- 1Marine Resources and Biodiversity Research Group (GRMAR), Institute of Aquatic Ecology, University of Girona, Girona, Spain
- 2Centre d’Estudis Avançats de Blanes, Consejo Superior de Investigaciones Científicas (CEAB-CSIC), Blanes, Spain
Morphological plasticity can enable algae to adapt to environmental change and increase their invasibility when introduced into new habitats. Nevertheless, there is still a lack of knowledge on how such plasticity can affect the invasion process of an invasive species. In this context, the high plasticity in the genus Caulerpa is well documented. However, after an extremely hot summer, a previously unreported filamentous morphology of Caulerpa cylindracea was detected; indeed, this morphology could only be confirmed taxonomically after in-depth morphological characterization and molecular analysis with the genetic marker tufA. We describe an ex situ culture experiment which showed that stressful conditions, such as high temperatures, can trigger this morphological change. Almost all of the thalli maintained at a constant extreme temperature of 29°C died, but after being returned to optimum temperature conditions, the filamentous morphology began to develop from the surviving microscopic tissue. In contrast, thalli at a control temperature of 21°C maintained the regular morphology throughout the experiment. When C. cylindracea develops this filamentous morphology, it may act as a cryptic invader because it is difficult to detect in the field. Furthermore, the filaments likely improve C. cylindracea’s invasive capabilities with regard to resistance, persistence and dispersion and may have an important role in the re-colonization process, after a population disappears following a period of stressful conditions. Possibly, C. cylindracea’s ability to respond plastically to stressful conditions might explain its remarkable success as an invasive species.
Introduction
Biological invasions refer to the process by which different organisms, commonly known as invasive species, can arrive and establish in a new habitat, where they disrupt the normal functioning of the system. Currently, these invasions are considered one of the main drivers of global change due to their adverse effects on biodiversity, habitat structure and native ecosystem functioning (Mack et al., 2000; Stachowicz and Byrnes, 2006; Simberloff et al., 2013; Bellard et al., 2016). Additionally, the establishment of invasive species it is often associated to great economic costs (Pimentel et al., 2001, 2005) due to their alteration of several ecosystem services (Pejchar and Mooney, 2009; Vilà et al., 2010). The impacts of invasive species are especially important and noticeable in marine ecosystems, where biological invasions are on the rise due to the increases in their main vectors of introduction, such as shipping traffic, the aquarium trade, or the opening and widening of new corridors (Katsanevakis et al., 2013; Seebens et al., 2013; Galil et al., 2017); and in the future, the establishment of non-native species is expected to continue increasing due to climate change (Stachowicz et al., 2002; Lejeusne et al., 2010).
Species invasiveness depends on the features that enable a non-native organism to invade a certain habitat (Richardson et al., 2011) with the main influence being the life-history traits of the invader (Grotkopp et al., 2002; Pyšek and Richardson, 2008; Van Kleunen et al., 2010). Several studies have suggested that phenotypic plasticity is one of the most important of such features for invasive species (Richards et al., 2006; Davidson et al., 2011). Plants and algae can adapt by modifying, among other things, photosynthetic traits (Molina-Montenegro et al., 2012; Zanolla et al., 2015), leaf-area and shoot allocation (Arenas et al., 2002; Liu and Su, 2016) and growth form (Van Kleunen and Fisher, 2001; Monro and Poore, 2009) allowing them to respond to changes in light, temperature or herbivory pressure (Lewis et al., 1987; Monro and Poore, 2005; Nicotra et al., 2010). In this sense, understanding how this phenotypic plasticity affects the success of an invasive species is crucial to our understanding of its invasion process (Schaffelke et al., 2006; Theoharides and Dukes, 2007) and might have important implications for the successful management of the species (Hobbs, 2000; Simberloff et al., 2005).
Caulerpa cylindracea is a siphonaceous green macroalga, native to Western Australia, that has become one of the most widespread non-native algae in the Mediterranean Sea (Piazzi et al., 2005; Klein and Verlaque, 2008; Montefalcone et al., 2015). Indeed, C. cylindracea is currently considered the most invasive species within the Mediterranean basin (Katsanevakis et al., 2016) and has also invaded areas in the Atlantic Ocean (Verlaque et al., 2004) and in Southern Australia (Klein and Verlaque, 2008). Still, it is not clear which has been the source of the primary introduction in the Mediterranean Sea, although it is quite likely that it was through the aquarium trade, with shipping traffic and fishing gear being the main sources of the secondary introductions within the basin (Verlaque et al., 2003). Ecologically, C. cylindracea spread causes the homogenization of native communities due to the formation of dense and continuous meadows (Klein and Verlaque, 2008), which has negative implications for the native macroalgal assemblages and the diversity of the communities (Piazzi et al., 2001; Piazzi and Ceccherelli, 2006; Klein and Verlaque, 2011). Also, this species affects the sedimentation rates, the carbon turnover, the organic matter composition and the quality of the invaded sediments (Piazzi et al., 2007; Holmer et al., 2009; Pusceddu et al., 2016; Rizzo et al., 2017). Morphologically, C. cylindracea is characterized by a simple morphology, formed by creeping stolons and erect shoots with grape-like ramuli (also called branchlets) that can be arranged radially or distichously (Klein and Verlaque, 2008). In addition, it has been reported that species in the genus Caulerpa show a high degree of morphological plasticity in response to environmental conditions (Peterson, 1972; Calvert, 1976; Coppejans and Beeckman, 1989; Collado-Vides, 2002b), allowing these species to adapt to different environments and thus increasing their invasive potential (Collado-Vides, 2002b; Raniello et al., 2004; Smith, 2009). Several factors such as temperature, light or depth, can trigger subtle morphological changes in stolon and ramuli shape (Peterson, 1972; Calvert, 1976; Ohba and Enomoto, 1987; Ohba et al., 1992), photosynthetic traits (Raniello et al., 2004, 2006) and the interspace between erect axes (Collado-Vides, 2002b; De Senerpont Domis et al., 2003). However, more acute morphological changes have been detected for the first time in C. cylindracea during a recent field survey (Figure 1). The thalli of these specimens consisted only of thin vertical filaments, which were impossible to identify as Caulerpa species until morphological and molecular characterization confirmed their identity. At present, the exact conditions that trigger this morphological change are unknown but, considering that the filamentous form was found in Montenegro after the extremely warm summer of 2018 (Figure 2), it would appear that stressful conditions brought about by high temperatures could be involved. A better understanding of the conditions that trigger this morphological shift—which allows C. cylindracea to become a cryptic invader—will greatly enhance our understanding of the invasive process, the collapses and the recoveries of this species.
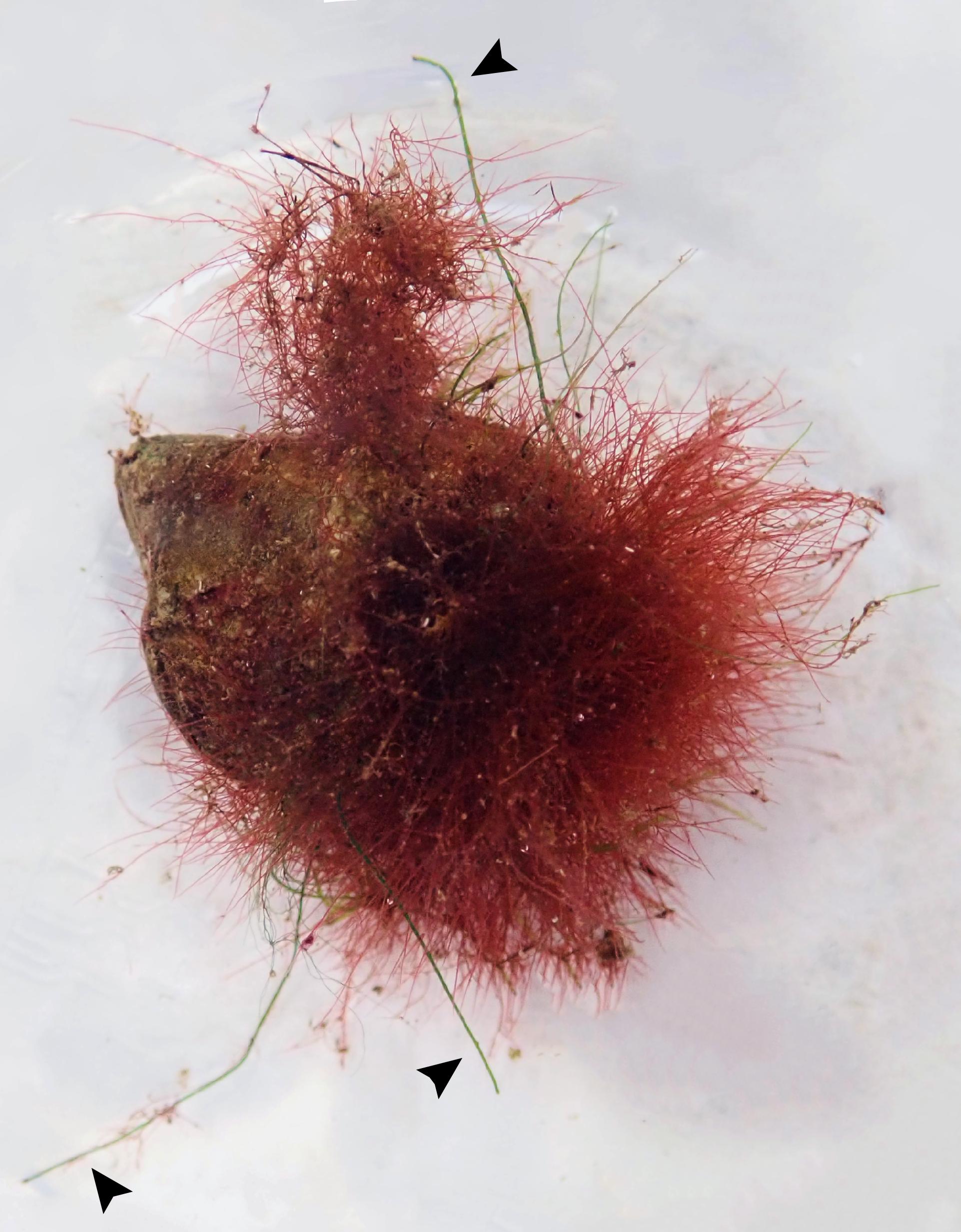
Figure 1. Macroscopic picture of a rock covered by a thick patch of Womersleyella setacea turf with the filamentous form of Caulerpa cylindracea growing from beneath it (black arrows).
