- 1Department of Marine Animal Ecology, Wageningen University & Research, Wageningen, Netherlands
- 2Department of Fisheries and Oceans, Bedford Institute of Oceanography, Dartmouth, NS, Canada
- 3Centre for Geobiology, Department of Biology, University of Bergen, Bergen, Norway
Plumes of re-suspended sediment potentially smother and clog the aquiferous system of filter-feeding sponges with unknown implications for their health. For the first time, we examined the physiological responses of repeated exposure to natural sediment in the glass sponge Vazella pourtalesii, which forms dense sponge grounds in Emerald Basin off Nova Scotia, Canada. Ex situ chamber-based measurements of bacterial clearance and oxygen consumption (respiration) rates indicated that individuals subjected to elevated concentrations of suspended sediment expressed normal clearance and respiration rates over 7 days of sediment exposure, indicating an ability to cope with elevated concentrations of indigestible sediment particles. However, clearance rates significantly declined after 14 days of sediment exposure, suggesting an inability to cope with long-term exposure to increased sediment load. Therefore, long-term exposure to elevated concentrations of suspended sediment should be avoided in order to minimize adverse effects on the abundant Vazella sponge grounds.
Introduction
Sponges (Porifera) play a pivotal role within the marine ecosystems they inhabit from tropical shallow waters to abyssal depths. Providing three-dimensional structures and secondary substrates through a diversity of growth forms, they function as microhabitats themselves, increasing local faunal biodiversity (Beazley et al., 2013, 2015; Hawkes et al., 2019). Sponges are also key players in benthic-pelagic energy transfer and biochemical processing by filtering large volumes of seawater (Maldonado et al., 2012, 2017; De Goeij et al., 2013; Pham et al., 2019). The use of advanced technologies such as remotely operated vehicles (ROVs) has provided evidence of the vast diversity and abundance of sponges in the majority of deep-sea ecosystems (Maldonado et al., 2017). In specific oceanographic settings, they can represent up to 94% of the benthic biomass (Murillo et al., 2012). Over the past decade, such sponge-dominated deep-sea habitats have been characterized as sponge grounds (Hogg et al., 2010).
With the increasing discovery of these important sponge communities in the deep-sea also comes evidence of their destruction, caused mainly by the use of bottom-tending fishing gears (Hogg et al., 2010). Bottom trawling has direct and indirect effects on sessile, filter-feeding sponges. In addition to direct removal, damage, or burying, fishing gear re-suspends bottom sediments (Hogg et al., 2010). Depending on the gear used, seabed structure, and distance from the impact site, particle concentrations of up to 500 mg L–1 can be reached behind the path of a trawl (Durrieu de Madron et al., 2005). In a disturbance experiment in the Mediterranean Sea, the average concentration behind a trawl was measured at 50 mg L–1, with the predominant proportion of re-suspended particles made up of silty clay (grain size 1–63 μm) (Durrieu de Madron et al., 2005). Plumes of particles within the micron scale may stay suspended for days and be transported by oceanic currents over vast distances (Puig et al., 2012). Thus, generated plumes have the potential to indirectly impact habitats kilometers away from the impact site over an extended period of time (Hogg et al., 2010; Mengual et al., 2016; Grant et al., 2019).
Previous studies have found that sponges are negatively impacted by low concentrations of suspended particles. Exposure to suspended particle concentrations between 10 and 95 mg L–1 has resulted in decreased pumping efficiency and filtering capacity in some species (Bell et al., 2015; Grant et al., 2018). Strehlow et al. (2017) showed that the demosponge Ianthella basta (Pallas, 1766) (Ianthellidae) incorporated suspended sediment particles as small as 4.2 μm from the surrounding sea water into its mesohyl. The authors postulated that this particle size is well within the range of bacterioplankton that filter-feeding sponges predominantly utilize as a food source. Its uptake therefore could cause serious deleterious effects on sponge health. At the same time, some sponge species have developed mechanisms to exclude or excrete ingested sediment. These mechanisms include mucus production, exclusion of particles by incurrent pores, closure of oscula and pumping cessation, expulsion of particles from the aquiferous system, and structural component regression to reduce the volume of the aquiferous system and limit pumping and clearance capacity (Strehlow et al., 2017). These active mechanisms require additional energetic expenditures, and it is unknown if sponges can sustain those over long time periods (Bell et al., 2015; Schönberg, 2016a,b; Strehlow et al., 2016).
While the effects of sediment exposure on glass sponges are less understood compared to demosponges, Tompkins-Macdonald and Leys (2008) demonstrated that Rhabdocalyptus dawsoni (Lambe, 1893) (Rossellidae) arrests pumping when subjected to suspended particle concentrations of 36 mg L–1, confirming a response of hexactinellids to suspended particles. An in situ study (Grant et al., 2018) on the reef-forming glass sponge Aphrocallistes vastus Schulze, 1886 (Aphrocallistidae) from the northeast Pacific showed that suspended particles in concentrations as low as 4.4 mg L–1 caused sponges to arrest their pumping with prolonged phases of “coughing” patterns in pumping activities. The arrests and “coughing” patterns are thought to protect the sponge’s aquiferous system from clogging and help remove ingested particles. At the same time, reduced pumping and thus filtering activity limit feeding and waste product excretion and can result in adverse effects on overall metabolism and health of individual sponges and consequently the biogenic habitats they form (Grant et al., 2018).
The hexactinellid sponge V. pourtalesii (Schmidt, 1870) (Rossellidae) is distributed along the continental margin of eastern North America, from the Florida Keys in the southeastern United States to the Scotian Shelf off Nova Scotia, Canada, where it forms extensive sponge grounds between 160 and 200 m depth (Figure 1; Beazley et al., 2018). The densest known aggregations of V. pourtalesii occur in Emerald Basin, a deep-water basin on the central Scotian Shelf, where this species reaches heights (up to 40 cm) and densities (up to 4 individuals per m2) (Maldonado et al., 2020) not observed elsewhere across its distribution range. The presence of V. pourtalesii sponge grounds in Emerald Basin was first noted by fishermen when fishing for pollock and redfish using otter trawl gear (Fuller, 2011; Beazley et al., 2018).
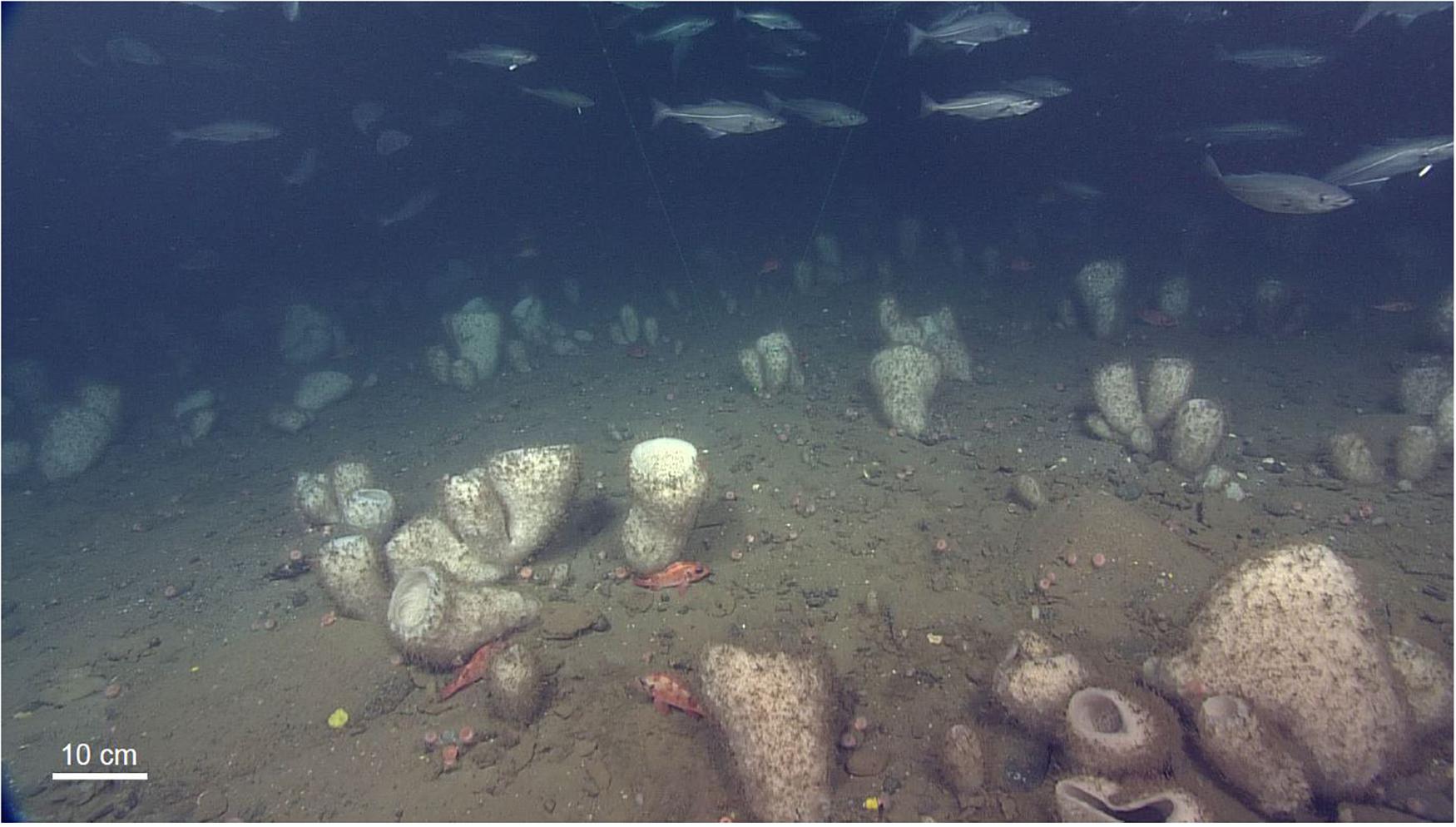
Figure 1. Sponge grounds formed by Vazella pourtalesii in the Sambro Bank Sponge Conservation Area, Emerald Basin, Nova Scotia, Canada. Image is a frame grab from video collected using the ROV ROPOS in 2017. Depth is 155 m, and location is 43°53.6513 N, −63°4.6513 W.
The highest biomass/densities of Vazella pourtalesii from commercial bycatch (where catch biomass up to 5900 kg in a single tow has been reported), research vessel trawl catch data, and in situ camera surveys (described in Beazley et al., 2018) are reported to occur in two localized areas of Emerald Basin: the eastern flank of Sambro Bank, and on a shallower saddle between Emerald Basin’s Main and Northern Basins, to the west of the area known to fisherman as “The Patch” (see Figure 2; Fuller, 2011; Beazley et al., 2018). Conservation of sponge grounds has gained international attention since they were listed as species vulnerable to bottom contact fishing gears by the Food and Agriculture Organization of the United Nations (FAO, 2009) in the international guidelines written in response to the United Nations General Assembly resolutions aimed at protecting vulnerable marine ecosystems. Deep-sea sponge aggregations are included in the OSPAR List of threatened and/or declining species and habitats (OSPAR agreement 2008-6), and regional fisheries management organizations such as the North Atlantic Fisheries Organization have introduced management measures to protect sponge habitats (NAFO, 2021). In Canada, sponge grounds may qualify as sensitive benthic areas, the Canadian equivalent of vulnerable marine ecosystems (DFO, 2017a). In order to protect these dense aggregations from adverse impacts of bottom trawling, in 2013, Fisheries and Oceans Canada (DFO) implemented two sponge conservation areas (the Sambro Bank and Emerald Basin Sponge Conservation Areas, referred to herein as SCAs) prohibiting the use of bottom-tending gears. However, groundfish fishing activities continue to occur almost immediately adjacent to the borders of these two conservation areas (DFO, 2017b), potentially affecting the sponge grounds and abundant megafaunal communities within the SCAs (Hawkes et al., 2019) via re-suspension of sediment.
