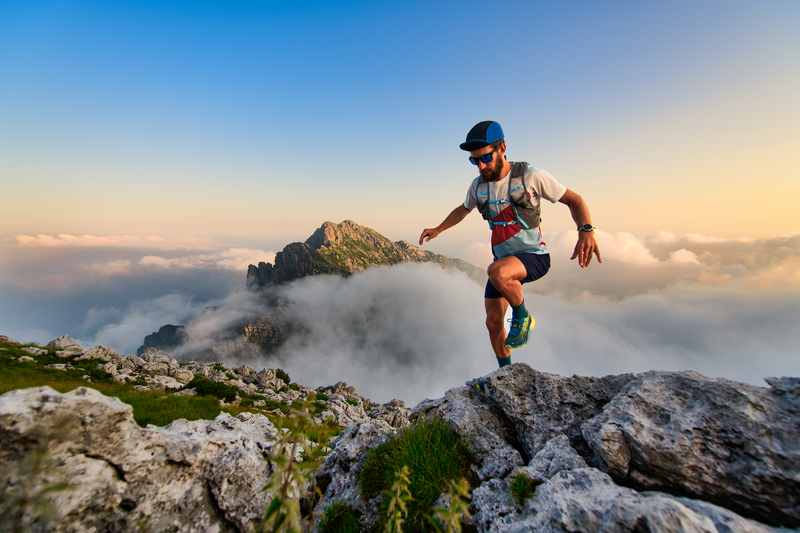
95% of researchers rate our articles as excellent or good
Learn more about the work of our research integrity team to safeguard the quality of each article we publish.
Find out more
BRIEF RESEARCH REPORT article
Front. Mar. Sci. , 22 March 2021
Sec. Marine Conservation and Sustainability
Volume 8 - 2021 | https://doi.org/10.3389/fmars.2021.611310
This article is part of the Research Topic The Dolphins of Sarasota Bay: Lessons from 50 years of Research and Conservation View all 14 articles
Exposure of common bottlenose dolphins (Tursiops truncatus) to brevetoxins (PbTx) produced by blooms of the toxic phytoplankton Karenia brevis frequently results in severe health impacts, including illness and large-scale mortality events. Although PbTx accumulation in dead-stranded dolphins is well documented, there are limited data for corresponding brevetoxin exposure in live dolphins. In addition, the severity of impacts on living survivors of such toxic blooms is difficult to assess due to a lack of data on the relationship between K. brevis bloom severity and corresponding PbTx concentrations in exposed animals. Here we present results of PbTx analysis of urine, serum, milk, gastric fluid, and feces samples collected from live, free-ranging dolphins (n = 253) from Sarasota Bay, Florida during 2000–2018, and investigate the relationship between PbTx concentrations detected and corresponding K. brevis cell abundances that are temporally (within 30 days) and spatially (within 16 km) associated with each individual. We found that 28% of dolphins were associated with elevated K. brevis abundances (10,000–60,000,000 cells/L), with 41% (n = 104) of dolphins testing positive for PbTx in at least one sample type. The proportion of PbTx-positive animals was significantly greater in animals exposed to elevated cell abundances vs. those exposed to background cell abundances (<10,000 cells/L), with 60 and 34% testing positive, respectively (p < 0.001). PbTx was detected most frequently in feces (57%, n = 38), followed by gastric (35%, n = 37), urine (32%, n = 55), and blood (7%, n = 17). PbTx concentrations by sample type were highest in feces (2–231 ng/g; mean 46), followed by urine (0.8–90 ng/g; mean 7.2), gastric (0.8–61 ng/g; mean 12), and blood (0.3–5 ng/g; mean 1.3). Regression analyses of K. brevis cell abundance as an index of exposure vs. corresponding PbTx concentration found no statistically significant relationship for feces (p = 0.120), gastric (p = 0.349), urine (p = 0.053), or blood (p = 0.729) samples. PbTx concentrations typically ranged over two orders of magnitude between minimum and maximum values and did not scale with corresponding indices of exposure, which ranged over three orders of magnitude or more. Our results indicate that K. brevis cell abundance alone is a poor predictor of brevetoxin accumulation in bottlenose dolphins, and suggest that alternative methods (e.g., endocrine or immunological biomarkers) should be investigated as more appropriate methods for determining the severity of health impacts due to red tides.
