- 1MARBEC, Université Montpellier, Ifremer, CNRS, IRD, Montpellier, France
- 2School of Natural Sciences, National University of Ireland Galway, Galway, Ireland
- 3Okeanos Research Centre, Universidade dos Açores, Horta, Portugal
- 4IMAR Instituto do Mar, Universidade dos Açores, Horta, Portugal
- 5Scottish Association for Marine Science, Scottish Marine Institute, Oban, United Kingdom
- 6Changing Oceans Group, School of GeoSciences, Grant Institute, University of Edinburgh, Edinburgh, United Kingdom
- 7Seascape Consultants Ltd., Romsey, United Kingdom
- 8Department of Biological Sciences, University of Rhode Island, Kingston, RI, United States
- 9Bedford Institute of Oceanography, Fisheries and Oceans Canada, Dartmouth, NS, Canada
- 10Instituto Español de Oceanografía (IEO), Centro Oceanográfico de Gijón, CSIC, Gijón, Spain
- 11Ifremer, EEP, Centre de Bretagne, Brest, France
Designing conservation networks requires a well-structured framework for achieving essential objectives such as connectivity, replication or viability, and for considering local management and socioeconomic stakes. Although systematic conservation planning (SCP) approaches are increasingly used to inform such networks, their application remains challenging in large and poorly researched areas. This is especially the case in the deep sea, where SCP has rarely been applied, although growing awareness of the vulnerability of deep-sea ecosystems urges the implementation of conservation measures from local to international levels. This study aims to structure and evaluate a framework for SCP applicable to the deep sea, focusing on the identification of conservation priority networks for vulnerable marine ecosystems (VMEs), such as cold-water coral reefs, sponge grounds, or hydrothermal vents, and for key demersal fish species. Based on multi-objective prioritization, different conservation scenarios were investigated, allowing the impact of key elements such as connectivity and conservation cost to be evaluated. Our results show that continental margin slopes, the Mid-Atlantic Ridge, and deeper areas of large and productive shelves housing fishing grounds appeared as crucial zones for preserving the deep-sea biodiversity of the North Atlantic, and within the limitations imposed by the data available, of the Mediterranean. Using biologically-informed connectivity led to a more continuous and denser conservation network, without increasing the network size. Even when minimizing the overlap with socioeconomic activities, the inclusion of exploited areas was necessary to fulfil conservation objectives. Such areas included continental shelf fishing grounds for demersal fish species, and areas covered by deep-sea mining exploration contracts for hydrothermal vent communities. Covering 17% of the study area and protecting 55% of each feature on average, the identified priority network held a high conservation potential. However, these areas still suffer from poor protection, with 30% of them benefiting from some form of recognition and 11% only from protection against trawling. Integrating them into current marine spatial planning (MSP) discussions could foster the implementation of a basin-scale conservation network for the deep sea. Overall, this work established a framework for developing large-scale systematic planning, useful for managing Areas Beyond National Jurisdiction (ABNJ).
Introduction
The deep sea hosts a large diversity of ecosystems and species (Gage and Tyler, 1991; Tyler, 2003; Danovaro et al., 2010; Ramirez-Llodra et al., 2010). The bathyal domain, which comprises continental margins and oceanic rises from about 200 m down to 3,500 m deep (UNESCO, 2009), is topographically complex, including canyons, seamounts and ridges, known to represent hotspots of benthic and demersal biodiversity (Rogers et al., 2007; De Leo et al., 2010; Würtz, 2012; Morato et al., 2013; Fernandez-Arcaya et al., 2017). These habitats offer diverse substrates for species to attach to, but also a plentiful food supply for benthic filter- and suspension-feeders, as plankton is trapped by currents on their steep slopes (Frederiksen et al., 1992; Gili et al., 1999; Genin, 2004; White et al., 2005; Mienis et al., 2007). The presence of habitat-forming species highly influences the structure and functioning of such benthic ecosystems. Cold-water corals and deep-sea sponges facilitate the development of complex benthic communities, including coral reef frameworks, coral gardens, sea-pen fields and sponge grounds, among others (Roberts et al., 2006, 2009; Buhl-Mortensen et al., 2010; Baker et al., 2012; Beazley et al., 2013; Rueda et al., 2019). Hydrothermal vents and cold seeps are other types of highly specific and unique communities whose components rely on chemosynthesis achieved by the symbiotic association of organisms (Fisher et al., 2007; Palomino et al., 2019). This mosaic of deep-sea ecosystems offers a wide array of ecological functions beyond structuring benthic communities, such as the provision of habitat, refuge and feeding resources for many demersal fish species (Baillon et al., 2012; Henry et al., 2013; Pham et al., 2015; Buhl-Mortensen et al., 2017; D’Onghia, 2019) and influences oceanic functioning through the carbon pump (Cathalot et al., 2015; Soetaert et al., 2016). Many of such ecosystem functions translate into ecosystem services, which ultimately benefit humankind (Thurber et al., 2014).
Deep-sea ecosystems are under increasing pressure from human activities (Davies et al., 2007; Ramirez-Llodra et al., 2011; Pianté and Ody, 2015; Halpern et al., 2019), whose cumulative impacts remain highly uncertain (Armstrong et al., 2019). Deep-sea fishing is known to deplete deep-water fish stocks, and to adversely impact entire benthic habitats (Koslow et al., 2000; Althaus et al., 2009; Bailey et al., 2009; Maynou and Cartes, 2012; Pusceddu et al., 2014; Aguilar et al., 2017; Clark et al., 2019). Recent advances in technology have opened the way for the biotechnology industry to access and exploit the potential of deep-sea biochemical compounds (Synnes, 2007; Skropeta and Wei, 2014). The oil and gas industry has a long history of activity in the deep sea (LaBelle, 2001) and may soon be followed by the mining industry (Sharma, 2017). Deep-sea mining targets minerals that are often found in areas supporting high biomass, such as hydrothermal vents, and is therefore likely to cause adverse impacts on these ecosystems (Van Dover, 2011; Gollner et al., 2017; Niner et al., 2018; Van Dover et al., 2018). In addition to these growing exploitation threats, the future of deep-sea species and habitats will also be influenced by forecasted changes in climate conditions, which will widely affect deep-sea ecosystem structure and functioning (Parravicini et al., 2015; Sweetman et al., 2017; Levin et al., 2020; Morato et al., 2020). In the face of these threats, certain deep-sea ecosystems have now been designated as vulnerable marine ecosystems (VMEs) following the criteria proposed by the Food and Agricultural Organization (FAO) of the United Nations (FAO, 2009). These criteria include: (1) uniqueness or rarity; (2) functional significance of the habitat; (3) fragility; (4) life history traits of component species that make recovery difficult; and (5) structural complexity. VMEs are generally identified through the type and abundance of indicator taxa such as large cold-water corals and sponges, chemosynthetic species and certain types of mud and sand fauna.
The challenge of progressing toward a sustainable exploitation of deep-water resources and the inherent fragility of deep-sea ecosystems reinforce the need to establish an adequate conservation and governance framework for the deep sea (Mengerink et al., 2014; Manea et al., 2020; Orejas et al., 2020). In recent years, a growing number of regional or international agreements, conventions and laws have addressed the need to conserve deep-sea biodiversity (Ardron et al., 2014). These include: (i) the UN Convention on Biological Diversity (CBD) leading a global effort to identify Ecologically or Biologically Significant Areas (EBSAs); (ii) the implementation by Regional Fisheries Management Organizations (RFMOs) of the United Nation General Assembly resolutions (61/105 and 64/72) calling on sovereign states to protect VMEs from fishing; (iii) the identification and protection of Areas of Particular Environmental Interest by the International Seabed Authority (ISA) in areas licensed for seabed mining; (iv) the regional designation of marine protected areas (MPAs) by consortiums of nations such as the OSPAR Commission in the North-East Atlantic and the UNEP-MAP Protocol of the Barcelona Convention in the Mediterranean (Barcelona Convention, 1995; MedPAN & SPA/RAC, 2019); and (v) the ongoing negotiations on the new legally-binding UN Treaty to protect Biodiversity Beyond National Jurisdictions (the UN BBNJ treaty for short, Wright et al., 2019).
Despite the various measures outlined above, less than 2% of Areas Beyond National Jurisdiction (ABNJ), which are almost exclusively constituted by deep sea, are protected in the world, and the integration of the deep sea in national protection networks remains uneven and lacks foundation. Hence there is an urgent need to provide objective guidance if global marine protection objectives are to be met (i.e., the Aichi Target 11 of 10% of the oceans protected by 2020; and prospective 30% targets in the draft CBD post 2020 Global Biodiversity Framework). Meanwhile, systematic approaches for conservation planning have been called for (Ban et al., 2014; Manea et al., 2020) but only sparsely applied to the deep sea (Evans et al., 2015), essentially for sectoral purposes (deep-sea mining, see Wedding et al., 2013; Dunn et al., 2018), or in ABNJ in general (Álvarez-Romero et al., 2018a; O’Leary et al., 2019; Visalli et al., 2020).
Systematic conservation planning (SCP) is an explicit, objective-based and quantitative approach for allocating areas for biodiversity conservation (Margules and Pressey, 2000). Although it is widely used to design terrestrial protected areas and coastal MPAs, a major constraint for the development of this method at an ocean basin scale, particularly in the deep sea, is the lack of baseline data on the distribution, biogeography and connectivity of both vulnerable and exploited species (IUCN, 2019; Wright et al., 2019; Manea et al., 2020). The deep North Atlantic and the Mediterranean are some of the most sampled areas of the deep sea and thus are suitable regions to implement SCP at large spatial scales. To date, as elsewhere, the protection of these basins has been designed by incrementally protecting important and well-studied areas, and then iteratively adding ad hoc new areas to enhance conservation, without considering overall network coherence (Ardron et al., 2014, MedPAN & SPA/RAC, 2019). While a few studies have investigated aspects of the efficiency of the current MPA network to protect deep-sea ecosystems in parts of the North Atlantic (e.g., Johnson et al., 2014; Evans et al., 2015; Ross et al., 2017; Kenchington et al., 2019b), and the Mediterranean (Abdulla et al., 2009; Amengual and Alvarez-Berastegui, 2018; MedPAN & SPA/RAC, 2019; Manea et al., 2020), conservation planning has seldom been addressed over the full basin even though it represents the most relevant ecological scale especially for widely distributed species. Besides, climate change is likely to severely limit the effectiveness of currently existing protection measures for the deep sea (Johnson et al., 2018b) by causing significant species distribution shifts, contractions and local extinctions. This emphasizes the need to consider a large spatial scale, at which ecological and evolutionary processes take place, and it appears then crucial to propose conservation options that will provide long-term network viability and identify areas of refugia that are resilient to climate change (Johnson and Kenchington, 2019; Manea et al., 2020).
This study draws upon recent research that identified a series of ecological principles that should be used to guide the implementation of MSP for the deep environments of the Mediterranean (Manea et al., 2020), the Mid-Atlantic Ridge (Dunn et al., 2018), and addressing the conservation of VMEs (Morato et al., 2018). Even though the narrow Strait of Gibraltar can represent a dispersal barrier for several species or populations (Pascual et al., 2017), the natural connection between the North Atlantic and the Mediterranean links the deep-sea ecosystems of the two basins, for instance through the Mediterranean Outflow Water (MOW) (e.g., Boavida et al., 2019; Mosquera Giménez et al., 2019). This interdependency makes it ecologically relevant to conduct this study considering the two basins together particularly in terms of network connectivity in the face of climate change. Moreover the North Atlantic and the Mediterranean share several riverine states and a common supra-national body (EU) for law enforcement, that reinforce the relevance of addressing them together regarding MSP.
Our aim is to apply a SCP exercise to address conservation management gaps for the deep-sea biodiversity in the North Atlantic, and within the limits of data available, in the Mediterranean, in the context of climate change and ongoing international discussions on conservation management policies. Our study is thus not attempting to design a fully ecologically representative conservation network (including exhaustive pelagic, demersal and benthic compartments) but rather focuses on designing and evaluating a framework that can be re-used to better conserve deep-sea VMEs. This will be achieved by:
(1) Identifying zones of conservation importance for VMEs (especially habitat-forming cold-water corals and sponges) and commercially important demersal fish species, by taking into account predicted climate change;
(2) Evaluating the implications of considering connectivity in the conservation network design;
(3) Evaluating the implications of considering current management of human activities in the conservation network design;
(4) Assessing the efficiency of current conservation efforts and identifying priority areas that could set the baseline for the design of a robust network of MPAs.
Materials and Methods
In the framework of two EU projects funded under the Horizon 2020 programme (ATLAS and SponGES), the generation and synthesis of (1) knowledge on the actual distribution of VMEs in the Atlantic and the Mediterranean (Supplementary Figure 1, Combes et al., 2019; Morato et al., 2021), (2) predictive modelling of the current and future distributions of VME indicator taxa and deep-sea fishes (Morato et al., 2020; González-Irusta, unpublished data), and (3) the modelling of larval dispersal at the scale of the North Atlantic (Gary et al., 2020) provided the basis to test the relevance of SCP to guide MSP. Furthermore, this information was augmented with existing datasets on seafloor geomorphology and biogeography, human uses of the deep sea and the current conservation management measures being implemented over the full basin.
Systematic Conservation Planning
For a given region (i.e., an area to be managed), SCP is based primarily on an analysis of the distribution of biodiversity (species and/or habitats) while taking into account existing management of human activities. By applying spatial prioritization calculations, an SCP framework aims to select a network of areas that, if protected, would satisfy specific and quantitative conservation objectives. Various types of objectives can be set for a conservation network, such as protecting a set of species, representing the diversity of habitats, maximizing the connectivity between conservation units, or minimizing the socioeconomic cost of implementing the network (e.g., minimizing the amount of fishing grounds to close). The conservation solution emerging from prioritization depicts a suggested network of marine reserves, fishing closures, or any other designation that can suggest management measures ensuring the protection of the features of interest.
The spatial prioritization approach used in this study is similar to the widely used Marxan framework (Ball et al., 2009), and follows the steps outlined in the conceptual flow diagram shown in Figure 1 and explained in more detail in the following subsections. Technical key terms of the prioritization approach are outlined in this paragraph.
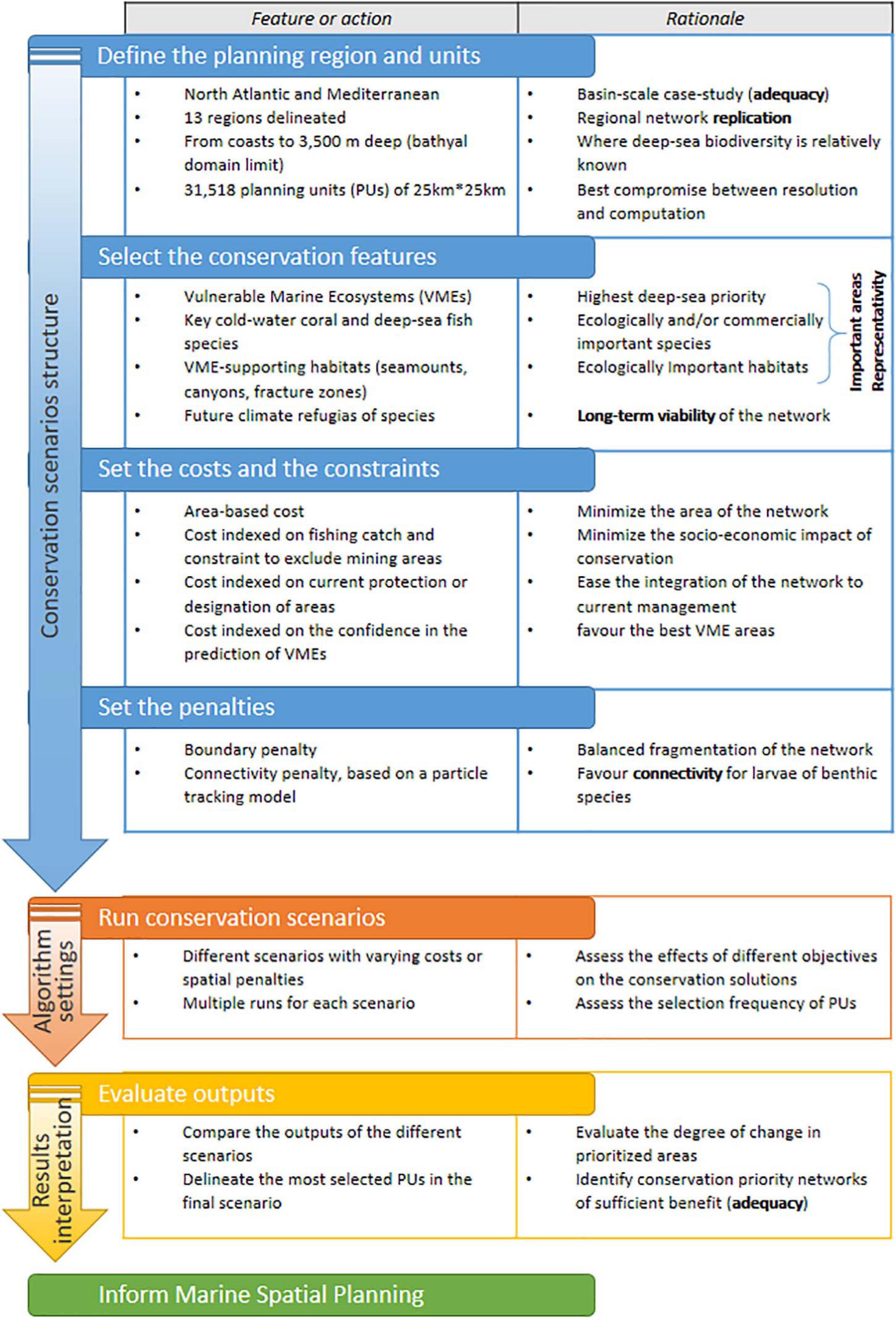
Figure 1. Conceptual flow diagram of the prioritization steps of the framework. The five network criteria for ecological coherence (important areas, representativity, connectivity, replication, and adequacy and viability) as identified by the Convention on Biological Diversity (CBD, 2008), are outlined in bold.
Spatial planning scenarios are developed for a given planning region. The planning region is subdivided into a series of spatial grid cells known as planning units (PUs) that can be either selected or not selected by a prioritization algorithm to reach a conservation solution. Spatial prioritization was undertaken using an integer linear programming (ILP) algorithm available in the Prioritizr package (Hanson et al., 2019b) in the R environment (R Core Team, 2018). A number of different conservation scenarios were constructed to determine the optimal conservation network design in terms of MPA size, number and location while minimizing disruption to the existing economic activity, i.e., fishing for the planning region.
The conservation features, e.g., vulnerable species or habitats, are each assigned a conservation target, corresponding to the minimum percentage area of the feature distribution that is required to be protected in the planning region. Besides setting conservation targets, the other essential element of SCP is determining the socio-economic cost of protecting a PU. When no socioeconomic costs are available, an area-based cost (i.e., proportional to the area of each PU) can be used, which is identical for all the PUs within a regular grid. In order to balance conservation goals with socio-economic or spatial management needs, the cost of PUs can be varied according to acquisition costs, the value of foregoing economic activity (opportunity cost), or any relative measure of easiness-to-implement management (Ban and Klein, 2009). Here, the cost layer was varied according to the scenarios’ objectives (Figure 1). Furthermore, spatial constraints and penalties can be factored into the solution of the conservation problem by influencing the location, size and spacing of the areas selected as part of the conservation solution. A constraint is a spatial requirement applied to the conservation solution that excludes or includes certain PUs (e.g., to include existing MPAs in the final solution). Penalties are associated with a specific metric, which acts as a trade-off between the cost of PUs. Two penalties were used here: (1) a boundary penalty, which increases the cost for more spatially fragmented solutions and thus favours larger, less numerous areas (through “clumping” of PUs) as the penalty is increased; and (2) a connectivity penalty, which applies a connectivity metric to promote better connectivity among conservation units (Figure 1).