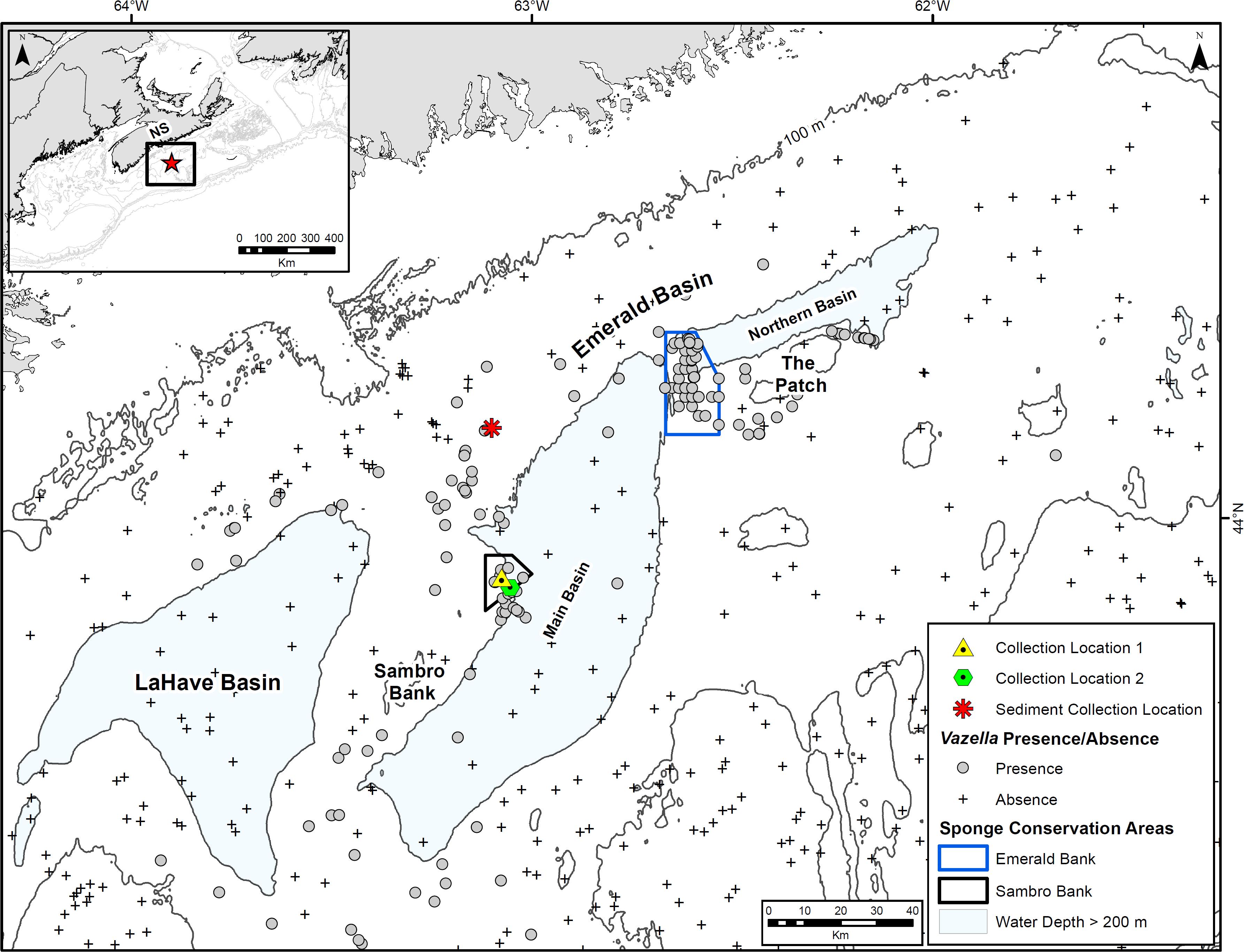
Figure 2. Location of collection sites for ex situ experiments in the Sambro Bank Sponge Conservation Area, Emerald Basin, Nova Scotia. Also shown is the location of sediment collection north of Emerald Basin, and the presence–absence of Vazella pourtalesii from commercial bycatch records, and DFO optical (camera) and multispecies research vessel trawl surveys.
Here, in a laboratory setting, we report for the first time the physiological response of V. pourtalesii to long-term exposure of an ecologically relevant concentration (50 mg L–1) (Tjensvoll et al., 2013; Kutti et al., 2015) of suspended natural bottom sediment, thereby evaluating an indirect effect of bottom trawling activity. Animal performance was quantified by assessing oxygen removal rates and clearance rates for bacterioplankton, thus providing the first insight into the physiological responses of this species to sediment exposure. Subsequent recovery after sediment exposure was evaluated using scanning electron microscopy (SEM) techniques and qualitative observations. Knowledge on the responses of V. pourtalesii to repeated sediment exposure may help provide insight into the biological considerations needed for effective closure area design and management of glass sponge grounds in the northwest Atlantic.
Materials and Methods
Collection of Animals
During an oceanographic mission to the Vazella sponge grounds of Emerald Basin in 2017 (Figure 2; see Beazley et al., 2017 for mission details) in support of the EU-funded Horizon 2020 project SponGES, the ROV ROPOS1, a 40 hp Science/Work Class ROV owned and operated by the non-profit Canadian Scientific Submersible Facility (CSSF), was deployed from the Canadian Coast Guard Ship Martha L. Black to collect specimens of V. pourtalesii for ex situ experimentation. A total of 23 sponges were collected over four separate deployments of ROPOS, three of which occurred in the center of the Sambro Bank SCA at approximately 160 m depth (Collection Location 1 in Figure 2), and one that spanned across the southern border of the closure at approximately 217 m depth (Collection Location 2, Figure 2). During collection, the manipulator arm of ROPOS was carefully used to collect sponges by their substrate attachment point (pebbles and cobbles) and placed them in the ROPOS “biobox” where they remained submerged upon recovery. Once onboard, the sponges were transferred in a submerged state to an insulated 500 L polyethylene holding tank inside a refrigerated shipping container. The sponges were placed in individual compartments (10 cm by 10 cm) of a grid at the bottom of the holding tank to help keep them stationary. Subsurface water (<5 m) was pumped into a tank on deck using a portable pump, which was then distributed to a tank inside the refrigerated container, chilled to 9 °C, and then slowly pumped via a peristaltic pump (1.2 L min–1) to the holding tank containing the sponge specimens. The chilled water tank was refilled twice a day, resulting in four water exchanges per day.
Sampling deep-water fauna for experimentation is a logistical and technological challenge. Sampling via the ROV’s manipulator arm required delicate and precise handling of sponges within the target size range. Due to this time-consuming process, along with impediments to sampling from inclement weather, only a limited number of individuals could be collected. At the same time, V. pourtalesii has been recognized as a vulnerable species (Beazley et al., 2018) and as a result, removal of animals for experimentation should be kept to a minimum. Due to these limitations and challenges, the study was performed with a minimum number of individuals to account for independent replication.
Acclimatization to ex situ Experimental Conditions
The sponges were transferred to facilities at the Bedford Institute of Oceanography (BIO), Nova Scotia, Canada, where they were maintained in a 500 L holding tank supplied with coarsely filtered saltwater from the adjacent Bedford Basin, the intake of which is 200 m from shore and at a depth of 17 m at low tide (∼3 m off bottom). Salinity was 30.5 ± 0.2 PSU over the course of 10 months. To ensure full recovery from sampling and transportation, sponges were acclimatized under the above conditions for 7 months. A non-axenic phytoplankton culture was routinely added to the sponge holding system to stimulate bacterial growth in the header tank during this time period. Two to three times per week, 3–7 L of cultured phytoplankton at 1–5 × 106 cells mL–1 was added to the header tank that supplied the sponge holding tank.
Collection of Sediments
In order to emulate the natural sediment regime of the Vazella sponge grounds, sediment for use in the exposure trials was collected 22 nautical miles north of the sponge collection locations in Emerald Basin (Figure 2). There, a Van Veen grab sampler was deployed at 170 m depth until 100 L of sediment was collected. The surficial sediments here are described as LaHave Clay, a loosely compacted silty clay that is mainly confined to the basins and depressions of the Scotian Shelf (King, 1970). LaHave Clay overlays Emerald Silt and Scotian Shelf Drift (i.e., glacial till), the latter of which the densest aggregations of V. pourtalesii are most associated with (Beazley et al., 2018). This location was chosen due to its proximity to several presence records of V. pourtalesii. Prior to exposure to the sponges, the collected sediment was sieved to a fraction < 63 μm in the laboratory and kept cool (4°C).
Suspended Sediment Exposure
Of the 23 sponges collected, three perished during the 7-month acclimatization period, leaving 20 specimens for experimentation. Numbers from 1 to 20 were assigned to the sponges for identification throughout the experiment. After acclimatization, 10 40 L tanks with a flow-through sea water supply were set up as experimental units for replication between two treatment groups. Sea water (9°C) was supplied to each individual tank with a flow rate of ∼0.5 L min–1. Water temperature in the experimental tanks was stable over the course of the experiment (mean = 8.96 ± 0.05 °C). Equally-sized sponges were distributed randomly between two treatments, with each of the control and treatment (i.e., sediment-exposed) groups consisting of 10 individuals each, with two individuals per tank (Figure 3).
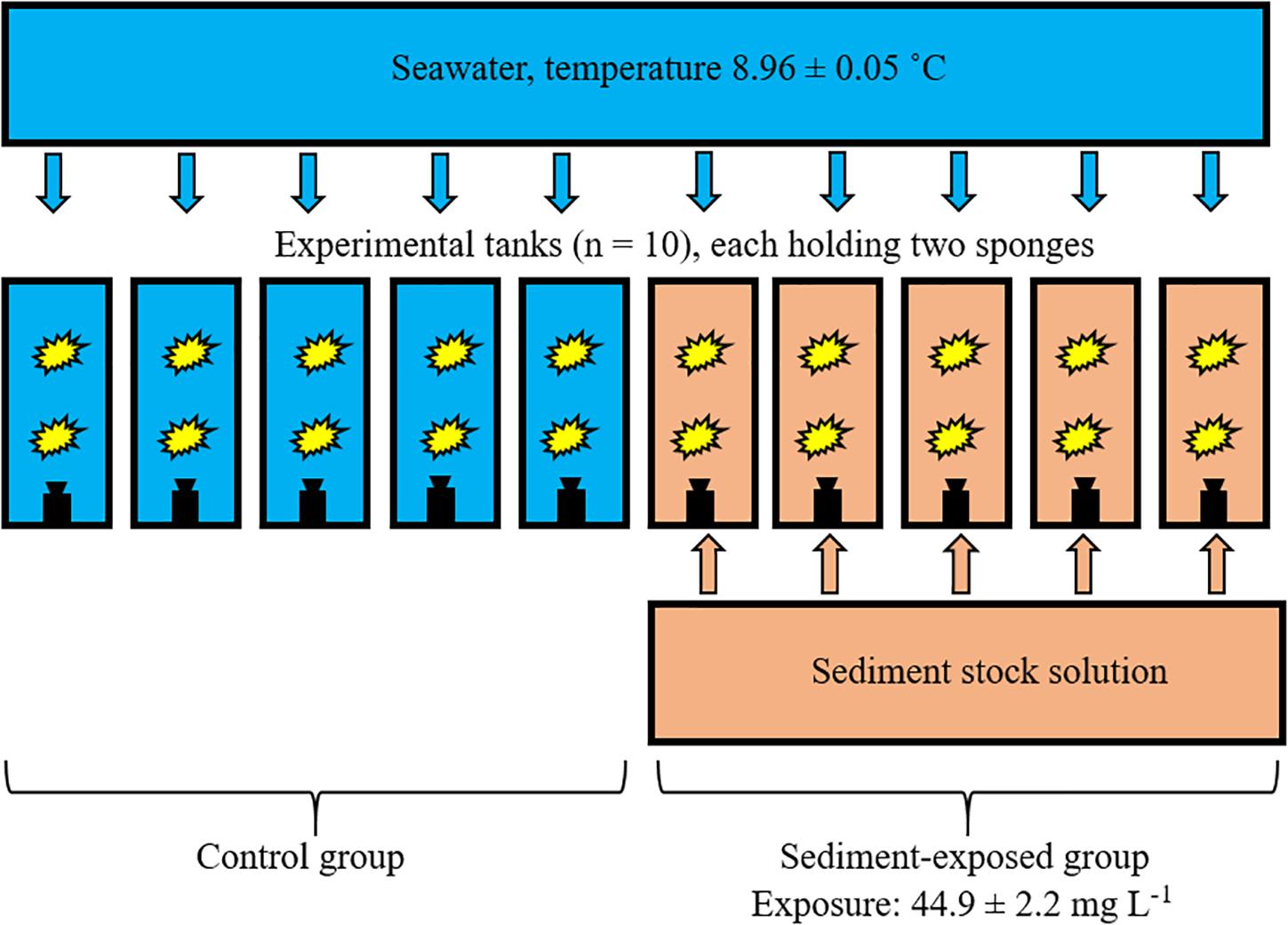
Figure 3. Experimental design used in this study. Ten individual tanks, each holding two sponges (yellow shapes), were supplied with sea water. Each tank was equipped with a pump (black shapes). Five tanks received dosages of a sediment stock solution for 12 h per day.
