- 1Thünen Institute of Baltic Sea Fisheries, Rostock, Germany
- 2Department of Aquatic Ecology, University of Rostock, Rostock, Germany
- 3Institute for Marine Ecosystems and Fisheries, University of Hamburg, Hamburg, Germany
- 4Centre for Clinical Veterinary Medicine, Faculty of Veterinary Medicine, Ludwig-Maximilians University, Munich, Germany
Shallow estuaries, bays, and lagoons are generally considered hot spots of ocean productivity that often adjust rapidly to seasonal variations in atmospheric temperatures. During spring when biological reproductive processes begin in the temperate zones, regional climate variability can be immense and uncovering a non-linear biological response, such as fish recruitment to changing temperature regimes might be challenging. Using herring as a paradigm for a response of coastal spring productivity to regional climate drivers, we demonstrated how the annual timing of spawning periods can significantly affect the reproductive success of spring-spawning herring (Clupea harengus) in the western Baltic Sea. An investigation of spawning phenology in consecutive years indicated a temperature threshold range of 3.5–4.5°C triggering initial spawning in the coastal zone. Based on this finding, we analyzed the timing of larval hatching peaks, larval survival and recruitment to the adult population relative to multi-decadal time-series of seasonal sea-surface temperatures. The results revealed that the late seasonal onset of cold periods the corresponding elongation of the period where larvae hatch from the eggs and early larval hatching peaks significantly reduced larval production in a coastal nursery area and finally lead to a reduced abundance of juveniles in the entire distribution area. Using a combination of field research and time series analysis, we presented precedence for shifting regional winter regimes providing a present-day stressor to reproductive capacity of a central component of the coastal food web.
Introduction
There is increasing evidence that spring temperatures are rising in the temperate zones (Schwartz et al., 2006). As a result, shifts in the phenology of species in flora and fauna become increasingly obvious, especially in terrestrial ecosystems where many phenomena, such as flowering of plants or bird migration, are commonly observed (Kaspar et al., 2015; Socolar et al., 2017). On land, the immediate effect of changing climate regimes on the timing of seasonal succession and processes in nature is noticed as a present-day threat to species’ reproductive success (Nature Climate Change, 2018). In the marine environment, however, most attention is paid to the direct physiological effects of global ocean warming and acidification (Catalán et al., 2019). In aquatic systems, shifts in the seasonal order of natural phenomena are not as readily visible as in terrestrial systems, but have been reported from a variety of temperate oceans particularly for fish species subjected to a seasonal gradient in migration, feeding, and reproduction (Platt et al., 2003; Edwards and Richardson, 2004; Rogers and Dougherty, 2019). Recently, Bates et al. (2018) suggested that ecologists include regional climate regimes in their studies rather than relating changes in biota to average global warming. This criticism seems well justified considering that species phenology is often subjected to regional climate regimes and inshore, coastal systems are globally known as hot spots of ocean productivity and biodiversity (Cloern et al., 2014; Simcock, 2017). In the temperate zones of higher latitudes, seasonal temperature regimes in shallow estuaries, bays, and lagoons fluctuate greatly owing to the effect of local air temperatures and wind mixing. The high temperature variability of transitional waters as a response to local weather regimes might obscure present effects of global ocean warming on coastal biota. However, shorter winters and steep spring temperature progressions might already affect these systems greatly, potentially influencing keystone species of coastal food webs with determined seasonal timing of reproduction processes.
The search for the drivers of fish recruitment variability of ecological and economic keystone species such as Atlantic herring dates to the beginning of fishery science. Since Johan Hjort (1914) related the survival of early larval stages to the year-class strength of recruits, it has generally been accepted that larval fish mortality represents a major bottleneck in recruitment (Houde, 2008; Oeberst et al., 2009b). In addition to biotic top–down and bottom–up control, the physico-chemical environment is of superior importance to larval growth and survival. The emerging awareness of climate-change-induced warming of the oceans fueled an abundance of studies of the physiological and metabolic responses of fish against rising ocean temperatures (Pörtner and Knust, 2007; Pörtner and Peck, 2010; Moyano et al., 2020). In addition to the direct temperature effect on the metabolism of the individual, changes in the temperature regime might greatly affect the reproduction phenology on the population level and, therefore, the match of susceptible early life stages with ambient environmental conditions.
Western Baltic spring-spawning herring is considered a meta-population of Clupea harengus (Bekkevold et al., 2005), that performs extensive migration from summer feeding grounds in the North Sea to constrained overwintering areas in the western Baltic Sea (i.e., Øresund, DK, SWE). In spring, they enter the inshore estuaries, bays, lagoons, and even artificial canals along the west and south coasts of the Baltic Sea for spawning. In late winter and early spring, herring schools aggregate in front of the inlets to inshore spawning grounds prior to spawning. Such aggregations are harvested by an industrial trawl fishery, while fish entering the shallow spawning ground are targeted by an artisanal gillnet fishery. For the early herring life stages those shallow systems are important nursery areas because they pass through the entire early life history in the area before they metamorphose and relocate to shore zone habitats of the outer coastal zone (Polte et al., 2017). As particular nurseries and inherent spawning grounds often make a greater-than-average contribution to the adult populations, local impacts can drastically affect the large-scale population level (Beck et al., 2001). Therefore, shallow coastal fish nursery areas may provide suitable model systems to identify mechanisms and ecological pathways of climate effects from the very early life history at the inner coast until recruitment to the population in the ocean.
Using herring as a paradigm for the effect of regional climate on coastal productivity, we investigated the sensitivity of Atlantic herring reproduction in the Baltic Sea against regional winter characteristics. By complementing previous studies that related global climate indices to fish recruitment dynamics (Cardinale et al., 2009; Gröger et al., 2014; Polte et al., 2014), the novel approach of this study was to identify an in situ temperature threshold for the onset of spawning that was then used to carefully dissect winter temperature patterns according to explanatory power for herring reproductive success.
By combining systematic ecological observation on successive early life stages (eggs-larvae-juveniles) with an information-theory-based selection of regional winter descriptors, we addressed the following hypotheses: (i) initial spawning is subjected to regional temperature thresholds, and (ii) onset and duration of cold periods relative to those thresholds can explain a significant part of recruitment dynamics.
Materials and Methods
Study System
Already in the 1930s Greifswald Bay, a shallow lagoon near the German island of Rügen (Figure 1) was documented as a major spawning area for western Baltic spring-spawning herring (Biester, 1989). The bay covers an area of 514 km2, and the average water depth is 5.8 m with a maximum of 13.6 m (Reinicke, 1989). Currents are predominantly wind-driven because tidal amplitudes are marginal (<10 cm, semi-diurnal). The annual mean salinity is 7.3 (Kell, 1989). Seasonal temperature fluctuations are large, ranging from regular sub-zero °C sea-surface temperatures (SST), with a closed ice sheet in winter, to more than 20°C SST during summer. Owing to the shallowness and frequent wind mixing, no thermoclines exist during spring, and there is no relevant difference between SST and bottom temperatures. Although the system is affected by eutrophication (Munkes, 2005), extensive mixing by wind forcing results in dissolved oxygen content close to 100% at the seabed.
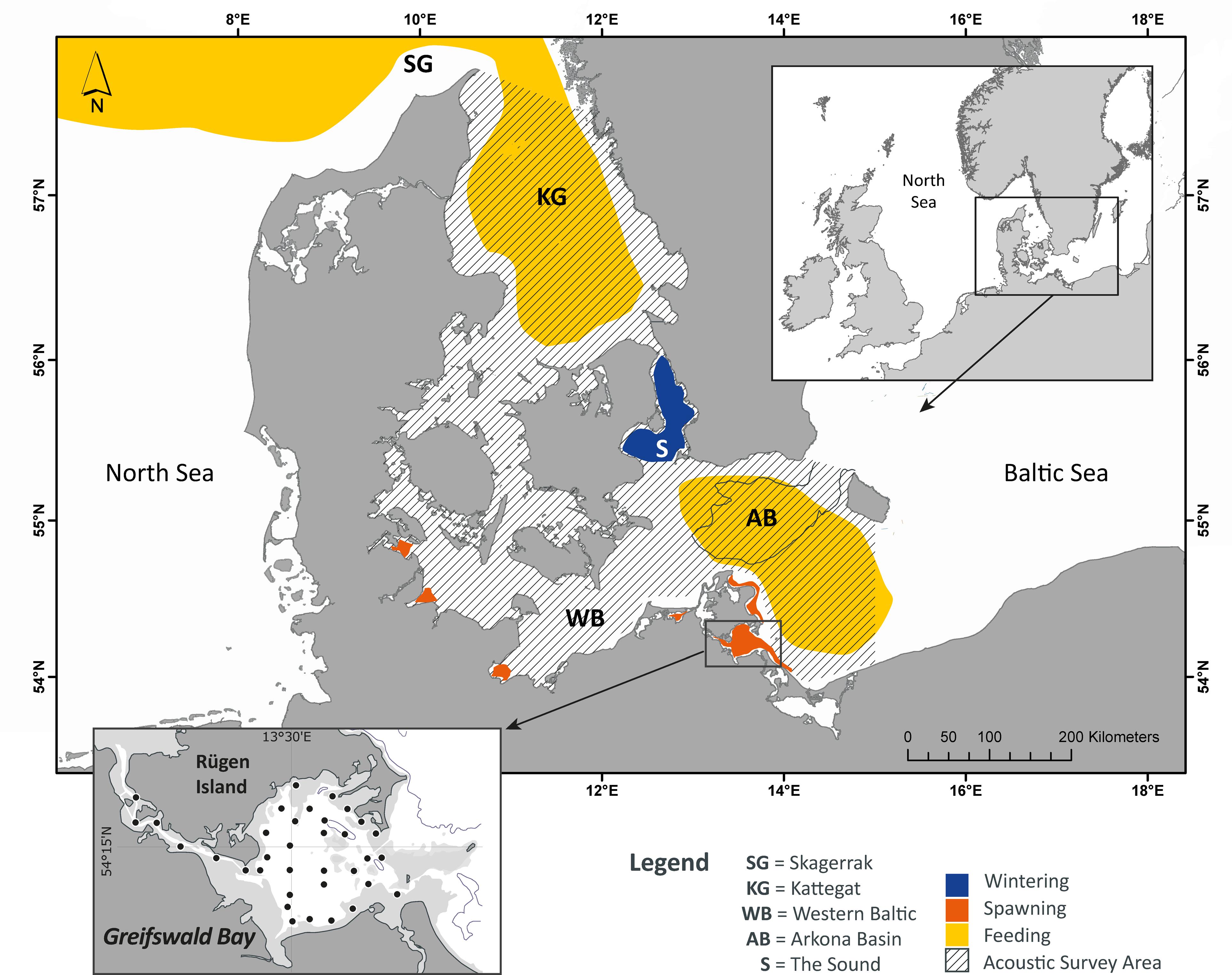
Figure 1. Study area, including the sampling area of the German acoustic survey (GERAS) in the western Baltic Sea (gray shading) and the sampling stations of the Rügen herring larvae survey (black dots) in Greifswald Bay. Spawning sites are not considered exclusive but represent the current state of knowledge.