The optimal conservation solution is determined by applying a “minimum set,” i.e., a Marxan-like objective function, whose objective is to minimize the cost of the solution whilst ensuring that all targets (and other constraints, if any) are met (Ball et al., 2009; Hanson et al., 2019b). This can be expressed in matrix notation as:
where x is a vector of decision variables (here, whether to select or not a PU), c is a vector of costs for each PU, A is the constraint matrix that stores the amount of each feature in each PU, b is a vector of targets for each conservation feature, the ≥ symbol indicates that the total amount of each feature in the solution must exceed the quantities in b. A conservation problem can be further modified by adding penalties acting on c (e.g., the connectivity penalty that decreases the cost of well-connected PUs), or constraints acting on A (e.g., the exclusion of certain PUs).
In this study, this objective function was further adjusted by imposing the completion of conservation targets non-solely on the total planning area, but also within 13 sub-regions (Figure 2) in order to allocate conservation units across the entire ocean basin and enhance the network replication and capture some element of geographic representativeness. Each conservation problem was solved a number of times so that it was possible to obtain a selection frequency with which individual PUs appeared in each solution, indicating the relative importance of PUs for achieving the conservation objectives. The resulting proposed conservation priority networks thus include those PUs that reached a high selection frequency, i.e., that were present in more than half or 75% of the solutions.
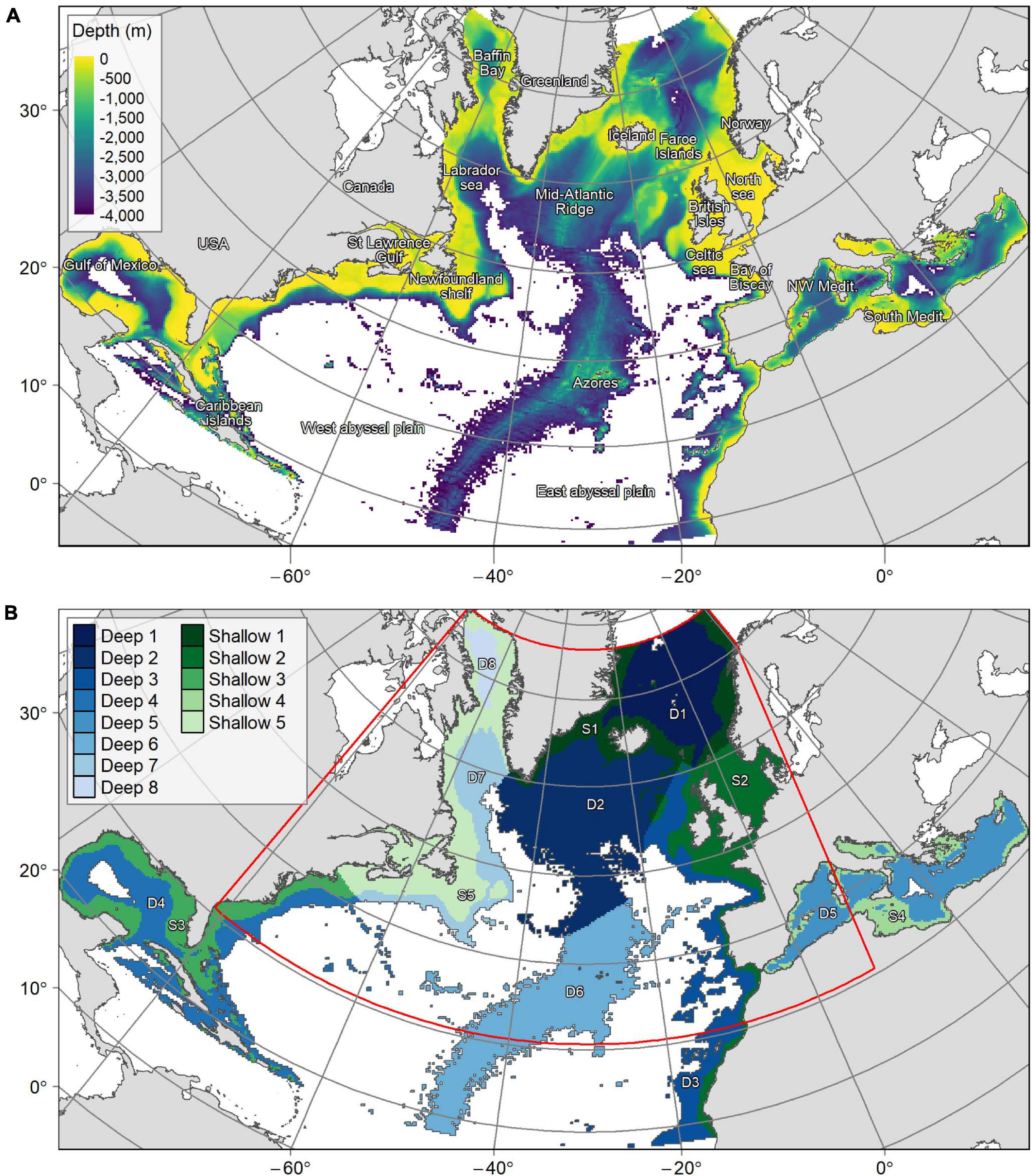
Figure 2. (A) Bathymetry over the planning region. (B) Delimitation of the planning region into 13 “provinces.” “Deep” regions represent areas with an average depth below 800 m whereas “shallow” regions have an average depth between 0 and 800 m. The red line shows the limits of the smaller planning area used for connectivity scenarios.
Planning Region
The planning region covered the Atlantic and Mediterranean basins from 18°N to 76°N and 36°E to 98°W (Figure 2). This area was sub-divided divided using a grid of 25 × 25 km cells, with each square representing a PU. Units that only contained seafloor deeper than 3,500 m (abyssal and hadal environments) were excluded because target VME and fish species do not occur at these depths. In contrast, shallow areas (<200 m depth) were retained, since demersal fish species occur both on margin slopes and continental shelves, and cold-water corals are often found in shallower waters on continental shelves and in fjords (Freiwald et al., 2004; Roberts et al., 2009; Buhl-Mortensen et al., 2015; Chimienti et al., 2019). PUs containing more than 20% terrestrial land cover were also excluded, leaving a total of 31,518 PUs covering 19,698,750 km2 of the North Atlantic and the Mediterranean (Figure 2). Scenarios that included connectivity were run on a reduced area matching the boundaries of the VIKING20 oceanographic model (Böning et al., 2016) used as input for Lagrangian larval dispersal modelling (Gary et al., 2020). This smaller area comprised 22,347 PUs (70.9% of the total planning region, Figure 2). An Albers equal-area conic projection centered in the study area (origin of latitude 30°N and longitude 30°W) was used during spatial data extraction, conservation prioritization and mapping.
Given its large spatial scale, the planning region was divided into 13 sub-regions or provinces (11 in the North Atlantic and 2 in the Mediterranean) to target a geographically representative protection of species and habitats, and enhance conservation network replication. These 13 provinces (Figure 2) were delineated with reference to three criteria that capture large scale habitat heterogeneity: (1) the lower bathyal Global Open Oceans and Deep Seabed (GOODS) biogeographic provinces classification (UNESCO, 2009); (2) the identification of major geographical barriers separating the abyssal plains located east and west of the Mid-Atlantic Ridge, as well as the Gibraltar Strait separating the Atlantic from the Mediterranean; and (3) depth, distinguishing between shallow (<800 m) and deep (>800 m) areas corresponding to the upper and lower bathyal biogeographic regions (UNESCO, 2009). The shallow provinces thus include the upper bathyal zone as well as the continental shelf (<200 m), while the deep provinces represent the lower bathyal zone down to where it transitions into the abyssal domain (ca. 3,500 m). Conservation targets for each conservation feature were applied in each of the 13 provinces. This ensured that in all solutions the same percentage of each conservation feature was protected within each province across the whole planning region.
Conservation Features and Targets
Conservation Features
In our study, we considered four main types of conservation features across the North-Atlantic and the Mediterranean: (1) known bona fide VMEs and areas where VMEs are likely to occur, (2) predicted suitable habitat of VME indicators, (3) predicted climate change refugia for VME indicators and deep-sea fish, and (4) geomorphologic features.
Vulnerable marine ecosystems
We assembled datasets of records from both the Atlantic and the Mediterranean (Combes et al., 2019; Morato et al., 2021), based on the lists of VME indicator taxa or VME habitat defined by the ICES Working Group on Deep-water Ecology (WGDEC) and GFCM Working Group on VMEs (ICES, 2016, 2019; GFCM, 2018). Such records were compiled from the ATLAS “North Atlantic Ocean basin-scale VME index dataset” (Morato et al., 2021), which is currently comprised of ∼455,000 records distributed in both sides of the North Atlantic and within the Mediterranean: 38,400 records from the ATLAS partners1, 315,000 from the Ocean Biogeographic Information System portal2 (OBIS), 71,500 from the NOAA Deep Sea Coral Data Portal3, 30,000 from the ICES VME database4 and 100 from the InterRidge Vents Database v.3.45, and further complemented by a literature review to list all known cold-seep ecosystems in the North Atlantic and the Mediterranean, of which 41 occurred in the planning region (Supplementary Text 1).
Known bona fide VMEs were defined as records for which there is unequivocal evidence for a VME, e.g., hydrothermal vents and cold seeps, in-situ ROV observations of a coral reef or significant bycatch of a VME indicator species. On the other hand, areas where VMEs are likely to occur were defined as records of VME indicators that suggest the presence of a VME with varying degrees of uncertainty. Based on these records, the VME index, originally described by Morato et al. (2018), involved the calculation of two indices: (1) a VME index score based on indicator species taxonomy and abundance giving an indication of the likelihood that a PU could contain VME habitat and (2) an associated confidence score based on source data quality. Based on the combined scores, individual records could then be assigned to one one of three levels of VME likelihood (low, medium, or high) using the Jenks natural breaks classification method (Jenks, 1967). In our study, the known VMEs and the areas categorised with a “high,” “medium,” and “low” VME likelihood (Supplementary Figure 1) were each associated with a conservation target.
Vulnerable marine ecosystems species and demersal fishes: present and future predicted habitat suitability
Because the spatial distribution of VME indicators in the deep sea is still poorly known, it is common practice to incorporate the outputs of species distribution models (or habitat suitability models) in prioritization exercises (Kenchington et al., 2019a). In this study, we included the predicted habitat suitability index of a number of VME indicator species and deep-sea fish species (González-Irusta, unpublished data; Morato et al., 2020; sponge HSM unpublished data) in the prioritization approach. Six coral species and one sponge species were selected as VME indicator species because they can form diverse VME habitat types in the North Atlantic and in the Mediterranean (Supplementary Figures 2,3). The coral species included three scleractinians with aragonitic skeletons (the reef-building colonial species Lophelia pertusa6 and Madrepora oculata, and the solitary cup coral Desmophyllum dianthus), and three octocorals with calcitic skeletons (Acanella arbuscula, Acanthogorgia armata, and Paragorgia arborea). The selected sponge species Geodia barretti is a boreal species forming sponge grounds on both sides of the Atlantic (Cárdenas et al., 2013). Six demersal fish species, occurring below 200 m depth and where the largest bottom-fisheries take place on the North Atlantic (Watson and Tidd, 2018), were also included, as securing the stocks of these highly targeted species appears essential to work toward bottom-fishing sustainability. These species were the roundnose grenadier (Coryphaenoides rupestris), the Atlantic cod (Gadus morhua), the bluemouth rockfish (Helicolenus dactylopterus), the American plaice (Hippoglossoides platessoides), the Greenland halibut (Reinhardtius hippoglossoides) and the beaked redfish (Sebastes mentella). Most occurrence records of these fish are in the northern half of the North Atlantic, thus the use of such fisheries-targeted species would only affect the SCP in this constrained latitudinal range, in contrast with the other types of conservation features that were spread through the whole planning region.
In order to assess possible climate change impacts, Morato et al. (2020) used habitat suitability modelling to forecast potential shifts in species distribution from the present-day (1951–2000) to future climate change RCP 8.5 scenarios (2081–2100) for the 12 species used in the study. The modelling approach hence resulted, for each species, in two maps of the predicted suitable habitat under both present and future environmental conditions (Morato et al., 2020). Given uncertainties associated with predicting future conditions and, hence, habitat suitability, future predicted species distributions were only used to identify potential species climate refugia. Climate refugia are defined as areas predicted as suitable habitat for the modelled species both during the present (1951–2000) and under future conditions (2081–2100), i.e., areas of suitable habitat that overlap between the two time periods.
While the emphasis of our study is on VME species, it is useful to consider the longer-term evolution of fisheries by including predicted climate refugia for commercial fish species. Thus, conservation scenarios included: (1) for the VME indicator species, predictions of both present suitable habitat (Supplementary Figure 2) and future climate refugia (Supplementary Figure 3) and (2) for fish species, only the prediction of climate refugia (Supplementary Figure 4).
Geomorphologic features
Three geomorphologic features known to provide a physical environment for VME indicator species were also considered in our planning scenarios (Supplementary Figure 5) (1) the seamounts extracted from Yesson et al. (2011), (2) the fracture zones along the Mid-Atlantic Ridge, determined from the GEBCO Gazetteer of Undersea Features Names7, and (3) the shelf-incising canyons (i.e., which have a shelf origin and continue toward the margin slope), measured by their total length (km) in each PU, calculated from the dataset of Harris and Whiteway (2011).
Conservation Targets
The attribution of targets for all conservation features was based on the application of simple decision rules (Table 1). Firstly, conservation targets were based on the rarity of the conservation feature within the planning region (Supplementary Table 1); higher conservation targets being set for rarer features. The conservation targets were fixed at 60, 50, or 30% for features covering less than 5, 5–10, or 10–25% of the planning area, respectively (Table 1). Secondly, targets were adopted to conserve areas predicted to act as species’ climate refugia. The potential reduction of species habitat in the future was estimated by comparing current and future predictions of suitable habitat for each species (Figure 3). Climate refugia were here chosen as the most conservative (or restrictive) representation of the future distribution of the species, as if species could not extend their habitat in areas that are currently unsuitable, thus resulting in the highest future potential reduction. For five species that were predicted to have a suitable habitat reduction under future environmental conditions of at least 70% (the three gorgonian corals, one scleractinian coral and the sponge), climate refugia were regarded as crucial areas for the species viability and thus these areas were prioritised by increasing their conservation targets by a further 20% (Table 1). Finally, for areas where VMEs are likely to occur a target of 15, 30, or 50% were assigned to those PUs with low, medium, or high VME index, respectively. Hence, the higher conservation targets of 70–80% were assigned to rare features (<10% cover) that act as climate refugia. Such targets were high relative to those generally used in conservation planning, from the 10% Aichi targets (CBD/COP10) to the 50% targets (Wilson, 2016; Dinerstein et al., 2017), but were here regarded as a scientific recommendation to ensure protection and buffer the uncertainties associated with habitat models and climate predictions (in line with our general approach of adopting the more conservative methods for prioritization).
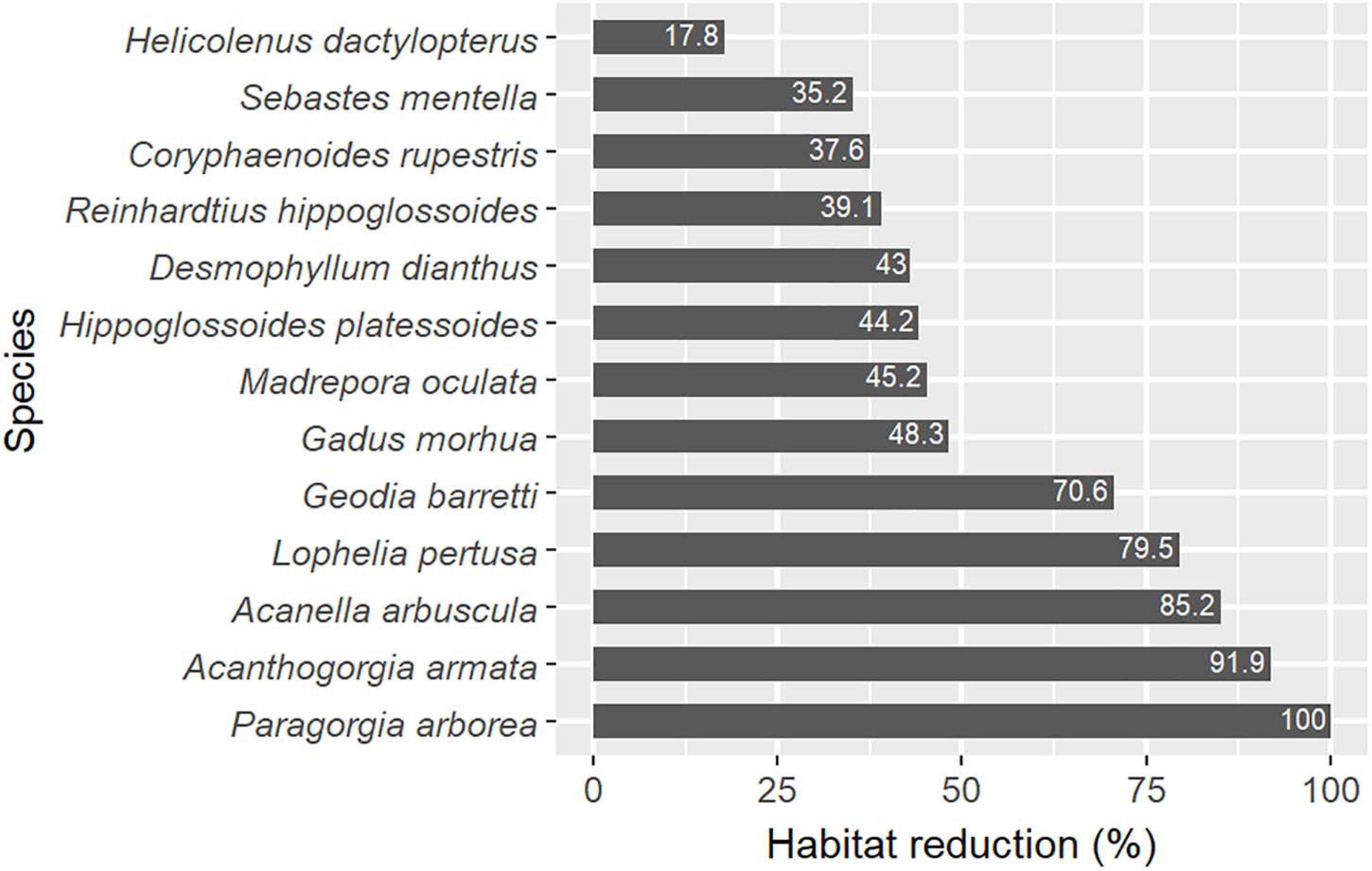
Figure 3. Relative habitat reduction between present suitable habitat and future climate refugia for the 13 selected species.
Costs and Constraints
A common objective during SCP is to minimize conservation costs while achieving conservation targets (Ban and Klein, 2009). One way to achieve this is to favour solutions that promote cost-efficient management and/or minimize socio-economic impacts. In this study, we produced different scenarios based on two cost and constraints approaches: (1) constant area-based cost and (2) varying cost that accounts for current conservation measures and current bottom-fishing activities.
Area-Based Cost
A constant area-based cost, minimizing the total area of the network, is used as all the PUs have identical areas. In that case, the prioritization does not account for spatial heterogeneity in management or socio-economic activities. A cost-calibration analysis showed that increasing the value of a constant area-based cost constrained the number of PUs in solutions. The area-based cost was set either to 1 (low cost) where PUs selected to provide a solution were lowly restricted, or to 10 (high cost) which imposed a more restricted selection of PUs and a higher influence of combined costs and penalties on the solution.