Prior to the start of the experiment, sponges in the control were unintentionally exposed to air bubbles that potentially formed due to temperature-induced off gassing of oxygen-saturated seawater (temperature difference of 0.5 °C from the header tank to the experimental unit). Upon notice of microbubbles, sponges were returned to the 500 L maintenance tank until the experimental setup had been improved. Sponges assigned to the sediment-exposed group remained in the 500 L holding tank at all times during this time period. Once the experimental setup was operational, sponges were placed back in their respective tanks the experiment commenced. No deleterious effects of microbubble exposure were evident in the control sponges upon the start of the experiment. We subsequently acquired control data on the respiration and clearance rate from colleagues working on this same species in the same aquaria facilities at another time (Bart et al., 2020) in order to compare these rates with our potentially compromised control sponges.
The sediment-exposed group received a concentration of suspended natural bottom sediments of 50 mg L–1 for 12 h per day for 21 days, while the control group remained untouched. The 12 h exposure cycle was chosen to emulate the natural tidal oscillation experienced off Nova Scotia. Each of the five sediment treatment tanks received pulses of high concentration sediment stock solution (Figure 3). Pulses of stock solution were delivered by five membrane pumps (EW-F31VC-20EPF5, IWAKI). Average frequency was one pulse of 1-min length per 5 min, resulting in 25 mL stock solution being added per pulse to each of the tanks in the exposed group. Aquarium pumps (CompactON 300, Eheim) kept dosed sediment volumes in suspension. The end concentrations of suspended sediment and particle size distribution in each of the five tanks were monitored with a particle analyzer (LISST-100X, Sequoia) at days 0, 7, 14, and 21. Settled sediment was removed from the bottom of each tank by careful siphoning after each exposure cycle of 12 h.
Physiological Measurements
The physiological performance (respiration rate and bacterial clearance rate) of V. pourtalesii assigned to the control and sediment-exposed groups was assessed with chamber-based incubations after 7, 14, and 21 days. Before each incubation, sediment-exposed sponges were given a 3 h recovery period after the last pulse of sediment. During this period, the tank volume was turned over by fresh seawater approximately four times, so that sponges were exposed to clear water prior to the start of each incubation. The bottom plate of the incubation chamber was put in its respective tank, and a sponge was placed on the plate. Sponges were left to recover for 1 h before an acrylic chamber that sealed to the bottom plate was placed over each sponge. The lid of the chamber was equipped with a stirrer for circulation and a dissolved oxygen sensor (HQ30D equipped with LDO sensors, Hach) calibrated to 100% saturation according to the manufacturer. Dissolved oxygen concentrations were measured every minute over 4 h, and respiration rates (R) in μmol O2 h–1 gDM–1 were calculated as outlined by Tjensvoll et al. (2013) using the following equation:
where c1 and c2 are the concentration of dissolved oxygen at start and end of the incubation in μmol O2 L–1, t is the time of the incubation in hours, Vnet is the volume of the incubation chamber after subtracting the volume of the respective sponge in mL, and gDM is the total dry mass of the incubated animal in grams. Wet mass was measured on a scale after taking the sponge out of the water and allowing the water to drain for 10 s. Volume of individual sponges was measured by water replacement in a graded beaker prior to sampling for microscopy approaches (see section “Recovery Potential and Electron Microscopy”). To make the rates assessed in this study comparable to published datasets, we used the conversion factor (mL:gDM = 5.2) from Bart et al. (2020) to calculate dry mass from sponge volume.
Water samples were drawn from the chamber at the beginning and the end of the 4 h incubations via tubes inserted through the lid. Samples for bacterioplankton quantification were fixed with glutaraldehyde (end-concentration 0.5%) for 10 min before flash freezing in liquid nitrogen and −80 °C storage. Bacterial concentration in the water samples was assessed by flow cytometry (Brussaard et al., 2010) and clearance rates for bacterioplankton in mL water cleared per mL sponge volume per minute (mL mL–1 min–1) were calculated as outlined by Robertson et al. (2017) using the following equation:
where Vnet is the volume of the incubation chamber after subtracting the volume of the respective sponge in mL, t is the time of the incubation in min, nstart and nend are the concentrations of bacterioplankton at start and end of the incubation in counts mL–1, and Vsponge is the total volume of the incubated animal in milliliters. Empty chamber incubations (n = 2 per treatment group) without a sponge were performed twice throughout the experiment to account for background respiration and non-sponge related dynamics in bacterioplankton. The average of the four empty chamber incubations was subtracted from the sponge incubations of the respective treatment.
Data Analysis
Statistical tests were performed with GraphPad Prism 8 Version 8.2.1(441), August 20, 2019 using a general linear model analysis of variance (Sediment; two levels nominal 0 or 50 mg L–1, n = 5) with multiple comparisons. For analysis, measurements of individual sponges were averaged per tank resulting in five independent replicates per treatment. We tested for tank effects prior to the analysis (Kutti et al., 2015), and influence of tank was identified as low (Control group: clearance rate: p = 0.12; respiration rate: p = 0.14; Sediment-exposed group: clearance rate: p = 0.78; respiration rate: p = 0.20). Values are given in mean ± standard deviation (SD) if not stated otherwise.
Recovery Potential and Electron Microscopy
After 3 weeks of exposure to suspended sediment, we followed the potential recovery of exposed individuals of V. pourtalesii for up to 38 days after the last exposure, with unexposed sponges as the control group. In order to determine the level of clogging by sediment in the aquiferous system, structural components from sediment-exposed sponges were collected for examination using SEM techniques. Due to financial limitations, analysis of structural components of all sponges was unfeasible and only a minimum number of replicates were analyzed to account for individual differences between sponges. Three hours after the last exposure to sediment (considered day 0 of the recovery phase), one sediment-exposed sponge was removed from its tank and subsampled by extracting a ∼1 cm3 sample through the body wall of the lower and upper halves of the sponge (specimens were halved at their mid-point based on total height). Two control sponges were sampled after 3 and 12 days of the recovery phase. On the final day of the recovery phase (day 38), two sediment-exposed sponges (Sponges 14 and 19) and one control (Sponge 8) were subsampled for examination using SEM to determine if sediment was still present in their body.
Subsamples were preserved in a 1:4:5 ratio of 25% glutaraldehyde, 0.34 M sodium chloride (NaCl), and 0.4 M phosphate-buffered saline (PBS) (i.e., “Fixative 1”). The Fixative 1 solution was changed after 0.5 h, and the samples were stored in 4°C for ∼2 months. The final step for SEM fixation involved rinsing the samples of Fixative 1 using a 1:1 ratio of 0.4 M PBS and 0.6 M NaCl and fixing the samples in a 1:1 ratio of 4% osmium tetroxide and 0.6 M PBS (i.e., “Fixative 2”) for approximately 2 h. Samples were then rinsed of Fixative 2 three times in distilled water for 10 min each and dehydrated through a series of ascending alcohol concentrations diluted from 100% anhydrous ethyl alcohol: 50 and 70% alcohol (10 min each), 80, 90, 95, and 100% alcohol (3 × 10 min each). In order to create even surfaces from which the intact aquiferous system could be observed, the 1 cm3 subsamples were freeze fractured in liquid nitrogen. Fractured pieces were then dried using liquid carbon dioxide in a Leica EM CPD300 (Critical Point Dryer) at the Scientific Imaging Suite at Dalhousie University, Halifax, Nova Scotia.
After critical point drying, samples were mounted on SEM stubs using a carbon-based paste, and sputter coated using a Leica EM ACE200 vacuum coater at a speed of 30 mA for 325 s, which equated to a 25 nm-thick coating of gold/palladium. To determine the degree of clogging, the coated samples were initially viewed and imaged using a Zeiss 1455VP scanning electron microscope operating with an acceleration voltage of 20 kV, and SmartSEM V05.05 SEM operating software.
Initial SEM examination of the sediment-exposed sponge sampled on recovery day 1 (Sponge 16) revealed poor results from freeze fracturing, likely due to the high density of spicules. Instead of freeze fracturing with liquid nitrogen, the 1 cm3 subsamples collected on all subsequent days were sectioned into smaller pieces using a sharp razor to create both cross sections across the atrial surface, and longitudinal sections through the body wall from the pinacoderm to atrial surfaces. Both the longitudinal and cross sections were fixed for SEM according to the techniques described above.
To identify the potential presence of accumulated particles in sediment-exposed sponge structural components, the elemental composition was examined using SEM/energy dispersive X-ray spectroscopy (EDS) techniques. Samples were examined and imaged using a Hitachi S-4700 Cold-Field Emission SEM operating at 10 kV (also at Dalhousie University). The atomic weight as a percentage of total weight was calculated for each of four or five randomly chosen point locations over the unknown matter. Samples of the matter were taken using an attached 80 mm2 X-Max EDS detector (Oxford Instruments, Concord, MA, United States) and associated Inca software. All elements were analyzed (normalized). Given that the mounted structural components of V. pourtalesii were not flat, polished, or homogeneous, which are requirements for standardized quantitative analysis using EDS, we chose to convert the atomic weight information into elemental presence/absence. Due to the use of carbon-based mounting media, the presence of carbon in the samples must be discounted, as well as the presence of osmium, which is likely a remnant of the fixation process. The elemental composition of the sediment used in the experiment was also examined using SEM/EDS techniques to serve as a comparison to the matter found in the sponge’s structural components. Sediment (unfixed) was mounted on an SEM stub using carbon-coated double-sided tape, and gold/palladium coated to a thickness of 15 nm.
Results
Survival
All 10 sponges in the treatment group survived repeated exposure to suspended sediment over the course of the 21-day experiment (indicated by observations of active pumping and by respiration and clearance rate assessments) and no structure necrosis or other signs of decreased health status were observed in the treatment group over the course of the experiment. Initially, sediments accumulated only on the long spicules of the exposed individuals, but with repeated exposure, the atrial surfaces eventually became covered in sediment particles and turned brown in color (Figure 4). In the control group, three sponges showed signs of necrosis (gray/black colored structural components) after 10 days in the experimental tanks and eventually perished (Supplementary Figure 1). These sponges deteriorated relatively quickly (within 3 days after the initial signs of discoloration), and no signs of necrosis were evident in the remaining sponges throughout the 21-day experiment. It cannot be ruled out that the mortality in the control group was induced by a short exposure to micro-bubbles at the beginning of the experiment. Thus, all sponges from the control group may have been compromised in their physiological performance. For this reason, data from the control group evaluated in this study were augmented by a published dataset (Bart et al., 2020) on the physiological rates of V. pourtalesii. Respiration and clearance rates of V. pourtalesii described by Bart et al. (2020) (n = 7) were assessed in a similar manner as in this study (chamber-based incubations) and were performed in the same aquaria facilities.