Because the entire early herring life history, from spawning to advanced larval stages (post-flexion), can be investigated in Greifswald Bay, it represents an ideal model system for in situ studies of survival bottlenecks (Polte et al., 2014, 2017).
Investigating the Temperature Range for Initial Spawning
To determine if the onset of initial spawning is related to a particular temperature range (initial spawning range, ISR), we monitored defined transects located parallel to the southern shoreline of Greifswald Bay (Figure 1) and documented the first occurrence of eggs during four consecutive years. The general location of the spawning zone was surveyed by a towed video camera transmitting real-time images of the seabed and was assigned to three transects, representing different depth zones. Each transect consisted of six fixed sampling stations, stretching on a latitudinal axis (from west to east) of 125 m total length (distance between sampling stations: 25 m). Benthic eggs were systematically sampled with a van Veen grab (250–400 cm2). Each transect was sampled weekly from March to late May/early June in 2012–2015. If conditions did not allow for systematic sampling early in the season (e.g., due to extensive ice cover), a visual presence–absence control was conducted to make sure no spawning occurred before the transect sampling started. Due to harsh weather conditions no sampling could be conducted before March 18th in 2015. Herring eggs were counted from the grab samples and raised to mean egg concentration per square meter (Moll et al., 2018).
Early Life Stage Abundance Indices
Two fishery-independent proxies for herring reproductive success in the western Baltic Sea (ICES, 2018) were used in this analysis as response variables to determine the effects of the regional winter regime on western Baltic spring-spawning herring recruitment on different spatial scales.
(1) Larvae abundance: The “N20” larval herring index is an established metric of annual herring reproduction in the major nursery areas included as the index for juvenile year class strengths in the international stock assessment for the Western Baltic spring spawning herring since 2008 (ICES, 2018). The rationale for the index is based on the observation that herring larvae, and so their number, strongly correlate with later juvenile stages. Based on weekly ichthyoplankton samples taken over a 3-month period, the N20 larval herring index is an annual estimation of larvae reaching a total length of 20 mm, having survived major early life stage mortality. The abundance of this particular size class provided a solid estimate for the year-class strength of recruits (see Oeberst et al., 2009a,b for details). The N20 larvae index time-series dates back to 1992. Weekly ichthyoplankton surveys include a grid of 35 stations in Greifswald Bay sampled from mid-April to late June (1992–2006) and from March to June (2007–2017, Figure 1). Herring larvae were sampled using a Bongo net (diameter 60 cm, mesh size 335 μm) equipped with flowmeters (HYDRO-BIOS). In the laboratory, all herring larvae were counted and measured to the nearest millimeter total length. If the number of larvae in the samples exceeded 1000 individuals, a random subsample of 600 larvae was measured to receive a weekly length distribution.
(2) Juvenile abundance: The German autumn acoustic survey (GERAS) is part of an international program that provides annual information on stock size of small pelagic fish in the Baltic Sea coordinated by the International Council of Exploration of the Seas (ICES). The GERAS covered the western Baltic Sea from the inlets to the North Sea to the central Baltic basin of the Arkona Sea (Figure 1). Survey details are provided at http://www.ices.dk/community/groups/Pages/WGBIFS.aspx. The abundance index GERAS first winter ring (1-wr) includes 1-year-old juveniles, as defined by the 1-wr microscopically detected in the otolith, as a proxy for herring recruitment in the entire distribution area. The GERAS 1-wr index follows on the larval production of the above N20 larvae index with a 1-year lag phase of fish metamorphosis and growth. Accordingly, the time-series 1993–2017 was used to determine if effects of local winter regimes, acting on the scale of a major nursery area (N20 larvae index), can be tracked to the large scale of recruitment in the western Baltic Sea.
Timing of Larval Hatch
Weekly sampling of ichthyoplankton (see above) generally allowed a relatively exact determination of larval hatching periods. To determine if the temperature regime affected the timing of larval hatch, two particular response variables focused on the earliest larvae stages (yolk-sac larvae). Previous studies indicated that early stage larvae are retained inside the Bay (Bauer et al., 2013; Polte et al., 2017). Therefore, significant export of larvae by current regimes was considered negligible. According to literature on the temperature-dependent duration of yolk consumption (Klinkhardt, 1986) all larvae within a total length range of 5–9 mm were considered hatchlings in the yolk-sac stage (Polte et al., 2017; Dodson et al., 2019). For this study, seasonal shifts of (i) peak hatching and (ii) hatching duration were investigated, based on the occurrence of maximum abundance of yolk-sac larvae and the number of days where yolk-sac larvae could be found in the system. Two periods totaling 16 years with full seasonal coverage of in situ data (periods 1992–1996 and 2007–2017) were included in the analysis.
The potential effects of the timing of yearly hatching peaks and the entire hatching duration might have on larvae abundance (N20 index) were individually assessed for hatching peaks and hatch duration using generalized linear models (McCullagh and Nelder, 1989).
Where β0 is the model intercept, β1 is the coefficient associated to either hatching peak or hatching duration, and subindex i refers to each observation year. The N20 was related to the predictors by a log link function. The first modeling approach assumed a Poisson distribution of the residuals. In case of model overdispersion (Deviance>>>d.o.f), a negative binomial distribution was applied.
To determine the response of the larval index to seasonal timing of hatching peaks and the hatching duration, we focused on the annual photoperiod instead of calendar periods. In contrast to the calendar scale, the light conditions are evidently a major driver of Atlantic herring maturation processes (McPherson and Kjesbu, 2012). Accordingly, the study period was set between the autumn equinox of the year y-1 (September 22) and the first solstice of year y (June 21; Figure 2).
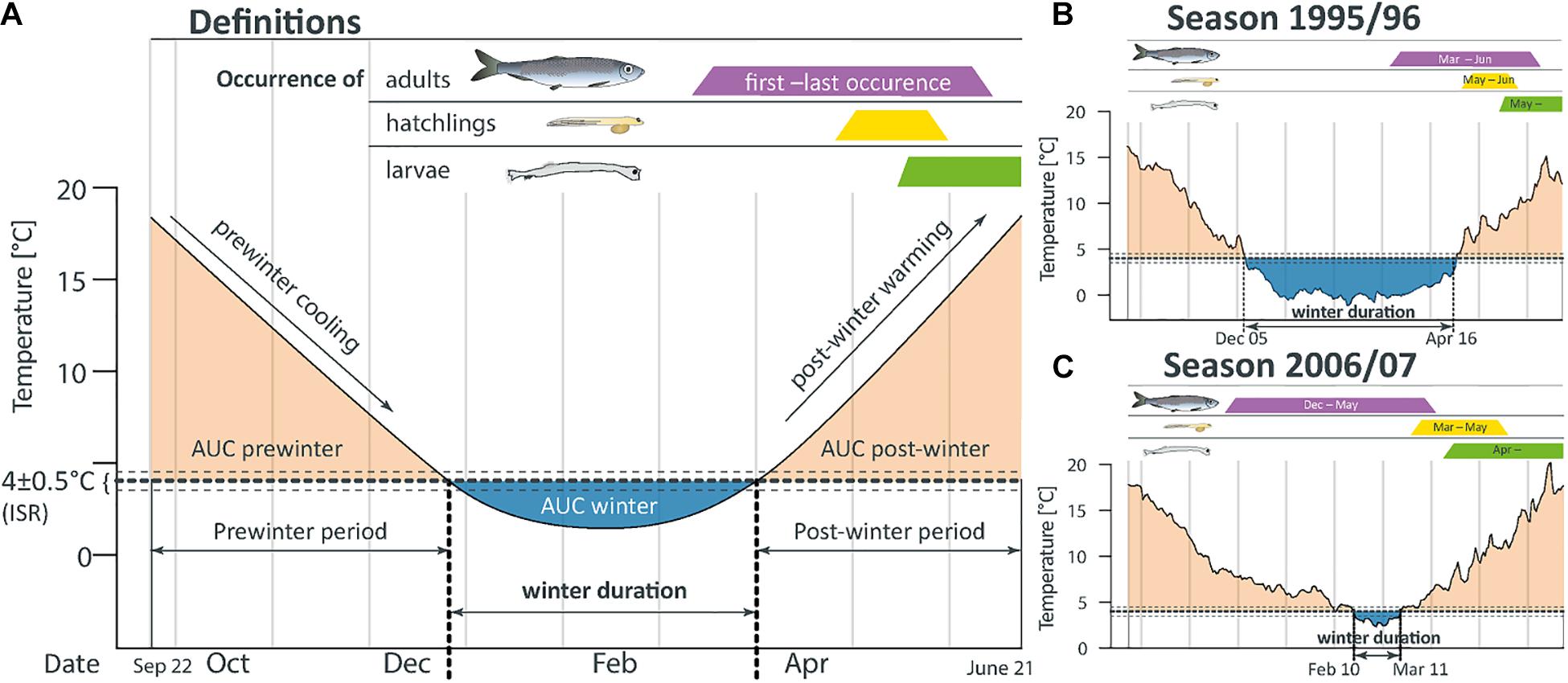
Figure 2. (A) Schematic of the best candidate winter descriptors identified by the model. Dashed horizon indicates the initial spawning range (ISR). AUC represents the area under the curve. Exact definitions of the descriptors are provided in Supplementary Table S1. The particular seasonal periods are assigned to herring life stages in the system. Two opposing extreme years with differing regional SST curves are presented in (B,C) to illustrate the application of the concept. The full set of SST curves for the 26 years studied is provided in Supplementary Figure S2.