Existing Conservation Measures and Ecologically or Biologically Significant Areas
Protected areas are often forcibly included in conservation solutions, so the prioritization algorithm complements the existing conservation network with new areas. In this SCP framework, we adopted a more flexible approach, by not locking-in, but rather favouring areas that already benefit from legal protection (MPAs) or that are considered as priority for conservation due to their ecological significance (EBSAs). Thus, we created an inventory of all the MPAs and EBSAs that were in place in the Atlantic and Mediterranean in 2019. These included fishing closures implemented to protect VMEs by the north Atlantic RFMOs (NAFO and NEAFC) and the GFCM in the Mediterranean, MPAs from the World Database on Protected Areas8 (WDPA) complemented by national databases for Norway9, Canada10 and the United States11, and CBD12 EBSAs in the northwest Atlantic, Caribbean, and Mediterranean regions. These areas were subsequently categorised according to their level of protection (Supplementary Figure 6): (1) High – for areas restricting human activities, e.g., RFMOs’ fishing closures and “marine reserves,” i.e., MPAs providing full no-take zones, closed to bottom-fishing, and/or those corresponding to IUCN category “Ia” or “Ib”; (2) Medium – for areas with less restrictions such as MPAs not classified as “reserves” and a GFCM fishing restricted areas in the Gulf of Lions, France; (3) Low – for CBD EBSAs, which are indicative areas and not under any management regime. When different protection measures overlapped in the same area, the stricter protection category was retained.
Bottom-Fishing Activities
Planning units that contain areas of high economic value (e.g., fisheries) can be assigned a higher cost so they are less likely to be included in conservation solutions. We extracted the fishing catch landing value for towed bottom-impacting gears (dredges, bottom trawls, and Danish seines) using the Global Fisheries Landings database (Watson, 2017; Watson and Tidd, 2018). The 2010–2015 average annual catch rate (tonnes per square km of ocean) was calculated for each PU and then log transformed to lower the weight of extremely high catch values (Supplementary Figure 7). Passive bottom impacting fishing gears (e.g., traps or bottom longline) were not considered as they were fairly localised comparatively to the working spatial resolution used (25 km), their catch levels were negligible in respect to total catches, and pelagic and bottom gears were not always distinguished in the available data.
Combined Costs
The layer displaying varying costs was computed from three sets of data, which allowed the inclusion of socio-economic and spatial management aspects in the PU selection process. First, PUs cost was negatively indexed to the three levels of protection in order to favour zones that already benefit from conservation designations and acknowledge the management efforts in place. Second, PUs cost was increased in proportion to the bottom fishing catch value to penalize the selection of fishing grounds, and thus, limit the economic loss due to implementing restrictions in fished areas selected in conservation solutions (i.e., fishing considered an opportunity cost). Third, PUs containing known VMEs or assigned with a medium to high VME confidence index score were given a lower cost value and were preferentially selected to PUs with low VME confidence due to a reduced requirement to confirm the presence of VME before designating an area as an MPA.
The PU values of the cost layer were calculated following the formula:
where b is the PU raster layer; n is the number of features in the scenario (n = 24); f1, f2, and f3 are the number of features affected by protection measures (f1 = 24), fishing (f2 = 21) or VME confidence index (f3 = 1); c1, c2 and c3 are raster layers with protection (c1), fishing (c2) or VME confidence (c3) cost indices rescaled within [0,0.8].
The cost layer was computed from an area-based baseline value (b) of 10, which then was made to vary according to the weighted sum of three cost indices (c1, c2, and c3). These latter described the range of cost variation applied to the baseline, from 0 to 80%, either following fixed values per categories (0.25, 0.5, and 0.8 for lower to higher levels of protection; 0.5 and 0.8 for medium and high VME confidence) or a continuous gradient (fishing catch). The three indices were weighted depending on the proportion of features likely affected by each kind of cost (). The protection and fishing cost indices had a high weight (1 and 0.88, respectively) as these activities potentially impact many species and habitats. On the contrary, the VME confidence index was only related to the VME feature and thus had a low importance in the final cost value (weight of 0.04).
Deep-Sea Mining Constraint
Finally, a spatial constraint consisted in the exclusion from the solutions of 106 PUs that contained deep-sea mining exploration zones, under contract with the ISA (Supplementary Figure 7). Scenarios including this constraint considered that SCP could not be implemented in these areas although efforts are underway to develop a Regional Environmental Management Plan for the Atlantic which will include conservation areas (International Seabed Authority, 2018).
Spatial Penalties
Spatial penalties enable trade-offs on the cost of PUs. We applied a “boundary penalty,” known as the boundary length modifier (BLM) in Marxan, that penalizes solutions that are excessively fragmented (Hanson et al., 2019b). A penalty value of 0.0001 was found to provide a suitable compromise between too fragmented and too clumped solutions and was applied in all scenarios run without connectivity.
Connectivity was addressed by adding penalties to favor solutions that selected combinations of PUs with high connectivity between them (Hanson et al., 2019b). A matrix of the probability of connectivity between each pair of PUs was derived from the outputs of the ARIANE Lagrangian particle tracking model (see Gary et al., 2020). This model tracked the trajectories of almost 500 million hypothetical pelagic larvae of benthic species with ocean circulation conditions over a 50-year period (1959–2008) generated by the VIKING20 model (Böning et al., 2016). Particles were released in each PU, at the surface (20 m) and at the seabed, and at 1,000 m if water depths were deeper than 1,000 m. The different release depths were a simplified way to model different larval behaviors which have been observed in deep-sea benthic species: drifting near the seabed, swimming up to the surface, swimming clear of the seabed but not right up to the surface. Particles were passively drifting with the prevailing currents for 20 days, corresponding to the shorter end of the range of many deep-sea pelagic larval durations (PLDs) (Hilário et al., 2015). This was of sufficient duration to identify connectivity pathways that could be factored into our models, but it should be noted that in addition to the PLD, the competency period (i.e., at which time larvae start heading downward to find somewhere to settle) and larval behaviour will have significant influence on actual connectivity patterns (Gary et al., 2020). The degree of connection between PUs was calculated as the proportion of particles released from a source PU passing through any other PU within 20 days. The connectivity penalty thus favoured solutions that maximise the number of larvae retained within the conservation network.
Spatial Prioritization
Scenarios
A total of 47 scenarios were formulated for the ATLAS SCP approach, for which conservation features, conservation targets, penalties (costs and connectivity) and constraints vary. Four of the more complex scenarios are presented and compared in this paper (Table 2). For the “Base” scenario, the 24 features and associated conservation targets were replicated within the 13 provinces across the full planning region, and an area-based cost with a low value (1) was used during the planning exercise. The “Base” scenario was rerun in the smaller planning region used for the “Connectivity” scenario (Figure 2B) that was limited by the coverage of the ocean circulation model used in particle tracking and called the “Reduced Base” scenario. In the “Connectivity” scenario, the replacement of the boundary penalty by the connectivity penalty allowed us to consider larval dispersal to achieve a more resilient conservation network. The area-based cost was increased to 10 in this scenario to increase the influence of the connectivity penalty on PUs selection. The area-based cost was similarly increased for the “Reduced Base” scenario to facilitate comparisons. Finally, the “Management” scenario involved more complex costs and constraints to prioritize areas according to the current protection and uses of the deep sea. The aim was therefore to find solutions that maximize the spatial overlap with current protection measures (PUs with low cost), while minimizing the overlap with fishing (PUs with high cost) and deep-sea mining (excluded PUs).
Solving and Evaluation
The Gurobi solver (Gurobi Optimization LLC, 2018) was used to solve the prioritization problems. For most scenarios, 100 runs were required using the “cuts” portfolio method (Rodrigues et al., 2000; Hanson et al., 2019b), and the 30 best solutions, i.e., the less costly solutions, were picked from the 100 initial runs (see Supplementary Figure 8 for cost evolution through solving). Connectivity problems were computationally heavy and particularly long to solve thus limits were placed on the calculations: only 30 runs with a computational time limit of 10,000 or 15,000 seconds were permitted. Outputs were evaluated using the selection frequency of PUs, reflecting how often they were included within the 30 best solutions. For each scenario, the dispersion of the number of selected PUs across the selected solutions was assessed using boxplots. The selection frequencies approach was preferred to using the binary output of a single solution, because it provides more flexibility in suggesting a conservation network solution. Thresholds of PUs selection frequency were used to determine the importance of their contribution toward the conservation objectives:
• PUs that were selected at least once across solutions contribute to the objectives;
• PUs that were selected in at least 50% of solutions make a relatively important contribution to the objectives;
• PUs that were selected in at least 75% of solutions make a highly important contribution to the objectives.
Finally, the PUs selected in at least 50–75% of solutions within the “Management” scenario were used to delineate two suggested priority networks for ocean-basin conservation. The representativeness of the prioritization elements (features, protection levels, and fishing catch) was assessed in these two priority networks and their conservation value compared with existing MPAs and CBD EBSAs.
Data Management and Access
The R code used to structure and run the scenarios, together with links to the scenarios’ input layers, the conservation targets table, the connectivity matrix resulting from the 20 days PLD larvae drift modelling and the final scenarios’ outputs are available online (Combes and Vaz, 2019).
Results
Base Scenario
This conservation planning scenario covered the whole planning region (Figure 2). The PUs most frequently selected in the solutions were mainly located along the slopes of the continental margins on both sides of the Atlantic, on the southern coasts of Greenland and Iceland, along the Mid-Atlantic Ridge, on the north of the Gulf of Mexico, and for the Mediterranean, along its western coasts, the Tyrrhenian Sea and the north and west of the Ionian sea (Figure 4). Large shallower expanses of the upper slopes and continental shelves (northern part of the North Sea, north of the British Isles and the Canadian shelf from the St. Lawrence Gulf to Baffin Bay) were also selected but with more moderate frequency (Figure 4). The individual solutions computed for this scenario covered approximately 19% of the planning region on average. This distribution of PU selection frequencies in the “Base scenario” seems to fit the overall distribution pattern of the selected features (Combes and Vaz, 2019; Morato et al., 2020). With regards to the invertebrate species, their present-day distribution and particularly their future climate refugia, appear mostly on margin slopes, sometimes predicted exclusively in those areas (Supplementary Figures 2,3). For instance, the refugia of A. arbuscula, A. armata, L. pertusa, and G. barretti, which were all small in extent and thus had high conservation value, formed thin ribbons of highly selected PUs along the North-Atlantic margins. In general, fish species’ climate refugia were located on the northern continental shelves above 500 m depth (Supplementary Figure 4), with some of them (R. hippoglossoides and S. mentella) exclusively found in cold waters as far as the Arctic. VME habitat and geomorphotype features displayed a more scattered distribution, mainly along margin slopes of the Atlantic, on the Mid-Atlantic Ridge and on the northern part of the Mediterranean (Supplementary Figures 1, 5).
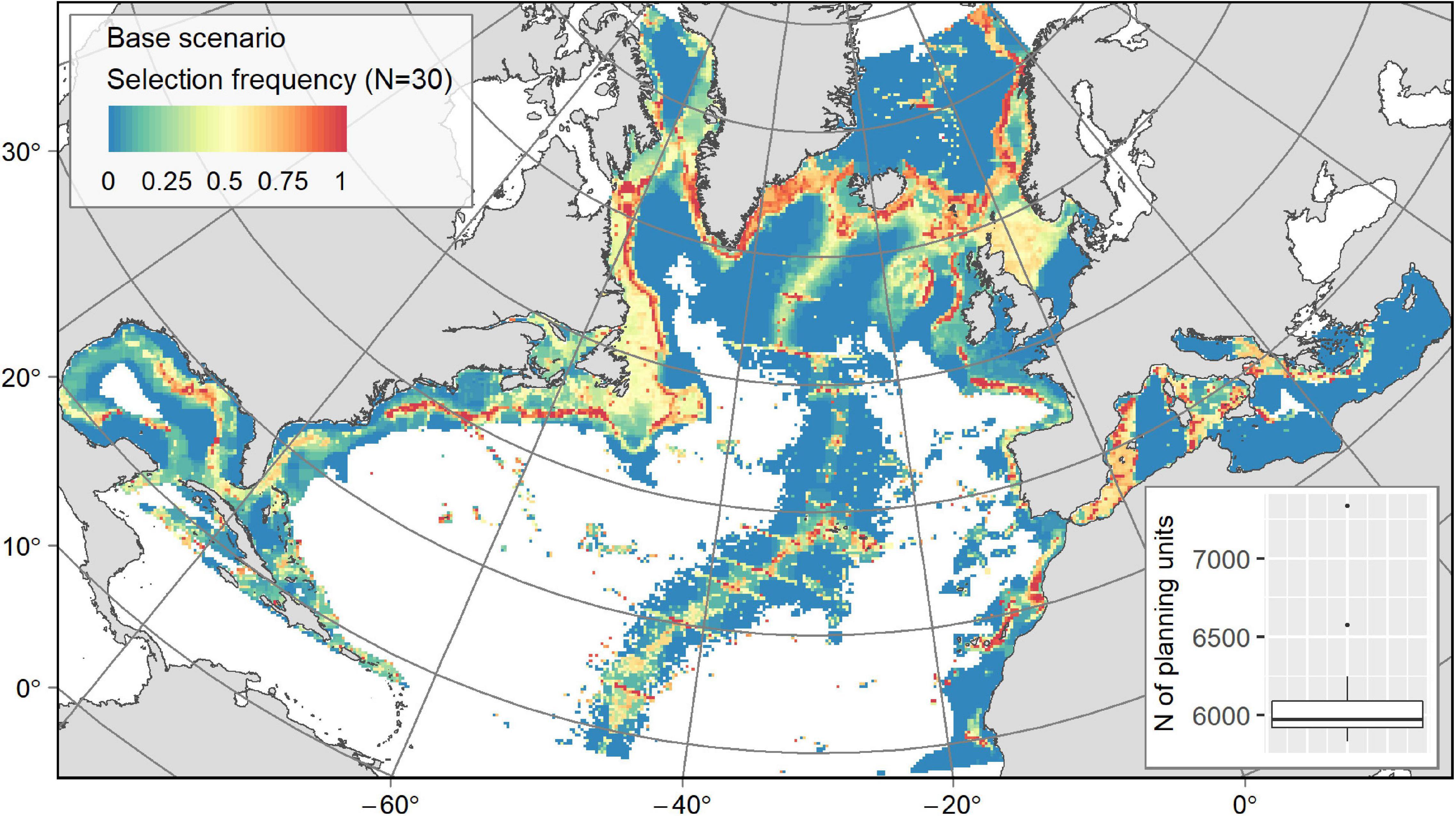
Figure 4. Output from the “Base” scenario: map of PU selection frequency from the 30 solutions with a boxplot showing the number of PUs in the solutions.
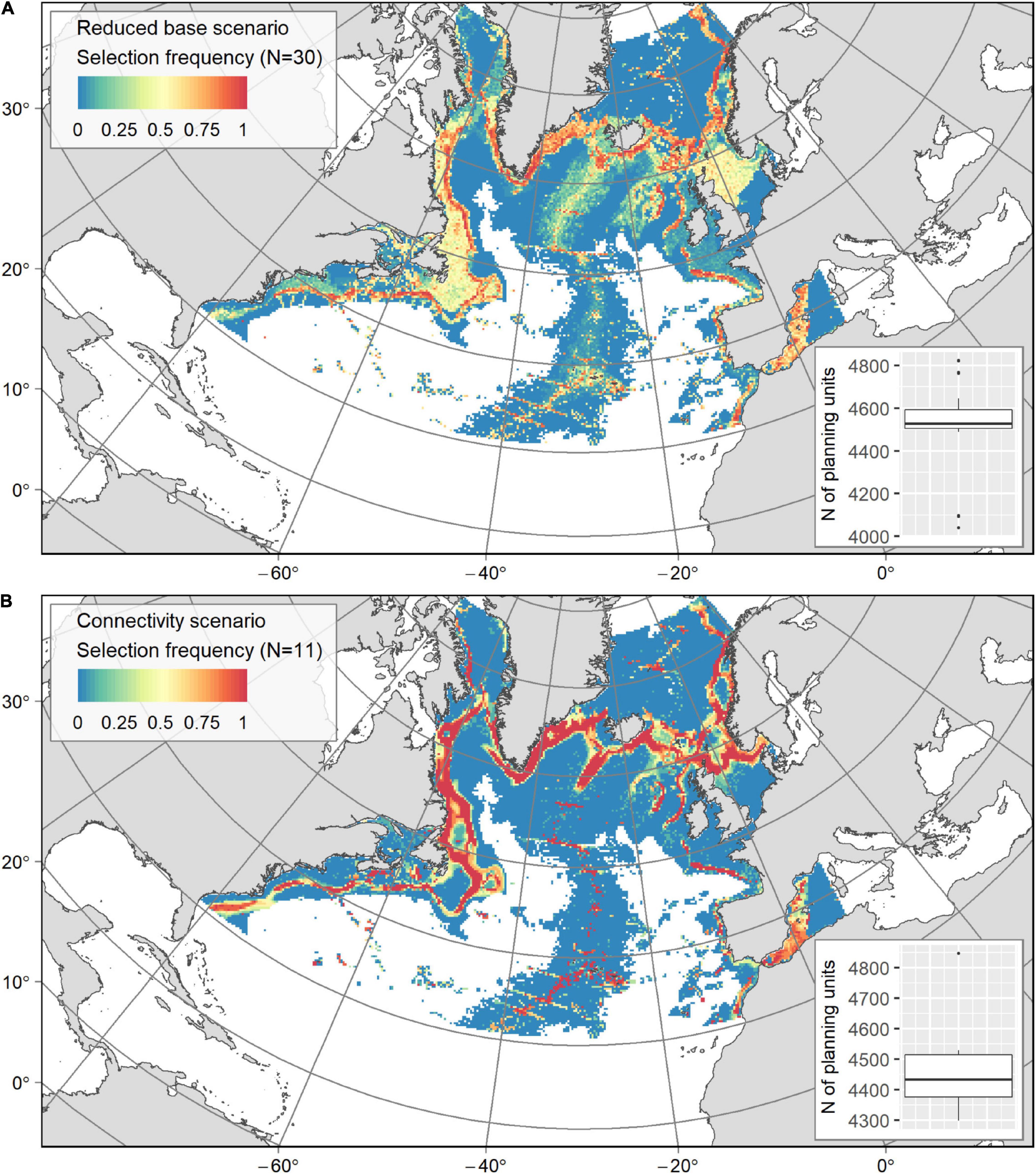
Figure 5. Outputs from (A) the “Reduced Base” scenario and (B) the “Connectivity” scenario: maps of PU selection frequency from the N solutions with a boxplot showing the number of PUs in the solutions.
Connectivity Scenario
In order to test the influence of including connectivity in our SCP solutions, a “Reduced Base” scenario was re-run within the smaller planning region used for the connectivity scenario. The output was similar to the larger area “Base” scenario. The “Reduced Base” output (Figure 5A) was compared with the “Connectivity” scenario output (Figure 5B) that maximised connectivity. Replacing the boundary penalty with a connectivity penalty did not change the broad distribution of conservation solutions but tended to constrain selection frequencies so that less PUs contributed to the solutions (Table 3) resulting in highly selected areas systematically forming a continuous and compact conservation network (Figure 5B). Hence, the connectivity scenario conserved much less of the planning region when focusing on the PUs with selection frequencies of ≥50% or those that were selected at least once (21 vs. 16% and 50 vs. 32%, respectively). Both the “Reduced Base” and “Connectivity” scenarios conserved c. 10% of the planning region when only PUs with a selection frequency of >75% were retained. However, the use of connectivity did not affect the number of PUs in individual solutions, ranging approximately from 4,400 to 4,600 PUs (19.7–20.6% of the planning area) for both scenarios (Figures 5A,B).
