- 1Israel Oceanographic and Limnological Research, Haifa, Israel
- 2Leon H. Charney School of Marine Sciences, The Leon Recanati Institute for Maritime Studies, University of Haifa, Haifa, Israel
- 3Department of Earth and Planetary Sciences, Weizmann Institute of Science, Rehovot, Israel
Submarine groundwater discharge (SGD) has been shown to be an important source of nutrients in coastal environments, especially nitrogen and silica, and thereby relive nutrient limitation to phytoplankton. Here, we followed autotrophic microbial biomass, activity, and community composition at a site strongly influenced by SGD and a nearby nutrients-poor reference site at the oligotrophic Israeli shallow rocky coast [southeastern Mediterranean Sea (SEMS)] between 2011 and 2019. The surface water at the SGD-affected area had significantly higher NO3 + NO2 (∼10-fold) and Si(OH)4 (∼2-fold) levels compared to the reference site, while no significant differences were observed for PO4 or NH4. This resulted in a significant increase in algae biomass (∼3.5-fold), which was attributed to elevated Synechococcus (∼3.5-fold) and picoeukaryotes (∼2-fold) at the SGD-affected site, and in elevated primary production rates (∼2.5-fold). Contrary to most SGD-affected coastal areas, diatoms biomass remained unchanged between sites, despite the elevated N and Si, suggesting the dominance of picophytoplankton over microphytoplankton at the SEMS. DNA sequencing of the 16S and 18S rDNA supported these findings. These results highlight the influence of SGD on shallow-water microbial populations. Our observations are consistent with recent studies showing that phytoplankton along the Israeli coast are likely nitrogen + silica limited, and may have important ecological and regulatory implications for environmental policy and management of coastal aquifers.
Introduction
The southeastern Mediterranean Sea (SEMS) is an impoverished environment (Siokou-Frangou et al., 2010; Berman-Frank and Rahav, 2012). Phytoplankton are mainly inorganic nitrogen and phosphorus co-limited (Kress et al., 2005; Zohary et al., 2005), while heterotrophic bacteria are limited by PO4 (Tanaka et al., 2007; Tsiola et al., 2016) or by dissolved organic carbon (Hazan et al., 2018; Rahav et al., 2019). Consequently, phytoplankton biomass is low and dominated mostly by cyanobacterial species (Mella-Flores et al., 2011; Rahav et al., 2013; Hazan et al., 2018) and the annual primary productivity rates are among the lowest in the world (Psarra et al., 2000; Siokou-Frangou et al., 2010; Berman-Frank and Rahav, 2012). Contrary to the offshore regions in the SEMS, higher nutrient levels are often observed in nearshore areas (Herut et al., 2000a; Kress et al., 2019), which may lead to higher phytoplankton/bacterial biomass and activity (Raveh et al., 2015; Rahav et al., 2018b), with different nutrient limitations (Rahav et al., 2016a, 2018b; Sisma-Ventura and Rahav, 2019). Nutrient enrichment of coastal waters occurs through inputs from estuaries (Herut et al., 2000b; Kress and Herut, 2001) and other coastal streams (Krom et al., 2014), sporadic terrestrial runoff (Rahav and Bar-Zeev, 2017), aerosol deposition (Rahav et al., 2016b, 2018a), and other anthropogenic inputs (Belkin et al., 2017; Frank et al., 2019; Raveh et al., 2019), as well as submarine groundwater discharge (SGD) (Garcés et al., 2011; Weinstein et al., 2011; Rodellas et al., 2015). Among these external nutrient inputs, the effect of SGD on phytoplankton biomass/activity has been far less studied in Israel coastal waters (SEMS), despite being a potentially important source of nutrients (Weinstein et al., 2011). SGD is enriched in allochthonous nutrients (e.g., nitrogen, phosphorous, and silica) and micronutrients (e.g., iron, nickel, and zinc) (Lecher and Mackey, 2018; Wang et al., 2018). A previous study demonstrated that the yearly N, P, and Si inputs from SGD to the Mediterranean Sea is comparable to riverine and atmospheric inputs combined (Rodellas et al., 2015), suggesting this process may have a significant impact on primary production in coastal waters.
In ultra-oligotrophic regimes, such as the SEMS, external inputs of limiting nutrients from SGD or other sources may greatly affect phytoplankton and bacterial communities, as well as the specific interactions between them. This is especially true given that recent studies demonstrated that N and Si additions (either as pure nutrients or as well-amelioration brines) may lead to cyanobacterial and phytoplankton proliferation in the SEMS coast (Rahav et al., 2018b; Raveh et al., 2019). This suggests that SGD, which is also rich in these compounds, is likely to lead to similar responses.
The aim of this study was to investigate the in situ responses of autotrophic microbial populations to nutrient enrichment by SGD at shallow rocky coastal water sites in the SEMS. Rocky shores are “biological hotspots” comprising ∼33% of the Israeli coast (Rilov, 2016), and are therefore of great ecological value. Surface waters from two rocky-shore sites; Achziv (highly affected by SGD; Weinstein et al., 2011; Paldor et al., 2019) and Tel-Shikmona (an oligotrophic environment with little SGD input) were sampled between 2013 and 2016. Inorganic nutrient concentrations, phytoplankton biomass, phytoplankton taxonomic identification, and primary production were recorded for the purpose of a comparison among these two sites. Additional “background” characterization of the sites included total alkalinity (TA) and inorganic nutrient measurements that were carried out monthly, from 2011 to 2019 at these two sites by the Israeli National Monitoring Program in Mediterranean waters (Herut et al., 2020). These time series were used to demonstrate the difference in surface seawater chemical signatures of SGD inputs in similar proximity to the shoreline at both sites.