Sea-Surface Temperature Descriptors
Average daily SST data were extracted from NOAA daily Optimum Interpolation Sea Surface Temperature with a spatial resolution of 0.25 × 0.25 degree and a temporal resolution from 1992 to 2017, available at https://www.esrl.noaa.gov/psd/data/gridded/data.noaa.oisst.v2. The original dataset was limited to those months potentially influencing herring migration, spawning, hatching, and larvae development (Polte et al., 2017). No in situ temperature data were available for the winter period previous to the beginning of the larval herring survey. Therefore, the used remote sensing data were calibrated against CTD measurements during the survey period (Supplementary Figure S1) and subsequently assessed suitable.
To characterize the annual variation of temperature regimes, we calculated several punctual descriptors within three different SST periods, defined as follows: The first period elapses from September 22, year y-1, until the day the SST falls into the in situ range for initial spawning ISR which was to be discovered as a result of this study (see above: egg sampling). The second period starts the day the SST consistently falls below the lower limit of the ISR for seven consecutive days, and ends on the day the SST consistently ascends above the lower limit of the ISR for seven consecutive days. Finally, the third period elapses from the end of the second period until June 21 from year y. These three periods are referred to hereafter as prewinter, winter, and post-winter, respectively (Figure 2). Annual SST descriptors used included the following: winter onset (number of days of the prewinter period), duration of the SST remaining within the ISR, winter duration, and the post-winter duration. Other descriptors extracted from each period are the mean SST and standard SST deviation, the area under the SST curve (AUC) relative to the ISR or the SST slopes in prewinter and post-winter. The AUCs were calculated for each season by applying numerical integration based on the composite trapezoid rule, and the SST slopes were calculated using the Sens’s method (Sen, 1968). The complete list of descriptors extracted from the SST curves can be found in Supplementary Table S1.
Selection of SST Predictors
The above SST descriptors were analyzed for their potential as predictors in regression models to determine the influence of temperature regimes in Greifswald Bay on the local level of the nursery (N20 larvae index) and the larger spatial scale of the western Baltic Sea (GERAS 1-wr index). To determine climate effects on reproduction timing, the duration of the hatching season and the day of the year (counting from September 22 from year y-1), when the hatching peak was observed, were included as response variables. The selection of SST descriptors to be used in the modeling section was conducted in two steps. First, an exploratory data analysis was conducted to identify significant pairwise linear correlation between the SST descriptors (Supplementary Table S1) and the biological responses. Based on this exploration, only those descriptors demonstrating significant linear correlation were initially considered as predictors to model a given response variable z. In a second step, we assessed potential problems of multi-collinearity among the descriptors selected in the first step. This assessment was conducted based on the procedures described in Hair et al. (2013). Predictors indicating a variance inflation factor (VIF) greater than a value of 4.5 were directly discarded, while predictors indicating values within the range 3–4.5 were subjected to a deeper assessment to decide if they should be included in the model matrix (Hair et al., 2013). The remaining descriptors after the above two-step selection process were used to establish a full matrix to model the annual abundance indices and hatching characteristics.
Modeling the Biological Response to Regional Winter Characteristics
The biological responses (N20 larvae index, GERAS 1-wr, hatching duration, and hatching peak) were modeled using generalized linear models (McCullagh and Nelder, 1989),
In Eq. 2, μi is the expected value of the biological response z at year i, g is a monotonic link function, Xi is a vector of predictors (added alone or interacting with other predictors), selected after the procedure described in the previous section, and β is a vector of unknown coefficients associated with Xi. The N20 larvae and GERAS 1-wr indices were modeled using the log link function. The two hatching variables were modeled assuming a Gaussian distribution of the residuals, and the link function was the response identity [g(μi) = μi].
The model matrix Xi in Eq. 2 included the intercept term and a vector of descriptors of length p. Considering the model in Eq. 2 as the full model, 2p different submodels could be formulated leaving out one or more descriptors at a time, all of them considered potential candidates to model the variation of the biological response z. The candidate models were automatically ranked by a decreasing value of AICc (Hurvich and Tsai, 1989), an Akaike Information Criterion (AIC) especially developed to avoid over-fitting when modeling data with small sampling sizes. The model with the lowest AICc was selected as the best candidate to describe variability of z.
Results
SST Range for Initial Spawning
In three consecutive years (2012–2014), a particular temperature range for initial spawning (ISR) could be identified at 3.5–4.5°C (Figure 3). In 2015 harsh weather conditions did not allow for sampling until March 18th when eggs were already found present on the initial sampling at 4.5°C. Although peak spawning in some years (e.g., 2012) occurred approximately a month later at an SST of >10°C, during the above ISR the first eggs of the season were discovered by the systematic herring-egg survey on the spawning beds. Therefore, we used the ISR (3.5–4.5°C) to define the three SST periods (prewinter, winter, and post-winter; Figure 2). Owing to the weekly sampling interval, the exact day of spawning could not always be determined. Regular controls on the spawning beds did not reveal any significant spawning underneath ice sheets or relevant spawning activity before the ISR was reached. The respective SST curves relative to the detected ISR for each of the investigated 26 years are provided in Supplementary Figure S2.
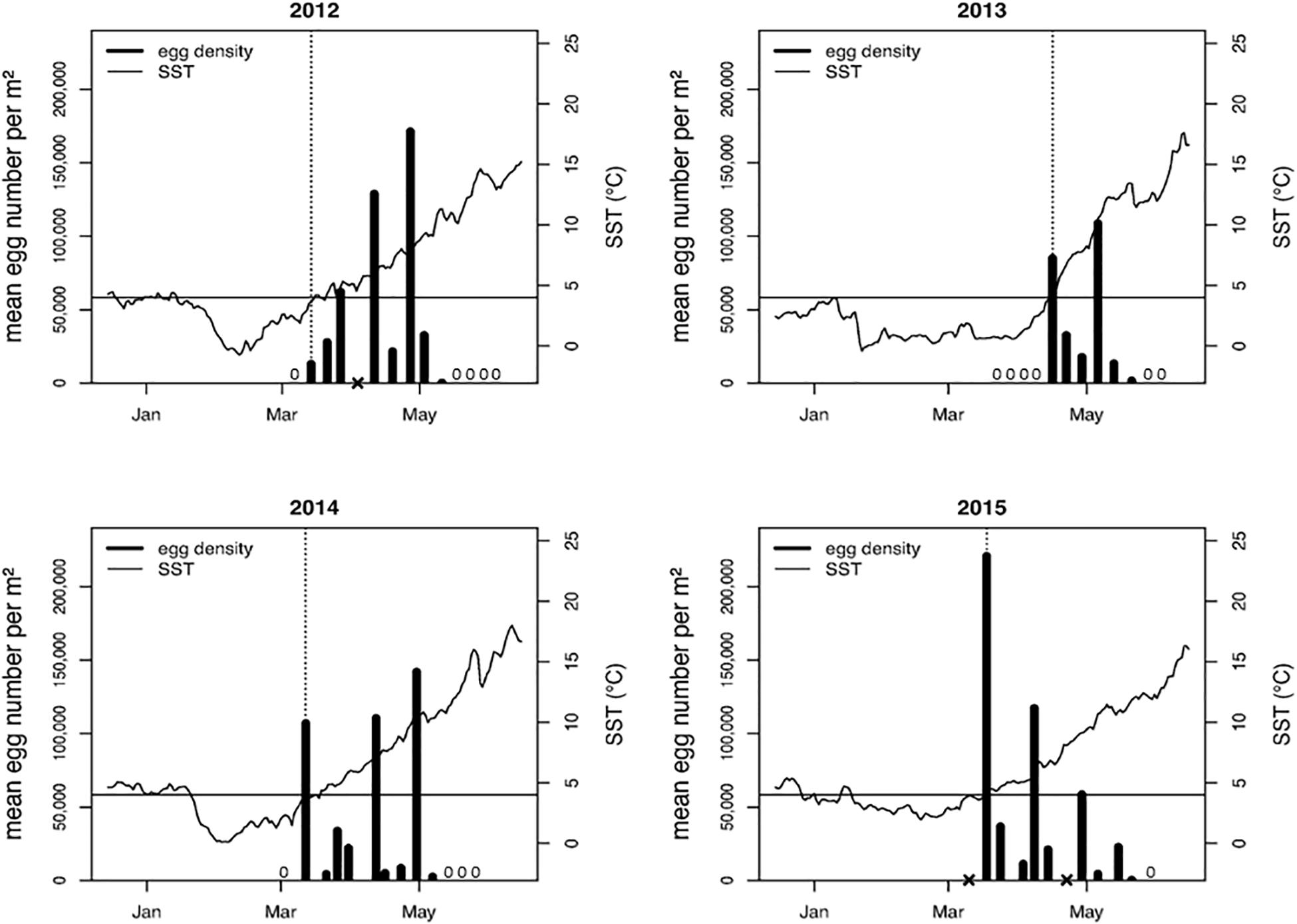
Figure 3. Mean number of herring eggs m–2 on a major spawning bed of ca. 1.5 m depth relative to SST (right y-axis) located in southern Greifswald Bay. Egg sampling was conducted weekly throughout four spawning seasons. A presence-absence sampling revealed no spawning activity prior to initial spawning (indicated by “zero”). The black line indicates the 4°C literature value for initial spawning. Initial spawning was observed in 2012 at 3.6°C, 2013 at 4.3°C and 2014 at 3.7°C. A cross on the x-axis indicates missing data. In these particular weeks, sampling could not be implemented due to harsh weather conditions. First egg sampling in 2015 was conducted on 18th March at 4.5°C.