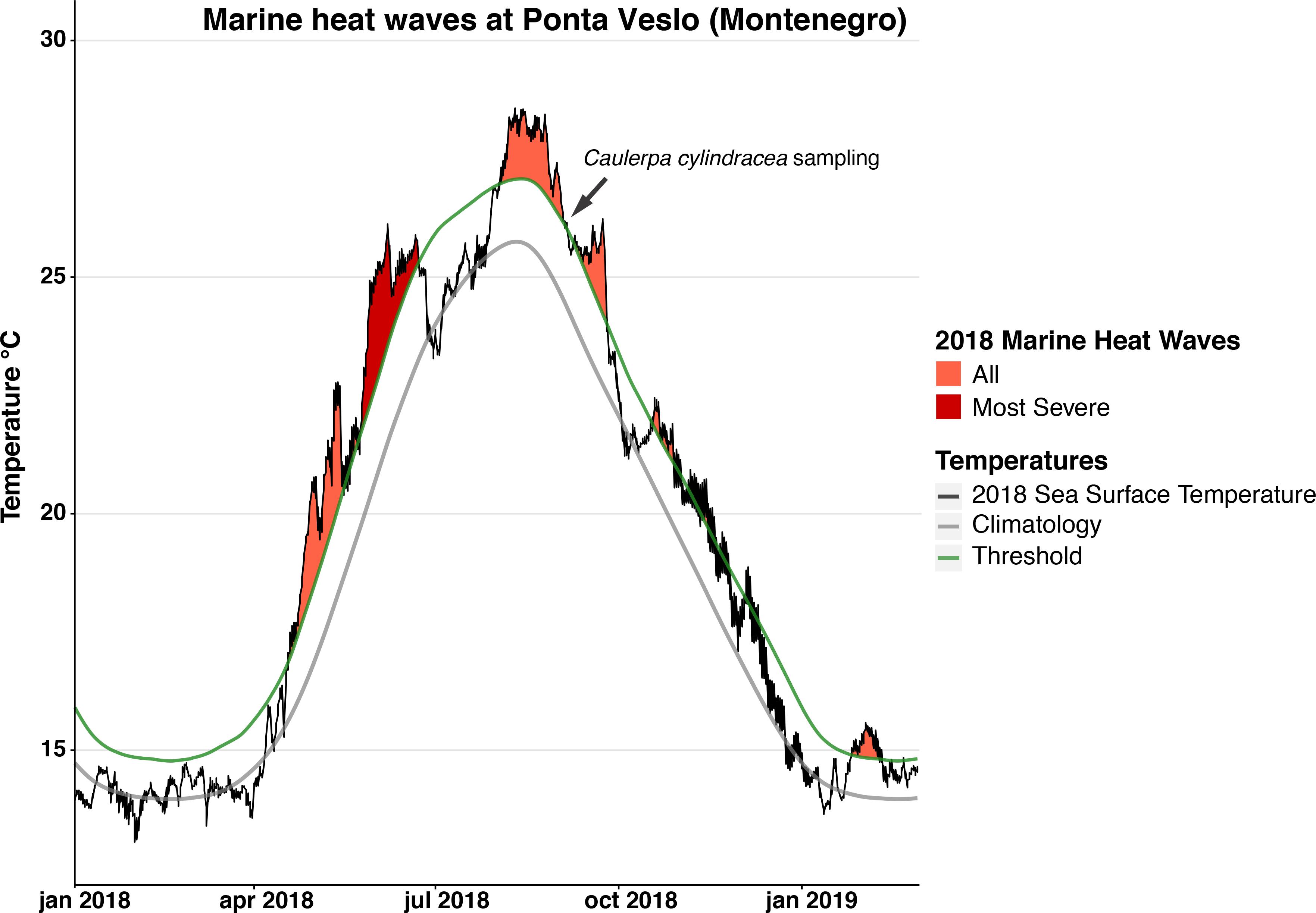
Figure 2. Marine heat wave (MHW) events during 2018 at Ponta Veslo, Montenegro (42° 22′ 5.15″N; 18° 36′ 22.50″E), calculated with the heatwaveR package (Schlegel and Smit, 2018) using Reynolds Optimally Interpolated Sea Surface Temperature (OISST) data (Reynolds et al., 2007). The gray line represents the SST climatology for the last 35 years; the green line indicates the 90th percentile MHW threshold; and the black line shows the SST during 2018. The dark red filled area indicates the most severe MHW event during 2018, while the orange filled areas indicate all the other MHW events identified over the same time period. The dark gray arrow indicates the day of the year when the filamentous morphology of Caulerpa cylindracea was sampled from the field (5th of September).
In this study, our aim was to determine whether extreme temperature conditions can trigger the formation of a filamentous morphology in Caulerpa cylindracea similar to that observed in the field. To do so, the morphological plasticity of this species was studied through culture experiments at contrasting temperatures. In addition, in order to confirm the taxonomical identity of the specimens, all thalli (from both cultured and natural populations) were genetically characterized with a chloroplast molecular marker (tufA), which had been used previously for the genus Caulerpa (Famà et al., 2002; Kazi et al., 2013; Sauvage et al., 2013).
Materials and Methods
Study Sites and Culture
Extreme Temperature Laboratory Experiment
To study the effects of extreme temperatures on C. cylindracea, specimens from a population in Spain (Roses: 42° 14′ 18.26″N; 3° 12′ 25.74″E) were sampled in February 2019. Once in the laboratory, samples were cleaned with sterilized seawater to remove all the epiphytes and detritus (such as dead Posidonia oceanica rhyzomes and dead shells). For acclimation, Caulerpa samples were placed in aquariums (12 L) with sterile seawater and in a Radiber AGP-360 growth chamber at 12°C and a 12:12 (L:D) cycle at 200 μmol photons m–2 s–1 to simulate natural conditions of irradiance and temperature for 1 week. After the acclimation period, algal cultures were prepared for a period of 170 days under either control conditions or extreme (i.e., very warm) conditions (Figure 3). Six fragments of C. cylindracea (≈4 cm2 each) were randomly transferred to six plastic beakers (1 L): three control treatments and three extreme-temperature treatments, each containing 200 g of sterilized gross sand and 0.5 L of sterilized seawater to which was added 5 ml/l of K-medium (Keller et al., 1987). The temperature treatments for the experiment were as follows: “control” (21°C) based on the average summer seawater temperature recorded in the sampled area and “extreme” (29°C) based on abnormally high summer seawater temperatures recorded in the Mediterranean Sea1. After an adaptation period of 7 days at 12°C in the growth chamber, the temperature was progressively increased (by 1°C every 2 days) in all six treatment beakers for 18 days until a temperature of 21°C was reached in the growth chamber. At this point, the beakers were split into two Radiber AGP-360 growth chambers, one to keep the “control” beakers and the other to keep the “extreme” beakers throughout the experiment. Following this, the “control” beakers were maintained at 21°C for the remaining 152 days of the experiment in the growth chamber; whereas, in the case of the “extreme” beakers, the temperature was raised in the other growth chamber by 1°C every 5 days for the next 40 days until a temperature of 29°C was reached; this temperature was then maintained for a further 14 days and then gradually lowered by 1°C each day back to 21°C and kept at this temperature for the remaining 90 days of the experiment (Figure 3). Throughout the experiment, the seawater and growth medium mixture was renewed once a week.

Figure 3. Water temperature variation over the course of the experiment in “control” conditions (above) and “extreme” conditions (below). The numbers indicate the days of culture and each change in color represents a 1°C change in temperature.
Field Sampling for Morphological and Taxonomical Characterization
Samples of C. cylindracea were collected from natural populations in Spain (Roses: 42° 14′ 18.26″N; 3° 12′ 25.74″E); Croatia (Funtana: 45° 10′ 40.16″N; 13° 35′ 32.31′ E and Split: 43° 30′ 28.79″N; 16° 23′ 17.56″E); Montenegro (Ponta Veslo: 42° 22′ 5.15″N; 18° 36′ 22.50′E); and Albania (Kallm: 41° 19′ 27.88″N; 19° 25′ 19.31″E) by scuba-divers at depths of between 5 and 12 m based on previous knowledge on the presence of the invasive alga in these locations. All samples were transported in zip bags within a thermal box to maintain a constant seawater temperature until the morphological characterization was performed in the laboratory. All samples showed the typical morphology of the species (Figure 4B). These samples were also used for the taxonomic characterization of the populations (see “Molecular Analysis” section).