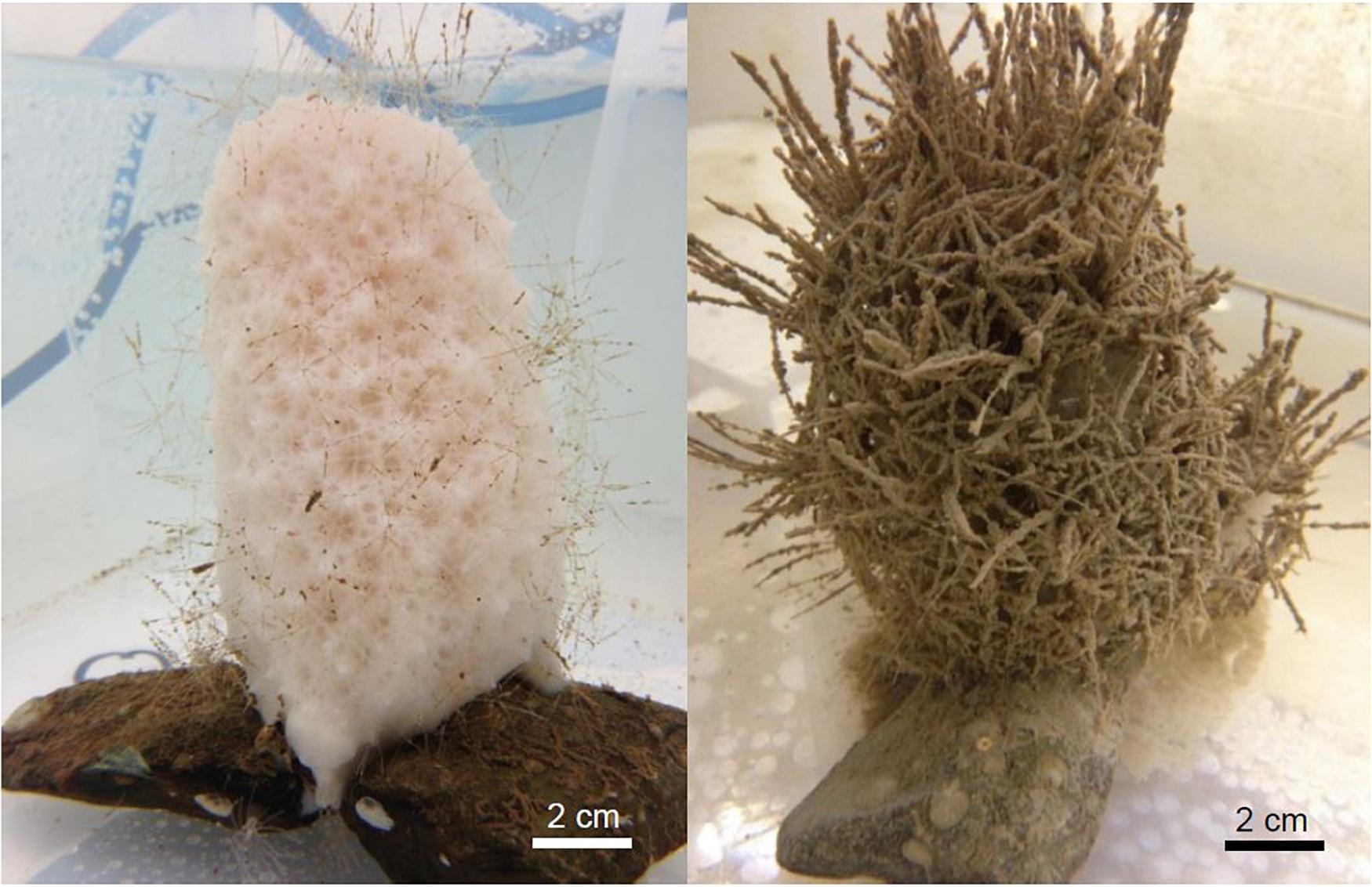
Figure 4. Individuals of Vazella pourtalesii from the control (left) and the exposure (right) group after 7 days in the experiment. The long spicules and atrial surface of the exposed sponges eventually became densely covered with sediment particles.
Suspended Sediment Exposure
The average suspended particle concentration in the treatment tanks during the 12 h exposure phase over the course of the 21-day experiment was 44.9 ± 2.2 mg L–1. Particle concentrations at the beginning of the experiment (33.6 ± 2.5 mg L–1) were below the targeted threshold of 50 mg L–1, but steadily increased up to 56.9 ± 2.1 mg L–1 at day 21 (Figure 5). The control treatment had an average suspended particle concentration of 1.9 ± 0.8 mg L–1. Particles < 6 μm were prevalent in the suspended sediment representing a fraction of 73% of the total concentration (mg L–1). A fraction of 10% was represented by particles as large as ∼184 μm (Figure 6).
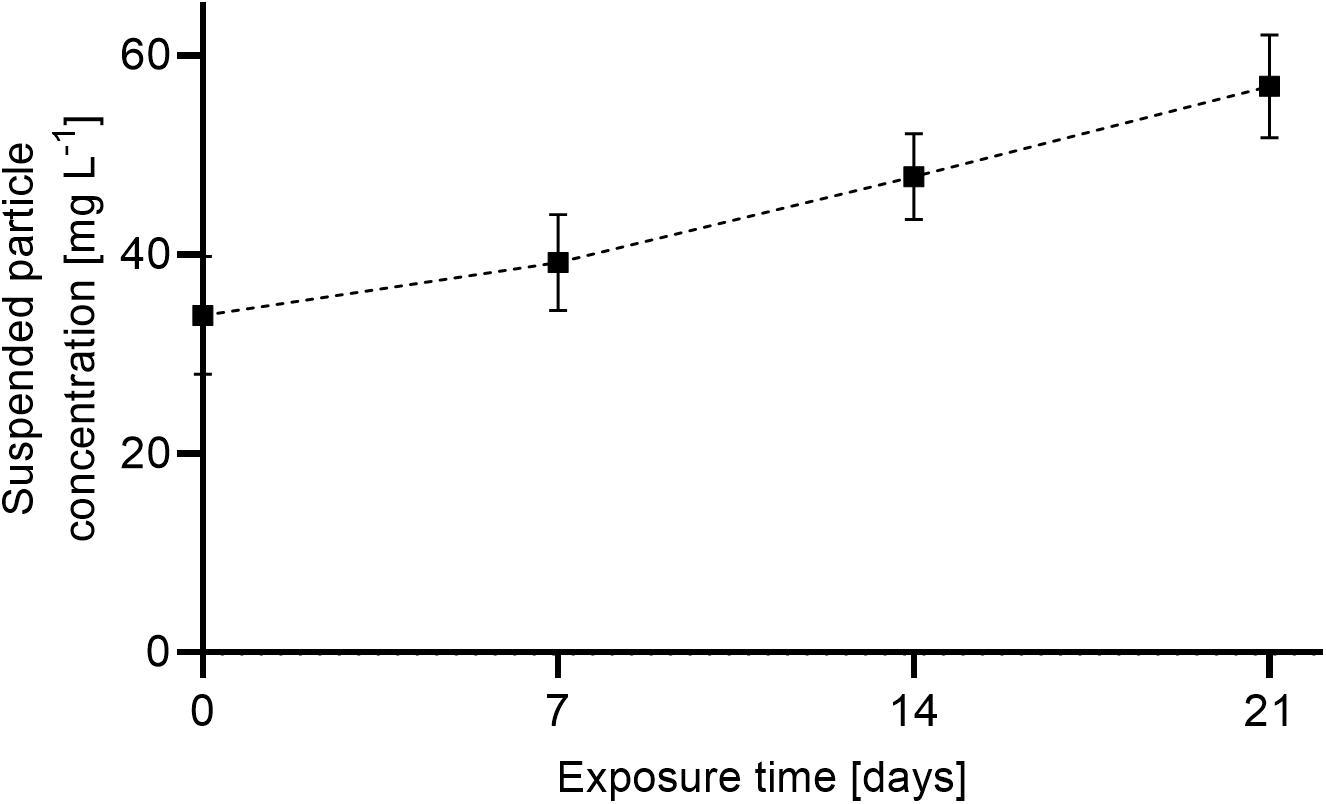
Figure 5. Average of end concentrations ± SD of suspended sediment in exposure (n = 5) and control tanks (n = 5) throughout the experiment. Overall average exposure level was 44.9 ± 2.2 mg L–1. Suspended sediment concentration in the control tanks was assessed once in the beginning of the experiment (1.9 ± 0.8 mg L–1).
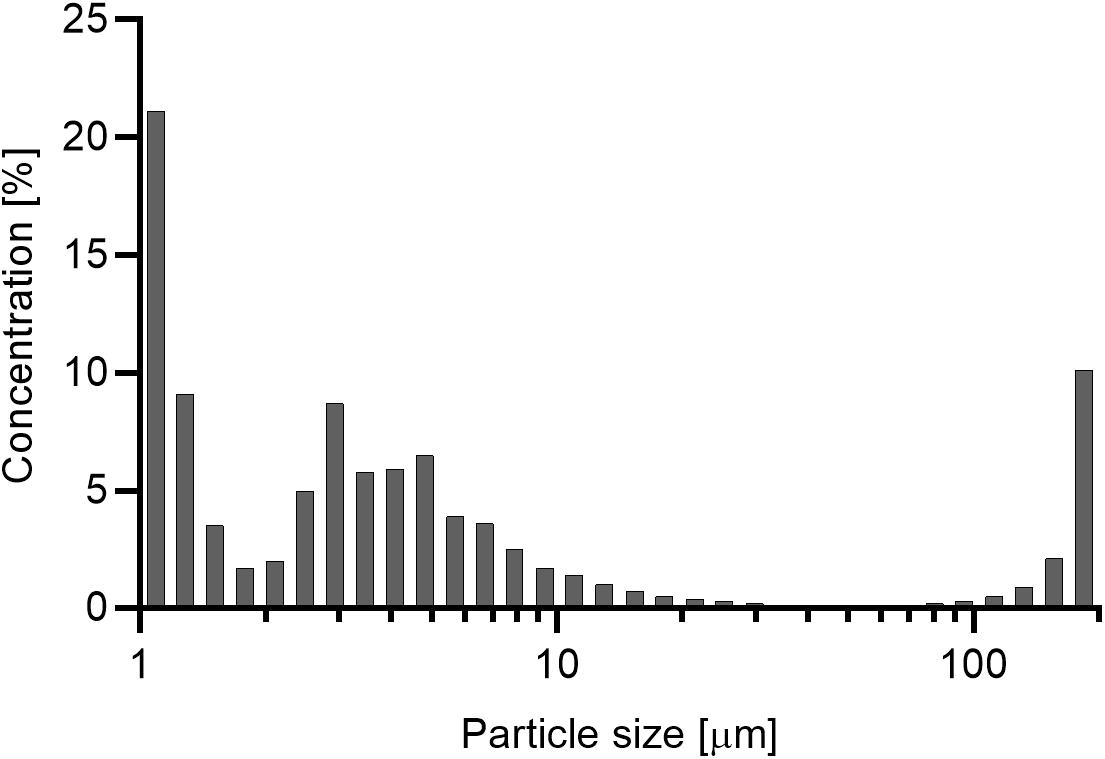
Figure 6. Percentage of concentration per size class of the suspended sediment in end-concentration. 73% of suspended particles are represented in the size class from 1 to 6 μm.
Physiological Performance
The respiration and clearance rates of V. pourtalesii in the two treatment groups measured during the chamber incubations on days 7, 14, and 21 are summarized in Table 1. Decrease in oxygen concentration in the closed chambers was linear over time. Respiration rates of individuals from the literature control and sediment-exposed groups were comparable at each of the three measured time points (days 7, 14, and 21; Figure 7). However, respiration rates increased slightly in the sediment exposed group over the 21-day experiment, with rates ranging from 1.89 ± 0.15 μmol O2 h–1 gDM–1 at day 7 to 2.66 ± 0.51 μmol O2 h–1 gDM–1 at day 21. No effect of sediment treatment or time of exposure was detected [F(3,18) = 1.96, p = 0.156]. Clearance rates (Figure 8) significantly differed between the sediment-exposed and the control group from Bart et al. (2020) on days 14 and 21 (p = 0.028 and p = 0.006, respectively; see Table 1). Clearance rates in the sediment-exposed group declined over time with rates ranging from 0.97 ± 0.17 mL mL–1 min–1 at day 7 to 0.53 ± 0.15 mL mL–1 min–1 at day 21. After 14 days of sediment exposure, clearance rates differed significantly from the control group and continued to decrease until the last assessment after 21 days [day 14: F(3,18) = 6.40, p = 0.028: day 21: F(3,18) = 6.40, p = 0.006].

Table 1. Overview of the assessed physiological parameters in Vazella pourtalesii under elevated concentrations of suspended particles (Sediment, n = 5) and the literature control group (n = 7) (Bart et al., 2020).
