use, distribution or reproduction in other forums is permitted, provided the original author(s) and the copyright owner(s) are credited and that the original publication in this journal is cited, in accordance with accepted academic practice. No use, distribution or reproduction is permitted which does not comply with these terms.
*Correspondence: Elizabeth Ann Lenz, ZWFsZW56QGhhd2FpaS5lZHU=
Disclaimer: All claims expressed in this article are solely those of the authors and do not necessarily represent those of their affiliated organizations, or those of the publisher, the editors and the reviewers. Any product that may be evaluated in this article or claim that may be made by its manufacturer is not guaranteed or endorsed by the publisher.
Research integrity at Frontiers
Learn more about the work of our research integrity team to safeguard the quality of each article we publish.