Materials and Methods
Sampling Design
Surface seawater samples (∼0.5 m) were collected from two coastal sites along the northern Israeli shore; Tel-Shikmona (Lat. 32° 49′34 N, Lon. 34° 57′20 E) and Achziv (Lat. 33° 3′52 N, Lon. 35° 6′14.94 E) by diving (Figure 1). Water chemistry (NO2 + NO3, Si(OH)4, PO4, and TA) was measured at the edge of the rocky shore in Achziv and Tel Shikmona on a monthly basis during the period 2011–2019 by the Israeli National Monitoring Program of Mediterranean waters. Together with measurements of salinity and NOx that were measured in May-June 2005 we were able to pinpoint the intensified nitrate-rich SGD location in Achziv and to choose an apparently less enriched site in Tel-Shikmona (Figure 1). Finally, dedicated water sampling for phytoplankton biomass/abundance, production and taxonomic identification was conducted during April 2014, March 2015, May 2015, October 2015, and March 2016. Surface water was collected from up to six locations at both sites (i.e., Achziv or Tel-Shikmona) during each sampling campaign.
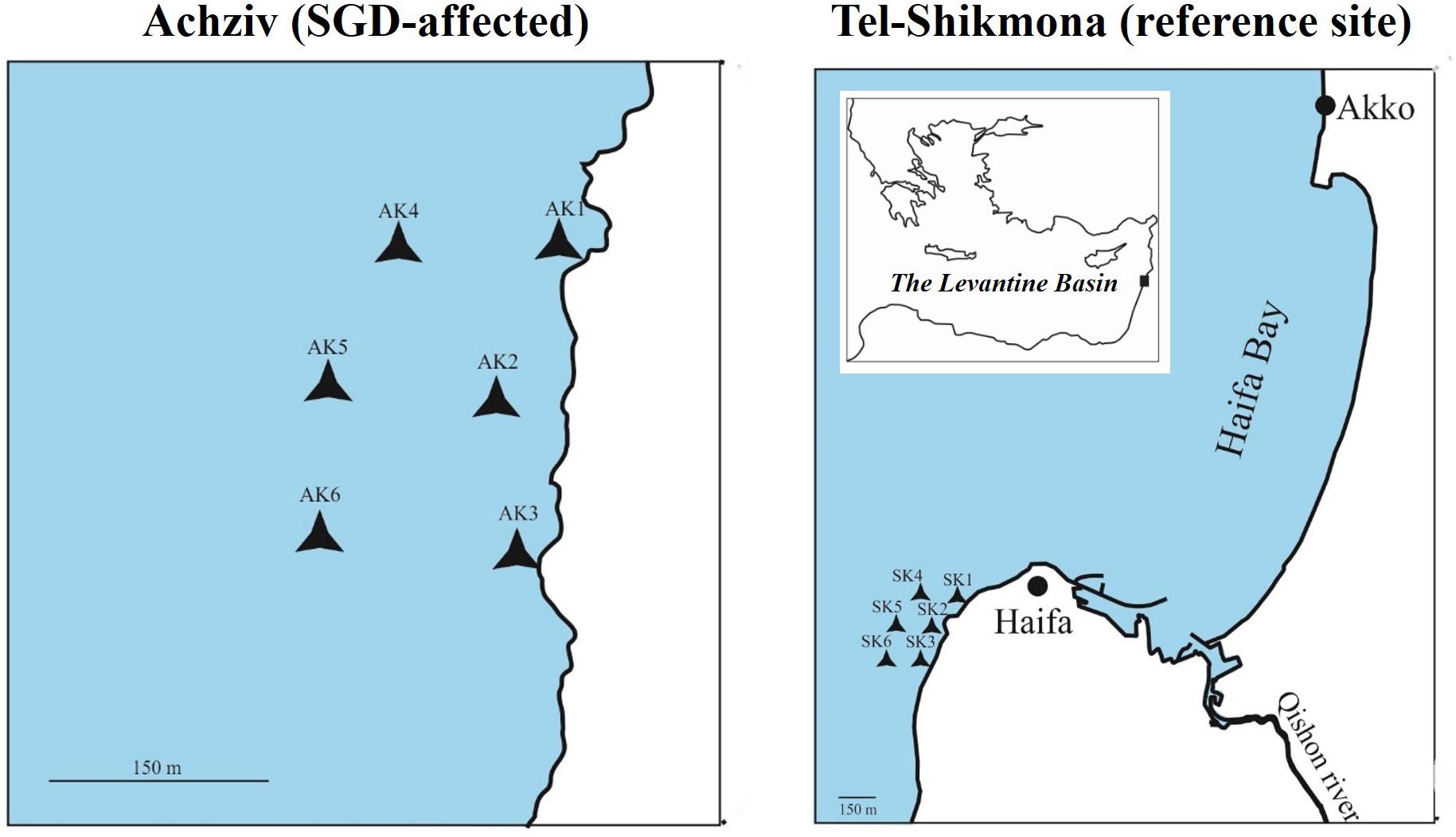
Figure 1. Map showing the two sampling areas at the Israeli coastline in the SE Mediterranean Sea. In each site, 6 surface water samples were collected by diving. The Achziv sampling stations (left panel) are considered to be strongly influenced by nutrient inputs from SGD year round, and the Tel-Shikmona stations (right panel) are less influenced by SGD nutrient input throughout the annual cycle. Scale bars within the expanded regions are 150 m.