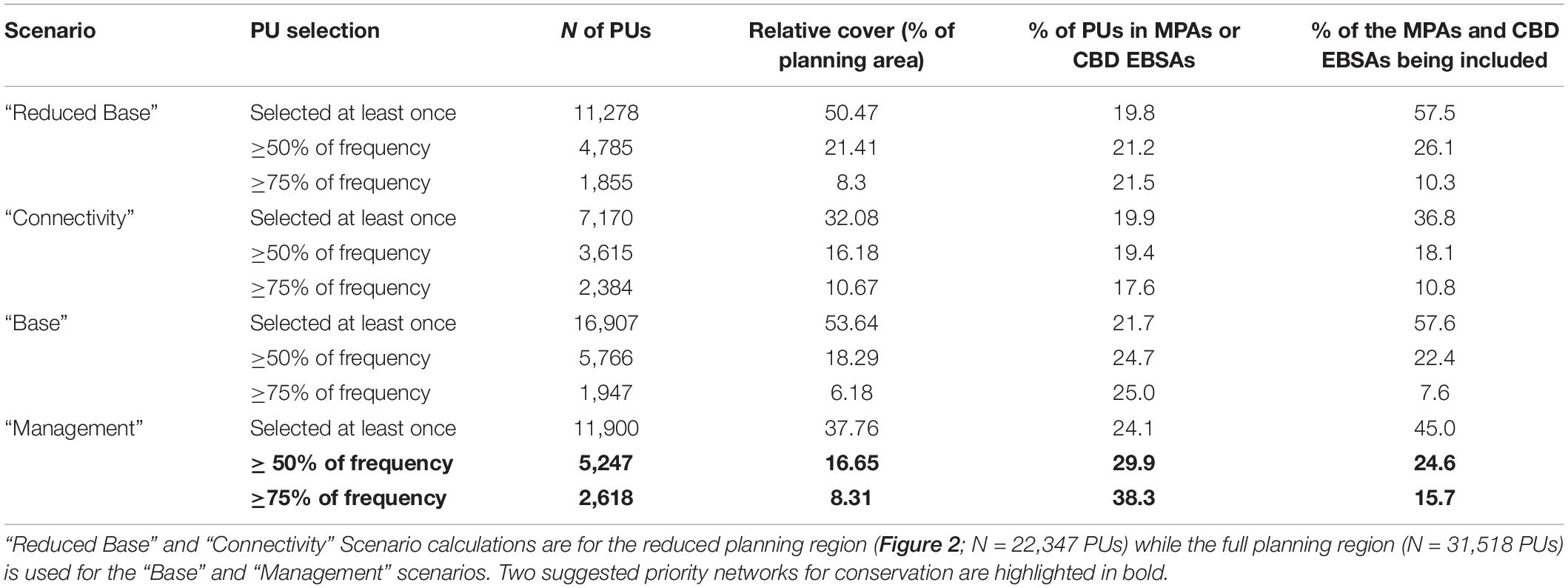
Table 3. Number of selected PUs in the different conservation solutions based on selection frequency thresholds (PUs selected at least once; in ≥50% of the solutions; or in ≥75% of the solutions).
Management Scenario
The “Management” scenario has the aim of finding solutions that maximize the selection of current conservation measures while minimizing the overlap with human activities. In this scenario, the socio-economic costs were assigned to PUs ranging between 1.8 and 17, with the baseline value of 10 predominating (Figure 6A) based on the value of fish caught or the presence of MPAs and likely VMEs. The lower cost values (least penalised) were attributed to MPAs and fishing closures in the High Seas where bottom-fishing was absent. The lowering of cost values for MPAs inside EEZs (Supplementary Figure 6) was partly counterbalanced due to fishing occurring in the same areas (e.g., parts of the US shelf, Celtic Sea and North Sea, northwest Mediterranean, Figure 6A). The higher cost values induced by high fishing activity were found along the Atlantic coasts and on large shelf areas, such as the North Sea, Celtic Sea and the Canadian shelf, and to a lesser extent in the Mediterranean, within the Alboran Sea and the coasts of Italy (Figure 6A). Existing MPAs, fishing closures or CBD EBSAs cover 19.7% of the planning region, of which 4.6% are fishing closures or marine reserves, 8.7% are MPAs with lesser protection (i.e., without no-take zones or strong fishing restrictions) and 6.4% are EBSAs (which do not mandate any management measures, Supplementary Figure 6). Current fishing closures and reserves with adequate enforcement protect from 0 to 16.5% of the target conservation features, with an average area extent of 7.2% (Supplementary Table 1). Importantly, existing levels of protection of potential climate refugia for 8 of the 13 species examined in this study are currently below 6% (Supplementary Table 1). Deep-sea mining areas are all situated in the same province (“Deep 6,” Figure 2), and overlap with the “hydrothermal vent fields” EBSA designated along the Mid-Atlantic Ridge almost entirely (Supplementary Figure 6).
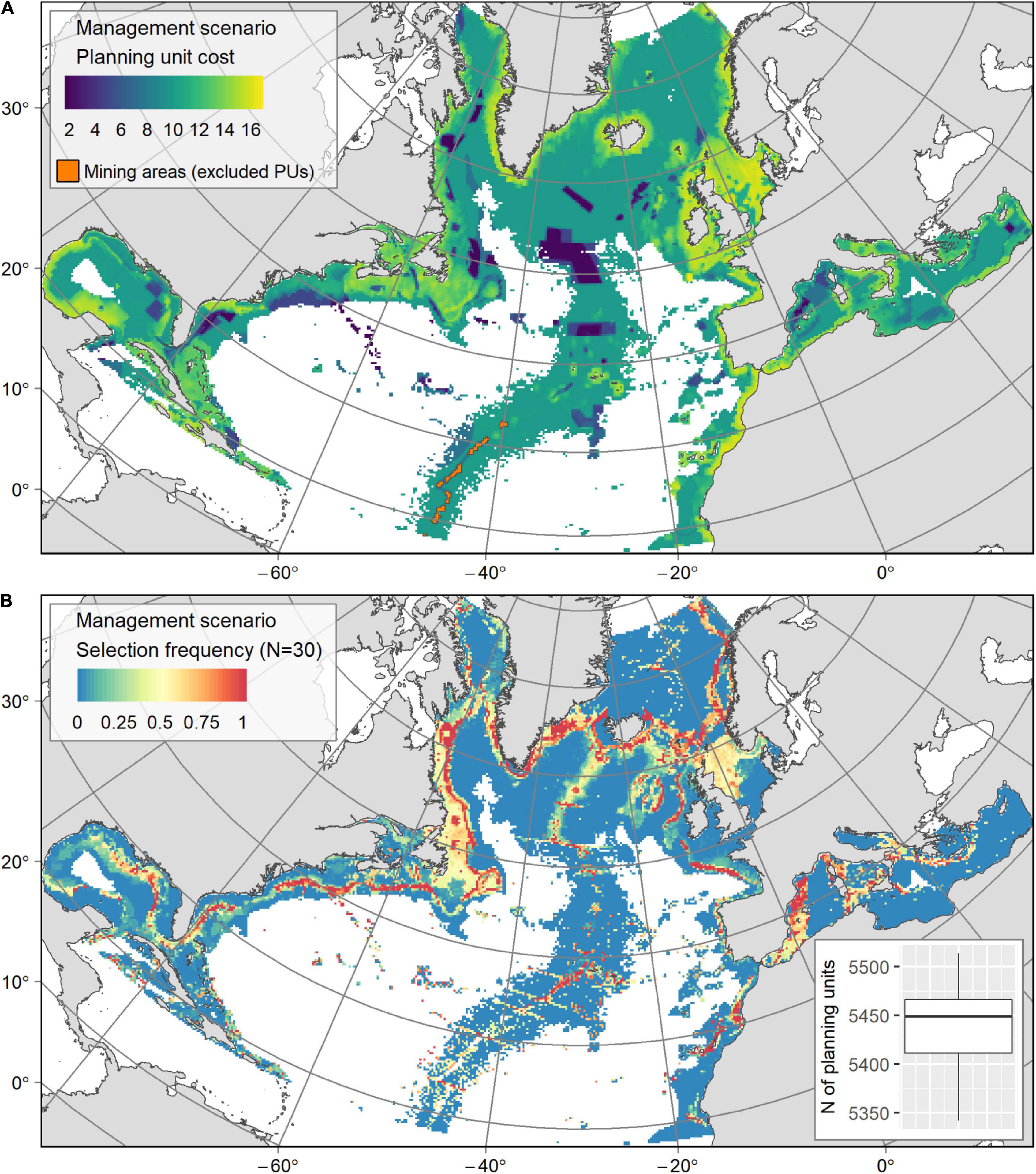
Figure 6. (A) Socio-economic cost layer used in the “Management” scenario, generated from the distribution of bottom fishing catch, the presence of MPAs or CBD EBSAs and the VME confidence index. Blue PUs (cost lower than 6) highlight the MPAs in which the fishing catch was relatively low, while yellow PUs reveal areas of high fishing catch. PUs containing deep-sea mining activities (in orange) were excluded from the conservation solutions. (B) Output from the “Management” scenario: map of PU selection frequency from the 30 solutions with a boxplot showing the number of PUs in the solutions.
While the areas selected as having conservation value in the “Management” and “Base” scenarios are broadly similar (Figures 4, 6B, respectively), their selection frequency varied (Table 3). Indeed, 30% less PUs were selected in solutions for the “Management” scenario, while the number of highly selected PUs (≥75% of selection frequency) increased by one third (Table 3) as would be expected existing conservation areas were favoured in solutions by their lower cost. The percentage of PUs selected in existing MPAs and EBSAs increased from 25% in the “Base” scenario to 38% in the “Management” scenario (Table 3). Similarly, this was reflected in the contribution of existing MPAs and EBSAs in the conservation solutions (an increase from 7 to 15.8 %) linked to their cost premium up to −80%. In the High Seas, solutions captured fishing closures in the NAFO regulatory area: Flemish Cap, New England and Corner seamounts; and in the NEAFC regulatory area: middle and northern Mid-Atlantic Ridge and Hatton Bank (Figure 6B). In EEZs, the selection of MPAs and EBSAs increased where the fishing pressure was relatively low, along the coasts of the US (mainly Florida, including its cold-water coral Habitat Areas of Particular Concern and snapper MPAs) and Canada (e.g., in several cold-water coral marine refuges and cod closures) and along the northern Mediterranean coast (e.g., in the large MPAs of the Pelagos Sanctuary and the Spanish Whale Migration corridor, that are cetaceans MPAs but encompass large deep-sea ecosystems and notably canyons) (Figures 6A,B).
The increase of cost indexed to fishing catch, which generally occurred in coastal areas and over large shelf areas (Supplementary Figure 7), reduced the selection frequency of these areas but did not exclude them from conservation solutions, likely because they hold significant potential for demersal fish conservation (Figure 6B). In both the “Base” and “Management” scenario solutions, median fishing catch values in selected PUs appeared to be higher than those obtained for the planning region as a whole (Figure 7).
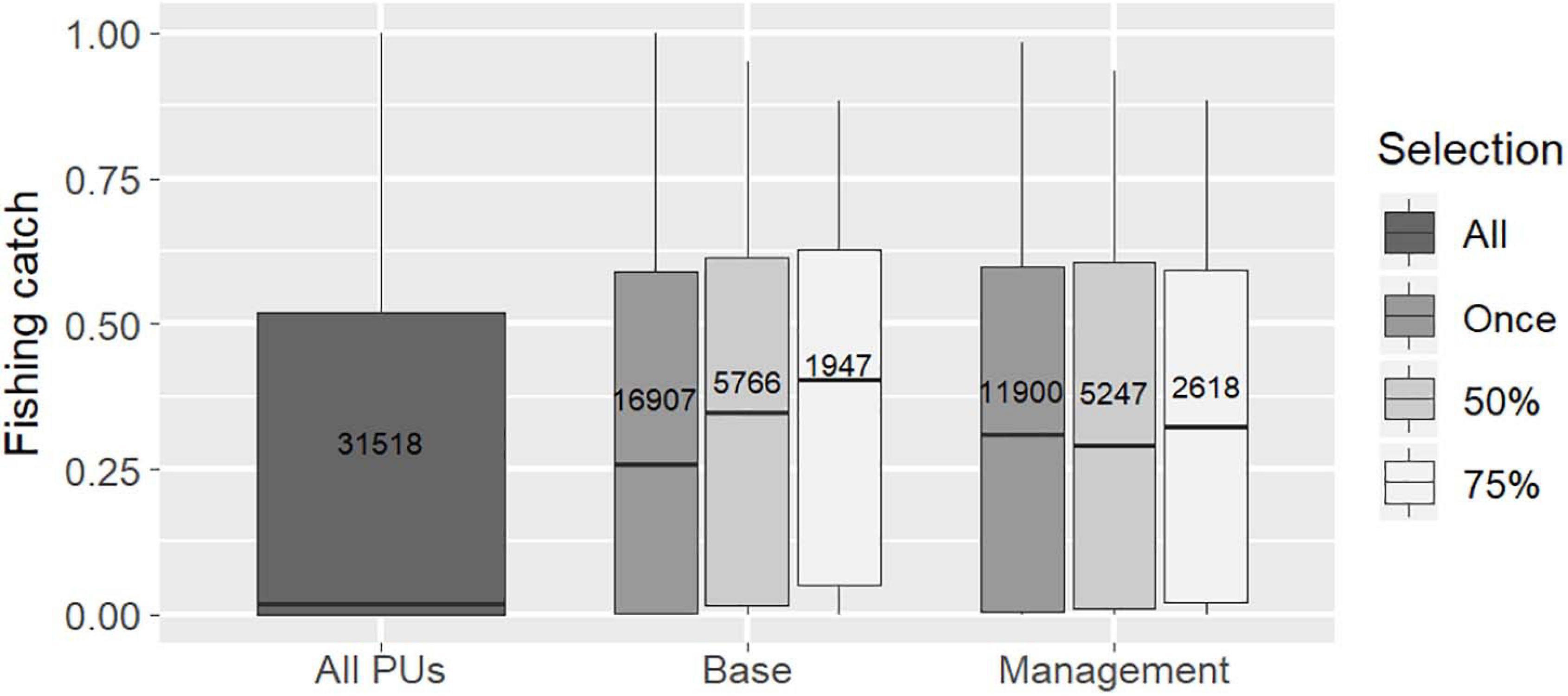
Figure 7. Distribution of costs associated with the fishing catch index (log of fishing catch +1, rescaled between 0 and 1) and total number of PUs selected (in box) for all PUs and for “Base” and “Management” scenario solutions (according to frequency of PU selection in solutions: selected at least once, ≥50% or ≥75%).
Finally, removing areas covered by existing sulphide mining exploration contracts from conservation solutions did not change the general output, because of the small size of the excluded area (Figure 6A). However, the loss of potential conservation area is compensated for by a strong selection of conservation PUs north of the mining exploration zone (Figure 6B). The “Deep 6” province contained 51.7% of all the PUs with hydrothermal vents. Sulphide exploration contracts cover 43.3% of PUs with hydrothermal vents in “Deep 6” and 23.2% of all the hydrothermal vent PUs at the basin scale. Therefore, excluding the licensed mining areas from conservation solutions removed the associated hydrothermal vents. So while the conservation target of 60% for these ecosystems –that were included in the “known VMEs”– could be achieved at the basin scale by selecting PUs further north, it was not possible to reach that target in the “Deep 6” province (with a maximum of 56.7% PUs available for conservation).
Conservation Priority Networks
Outputs from the “Management” scenario were used to identify a set of conservation priority areas (including certain existing measures) in the North Atlantic and the Mediterranean. Using selection frequency thresholds, it was possible to identify: (1) a “priority” network based on PUs that were selected in at least 50% of the scenario solutions, and (2) a “core priority” network based on PUs that were selected in at least 75% of the scenario solutions (Figure 8). The “priority” network, which covered 16.6% of the planning area (Table 3), included on average 55% of each conservation feature, exceeding the initial conservation targets in most cases (Supplementary Table 1). While the twice smaller “core priority” network included on average 37% of each conservation feature, falling short of the initial conservation targets, it still identified a conservation area extent 6 times higher than that currently covered by MPAs or EBSAs (Supplementary Table 1). The percentage of PUs in the “priority” and “core priority” networks that occurred in MPAs or EBSAs was 29.9–38.3%, respectively, with values dropping to 10.7 and 15.8%, respectively when only marine reserves and fishing closures were considered (Table 3 and Figure 8). Conversely, 75.4% (“priority” network) to 84.3% (“core priority” network) of the MPAs and EBSAs (out of which 61% and 71.2% for marine reserves and fishing closures, respectively) were not located in the selected priority networks (Table 3).
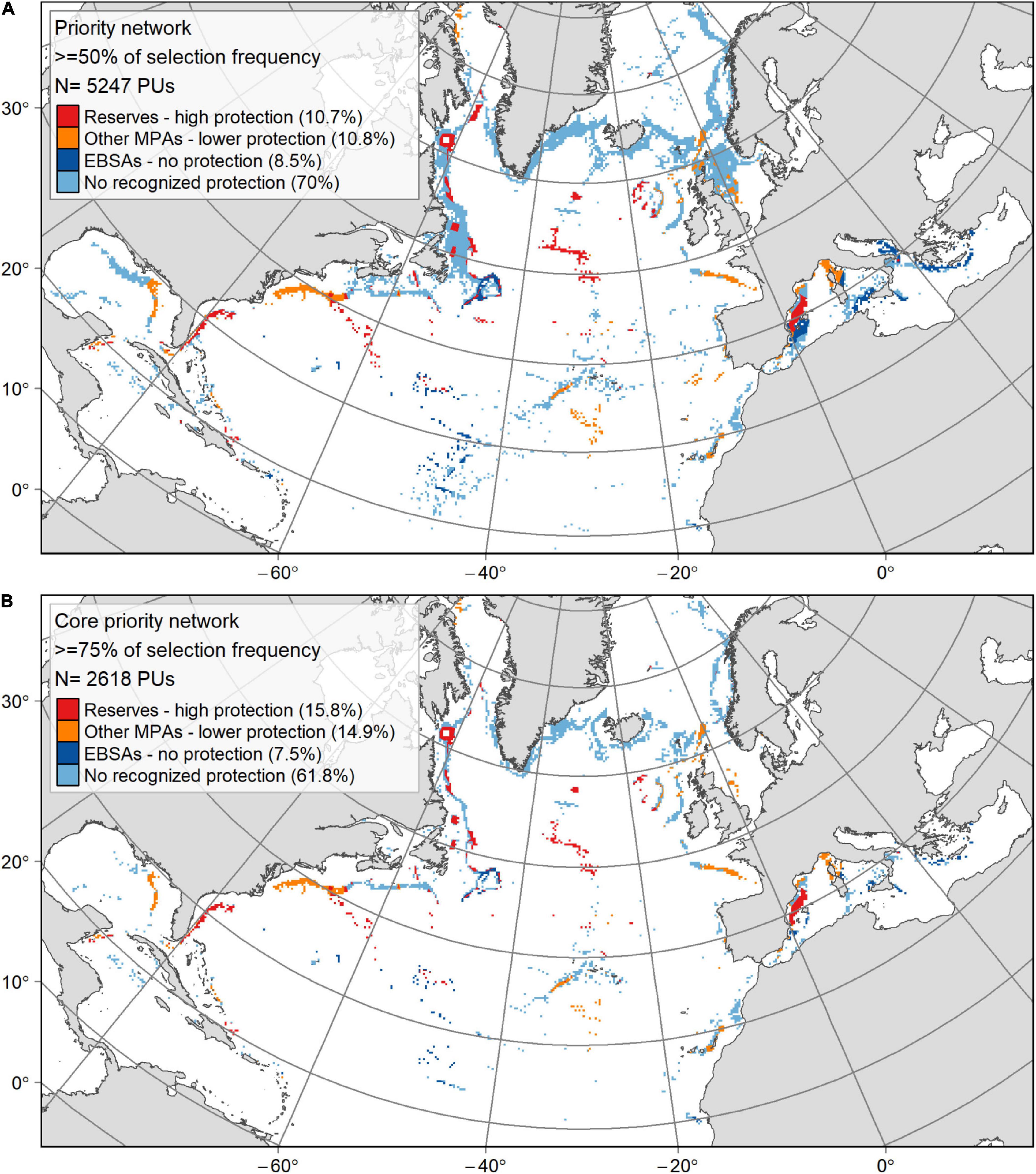
Figure 8. Maps showing the priority conservation networks selected in the “Management” scenario solutions, where PUs occurred in at least 50% (A) or 75% (B) of the selection frequency. The colour categories differentiate between current spatial management and designation categories together with their contribution (% of the priority network): (1) restrictive marine reserves and RFMOs fishing closures providing a high protection; (2) other MPAs with lower protection level; (3) CBD EBSAs with no legal protection; and (4) areas that have no protection of any kind or a protection that is not recognised as an MPA by the World Database of Protected Areas or as an EBSA by the CBD.