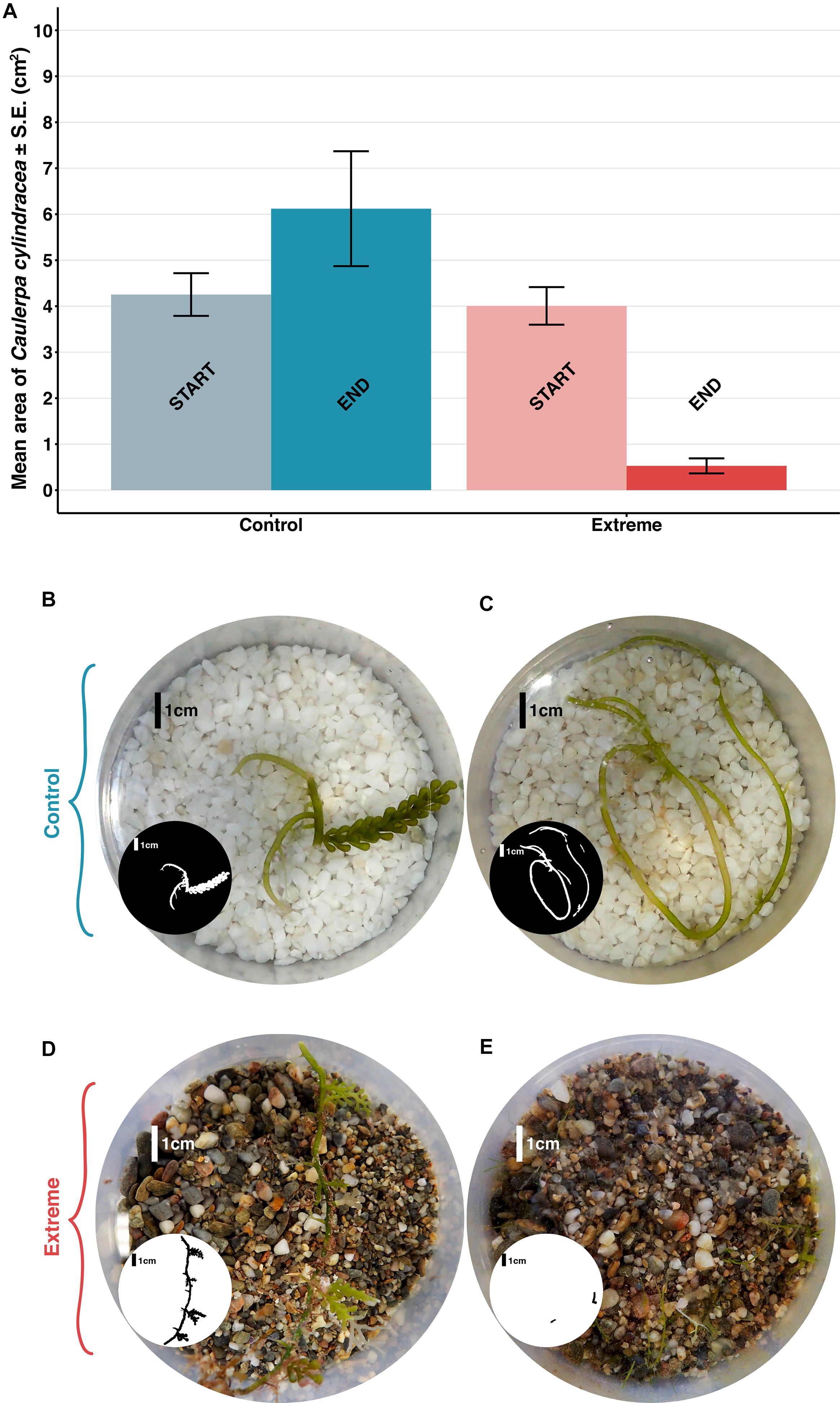
Figure 4. (A) Mean area ±S.E. of Caulerpa cylindracea in the beakers (n = 6, 3 control beakers and 3 extreme temperature beakers), at the beginning of the experiment, and after 72 days. Photographs (B,C) show control beakers at the beginning of the treatment and after 72 days, respectively. Photographs (D,E) show extreme temperature beakers at the beginning of the treatment and after 72 days, respectively. The small black and white circles highlight the C. cylindracea present in each beaker.
Data Collection and Analysis
Extreme Temperature Laboratory Experiment
The effects of the extreme temperatures we applied to C. cylindracea were assessed by measuring the macroscopic morphometric changes. The structure and area of Caulerpa were measured by means of macroscopic photographs taken with an Olympus TG-5 camera, which were then analyzed with Adobe Photoshop CC 2018. Living parts of C. cylindracea (green color) were manually selected and measured using the “analysis tool.” Then, to assess whether there were differences in the area of C. cylindracea between treatments at the end of the experiment, a linear mixed effect model (LMM) was fitted with “C. cylindracea area” as the response variable and “treatment” and “time” as the explanatory variables. The interaction between both explanatory variables was included in the model and a random term for “replicate” was used to take into account the repeated measures design (Harrison et al., 2018). To fit this model, the package lme4 (Bates et al., 2015) in the statistical environment R was used (R version 3.6.3) (R Core Team., 2018). Finally, to compare the effects between levels in the treatment factor (“control” and “extreme) at each time observation (“beginning” and “end”), Tukey post hoc tests were performed using the functions “pairs” and “emmeans” from the package emmeans (Lenth, 2018).
Morphological Characterization and Comparison
The morphological characterization of the cultured stolons and filaments was assessed by means of microscopic photographs, taken using a Zeiss AXIO Imager A.2 (Carl Zeiss, Berlin, Germany) equipped with an AxioCam MRc5 camera and a stereomicroscope Stemi 2000-C (Carl Zeiss, Berlin, Germany) equipped with an AxioCam ERc 5s camera; and the images were analyzed with Zen2011 software (Blue Edition). Also, to account for regional morphological variability, the mean stolon thickness of 10 randomly sampled stolons from each of the natural populations (Roses, Funtana, Split, Ponta Veslo, and Kallm) was compared to the thickness of filaments obtained at extreme temperatures.
To assess whether mean thickness of filaments differed from that of stolons, a linear model was fitted with “C. cylindracea thickness” as the response variable and “location_morphology” as the explanatory variable, in the statistical environment R. Finally, to compare between location_morphology levels (“Roses_filaments,” “Roses_stolons,” “Funtana_stolons,” “Split_stolons,” “Ponta Veslo_stolons,” and “Kallm_stolons”), Tukey post hoc tests were performed using the functions “pairs” and “emmeans” from the package emmeans.
Molecular Analysis
To determine species identity, a genetic analysis was performed on all sampled and incubated thalli, including both filamentous and typical morphology. DNA extraction was performed following the DNeasy Plant Mini Kit (QiaGen) protocol in order to obtain the best DNA quality. The primer used to amplify the genetic material was tufA (elongation factor A, from the chloroplast). The reactions were performed in 25 μL volumes and the master mix contained 5 mM of MgCl2, 0.3 mM of each primer, 0.2 mM of dNTPs, 0.5 units of Taq DNA polymerase and 1.0 μL of the extracted DNA. The PCR reaction consisted of 40 cycles of 94°C for 1 min (denaturation), 52°C for 1 min (primer annealing) and 72°C for 2 min (extension) (Famà et al., 2002). The PCR reaction was finalized with a final 5 min step at 72°C. The PCR products were purified and sequenced by Macrogen Spain.
Sequences analyses were performed using different R (R Core Team., 2018) packages: MUSCLE (Edgar, 2004) to align the sequences, and APE (Paradis et al., 2004) and PHANGORN (Schliep et al., 2017) to create phylogenetic trees based on statistical analyses (Bio neighbor-joining tree, k80 distance, with 10,000 replicates).
Results
Extreme Temperature Laboratory Experiment
In the model fitted to the data from the extreme temperature experiment, both “treatment” and the interaction term between “treatment” and “time” showed a significant effect on C. cylindracea area (p < 0.05, Table 1), being the area of C. cylindracea equal between treatments at the beginning of the experiment but being significantly different between “control” and “extreme” conditions at the end (p < 0.05, Table 2). Actually, after 72 days, the mean surface area of the Caulerpa cylindracea thalli under “control” conditions had increased by 44%, whereas that of the specimens exposed to the “extreme temperature” treatment (at 29°C for final 14 days of this 72 days period) had decreased significantly by 87% (Figure 4A). Furthermore, while the control specimens maintained the typical morphology of the species—characterized by thick rhizomes with some vertical vesicular fronds (Figure 4C)—almost all the thick parts of the specimens in the “extreme temperature treatment” had disappeared after 14 days at 29°C (Figure 4E).
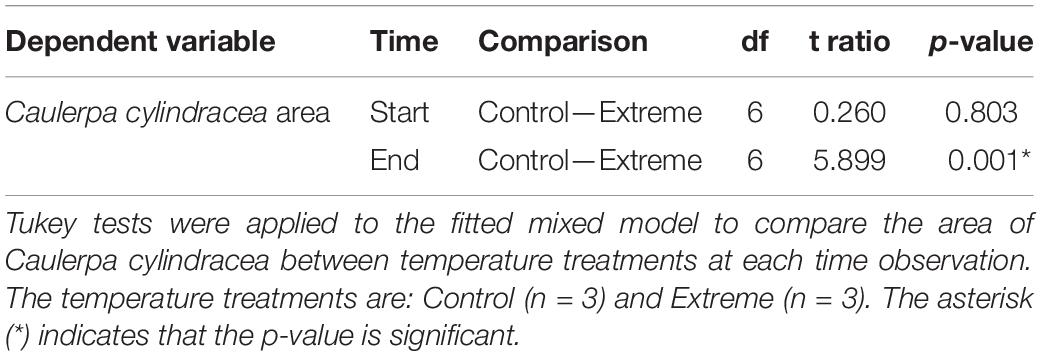
Table 2. Effect of temperature treatment on the area of Caulerpa cylindracea, at the beginning and end of the culture experiment.
When the cultures were returned to optimum conditions, new living parts emerged from the sediment in all the “extreme treatment” beakers, after 20 days. However, these new parts did not resemble the original C. cylindracea’s morphology. Instead, they presented a new type of structure characterized by erect thin filaments (Figure 5A), which for the next 3 months continued growing vertically and extending throughout the liquid in the beakers (Figure 5B).