Harmful algal blooms (HABs) are frequently associated with morbidity and large-scale mortalities of marine mammals, due to exposure to potent neurotoxins produced during such events (Broadwater et al., 2018; NOAA, 2020). Assessing the impacts of HAB toxins on marine mammals is often problematic, and is complicated by complex trophic interactions, time lags between blooms and observable impacts, and logistical constraints inherent in sampling free-ranging animals. Important marine sentinel species such as the common bottlenose dolphin (Tursiops truncatus) are apex predators that are typically distanced from toxic phytoplankton by several trophic links, with the organisms in each link having distinct rates of toxin accumulation and/or depuration (Landsberg, 2002; Doucette et al., 2006; Naar et al., 2007). Should a strong correlative relationship exist between the toxin concentration detected in an animal and the severity of the HAB to which it was exposed, this would serve as a useful tool to predict toxin accumulation in individuals or back-calculate toxic phytoplankton abundances associated with mortality events. Previous work has suggested that the abundance of toxic phytoplankton alone is a poor predictor of toxin accumulation rates and likelihood of exposure in dead-stranded marine mammals (Fire et al., 2007, 2020a; Landsberg et al., 2009). This is primarily due to the fact that apex predator marine mammals do not graze on phytoplankton nor ingest large amounts of seawater, accumulate negligible amounts of HAB toxins transdermally or via inhalation, leaving dietary intake (through large fish and invertebrates) as the only feasible route of biologically significant exposure. However, the relationship between phytoplankton abundance and toxin accumulation in exposed animals has not been specifically demonstrated for live, free-ranging marine mammals.
Bottlenose dolphins endemic to the west coast of Florida are frequently and severely impacted by blooms of the red tide organism Karenia brevis, which occur on a nearly annual basis (Steidinger et al., 1998; Steidinger, 2009) and produce a suite of neurotoxins known as brevetoxins (PbTx). These blooms typically occur over very large spatial areas (∼3,000 km2), can last for several months, and often result in mass mortality events of multiple species of marine megafauna (Zhao et al., 2013; Broadwater et al., 2018). This is of particular concern for populations of non-migratory, resident marine mammals that do not travel long distances and therefore reside in red tide-affected areas for the entire duration of each bloom. Although high PbTx tissue loads and acute PbTx exposure have been assigned as the cause of several large-scale dolphin mortality events in Florida waters, corresponding information for the impacts on living dolphins that survive such events is limited (Twiner et al., 2012; Litz et al., 2014). The present study addresses this lack of knowledge by (1) providing a long-term dataset of PbTx loads in resident dolphins from a region that frequently experiences severe K. brevis blooms, and (2) by investigating the relationship between K. brevis abundance associated with individual dolphins sampled in the field and their degree of PbTx exposure. Here we present PbTx data from live, free-ranging bottlenose dolphins resident to Sarasota Bay, Florida from 2000–2018, and test the utility of K. brevis abundance data as an index of exposure that corresponds to PbTx concentrations detected in various toxicologically important sample matrices.
Sarasota Bay, Florida (27°N, 82°W), a coastal barrier island estuary of approximately 125 km2, was selected as the location for the present study since it (1) experiences frequent, severe K. brevis blooms, (2) is a region of continuous HAB monitoring by multiple phytoplankton ecology research programs, and (3) is inhabited by a population of about 150 bottlenose dolphins that have been intensively studied for over 50 years (Scott et al., 1990; Wells, 2003; FWC, 2019; MML, 2019). Through long-term study of this population, the majority of these animals are individually identifiable in the field, have known sexes, ages, and spatial use and ranging patterns, and are therefore known to have high site fidelity to Sarasota Bay (Wells, 2014; Tyson and Wells, 2016). As a result of multiple years of photographic mark/recapture efforts on members of this population, the home ranges of most individuals confirm that they are year-round residents of Sarasota Bay, and therefore will be exposed to any K. brevis blooms present within the study area (Fire et al., 2020b).