Discussion
To our knowledge, this study is among the first SCP exercises to employ a strategic approach to the conservation of deep-sea biodiversity at an ocean-basin scale. The spatial prioritization process developed here shows this strategy has the potential to facilitate the identification of conservation priority networks across the whole of the North Atlantic, and into the Mediterranean, down to depths of c. 3,500 m. Conservation features included VME indicators, such as habitat-forming cold-water coral and sponge species and commercially-important demersal fishes, as well as habitat geomorphotype proxies. We carried out a multi-objective prioritization using different approaches: inclusion of climate refugia, replication, connectivity, different costs and constraints, in order to assess their applicability in basin scale SCP. Continental margin slopes of the Atlantic, the Mid-Atlantic Ridge, the Western Mediterranean, the north and west of the Ionian Sea, together with the largest continental shelves containing fishing grounds, were identified as crucial areas for conservation management. Interestingly, the priority networks identified ostensibly to protect the coral and sponge species included in this study also encompass much of the predicted distribution of a number of other VME indicator species not included in this study, mostly occurring between 500 and 2,500 m depth (Davies and Guinotte, 2011; Yesson et al., 2012, 2017; Knudby et al., 2013; Howell et al., 2016). Priority networks to protect the cold-temperate fish species selected focused on securing their future climate refugia and did not include species found in warm-temperate, tropical or Arctic zones. Notwithstanding caveats, such as the patchy data availability usual in the deep sea, the investigated scenarios used in this SCP exercise were able to identify gaps in the current conservation network employed to protect deep-sea VMEs and demersal fishes. This may help drive future data acquisition programs, particularly in the Mediterranean, notwithstanding preliminary attempts to identify knowledge gaps and the need for transnational conservation strategies (IUCN, 2019). Repeating a systematic conservation approach similar to that used in this study could add value to regional conservation planning processes in the North Atlantic and the Mediterranean.
Structuring a Resilient and Replicated Network
A growing number of SCP studies highlight the importance of designing resilient protection networks that integrate climate change considerations (Levy and Ban, 2013; Magris et al., 2014, 2017; Álvarez-Romero et al., 2018b; Manea et al., 2020). In our study, more than half of the conservation features considered consisted of species’ future climate refugia. Accompanying conservation targets were set high so that solutions preferentially selected these areas having high resilience toward climate change. Above all, in the context of drastic widespread environmental changes such as ocean acidification, warming and deoxygenation, VME engineers like most skeleton-forming invertebrates, are facing serious declines by 2100 potentially leading to extinction (Veron, 2008; Jackson et al., 2014; Roberts et al., 2016; Morato et al., 2020). In this study, refugia for coral and sponge species were restricted to steep margin slopes, that together with seamounts, appear to be essential areas for their future conservation because they provide a large range of environmental conditions in a relatively small area (Tittensor et al., 2010; Lo Iacono et al., 2018). Such climate refugia (for fish or invertebrates) may facilitate recolonisation or colonisation of new areas, thus fostering long-term species viability. Therefore their protection should be considered a high priority for the deep sea (Manea et al., 2020; Morato et al., 2020). The ability of species to show phenotypic plasticity or genomic adaptation that may enhance their tolerance to climate change was not considered here.
Subdividing the planning region into smaller sub-regional provinces appears to be an appropriate method to ensure network replication and resilience, as well as for capturing elements of geographic representativeness in such a large planning area. A similar approach has already been implemented in large planning regions such as the Mediterranean Sea (Giakoumi et al., 2013), the Caribbean and the Gulf of Mexico (Schill et al., 2015) and the Great Barrier Reef (Fernandes et al., 2005), ensuring higher potential resilience for the target species and habitats. Replicating the targets set for conservation features within each province fosters the selection of a range of small to large patches across the basin, and avoids the issue of focusing solely on areas where the targets are most densely represented. This in turn increases the likelihood that conservation solutions cover basin scale ecoregion variability. Furthermore, such an approach could increase the preservation of a greater range of spatially-structured intraspecific diversity. For instance, good genetic diversity and unhindered gene flow are needed to ensure conservation measures and networks function optimally (Laikre et al., 2010). However, these aspects are rarely considered in conservation planning frameworks because they still represent knowledge gaps (Hanson et al., 2019a).
Finally, basin-scale prioritization can highlight the relative importance of each province in contributing to conservation at the basin scale. Regional managers should be encouraged to work together, adopting standardised approaches to ensure conservation targets at the regional level are attained so that basin scale targets can be achieved.
Assessing Connectivity
Despite the fundamental importance of genetic connectivity in the local and regional persistence of metapopulations (Kritzer and Sale, 2004; Cowen, 2006), and the strong influence of oceanographic features on patterns of larval retention or dispersal (Dubois et al., 2016), very few algorithms, most of them rather recent (Daigle et al., 2018; Fox et al., 2019; Hanson et al., 2019b), include connectivity in marine conservation prioritization exercises. There are other methods that can generate connectivity metrics between PUs (e.g., betweenness centrality, Google PageRank, eigenvector centrality, see (Daigle et al., 2018) or optimize network-wide metrics such as population persistence or average shortest paths (Kininmonth et al., 2011; Fox et al., 2019), but these are very computationally heavy and would demand considerable computing power and time if used in large-scale conservation scenarios such as the one tackled here.
In this study, our approach to integrate connectivity into SCP aimed at maximizing the recruitment of benthic species larvae within the reserve network by favouring the selection of pairs of PUs with high connectivity between them (Hanson et al., 2019b). This allowed us to carry out a preliminary exploration of the influence of connectivity on planning solutions. Using modelled larval drift trajectories in SCP can substantially promote the viability of protected populations by favouring recruitment within the reserve network (Dubois et al., 2016; Magris et al., 2018; Álvarez-Romero et al., 2018b, Manea et al., 2020); and even benefit fisheries by replenishing fishing grounds (Krueck et al., 2017).
We used the Lagrangian particle models of Gary et al. (2020) to track the larval drift of hypothetical sessile VME indicator species, which were the main focus of this exercise. Many benthic species have spatially fragmented distributions and for the vast majority, their dispersal capability is linked to pelagic larval drift and duration (Cowen and Sponaugle, 2009; Hilário et al., 2015). The species studied here show a diversity of life-history traits and their PLD, when this information is available, can vary from days to months (Brooke and Young, 2005; Maldonado, 2006; Larsson et al., 2014; Hilário et al., 2015; Strömberg and Larsson, 2017; Kenchington et al., 2019b). Hence, accounting for the diversity of life history traits coexisting in marine communities is a very challenging target to achieve (Gaines et al., 2010; Moffitt et al., 2011; Jonsson et al., 2016). In this study, a non-specific and simple approach was applied to model larval connectivity, likely to provide broad results that would be more relevant information for prioritization than focusing on specific dispersal strategies. However, it is important to keep in mind that dispersal patterns may be largely deterministic as they are linked to individual species larval or adult reproductive behaviour.
Accounting for connectivity during SCP resulted in a relatively coherent and continuous network with the main priority areas linked together by “corridors” of PUs. This kind of output selects important larvae pathways and hubs (in Lagrangian dispersal modelling terms) that link populations. Certain PUs that were not retained under the “Base” scenario became very relevant in the “Connectivity” scenario. This suggests that when building a conservation network, some suboptimal areas (with a lower number or abundance of target conservation features) might be important to ensure connectivity between distant populations and maintain large-scale populations’ renewal and persistence. A strong connectivity across habitats enables larvae and propagules from source areas to supply degraded deep-sea ecosystems, thus improving resilience. Furthermore, results here demonstrate that a better connected conservation network could be achieved by prioritizing PUs with high connectivity potential without the need to increase the total area of the network. Other studies found similar prioritization results, where including larval connectivity favoured the selection of more numerous or larger reserves in close proximity without increasing the solution cost or area (Magris et al., 2018; Álvarez-Romero et al., 2018b).
Whereas this study did not evaluate the performance of the current MPA network in maintaining deep-sea benthic connectivity, similar particle-tracking model assessments have been undertaken quite recently at sub-basin scale in the North Atlantic (Fox et al., 2016; Ross et al., 2017; Kenchington et al., 2019b) and at smaller scale in the Mediterranean (Basterretxea et al., 2012; Clavel-Henry et al., 2019). While the NAFO VME fishing closures in the northwest Atlantic (Kenchington et al., 2019b) and MPAs in the EEZs such as the west of the United Kingdom and Ireland (Fox et al., 2016; Ross et al., 2017) and the Mediterranean (IUCN, 2019) form networks, these are the result of ad hoc additions of new fisheries closures and MPAs over time without any attempt to take network properties such as connectivity into account. As in other regions (Schill et al., 2015; Magris et al., 2018), these studies demonstrate that such ad hoc networks fail to maintain the larval exchange pathways that are essential to replenish benthic populations. Substantial conservation network improvements could be achieved in the North Atlantic and the Mediterranean by expanding MPA networks to allow for enhanced self-recruitment and to ensure the capture of likely important sources and sinks of larval recruitment. This would result in improved within-network exchange increasing genetic variability and hence overall resilience (Ross et al., 2017; Kenchington et al., 2019b; Manea et al., 2020). However, building networks that are highly connected is extremely challenging in the deep sea since life-history traits and the behaviour of deep-sea larvae remains largely unknown (Hilário et al., 2015; Clavel-Henry et al., 2019; Kenchington et al., 2019b), thus limiting the precision of predicted connectivity patterns. Approximate estimates may be modelled, as they were here, where different release depths were used to account for larval dispersion behaviour strategies rather than model specific swimming, floating and sinking parameters.
Assessing Spatial Management and Exploitation Activities
Factoring in the costs associated with the implementation of different sizes, numbers and locations of MPAs, as well as imposing constraints to avoid areas that are already exploited or have potential to be, led to prioritization solutions that favoured the selection of a conservation network with low implementation costs while minimizing the inclusion of areas that provide economic value (e.g., for deep-sea fishing and potentially mining). This widely used approach provides a solid basis for stakeholder negotiations and eases the subsequent implementation of conservation measures by reducing conflicts between stakeholders and managers (Klein et al., 2008; Ban and Klein, 2009; Mazor et al., 2014). While the objective of such an approach is to reach a compromise between biodiversity conservation and resource exploitation or management measures in place, it can lead to a selection of areas that are sub-optimal for conservation, yet still likely to provoke less stakeholder conflict. It is therefore important to evaluate how integrating socio-economic considerations into conservation prioritisation planning will influence the solutions. There are formal methods to approach these trade-offs using multi-objective optimization methods and calculation of the Pareto frontier (Lester et al., 2013; Rassweiler et al., 2014; Fox et al., 2019), but they are generally too resource-intensive to apply to a network of this size.
It is common practice during SCP to lock-in current MPAs to ensure they are part of the conservation solution. This approach often results in sub-optimal planning solutions (Evans et al., 2015; Moore et al., 2016) because current MPAs are often designated on an ad hoc basis that may differ from the conservation objectives employed in more systematic planning. However, including existing MPAs in conservation prioritisation planning should lead to reduced network implementation costs and acknowledges prior efforts to conserve biodiversity (Manea et al., 2020). Indeed, strengthening the management of existing MPAs may sometimes be less costly and easier for stakeholders to accept than creating a new MPA. When favouring the selection of existing MPAs rather than locking them, a priori, into the planning solution, the MPAs that contribute to the SCP conservation objectives are likely to be included while MPAs that make no contribution will be excluded. Hence this approach enables integrating existing MPAs into conservation planning based on their contribution to the conservation objectives rather than the fact that they already exist. By analysing the MPAs (or parts of MPAs) selected in the conservation solutions, their relative contribution to the conservation network can be assessed.
Our study shows that current MPAs or EBSAs in the North Atlantic and the Mediterranean are inadequate for the preservation of deep-sea biodiversity. Even with their cost decreased down to −80%, three quarters of the MPAs or EBSAs within the planning region did not fall into the priority conservation areas identified in the “Management” scenario. Even the marine reserves and fishing closures, most of them explicitly targeting VMEs in the North Atlantic and the Mediterranean, were predominantly excluded from the conservation solutions as only 29% of them were included in priority networks. These restrictive MPAs, with their small coverage (4.6%) and uneven distribution across the planning region, only conserve 7% of target species or habitats, a proportion that appears largely insufficient given global conservation target goals. These results agree with the previous studies in the northeast Atlantic (Johnson et al., 2014; Evans et al., 2015) and the Mediterranean (IUCN, 2019), which highlighted the lack of an efficient and representative MPA network for conserving deep-sea habitats.
While minimizing the selection of areas with socio-economic activity to avoid conflict is a goal in prioritization, it is worth noting that areas of high conservation value may be found where activities take place. In our study, this was particularly evident for Atlantic fish populations that are exploited by bottom fishing over large areas of the shelf and for specific ecosystems such as hydrothermal vents that generate polymetallic sulphides coveted by the mining industry (Fisher et al., 2007). According to the targets set during prioritization, conserving areas of future refugia for fish species could not occur without implementing new closures on the current bottom-fishing grounds. Similarly, areas currently licensed for deep-sea mining exploration are considered to be priority conservation areas in the “Base” scenario, simply because the licensed areas include all of the known hydrothermal vent fields south of the Azores EEZ (Van Dover et al., 2018) that form the largest part of the hydrothermal vent fields identified in the Mid-Atlantic Ridge EBSA. At the level of the planning region, excluding this relatively small area could be compensated for by increasing the amount of known VMEs (in particular hydrothermal vents) in other areas included in solutions. In the management scenario, however, it wasn’t possible to reach the 60% conservation target for hydrothermal vents in the “Deep 6” province while excluding areas already licensed for mining. To achieve 100% of the targets, the potential contribution of some of the existing licensed areas would need to be considered.
Hydrothermal vent communities show high rates of endemism, symbiotic association, and extreme environmental adaptation that makes them extremely rare. Since deep-sea mining impacts and the recovery potential of hydrothermal vent communities are still unknown (Gollner et al., 2017; Niner et al., 2018), exploration for polymetallic sulphides could represent a critical threat to these ecosystems (Boschen et al., 2016; Van Dover et al., 2018). Furthermore, the concentration of mining operations in a particular province may prove particularly high risk if the region contributes significantly to the overall network connectivity. Finally, the mining footprint might expand in the future and further increase the potential impact already facing these hydrothermal vent ecosystems (Boschen et al., 2016; Van Dover et al., 2018).
Limitations
Although efforts were made to ensure results would be as comprehensive as possible by diversifying the types of data and objectives included in the prioritization, several limitations emerged during the study that should be considered in order to judiciously use these outputs in conservation management and to improve future SCP of the region.
As expected, the results obtained underline several limitations due to data availability and accuracy, reflecting the uneven distribution of deep-sea scientific data available for the Atlantic and Mediterranean basins. In fact, the main priority networks identified correspond to areas where data on VMEs and fish species was most available, further highlighting the need to continue improving our scientific knowledge, especially in data-poor regions. For instance, results obtained here show that the north and western sides of the Mediterranean were often selected in conservation solutions, whereas the southern and eastern parts were less represented. Such underrepresentation very likely reflects the paucity of deep-sea data in such areas, linked to an imbalance in the resources dedicated to deep-sea research and regional geopolitical issues (IUCN, 2019; Manea et al., 2020). Deep-sea exploration is generally targeted, focusing on areas of interest for resources or “anomalies” (e.g., areas of chemosynthetic activity, geological interest, or high biomass concentration), leading to patchy information about the distribution of deep-sea species and habitats. Habitat suitability models can be used to overcome the lack of observations over large geographical extents, but their results are highly reliant on the availability of species records where environmental conditions are relatively well described. The identified priority networks should be useful in providing conservation options where VMEs are known or likely to occur but should not be used in areas that are under-explored.
Another data gap relates to knowledge of intraspecific diversity (i.e., genomic and physiological data) allowing an appraisal of the species capacity to adapt to forthcoming environmental changes (Buckley et al., 2011; Feng et al., 2019), either through phenotypic plasticity or through genetic adaptation, as this may confound predictions of future habitat shifts and the location of potential climate refugia. Equally, little is known about the reproductive cycle and larval biology of most VME indicator species (but see Larsson et al., 2014; Strömberg and Larsson, 2017), making it difficult to predict changes in connectivity patterns and population renewal capacity under climate change scenarios.
Considering the current management and socio-economic data used to create the cost layer, the accuracy and resolution of the data used in this study was limited by the need to cover the entire North-Atlantic basin and the Mediterranean. Indeed, even if the global MPA datasets used were complemented by national datasets, discrepancies were found (United States, Canada, and Norway). Furthermore, not all local datasets could be properly investigated, potentially resulting in the missing of information inside some national EEZs. An example might be national fishing closures that have a high level of protection but are not internationally recognised as MPAs. In the future, the recognition of Other Effective Conservation Measures (OECMs) as MPA equivalents (Diz et al., 2018) by the CBD (CBD/COP/DEC/14/8), as applied in Canada, will help to capture some of the areas missed. Similarly, the fishing catch dataset used was of a lower resolution than other fishing datasets, such as the bottom trawling footprint in European waters (Eigaard et al., 2017). Data homogeneity and comparability over the whole planning region, even with coarser resolution and more approximate proxies, was favoured instead of trying to integrate patchy high-resolution sub-regional datasets with the biases that it would create.
Finally, selecting PUs based on an analysis of their selection frequencies in order to identify priority networks must be done with caution. Networks delineated based on PU selection frequencies are not prioritization solutions per se and thus do not ensure that the conservation targets of each feature will be met. While each individual solution for a given scenario is optimised to meet the conservation targets, retaining only the PUs most often selected in the overall runs does not guarantee that all targets are met in the final network. It is then essential to evaluate if the level of target completion within the priority networks is sufficient when using this delineation method. In contrast, the selection frequency provides useful information regarding the importance of each PU through the number of times a scenario was run. For instance, a PU might be highly selected because it is irreplaceable in meeting one or several targets, contains numerous features, is essential to ensure connectivity, or is situated at the core of an important area for conservation.
Recommendations
The “priority” and “core priority” networks delineated by the selection of units with high conservation importance provide useful information to focus the attention of managers engaged in future conservation planning for the North-Atlantic and Mediterranean deep sea. Covering from 8 to 17% of the planning region, the “priority” and “core priority” networks contained on average 37–55% of each conservation feature, emphasizing their high conservation potential relative to other areas. However, ensuring an adequate conservation of all deep-sea species and habitats and not just those selected for this study will require even larger areas.
Our results reinforce the need to set more ambitious conservation targets for the ocean. The current CBD target to protect 10% of marine areas by 2020 (Aichi Target 11, CBD/COP10) will likely soon be replaced by the ambitious target of protecting 30% of marine areas by 2030 with 10% declared no-take zones (CBD/COP15, see CBD, 2020), with some already calling for a “30% by 2030” no-take target (e.g., United Kingdom, IUCN, Oceans Unite, see SCP examples for ABNJ in O’Leary et al., 2019; Visalli et al., 2020) or even to reach 50% of terrestrial and marine protection by 2050 (e.g., Nature Needs Half, see Wilson, 2016; Dinerstein et al., 2017).