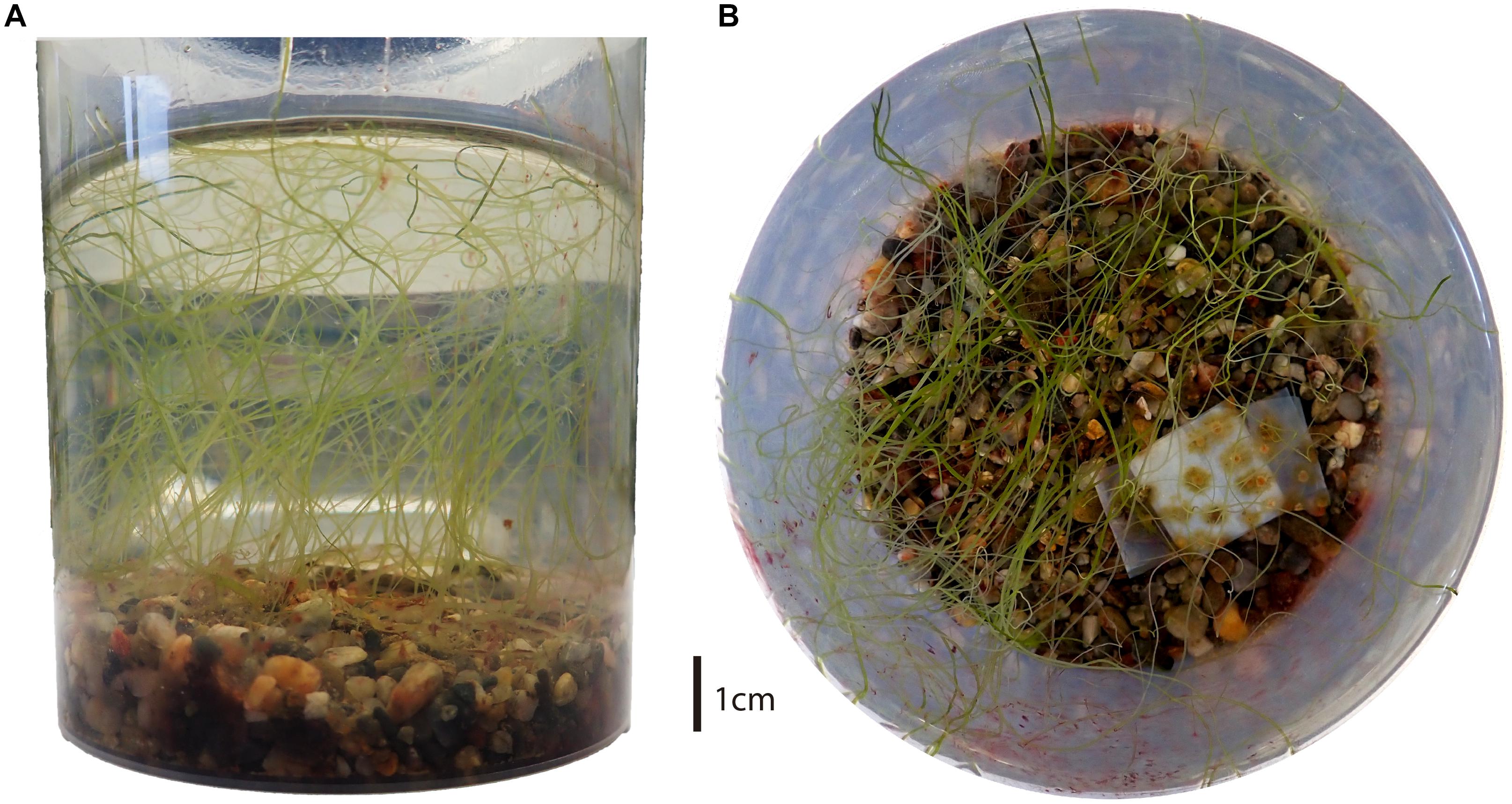
Figure 5. Lateral (A) and vertical (B) view of one beaker with the filamentous morphology of Caulerpa cylindracea. The white square on the bottom of the beaker was added at the end of the experiment, to use it as a scale.
Macroscopically, these filaments, which grow vertically from the substrate, are long (between 5 and 10 cm) and thin, and occasionally branched. Each filament has rhizoids in its basal part (which is without plastids) that serve to attach the filament to the substrate. The upper part of the filament (with plastids) is light green and in all cases devoid of rhizoids or vesicles, which contrasts with the typical morphology of C. cylindracea (Figures 4B,D).
These filaments contrasted with the C. cylindracea thalli cultured under control conditions which, throughout the experiment (Figures 4B,C), maintained the typical morphology described for this species: the thalli were characterized by thick, prostrate stolons with interspaced rhizoids for anchoring, even where they grew unattached to the substrate. Vesicular fronds could also be observed, although these were smaller than in the field.
Morphological Comparison
In the model fitted to the morphological data, the variable “location_morphology” showed a significant effect on C. cylindracea thickness (p < 0.05, Table 3), with filament thickness being significantly different to stolon thickness (p < 0.005, Table 4) while stolon thickness was similar between populations (Table 4). Actually, microscopically, there was an almost 10-fold difference in mean thickness between the filaments (222.42 ± 9.78 μm) in the extreme temperature beakers and the stolons (2,093.38 ± 31.50 μm) sampled at different natural populations. This is amply illustrated in Figure 6.
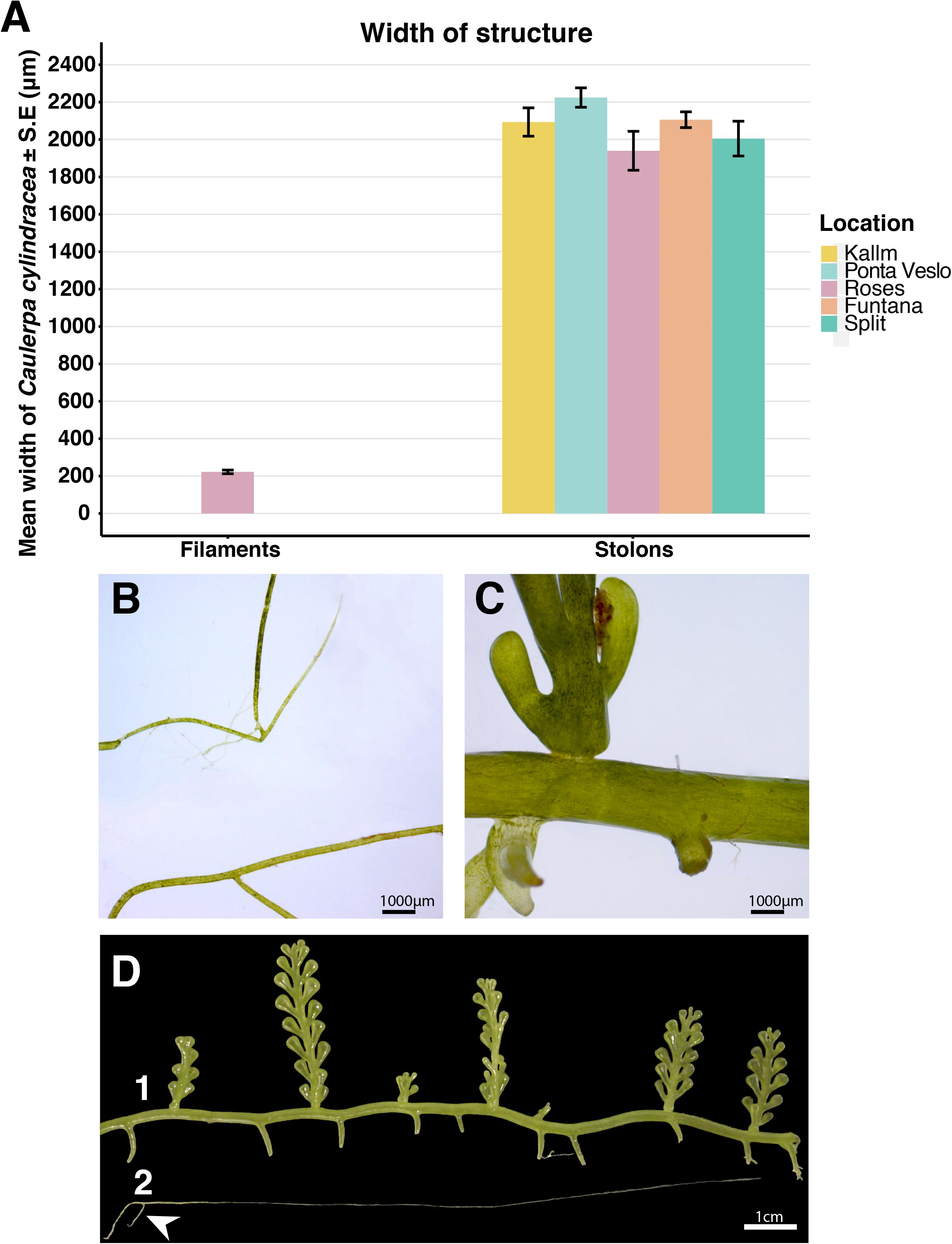
Figure 6. (A) Mean width ±S.E. of Caulerpa cylindracea filaments (left) and stolons (right) from different populations (n = 10 for each population). (B) View of two filaments under the stereomicroscope at 1.0×. (C) View of a stolon with a vertical vesicular frond under the stereomicroscope at 1.0×. (D) (1) Macroscopic view of C. cylindracea with the regular morphology and (2) macroscopic view of the filamentous form of C. cylindracea. The white arrow shows the rhizoids that the filaments use for attaching themselves to the substrate.
Although erect filaments are rarely ascribed to Caulerpa genus, the presence of trabeculae—slender strands traversing the lumen of the thallus (Lamouroux, 1809; Womersley, 1984; Wynne and Bold, 1985)—confirms the identity of the thalli. The anatomical morphology of this structure in the filaments is identical to that of the regular stolons, as they traverse the interior of the filament going from one side to the other of a circular section and attach to the wall with a thickened structure that resembles a suction cup. However, the trabeculae in the filaments are much thinner and less numerous than in the stolons, which might explain why the filaments are so weak.
Taxonomical Characterization
For the genetic analysis, a total of 14 high quality tufA sequences of up to 820 bp. were obtained and amplified from five specimens with the filamentous shape, eight specimens from the Mediterranean Sea with the common morphology of Caulerpa cylindracea and an additional sequence from C. sertularioides, which was obtained from the Caribbean Sea (Table 5). In addition, several C. cylindracea sequences were obtained from GenBank, together with sequences from C. taxifolia and C. prolifera, in order to establish differences in the phylogenetic tree. In the Neighbor Joining tree (Figure 7), all sequences from C. cylindracea formed a highly supported cluster, grouped close together, which included both filamentous and typical thalli, thus indicating that they are the same entity.