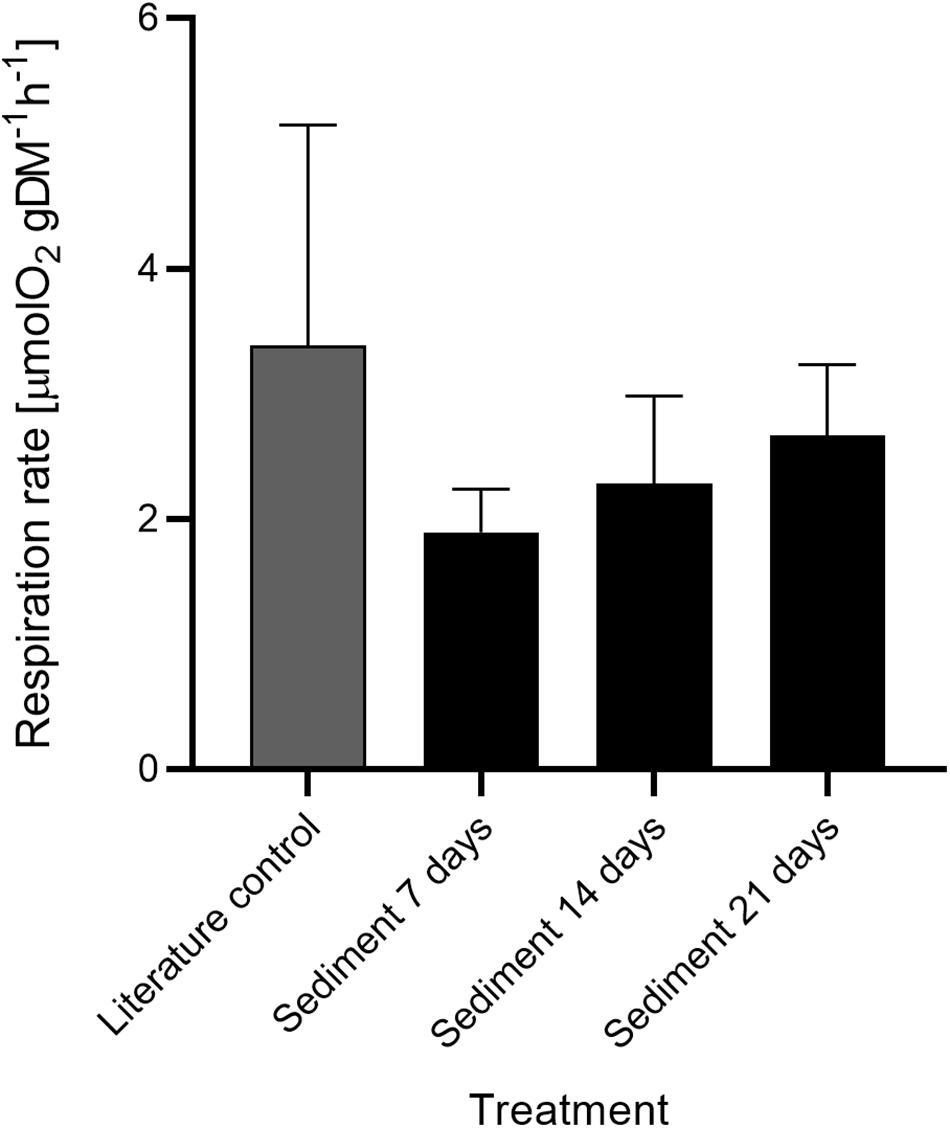
Figure 7. Respiration rate ± SD of Vazella pourtalesii in the treatment (n = 5 per timepoint) and literature control group (n = 7) (Bart et al., 2020) over time.
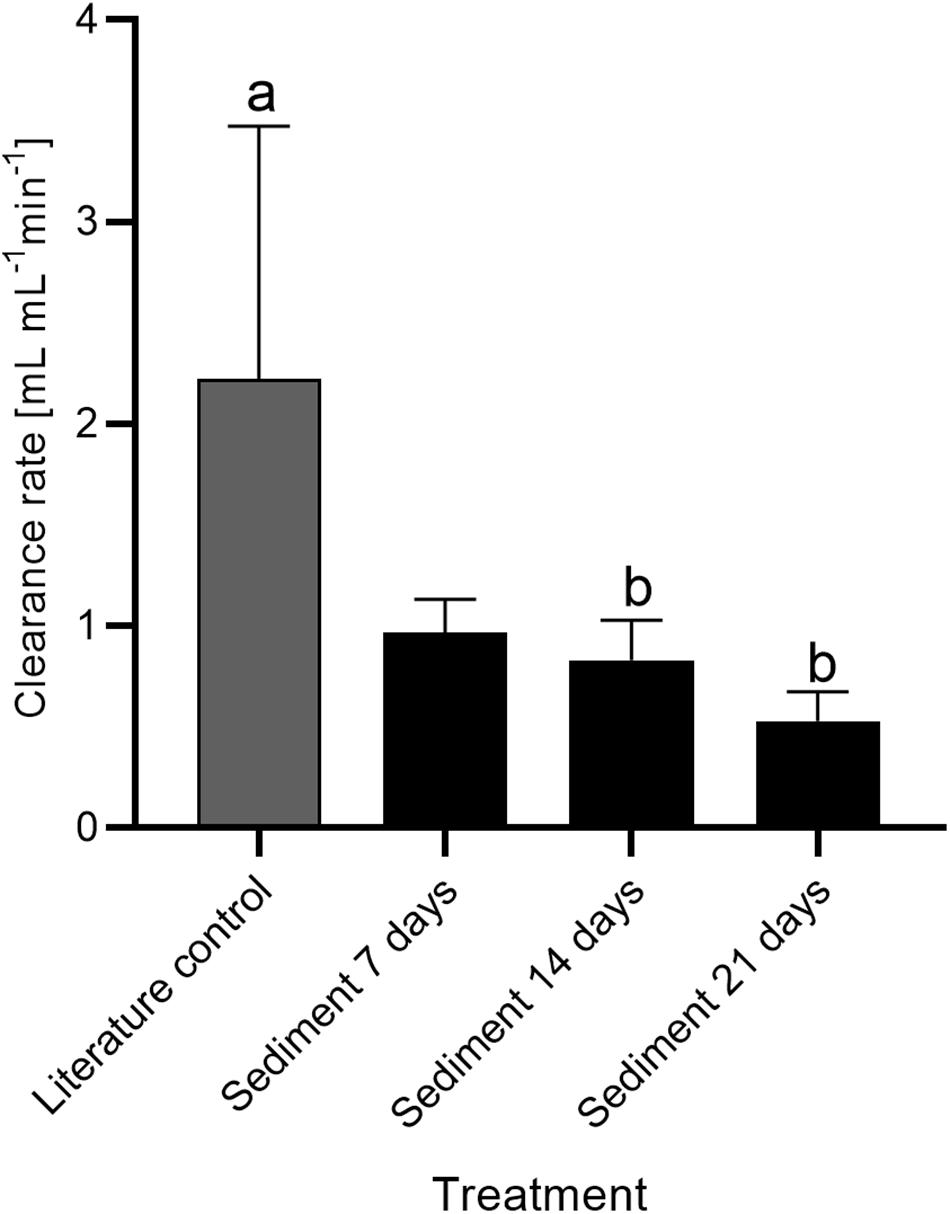
Figure 8. Clearance rate ± SD of Vazella pourtalesii in the sediment treatment group over time compared to a literature control (Bart et al., 2020). Pairs of letters (ab) indicate 2 cm significant differences (p < 0.05) among means (n = 5 per sediment treatment and n = 7 for literature control). Common letters indicate that no significant difference was detected.
Observations of Accumulated Material After Sediment Exposure
After each 12 h cycle of sediment exposure, settled sediment was removed from the bottom of each tank. Within 30 min after sediment removal, flocculate matter, possibly representing sloughed mucous-coated sediment, was observed accumulating next to the sponges (Figure 9). Particles had a flocculated appearance; small pellets of sediment clumped together by a translucent material and settled on the bottom of the aquaria. This matter was not present at the bottom of the tanks of the control group. Video observations of outflow of sediment-exposed individuals showed that the large accumulated particles were expelled with high velocity from the osculum (see Supplementary Video 1).
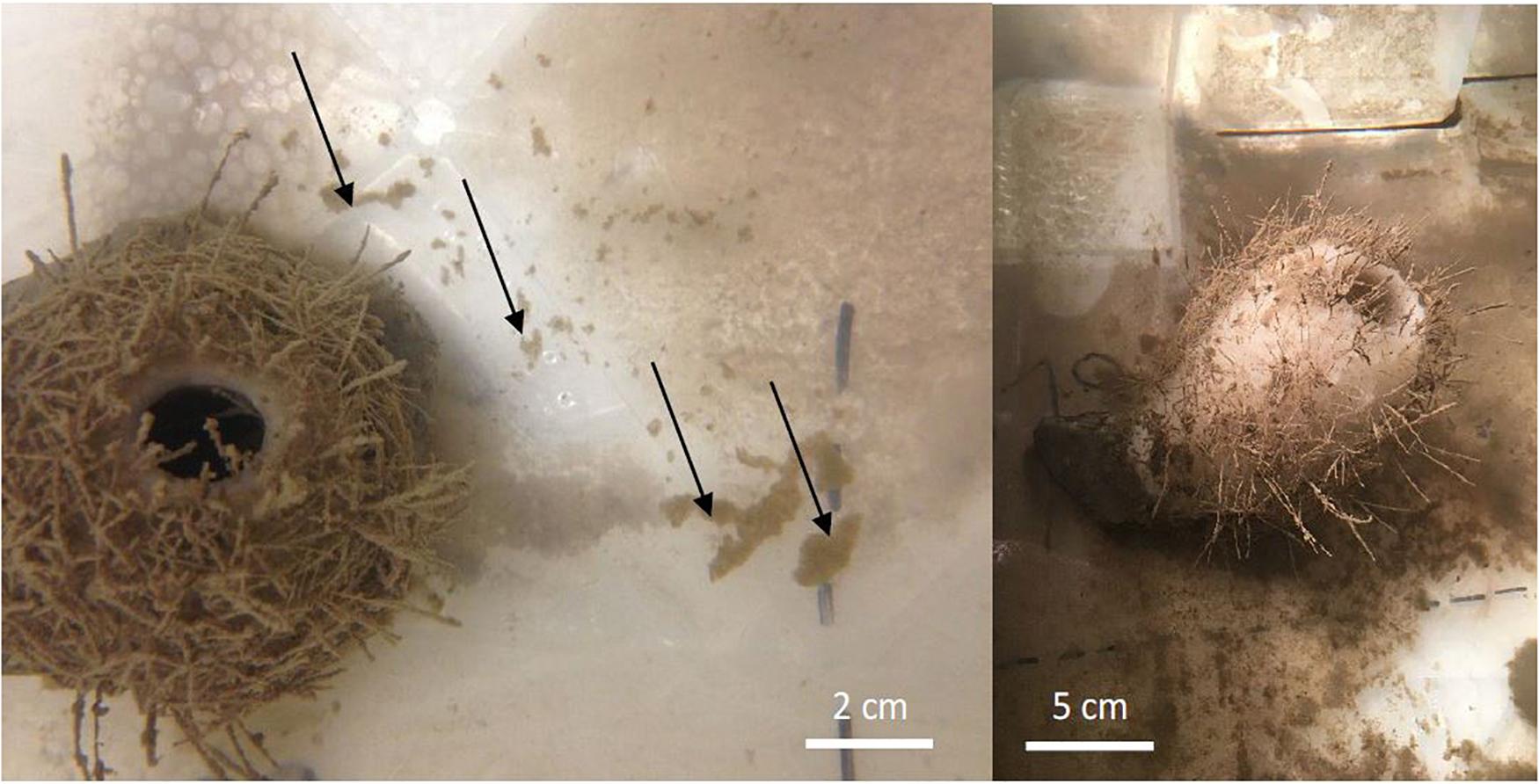
Figure 9. Left: Vazella pourtalesii in top view with flocculated matter (black arrows) accumulated in the tank after initial removal of settled sediment particles Right: V. pourtalesii in experimental tank with accumulated sediment particles on the tank bottom after 12 h of exposure.
Recovery Potential and Scanning Electron Microscopy Examination
The atrial surfaces of most individuals in the sediment-exposed group returned to their pre exposure state approximately 14 days after last exposure. Of the three sponges left to recover to day 38, all appeared to have cleared their pinacoderm of sediment completely. Two sediment-exposed sponges appeared to be in the process of dying, with dark sections of structural components indicating necrosis. Another sediment-exposed sponge (Figure 10), while appearing to nearly recover, self-dislodged from its basal attachment and floated to the top of the tank on day 38 and was dissected. Some structure necrosis was also evident in this individual.