Impact of Larval Hatch Timing on Reproductive Success
During the two periods (1992–1996 and 2007–2017) including data of the entire hatching period, the applied model assuming negative binomial distribution of the residuals, revealed a significant positive correlation between the N20 larvae index (Figure 4A) and the day in the photoperiod (September 22–June 21) where peak hatching occurred (R2 = 0.65, p < 0.001). This indicates a strong negative effect of early hatching on the reproductive success.
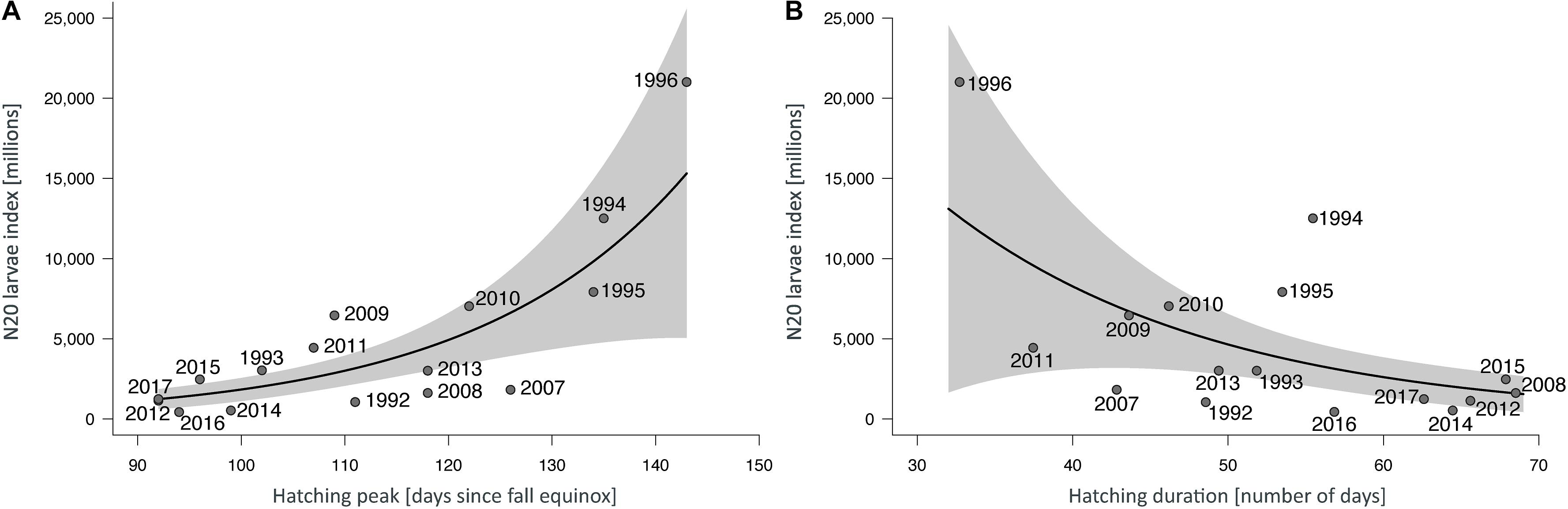
Figure 4. (A) Predicted effect of the date of the hatching peak in the period from the autumn equinox (9/22) to the first solstice (6/21) on larval herring production (N20 larvae index), and (B) predicted effect of the hatching duration in the same period on larval herring production (N20 larvae index). Points represent the annual estimates of the variables related to the empirical value of the post-winter descriptors. Shaded areas represent 95% confidence intervals.
Furthermore, a significant trend indicated that hatching peaks occurred earlier along the time-series (R2 = 0.38, p = 0.01). A direct comparison of the two investigation periods revealed a mean hatching peak at day 125 (SD ± 17.5) after the autumn equinox for the period 1992–1996, and day 106.6 (SD ± 12.8) for the past decade (2007–2017), indicating a mean of approximately 2.5 weeks of earlier occurrence. An analysis focusing on the past decade only resulted in a more pronounced significant trend toward earlier hatching (R2 = 0.64, p = 0.003) because of reduced inter-annual variability.
An extended hatching duration (Figure 4B) resulted in a significantly reduced N20 larvae index (R2 = 0.38, p = 0.002). During the two periods (1992–1996 and 2007–2017) no significant trend indicated that hatching duration became significantly extended over time (R2 = 0.14, p = 0.16). A direct comparison of the periods revealed a mean hatching duration of 48.4 days (SD ± 9.1) for the period 1992–1996, and 55.0 (SD ± 11.4) for the past decade (2007–2017), indicating an average extension of hatching duration of approximately 1 week.
Inspection of residuals obtained from time-trend analyses of hatching peaks and hatching duration revealed no significant auto-correlation across years.
Response of Hatching Phenology to Particular Winter Descriptors
The model results indicate that an increase in the area below the seasonal temperature slope (AUC-post-winter), as the integral of slope progression and the period in days until midsummer, indicate that steeper post-winter temperature curves in combination with shorter post-winter duration resulted in earlier hatching peaks (Table 1 and Figure 5A). The AUC-post-winter was found a significant (p < 0.034) descriptor of the seasonal setting of hatching peaks (Table 2).
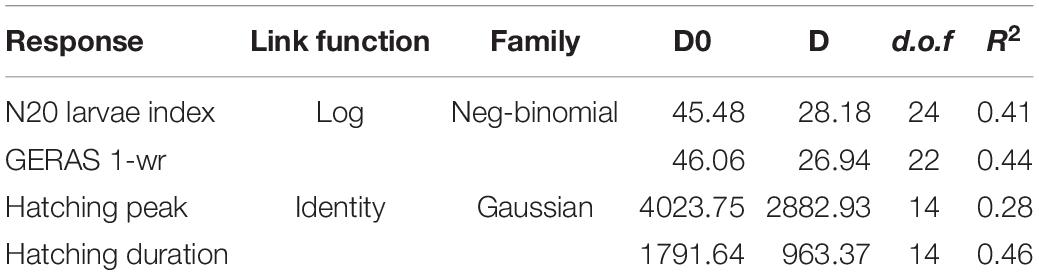
Table 1. Fit statistics of the best candidate models for the N20 larvae index, GERAS 1-wr, hatching peak, and hatching duration.
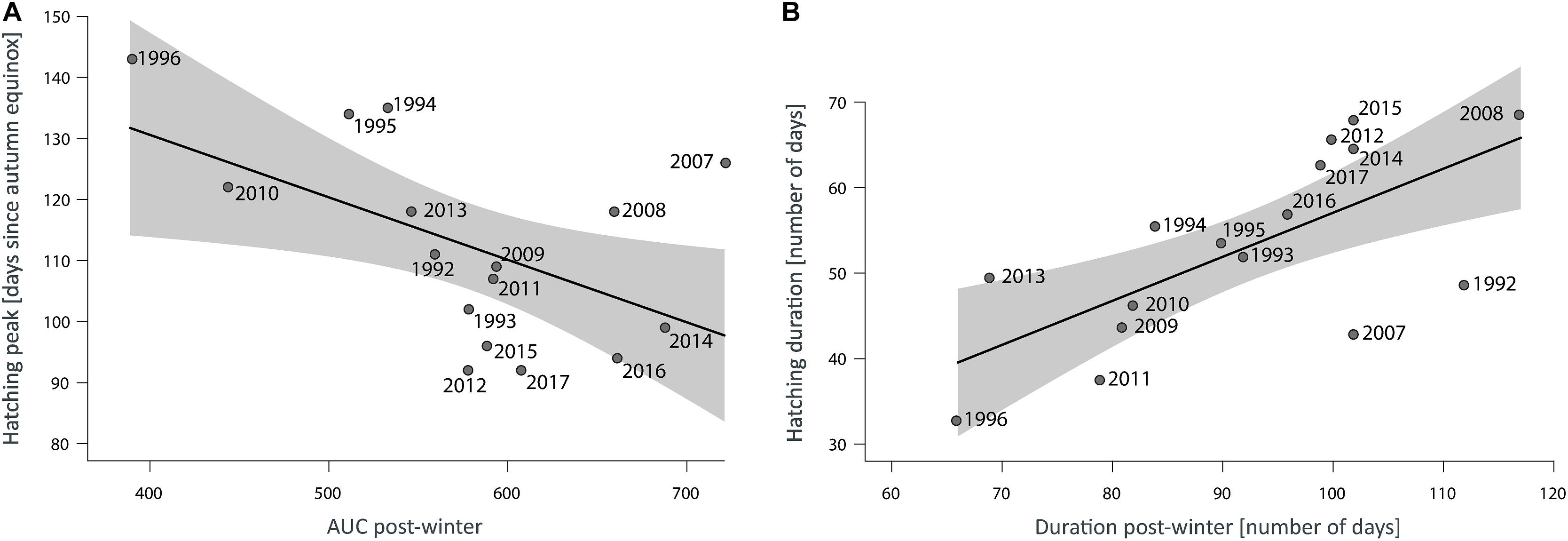
Figure 5. Predicted linear relationships (lines) and associated 95% CI (shadows) by the best candidate models on hatching responses. (A) Effect of AUC in the period after the increasing temperature curve crossed the initial spawning threshold range (post-winter) in the period from the autumn (9/22) equinox to the first solstice (6/21) when the major abundance of hatchlings (yolk-sac larvae) occurred. (B) Effect of the post-winter duration on the duration of the hatching period in days. Points represent the yearly estimates of the variables related to empirical value of the post-winter descriptors.