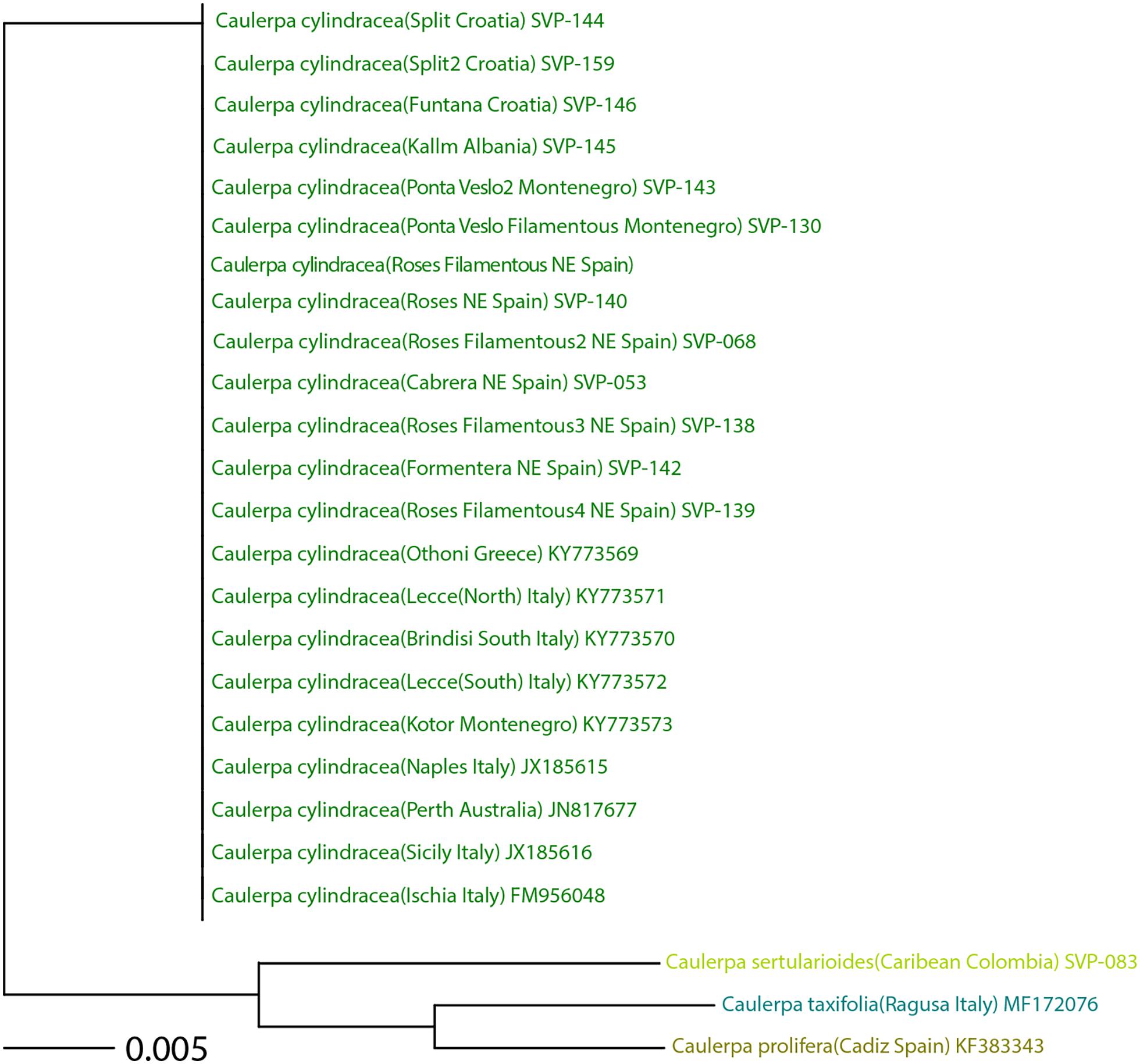
Figure 7. Phylogenetic Neighbor Joining (NJ) tree for Caulerpa cylindracea, obtained using the tufA marker. Three other species of Caulerpa (C. sertularioides, C. taxifolia, and C. prolifera) were used as closely related species to establish differences. GenBank accession numbers are given for each sequence. Values at nodes derived from the NJ. The sequences produced in the present study are highlighted in bold.
Discussion
Our culture experiments showed that extreme environmental conditions, in this case high temperatures maintained for long periods of time (i.e., 14 days at 29°C), affected the survival of Caulerpa cylindracea and triggered the development of a new morphology characterized by long, thin vertical filaments from the surviving tissues. This new morphology of C. cylindracea has also been found in the field and is described here for the first time.
The laboratory experiment showed the remarkable capacity of C. cylindracea to survive under stressful culture conditions as the temperature treatment chosen (29°C) is much higher than the usual temperatures found at both the native and the invaded range (Klein and Verlaque, 2008). Additionally, C. cylindracea showed a great ability to adapt to environmental change, because it developed a new filamentous morphology from the damaged tissue that survived to the unfavorable culture conditions, i.e., extreme temperatures. These morphological changes during vegetative development are a common strategy in sessile organisms such as plants (Dorn et al., 2000; Puijalon et al., 2008; Nicotra et al., 2010) and algae (Kübler and Dudgeon, 1996; Garbary et al., 2004; Monro and Poore, 2005; Fowler-Walker et al., 2006) to tolerate environmental change, and to improve their competitive and survival capacity (Bradshaw, 1972; Harper et al., 1986; Price and Marshall, 1999; Collado-Vides, 2002a). Actually, more or less acute morphological changes have been previously observed and described in different algae species (e.g., some Caulerpa species, Ulva prolifera, Chondrus crispus, Asparagopsis armata, Padina jamaicensis, Codium fragile, or Ecklonia radiata among others) under different culture conditions (Ohba and Enomoto, 1987; Ohba et al., 1992; Kübler and Dudgeon, 1996; De Senerpont Domis et al., 2003; Garbary et al., 2004; Monro and Poore, 2005; Gao et al., 2016) and in the field (Lewis et al., 1987; Meinesz et al., 1995; Collado-Vides, 2002b; Garbary et al., 2004; Fowler-Walker et al., 2006), as a response to changes in temperature, salinity, hydrodynamism, light, or herbivory pressure.
Taking into account that Caulerpa cylindracea may be one of the most widely studied invasive algae species around the world, the lack of a previous description of the filamentous morphology demonstrates that when C. cylindracea adopts this form, it clearly goes undetected. For instance, the filamentous morphology was sampled—accidentally—in the field within a macroalgae benthic community (Figure 1) and was only identified as Caulerpa after the samples were analyzed under the microscope. Thus it is clear that C. cylindracea filaments also develop under natural conditions. The variables that trigger such filaments in the field may, however, be multiple and in general, remain uncertain. Nevertheless, the culture experiments and field observations described here strongly indicate that stressful conditions may induce the formation of these structures.
The phenotypic plasticity observed in C. cylindracea and its ability to withstand extreme conditions (high temperatures) for a long time are characteristics that clearly influence the invasiveness of this species, and improve its persistence and resistance to stress. Actually, this resistance, together with the difficulty of observing the filaments underwater, might explain why C. cylindracea populations sometimes seem to disappear only to bounce back after a few months (García et al., 2016). In such situations, it may be that while most of the population dies following unfavorable conditions (Figure 8E; Klein and Verlaque, 2008), some small remnants survive, most probably hidden and undetectable in the sediment or sand. Then, as was the case in our culture experiment, the surviving parts may produce filaments that go unnoticed by divers and researchers (Figure 1), and, subsequently, the regular morphology of C. cylindracea returns when favorable conditions allow the population to recover (Figure 8G). Typically, colonization of a new area by C. cylindracea can take approximately 3 years, but the presence of this cryptic and resistant form of the species would explain the marked reduction—from 3 years to less than 1 year—in the time it takes C. cylindracea to re-colonize areas in which it had disappeared (García et al., 2016), thus highlighting the importance of this morphology in the re-colonization process (Figure 8). Furthermore, when adopting this cryptic phase, identification is extremely difficult or simply impossible, which has further implications for the management of the species, since early detection is one of the most important requirements for the successful management of invasive species (Lodge et al., 2006; Vander Zanden et al., 2010; Giakoumi et al., 2019). This misidentification of the filaments can also affect current estimations of C. cylindracea in the Mediterranean Sea as this species may be present at several locations in this latent, cryptic form. Since traditional methods of direct observation may not always be reliable in detecting filaments of C. cylindracea, as they can be misidentified or missed entirely, methods involving environmental DNA (Taberlet et al., 2012; Kelly et al., 2014; Thomsen and Willerslev, 2015) could be useful in detecting this species, as has been the case with the detection of other invasive species (Dejean et al., 2012; Ardura et al., 2015; Simmons et al., 2015).
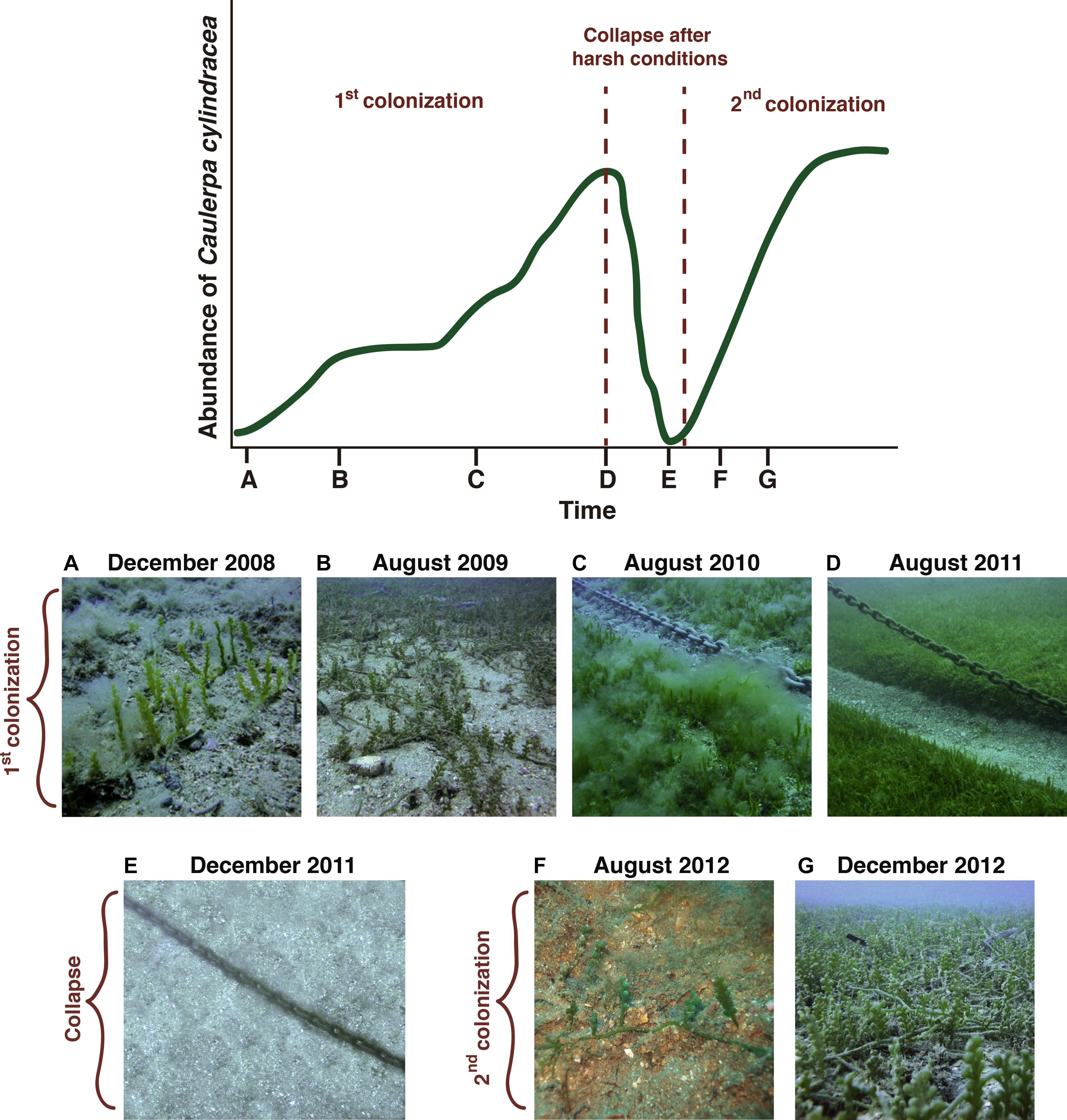
Figure 8. Evolution of the abundance of Caulerpa cylindracea over a period of 4 years in a population under study, close to Vilanova i la Geltrú (Spain). During the first colonization, C. cylindracea was first detected in December 2008 (A); by August 2009, C. cylindracea had expanded and had a patchy heterogeneous distribution (B); in August 2010, C. cylindracea covered a larger area forming a continuous meadow (C); and in August 2011, the abundance of C. cylindracea reached a peak, forming a dense and continuous meadow over more than 1,000 m2 (D). In December 2011, the population had collapsed after an exceptionally warm autumn and no sign of C. cylindracea could be found (E). The first report of C. cylindracea in the second colonization was in August 2012 (F); and just 3 months later, in December 2012, it was already forming a continuous meadow over a large area (G), with greater abundance than that of August 2010 (C).