Samples of urine, feces, blood, milk, and gastric fluid were collected from bottlenose dolphins sampled during health assessments occurring between June 2000 and June 2018, following methods described in Wells et al. (2004). Briefly, dolphins were captured by encircling them with a 500 m × 4 m seine net deployed in shallow (<2 m) waters and secured by a team of veterinarians, trained dolphin handlers, and biologists to ensure the safe restraint of the animals. Each sampled individual was transferred to a padded, shaded platform onboard a 9 m veterinary examination boat where body measurements and various health samples and data were collected, and then the dolphin was released on-site. Whole blood was collected via venipuncture of the fluke vasculature and was stored using blood spot collection cards as described by Maucher et al. (2007), or separated by centrifugation and the serum fraction collected for analysis. Urine samples were collected via the urethra using a sterile catheter. Gastric samples were collected using a small tube inserted via the esophagus into the stomach, from which stomach fluid was drained and stored. Milk samples were collected via negative pressure applied using a modified catheter-syringe device placed over the expressed mammary gland. Feces samples were taken during processing using a sterile catheter. All samples were collected into sterile polypropylene vials and frozen (−20°C for blood cards, −80°C for all other samples) until toxin analysis was conducted. Several dolphins were sampled multiple times during the study period, and duplicate data for animals sampled more than once during the same health assessment were excluded from the dataset. Sample sets from the same individual but collected during separate health assessments (≥1 year apart) were treated as independent sampling events for this study.
Samples were extracted according to methods described in Twiner et al. (2011) and Fire et al. (2019). Fluid samples (urine, serum, milk, or gastric fluid) were centrifuged at 10,000 × g for 10 min. The supernatant was collected and filtered using 0.45 μm syringe-driven glass fiber filters. Feces samples and sections of blood spot cards were mechanically disrupted using a handheld tissue homogenizer in three volumes of acetone (1:3 w/v). The resulting slurry was sonicated for 120 s, transferred to conical polypropylene tubes, and centrifuged at 3,400 × g at 15°C for 15 min. The pellet was re-extracted in an additional three volumes of acetone, centrifuged and the combined supernatants were filtered via a 0.45 μm syringe filter, evaporated, and resuspended in 6 mL of 80% aqueous methanol. This solution was solvent partitioned twice with 6 mL hexane, and the methanolic fraction collected, evaporated and resuspended in 2 mL of 100% methanol. Extracts were stored at −20°C until analysis.
The extracts and filtered samples were analyzed for PbTx using a commercially available enzyme-linked immunosorbent assay (ELISA), following procedures outlined by the manufacturer (Abraxis LLC, Warminster, PA, United States). The ELISA measures competition between free PbTx molecules and PbTx -enzyme conjugates for binding sites on anti-PbTx antibodies immobilized on a microtiter plate, in order to determine the total PbTx binding activity of the sample extract. A subset of samples was also analyzed using a PbTx radioimmunoassay (RIA) and/or liquid chromatography-tandem mass spectrometry (LC-MS/MS) methods described in Twiner (Twiner et al., 2011). The limit of detection varied among assays but was approximately 1.0 ng PbTx per gram of sample.
Quantitative cell abundance data from statewide K. brevis monitoring efforts occurring in Florida were obtained from the Harmful Algal BloomS Observing System (HABSOS) database maintained by the NOAA National Centers for Environmental Information (NOAA/NCEI, 2020). A subset of queried data corresponding to K. brevis cell counts (# cells/L of seawater) from Florida state waters for the period from 01 January 1999 to 31 December 2018 was used to assign numerical proxy values of bloom exposure to individual dolphins in the sample set. Associated metadata included date/time of sampling, latitude/longitude, county, and categorical abundance descriptions (not observed, 0 cells/L; very low, >0–10,000 cells/L; low, >10,000–100,000 cells/L; medium, >100,000–1,000,000 cells/L; high, >1,000,000 cells/L).