Physical and Chemical Properties
Temperature, salinity, and pH were measured at the time of sampling using a handheld WTW multiline 3410 sensor (WTW, Germany). Irradiance was measured at the sampling site using an Onset UA-002-64 instrument. Filtered (0.45 μm Minisart filters) seawater samples were collected at the study sites and the long term monitoring stations using acid-rinsed plastic vials and stored frozen for later analysis in the lab. These samples were analyzed for NO2 + NO3 (NOx), NH4, PO4, and Si(OH)4 using a segmented flow Seal Analytical AA-3 system (Sisma-Ventura and Rahav, 2019). The limits of detection for NOx, NH4, PO4, and Si(OH)4 were 0.08, 0.09, 0.008, and 0.03 μM, respectively. In addition, filtered (0.45 μm Minisart filters) seawater samples from the long term (2011–2019) national monitoring stations (AKE and SKE) were collected monthly from the sea surface into brown glass bottles that were refrigerated until analysis in the lab within a few days of collection. These samples were analyzed for density and TA. Analyses of TA samples were done with a Methrom 785 Titrino Plus titration system with ∼0.05 N HCl, based on the analytical procedures and calculations described by Sass and Ben-Yaakov (1977). TA measurements were calibrated and standardized using seawater CRMs from A. Dickson’s lab (Dickson et al., 2003). The precision of TA measurements was ±2 μmol/kg. Seawater density measurements were made with a 6-digit accuracy Anton Paar DMA-5000 densitometer at a known temperature (3 decimal places). Salinity values were calculated from the density data at the measurement temperature using the equation of state for seawater.
Trace Elements
Groundwater, seawater, and discharged groundwater were collected, filtered through 0.45 μm and acidified to pH∼2 with NHO3. Sr and Ba concentrations were analyzed by inductively coupled plasma–mass spectrometry (Agilent 7700s ICP-MS). The uncertainties of the method were <5%.
Chlorophyll a
Particulate matter in seawater samples (300 ml) collected at the study sites and monitoring stations was concentrated onto 25 mm glass fiber filters (Whatman) using low vacuum (<150 mmHg) immediately after sampling. Chlorophyll (Chl a) was extracted from these filters using 90% acetone overnight (Welschmeyer, 1994). After extraction, fluorescence was measured with a Turner Designs Trilogy® fluorometer at 436 nm excitation and 680 nm emission.
Cyanobacterial and Picoeukaryotes Abundances
Seawater samples (1.8 ml) were fixed with cytometry-grade glutaraldehyde (Sigma G-7651, 0.02% final concentration), snap-frozen in liquid nitrogen, and kept at −80°C until analysis. Prior to analysis, the samples were fast-thawed at 37°C in a water bath (Marie et al., 1997). Autotrophic microbes were enumerated by an Attune flow cytometer (Attune acoustic flow-cytometer, 100 μl min–1). Cell discrimination was done based on side-scatter, forward scatter, red fluorescence (Chl a, 630 nm), and orange fluorescence (phycoerythrin, 585 nm, only cyanobacteria).
Pigment Markers Analyses
High Performance Liquid Chromatography (HPLC) was used to quantify the relative biomass of diatoms and dinoflagellates in the samples based on biomarker photo-pigment concentrations (fucoxanthin and peridinin, respectively). Water samples (∼10 L) were filtered through Whatman GF/F filters and kept frozen (−20°C) in dark until analysis. Frozen filters were thawed over ice in screw capped glass tubes and extracted with 2 ml of 90% acetone overnight. The filters were homogenized under subdued light using a stainless steel spatula and then sonicated in an ultrasonic bath (SYMPHONY) for 5 min (Zapata et al., 2000). Following a further extraction of 24 h at 4°C, the extracts were filtered through a 0.45 μm Teflon syringe filter (Thermo) and transferred into glass HPLC vials. The extracts (1 ml) were analyzed using an Agilent 1220 HPLC system equipped with a diode array and fluorescence detectors. Pigments were analyzed using a 40 min Ethyl acetate methanol gradient method (Jeffrey et al., 1997). Selected pigment standards (DHI Labs) were used for verification of the spectra and retention times. The data presented are the relative peak areas (mAU’s) of fucoxanthin or peridinin out of the total Chl a area.
Primary Production (PP)
Photosynthetic carbon fixation rates were measured using 14C incorporation (Steemann-Nielsen, 1952). Seawater samples (50 ml) were collected in triplicates into transparent polycarbonate bottles during the morning (∼08:00), spiked with 5 μCi of NaH14CO3 (Perkin Elmer, specific activity 1 mCi ml–1) and incubated under ambient light and temperature for 4 h. Blank (dark) runs were also conducted. Incubations were terminated by concentrating the particulate matter onto GF/F filters and removing unbound inorganic carbon by exposing the filters to 32% HCl vapors overnight. The total radioactivity was determined by liquid scintillation counting (Packard Tri carb 2100 TR liquid scintillation analyzer).
DNA Extraction, PCR Amplification and Sequence Processing
Water samples (4L) were filtered onto Sterivex filters (0.22 μm, Merck) using a peristaltic pump and placed in a sterile DNase/RNase Free Whirl-Pak bag. Lysis buffer (1.2 ml) was added to the filters before these were sealed and stored at −80°C until analyses. DNA extractions were done directly from the Sterivex filters as previously described in Raveh et al. (2019), followed by amplification and sequencing of the 16S and 18S rRNA gene regions. Prokaryote 16S rRNA genes (V4–V5 hypervariable region) were amplified using the 16S rRNA gene V4 variable region PCR primers 515F (Caporaso et al., 2011). Eukaryotic 18S rRNA genes were amplified using the universal primers Euk-A (Medlin et al., 1988) targeting the gene V3 variable region with barcode on the forward primer in a 28 cycle PCR using the HotStarTaq Plus Master Mix Kit (Qiagen, United States) under the following conditions: 94°C for 3 min, followed by 28 cycles of 94°C for 30 s, 53°C for 40 s, and 72°C for 1 min, after which a final elongation step at 72°C for 5 min was performed. After amplification, PCR products were checked in 2% agarose gel to determine the success of amplification and the relative intensity of bands. Pooled and purified PCR products were used to prepare illumina DNA libraries. Sequencing was performed at MR DNA1 (Shallowater, TX, United States) on a MiSeq system following the manufacturer’s guidelines. Sequence data were processed using MR DNA analysis pipeline (MR DNA, Shallowater, TX, United States). Reads were truncated based on quality plots, checked for chimeras, merged and grouped into operational taxonomic units (OTUs) using the QIIME platform. OTUs were defined by clustering at 3% divergence (97% similarity). Final OTUs were taxonomically classified using BLASTn against a curated database derived from RDPII and NCBI2. All sequences were deposited in NCBI reference number PRJNA671735.