Dispersion is another process that might also be favored by C. cylindracea filaments, because these long and weak vertical structures are more easily broken upon contact or by water movement than the regular thallus. This will release living fragments that can be transported by currents or attached to drifting objects, favoring dispersal of C. cylindracea and the potential for secondary introductions. Actually, in Caulerpa species, the fragmentation process is one of its most important reproductive strategies (Fralick and Mathieson, 1972; Ceccherelli and Cinelli, 1999; Smith and Walters, 1999; Ceccherelli and Piazzi, 2001) and allows these species to spread really fast.
To conclude, this newly identified filamentous morphology of C. cylindracea could act as a potential jack-of-all-trades that further improves this species’ capacity as an invader. The filaments described here are involved in the persistence, resistance, and dispersion of this invasive species and have characteristics that allow this species to withstand harsh abiotic conditions and which may help to explain its successful expansion in the Mediterranean Sea.
Data Availability Statement
The datasets generated for this study and the code to analyse the data can be found here: https://github.com/JorgeSantamaria/Filamentous-Morphology-Caulerpa. The genetic sequences amplified in this study are deposited in the GenBank repository and can be accessed with the accession numbers shown in Table 5.
Author Contributions
JS, EC, and AV conceived the ideas and the experimental design. JS, RG, and MG collected the samples. JS and RG analyzed the data. All authors drafted the manuscript, contributed substantially to revisions, and accepted the final version before submission.
Funding
This project was funded by the Spanish Ministry of Science, Innovation and Universities (project ANIMA, ref. CGL2016-76341-R). JS received the support of a fellowship from “La Caixa” Foundation (ID 100010434) with code (LCF/BQ/DE17/11600001). RG benefited from a pre-doctoral fellowship from the Spanish Ministry of Science (ref. BES-2017-079907).
Conflict of Interest
The authors declare that the research was conducted in the absence of any commercial or financial relationships that could be construed as a potential conflict of interest.
Acknowledgments
We would like to thank Jana Verdura, Ljiljana Iveša, Petra Lučić, Vesna Mačić, and Jerina Kolitari for support during field collection of the samples. We acknowledge the role of the project AFRIMED (EASME/EMFF/2017/1.2.1.12/S4). JS, RG, EC, and AV are members of the Marine Conservation Research Group (d3d3Lm1lZHJlY292ZXIub3Jn; 2017 SGR 1521) from the Generalitat de Catalunya. Finally, we would like to thank the reviewers for their valuable and constructive comments in this manuscript.
Footnotes
References
Ardura, A., Zaiko, A., Martinez, J. L., Samulioviene, A., Semenova, A., and Garcia-Vazquez, E. (2015). eDNA and specific primers for early detection of invasive species - A case study on the bivalve Rangia cuneata, currently spreading in Europe. Mar. Environ. Res. 112, 48–55. doi: 10.1016/j.marenvres.2015.09.013
Arenas, F., Viejo, R. M., and Fernández, C. (2002). Density-dependent regulation in an invasive seaweed: responses at plant and modular levels. J. Ecol. 90, 820–829. doi: 10.1046/j.1365-2745.2002.00720.x
Bates, D., Mächler, M., Bolker, B., and Walker, S. (2015). Fitting linear mixed-effects models using lme4. J. Stat. Softw. 67, 1–48. doi: 10.18637/jss.v067.i01
Bellard, C., and Cassey, P. and Blackburn, T. M. (2016). Alien species as a driver of recent extinctions. Biol. Lett. 12:0623. doi: 10.1098/rsbl.2015.0623
Bradshaw, A. D. (1972). Some of the evolutionary consequences of being a plant. Evol. Biol. 5, 25–47. doi: 10.1007/978-1-4757-0256-9_2
Calvert, H. E. (1976). Culture studies on some Florida species of Caulerpa: Morphological responses to reduced illumination. Br. Phycol. J. 11, 203–214. doi: 10.1080/00071617600650481
Ceccherelli, G., and Cinelli, F. (1999). The role of vegetative fragmentation in dispersal of the invasive alga Caulerpa taxifolia in the Mediterranean. Mar. Ecol. Prog. Ser. 182, 299–303. doi: 10.3354/meps182299
Ceccherelli, G., and Piazzi, L. (2001). Dispersal of Caulerpa racemosa Fragments in the Mediterranean: Lack of Detachment Time Effect on Establishment. Bot. Mar. 44, 209–213. doi: 10.1515/BOT.2001.027
Collado-Vides, L. (2002a). Clonal architecture in marine macroalgae: ecological and evolutionary perspectives. Evol. Ecol. 15, 531–545. doi: 10.1007/978-94-017-1345-0_17
Collado-Vides, L. (2002b). Morphological plasticity of Caulerpa prolifera (Caulerpales, Chlorophyta) in relation to growth form in a coral reef lagoon. Bot. Mar. 45, 123–129. doi: 10.1515/BOT.2002.013
Coppejans, E., and Beeckman, T. (1989). Caulerpa section Sedoideae (Chlorophyta, Caulerpales) from the Kenyan coast. Nov. Hedwigia 49, 381–393. doi: 10.1127/nova.hedwigia/50/1990/111
Davidson, A. M., Jennions, M., and Nicotra, A. B. (2011). Do invasive species show higher phenotypic plasticity than native species and, if so, is it adaptive? A meta-analysis. Ecol. Lett. 14, 419–431. doi: 10.1111/j.1461-0248.2011.01596.x
De Senerpont Domis, L. N., Famà, P., Bartlett, A. J., Prud’homme Van, Reine, W. F., Espinosa, C. A., et al. (2003). Defining taxon boundaries in members of the morphologically and genetically plastic genus Caulerpa (Caulerpales, Chlorophyta). J. Phycol. 39, 1019–1037. doi: 10.1111/j.0022-3646.2003.02-203.x
Dejean, T., Valentini, A., Miquel, C., Taberlet, P., Bellemain, E., and Miaud, C. (2012). Improved detection of an alien invasive species through environmental DNA barcoding: The example of the American bullfrog Lithobates catesbeianus. J. Appl. Ecol. 49, 953–959. doi: 10.1111/j.1365-2664.2012.02171.x
Dorn, L. A., Pyle, E. H., and Schmitt, J. (2000). Plasticity to light cues and resources in Arabidopsis thaliana: testing for adaptive value and costs. Evolution 54, 1982–1994. doi: 10.1111/j.0014-3820.2000.tb01242.x
Edgar, R. C. (2004). MUSCLE: Multiple sequence alignment with high accuracy and high throughput. Nucleic Acids Res. 32, 1792–1797. doi: 10.1093/nar/gkh340
Famà, P., Wysor, B., Kooistra, W. H. C. F., and Zuccarello, G. C. (2002). Molecular phylogeny of the genus Caulerpa (Caulerpales, Chlorophyta) inferred from chloroplast tufA gene. J. Phycol. 38, 1040–1050. doi: 10.1046/j.1529-8817.2002.t01-1-01237.x
Fowler-Walker, M. J., Wernberg, T., and Connell, S. D. (2006). Differences in kelp morphology between wave sheltered and exposed localities: morphologically plastic or fixed traits? Mar. Biol. 148, 755–767. doi: 10.1007/s00227-005-0125-z
Fralick, R. A., and Mathieson, A. C. (1972). Winter fragmentation of Codium fragile (Suringar) Hariot ssp. tomentosoides (van Goor) Silva (Chlorophyceae, Siphonales) in New England. Phycologia 11, 67–70. doi: 10.2216/i0031-8884-11-1-67.1
Galil, B., Marchini, A., Occhipinti-Ambrogi, A., and Ojaveer, H. (2017). The enlargement of the Suez Canal—Erythraean introductions and management challenges. Manag. Biol. Invasions 8, 141–152. doi: 10.3391/mbi.2017.8.2.02
Gao, G., Zhong, Z., Zhou, X., and Xu, J. (2016). Changes in morphological plasticity of Ulva prolifera under different environmental conditions: A laboratory experiment. Harmful Algae 59, 51–58. doi: 10.1016/j.hal.2016.09.004
Garbary, D. J., Fraser, S. J., Hubbard, C., and Kim, K. Y. (2004). Codium fragile: Rhizomatous growth in the Zostera thief of eastern Canada. Helgol. Mar. Res. 58, 141–146. doi: 10.1007/s10152-004-0173-7
García, M., Weitzmann, B., Pinedo, S., Cebrian, E., and Ballesteros, E. (2016). First report on the distribution and impact of marine alien species in coastal benthic assemblages along the catalan coast. Handb. Environ. Chem. 43, 249–270. doi: 10.1007/698-2015-411
Giakoumi, S., Katsanevakis, S., Albano, P. G., Azzurro, E., Cardoso, A. C., Cebrian, E., et al. (2019). Management priorities for marine invasive species. Sci. Total Environ. 688, 976–982. doi: 10.1016/j.scitotenv.2019.06.282
Grotkopp, E., Rejmánek, M., and Rost, T. L. (2002). Toward a causal explanation of plant invasiveness: Seedling growth and life-history strategies of 29 pine (Pinus) species. Am. Nat. 159, 396–419. doi: 10.1086/338995
Harper, J. L., Rosen, B. R., and White, J. (1986). The growth and form of modular organisms in The growth and form of modular organisms. London: Royal Society.