Despite their obvious importance, the priority areas identified in our study are currently poorly protected since existing fishing closures and reserves cover less than 16% of their extent. Moreover, our results show that bottom fishing, particularly on continental shelves, occurs within MPAs that have low levels of restriction and of course within EBSAs, as these areas are indicative and do not have any legal protection. Marine reserves permanently closed to demersal fishing appear to be the most effective tool to guarantee the long-term conservation of deep seabed ecosystems, given that benthic ecosystems may struggle to completely recover from the impacts of even infrequent bottom-fishing events (Althaus et al., 2009; Huvenne et al., 2016; Aguilar et al., 2017; Clark et al., 2019). Moreover, reinforcing and expanding the network of no-take marine reserves in the North Atlantic and Mediterranean could also contribute to local rebuilding of commercially exploited demersal fish stocks as well as by-catch species caught during fishing (Murawski et al., 2000; Kincaid and Rose, 2017; del Otero and Marin, 2019).
In addition to the results produced above, we have developed a SCP framework that may be used by decision makers to achieve future conservation goals. The framework enables the identification of priority networks based on science that can inform various MSP and management processes at the ocean-basin scale. SCP for the deep sea can help fast-track consensus around the expansion of an MPA network by facilitating engagement with stakeholders. Identified priority networks due to their conservation importance are likely to require more stringent environmental impact assessment (EIA) thresholds with accompanying mitigation measures.
Understanding where deep-sea protective measures are most needed and what level of management is required for them to achieve their conservation goals is imperative to support the setting of more ambitious conservation targets for the ocean. The SCP framework employed here and the priority conservation networks we identified can potentially support the implementation of marine spatial planning (MSP) in the deep sea via a number of avenues:
(1) The on-going negotiation of a legally binding instrument for the conservation and sustainable use of biodiversity beyond national jurisdictions (the BBNJ process), coordinated by the UN Division of the Law of the Sea (UNGA, 2017). This BBNJ process aims to set global protection goals for ABNJ and will include guidelines for the use of area-based management tools (including MPAs) and implementation of EIA;
(2) A further iteration of the CBD EBSA process (Johnson et al., 2018a), notably to foster the description of EBSAs in ABNJ. Whilst in the Northwest Atlantic, Mediterranean and Northeast Atlantic Regional EBSA Workshops the focus was predominantly on deeper waters including ABNJ, other Regional EBSA Workshops concentrated on shallower coastal locations within national jurisdiction. A thematic deep-sea review of EBSAs, taking into account SCP suggestions could further highlight gaps and opportunities.
(3) Informing competent regional and/or sectoral organizations with a mandate to protect biodiversity – notably NAFO, NEAFC, OSPAR, the Barcelona Convention and ISA13 – which should all aim to consolidate and expand their conservation networks to meet strategic obligations. For instance, deep-sea priority areas could inform the extension of the North-Atlantic fishing closure network by the relevant sectoral (RFMOs) and local (nations, EU) jurisdictional bodies. Similarly, our priority networks could contribute to the regulation of deep-sea mining regulation via the Atlantic Regional Environmental Management Plan (REMP). The ISA is required to implement the REMP and is mandated to designate Areas of Particular Environmental Interest (APEI) using SCP (Wedding et al., 2013; Lodge et al., 2014; Dunn et al., 2018) to protect specific features such as hydrothermal vents (Johnson, 2019).
(4) Informing national conservation planning exercises in many different countries by highlighting the potential contribution national conservation initiatives could make to the support of basin-scale priority conservation networks.
Finally, these results can help direct future research. The UN Decade of Ocean Science for Sustainable Development will undoubtedly have a deep-sea component. Building baseline data, ground-truthing identified priority networks, confirming their potential to promote resilience and provide refugia from climate change, should all be featured in future research programs informed by SCP. From a viability and resilience perspective, it is recommended that connectivity and climate change should be factored into the design of future conservation networks (Magris et al., 2014). By investigating in more detail the connectivity pathways of benthic species, broadly addressed here, and including areas identified as sources and sinks from population genetic studies, more robust conservation networks are possible. This study has shown that few areas that could be considered as potential climate refugia for deep-sea species currently benefit from some form of protection. Our results support the conclusions of Johnson et al. (2018b) that climate change pressures are likely to affect deep-sea oceanography and biodiversity, and thus the ability of the current protection network to preserve them.
Summary/Conclusions
This study adds to previous research that promotes the use of SCP based on multi-objective prioritization (Beger et al., 2015; Magris et al., 2017; Álvarez-Romero et al., 2018b; Manea et al., 2020). It factors in multi-scale representativity (species, ecosystems, and physical habitats), regional replication, climate change resilience and population connectivity while taking into account existing spatial management and socio-economic interests (fisheries and mining).
The key findings that emerged from both the process of structuring the prioritization framework and the resulting conservation solutions are as follows:
• An in-depth and exhaustive data mining exercise is a prerequisite for successful application of SCP. Diverse data types should be collated including habitat types, species occurrence records and predicted distributions based on the outputs of habitat suitability models models. The latter facilitates testing the impact of various climate change scenarios on the future distribution of species and habitats.
• It is important to consider climate change effects during MPA design. The inclusion of predicted species climate refugia as conservation features during the planning process provides a degree of future proofing, ensuring the long-term viability, and resilience of the conservation network.
• Promoting conservation network replication by dividing the planning region into distinct sub-regions promotes environmental and geographic representativeness and potentially increases intraspecific diversity, thus strengthening overall network resilience.
• Adjusting targets to prioritize conservation features according to their rarity, ecological importance or vulnerability, or degree of data confidence provides more flexibility and accuracy than applying blanket targets across all features.
• The inclusion of connectivity in SCP is challenging, especially for remote and poorly known areas or species. Including it during prioritization can help to identify areas that do not appear as important at first sight, but play an essential role in ensuring connectivity and network coherence.
• Weighting in favour of, rather than “locking-in,” current protected areas and EBSAs during prioritization builds on the existing networks while excluding any individual MPAs or EBSAs that do not contribute to the proposed SCP network.
• Taking into account economic activity in the SCP design is essential so that the selection of the conservation network minimizes the socio-economic costs of conservation, but also to assess whether prioritised conservation areas overlap with those activities which may prevent them from contributing to meeting the conservation targets. This was the case for most fish refugia that were located on existing fishing grounds, and for hydrothermal vents located within licenced deep-sea mining exploration areas.
• Identifying the priority conservation zones that most strongly contribute to the SCP objectives is a fundamental step in the development and implementation of a robust MSP approach.
• This study highlights the value of SCP in that the relatively small proportion of the planning region – here around 17% – proposed for the network ensured that on average 55% of each feature would be conserved, thus banking an extensive conservation capital.
• While VMEs are considered amongst the ecosystems most at risk from climate change and considered as a top-priority for conservation management, deep-sea biodiversity in the North Atlantic and Mediterranean is still inadequately protected.
In conclusion, this multi-objective prioritization allowed us to determine options for developing a coherent VME conservation network for the whole of the North Atlantic and the Mediterranean that could be updated as new data is gathered. The SCP approach adopted here could be employed by stakeholders and policymakers to provide the foundation for basin-scale MSP, for example, in ABNJ, where a framework to coordinate the actions of different regional authorities and sectoral bodies is still lacking (Ardron et al., 2014; Ban et al., 2014; Wright et al., 2019). A similar approach could be used to extend such planning into adjacent areas, such as the Arctic. This study demonstrates the value of adopting a basin-wide management strategy to protect the vulnerable habitats and species found in the deep sea in a more systematic way to improve mitigation of the threats posed by bottom fishing, mining and climate change.
Data Availability Statement
The datasets presented in this study can be found in online repositories. The names of the repository/repositories and accession number(s) can be found below: https://doi.org/10.17882/62541.
Author Contributions
SV and MC structured the scenarios building on discussions held with participants during a workshop “Conservation management issues in ATLAS,” held in Sète, France, in January 2019, with subsequent review by LM and SA-H. CD-C, AF, JG-I, and TM produced parts of the data. MC, LM, and TM compiled the data. MC conducted the analysis and wrote the first draft of the manuscript. All authors structured the objectives, framework of the study, and revised the manuscript.
Funding
This work has received funding from the European Union’s Horizon 2020 Research and Innovation Program under grant agreements Nos. 678760 (ATLAS), 679849 (SponGES), and 818123 (iAtlantic). This output reflects the authors’ views and the European Union is not responsible for any use that may be made of the information it contains.
Conflict of Interest
DJ was employed by the company Seascape Consultants Ltd.
The remaining authors declare that the research was conducted in the absence of any commercial or financial relationships that could be construed as a potential conflict of interest.
Acknowledgments
We would like to thank data contributors and all participants of the workshop “Conservation management issues in ATLAS” for their input: Luís Rodrigues, Javier Murillo-Perez, Lea-Anne Henry, Xuan Bui, Pascal Laffargue, David Stirling, and Mathieu Woillez. We thank Camille Lirette and Steve W. Ross for assistance in identifying Atlantic MPAs in Canada and the United States. MC and SV are grateful to Jeffrey Hanson for his guidance in the use of the Prioritizr software package. We thank MPA practitioner, Marty King, Fisheries and Oceans Canada, for his insightful comments on an earlier draft of this manuscript. We dedicate this paper to the memory of Hans Tore Rapp.
Supplementary Material
The Supplementary Material for this article can be found online at: https://www.frontiersin.org/articles/10.3389/fmars.2021.611358/full#supplementary-material
Footnotes
- ^ The ATLAS data calls were sent to partners to inventory the VMEs emerging from their institutional databases or through ATLAS research activities. This database included bona fide VMEs and VME indicator taxa records for both the Atlantic and Mediterranean. Several of these VMEs have been discovered recently thus are not yet available on public data portals (Combes et al., 2019).
- ^ www.iobis.org
- ^ https://deepseacoraldata.noaa.gov/
- ^ http://www.ices.dk/marine-data/data-portals/Pages/vulnerable-marineecosystems
- ^ http://vents-data.interridge.org
- ^ Recently synonymised to Desmophyllum pertusum (Addamo et al., 2016).
- ^ www.gebco.net
- ^ www.protectedplanet.net
- ^ http://maps.imr.no/geoserver/web/. Map “coralmpa”: cold-water corals MPAs in the EEZ of Norway.
- ^ https://open.canada.ca/data/en/dataset/a1e18963-25dd-4219-a33f-1a38c4971250; https://open.canada.ca/data/en/dataset/44769543-7a23-4991-a53f-c2cf7c7a946f
- ^ https://marineprotectedareas.noaa.gov/dataanalysis/mpainventory/; https://safmc.net/ecosystem-management/mapping-and-gis-data/. The national NOAA dataset of protected areas was complemented by the deepwater coral Habitat Areas of Particular Concern (HAPC) and grouper Special Management Zones managed on the Atlantic coast by the SAFMC.
- ^ www.cbd.int/ebsa/ebsas. Considering the North-East Atlantic, a first workshop to describe EBSAs was held by the OSPAR Commission and the North East Atlantic Fisheries Commission in 2011. In 2019, a new workshop, convened by the CBD, and with input from ATLAS Partners, has taken place (CBD/SBSTTA/23/7 and 23/7/ADD1) and described 17 potential EBSAs, but have yet to be formally endorsed by CBD COP and have therefore not been included in this analysis.
- ^ Beyond these mentioned sectoral and regional organizations, it should be noted that there are regional governance gaps in the North Atlantic. For instance the northwest Atlantic has no Regional Seas Convention and WECAFC (Western Central Fishery Commission) can provide fisheries advice but has no mandate to designate VMEs.
References
Abdulla, A., Gomei, M., Hyrenbach, D., Notarbartolo-di-Sciara, G., and Agardy, T. (2009). Challenges facing a network of representative marine protected areas in the Mediterranean: prioritizing the protection of underrepresented habitats. ICES J. Mar. Sci. 66, 22–28. doi: 10.1093/icesjms/fsn164
Addamo, A. M., Vertino, A., Stolarski, J., García-Jiménez, R., Taviani, M., and Machordom, A. (2016). Merging scleractinian genera: the overwhelming genetic similarity between solitary Desmophyllum and colonial Lophelia. BMC Evol. Biol. 16:108. doi: 10.1186/s12862-016-0654-8
Aguilar, R., Perry, A. L., and López, J. (2017). “Conservation and management of vulnerable marine benthic ecosystems,” in Marine Animal Forests: The Ecology of Benthic Biodiversity Hotspots, eds S. Rossi, L. Bramanti, A. Gori, and C. Orejas (Cham: Springer International Publishing), 1165–1207.
Althaus, F., Williams, A., Schlacher, T. A., Kloser, R. J., Green, M. A., Barker, B. A., et al. (2009). Impacts of bottom trawling on deep-coral ecosystems of seamounts are long-lasting. Mar. Ecol. Prog. Ser. 397, 279–294. doi: 10.3354/meps08248
Álvarez-Romero, J. G., Mills, M., Adams, V. M., Gurney, G. G., Pressey, R. L., Weeks, R., et al. (2018a). Research advances and gaps in marine planning: towards a global database in systematic conservation planning. Biol. Conserv. 227, 369–382. doi: 10.1016/j.biocon.2018.06.027
Álvarez-Romero, J. G., Munguía-Vega, A., Beger, M., del Mancha-Cisneros, M., Suárez-Castillo, A. N., Gurney, G. G., et al. (2018b). Designing connected marine reserves in the face of global warming. Glob. Change Biol. 24, e671–e691. doi: 10.1111/gcb.13989
Amengual, J., and Alvarez-Berastegui, D. (2018). A critical evaluation of the aichi biodiversity target 11 and the mediterranean MPA network, two years ahead of its deadline. Biol. Conserv. 225, 187–196. doi: 10.1016/j.biocon.2018.06.032
Ardron, J. A., Rayfuse, R., Gjerde, K., and Warner, R. (2014). The sustainable use and conservation of biodiversity in ABNJ: What can be achieved using existing international agreements? Mar. Policy 49, 98–108. doi: 10.1016/j.marpol.2014.02.011
Armstrong, C. W., Vondolia, G. K., Foley, N. S., Henry, L.-A., Needham, K., and Ressurreição, A. (2019). Expert assessment of risks posed by climate change and anthropogenic activities to ecosystem services in the deep North Atlantic. Front. Mar. Sci. 6:158. doi: 10.3389/fmars.2019.00158
Bailey, D. M., Collins, M. A., Gordon, J. D. M., Zuur, A. F., and Priede, I. G. (2009). Long-term changes in deep-water fish populations in the northeast Atlantic: a deeper reaching effect of fisheries? Proc. R. Soc. B Biol. Sci. 276, 1965–1969. doi: 10.1098/rspb.2009.0098
Baillon, S., Hamel, J.-F., Wareham, V. E., and Mercier, A. (2012). Deep cold-water corals as nurseries for fish larvae. Front. Ecol. Environ. 10:351–356. doi: 10.1890/120022
Baker, K. D., Wareham, V. E., Snelgrove, P. V. R., Haedrich, R. L., Fifield, D. A., Edinger, E. N., et al. (2012). Distributional patterns of deep-sea coral assemblages in three submarine canyons off Newfoundland. Canada. Mar. Ecol. Prog. Ser. 445, 235–249. doi: 10.3354/meps09448
Ball, I. R., Possingham, H. P., and Watts, M. (2009). “Marxan and relatives: software for spatial conservation prioritisation,” in Spatial Conservation Prioritisation: Quantitative Methods and Computational Tools, eds A. Moilanen, K. A. Wilson, and H. P. Possingham (Oxford: Oxford University Press), 185–195.
Ban, N. C., and Klein, C. J. (2009). Spatial socioeconomic data as a cost in systematic marine conservation planning. Conserv. Lett. 2, 206–215. doi: 10.1111/j.1755-263x.2009.00071.x
Ban, N. C., Bax, N. J., Gjerde, K. M., Devillers, R., Dunn, D. C., Dunstan, P. K., et al. (2014). Systematic conservation planning: a better recipe for managing the high seas for biodiversity conservation and sustainable use. Conserv. Lett. 7, 41–54. doi: 10.1111/conl.12010
Barcelona Convention (1995). Protocol Concerning Specially Protected Areas and Biological Diversity in the Mediterranean. Barcelona: Barcelona Convention.
Basterretxea, G., Jordi, A., Catalán, I. A., and Sabatés, A. (2012). Model-based assessment of local-scale fish larval connectivity in a network of marine protected areas: Local-scale connectivity in a network of MPAs. Fish. Oceanogr. 21, 291–306. doi: 10.1111/j.1365-2419.2012.00625.x
Beazley, L. I., Kenchington, E. L., Murillo, F. J., Sacau, M., and del, M. (2013). Deep-sea sponge grounds enhance diversity and abundance of epibenthic megafauna in the Northwest Atlantic. ICES J. Mar. Sci. 70, 1471–1490. doi: 10.1093/icesjms/fst124
Beger, M., McGowan, J., Treml, E. A., Green, A. L., White, A. T., Wolff, N. H., et al. (2015). Integrating regional conservation priorities for multiple objectives into national policy. Nat. Commun. 6:8208. doi: 10.1038/ncomms9208
Boavida, J., Becheler, R., Addamo, A. M., Sylvestre, F., and Arnaud-Haond, S. (2019). “31 Past, present and future connectivity of mediterranean cold-water corals: patterns, drivers and fate in a technically and environmentally changing world,” in Mediterranean Cold-Water Corals: Past, Present and Future Coral Reefs of the World, eds C. Orejas and C. Jiménez (Cham: Springer International Publishing), 357–372. doi: 10.1007/978-3-319-91608-8_31
Böning, C. W., Behrens, E., Biastoch, A., Getzlaff, K., and Bamber, J. L. (2016). Emerging impact of Greenland meltwater on deepwater formation in the North Atlantic Ocean. Nat. Geosci. 9, 523–527. doi: 10.1038/ngeo2740
Boschen, R. E., Rowden, A. A., Clark, M. R., Pallentin, A., and Gardner, J. P. A. (2016). Seafloor massive sulfide deposits support unique megafaunal assemblages: Implications for seabed mining and conservation. Mar. Environ. Res. 115, 78–88. doi: 10.1016/j.marenvres.2016.02.005
Brooke, S., and Young, C. M. (2005). Embryogenesis and larval biology of the ahermatypic scleractinian Oculina varicosa. Mar. Biol. 146, 665–675. doi: 10.1007/s00227-004-1481-9
Buckley, L. B., Waaser, S. A., MacLean, H. J., and Fox, R. (2011). Does including physiology improve species distribution model predictions of responses to recent climate change? Ecology 92, 2214–2221. doi: 10.1890/11-0066.1
Buhl-Mortensen, L., Olafsdottir, S. H., Buhl-Mortensen, P., Burgos, J. M., and Ragnarsson, S. A. (2015). Distribution of nine cold-water coral species (Scleractinia and Gorgonacea) in the cold temperate North Atlantic: effects of bathymetry and hydrography. Hydrobiologia 759, 39–61. doi: 10.1007/s10750-014-2116-x
Buhl-Mortensen, L., Vanreusel, A., Gooday, A. J., Levin, L. A., Priede, I. G., Buhl-Mortensen, P., et al. (2010). Biological structures as a source of habitat heterogeneity and biodiversity on the deep ocean margins. Mar. Ecol. 31, 21–50. doi: 10.1111/j.1439-0485.2010.00359.x
Buhl-Mortensen, P., Buhl-Mortensen, L., and Purser, A. (2017). “Trophic ecology and habitat provision in cold-water coral ecosystems,” in Marine Animal Forests: The Ecology of Benthic Biodiversity Hotspots, eds S. Rossi, L. Bramanti, A. Gori, and C. Orejas (Cham: Springer International Publishing), 919–944.