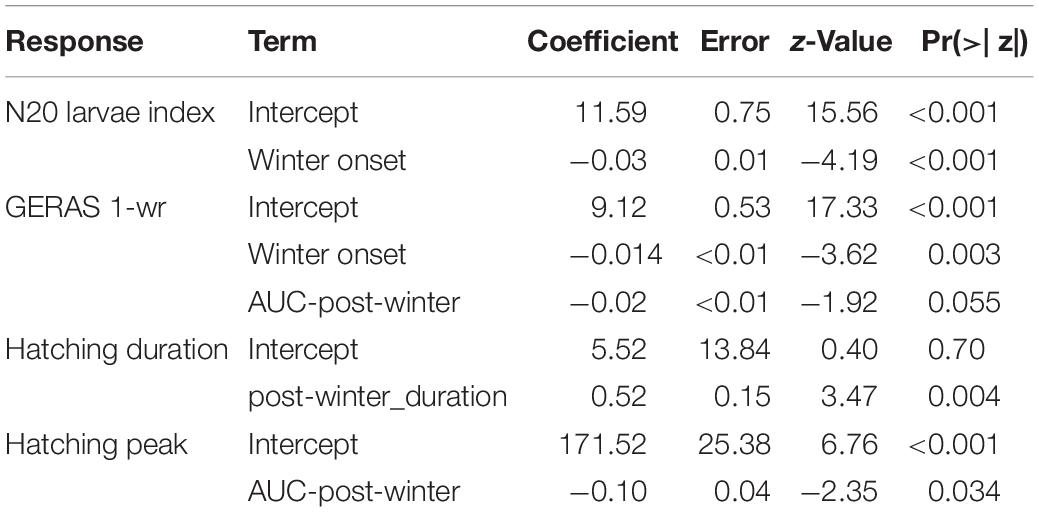
Table 2. Summary of terms from the best candidate models for the N20 larvae index, GERAS 1-wr index, hatching duration, and hatching peak.
The seasonal duration of the hatching period was found positively affected by the post-winter duration (R2 = 0.46, p < 0.004) (Table 2 and Figure 5B).
This demonstrated that the early onset of the post-winter period (i.e., an early ending of winter) resulted in an extension of the seasonal duration of hatching.
Response of Herring Productivity to Particular Winter Descriptors
Winter onset and winter duration both had a strong linear relationship with the N20 larvae index (Supplementary Figure S3). However, both descriptors were found highly correlated (R2 = 0.87; Supplementary Figure S4). Therefore, to avoid problems with co-linearity of predictors, only winter onset was used in the subsequent modeling approach. After selection by the exploratory data analysis, the remaining descriptors were used to establish the following full model:
N20 ∼ exp[winter onset∗(AUC-prewinter + AUC-post-winter + prewinter slope)] (3)
Equation 3 includes four main effects related to winter onset, AUC-prewinter, AUC-post-winter, prewinter slope, and the first-order interaction between winter onset and the remaining three descriptors included in the model. Therefore, the initial full model included a total of p = 7 predictors terms in addition to the model’s intercept. The initial model assuming Poisson distribution of the residuals, suffered from large overdispersion. Therefore, a negative binomial modeling was finally applied. Based on the full model, 27 = 128 different candidate submodels were successfully estimated and ranked by decreasing AICc (Supplementary Table S2). The best candidate model resulted in R2 = 0.41 (Table 1) and included only winter onset as SST predictor (p < 0.001; Table 2). A diagnosis of the residuals of the best candidate model is provided as Supplementary Figure S5.
The curve predicted by the N20 model reveals that a late seasonal drop of SST below 3.5°C (delay in winter onset) led to an average reduction in recruitment (Figure 6A) as derived by larval herring productivity and abundance of 1-year-old juveniles 1 year later. The back transformation of the model coefficients (Table 2) revealed an expected decrease of ∼3% (95% IC = 1.6–4.3%) in the N20 larvae index for each 1-day of delay in winter onset.
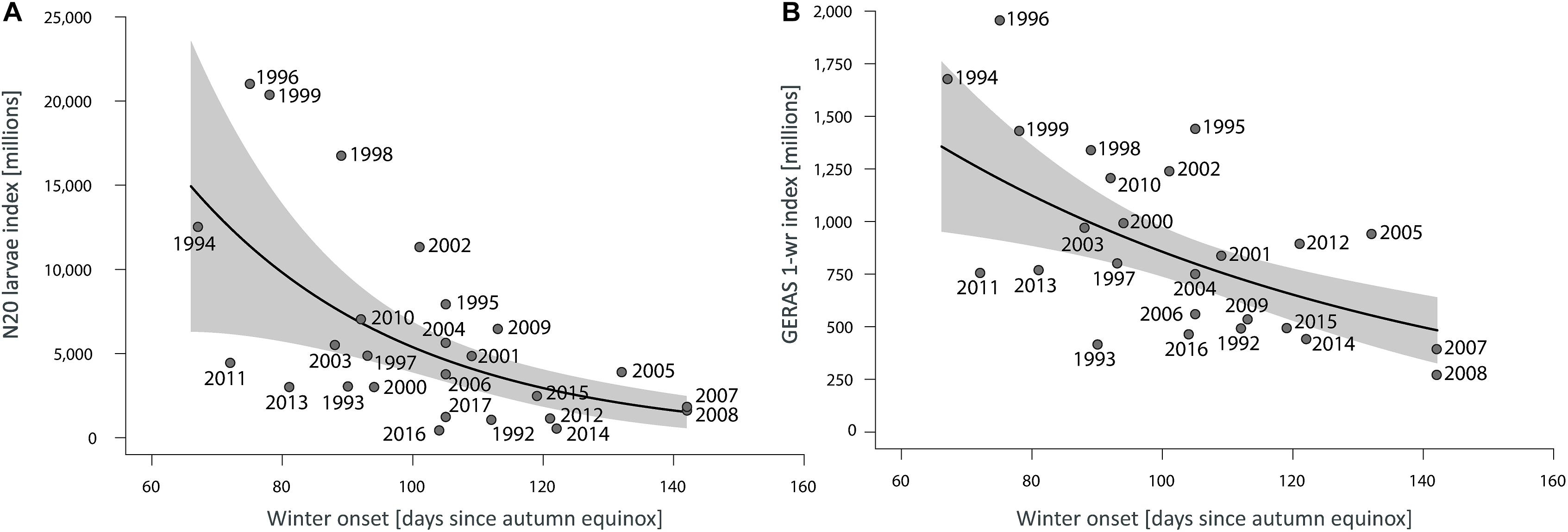
Figure 6. Predicted effect of winter onset (lines) and associated 95% CI (shadows) by the best candidate models on (A) the N20 larvae index in the nursery area, and (B) abundance of 1-year-old juveniles (GERAS 1-wr) in the western Baltic Sea. Points represent the annual estimates of the indices related to empirical values of the winter onset descriptor. In (B) the index is lagged 1 year in relation to the descriptor.
Respectively, winter onset as SST predictor for the 1-year juveniles in the outer Western Baltic Sea, GERAS 1-wr (Supplementary Table S3) resulted in R2 = 0.44 (Table 1).
The GERAS 1-wr model predicted an expected decrease of ∼1.3% (0.6–2.0%) in the abundance index for each 1-day delay in winter onset (Figure 6B).
Discussion
Earlier studies reveal a significant influence on variations in large-scale climate regimes on the reproductive success of fishes, particularly on species that spawn all at once in a single sequence determined by a particular season, such as spring-spawning herring in the Baltic Sea (Cardinale et al., 2009; Gröger et al., 2014). However, the ecological mechanisms responsible for this relationship among global climate indices and local fish recruitment largely remained subject of speculation. In general, our results now demonstrate that fish reproductive success in temperate coastal zones is subject to particular regional characteristics of seasonal temperature regimes relative to species- or population-specific temperature thresholds for reproduction phenology.
During three consecutive years with differing winter conditions, we found a consistent 4°C temperature threshold (±0.5°C) for initial spawning. The fourth year 2015 is also indicative for this pattern but eggs were already found at the initial inspection after storms and harsh weather conditions finally allowed for sampling conducted by grab samples from small boats. Our observations on the initial egg occurrence are in line with anecdotal observations from the past (Klinkhardt, 1996). Additionally, we found no evidence of herring spawning underneath a closed ice sheet in the bay as has often been anecdotally assumed. The identification of temperature-based spawning thresholds in our study is an important finding that might generally occur in other herring populations, although the precise temperature range might differ between populations according to latitude and season of spawning (Sinclair and Tremblay, 1984). This study demonstrates that such phenological thresholds, when identified, provide a suitable baseline for the analysis of climate-induced response in recruitment success. In the open ocean, a response of fishes to climate change is generally related to future ocean warming scenarios (e.g., Bindoff et al., 2019; Dahlke et al., 2020). In contrast this study shows that fish reproduction in shallow coastal systems is already affected for more than a decade by changing regional winter regimes.
The quick response of such systems to fluctuating air temperatures and the corresponding SST variability adds much complexity to an approach to identify climate effects as the response of biota does not necessarily manifest as a linear correlation to a gradual increase of average ocean temperatures during a relatively limited time series of about three decades. In our approach, different periods during winter and spring temperature gradients were selected, covering important stages in the reproduction process and bottlenecks in the survival of early herring life stages.