Harrison, X. A., Donaldson, L., Correa-Cano, M. E., Evans, J., Fisher, D. N., Goodwin, C. E. D., et al. (2018). A brief introduction to mixed effects modelling and multi-model inference in ecology. PeerJ 2018, 1–32. doi: 10.7717/peerj.4794
Hobbs, R. J. (2000). “Invasive species,” in A changing world, eds H. A. Mooney and R. J. Hobbs (Washington, DC:Island Press).
Holmer, M., Marbà, N., Lamote, M., and Duarte, C. M. (2009). Deterioration of sediment quality in seagrass meadows (Posidonia oceanica) invaded by macroalgae (Caulerpa sp.). Estuar. Coasts 32, 456–466. doi: 10.1007/s12237-009-9133-4
Katsanevakis, S., Tempera, F., and Teixeira, H. (2016). Mapping the impact of alien species on marine ecosystems: The Mediterranean Sea case study. Divers. Distrib. 22, 694–707. doi: 10.1111/ddi.12429
Katsanevakis, S., Zenetos, A., Belchior, C., and Cardoso, A. C. (2013). Invading European Seas: Assessing pathways of introduction of marine aliens. Ocean Coast. Manag. 76, 64–74. doi: 10.1016/j.ocecoaman.2013.02.024
Kazi, M. A., Reddy, C. R. K., and Jha, B. (2013). Molecular Phylogeny and Barcoding of Caulerpa (Bryopsidales) Based on the tufA, rbcL, 18S rDNA and ITS rDNA Genes. PLoS One 8:0082438. doi: 10.1371/journal.pone.0082438
Keller, M. D., Selvin, R. C., Claus, W., and Guillard, R. R. L. (1987). Media for the culture of oceanic ultraphytoplankton. J. Phycol. 23, 633–638. doi: 10.1111/j.1529-8817.1987.tb04217.x
Kelly, R. P., Port, J. A., Yamahara, K. M., Martone, R. G., Lowell, N., Thomsen, P. F., et al. (2014). Harnessing DNA to improve environmental management. Science 344, 1455–1456. doi: 10.1126/science.1251156
Klein, J. C., and Verlaque, M. (2011). Experimental removal of the invasive Caulerpa racemosa triggers partial assemblage recovery. J. Mar. Biol. Assoc. UK 91, 117–125. doi: 10.1017/S0025315410000792
Klein, J., and Verlaque, M. (2008). The Caulerpa racemosa invasion: A critical review. Mar. Pollut. Bull. 56, 205–225. doi: 10.1016/j.marpolbul.2007.09.043
Kübler, J. E., and Dudgeon, S. R. (1996). Temperature dependent change in the complexity of form of Chondrus crispus fronds. J. Exp. Mar. Bio. Ecol. 207, 15–24. doi: 10.1016/S0022-0981(96)02651-2
Lamouroux, J. V. F. (1809). Mémoires sur les Caulerpes, nouveau genre de la famille des algues marines. J. Bot. 2, 136–146.
Lejeusne, C., Chevaldonné, P., Pergent-Martini, C., Boudouresque, C. F., and Pérez, T. (2010). Climate change effects on a miniature ocean: The highly diverse, highly impacted Mediterranean Sea. Trends Ecol. Evol. 25, 250–260. doi: 10.1016/j.tree.2009.10.009
Lenth, R. (2018). emmeans: Estimated Marginal Means, aka Least-Squares Means. R package version 1.3.0.
Lewis, S. M., Norris, J. N., and Searles, R. B. (1987). The Regulation of Morphological Plasticity in Tropical Reef Algae by Herbivory. Ecology 68, 636–641. doi: 10.2307/1938468
Liu, W., and Su, J. (2016). Effects of light acclimation on shoot morphology, structure, and biomass allocation of two Taxus species in southwestern China. Sci. Rep. 6, 1–9. doi: 10.1038/srep35384
Lodge, D. M., Williams, S., Macisaac, H. J., Hayes, K. R., Leung, B., Reichard, S., et al. (2006). Biological Invasions: Recommendations for U.S. Policy and Management. 2Ecological Appl. 16, 2035–2054.
Mack, R. N., Simberloff, D., Lonsdale, M. W., Evans, H., Clout, M., and Bazzaz, F. A. (2000). Biotic invasions: causes, epidemiology, global consequences and control. Ecol. Appl. 10, 689–710. doi: 10.3906/yer-1001-30
Meinesz, A., Benichou, L., Blachier, J., Komatsu, T., Lemée, R., Molenaar, H., et al. (1995). Variations in the Structure, Morphology and Biomass of Caulerpa taxifolia in the Mediterranean Sea. Bot. Mar. 38, 499–508. doi: 10.1515/botm.1995.38.1-6.499
Molina-Montenegro, M. A., Peñuelas, J., Munné-Bosch, S., and Sardans, J. (2012). Higher plasticity in ecophysiological traits enhances the performance and invasion success of Taraxacum officinale (dandelion) in alpine environments. Biol. Invasions 14, 21–33. doi: 10.1007/s10530-011-0055-2
Monro, K., and Poore, A. G. B. (2005). Light quantity and quality induce shade-avoiding plasticity in a marine macroalga. J. Evol. Biol. 18, 426–435. doi: 10.1111/j.1420-9101.2004.00826.x
Monro, K., and Poore, A. G. B. (2009). Performance benefits of growth-form plasticity in a clonal red seaweed. Biol. J. Linn. Soc. 97, 80–89. doi: 10.1111/j.1095-8312.2008.01186.x
Montefalcone, M., Morri, C., Parravicini, V., and Bianchi, C. N. (2015). A tale of two invaders: divergent spreading kinetics of the alien green algae Caulerpa taxifolia and Caulerpa cylindracea. Biol. Invasions 17, 2717–2728. doi: 10.1007/s10530-015-0908-1
Nicotra, A. B., Atkin, O. K., Bonser, S. P., Davidson, A. M., Finnegan, E. J., Mathesius, U., et al. (2010). Plant phenotypic plasticity in a changing climate. Trends Plant Sci. 15, 684–692. doi: 10.1016/j.tplants.2010.09.008
Ohba, H., and Enomoto, S. (1987). Culture studies on Caulerpa (Caulerpales, Chlorophyceae) II. Morphological variation of C. racemosa var. laetevirens under various culture conditions. Japanese J. Phycol. 35, 178–188. doi: 10.1007/BF02489433
Ohba, H., Nashima, H., and Enomoto, S. (1992). Culture Studies on Caulerpa (Caulerpales, Chlorophyceae) III. Reproduction, Deverlopment and Morphological Variation of Laboratory-cultured C. racemosa var. peltata. Bot. Mag. Tokyo 105, 589–600. doi: 10.1007/bf02489433
Paradis, E., Claude, J., and Strimmer, K. (2004). APE: Analyses of phylogenetics and evolution in R language. Bioinformatics 20, 289–290. doi: 10.1093/bioinformatics/btg412
Pejchar, L., and Mooney, H. A. (2009). Invasive species, ecosystem services and human well-being. Trends Ecol. Evol. 24, 497–504. doi: 10.1016/j.tree.2009.03.016
Peterson, R. D. (1972). Effects of Light Intensity on the Morphology and Productivity of Caulerpa racemosa. Micronesica 8, 63–86.
Piazzi, L., Balata, D., Foresi, L., Cristaudo, C., and Cineli, F. (2007). Sediment as a constituent of Mediterranean benthic communities dominated by Caulerpa racemosa var. cylindracea. Sci. Mar. 71, 129–135. doi: 10.3989/scimar.2007.71n1129
Piazzi, L., and Ceccherelli, G. (2006). Persistence of biological invasion effects: Recovery of macroalgal assemblages after removal of Caulerpa racemosa var. cylindracea. Estuar. Coast. Shelf Sci. 68, 455–461. doi: 10.1016/j.ecss.2006.02.011
Piazzi, L., Ceccherelli, G., and Cinelli, F. (2001). Threat to macroalgal diversity: effects of the introduced green alga Caulerpa racemosa in the Mediterranean. Mar. Ecol. Prog. Ser. 210, 149–159. doi: 10.3354/meps210149
Piazzi, L., Meinesz, A., Verlaque, M., Akçali, B., Antoliæ, B., Argyrou, M., et al. (2005). Invasion of Caulerpa racemosa var. cylindracea (Caulerpales, Chlorophyta) in the Mediterranean Sea: An assessment of the spread. Cryptogam. Algol 26, 189–202.
Pimentel, D., McNair, S., Janecka, J., Wightman, J., Simmonds, C., O’Connell, C., et al. (2001). Economic and environmental threats of alien plant, animal, and microbe invasions. Agric. Ecosyst. Environ. 84, 1–20. doi: 10.1016/S0167-8809(00)00178-X
Pimentel, D., Zuniga, R., and Morrison, D. (2005). Update on the environmental and economic costs associated with alien-invasive species in the United States. Ecol. Econ. 52, 273–288. doi: 10.1016/j.ecolecon.2004.10.002
Price, E. A. C., and Marshall, C. (1999). Clonal plants and environmental heterogeneity: an introduction to the proceedings. Plant Ecol. 141, 3–7. doi: 10.1023/A:1009838300691
Puijalon, S., Léna, J. P., Rivière, N., Champagne, J. Y., Rostan, J. C., and Bornette, G. (2008). Phenotypic plasticity in response to mechanical stress: hydrodynamic performance and fitness of four aquatic plant species. N. Phytol. 177, 907–917. doi: 10.1111/j.1469-8137.2007.02314.x
Pusceddu, A., Fraschetti, S., Scopa, M., Rizzo, L., and Danovaro, R. (2016). Meiofauna communities, nematode diversity and C degradation rates in seagrass (Posidonia oceanica L.) and unvegetated sediments invaded by the algae Caulerpa cylindracea (Sonder). Mar. Environ. Res. 119, 88–99. doi: 10.1016/j.marenvres.2016.05.015
Pyšek, P., and Richardson, D. M. (2008). Traits associated with invasiveness in alien plants: where do we stand? in Biological invasions. Ecological studies (Analysis and Synthesis). Berlin: Springer, 97–125.