Statistical Analyses
The differences between the chemical and biological characteristics of the surface seawater in Tel-Shikmona and Achziv were evaluated using a two-tailed student’s t-test (α = 0.05) for each sampling event and then pooled together in a box-plot for visualization. Prior to the analyses, the normality and the heterogeneity of variances of the data were examined. Statistical testing was done using SPSS or XLSTAT software.
Results
Physical and Chemical Characteristics of the Study Sites
NOx and Si(OH)4 concentrations were ∼10-fold and ∼2-fold higher at Achziv site compared to the Tel-Shikmona site, respectively (Figures 2A,B and Supplementary Figure 1). Similarly, the averaged TA was higher at Achziv (2,573–2,749 μmole kg–1) than in Tel-Shikmona (2,523–2,736 μmole kg–1) by 35 ± 14 μmole kg–1 (Figure 2C and Supplementary Figure 1), indicating the stronger influence of alkalinity-enriched groundwater at the Achziv site. While there was very little difference between PO4 (Figure 2D and Supplementary Figure 1) or NH4 (Table 1) levels at the sites, there were 10-fold higher NOx:PO4 ratios (∼212:1 vs. 20:1) and fivefold higher NOx:Si(OH)4 ratios (3.4:1 vs. 0.7:1) in Achziv than in Tel-Shikmona, respectively. There was notable seasonal variability at the Achziv site, with a drop in TA and Si(OH)4 from January till May and then increasing again until December. Similarly, NOx, decreased from January until July and then increased again until December (Supplementary Figure 1).
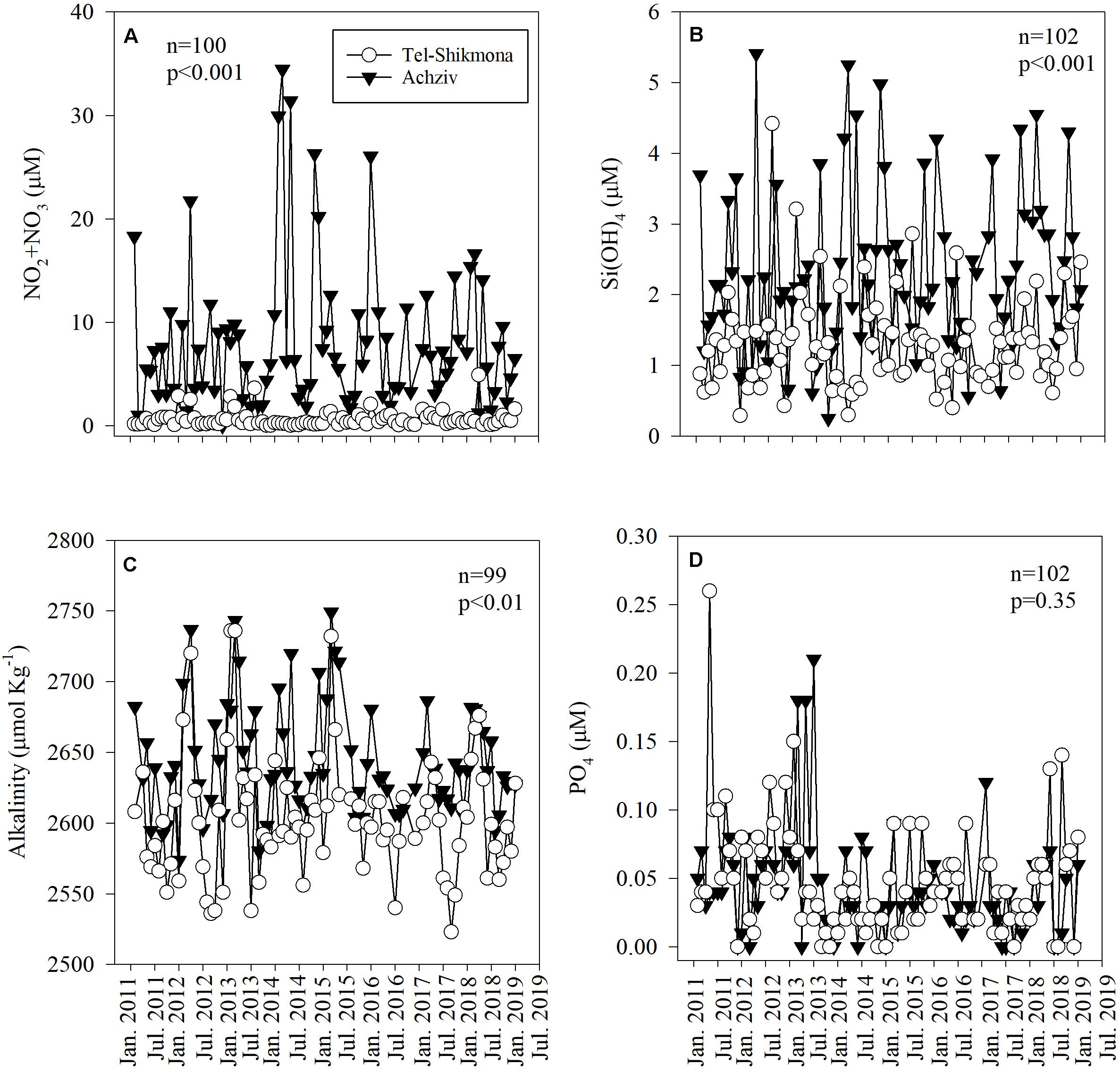
Figure 2. The temporal variability of NOx (A), Si(OH)4 (B), total alkalinity normalized to a constant salinity of 39.234 PSU (multi annual average salinity measured at both sites) (C), and PO4 (D) collected by the Israeli National Monitoring Program in the surface water in Achziv (black triangle) and Tel Shikmona (white circle) during the period 2011–2019.