Dissecting the SST curve along the light period from autumn equinox to first solstice into multiple variables inevitably resulted in a high level of co-linearity. In the process of selecting the descriptor variable for the model, co-linear variables were filtered out by keeping the ones with the highest correlation coefficient. This way, e.g., “winter onset,” was selected and “winter duration” abandoned, because those variables are strongly co-linear. However, a late winter onset and a short winter duration have an equally negative effect on herring reproductive success. Our application of the ISR to define not only the winter ending but also the winter onset might appear somewhat arbitrary as a technical requirement of the winter descriptor model used. However, there is an indication that herring migration can indeed be initiated by temperature thresholds in the range 3–4°C (Jakobsson, 1969 and citations therein). Additionally, in the fishery data we observe an early (premature) arrival of herring in the area, where they aggregate before spawning. A late winter onset is therefore considered to affect the spawning phenology of the adult fish. This seems to be in contrast to the maturation phenology as it is known that spawn timing is determined early in the annual maturation cycle (Slotte et al., 2000; Neuheimer and MacKenzie, 2014). However, Western Baltic spring spawning herring aggregate at the entrance to the spawning area in a pre-spawning maturity stage (stage 4, maturity scales Heincke, 1898; Bowers and Holliday, 1961). In this stage fish are caught by the industrial trawl net fishery in the Pommeranian Bay. When the fish finally enter the spawning area they reach maturity stages 5/6 indicating full spawning condition and this final process seem to follow the investigated temperature threshold. If the threshold is not reached in a certain time period, the amount of females with harmful levels of atresia is increasing (unpublished observation) resulting from ovaries full of eggs that cannot either be spawned or resorbed (Ojaveer et al., 2015).
From this, relative indication for the seasonal timing of the initial spawning process of western Baltic spring-spawning herring can be derived by the annual onset of the trawl net fishery on pre-spawning areas, because this fishery targets aggregations of the initial cohort of spawning fish in particular. Although the size of the fleet and number of trips per vessel remained unchanged, the fish biomass retrieved by the trawl fishery as early as January increased from 135 metric tons (2% of the total annual catch) in 2002 to 1500 metric tons (15% of the total annual catch) in 2017 (Supplementary Figure S6). Since 2012, January landings have increased sharply by >100% of the previous amount. As the gillnet fishery targets fish in full spawning condition, an earlier start of the gillnet fishery indirectly implies an earlier maturation of fish during the past decade. Similar to the shift in timing in the trawl net fishery, the amount taken early in the season (i.e., January) has increased, particularly during the two most recent years, 2016 and 2017 (Supplementary Figure S7).
The winter onset explained a significant amount of the variability of larval herring production in the major nursery area. This was reflected to the same degree in the abundance of 1-year-old juveniles on the larger spatial scale of the entire western Baltic Sea. The distribution of the years in the model result implicitly indicate that those relatively mild winter periods with a late winter onset occurred more frequently during the past decade. Recent estimates of the effect of climate change on the Baltic Sea identify an annual warming trend of 0.08°C per decade, which is observed as a decrease in the number of very cold days during winter (HELCOM, 2013).
Although the 27 years herring larvae time-series did not (yet) reveal a significant linear trend of shorter winters, our results demonstrate that each day of winter delay resulted in a 3% reduction in the N20 larvae index and 1.3% of GERAS 1-wr, respectively.
In contrast to observations of different fish species and ecosystems (Rogers and Dougherty, 2019), for herring in coastal spawning grounds, the occurrence of larval hatching peaks must not necessarily reflect the timing of spawning. For example, if early spawning resulted in increased egg mortality of initial cohorts, then a potential appearance of later hatching peaks produced by later cohorts would not reflect this phenological shift. This might explain why the timing of hatching peaks was affected by the predictor “AUC-post-winter” (summarizing: onset, duration, and progression of the warming SST curve after crossing ISR until the first solstice in summer). As spawning and the entire embryonic development are subjected to this season descriptor, hatch timing is probably a result of the combination of spawn timing and egg survival.
Earlier hatching was negatively correlated with larval production (N20 larvae index) indicating that a reduction in recruitment is mechanistically related to a phenological shift, potentially causing a temporal mismatch with adequate plankton prey. Testing the match-mismatch hypothesis (Cushing, 1969; Durant et al., 2007) for herring larvae in Greifswald Bay must remain subject of future research but preliminary results indicate a lack of suitable zooplankton prey during the larval herring first feeding periods since 2013 (L. Livdâne, unpublished).
This study found the annual duration of larval hatching slightly extended with negative consequences for reproductive success. The negative effect of an elongated hatching duration is somewhat counterintuitive, because theoretically a spread of hatching over a longer period should result in increased potential to meet favorable conditions for growth and survival. This phenomenon potentially compensates for the disadvantages of premature hatching of the initial cohort by increasing the chances of hatchlings meeting favorable environmental conditions. Indeed, an increased contribution of larvae from later cohorts to overall production was observed in recent years but this occurred simultaneously with decreasing reproductive success (Polte et al., 2014). However, the extension of the hatching duration is the result of an earlier reproduction phase. In a scenario where initial hatchlings would starve as a consequence, the survivors would recruit from later cohorts, but overall productivity would potentially decrease. Rogers and Dougherty (2019) pointed out that, although the spawning of walleye pollock (Gadus chalcogrammus) in Alaskan waters shifted toward earlier spawning, the effects of timing on reproduction might be mitigated by fish demography. This refers to the situation where consecutive spawning is structured by multiple cohorts differing in size and age. Similarly, Baltic Sea spring-spawning herring enters the spawning grounds in successive cohorts where the initial cohort is represented by the largest, very fecund animals. However, it is not entirely clear if those size differences de facto represent different age groups or if they are caused by cohorts with differing growth patterns. A potential advantage for later larvae cohorts is the chance to encounter better feeding conditions as they hatch closer to the peak of the spring plankton bloom and are experiencing elongated daylight periods promoting their success in plankton feeding (Hufnagl and Peck, 2011). However, under current scenarios of steeper spring temperature gradients (Schwartz et al., 2006), later cohorts will be increasingly exposed to physiological thermal limits (Moyano et al., 2020). Additionally, we see high spring temperatures acting in synergy with high eutrophication levels on the littoral spawning beds. This synergy is already manifest in complex ecosystem cascades. A strong example is the retreat of aquatic plants, a major herring-spawning substratum, to a vertical depth limit of 3.5 m (Munkes, 2005; Kanstinger et al., 2018). As a result, herring eggs are extremely exposed to storm-induced wave action, and increasing storm frequencies greatly affect egg mortality (Moll et al., 2018). Furthermore, some filamentous, epiphytic brown algae, such as Pilayella littoralis, can cause drastic egg mortality on herring-spawning beds (Aneer, 1987; von Nordheim et al., 2020). The sum of the direct and indirect stressors outlined above, together with additional top–down effects, especially by egg predation (Kotterba et al., 2014, 2017), demonstrates the diverse suite of environmental factors affecting herring reproductive success in coastal systems. Therefore, we consider the amount of 40% of the variability in larval production explained by the “winter onset” as a very strong effect. The impact is even intensified because it is transported to the population level as expressed by a similar effect on the 1-year-old juveniles on the large spatial scale.
Considering the high plasticity of Atlantic herring-spawning modes and- seasons, the universality of our findings on the species level might be limited. However, the core of the subject – underlining the present impact of local climate regimes on coastal productivity and economy – is underlined by the precedence we presented.
The low spawning stock biomass of herring in the western Baltic Sea is considered a consequence of reduced recruitment then vice versa since ca. 2002 (ICES, 2019). In fact, the SSB decreased drastically toward the mid-1990s before international management of the fishery was instituted (ICES, 2018). After a certain transition period from 2000 to 2003, a particular period of low recruitment (<25% quantile of the time-series means) can be defined starting in 2004 and persisting until today. Today it can only be speculated that the fishery pressure in the past led to reduced resilience and reproductive capacity of the population. The exact portion of the overall spawning stock biomass (SSB) entering the studied spawning ground cannot be estimated as the larval size class used for the N20 index is not suitable as a regional SSB index and the drivers for egg mortality as outlined above are quite significant in the system. However, the N20 index is used as important metric for the year class strength of recruits to the entire stock (Oeberst et al., 2009a,b; ICES, 2019) but shows no relation with the respective SSB of Western Baltic spring spawning herring. Similar is the case for the GERAS 1-wr index. Since about 2002 the herring population dynamics in the Western Baltic Sea is considered to be driven by low recruitment instead of decreasing spawning stock biomass (ICES, 2019). Although a decreasing SSB as alternative hypothesis for the general decrease of recruitment cannot be entirely excluded, we do not consider this to affect the results of this study. The observed winter effects can explain a significant part of year-class variability in juvenile herring in times with high SSB in the 1990s. In the framework of analyzing climate effects on herring reproduction, SSB could have introduced spurious results to our approach only if SSB would also correlate with the selected winter descriptors. Therefore, potential correlations between SSB and winter onset were evaluated, revealing no indication that the status of herring adult biomass is driving the N20 patterns predicted as a function of winter onset (Supplementary Figure S8). This underlines the current understanding that unfavorable environmental conditions such as those outlined in this study currently prevent recovery to strong year classes of recruits. The current situation, however, might not reflect to what extent the drastic decrease of SSB in the past potentially affected fish demography in a way contributing to the observed climate effects on reproductive success. It is documented for multiple fish populations that fecundity, egg size and – number as well as additional maturity parameters are positively related to fish size and maternal age and therefore larger fish have a greater reproductive potential (e.g., Murawski et al., 2001; Berkeley et al., 2004; Carbonara et al., 2015). In addition to the climate drivers outlined in this study, future studies should address the question whether decades of fishery pressure on the larger fish forming the first, seasonal spawning cohorts could have decreased the fitness of herring larvae in the system and to what degree a potential demographic shift in SSB affect the resilience of the population.