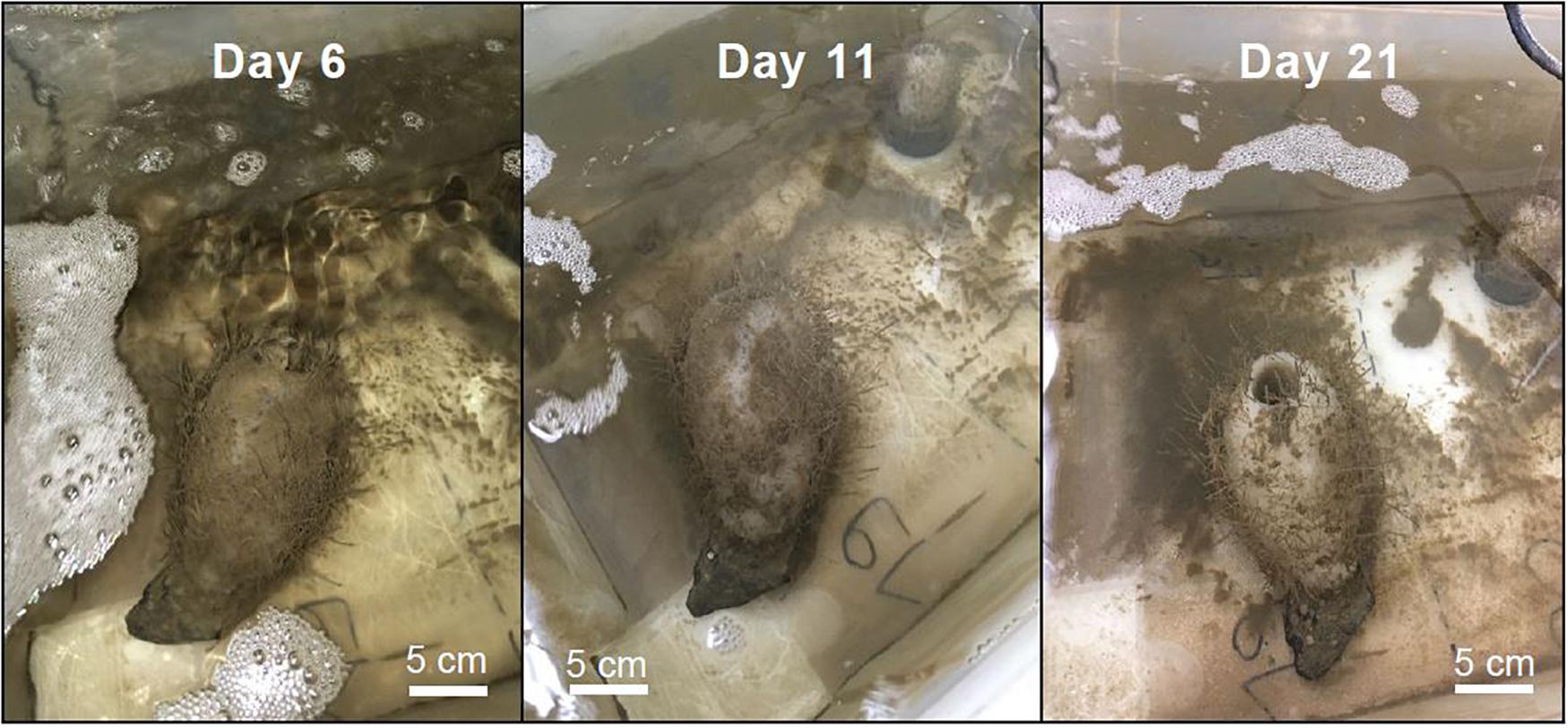
Figure 10. Succession of recovery of a sediment-exposed sponge at 6, 11, and 21 days after sediment exposure stopped. This sponge was sampled for SEM/EDS analysis 38 days after sediment exposure.
Scanning electron microscopy/EDS analysis (Supplementary Table 1) revealed that the sediment used in the exposure experiment consisted of a wide variety of grains and elemental composition, with textural differences between grains observed. The presence of aluminum, silicate, potassium, iron, magnesium, and chloride was evident in the grains. The presence of both calcium and silicate suggests that the sediment grains are both calcareous and siliceous in origin. These grains were largely absent from the three control sponges sampled, where the observed matter was comprised mostly of carbon (although note contamination from mounting media) and oxygen.
Due to the poor results of freeze fracturing and manual razor sectioning of the structural components, observations of the exact location of sediments within the aquiferous system of the sponges were not possible. Nonetheless, observations on the composition/origin of matter observed among the spicules of the mesohyl were made to assess recovery. The elemental composition of the matter observed within the mesohyl of a sediment-exposed sponge (sampled on day 1 of the recovery phase) was most similar to that of the two analyzed sediment samples. Here, iron, aluminum, magnesium, and potassium were all constituents of the sample, suggesting the presence of sediments inside the sponge. Examination of the sediment-exposed sponges (Sponges 14 and 19) sampled on day 38 revealed an elemental composition more similar to control Sponge 8 (sampled on day 38) than sediment-exposed Sponge 16 and the two controls. For sediment-exposed Sponge 19, the observed matter was comprised of carbon, nitrogen, oxygen, sodium, biogenic silica, aluminum, and sulfur. The sulfur was possibly related to the decaying state of the sponge prior to sampling.
The similar elemental composition found in the sediment-exposed and the control sponges (longitudinal sections), and the lack of iron, potassium, aluminum, and calcium, which were indicative of the sediments, indicate that sediment-exposed individuals had mostly cleared the sediment from their structural components over the course of the 38-day recovery period. However, observations of the cross-section sample from sediment-exposed Sponge 14 revealed the presence of matter with a similar elemental composition to that of the sediment, suggesting high variability in the location of sediment within the body of the same individual.
Discussion
Physiological Response of Vazella pourtalesii to Natural Suspended Sediment
Under the laboratory conditions of this study, V. pourtalesii survived repeated exposure to seawater with particle concentrations up to 57 mg L–1 over the course of 21 days. There was no difference in respiration rates between the sediment-exposed and literature control group throughout the experiment. These findings are in line with experimental work on deep-water demosponges exposed to a similar sediment concentration as used in this study. For example, Kutti et al. (2015) found that oxygen consumption rates in Geodia barretti Bowerbank, 1858 (Geodiidae) remained stable over time when exposed to natural suspended sediment (50 mg L–1) for up to 50 days, indicating that this species is adapted to cope with periods of higher natural water turbidity.
However, in this study, V. pourtalesii individuals exposed to sediment expressed reduced bacterial clearance rates after 14 days of exposure. In situ and ex situ studies showed that some species of glass sponges arrest pumping when exposed to different concentrations of natural sediment, whereas others do not. For instance, the glass sponge A. vastus maintained pumping during exposure to sediment concentrations up to 36 mg L–1 in an aquaria-based study (Tompkins-Macdonald and Leys, 2008), while Grant et al. (2019) found that concentrations as low as 2.8–6.4 mg L–1 caused arrests in the glass sponge R. dawsoni from the Hecate Strait reef complex, but no arrests were observed in Farrea occa Bowerbank, 1862 (Farreidae) when exposed to similar concentrations as reached in our study (57 mg L–1). Grant et al. (2018) reported short-term arrests of pumping activities in glass sponges as a response to elevated particle concentrations. These reported arrests never exceeded intervals of ∼80 min, likely to avoid oxygen depletion in the sponge’s structural components. While we have not directly measured or observed whether short-term cessation in pumping occurred in our experiment, the increasing brown coloration of the sponges over the course of the experiment, as well as the presence of sediments in the structural components of exposed V. pourtalesii individuals as indicated by SEM, suggests that the animals were actively pumping during the 12 h exposure cycles.
Sponges respond to sediment stress via closure of incurrent pores (the ostia) and osculae and the reduction of the aquiferous system via structure regression. These morphological responses result in the cessation of pumping (Tompkins-Macdonald and Leys, 2008). In addition, expulsion of particles from the aquiferous system, and mucus production (Strehlow et al., 2017) have been observed after sediment exposure. Particles up to 1 mm in diameter were observed in the excurrent flow of the sponges after sediment exposure (Supplementary Video 1, osculum diameter ∼2 cm). Particles had a flocculated appearance, and small pellets of sediment clumped together by a translucent material and settled on the bottom of the aquaria. No accumulation of such particles was observed at any time throughout the experiment in the control sponges. Excretion of pellets of indigestible particulate matter is linked to an increase in mucus production, a known stress response exhibited by both demosponges (Strehlow et al., 2017; Grant et al., 2019) and hexactinellids (Kahn et al., 2018). However, mucus production is an energy-demanding process (McGrath et al., 2017), and prolonged exposure to suspended natural sediment likely has an adverse effect on a sponge’s ability to maintain this coping mechanism. A decrease in bacterial clearance rates with sediment exposure, as observed in our study, is indicative for sediment induced cessation of pumping. If the sponges reduce their pumping activity, energy input will decrease and hence, continued coping with sediment will start to consume energy at the expense of other processes. Thus, processes such as growth, structural component maintenance (Shore, 1971; De Goeij et al., 2009), reproduction, and cell renewal (Bell, 2002; Alexander et al., 2014) might be compromised under prolonged events of elevated suspended particle concentrations. This could result in an energy deficiency and adverse effects on overall sponge health (Grant et al., 2019).
Recently, V. pourtalesii has been shown to predominantly utilize dissolved organic carbon to fuel its metabolic requirements (Bart et al., 2020). It remains to be investigated whether this species can increase the uptake of dissolved organic matter to counteract the reduced uptake of bacterioplankton resulting from repeated sediment exposure. Also unknown is to what extent pathways for dissolved organic matter uptake in V. pourtalesii are dependent on pumping and as such affected by the presence of elevated levels of natural suspended sediment. Additionally, a decrease in pumping could result in a reduced capacity to evacuate indigestible sediment particles and accumulation within the sponge’s structural components.
Recovery After Sediment Exposure
Individuals left to recover for up to 38 days after last sediment exposure appeared to clear their pinacoderm of sediment and were similar in appearance to sponges in the control group. However, SEM/EDS techniques indicated that after 38 days, sediment particles were still present in certain structural component sections of one individual, suggesting high variability in the location of sediment within the structural components and possibly high variability between individuals in the ability to expel accumulated, indigestible particles. Visual observations made during the experiment indicated that sediments tended to accumulate inside and at the base of the atrium of this barrel-shaped species. It is likely that structural components closer to the base will take longer to clear indigestible particles. In our study, all samples for SEM were extracted from the bottom portion of the sponge (based on total height) suggesting that structural components located further away from the base of the sponge might have been cleaned more efficiently from accumulated sediment particles than the sampled regions of sponge structural components. Despite the visual recovery of some individuals, a number of V. pourtalesii perished over the course of the 38-day recovery phase. As some individuals in the control group also showed signs of structure necrosis and perished, the cause of death cannot evidently be linked solely to exposure to suspended particles. To assess the physiological recovery potential of V. pourtalesii after long-term sediment exposure, parameters such as oxygen uptake and bacterial clearance rates should also be monitored throughout the recovery period in future studies.
Potential Adaptations to a Dynamic Environment
It is hypothesized that glass sponges in deep-sea environments may utilize sediment-borne bacteria as an additional food source. Stable carbon and nitrogen isotope examinations of glass sponges collected off the Pacific coast of Canada showed ingestion of sediment-borne bacteria re-suspended by tidal oscillations (Kahn et al., 2018). Sediments in deep-sea environments have been found to be 100–1000 times more rich in heterotrophic bacteria than the overlying water column (Kuwae and Hosokawa, 1999). Furthermore, Grant et al. (1987) showed that the fine silty sediments on the Scotian Shelf and particularly in Emerald Basin contain a high proportion of easily re-suspended organic particles and postulated that lateral transport of organic material makes the Scotian Shelf a sink for organic carbon. The potential trophic interaction of V. pourtalesii and sediment borne re-suspended organic matter has not been investigated up to date. However, our incubation-based assessments of physiological parameters suggest that V. pourtalesii tolerates re-suspended particles, rather than utilizing and benefiting from the potentially bacteria-and particle-enriched water layers. The assessed respiration rates suggest that there is no metabolic benefit of the presence of suspended sediment enriched seawater for these sponges.
Emerald Basin is subjected to higher tidal variability and mixing compared to other areas of the Scotian Shelf (Petrie and Smith, 1977). Tidal oscillations and storm events have the potential to re-suspend small grain particles that may remain suspended in the water column for several days (Bogucki et al., 1997; Boegman and Stastna, 2019). In addition to seasonal short-term resuspension events, persistent nepheloid (sediment enriched) layers with particle concentrations of up to 8 mg L–1 have been described to occur on the Scotian Shelf (Spinrad et al., 1983; Pilskaln et al., 1998). Similar resuspension events with a duration of several days were observed during a 10-month deployment of an autonomous benthic lander in the Sambro Bank Sponge Conservation Area (Hanz et al., 2021; see Beazley et al., 2017 for deployment details). Dynamic oceanographic characteristics and sponge occurrences are often linked in the North Atlantic. For instance, the distribution of the glass sponge Pheronema carpenteri (Thomson, 1869) (Pheronematidae) at the Porcupine Seabight (northeast Atlantic) is thought to be linked to areas where bottom currents are intensified due to internal waves (Rice et al., 1990). The causal link was suggested to be an increase in the supply of food related to the occurrence of the internal waves. The same oceanographic settings might be a possible explanation for the occurrence of dense demosponge grounds in the Faroe-Shetland basin (Davison et al., 2019). Our findings suggest that V. pourtalesii might be adapted to tolerate natural suspended sediment up to a certain concentration and exposure time. This is corroborated by the proliferation of this species in this highly dynamic environment subjected to periodic re-suspension events. However, the observed reduction in clearance efficiency with sediment exposure over time indicates that potential coping mechanisms and adaptations are compromised when sediment exposure occurs over a longer time scale.