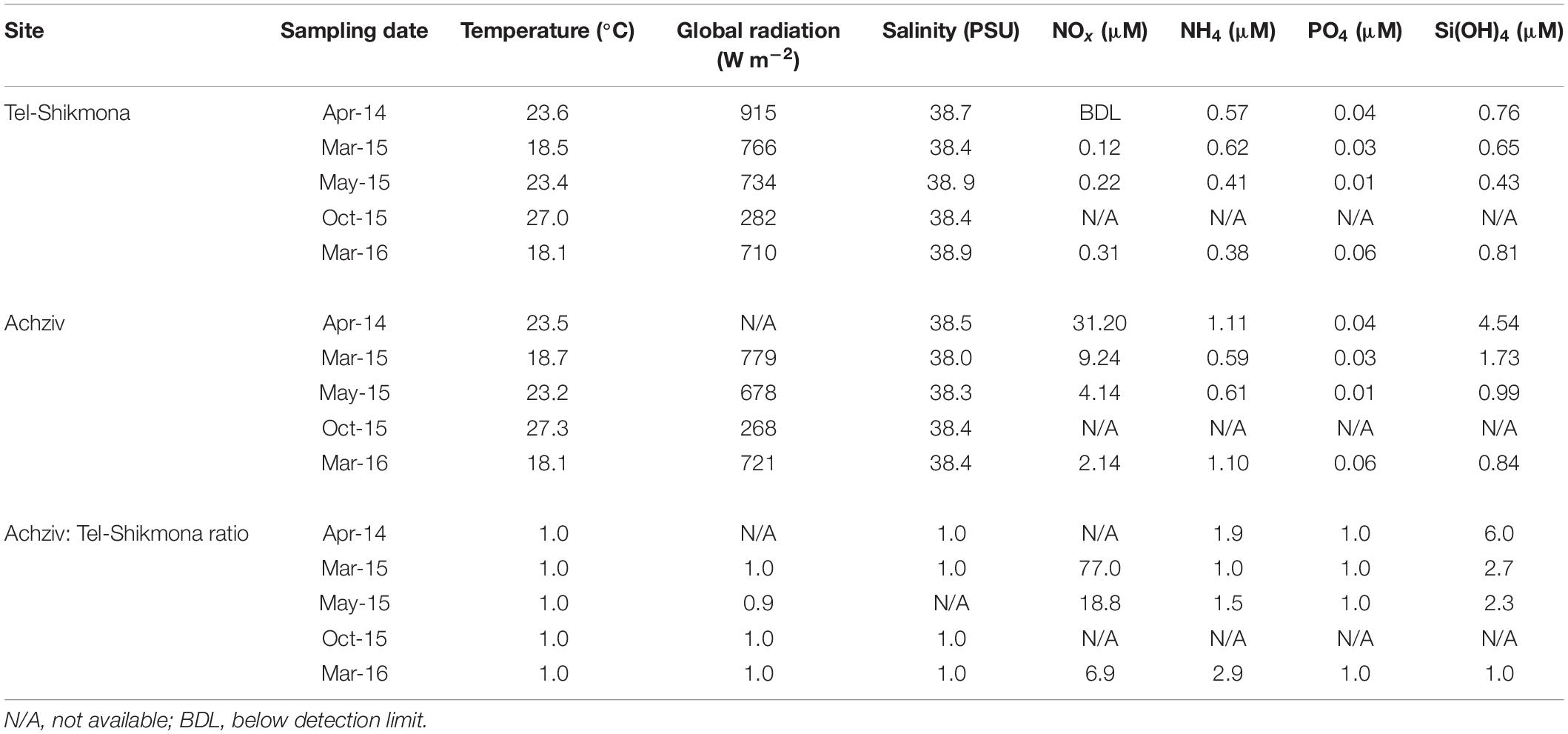
Table 1. Temperature, radiation, and salinity levels in surface waters (∼0.5 m) of Achziv and Tel-Shikmona during the phytoplankton collection campaigns, and the calculated ratio between variables.
At both sites, the highest water temperature was measured in the fall (∼27°C, October 2015), whereas the lowest temperature was measured at the end of winter (∼18°C, March 2015 and March 2016) (Table 1). Global radiation levels were overall high during most samplings (∼700 W m–2), except for October 2015 where lower values were recorded due to cloudy conditions (∼250 W m–2) (Table 1). A significant inverse relationship between NOx and salinity was observed during a tidal cycle at a shoreline location in Achziv (r2 = 0.74, p < 0.01, Figure 3A), while a direct-linear relationship was observed between NOx and alkalinity (r2 = 0.51, p < 0.01, Figure 3B). Moreover, trace element analysis of Sr and Ba collected from the discharge point at Achziv were higher relative to Tel-Shikmona (Figure 4), indicating a significant SGD signal at Achziv (Moore, 1996; Trezzi et al., 2017). In contrast, no such relationship was found in Tel-Shikmona (Figures 3A,B), suggesting little/no SGD input near our sampling sites.