Dolphin sampling dates and locations were cross-referenced with HABSOS K. brevis abundance data to assign each individual to one of two bloom exposure groups, based on the following criteria: (1) the “Exposed” group included animals that were temporally and spatially associated with any K. brevis abundance value of ≥10,000 cells/L and (2) the “Baseline” group included all remaining animals, including those for which no corresponding K. brevis abundance values were available. Since the K. brevis dataset is the result of primarily event-response sampling, it was assumed for this study that an absence of K. brevis data represented background cell abundances. A custom script to match K. brevis abundance data to each individual’s stranding date and location was generated using Google Visualization API Query Language (version 0.7) and a corresponding query in a Google Sheets worksheet (Google LLC, Mountain View, CA, United States). The script utilized the Haversine formula for determining the distance between two latitude/longitude points (Robusto, 1957) to select only those K. brevis data points that fell within a 16-km radius of a given dolphin’s sampling location. This subset of data was further restricted to include only values occurring within the 30-day window prior to the dolphin sampling date. The 16-km radius was selected based on estimated maximum travel distances for resident bottlenose dolphins whose movements are known to be restricted to Sarasota Bay, which has a maximum linear distance of approximately 32 km. The 30-day date range criterion for the Exposed group was selected based on previous studies describing the persistence of lipophilic toxins such as PbTx in animal dose-response models, suggesting a residence time/lag time of up to several weeks in exposed prey items of bottlenose dolphins (Naar et al., 2007; Fire et al., 2008a; Hinton and Ramsdell, 2008). Frequencies of PbTx-positive animals (presence/absence of PbTx in at least one sample type per individual) between exposure groups were compared using logistic regression. PbTx concentrations by sample type (blood, urine, gastric, and feces) were also compared between exposure groups, using Welch’s t-test. Due to the wide range of values detected, PbTx concentrations were log-transformed prior to analysis (ln + 1) and concentrations reported as below the assay limit of detection (<dl) were assigned zero values.
Karenia brevis abundance data were used to investigate any dose-response relationship between K. brevis exposure and PbTx concentrations detected for each sample type. The maximum cell abundance value from each animal’s set of filtered values was assigned as an index of exposure for each animal, and compared using logistic regression as above, with the index of exposure as the independent variable and the PbTx concentration in each sample type as the dependent variable. Animals for which no corresponding K. brevis data were found were assigned zero values for their index of exposure. PbTx concentrations reported as <dl were assigned zero values for these analyses.
A logistic regression model was used to determine if the probability of PbTx detection in an animal can be predicted using the index of exposure, with the index (cells/L) as the independent variable and presence/absence of PbTx in the same individual as the dependent variable. In addition, a Spearman correlation was performed between the index of exposure and PbTx concentration for each sample type. Index of exposure values and PbTx concentrations used to determine presence/absence in these tests were assigned using the criteria above. Analyses were conducted using R 3.6.3 (R Core Team, 2020). All significance values were set at α = 0.05.
In total, 253 dolphins were tested for PbTx between 2000 and 2018, yielding 599 samples of urine, feces, gastric, milk or blood. Twenty-eight percent of dolphins (n = 70) were associated with K. brevis abundance values of ≥10,000 cells/L, and were therefore assigned to the Exposed group; the remaining 72% (n = 183) were assigned to the Baseline group. Maximum K. brevis cell abundances associated with each dolphin ranged from background levels (≤1,000 cells/L) to 60,000,000 cells/L (Supplementary Table 1). Overall, 41% (n = 104) of dolphins tested positive for PbTx in at least one sample type (Table 1). Comparing between exposure groups, the proportion of PbTx-positive animals was significantly greater in the Exposed group relative to the Baseline group, with 60% and 34% testing positive, respectively (p < 0.001).
Table 1. Proportions of PbTx-positive dolphins and sample types by exposure category, and PbTx concentrations detected.
PbTx was detected most frequently in feces (57%, n = 38), followed by gastric (35%, n = 37), urine (32%, n = 55), and blood (7%, n = 17) (Table 1). PbTx concentrations by sample type were highest in feces (2–231 ng/g; mean 46), followed by urine (0.8–90 ng/g; mean 7.2), gastric (0.8–61 ng/g; mean 12), and blood (0.3–5 ng/g; mean 1.3). PbTx was not detected in milk samples, which were therefore excluded from statistical analyses.
PbTx concentrations were significantly higher in the Exposed group relative to the Baseline group for feces (p < 0.001), gastric (p = 0.032), and urine (p = 0.013) samples, but were not significantly different for blood samples (p = 0.133; Figure 1).