Climate-induced changes in the species’ phenology, including the seasonal timing of reproduction, might pose a present threat to biodiversity and sustainability of natural resources. Therefore, the mechanisms and consequences should become the focus of research. Although we cannot reverse current climate-change processes in the short term, directed coastal-zone management could mitigate the synergistic effects of shifting seasons with coastal eutrophication. To reduce the pressure on fish early life stages and maintain the functioning of nursery areas, eutrophication of coastal waters should be further restricted, and harvesting of affected fauna should be adjusted carefully under close observation of recruitment success.
Data Availability Statement
The datasets presented in this study can be found in online repositories. The names of the repository/repositories and accession number(s) can be found below: The data sets on abundance of herring larvae analyzed during the current study are available in the ICES Eggs & Larvae database available at http://www.ices.dk/marine-data/data-portals/Pages/Eggs-and-larvae.aspx. The data on the N20 larvae index, as well as the data on SSB, are available at http://www.ices.dk/community/groups/Pages/HAWG.aspx. The datasets on the GERAS 1-wr index analyzed during the current study are available at http://www.ices.dk/community/groups/Pages/WGBIFS.aspx. The data sets on fishery landings are compiled by the German Federal Office for Agriculture and Food and are available from the corresponding author upon reasonable request. The data sets on herring-egg densities on the spawning beds, as well as abundance data on the yolk-sac larvae generated in the current study, are available from the corresponding author upon reasonable request.
Ethics Statement
Ethical review and approval was not required for the animal study because all methods were carried out in accordance with relevant guidelines and regulations. All field samplings of fish were conducted under current licenses for wild fish sampling and approved by the relevant institutions (Mecklenburg-Western Pomeranian, Germany fishery law, §11 LFischG, Landesamt für Landwirtschaft, Lebensmittelsicherheit und Fischerei, and Mecklenburg- West Pomeranian).
Author Contributions
PP and PK designed and performed research. DM contributed data on herring-egg sampling. DM and LN analyzed data, participated in conceptualizing the study, and revised the manuscript. JS developed and performed the modeling approach. TG contributed data and analyses of juvenile herring sampling. PR-T and YZ analyzed the data and contributed to the data modeling. CZ contributed with conceptual development and design of graphics. PP and JS wrote the manuscript. All authors contributed to the article and approved the submitted version.
Funding
Funding for this work was provided by the EU-Data Collection Framework. Additional funding was received by DM from the project balt_ADAPT (03F0863D), funded by the Federal Ministry of Education and Research of Germany (BMBF). LN received funds from the German Federal Environmental Foundation (DBU).
Conflict of Interest
The authors declare that the research was conducted in the absence of any commercial or financial relationships that could be construed as a potential conflict of interest.
Acknowledgments
The authors thank the laboratory of the Thünen Institute of Baltic Sea Fisheries, in particular Dagmar Stephan, and Anne Georgi and Ina Hennings. Thanks are extended to Annemarie Schütz and Nakula Plantener for technical support with graphics and databases. The authors thank the crew of the research vessel FRV CLUPEA. The authors wish to thank the reviewers and the handling editor for their valuable comments on the manuscript. High Resolution SST data were provided by the NOAA/OAR/ESRL PSD, Boulder, CO, United States, from their website at https://www.esrl.noaa.gov/psd/. The content of this manuscript has been published as part of the thesis of von Nordheim (2019).
Supplementary Material
The Supplementary Material for this article can be found online at: https://www.frontiersin.org/articles/10.3389/fmars.2021.589242/full#supplementary-material
References
Aneer, G. (1987). High natural mortality of Baltic herring (Clupea harengus) eggs caused by algal exudates? Mar. Biol. 94, 163–169. doi: 10.1007/BF00392928
Bates, A. E., Helmuth, B., Burrows, M. T., Duncan, M. I., Garrabou, J., Guy-Haim, T., et al. (2018). Biologists ignore ocean weather at their peril. Nature 560, 299–301. doi: 10.1038/d41586-018-05869-5
Bauer, R. K., Stepputtis, D., Gräwe, U., Zimmermann, C., and Hammer, C. (2013). Wind-induced variability in coastal larval retention areas: a case study on Western Baltic spring-spawning herring. Fish. Oceanogr. 22, 388–399. doi: 10.1111/fog.12029
Beck, M. W., Heck, K. L., Able, K. W., Childers, D. L., Eggleston, D. B., Gillanders, B. M., et al. (2001). The identification, conservation, and management of estuarine and marine nurseries for fish and invertebrates. BioScience 51, 633–641. doi: 10.1641/0006-3568(2001)051[0633:ticamo]2.0.co;2
Bekkevold, D., André, C., Dahlgren, T. G., Clausen, L. A. W., Torstensen, E., Mosegaard, H., et al. (2005). Environmental correlates of population differentiation in atlantic herring. Evolution 59, 2656–2668. doi: 10.1111/j.0014-3820.2005.tb00977.x
Berkeley, S. A., Chapman, C., and Sogard, S. M. (2004). Maternal age as a determinant of larval growth and survival in a marine fish, Sebastes melanops. Ecology 85, 1258–1264. doi: 10.1890/03-0706
Biester, E. (1989). The distribution of spring-spawning herring larvae in coastal waters of the German Democratic Republic. Rapp. Réun. Cons. Int. Explor. Mer. 190, 109–112.
Bindoff, N. L., Cheung, W. W. L., Kairo, J. G., Arístegui, J., Guinder, V. A., Hallberg, R., et al. (2019). “Changing ocean, marine ecosystems, and dependent communities,” in IPCC Special Report on the Ocean and Cryosphere in a Changing Climate, eds H.-O. Pörtner, D. C. Roberts, V. Masson-Delmotte, P. Zhai, M. Tignor, E. Poloczanska, et al. (Geneva: IPCC).
Bowers, A. B., and Holliday, F. G. T. (1961). Histological changes in the gonad associated with the reproductive cycle of the herring (Clupea harengus L.). Marine Research Department of Agriculture and Fisheries for Scotland. Mar. Res. 5, 1–16.
Carbonara, P., Intini, S., Modugno, E., Maradonna, F., Spedicato, M. T., Lembo, G., et al. (2015). Reproductive biology characteristics of red mullet (Mullus barbatus L., 1758) in Southern Adriatic Sea and management implications. Aquat. Living Resour. 28, 21–31. doi: 10.1051/alr/2015005
Cardinale, M., Möllmann, C., Bartolino, V., Casini, M., Kornilovs, G., Raid, T., et al. (2009). Effect of environmental variability and spawner characteristics on the recruitment of Baltic herring Clupea harengus populations. Mar. Ecol. Prog. Ser. 388, 221–234. doi: 10.3354/meps08125
Catalán, I. A., Auch, D., Kamermans, P., Morales-Nin, B., Angelopoulos, N. V., Reglero, P., et al. (2019). Critically examining the knowledge base required to mechanistically project climate impacts: A case study of Europe’s fish and shellfish. Fish Fish. 20, 501–517. doi: 10.1111/faf.12359
Cloern, J. E., Foster, S., and Kleckner, A. E. (2014). Phytoplankton primary production in the world’s estuarine-coastal ecosystems. Biogeosciences 11, 2477–2501. doi: 10.5194/bg-11-2477-2014
Cushing, D. H. (1969). The regularity of the spawning season of some fishes. ICES J. Mar. Sci. 33, 81–92. doi: 10.1093/icesjms/33.1.81
Dahlke, F. T., Wohlrab, S., Butzin, M., and Pörtner, H.-O. (2020). Thermal bottlenecks in the life cycle define climate vulnerability of fish. Science 369, 65–70. doi: 10.1126/science.aaz3658
Dodson, J. J., Daigle, G., Hammer, C., Polte, P., Kotterba, P., Winkler, G., et al. (2019). Environmental determinants of larval herring (Clupea harengus) abundance and distribution in the western Baltic Sea. Limnol. Oceanogr. 64, 317–329. doi: 10.1002/lno.11042
Durant, J. M., Hjermann, D. Ø, Ottersen, G., and Stenseth, N. C. (2007). Climate and the match or mismatch between predator requirements and resource availability. Clim. Res. 33, 271–283. doi: 10.3354/cr033271
Edwards, M., and Richardson, A. J. (2004). Impact of climate change on marine pelagic phenology and trophic mismatch. Nature 430, 881–884. doi: 10.1038/nature02808
Gröger, J. P., Hinrichsen, H.-H., and Polte, P. (2014). Broad-scale climate influences on spring-spawning herring (Clupea harengus. L.) recruitment in the Western Baltic Sea. PLoS One 9:e87525. doi: 10.1371/journal.pone.0087525
Hair, J. F., Black, W. C., Babin, B. J., and Anderson, R. E. (2013). Multivariate Data Analysis, 7th Edn. Essex: Pearson Education Limited.
Heincke, F. (1898). “Naturgeschichte des Herings. Teil 1: die Lokalformen und die Wanderungen des Herings in den europäischen Meeren,” in Abhandlungen des Deutschen Seefischereivereins (Berlin: O. Salle).
HELCOM (2013). “Climate change in the baltic sea area: HELCOM thematic assessment in 2013,” in : Baltic Sea Environment Proceedings. No. 137, (Helsinki: HELCOM).