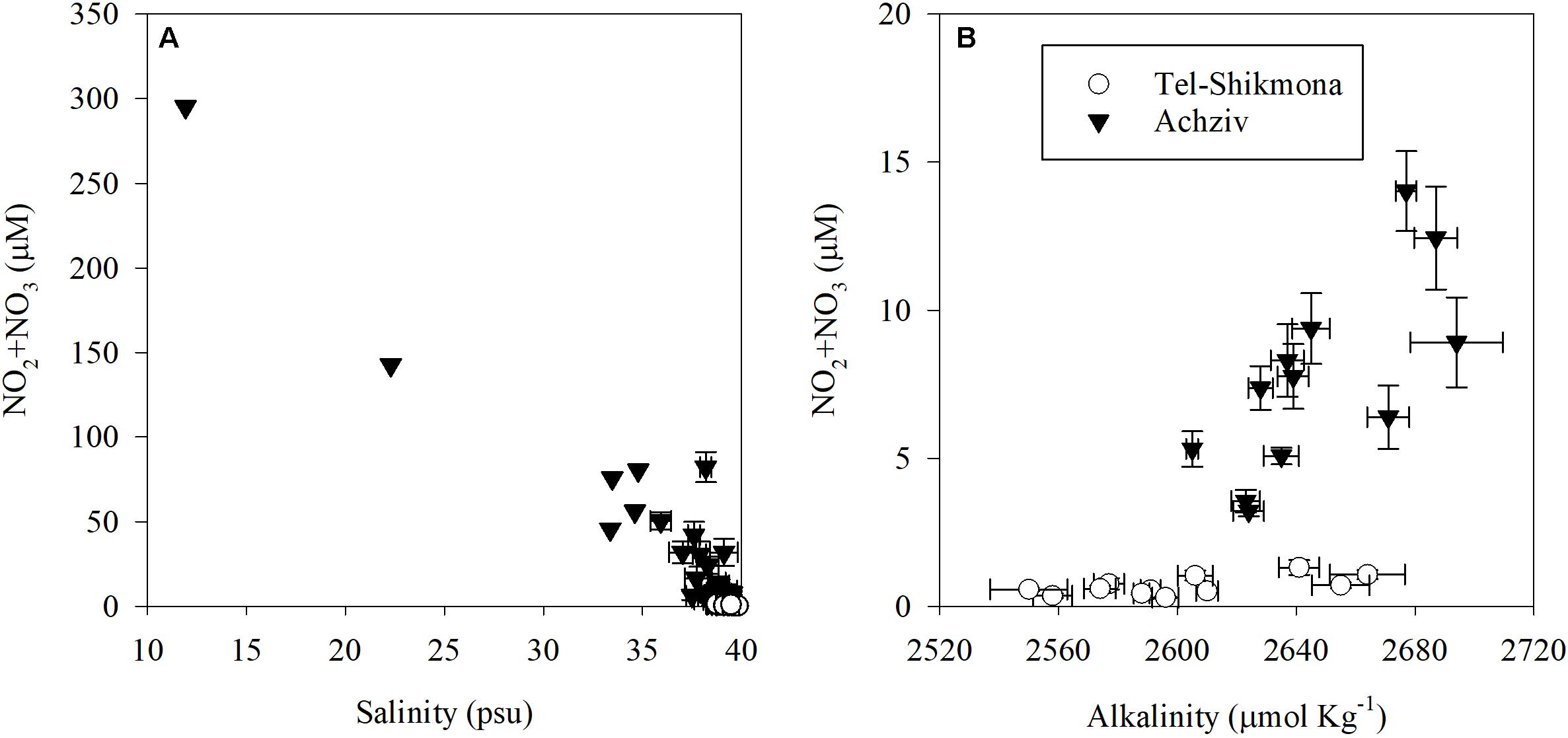
Figure 3. Relationships between NO2 + NO3 concentrations and salinity [R = 0.86, p < 0.01, (A)] or total alkalinity [R = 0.71, p < 0.01, (B)] at a shoreline location in Achziv (black triangle) and Tel-Shikmona (while circle). Values presented in panel (A) show a tidal time series measured during May and June 2005, April 2014, March 2015, May 2015, October 2015 and March 2016, and monthly averages between 2011 and 2019. Data shown in panel (B) was derived from multiannual monthly averages measured in the period 2011–2019. Note the different scales of the Y-axis between panels.