Simple regression analyses of K. brevis cell abundance as an index of exposure vs. corresponding PbTx concentration found no statistically significant relationship for feces (p = 0.120), gastric (p = 0.349), urine (p = 0.053), or blood (p = 0.729) samples. Spearman correlation analyses indicated that while correlation was statistically significant for all sample types, correlation coefficients were close to zero for all sample types except feces (rho = 0.715 feces; 0.365 gastric; 0.399 urine; 0.256 blood; Figure 2). Regression analysis of index of exposure vs. presence/absence of PbTx in individual dolphins indicated no statistically significant relationship (p = 0.580; data not shown).
Overall, our findings indicate that the majority of live, free-ranging bottlenose dolphins exposed to K. brevis cell abundances of >10,000 cells/L accumulate detectable levels of PbTx, and do so at approximately twice the frequency of individuals sampled at background K. brevis abundances. Similarly, the concentrations of PbTx detected in three of four sample types were also higher in dolphins sampled during elevated levels of K. brevis vs. those sampled during baseline conditions. This complements earlier work demonstrating large differences in the PbTx concentrations detected and proportion of PbTx-positive dolphin carcasses recovered during red tides vs. those recovered during non-bloom conditions (Fire et al., 2007). However, our results contrast with previous findings demonstrating that live dolphins sampled during non-bloom conditions show no evidence of PbTx exposure (Fire et al., 2008b). These conflicting results are likely due to differences in sampling design and sample size, as the previous preliminary effort included only a handful of non-bloom individuals, all of which were sampled during the same 2-week health assessment. We suggest that the results of the present study are a more accurate representation of degree and frequency of PbTx exposure in live dolphins from this region.
Our results indicate that K. brevis is a poor predictor of detectable PbTx concentrations in gastric, urine and blood samples, and a weak predictor of PbTx concentration in feces samples. While analysis of some sample types showed significant correlation with index of exposure, correlation coefficients approaching zero indicate that these relationships were not biologically meaningful. We found relatively low prevalence of PbTx in blood samples, and narrow ranges of PbTx concentrations in feces, gastric, and urine samples despite high variability in their associated K. brevis abundance values. PbTx concentrations typically ranged over two orders of magnitude between minimum and maximum values and did not scale with corresponding indices of exposure which ranged over three orders of magnitude or more.
These findings indicate that despite the feasibility of collecting feces, urine, gastric, and blood samples from live, free ranging dolphins, and their utility in determining presence/absence of PbTx in exposed individuals, they have limited use in estimating the degree of exposure to K. brevis blooms. This is likely due to the uncertainty with which K. brevis exposure levels were assigned to the animals in the present study, as the 30-day window used to assign indices of exposure to each dolphin was based on limited information on estimated PbTx transfer rates through the food web (Landsberg, 2002; Doucette et al., 2006; Naar et al., 2007). Likewise, the 16-km buffer used to assign K. brevis cell counts to each dolphin sampling event may be insufficiently precise to account for the actual movements of individuals used in this study. In addition, the spatial distribution of K. brevis is highly heterogeneous at scales of a few kilometers, and the discontinuous, patchy nature of these blooms may account for much of the variation observed in the indices of exposure used herein (Tester and Steidinger, 1997; Zhao et al., 2013). The type of sample able to be collected safely from living, wild dolphins may also be a limitation in estimating exposure. Ideally, liver tissue is very useful for addressing PbTx detoxification and depuration rates in experimental dosing models, as well intestinal tissue for determining how much PbTx is assimilated from the gastrointestinal tract, however, these samples were not available from the live animals in this study. While PbTx concentrations in blood are theoretically at equilibrium with the rest of the tissues in an exposed organism, serum PbTx values in bottlenose dolphins are typically at concentrations near the limit of detection for most PbTx analytical methods, and may often go undetected.