Implications for Conservation Management
While the results of this study indicate no adverse physiological effects (respiration and clearance rates) of short-term sediment exposure (7 days), prolonged (14–21 days) exposure to re-suspended sediment from bottom trawling may impair physiological rates in V. pourtalesii as a response mechanism. Over time, this may result in an energy deficit and reduction in overall sponge health. With a concentration well below the described re-suspension potential of a trawl (up to 500 mg L–1, see section “Introduction”), these likely negative impacts on the physiology of V. pourtalesii could be amplified in its natural setting if exposed to re-suspended sediments from trawling. Future studies should include different concentrations of suspended sediment in combination with longer exposure times to detect potential thresholds for adverse effects of suspended sediments on V. pourtalesii.
The Sambro Bank and Emerald Basin Sponge Conservation Areas were designed to protect two of the more significant concentrations of V. pourtalesii on the Scotian Shelf identified from biomass/density observations from commercial bycatch records, research vessel trawl catch data, and in situ camera observations. However, kernel density analyses applied to research vessel trawl survey catch data to identify hotspots in sponge biomass (all Porifera, including V. pourtalesii; Kenchington et al., 2016), indicated that less than 2% of the significant concentrations formed primarily by V. pourtalesii in the area known as the Scotian Gulf, which includes Emerald Basin, are encompassed by the closed areas. Similar results were found when examining the proportion of protected suitable habitat of V. pourtalesii generated from random forest species distribution modeling (see Beazley et al., 2018). The percentage overlap between the significant concentrations of all Porifera, including V. pourtalesii, and groundfish fishing effort using mobile-gears revealed that 29.4% of the area occupied by these significant concentrations was subjected to some level of fishing (DFO, 2017b; Koen-Alonso, 2018), with most effort occurring in the lowest percentile (low effort). Nonetheless, this lower effort does not imply that significant adverse impacts of fishing to V. pourtalesii are absent in these sensitive benthic areas (Koen-Alonso, 2018). Depending on the type of gear and composition of the benthic community, few interactions can cause serious irreversible damage (NAFO, 2011), and the greatest impacts to these ecosystems, such as irreversible damage or complete removal, are often caused by the first fishing events (DFO, 2006; Pham et al., 2019).
Grant et al. (2018) modeled the indirect footprint of a trawl adjacent to an existing bottom fishing closure and estimated that the re-suspended sediment plumes spread as far as 6 km from the initial trawling site. They concluded that fishing effort along the boundaries of sponge conservation area rendered the dense sponge grounds inside it vulnerable to suspended sediments. Although the trajectory and retention rates of sediment plumes from bottom fishing in Emerald Basin were not measured in this study, the close proximity of existing bottom fishing to the SCAs on the Scotian Shelf (Koen-Alonso, 2018) may have the potential to impact the dense Vazella grounds that reside within them. Here, buffer zones of a sufficient dimension could be established around the SCAs to minimize the indirect impact of downstream sediment re-suspension by bottom gear to the sponge ground community inside the closed areas. Although there was no adverse effect evident the first 7 days of sediment exposure, an additional mitigation measure could be a temporal staggering of fishing activities within the same area to allow the sponges to recover following trawling events. In this area, the groundfish fishery operates year-round, from April 1 to March 31 but has a 3-month period of high fishing activity from July to September with individual trips occurring on the scale of 5 days and with multiple sets spread out over multiple areas (D. Fenton, Fisheries and Oceans Canada, pers. comm.). Thus, the 21-day period chosen for this experiment likely represents a worst-case scenario of sustained fishing activity from different vessels in the vicinity of the sponge grounds within the peak fishing period. The Scotian Shelf is subjected to ongoing structural developments such as offshore oil and gas extraction (Breeze and Horsman, 2005). The impacts of sediments contaminated with drilling muds or potential spills of crude oil on glass sponges are at present poorly understood. The present study on the physiological response of V. pourtalesii to a concentration of natural suspended sediment may act as a blueprint for the design of these elaborate experiments.
Conclusion
In this aquaria-based ex situ study, V. pourtalesii was found to cope with increased levels of natural suspended sediment for a period of 7 days. As this glass sponge species thrives in a dynamic shelf environment, V. pourtalesii is likely adapted to periodic, current-induced re-suspension events. However, our observations of decreasing clearance rates over time could indicate impaired filtration capacities cumulating in negative impacts on biological functions such as growth, structural components repair mechanisms and reproduction, and a metabolic deficit with adverse implications for overall animal health. Following the precautionary approach, the application of buffer zones to the current management measures for this species on the Scotian Shelf, and temporal coordination of sediment re-suspending activities may help mitigate the potentially adverse long-term effects on the unique ecosystem the Vazella grounds represent on the Scotian Shelf.
Data Availability Statement
The original contributions presented in the study are included in the article/Supplementary Material, further inquiries can be directed to the corresponding author/s.
Author Contributions
EW: conceptualization, methodology, formal analysis, data curation, writing—original draft, review and editing, and visualization. LB: investigation, resources, data curation, writing—original draft, writing—review and editing, visualization, and funding acquisition. BM: investigation, resources, and writing—review and editing. EK: conceptualization, resources, writing—review and editing, supervision, project administration, and funding acquisition. RO: conceptualization, methodology, resources, writing—original draft, writing—review and editing, supervision, project administration, and funding acquisition. All authors contributed to the article and approved the submitted version.
Funding
This work was funded through the SponGES—Deep-sea Sponge Grounds Ecosystems of the North Atlantic: An Integrated Approach Towards Their Preservation And Sustainable Exploitation, under H2020—the EU Framework Programme for Research and Innovation (Grant Agreement No. 679849) with Canadian contribution from Fisheries and Oceans Canada Strategic Program for Ecosystem-Based Research and Advice (SPERA) and International Governance Strategy (IGS).
Conflict of Interest
The authors declare that the research was conducted in the absence of any commercial or financial relationships that could be construed as a potential conflict of interest.
Acknowledgments
We thank the captain, crew, and scientific participants of the cruise on the Canadian Coast Guard vessel Martha L. Black for successful operations and maintenance of sampled sponges onboard. Thanks to the ROPOS team for efficient and delicate sampling of sponges by remotely operated vehicle (ROV). We thank Ping Li and Patricia Scallion from Dalhousie University’s Scientific Imaging Suite and SEM-FIB Facilities, respectively, for their training and assistance in SEM/EDS analyses, and Marieve Bouchard-Marmen, Emily Baker (both DFO), and Gabrielle Tompkins (Dalhousie University) for their assistance in preparing samples for SEM/EDS analysis. We also thank Diede Maas and Tim Wijgerde (Wageningen University and Research, WUR) for their invaluable assistance with the data analysis. We thank Anna Noordeloos (Royal Netherlands Institute for Sea Research, NIOZ), Jasper de Goeij (University of Amsterdam, UvA), and Martijn Bart (UvA) for their assistance in establishing the flow cytometry analysis and frequent content meetings. We thank Furu Mienis (NIOZ) and Ulrike Hanz (NIOZ) for support with analyzing the sediment characteristics and sharing insights into the particle dynamics on the Scotian shelf. We thank the Institute for Marine Research, Bergen and namely Raymond Bannister, for the supplies of experimental equipment. Finally, we thank Detmer Sipkema (WUR) and Rob Joosten (WUR) for generously sharing the flow cytometer and help with analyzing samples.
Supplementary Material
The Supplementary Material for this article can be found online at: https://www.frontiersin.org/articles/10.3389/fmars.2021.611539/full#supplementary-material
Footnotes
References
Alexander, B. E., Liebrand, K., Osinga, R., van der Geest, H. G., Admiraal, W., Cleutjens, J. P. M., et al. (2014). Cell turnover and detritus production in marine sponges from tropical and temperate benthic ecosystems. PLoS One 9:e109486. doi: 10.1371/journal.pone.0109486
Bart, M. C., Mueller, B., Rombouts, T., Ven, C., Tompkins, G., Osinga, R., et al. (2020). Dissolved organic carbon (DOC) is essential to balance the metabolic demands of four dominant North-Atlantic deep-sea sponges. Limnol. Oceanogr. 9999, 1–14. doi: 10.1002/lno.11652
Beazley, L., Kenchington, E. L., Murillo, F. J., and Sacau, M. D. M. (2013). Deep-sea sponge grounds enhance diversity and abundance of epibenthic megafauna in the Northwest Atlantic. ICES J. Mar. Sci. 70, 1471–1490. doi: 10.1093/icesjms/fst124
Beazley, L., Kenchington, E., Yashayaev, I., and Murillo, F. J. (2015). Drivers of epibenthic megafaunal composition in the sponge grounds of the Sackville Spur, northwest Atlantic. Deep Sea Res. Part I Oceanogr. Res. Pap. 98, 102–114. doi: 10.1016/j.dsr.2014.11.016
Beazley, L., Pham, C., Murillo, J., and Kenchington, E. (2017). Cruise Report for the DFO/SponGES CCGS Martha L. Black Oceanographic Mission (MLB2017001), August 31 to September 7, 2017. Canadian Technical Report of Fisheries and Aquatic Sciences 3242. Dartmouth, NS: Bedford Institute of Oceanography.
Beazley, L., Wang, Z., Kenchington, E., Yashayaev, I., Rapp, H. T., Xavier, J. R., et al. (2018). Predicted distribution of the glass sponge Vazella pourtalesi on the Scotian Shelf and its persistence in the face of climatic variability. PLoS One 13:e0205505. doi: 10.1371/journal.pone.0205505
Bell, J. J. (2002). Regeneration rates of a sublittoral demosponge. J. Mar. Biol. Assoc. UK 82, 169–170. doi: 10.1017/S0025315402005295
Bell, J. J., McGrath, E., Biggerstaff, A., Bates, T., Bennett, H., Marlow, J., et al. (2015). Sediment impacts on marine sponges. Mar. Pollut. Bull. 94, 5–13. doi: 10.1016/j.marpolbul.2015.03.030
Boegman, L., and Stastna, M. (2019). Sediment resuspension and transport by internal solitary waves. Annu. Rev. Fluid Mech. 51, 129–154. doi: 10.1146/annurev-fluid-122316-045049
Bogucki, D., Dickey, T., and Redekopp, L. G. (1997). Sediment resuspension and mixing by resonantly generated internal solitary waves. J. Phys. Oceanogr. 27, 1181–1196. doi: 10.1175/1520-04851997027<1181:SRAMBR<2.0.CO;2
Breeze, H., and Horsman, T. (2005). The Scotian Shelf: An Atlas of Human Activities. Dartmouth, NS: Fisheries and Oceans Canada.
Brussaard, C. P. D., Payet, J. P., Winter, C., and Weinbauer, M. G. (2010). Quantification of aquatic viruses by flow cytometry. Man. Aquat. Viral Ecol. 11, 102–107. doi: 10.4319/mave.2010.978-0-9845591-0-7.102
Davison, J. J., van Haren, H., Hosegood, P., Piechaud, N., and Howell, K. L. (2019). The distribution of deep-sea sponge aggregations (Porifera) in relation to oceanographic processes in the Faroe-Shetland Channel. Deep Sea Res. Part I Oceanogr. Res. Pap. 146, 55–61. doi: 10.1016/j.dsr.2019.03.005
De Goeij, J. M., de Kluijver, A., van Duyl, F. C., Vacelet, J., Wijffels, R. H., de Goeij, A. F. R. M., et al. (2009). Cell kinetics of the marine sponge Halisarca caerulea reveal rapid cell turnover and shedding. J. Exp. Biol. 212, 3892–3900. doi: 10.1242/jeb.034561
De Goeij, J. M., van Oevelen, D., Vermeij, M. J. A., Osinga, R., Middelburg, J. J., de Goeij, A. F. P. M., et al. (2013). Surviving in a marine desert: the sponge loop retains resources within coral reefs. Science 342, 108–110. doi: 10.1126/science.1241981
DFO (2006). Impacts of Trawl Gears and Scallop Dredges on Benthic Habitats, Populations and Communities. DFO Canadian Science Advisory Secretariat Science Advisory Report 2006/025. Dartmouth: DFO.