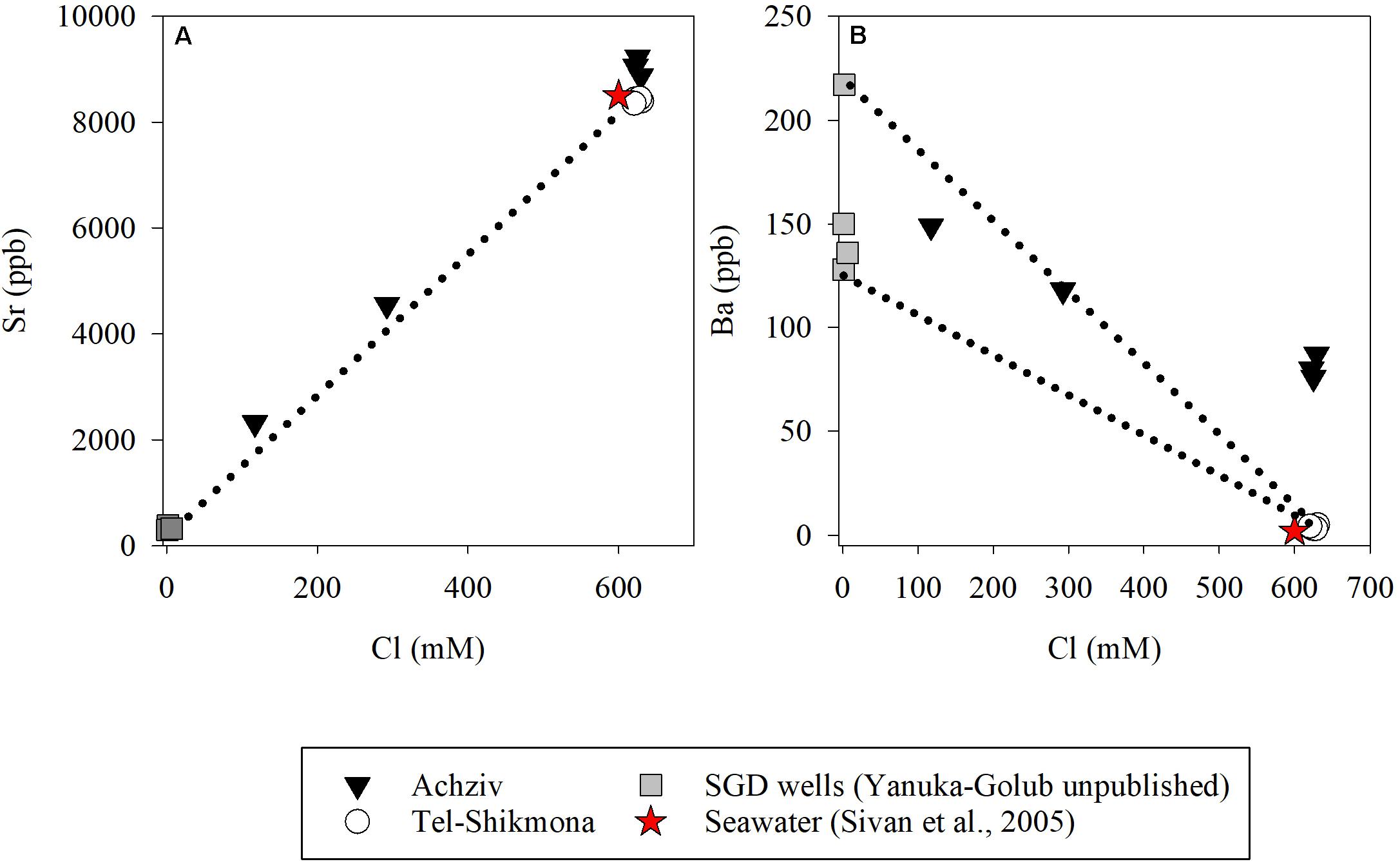
Figure 4. Concentrations of Sr (A) and Ba (B) vs. Cl in Achziv (black triangle) and Tel-Shikmona (while circle). Gray squares show values measured in SGD wells near Achziv (Yanuka-Golub, unpublished) and the red star indicate a seawater mean value based on Sivan et al. (2005) at the Israeli coast. Dash lines indicates the freshwater-seawater mixing line (Sivan et al., 2005).
Phytoplankton Abundance, Composition, and Activity at Achziv and Tel-Shikmona
Phytoplankton biomass, derived from Chl a measurements, was consistently higher at Achziv than at Tel-Shikmona (median 0.69 vs. 0.19 μg L–1, respectively, Figure 5A), as were cyanobacteria (Synechococcus + Prochlorococcus, median ∼14,000 vs. ∼6,500 cells ml–1, respectively, Figure 5B) and picoeukaryotes (median ∼4,000 vs. ∼2,000 cells ml–1, respectively, Figure 5C). Microphytoplankton biomass, determined by pigment markers, exhibited a different trend than that of the picophytoplankton (Figures 5D,E). Peridinin, a pigment marker of dinoflagellates, was significantly lower in Achziv than in Tel-Shikmona (median ∼5 vs. ∼24% of total Chl a, respectively, Figure 5D), while fucoxanthin, a pigment marker of diatoms, showed no difference between the sites (∼50% of total Chl a, Figure 5E). Concurrent with the overall differences in phytoplankton biomass and composition between sites, primary production rates were lower in Tel-Shikmona (median 0.19 μg C L–1 h–1) than in Achziv (median 0.52 μg C L–1 h–1) throughout the study (Figure 5F).