Further efforts to assess the relationship between K. brevis exposure and PbTx accumulation in live dolphins may benefit from more physiologically relevant biomarkers of toxin exposure, potentially including plasma cortisol concentration or immune response. Feces and gastric samples are only proxies for uptake and assimilation of toxins, and feces and urine as indicators of detoxification and depuration via renal and biliary routes do not provide information on real-time health impacts. In vitro exposure of dolphin peripheral blood cells to PbTx is known to increase proliferation of multiple immune cell types, and this merits investigation as a complementary data set to those used in the present study (Gebhard et al., 2015). In addition, targeted fine-scale seawater sampling combined with tracking movements of live dolphins immediately prior to health assessments may give more accurate estimates of individual exposure to K. brevis cells and reduce the impact of patchy cell distribution on future analyses.
The original contributions presented in the study are included in the article/Supplementary Material, further inquiries can be directed to the corresponding author/s.
The animal study was reviewed and approved by Mote Marine Laboratory annual Institutional Animal Care and Use Committee.
SF designed and oversaw all aspects of the study. GM provided statistical guidance and analyses, and assisted with development of the manuscript. ES carried out laboratory analyses and assisted with development of the manuscript. RW provided field logistical support, field sampling design, sample collection, and assisted with development of the manuscript. All authors contributed to the article and approved the submitted version.
Funding was provided by the National Marine Fisheries Service, Dolphin Quest, and Disney’s Animal Programs. Publication of this article was funded in part by the Florida Tech Open Access Subvention Fund and the John H. Evans Library.
The authors declare that the research was conducted in the absence of any commercial or financial relationships that could be construed as a potential conflict of interest.
We would like to thank the staff, volunteers, and various collaborators of the Chicago Zoological Society’s Sarasota Dolphin Research Program for assistance with animal care and handling, sample collection and processing, and logistical support. We would also like to thank the Mote Marine Laboratory Phytoplankton Ecology Program and the Florida Fish and Wildlife Conservation Commission for use of K. brevis cell count datasets. We would also further like to thank H. Giddens, J. Maucher, T. Leighfield, S. Powers, L. Symon, and V. Palubok for technical assistance with laboratory analyses and enumeration of phytoplankton samples. All efforts were conducted under National Marine Fisheries Service Scientific Research Permits No. 522-1569, No. 522-1785, No. 15543, and No. 20455 issued to RSW and approved through Mote Marine Laboratory annual Institutional Animal Care and Use Committee (IACUC) reviews.
The Supplementary Material for this article can be found online at: https://www.frontiersin.org/articles/10.3389/fmars.2021.611310/full#supplementary-material
Broadwater, M. H., Van Dolah, F. M., and Fire, S. E. (2018). “Vulnerabilities of marine mammals to harmful algal blooms,” in Harmful Algal Blooms, Vol. 8, eds S. E. Shumway, J. M. Burkholder, and S. L. Morton (Hoboken, NJ: John Wiley & Sons, Ltd), 191–222. doi: 10.1002/9781118994672.ch5
Doucette, G., Maneiro, I., Riveiro, I., and Svensen, C. (2006). “Phycotoxin pathways in aquatic food webs: transfer, accumulation and degradation,” in Ecology of Harmful Algae, eds E. Graneli and J. T. Turner (Berlin: Springer Verlag), 283–295. doi: 10.1007/978-3-540-32210-8_22
Fire, S., Fauquier, D., Flewelling, L., Henry, M., Naar, J., Pierce, R., et al. (2007). Brevetoxin exposure in bottlenose dolphins (Tursiops truncatus) associated with Karenia brevis blooms in Sarasota Bay, Florida. Mar. Biol. 152, 827–834. doi: 10.1007/s00227-007-0733-x
Fire, S. E., Browning, J. A., Durden, W. N., and Stolen, M. K. (2019). Comparison of during-bloom and inter-bloom brevetoxin and saxitoxin concentrations in Indian River Lagoon bottlenose dolphins, 2002-2011. Aquat. Toxicol. 218:105371. doi: 10.1016/j.aquatox.2019.105371
Fire, S. E., Flewelling, L. J., Naar, J., Twiner, M. J., Henry, M. S., Pierce, R. H., et al. (2008a). Prevalence of brevetoxins in prey fish of bottlenose dolphins in Sarasota Bay, Florida. Mar. Ecol. Prog. Ser. 368, 283–294. doi: 10.3354/meps07643
Fire, S. E., Flewelling, L. J., Wang, Z., Naar, J., Henry, M. S., Pierce, R. H., et al. (2008b). Florida red tide and brevetoxins: association and exposure in live resident bottlenose dolphins Tursiops truncatus in the eastern Gulf of Mexico, U.S.A. Mar. Mamm. Sci. 24, 831–844.