Cárdenas, P., Rapp, H. T., Klitgaard, A. B., Best, M., Thollesson, M., and Tendal, O. S. (2013). Taxonomy, biogeography and DNA barcodes of Geodia species (Porifera, Demospongiae, Tetractinellida) in the Atlantic boreo-arctic region. Zool. J. Linn. Soc. 169, 251–311. doi: 10.1111/zoj.12056
Cathalot, C., Van Oevelen, D., Cox, T. J. S., Kutti, T., Lavaleye, M., Duineveld, G., et al. (2015). Cold-water coral reefs and adjacent sponge grounds: hotspots of benthic respiration and organic carbon cycling in the deep sea. Front. Mar. Sci. 2:37. doi: 10.3389/fmars.2015.00037
CBD (2008). IX/20. Marine and coastal biodiversity, in Decision Adopted by the Conference of the Parties to the Convention on Biological Diversity at its Ninth Meeting. Available online at: www.cbd.int/decision/cop/default.shtml?id=11663 (accessed April 6, 2021).
CBD (2020). Zero Draft of the Post-2020 Global Biodiversity Framework. Available online at: https://www.cbd.int/doc/c/efb0/1f84/a892b98d2982a829962b6371/wg2020-02-03-en.pdf (accessed April 6, 2021)
Chimienti, G., Bo, M., Taviani, M., and Mastrototaro, F. (2019). “19 occurrence and biogeography of mediterranean cold-water corals,” in Mediterranean Cold-Water Corals: Past, Present and Future Coral Reefs of the World, eds C. Orejas and C. Jiménez (Cham: Springer International Publishing), 213–243. doi: 10.1007/978-3-319-91608-8_19
Clark, M. R., Bowden, D. A., Rowden, A. A., and Stewart, R. (2019). Little evidence of benthic community resilience to bottom trawling on seamounts after 15 years. Front. Mar. Sci. 6:63. doi: 10.3389/fmars.2019.00063
Clavel-Henry, M., Solé, J., Ahumada-Sempoal, M. -Á, Bahamon, N., Briton, F., Rotllant, G., et al. (2019). Influence of the summer deep-sea circulations on passive drifts among the submarine canyons in the northwestern Mediterranean Sea. Ocean Sci. 15, 1745–1759. doi: 10.5194/os-15-1745-2019
Combes, M., and Vaz, S. (2019). Systematic Conservation Planning for the North-Atlantic Deep Sea. Brest: IFREMER, doi: 10.17882/62541
Combes, M., Vaz, S., Morato, T., Fauconnet, L., Arnaud-Haond, S., Dominguez-Carrió, C., et al. (2019). ATLAS D3.4 Conservation Management Issues in ATLAS. Basin-Scale Systematic Conservation planning: Identifying Suitable Networks for VMEs Protection. doi: 10.5281/zenodo.4658788
Cowen, R. K. (2006). Scaling of connectivity in marine populations. Science 311, 522–527. doi: 10.1126/science.1122039
Cowen, R. K., and Sponaugle, S. (2009). Larval dispersal and marine population connectivity. Annu. Rev. Mar. Sci. 1, 443–466. doi: 10.1146/annurev.marine.010908.163757
D’Onghia, G. (2019). “30 Cold-Water corals as shelter, feeding and life-history critical habitats for fish species: ecological interactions and fishing impact,” in Mediterranean Cold-Water Corals: Past, Present and Future, eds C. Orejas and C. Jiménez (Cham: Springer), 335–356.
Daigle, R. M., Metaxas, A., Balbar, A., McGowan, J., Treml, E. A., Kuempel, C. D., et al. (2018). Operationalizing ecological connectivity in spatial conservation planning with Marxan Connect. bioRxiv [preprint] doi: 10.1101/315424
Danovaro, R., Corinaldesi, C., D’Onghia, G., Galil, B., Gambi, C., Gooday, A. J., et al. (2010). Deep-sea biodiversity in the Mediterranean Sea: the known, the unknown, and the unknowable. PLoS One 5:e11832. doi: 10.1371/journal.pone.0011832
Davies, A. J., and Guinotte, J. M. (2011). Global habitat suitability for framework-forming cold-water corals. PLoS One 6:e18483. doi: 10.1371/journal.pone.0018483
Davies, A. J., Roberts, J. M., and Hall-Spencer, J. (2007). Preserving deep-sea natural heritage: Emerging issues in offshore conservation and management. Biol. Conserv. 138, 299–312. doi: 10.1016/j.biocon.2007.05.011
De Leo, F. C., Smith, C. R., Rowden, A. A., Bowden, D. A., and Clark, M. R. (2010). Submarine canyons: hotspots of benthic biomass and productivity in the deep sea. Proc. R. Soc. B Biol. Sci. 277, 2783–2792. doi: 10.1098/rspb.2010.0462
del Otero, M., and Marin, P. (2019). “Chap. 46 conservation of cold-water corals in the mediterranean: current status and future prospects for improvement,” in Mediterranean Cold-Water Corals: Past, Present and Future, eds C. Orejas and C. Jiménez (Cham: Springer International Publishing), 535–545. doi: 10.1007/978-3-319-91608-8_46
Dinerstein, E., Olson, D., Joshi, A., Vynne, C., Burgess, N. D., Wikramanayake, E., et al. (2017). An ecoregion-based approach to protecting half the terrestrial realm. BioScience 67, 534–545. doi: 10.1093/biosci/bix014
Diz, D., Johnson, D., Riddell, M., Rees, S., Battle, J., Gjerde, K., et al. (2018). Mainstreaming marine biodiversity into the SDGs: The role of other effective area-based conservation measures (SDG 14.5). Mar. Policy 93, 251–261. doi: 10.1016/j.marpol.2017.08.019
Dubois, M., Rossi, V., Ser-Giacomi, E., Arnaud-Haond, S., López, C., and Hernández-García, E. (2016). Linking basin-scale connectivity, oceanography and population dynamics for the conservation and management of marine ecosystems. Glob. Ecol. Biogeogr. 25, 503–515. doi: 10.1111/geb.12431
Dunn, D. C., Dover, C. L. V., Etter, R. J., Smith, C. R., Levin, L. A., Morato, T., et al. (2018). A strategy for the conservation of biodiversity on mid-ocean ridges from deep-sea mining. Sci. Adv. 4:eaar4313. doi: 10.1126/sciadv.aar4313
Eigaard, O. R., Bastardie, F., Hintzen, N. T., Buhl-Mortensen, L., Buhl-Mortensen, P., Catarino, R., et al. (2017). The footprint of bottom trawling in European waters: distribution, intensity, and seabed integrity. ICES J. Mar. Sci. 74, 847–865. doi: 10.1093/icesjms/fsw194
Evans, J. L., Peckett, F., and Howell, K. L. (2015). Combined application of biophysical habitat mapping and systematic conservation planning to assess efficiency and representativeness of the existing High Seas MPA network in the Northeast Atlantic. ICES J. Mar. Sci. 72, 1483–1497. doi: 10.1093/icesjms/fsv012
FAO. (2009). Report of the Technical Consultation on International Guidelines for the Management of Deep-sea Fisheries in the High Seas: Food and Agriculture Organization of the United Nations Fisheries and Aquaculture Report 881. Rome: FAO, 86.
Feng, X., Liang, Y., Gallardo, B., and Papeş, M. (2019). Physiology in ecological niche modeling: using zebra mussel’s upper thermal tolerance to refine model predictions through Bayesian analysis. Ecography 43, 270–282. doi: 10.1111/ecog.04627
Fernandes, L., Day, J., Lewis, A., Slegers, S., Kerrigan, B., Breen, D., et al. (2005). Establishing representative no-take areas in the great barrier reef: large-scale implementation of theory on marine protected areas. Conserv. Biol. 19, 1733–1744. doi: 10.1111/j.1523-1739.2005.00302.x
Fernandez-Arcaya, U., Ramirez-Llodra, E., Aguzzi, J., Allcock, A. L., Davies, J. S., Dissanayake, A., et al. (2017). Ecological role of submarine canyons and need for canyon conservation: a review. Front. Mar. Sci. 4:5. doi: 10.3389/fmars.2017.00005
Fisher, C. R., Takai, K., and Le Bris, N. (2007). Hydrothermal vent ecosystems. Oceanography 20, 14–23. doi: 10.5670/oceanog.2007.75
Fox, A. D., Corne, D. W., Mayorga Adame, C. G., Polton, J. A., Henry, L.-A., and Roberts, J. M. (2019). An efficient multi-objective optimization method for use in the design of marine protected area networks. Front. Mar. Sci. 6:17. doi: 10.3389/fmars.2019.00017
Fox, A. D., Henry, L.-A., Corne, D. W., and Roberts, J. M. (2016). Sensitivity of marine protected area network connectivity to atmospheric variability. R. Soc. Open Sci. 3:160494. doi: 10.1098/rsos.160494
Frederiksen, R., Jensen, A., and Westerberg, H. (1992). The distribution of the scleractinian coral Lophelia pertusa around the Faroe islands and the relation to internal tidal mixing. Sarsia 77, 157–171. doi: 10.1080/00364827.1992.10413502
Freiwald, A., Helge Fosså, J., Grehan, A., Koslow, T., and Roberts, J. M. (2004). Cold-Water Coral Reefs: Out of Sight - No Longer out of Mind. Cambridge: UNEP-WCMC.
Gage, J. D., and Tyler, P. A. (1991). Deep-Sea Biology: A Natural History of Organisms at the Deep-Sea Floor. Cambridge: Cambridge University Press.
Gaines, S. D., White, C., Carr, M. H., and Palumbi, S. R. (2010). Designing marine reserve networks for both conservation and fisheries management. Proc. Natl. Acad. Sci. U.S.A. 107, 18286–18293. doi: 10.1073/pnas.0906473107
Gary, S. F., Fox, A. D., Biastoch, A., Roberts, J. M., and Cunningham, S. A. (2020). Larval behaviour, dispersal and population connectivity in the deep sea. Sci. Rep. 10:10675. doi: 10.1038/s41598-020-67503-7
Genin, A. (2004). Bio-physical coupling in the formation of zooplankton and fish aggregations over abrupt topographies. J. Mar. Syst. 50, 3–20. doi: 10.1016/j.jmarsys.2003.10.008
GFCM (2018). Report of the Second Meeting of the Working Group on Vulnerable Marine Ecosystems (WGVME). Available online at: http://www.fao.org/gfcm/technical-meetings/detail/ar/c/1142043/ (accessed April 6, 2021).
Giakoumi, S., Sini, M., Gerovasileiou, V., Mazor, T., Beher, J., Possingham, H. P., et al. (2013). Ecoregion-based conservation planning in the mediterranean: dealing with large-scale heterogeneity. PLoS One 8:e76449. doi: 10.1371/journal.pone.0076449
Gili, J.-M., Bouillon, J., Pagès, F., Palanques, A., and Puig, P. (1999). Submarine canyons as habitats of prolific plankton populations: three new deep-sea Hydroidomedusae in the western Mediterranean. Zool. J. Linn. Soc. 125, 313–329. doi: 10.1111/j.1096-3642.1999.tb00595.x
Gollner, S., Kaiser, S., Menzel, L., Jones, D. O. B., Brown, A., Mestre, N. C., et al. (2017). Resilience of benthic deep-sea fauna to mining activities. Mar. Environ. Res. 129, 76–101. doi: 10.1016/j.marenvres.2017.04.010
Gurobi Optimization LLC (2018). Gurobi Optimizer Reference Manual. Available online at: http://www.gurobi.com (accessed June 6, 2019).
Halpern, B. S., Frazier, M., Afflerbach, J., Lowndes, J. S., Micheli, F., O’Hara, C., et al. (2019). Recent pace of change in human impact on the world’s ocean. Sci. Rep. 9:11609. doi: 10.1038/s41598-019-47201-9
Hanson, J. O., Fuller, R. A., and Rhodes, J. R. (2019a). Conventional methods for enhancing connectivity in conservation planning do not always maintain gene flow. J. Appl. Ecol. 56, 913–922. doi: 10.1111/1365-2664.13315
Hanson, J. O., Schuster, R., Morrell, N., Strimas-Mackey, M., Watts, M. E., Arcese, P., et al. (2019b). Prioritizr: Systematic Conservation Prioritization in R. Available online at: https://CRAN.R-project.org/package=prioritizr (accessed June 6, 2019).
Harris, P. T., and Whiteway, T. (2011). Global distribution of large submarine canyons: Geomorphic differences between active and passive continental margins. Mar. Geol. 285, 69–86. doi: 10.1016/j.margeo.2011.05.008
Henry, L.-A., Navas, J. M., Hennige, S. J., Wicks, L. C., Vad, J., and Murray Roberts, J. (2013). Cold-water coral reef habitats benefit recreationally valuable sharks. Biol. Conserv. 161, 67–70. doi: 10.1016/j.biocon.2013.03.002
Hilário, A., Metaxas, A., Gaudron, S. M., Howell, K. L., Mercier, A., Mestre, N. C., et al. (2015). Estimating dispersal distance in the deep sea: challenges and applications to marine reserves. Front. Mar. Sci. 2:6. doi: 10.3389/fmars.2015.00006
Howell, K.-L., Piechaud, N., Downie, A.-L., and Kenny, A. (2016). The distribution of deep-sea sponge aggregations in the North Atlantic and implications for their effective spatial management. Deep Sea Res. Part Oceanogr. Res. Pap. 115, 309–320. doi: 10.1016/j.dsr.2016.07.005
Huvenne, V. A. I., Bett, B. J., Masson, D. G., Le Bas, T. P., and Wheeler, A. J. (2016). Effectiveness of a deep-sea cold-water coral Marine Protected Area, following eight years of fisheries closure. Biol. Conserv. 200, 60–69. doi: 10.1016/j.biocon.2016.05.030
ICES (2016). Report of the Joint ICES/NAFO Working Group on Deep-water Ecology (WGDEC), ICES CM 2016/ACOM:28. Copenhagen: ICES, 82.
ICES (2019). Report of the ICES/NAFO Joint Working Group on Deep-water Ecology (WGDEC). ICES Sci. Rep. 119, Copenhagen: ICES. doi: 10.17895/ices.pub.5567
International Seabed Authority (2018). Preliminary Strategy for the Development of Regional Environmental Management Plans for the Area. Available online at: https://isa.org.jm/files/files/documents/isba24-c3-e.pdf (accessed June 16, 2020).
IUCN (2019). Thematic Report – Conservation Overview of Mediterranean Deep-Sea Biodiversity: A Strategic Assessment. Spain: IUCN Gland, Switzerland and Malaga, 122.
Jackson, E. L., Davies, A. J., Howell, K. L., Kershaw, P. J., and Hall-Spencer, J. M. (2014). Future-proofing marine protected area networks for cold water coral reefs. ICES J. Mar. Sci. 71, 2621–2629. doi: 10.1093/icesjms/fsu099
Jenks, G. F. (1967). The data model concept in statistical mapping. Int. Yearb. Cartogr. 7, 186–190.
Johnson, D. E. (2019). Protecting the lost city hydrothermal vent system: All is not lost, or is it? Mar. Policy 107:103593. doi: 10.1016/j.marpol.2019.103593
Johnson, D. E., and Kenchington, E. L. (2019). Should potential for climate change refugia be mainstreamed into the criteria for describing EBSAs? Conserv. Lett. 12:e12634. doi: 10.1111/conl.12634
Johnson, D. E., Ardron, J., Billett, D., Hooper, T., Mullier, T., Chaniotis, P., et al. (2014). When is a marine protected area network ecologically coherent? A case study from the North-east Atlantic. Aquat. Conserv. Mar. Freshw. Ecosyst. 24, 44–58. doi: 10.1002/aqc.2510
Johnson, D. E., Barrio Froján, C., Turner, P. J., Weaver, P., Gunn, V., Dunn, D. C., et al. (2018a). Reviewing the EBSA process: Improving on success. Mar. Policy 88, 75–85. doi: 10.1016/j.marpol.2017.11.014
Johnson, D. E., Ferreira, M. A., and Kenchington, E. (2018b). Climate change is likely to severely limit the effectiveness of deep-sea ABMTs in the North Atlantic. Mar. Policy 87, 111–122. doi: 10.1016/j.marpol.2017.09.034
Jonsson, P. R., Jacobi, M. N., and Moksnes, P.-O. (2016). How to select networks of marine protected areas for multiple species with different dispersal strategies. Divers. Distrib. 22, 161–173. doi: 10.1111/ddi.12394
Kenchington, E. L., Callery, O., Davidson, F., Grehan, A., Morato, T., Appiott, J., et al. (2019a). Use of Species Distribution Modeling in the Deep Sea. Canadian Technical Report of Fisheries and Aquatic Sciences 3296. Available online at: http://rgdoi.net/10.13140/RG.2.2.12433.28003 (accessed April 6, 2021).
Kenchington, E. L., Wang, Z., Lirette, C., Murillo, F. J., Guijarro, J., Yashayaev, I., et al. (2019b). Connectivity modelling of areas closed to protect vulnerable marine ecosystems in the northwest Atlantic. Deep Sea Res. Part Oceanogr. Res. Pap. 143, 85–103. doi: 10.1016/j.dsr.2018.11.007
Kincaid, K., and Rose, G. (2017). Effects of closing bottom trawling on fisheries, biodiversity, and fishing communities in a boreal marine ecosystem: the Hawke Box off Labrador, Canada. Can. J. Fish. Aquat. Sci. 74, 1490–1502. doi: 10.1139/cjfas-2016-0343
Kininmonth, S., Beger, M., Bode, M., Peterson, E., Adams, V. M., Dorfman, D., et al. (2011). Dispersal connectivity and reserve selection for marine conservation. Ecol. Model. 222, 1272–1282. doi: 10.1016/j.ecolmodel.2011.01.012
Klein, C. J., Chan, A., Kircher, L., Cundiff, A. J., Gardner, N., Hrovat, Y., et al. (2008). Striking a balance between biodiversity conservation and socioeconomic viability in the design of marine protected areas. Conserv. Biol. 22, 691–700. doi: 10.1111/j.1523-1739.2008.00896.x
Knudby, A., Kenchington, E., and Murillo, F. J. (2013). Modeling the distribution of geodia sponges and sponge grounds in the northwest Atlantic. PLoS One 8:e82306. doi: 10.1371/journal.pone.0082306
Koslow, J. A., Boehlert, G. W., Gordon, J. D. M., Haedrich, R. L., Lorance, P., and Parin, N. (2000). Continental slope and deep-sea fisheries: implications for a fragile ecosystem. ICES J. Mar. Sci. 57, 548–557. doi: 10.1006/jmsc.2000.0722
Kritzer, J. P., and Sale, P. F. (2004). Metapopulation ecology in the sea: from Levins’ model to marine ecology and fisheries science. Fish Fish. 5, 131–140. doi: 10.1111/j.1467-2979.2004.00131.x
Krueck, N. C., Ahmadia, G. N., Green, A., Jones, G. P., Possingham, H. P., Riginos, C., et al. (2017). Incorporating larval dispersal into MPA design for both conservation and fisheries. Ecol. Appl. 27, 925–941. doi: 10.1002/eap.1495
LaBelle, R. (2001). Overview of US minerals management service activities in deepwater research. Mar. Pollut. Bull. 43, 256–261. doi: 10.1016/s0025-326x(01)00056-x
Laikre, L., Allendorf, F. W., Aroner, L. C., Baker, C. S., Gregovich, D. P., Hansen, M. M., et al. (2010). Neglect of genetic diversity in implementation of the convention on biological diversity. Conserv. Biol. 24, 86–88. doi: 10.1111/j.1523-1739.2009.01425.x
Larsson, A. I., Järnegren, J., Strömberg, S. M., Dahl, M. P., Lundälv, T., and Brooke, S. (2014). Embryogenesis and larval biology of the cold-water coral Lophelia pertusa. PLoS One 9:e102222. doi: 10.1371/journal.pone.0102222
Lester, S. E., Costello, C., Halpern, B. S., Gaines, S. D., White, C., and Barth, J. A. (2013). Evaluating tradeoffs among ecosystem services to inform marine spatial planning. Mar. Policy 38, 80–89. doi: 10.1016/j.marpol.2012.05.022
Levin, L. A., Wei, C., Dunn, D. C., Amon, D. J., Ashford, O. S., Cheung, W. W. L., et al. (2020). Climate change considerations are fundamental to management of deep-sea resource extraction. Glob. Change Biol. 26, 4664–4678. doi: 10.1111/gcb.15223
Levy, J. S., and Ban, N. C. (2013). A method for incorporating climate change modelling into marine conservation planning: An Indo-west Pacific example. Mar. Policy 38, 16–24. doi: 10.1016/j.marpol.2012.05.015
Lo Iacono, C., Robert, K., Gonzalez-Villanueva, R., Gori, A., Gili, J.-M., and Orejas, C. (2018). Predicting cold-water coral distribution in the Cap de Creus Canyon (NW Mediterranean): Implications for marine conservation planning. Prog. Oceanogr. 169, 169–180. doi: 10.1016/j.pocean.2018.02.012
Lodge, M., Johnson, D., Le Gurun, G., Wengler, M., Weaver, P., and Gunn, V. (2014). Seabed mining: international seabed authority environmental management plan for the Clarion–Clipperton Zone. A partnership approach. Mar. Policy 49, 66–72. doi: 10.1016/j.marpol.2014.04.006
Magris, R. A., Andrello, M., Pressey, R. L., Mouillot, D., Dalongeville, A., Jacobi, M. N., et al. (2018). Biologically representative and well-connected marine reserves enhance biodiversity persistence in conservation planning. Conserv. Lett. 11:e12439. doi: 10.1111/conl.12439
Magris, R. A., Pressey, R. L., Mills, M., Vila-Nova, D. A., and Floeter, S. (2017). Integrated conservation planning for coral reefs: Designing conservation zones for multiple conservation objectives in spatial prioritisation. Glob. Ecol. Conserv. 11, 53–68. doi: 10.1016/j.gecco.2017.05.002
Magris, R. A., Pressey, R. L., Weeks, R., and Ban, N. C. (2014). Integrating connectivity and climate change into marine conservation planning. Biol. Conserv. 170, 207–221. doi: 10.1016/j.biocon.2013.12.032
Maldonado, M. (2006). The ecology of the sponge larva. Can. J. Zool. 84, 175–194. doi: 10.1139/z05-177
Manea, E., Bianchelli, S., Fanelli, E., Danovaro, R., and Gissi, E. (2020). Towards an ecosystem-based marine spatial planning in the deep mediterranean sea. Sci. Total Environ. 715:136884. doi: 10.1016/j.scitotenv.2020.136884
Margules, C. R., and Pressey, R. L. (2000). Systematic conservation planning. Nature 405:243. doi: 10.1038/35012251
Maynou, F., and Cartes, J. E. (2012). Effects of trawling on fish and invertebrates from deep-sea coral facies of Isidella elongata in the western Mediterranean. J. Mar. Biol. Assoc. United Kingdom 92, 1501–1507. doi: 10.1017/S0025315411001603
Mazor, T., Giakoumi, S., Kark, S., and Possingham, H. P. (2014). Large-scale conservation planning in a multinational marine environment: cost matters. Ecol. Appl. 24, 1115–1130. doi: 10.1890/13-1249.1
MedPAN & SPA/RAC (2019). in The 2016 Status of Marine Protected Areas in the Mediterranean, eds B. Meola and C. Webster Tunis (Tunis: SPA/RAC & MedPAN). Available online at: https://www.um.edu.mt/library/oar/handle/123456789/69286 (accessed April 6, 2021).