DFO (2017a). Delineation of Significant Areas of Coldwater Corals and Sponge-Dominated Communities in Canada’s Atlantic and Eastern Arctic Marine Waters and their Overlap with Fishing Activity. DFO Canadian Science Advisory Secretariat Science Advisory Report 2017/007. Dartmouth: DFO.
DFO (2017b). Guidance on the Level of Protection of Significant Areas of Coldwater Corals and Sponge-Dominated Communities in Newfoundland and Labrador Waters. DFO Canadian Science Advisory Secretariat Science Advisory Report 2017/030. Dartmouth: DFO.
Durrieu de Madron, X., Ferré, B., Le Corre, G., Grenz, C., Conan, P., Pujo-Pay, M., et al. (2005). Trawling-induced resuspension and dispersal of muddy sediments and dissolved elements in the Gulf of Lion (NW Mediterranean). Cont. Shelf Res. 25, 2387–2409. doi: 10.1016/j.csr.2005.08.002
FAO (2009). International Guidelines for the Management of Deep-Sea Fisheries in the High Seas. Rome: FAO, 73.
Fuller, S. (2011). Diversity of Marine Sponges in the Northwest Atlantic. Ph.D. thesis, Dalhousie University, Halifax, NS.
Grant, J., Volckaert, F., and Roberts-Regan, D. L. (1987). Resuspendable organic matter in nova scotian shelf and slope sediments. Cont. Shelf Res. 7, 1123–1138. doi: 10.1016/0278-4343(87)90102-6
Grant, N., Matveev, E., Kahn, A. S., and Leys, S. P. (2018). Suspended sediment causes feeding current arrests in situ in the glass sponge Aphrocallistes vastus. Mar. Environ. Res. 137, 111–120. doi: 10.1016/j.marenvres.2018.02.020
Grant, N., Matveev, E., Kahn, A. S., Archer, S. K., Dunham, A., Bannister, R. J., et al. (2019). Effect of suspended sediments on the pumping rates of three species of glass sponge in situ. Mar. Ecol. Prog. Ser. 615, 79–100. doi: 10.3354/meps12939
Hanz, U., Beazley, L., Kenchington, E., Duineveld, G., Rapp, H. T., and Mienis, F. (2021). Seasonal variability in near-bed environmental conditions in the Vazella pourtalesii glass sponge grounds of the Scotian Shelf. Front. Mar. Sci. 7:1179. doi: 10.3389/fmars.2020.597682
Hawkes, N., Korabik, M., Beazley, L., Rapp, H. T., Xavier, J. R., and Kenchington, E. (2019). Glass sponge grounds on the Scotian Shelf and their associated biodiversity. Mar. Ecol. Prog. Ser. 614, 91–109. doi: 10.3354/meps12903
Hogg, M. M., Tendal, O. S., Conway, K. W., Pomponi, S. A., van Soest, R. W. M., Gutt, J., et al. (2010). Deep Sea Sponge Grounds: Reservoirs of Biodiversity. UNEP-WCMC Biodiversity Series No: 32. Cambridge: UNEP-WCMC, 27–31. doi: 10.1016/S0378-777X(80)80057-6
Kahn, A. S., Chu, J. W. F., and Leys, S. P. (2018). Trophic ecology of glass sponge reefs in the Strait of Georgia, British Columbia. Sci. Rep. 8:756. doi: 10.1038/s41598-017-19107-x
Kenchington, E., Beazley, L., Lirette, C., Murillo, F., Guijarro, J., Wareham, V., et al. (2016). Delineation of Coral and Sponge Significant Benthic Areas in Eastern Canada Using Kernel Density Analyses and Species Distribution Models. Canadian Science Advisory Secretariat Research Document 2016/093. Dartmouth, NS: Fisheries and Oceans Canada, 178.
King, L. (1970). Surficial Geology of the Halifax-Sable Island Map Area. Ottawa, ON: Marine Science Branch, Canada Department of Energy, Mines and Resources, 16.
Koen-Alonso, M. (2018). Analysis of the Overlap between Fishing Effort and Significant Benthic Areas in Canada’s Atlantic and Eastern Arctic Marine Waters. Canadian Science Advisory Secretariat (CSAS). Research Document 2018/015. Dartmouth, NS: Fisheries and Oceans Canada.
Kutti, T., Bannister, R. J., Fosså, J. H., Krogness, C. M., Tjensvoll, I., and Søvik, G. (2015). Metabolic responses of the deep-water sponge Geodia barretti to suspended bottom sediment, simulated mine tailings and drill cuttings. J. Exp. Mar. Biol. Ecol. 473, 64–72. doi: 10.1016/j.jembe.2015.07.017
Kuwae, T., and Hosokawa, Y. (1999). Determination of abundance and biovolume of bacteria in sediments by dual staining with 4′,6-diamidino-2-phenylindole and acridine orange: relationship to dispersion treatment and sediment characteristics. Appl. Environ. Microbiol. 65, 3407–3412.
Maldonado, M., Aguilar, R., Bannister, R. J., Bell, J. J., Conway, K. W., Dayton, P. K., et al. (2017). “Sponge grounds as key marine habitats: a synthetic review of types, structure, functional roles, and conservation concerns,” in Marine Animal Forests: The Ecology of Benthic Biodiversity Hotspots, eds S. Rossi, L. Bramanti, A. Gori, and C. Orejas (Cham: Springer International Publishing AG), 14–25. doi: 10.1007/978-3-319-21012-4_24
Maldonado, M., Beazley, L., López-Acosta, M., Kenchington, E., Casault, B., Hanz, U., et al. (2020). Massive silicon utilization facilitated by a benthic-pelagic coupled feedback sustains deep-sea sponge aggregations. Limnol. Oceanogr. 66, 366–391. doi: 10.1002/lno.11610
Maldonado, M., Ribes, M., and van Duyl, F. C. (2012). Nutrient fluxes through sponges. biology, budgets, and ecological implications. Adv. Mar. Biol. 62, 113–182. doi: 10.1016/B978-0-12-394283-8.00003-5
McGrath, E. C., Smith, D. J., Jompa, J., and Bell, J. J. (2017). Adaptive mechanisms and physiological effects of suspended and settled sediment on barrel sponges. J. Exp. Mar. Biol. Ecol. 496, 74–83. doi: 10.1016/j.jembe.2017.07.013
Mengual, B., Cayocca, F., Le Hir, P., Draye, R., Laffargue, P., Vincent, B., et al. (2016). Influence of bottom trawling on sediment resuspension in the ‘Grande-Vasière’ area (Bay of Biscay, France). Ocean Dyn. 66, 1181–1207. doi: 10.1007/s10236-016-0974-7
Murillo, F. J., Muñoz, P. D., Cristobo, J., Ríos, P., González, C., Kenchington, E., et al. (2012). Deep-sea sponge grounds of the Flemish Cap, Flemish Pass and the Grand Banks of Newfoundland (Northwest Atlantic Ocean): distribution and species composition. Mar. Biol. Res. 8, 842–854. doi: 10.1080/17451000.2012.682583
NAFO (2011). Report of the 4th Meeting of the NAFO Scientific Council Working Group on Ecosystem Approach to Fisheries Management (WGEAFM). 30 November to 10 December 2011, Dartmouth, Canada. NAFO SCS Document 11/22. Serial No. N6006. Dartmouth, NS: NAFO.
NAFO (2021). Conservation and Enforcement Measures. NAFO/COM Doc. 21-10. Serial No. N7153. Dartmouth, NS: NAFO.
Petrie, B., and Smith, P. C. (1977). Low-frequency motions on the Scotian shelf and slope. Atmosphere 15, 117–140. doi: 10.1080/00046973.1977.9648435
Pham, C. K., Murillo, F. J., Lirette, C., Maldonado, M., Colaço, A., Ottaviani, D., et al. (2019). Removal of deep-sea sponges by bottom trawling in the Flemish Cap area: conservation, ecology and economic assessment. Sci. Rep. 9:15843. doi: 10.1038/s41598-019-52250-1
Pilskaln, C. H., Churchill, J. H., and Mayer, L. M. (1998). Resuspension of sediment by bottom trawling in the Gulf of Maine and potential geochemical consequences. Conserv. Biol. 12, 1223–1229. doi: 10.1046/j.1523-1739.1998.0120061223.x
Puig, P., Canals, M., Company, J. B., Martín, J., Amblas, D., Lastras, G., et al. (2012). Ploughing the deep sea floor. Nature 489, 286–289. doi: 10.1038/nature11410
Rice, A. L., Thurston, M. H., and New, A. L. (1990). Dense aggregations of a hexactinellid sponge, Pheronema carpenteri, in the Porcupine Seabight (northeast Atlantic Ocean), and possible causes. Prog. Oceanogr. 24, 179–196. doi: 10.1016/0079-6611(90)90029-2
Robertson, L. M., Hamel, J. F., and Mercier, A. (2017). Feeding in deep-sea demosponges: influence of abiotic and biotic factors. Deep Sea Res. Part I Oceanogr. Res. Pap. 127, 49–56. doi: 10.1016/j.dsr.2017.07.006
Schönberg, C. H. L. (2016a). Effects of Dredging on Benthic Filter Feeder Communities, with a Focus on Sponges. Report of Theme 6-Project 6.1.1 Prepared for the Dredging Science Node. Perth: Western Australian Marine Science Institution, 139.
Schönberg, C. H. L. (2016b). Happy relationships between marine sponges and sediments-a review and some observations from Australia. J. Mar. Biol. Assoc. UK 96, 493–514. doi: 10.1017/S0025315415001411
Shore, R. E. (1971). Growth and renewal studies of the choanocyte population in Hymeniacidon sinapium (Porifera: Demospongiae) using colcemid and 3-H thymidine. J. Exp. Zool. 177, 359–363. doi: 10.1002/jez.1401770310
Spinrad, R. W., Zaneveld, J. R. V., and Kitchen, J. C. (1983). A study of the optical characteristics of the suspended particles in the benthic nepheloid layer of the Scotian Rise (North Atlantic). J. Geophys. Res. 88, 7641–7645. doi: 10.1029/JC088iC12p07641
Strehlow, B. W., Jorgensen, D., Webster, N. S., Pineda, M. C., and Duckworth, A. (2016). Using a thermistor flowmeter with attached video camera for monitoring sponge excurrent speed and oscular behaviour. PeerJ 4:e2761. doi: 10.7717/peerj.2761
Strehlow, B. W., Pineda, M. C., Duckworth, A., Kendrick, G. A., Renton, M., Wahab, M. A. A., et al. (2017). Sediment tolerance mechanisms identified in sponges using advanced imaging techniques. PeerJ 5:e3904. doi: 10.7717/peerj.3904
Tjensvoll, I., Kutti, T., Fosså, J. H., and Bannister, R. J. (2013). Rapid respiratory responses of the deep-water sponge Geodia barretti exposed to suspended sediments. Aquat. Biol. 19, 65–73. doi: 10.3354/ab00522
Keywords: sponge grounds, Vazella pourtalesii, re-suspended sediment, bottom-trawling, marine conservation area
Citation: Wurz E, Beazley L, MacDonald B, Kenchington E, Rapp HT and Osinga R (2021) The Hexactinellid Deep-Water Sponge Vazella pourtalesii (Schmidt, 1870) (Rossellidae) Copes With Temporarily Elevated Concentrations of Suspended Natural Sediment. Front. Mar. Sci. 8:611539. doi: 10.3389/fmars.2021.611539
Received: 29 September 2020; Accepted: 02 March 2021;
Published: 24 March 2021.
Edited by:
Cristina Gambi, Marche Polytechnic University, ItalyReviewed by:
Andreu Santin Muriel, Institute of Marine Sciences (ICM-CSIC), SpainTina Molodtsova, P.P. Shirshov Institute of Oceanology (RAS), Russia
Copyright © 2021 Wurz, Beazley, MacDonald, Kenchington, Rapp and Osinga. This is an open-access article distributed under the terms of the Creative Commons Attribution License (CC BY). The use, distribution or reproduction in other forums is permitted, provided the original author(s) and the copyright owner(s) are credited and that the original publication in this journal is cited, in accordance with accepted academic practice. No use, distribution or reproduction is permitted which does not comply with these terms.
*Correspondence: Erik Wurz, ZXJpay53dXJ6QHd1ci5ubA==