Fire, S. E., Leighfield, T. A., Miller, G. A., Piwetz, S., Sabater, E. R., and Whitehead, H. (2020a). Association between red tide exposure and detection of corresponding neurotoxins in bottlenose dolphins from Texas waters during 2007–2017. Mar. Environ. Res. 162:105191. doi: 10.1016/j.marenvres.2020.105191
Fire, S. E., Miller, G. A., and Wells, R. S. (2020b). Explosive exhalations by common bottlenose dolphins during Karenia brevis red tides. Heliyon 6:e03525. doi: 10.1016/j.heliyon.2020.e03525
Gebhard, E., Levin, M., Bogomolni, A., and De Guise, S. (2015). Immunomodulatory effects of brevetoxin (PbTx-3) upon in vitro exposure in bottlenose dolphins (Tursiops truncatus). Harmful Algae 44, 54–62. doi: 10.1016/j.hal.2015.02.010
Hinton, M., and Ramsdell, J. S. (2008). Brevetoxin in two planktivorous fishes after exposure to Karenia brevis: implications for food-web transfer to bottlenose dolphins. Mar. Ecol. Prog. Ser. 356, 251–258. doi: 10.3354/meps07267
Landsberg, J. H. (2002). The effects of harmful algal blooms on aquatic organisms. Rev. Fish. Sci. 10, 113–390. doi: 10.1080/20026491051695
Landsberg, J. H., Flewelling, L. J., and Naar, J. (2009). Karenia brevis red tides, brevetoxins in the food web, and impacts on natural resources: decadal advancements. Harmful Algae 8, 598–607. doi: 10.1016/j.hal.2008.11.010
Litz, J. A., Baran, M. A., Bowen-Stevens, S. R., Carmichael, R. H., Colegrove, K. M., Garrison, L. P., et al. (2014). Review of historical unusual mortality events (UMEs) in the Gulf of Mexico (1990-2009): providing context for the multi-year northern Gulf of Mexico cetacean UME declared in 2010. Dis. Aquat. Organ. 112, 161–175. doi: 10.3354/dao02807
Maucher, J. M., Briggs, L., Podmore, C., and Ramsdell, J. S. (2007). Optimization of blood collection card method/enzyme-linked immunoassay for monitoring exposure of bottlenose dolphin to brevetoxin-producing red tides. Environ. Sci. Technol. 41, 563–567. doi: 10.1021/es0612605
Naar, J. P., Flewelling, L. J., Lenzi, A., Abbott, J. P., Granholm, A., Jacocks, H. M., et al. (2007). Brevetoxins, like ciguatoxins, are potent ichthyotoxic neurotoxins that accumulate in fish. Toxicon 50, 707–723. doi: 10.1016/j.toxicon.2007.06.005
NOAA (2020). Marine Mammal Unusual Mortality Events, 1991-2020. Washington, DC: National Oceanic and Atmospheric Administration Office of Protected Resources.
NOAA/NCEI (2020). Harmful Algal BloomS Observing System (HABSOS). Washington, DC: National Oceanic and Atmospheric Administration, National Centers for Environmental Information.
R Core Team (2020). R: A Language and Environment for Statistical Computing. Vienna: R Foundation for Statistical Computing.
Scott, M. D., Wells, R. S., and Irvine, A. B. (1990). “A long-term study of bottlenose dolphins on the west coast of Florida,” in The Bottlenose Dolphin, eds S. Leatherwood and R. R. Reeves (Cambridge, MA: Academic Press), 235–244. doi: 10.1016/b978-0-12-440280-5.50015-9
Steidinger, K. A. (2009). Historical perspective on Karenia brevis red tide research in the Gulf of Mexico. Harmful Algae 8, 549–561. doi: 10.1016/j.hal.2008.11.009
Steidinger, K. A., Vargo, G. A., Tester, P. A., and Tomas, C. R. (1998). “Bloom dynamics and physiology of Gymnodinium breve with emphasis on the Gulf of Mexico,” in Physiological Ecology of Harmful Algal Blooms, eds D. M. Anderson, A. D. Cembella, and G. M. Hallegraeff (Berlin: Springer-Verlag), 133–153.