Hjort, J. (1914). Fluctuations in the great fisheries of Northern Europe - viewed in the light of biological research. Rapp. P. V. Réun. Cons. Int. Explor. Mer. 20, 1–228. doi: 10.15578/ifrj.3.1.1997.1-7
Houde, E. D. (2008). Emerging from Hjort’s Shadow. J. Northwest Atl. Fish. Sci. 41, 53–70. doi: 10.2960/J.v41.m634
Hufnagl, M., and Peck, M. A. (2011). Physiological individual-based modelling of larval Atlantic herring (Clupea harengus) foraging and growth: insights on climate-driven life-history scheduling. ICES J. Mar. Sci. 68, 1170–1188. doi: 10.1093/icesjms/fsr078
Hurvich, C. M., and Tsai, C.-L. (1989). Regression and time series model selection in small samples. Biometrika 76, 297–307. doi: 10.1093/biomet/76.2.297
ICES (2019). Herring Assessment Working Group for the Area South of 62°N (HAWG). ICES Scientific Report (ICES, Copenhagen), 1:971. doi: 10.17895/ices.pub.5460
ICES (2018). “ICES Herring (Clupea harengus) in subdivision 20-24, spring spawners (Skagerrak, Kattegat, and western Baltic),” in Advice on Fishing Opportunities, Catch, and Effort Baltic Sea and Greater North Sea Ecoregions ICES Scientific Reports her.27.20-24 (ICES, Copenhagen), 12. doi: 10.17895/ices.pub.4390
Jakobsson, J. (1969). On herring migration in relation to changes in sea temperature. Jökull 19, 134–145.
Kanstinger, P., Beher, J., Grenzdörffer, G., Hammer, C., Huebert, K. B., Stepputtis, D., et al. (2018). What is left? Macrophyte meadows and Atlantic herring (Clupea harengus) spawning sites in the Greifswalder Bodden, Baltic Sea. Estuar. Coast. Shelf Sci. 201, 72–81. doi: 10.1016/j.ecss.2016.03.004
Kaspar, F., Zimmermann, K., and Polte-Rudolf, C. (2015). An overview of the phenological observation network and the phenological database of Germany’s national meteorological service (Deutscher Wetterdienst). Adv. Sci. Res. 11, 93–99. doi: 10.5194/asr-11-93-2014
Klinkhardt, M. (1986). Ergebnisse von Untersuchungen zur Schlupf- und Dottersackphase der Larven von Rügenschen Frühjahrsheringen (Clupea harengus L.). Fischerei-Forschung 24, 28–30.
Kotterba, P., Kühn, C., Hammer, C., and Polte, P. (2014). Predation of threespine stickleback (Gasterosteus aculeatus) on the eggs of Atlantic herring (Clupea harengus) in a Baltic Sea lagoon. Limnol. Oceanogr. 59, 578–587. doi: 10.4319/lo.2014.59.2.0578
Kotterba, P., Moll, D., Hammer, C., Peck, M. A., Oesterwind, D., and Polte, P. (2017). Predation on Atlantic herring (Clupea harengus) eggs by the resident predator community in coastal transitional waters. Limnol. Oceanogr. 62, 2616–2628. doi: 10.1002/lno.10594
McCullagh, P., and Nelder, J. A. (1989). Generalized Linear Models, 2nd Edn. London: Chapman & Hall, doi: 10.1201/9780203753736
McPherson, L. R., and Kjesbu, O. S. (2012). Emergence of an oocytic circumnuclear ring in response to increasing day length in Atlantic herring (Clupea harengus). Mar. Biol. 159, 341–353. doi: 10.1007/s00227-011-1812-6
Moll, D., Kotterba, P., von Nordheim, L., and Polte, P. (2018). Storm-induced atlantic herring (Clupea harengus) egg mortality in baltic sea inshore spawning areas. Estuar. Coast. 41, 1–12. doi: 10.1007/s12237-017-0259-5
Moyano, M., Illing, B., Polte, P., Kotterba, P., Zablotski, Y., Gröhsler, T., et al. (2020). Linking individual physiological indicators to the productivity of fish populations: a case study of Atlantic herring. Ecol. Indic. 113:106146. doi: 10.1016/j.ecolind.2020.106146
Munkes, B. (2005). Eutrophication, phase shift, the delay and the potential return in the Greifswalder Bodden, Baltic Sea. Aquat. Sci. 67, 372–381. doi: 10.1007/s00027-005-0761-x
Murawski, S. A., Rago, P. J., and Trippel, E. A. (2001). Impacts of demographic variation in spawning characteristics on reference points for fishery management. ICES J. Mar. Sci. 58, 1002–1014. doi: 10.1006/jmsc.2001.1097
Nature Climate Change (2018). Timing is everything. Nat. Clim. Change 8, 841–841. doi: 10.1038/s41558-018-0304-9
Neuheimer, A. B., and MacKenzie, B. R. (2014). Explaining life history variation in a changing climate across a species’ range. Ecology 95, 3364–3375. doi: 10.1890/13-2370.1
Oeberst, R., Dickey-Collas, M., and Nash, R. D. M. (2009a). Mean daily growth of herring larvae in relation to temperature over a range of 5–20°C, based on weekly repeated cruises in the Greifswalder Bodden. ICES J. Mar. Sci. 66, 1696–1701. doi: 10.1093/icesjms/fsp193
Oeberst, R., Klenz, B., Gröhsler, T., Dickey-Collas, M., Nash, R. D. M., and Zimmermann, C. (2009b). When is year-class strength determined in western Baltic herring? ICES J. Mar. Sci. 66, 1667–1672. doi: 10.1093/icesjms/fsp143
Ojaveer, H., Tomkiewicz, J., Arula, T., and Klais, R. (2015). Female ovarian abnormalities and reproductive failure of autumn-spawning herring (Clupea harengus membras) in the Baltic Sea. ICES J. Mar. Sci. 72, 2332–2340. doi: 10.1093/icesjms/fsv103
Platt, T., Fuentes-Yaco, C., and Frank, K. T. (2003). Spring algal bloom and larval fish survival. Nature 423, 398–399. doi: 10.1038/423398b
Polte, P., Kotterba, P., Hammer, C., and Gröhsler, T. (2014). Survival bottlenecks in the early ontogenesis of Atlantic herring (Clupea harengus, L.) in coastal lagoon spawning areas of the western Baltic Sea. ICES J. Mar. Sci. 71, 982–990. doi: 10.1093/icesjms/fst050
Polte, P., Kotterba, P., Moll, D., and Von Nordheim, L. (2017). Ontogenetic loops in habitat use highlight the importance of littoral habitats for early life-stages of oceanic fishes in temperate waters. Sci. Rep. 7:42709. doi: 10.1038/srep42709
Pörtner, H. O., and Knust, R. (2007). Climate change affects marine fishes through the oxygen limitation of thermal tolerance. Science 315, 95–97. doi: 10.1126/science.1135471
Pörtner, H. O., and Peck, M. A. (2010). Climate change effects on fishes and fisheries: towards a cause-and-effect understanding. J. Fish. Biol. 77, 1745–1779. doi: 10.1111/j.1095-8649.2010.02783.x
Reinicke, R. (1989). Der Greifswalder Bodden - geographisch-geologischer Überblick, Morphogenese und Küstendynamik. Meer Museum 5, 3–9.
Rogers, L. A., and Dougherty, A. B. (2019). Effects of climate and demography on reproductive phenology of a harvested marine fish population. Glob. Chang. Biol. 25, 708–720. doi: 10.1111/gcb.14483
Schwartz, M. D., Ahas, R., and Aasa, A. (2006). Onset of spring starting earlier across the Northern Hemisphere. Glob. Chang. Biol. 12, 343–351. doi: 10.1111/j.1365-2486.2005.01097.x
Sen, P. K. (1968). Estimates of the Regression Coefficient Based on Kendall’s Tau. J. Am. Stat. Assoc. 63, 1379–1389. doi: 10.1080/01621459.1968.10480934
Sinclair, M., and Tremblay, M. J. (1984). Timing of Spawning of Atlantic Herring (Clupea harengus harengus) Populations and the Match-Mismatch Theory. Can. J. Fish. Aquat. Sci. 41, 1055–1065. doi: 10.1139/f84-123
Slotte, A., Johannessen, A., and Kjesbu, O. S. (2000). Effects of fish size on spawning time in Norwegian spring-spawning herring. J. Fish. Biol. 56, 295–310. doi: 10.1006/jfbi.1999.1161
Socolar, J. B., Epanchin, P. N., Beissinger, S. R., and Tingley, M. W. (2017). Phenological shifts conserve thermal niches in North American birds and reshape expectations for climate-driven range shifts. Proc. Natl. Acad. Sci. U.S.A. 114, 12976–12981. doi: 10.1073/pnas.1705897114
von Nordheim, L. (2019). Effects of Coastal Habitat Characteristics on the Reproduction of Baltic Herring (Clupea harengus). Dissertation, University of Hamburg, Hamburg, Germany.
Keywords: herring phenology, climate change, fish reproduction success, Clupea harengus, Baltic Sea
Citation: Polte P, Gröhsler T, Kotterba P, von Nordheim L, Moll D, Santos J, Rodriguez-Tress P, Zablotski Y and Zimmermann C (2021) Reduced Reproductive Success of Western Baltic Herring (Clupea harengus) as a Response to Warming Winters. Front. Mar. Sci. 8:589242. doi: 10.3389/fmars.2021.589242
Received: 25 August 2020; Accepted: 05 January 2021;
Published: 25 January 2021.
Edited by:
Pierluigi Carbonara, COISPA Tecnologia & Ricerca, ItalyReviewed by:
Giuseppe Scarcella, National Research Council (CNR), ItalyCui Liang, Institute of Oceanology (CAS), China
Copyright © 2021 Polte, Gröhsler, Kotterba, von Nordheim, Moll, Santos, Rodriguez-Tress, Zablotski and Zimmermann. This is an open-access article distributed under the terms of the Creative Commons Attribution License (CC BY). The use, distribution or reproduction in other forums is permitted, provided the original author(s) and the copyright owner(s) are credited and that the original publication in this journal is cited, in accordance with accepted academic practice. No use, distribution or reproduction is permitted which does not comply with these terms.
*Correspondence: Patrick Polte, patrick.polte@thuenen.de