Raniello, R., Lorenti, M., Brunet, C., and Buia, M. C. (2004). Photosynthetic plasticity of an invasive variety of Caulerpa racemosa in a coastal Mediterranean area: Light harvesting capacity and seasonal acclimation. Mar. Ecol. Prog. Ser. 271, 113–120. doi: 10.3354/meps271113
Raniello, R., Lorenti, M., Brunet, C., and Buia, M. C. (2006). Photoacclimation of the invasive alga Caulerpa racemosa var. cylindracea to depth and daylight patterns and a putative new role for siphonaxanthin. Mar. Ecol. 27, 20–30. doi: 10.1111/j.1439-0485.2006.00080.x
Reynolds, R. W., Smith, T. M., Liu, C., Chelton, D. B., Casey, K. S., and Schlax, M. G. (2007). Daily high-resolution-blended analyses for sea surface temperature. J. Clim. 20, 5473–5496. doi: 10.1175/2007JCLI1824.1
Richards, C. L., Bossdorf, O., Muth, N. Z., Gurevitch, J., and Pigliucci, M. (2006). Jack of all trades, master of some? On the role of phenotypic plasticity in plant invasions. Ecol. Lett. 9, 981–993. doi: 10.1111/j.1461-0248.2006.00950.x
Richardson, D. M., Pyšek, P., and Carlton, J. T. (2011). “A Compendium of Essential Concepts and Terminology In Invasion Ecology,” in Fifty years of invasion ecology. The legacy of Charles Elton. Hoboken, NJ: Wiley. ed. D. M. Richardson 409. doi: 10.1002/9781444329988.ch30
Rizzo, L., Pusceddu, A., Stabili, L., Alifano, P., and Fraschetti, S. (2017). Potential effects of an invasive seaweed (Caulerpa cylindracea, Sonder) on sedimentary organic matter and microbial metabolic activities. Sci. Rep. 7, 1–10. doi: 10.1038/s41598-017-12556-4
Sauvage, T., Payri, C., Draisma, S. G. A., Prud’homme Van, Reine, W. F., Verbruggen, H., et al. (2013). Molecular diversity of the Caulerpa racemosa - Caulerpa peltata complex (Caulerpaceae, Bryopsidales) in New Caledonia, with new Australasian records for C. racemosa var. cylindracea (Phycologia (2013) 52:1 (6-13)). Phycologia 52, 6–13. doi: 10.2216/0031-8884-52.2.222
Schaffelke, B., Smith, J. E., and Hewitt, C. L. (2006). Introduced macroalgae - A growing concern. J. Appl. Phycol. 18, 529–541. doi: 10.1007/s10811-006-9074-2
Schlegel, R. W., and Smit, A. J. (2018). heatwaveR: A central algorithm for the detection of heatwaves and cold-spells. J. Open Source Softw. 3:821. doi: 10.21105/joss.00821
Schliep, K., Potts, A. J., Morrison, D. A., and Grimm, G. W. (2017). Intertwining phylogenetic trees and networks. Methods Ecol. Evol. 8, 1212–1220. doi: 10.1111/2041-210X.12760
Seebens, H., Gastner, M. T., and Blasius, B. (2013). The risk of marine bioinvasion caused by global shipping. Ecol. Lett. 16, 782–790. doi: 10.1111/ele.12111
Simberloff, D., Martin, J. L., Genovesi, P., Maris, V., Wardle, D. A., Aronson, J., et al. (2013). Impacts of biological invasions: What’s what and the way forward. Trends Ecol. Evol. 28, 58–66. doi: 10.1016/j.tree.2012.07.013
Simberloff, D., Parker, I. M., and Windle, P. N. (2005). Introduced species policy, management, and future research needs. Front. Ecol. Environ. 3:12–20. doi: 10.2307/3868440
Simmons, M., Tucker, A., Chadderton, W. L., Jerde, C. L., and Mahon, A. R. (2015). Active and passive environmental DNA surveillance of aquatic invasive species. Can. J. Fish. Aquat. Sci. 73, 76–83. doi: 10.1139/cjfas-2015-0262
Smith, C. M., and Walters, L. J. (1999). Fragmentation as a Strategy for Caulerpa Species: Fates of Fragments and Implications for Management of an Invasive Weed. Mar. Ecol. 20, 307–319. doi: 10.1046/j.1439-0485.1999.2034079.x
Smith, L. D. (2009). The Role of Phenotypic Plasticity in Marine Biological Invasions. Biol. Invas. Mar. Ecosyst. 2009, 177–202. doi: 10.1007/978-3-540-79236-9_10
Stachowicz, J. J., and Byrnes, J. E. (2006). Species diversity, invasion success, and ecosystem functioning: Disentangling the influence of resource competition, facilitation, and extrinsic factors. Mar. Ecol. Prog. Ser. 311, 251–262. doi: 10.3354/meps311251
Stachowicz, J. J., Terwin, J. R., Whitlatch, R. B., and Osman, R. W. (2002). Linking climate change and biological invasions: ocean warming facilitates nonindigenous species invasions. Proc. Natl. Acad. Sci. U. S. A. 99, 15497–15500. doi: 10.1073/pnas.242437499
Taberlet, P., Coissac, E., Hajibabaei, M., and Rieseberg, L. H. (2012). Environmental DNA. Mol. Ecol. 21, 1789–1793. doi: 10.1111/j.1365-294X.2012.05542.x
Theoharides, K. A., and Dukes, J. S. (2007). Plant invasion across space and time: factors affecting nonindigenous species success during four stages of invasion. N. Phytol. 176, 256–273. doi: 10.1111/j.1469-8137.2007.02207.x
Thomsen, P. F., and Willerslev, E. (2015). Environmental DNA - An emerging tool in conservation for monitoring past and present biodiversity. Biol. Conserv. 183, 4–18. doi: 10.1016/j.biocon.2014.11.019
Van Kleunen, M., and Fisher, M. (2001). Adaptive evolution of plastic foraging responses in a clonal plant. Ecology 82, 3309–3319. doi: 10.1890/0012-96582001082[3309:AEOPFR]2.0.CO;2
Van Kleunen, M., Weber, E., and Fischer, M. (2010). A meta-analysis of trait differences between invasive and non-invasive plant species. Ecol. Lett. 13, 235–245. doi: 10.1111/j.1461-0248.2009.01418.x
Vander Zanden, M. J., Hansen, G. J. A., Higgins, S. N., and Kornis, M. S. (2010). A pound of prevention, plus a pound of cure: early detection and eradication of invasive species in the Laurentian Great Lakes. J. Great Lakes Res. 36, 199–205. doi: 10.1016/j.jglr.2009.11.002
Verlaque, M., Afonso-Carrillo, J., Candelaria Gil-Rodríguez, M., Durand, C., Boudouresque, C. F., and Le Parco, Y. (2004). Blitzkrieg in a marine invasion: Caulerpa racemosa var. cylindracea (Bryopsidales, Chlorophyta) reaches the Canary Islands (North-East Atlantic). Biol. Invasions 6, 269–281. doi: 10.1023/b:binv.0000034589.18347.d3
Verlaque, M., Durand, C., Huisman, J. M., Boudouresque, C. F., and Le Parco, Y. (2003). On the identity and origin of the Mediterranean invasive Caulerpa racemosa (Caulerpales, Clorophyta). Eur. J. Phycol. 38, 325–339. doi: 10.1080/09670260310001612592
Vilà, M., Basnou, C., Pyšek, P., Josefsson, M., Genovesi, P., Gollasch, S., et al. (2010). How well do we understand the impacts of alien species on ecosystem services? A pan-European, cross-taxa assessment. Front. Ecol. Environ. 8:135–144. doi: 10.1890/080083
Womersley, H. B. S. (1984). The marine benthic flora of Southern Australia-Part I, ed. D. J. Woolman (South Australia: Department of botany, University of Adelaide).
Wynne, M. J., and Bold, H. C. (1985). Introduction to the algae: structure and reproduction. Upper Saddle River, NJ: Prentice-Hall, Incorporated.
Zanolla, M., Altamirano, M., Carmona, R., De La Rosa, J., Sherwood, A., and Andreakis, N. (2015). Photosynthetic plasticity of the genus Asparagopsis (Bonnemaisoniales, Rhodophyta) in response to temperature: implications for invasiveness. Biol. Invasions 17, 1341–1353. doi: 10.1007/s10530-014-0797-8
Keywords: cryptic invasions, morphological plasticity, resistance form, Mediterranean Sea, Caulerpa cylindracea
Citation: Santamaría J, Golo R, Cebrian E, García M and Vergés A (2021) Stressful Conditions Give Rise to a Novel and Cryptic Filamentous Form of Caulerpa cylindracea. Front. Mar. Sci. 8:548679. doi: 10.3389/fmars.2021.548679
Received: 03 April 2020; Accepted: 11 January 2021;
Published: 18 February 2021.
Edited by:
Nuria Marba, Consejo Superior de Investigaciones Científicas (CSIC), SpainReviewed by:
Anwesha Ghosh, Indian Institute of Science Education and Research Kolkata, IndiaMads Solgaard Thomsen, University of Canterbury, New Zealand
Copyright © 2021 Santamaría, Golo, Cebrian, García and Vergés. This is an open-access article distributed under the terms of the Creative Commons Attribution License (CC BY). The use, distribution or reproduction in other forums is permitted, provided the original author(s) and the copyright owner(s) are credited and that the original publication in this journal is cited, in accordance with accepted academic practice. No use, distribution or reproduction is permitted which does not comply with these terms.
*Correspondence: Jorge Santamaría, am9yZ2Uuc2FudGFtYXJpYUB1ZGcuZWR1