Tester, P. A., and Steidinger, K. A. (1997). Gymnodinium breve red tide blooms: initiation, transport, and consequences of surface circulation. Limnol. Oceanogr. 42(5, part 2), 1039–1051. doi: 10.4319/lo.1997.42.5_part_2.1039
Twiner, M. J., Fire, S., Schwacke, L., Davidson, L., Wang, Z., Morton, S., et al. (2011). Concurrent exposure of bottlenose dolphins (Tursiops truncatus) to multiple algal toxins in Sarasota Bay, Florida, USA. PLoS One 6:e17394. doi: 10.1371/journal.pone.0017394
Twiner, M. J., Flewelling, L. J., Fire, S. E., Bowen-Stevens, S. R., Gaydos, J. K., Johnson, C. K., et al. (2012). Comparative analysis of three brevetoxin-associated bottlenose dolphin (Tursiops truncatus) mortality events in the Florida Panhandle region (USA). PLoS One 7:e42974. doi: 10.1371/journal.pone.0042974
Tyson, R. B., and Wells, R. S. (2016). Sarasota Bay/Little Sarasota Bay Bottlenose Dolphin Abundance Estimates: 2015. Washington, DC: NOAA.
Wells, R. S. (2003). “Dolphin social complexity: lessons from long-term study and life history,” in Animal Social Complexity: Intelligence, Culture, and Individualized Societies, eds F. B. M. de Waal and P. L. Tyack (Cambridge, MA: Harvard University Press), 32–56. doi: 10.4159/harvard.9780674419131.c4
Wells, R. S. (2014). “Social structure and life history of bottlenose dolphins near Sarasota Bay, Florida: insights from four decades and five generations,” in Primates and Cetaceans: Field Research and Conservation of Complex Mammalian Societies, Primatology Monographs, eds J. Yamagiwa and L. Karczmarski (Berlin: Springer), 149–172. doi: 10.1007/978-4-431-54523-1_8
Wells, R. S., Rhinehart, H. L., Hansen, L. J., Sweeney, J. C., Townsend, F. I., Stone, R., et al. (2004). Bottlenose dolphins as marine ecosystem sentinels: developing a health monitoring system. EcoHealth 1, 246–254.
Keywords: HAB (harmful algal bloom), brevetoxin, Tursiops truncatus, bottlenose dolphin (Tursiops truncatus), biotoxins, phycotoxins
Citation: Fire SE, Miller GA, Sabater ER and Wells RS (2021) Utility of Red Tide (Karenia brevis) Monitoring Data as a Predictive Tool to Estimate Brevetoxin Accumulation in Live, Free-Ranging Marine Mammals. Front. Mar. Sci. 8:611310. doi: 10.3389/fmars.2021.611310
Received: 28 September 2020; Accepted: 04 March 2021;
Published: 22 March 2021.
Edited by:
Rochelle Diane Seitz, College of William & Mary, United StatesReviewed by:
Andrew M. Fischer, University of Tasmania, AustraliaCopyright © 2021 Fire, Miller, Sabater and Wells. This is an open-access article distributed under the terms of the Creative Commons Attribution License (CC BY). The use, distribution or reproduction in other forums is permitted, provided the original author(s) and the copyright owner(s) are credited and that the original publication in this journal is cited, in accordance with accepted academic practice. No use, distribution or reproduction is permitted which does not comply with these terms.
*Correspondence: Spencer E. Fire, c2ZpcmVAZml0LmVkdQ==
Disclaimer: All claims expressed in this article are solely those of the authors and do not necessarily represent those of their affiliated organizations, or those of the publisher, the editors and the reviewers. Any product that may be evaluated in this article or claim that may be made by its manufacturer is not guaranteed or endorsed by the publisher.
Research integrity at Frontiers
Learn more about the work of our research integrity team to safeguard the quality of each article we publish.