Mengerink, K. J., Dover, C. L. V., Ardron, J., Baker, M., Escobar-Briones, E., Gjerde, K., et al. (2014). A call for deep-ocean stewardship. Science 344, 696–698. doi: 10.1126/science.1251458
Mienis, F., de Stigter, H. C., White, M., Duineveld, G., de Haas, H., and van Weering, T. C. E. (2007). Hydrodynamic controls on cold-water coral growth and carbonate-mound development at the SW and SE Rockall Trough Margin, NE Atlantic Ocean. Deep Sea Res. Part Oceanogr. Res. Pap. 54, 1655–1674. doi: 10.1016/j.dsr.2007.05.013
Moffitt, E. A., Wilson White, J., and Botsford, L. W. (2011). The utility and limitations of size and spacing guidelines for designing marine protected area (MPA) networks. Biol. Conserv. 144, 306–318. doi: 10.1016/j.biocon.2010.09.008
Moore, C. H., Radford, B. T., Possingham, H. P., Heyward, A. J., Stewart, R. R., Watts, M. E., et al. (2016). Improving spatial prioritisation for remote marine regions: optimising biodiversity conservation and sustainable development trade-offs. Sci. Rep. 6:32029. doi: 10.1038/srep32029
Morato, T., González-Irusta, J.-M., Dominguez-Carrió, C., Wei, C.-L., Davies, A., Sweetman, A. K., et al. (2020). Climate-induced changes in the suitable habitat of cold-water corals and commercially important deep-sea fishes in the North Atlantic. Glob. Change Biol. 26, 2181–2202. doi: 10.1111/gcb.14996
Morato, T., Kvile, K. Ø, Taranto, G. H., Tempera, F., Narayanaswamy, B. E., Hebbeln, D., et al. (2013). Seamount physiography and biology in the north-east Atlantic and Mediterranean Sea. Biogeosciences 10, 3039–3054. doi: 10.5194/bg-10-3039-2013
Morato, T., Pham, C. K., Fauconnet, L., Taranto, G. H., Chimienti, G., Cordes, E., et al. (2021). North Atlantic Basin-Scale Multi-Criteria assessment database to inform effective management and protection of vulnerable marine ecosystems. Front. Mar. Sci. 8:637078. doi: 10.3389/fmars.2021.637078
Morato, T., Pham, C. K., Pinto, C., Golding, N., Ardron, J. A., Durán Muñoz, P., et al. (2018). A Multi criteria assessment method for identifying vulnerable marine ecosystems in the North-East Atlantic. Front. Mar. Sci. 5:460. doi: 10.3389/fmars.2018.00460
Mosquera Giménez, Á, Vélez-Belchí, P., Rivera, J., Piñeiro, S., Fajar, N., Caínzos, V., et al. (2019). Ocean circulation over north atlantic underwater features in the path of the mediterranean outflow water: the ormonde and formigas seamounts, and the Gazul Mud Volcano. Front. Mar. Sci. 6:702. doi: 10.3389/fmars.2019.00702
Murawski, S. A., Brown, R., Lai, H.-L., Rago, P. J., and Hendrickson, L. (2000). Large-scale closed areas as a fishery-management tool in temperate marine systems: The Georges Bank experience. Bull. Mar. Sci. 66:25.
Niner, H. J., Ardron, J. A., Escobar, E. G., Gianni, M., Jaeckel, A., Jones, D. O. B., et al. (2018). Deep-Sea mining with no net loss of biodiversity—an impossible aim. Front. Mar. Sci. 5:53. doi: 10.3389/fmars.2018.00053
O’Leary, B. C., Allen, H. R., Yates, K. L., Page, R. W., Tudhope, A. W., McClean, C., et al. (2019). 30x30: A Blueprint for Ocean Protection. Umweltstiftung: Umweltstiftung Greenpeace.
Orejas, C., Kenchington, E., Rice, J., Kazanidis, G., Palialexis, A., Johnson, D., et al. (2020). Towards a common approach to the assessment of the environmental status of deep-sea ecosystems in areas beyond national jurisdiction. Mar. Policy 121:104182. doi: 10.1016/j.marpol.2020.104182
Palomino, D., Rueda, J. L., Vázquez, J. T., Urra, J., Sánchez-Guillamón, O., González-García, E., et al. (2019). “24 cold-water corals in fluid venting submarine structures,” in Mediterranean Cold-Water Corals: Past, Present and Future, eds C. Orejas and C. Jiménez (Cham: Springer), 261–263.
Parravicini, V., Mangialajo, L., Mousseau, L., Peirano, A., Morri, C., Montefalcone, M., et al. (2015). Climate change and warm-water species at the north-western boundary of the Mediterranean Sea. Mar. Ecol. 36, 897–909. doi: 10.1111/maec.12277
Pascual, M., Rives, B., Schunter, C., and Macpherson, E. (2017). Impact of life history traits on gene flow: A multispecies systematic review across oceanographic barriers in the Mediterranean Sea. PLoS One 12:e0176419. doi: 10.1371/journal.pone.0176419
Pham, C. K., Vandeperre, F., Menezes, G., Porteiro, F., Isidro, E., and Morato, T. (2015). The importance of deep-sea vulnerable marine ecosystems for demersal fish in the Azores. Deep Sea Res. Part Oceanogr. Res. Pap. 96, 80–88. doi: 10.1016/j.dsr.2014.11.004
Pianté, C., and Ody, D. (2015). Blue growth in the Mediterranean Sea: The Challenge of Good Environmental Status. France: MedTrends Project, 192.
Pusceddu, A., Bianchelli, S., Martín, J., Puig, P., Palanques, A., Masqué, P., et al. (2014). Chronic and intensive bottom trawling impairs deep-sea biodiversity and ecosystem functioning. Proc. Natl. Acad. Sci. U.S.A. 111, 8861–8866. doi: 10.1073/pnas.1405454111
R Core Team (2018). R: A Language and Environment for Statistical Computing. Vienna: R Foundation for Statistical Computing.
Ramirez-Llodra, E., Brandt, A., Danovaro, R., De Mol, B., Escobar, E., German, C., et al. (2010). Deep, diverse and definitely different: unique attributes of the world’s largest ecosystem. Biogeosciences 7, 2851–2899. doi: 10.5194/bg-7-2851-2010
Ramirez-Llodra, E., Tyler, P. A., Baker, M. C., Bergstad, O. A., Clark, M. R., Escobar, E., et al. (2011). Man and the last great wilderness: human impact on the deep sea. PLoS One 6:e22588. doi: 10.1371/journal.pone.0022588
Rassweiler, A., Costello, C., Hilborn, R., and Siegel, D. A. (2014). Integrating scientific guidance into marine spatial planning. Proc. R. Soc. B Biol. Sci. 281:20132252. doi: 10.1098/rspb.2013.2252
Roberts, J. M., Murray, F., Anagnostou, E., Hennige, S., Gori, A., Henry, L.-A., et al. (2016). “Cold-Water corals in an era of rapid global change: are these the deep ocean’s most vulnerable ecosystems?,” in The Cnidaria, Past, Present and Future, eds S. Goffredo and Z. Dubinsky (Cham: Springer), 593–606. doi: 10.1007/978-3-319-31305-4_36
Roberts, J. M., Wheeler, A. J., and Freiwald, A. (2006). Reefs of the deep: the biology and geology of cold-water coral ecosystems. Science 312, 543–547. doi: 10.1126/science.1119861
Roberts, J. M., Wheeler, A., Freiwald, A., and Cairns, S. (2009). Cold-Water Corals: The Biology and Geology of Deep-Sea Coral Habitats. Cambridge: Cambridge University Press.
Rodrigues, A. S., Cerdeira, J. O., and Gaston, K. J. (2000). Flexibility, efficiency, and accountability: adapting reserve selection algorithms to more complex conservation problems. Ecography 23, 565–574. doi: 10.1111/j.1600-0587.2000.tb00175.x
Rogers, A. D., Baco, A., Griffiths, H., Hart, T., and Hall-Spencer, J. M. (2007). “Corals on seamounts,” in Seamounts: Ecology, Fisheries & Conservation, eds T. J. Pitcher, T. Morato, P. J. B. Hart, M. R. Clark, N. Haggan, and R. S. Santos (Oxford: Blackwell Publishing Ltd), 141–169. doi: 10.1002/9780470691953.ch8
Ross, R. E., Nimmo-Smith, W. A. M., and Howell, K. L. (2017). Towards ‘ecological coherence’: Assessing larval dispersal within a network of existing Marine Protected Areas. Deep Sea Res. Part Oceanogr. Res. Pap. 126, 128–138. doi: 10.1016/j.dsr.2017.06.004
Rueda, J. L., Urra, J., Aguilar, R., Angeletti, L., Bo, M., Garcia-Ruiz, C., et al. (2019). “29 Cold-Water coral associated fauna in the mediterranean sea and adjacent areas,” in Mediterranean Cold-Water Corals: Past, Present and Future: Understanding the Deep-Sea Realms of Coral, eds C. Orejas and C. Jiménez (Cham: Springer), 295–333.
Schill, S. R., Raber, G. T., Roberts, J. J., Treml, E. A., Brenner, J., and Halpin, P. N. (2015). No reef is an Island: integrating coral reef connectivity data into the design of regional-scale marine protected area networks. PLoS One 10:e0144199. doi: 10.1371/journal.pone.0144199
Sharma, R. (ed.) (2017). Deep-Sea Mining: Resource Potential, Technical and Environmental Considerations. New York, NY: Springer International Publishing.
Skropeta, D., and Wei, L. (2014). Recent advances in deep-sea natural products. Nat. Prod. Rep. 31, 999–1025. doi: 10.1039/c3np70118b
Soetaert, K., Mohn, C., Rengstorf, A., Grehan, A., and van Oevelen, D. (2016). Ecosystem engineering creates a direct nutritional link between 600-m deep cold-water coral mounds and surface productivity. Sci. Rep. 6:35057. doi: 10.1038/srep35057
Strömberg, S. M., and Larsson, A. I. (2017). Larval behavior and longevity in the cold-water coral Lophelia pertusa indicate potential for long distance dispersal. Front. Mar. Sci. 4:411. doi: 10.3389/fmars.2017.00411
Sweetman, A. K., Thurber, A. R., Smith, C. R., Levin, L. A., Mora, C., Wei, C.-L., et al. (2017). Major impacts of climate change on deep-sea benthic ecosystems. Elem. Sci. Anthr. 5:4. doi: 10.1525/elementa.203
Synnes, M. (2007). Bioprospecting of organisms from the deep sea: scientific and environmental aspects. Clean Technol. Environ. Policy 9, 53–59. doi: 10.1007/s10098-006-0062-7
Thurber, A. R., Sweetman, A. K., Narayanaswamy, B. E., Jones, D. O. B., Ingels, J., and Hansman, R. L. (2014). Ecosystem function and services provided by the deep sea. Biogeosciences 11, 3941–3963. doi: 10.5194/bg-11-3941-2014
Tittensor, D. P., Baco, A. R., Hall-Spencer, J. M., Orr, J. C., and Rogers, A. D. (2010). Seamounts as refugia from ocean acidification for cold-water stony corals. Mar. Ecol. 31, 212–225. doi: 10.1111/j.1439-0485.2010.00393.x
UNESCO (2009). Global Open Oceans and Deep Seabed (GOODS) Biogeographic Classification (IOC Technical Series, 84.). Paris: UNESCO-IOC.
UNGA (2017). United Nations General Assembly Resolution 72/249. Resolution adopted by the General Assembly. International Legally Binding Instrument Under the United Nations Convention on the Law of the Sea on the Conservation and Sustainable use of Marine Biological Diversity of Areas Beyond National Jurisdiction. 72nd Session, Agenda Item 77. UN doc 748 A/RES/72/249. New York, NY: UNGA
Van Dover, C. L. (2011). Mining seafloor massive sulphides and biodiversity: what is at risk? ICES J. Mar. Sci. 68, 341–348. doi: 10.1093/icesjms/fsq086
Van Dover, C. L., Arnaud-Haond, S., Gianni, M., Helmreich, S., Huber, J. A., Jaeckel, A. L., et al. (2018). Scientific rationale and international obligations for protection of active hydrothermal vent ecosystems from deep-sea mining. Mar. Policy 90, 20–28. doi: 10.1016/j.marpol.2018.01.020
Veron, J. E. N. (2008). Mass extinctions and ocean acidification: biological constraints on geological dilemmas. Coral Reefs 27, 459–472. doi: 10.1007/s00338-008-0381-8
Visalli, M. E., Best, B. D., Cabral, R. B., Cheung, W. W. L., Clark, N. A., Garilao, C., et al. (2020). Data-driven approach for highlighting priority areas for protection in marine areas beyond national jurisdiction. Mar. Policy 122:103927. doi: 10.1016/j.marpol.2020.103927
Watson, R. A. (2017). A database of global marine commercial, small-scale, illegal and unreported fisheries catch 1950–2014. Nat. Sci. Data 4:170039. doi: 10.1038/sdata.2017.39
Watson, R. A., and Tidd, A. (2018). Mapping nearly a century and a half of global marine fishing: 1869–2015. Mar. Policy 93, 171–177. doi: 10.1016/j.marpol.2018.04.023
Wedding, L. M., Friedlander, A. M., Kittinger, J. N., Watling, L., Gaines, S. D., Bennett, M., et al. (2013). From principles to practice: a spatial approach to systematic conservation planning in the deep sea. Proc. R. Soc. B Biol. Sci. 280:20131684. doi: 10.1098/rspb.2013.1684
White, M., Mohn, C., de Stigter, H., and Mottram, G. (2005). “Deep-water coral development as a function of hydrodynamics and surface productivity around the submarine banks of the Rockall Trough, NE Atlantic,” in Cold-Water Corals and Ecosystems Erlangen Earth Conference Series, eds A. Freiwald and J. M. Roberts (Berlin: Springer Berlin Heidelberg), 503–514. doi: 10.1007/3-540-27673-4_25
Wright, G., Gjerde, K. M., Johnson, D. E., Finkelstein, A., Ferreira, M. A., Dunn, D. C., et al. (2019). Marine spatial planning in areas beyond national jurisdiction. Mar. Policy 103384. doi: 10.1016/j.marpol.2018.12.003
Yesson, C., Bedford, F., Rogers, A. D., and Taylor, M. L. (2017). The global distribution of deep-water Antipatharia habitat. Deep Sea Res. Part II Top. Stud. Oceanogr. 145, 79–86. doi: 10.1016/j.dsr2.2015.12.004
Yesson, C., Clark, M. R., Taylor, M. L., and Rogers, A. D. (2011). The global distribution of seamounts based on 30 arc seconds bathymetry data. Deep Sea Res. Part Oceanogr. Res. Pap. 58, 442–453. doi: 10.1016/j.dsr.2011.02.004
Keywords: marine spatial planning, marine protected areas, biodiversity conservation, spatial prioritization, connectivity, vulnerable marine ecosystems, open ocean, high seas
Citation: Combes M, Vaz S, Grehan A, Morato T, Arnaud-Haond S, Dominguez-Carrió C, Fox A, González-Irusta JM, Johnson D, Callery O, Davies A, Fauconnet L, Kenchington E, Orejas C, Roberts JM, Taranto G and Menot L (2021) Systematic Conservation Planning at an Ocean Basin Scale: Identifying a Viable Network of Deep-Sea Protected Areas in the North Atlantic and the Mediterranean. Front. Mar. Sci. 8:611358. doi: 10.3389/fmars.2021.611358
Received: 28 September 2020; Accepted: 03 June 2021;
Published: 30 June 2021.
Edited by:
Lorenzo Angeletti, Institute of Marine Science, National Research Council (CNR), ItalyReviewed by:
Lucia Bongiorni, Institute of Marine Science, National Research Council (CNR), ItalyGermana Garofalo, Institute for Biological Resources and Marine Biotechnology, National Research Council (CNR), Italy
Copyright © 2021 Combes, Vaz, Grehan, Morato, Arnaud-Haond, Dominguez-Carrió, Fox, González-Irusta, Johnson, Callery, Davies, Fauconnet, Kenchington, Orejas, Roberts, Taranto and Menot. This is an open-access article distributed under the terms of the Creative Commons Attribution License (CC BY). The use, distribution or reproduction in other forums is permitted, provided the original author(s) and the copyright owner(s) are credited and that the original publication in this journal is cited, in accordance with accepted academic practice. No use, distribution or reproduction is permitted which does not comply with these terms.
*Correspondence: Sandrine Vaz, c2FuZHJpbmUudmF6QGlmcmVtZXIuZnI=