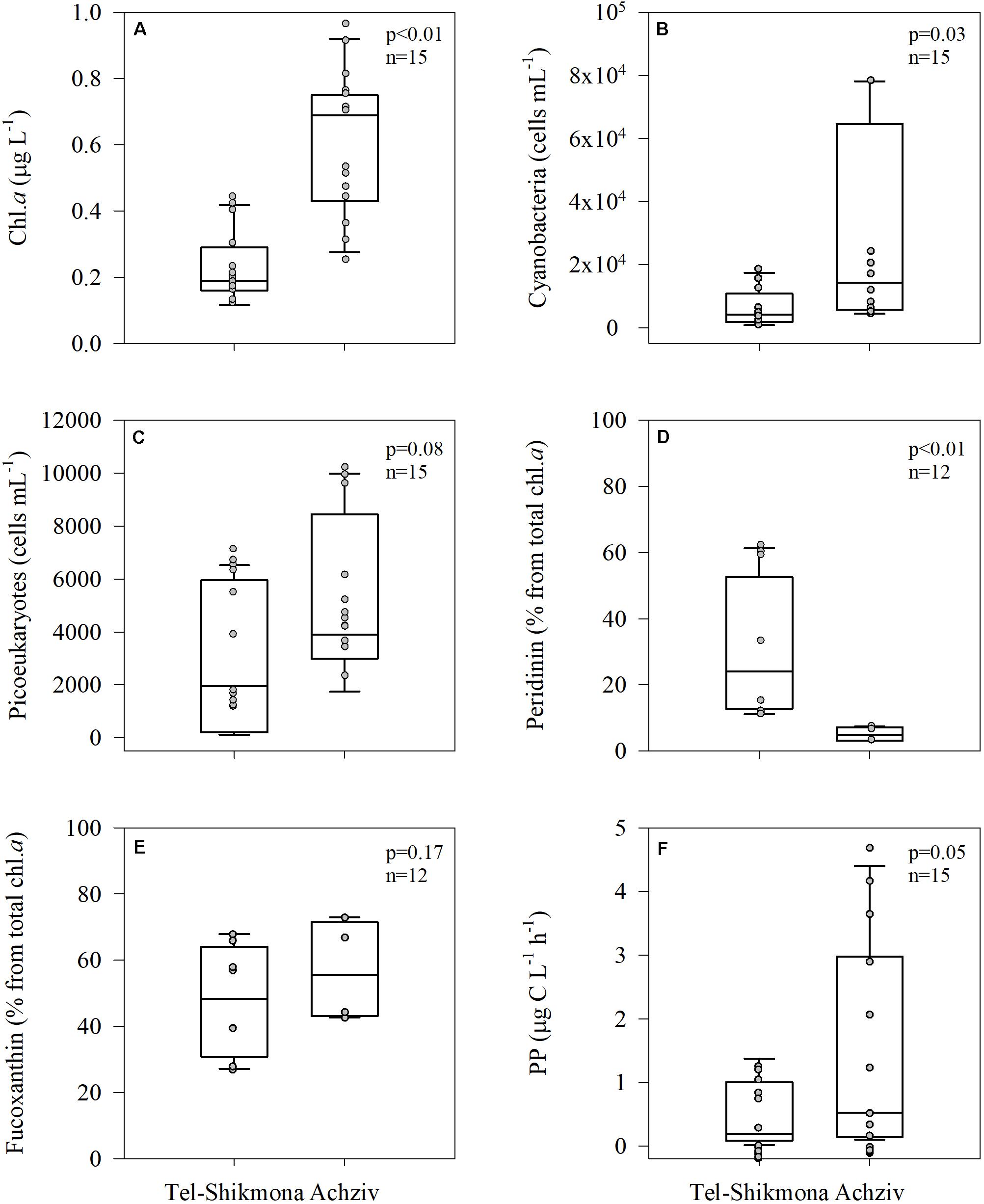
Figure 5. Box-Whisker plots showing the interquartile range (25th to 75th percentile) and median value (horizontal line within the box) of chlorophyll a (A), cyanobacterial abundance (B), picoeukaryotes abundance (C), peridinin (D), fucoxanthin (E), and primary production (PP) (F) in Tel-Shikmona and Achziv between 2013 and 2016. The measured values used to generate the box-plots are shown in gray.
DNA sequencing of the 16S and 18S rDNA showed insignificant differences in the relative OTU abundances of cyanobacteria, diatoms (Bacillariophyta), or dinoflagellates (Alveolates) at both sites (Figure 6). Nevertheless, the mean relative abundances of cyanobacteria and Bacillariophyta were higher in Achziv than in Tel-Shikmona (Figures 6A,B; 19 ± 17% vs. 11 ± 9% and 12 ± 17% and 5 ± 3%, respectively), and the relative abundance of dinoflagellates was higher at Tel-Shikmona (Figure 6C; 26% ± 35 vs. 7 ± 7%) than at Achziv.
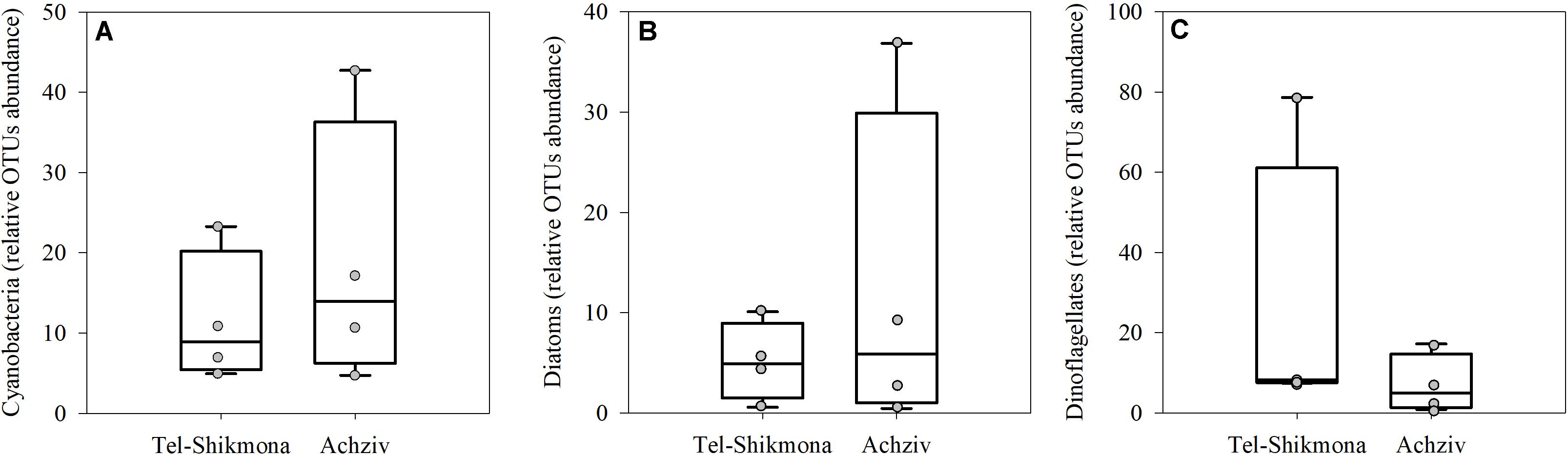
Figure 6. Box-Whisker plots showing the interquartile range (25th to 75th percentile) and median value (horizontal line within the box) of the relative OTU abundances of Cyanobacteria (A), Diatoms (B), and Dinoflagellates (C) in Tel-Shikmona (white) and Achziv (cyan) between 2014 and 2016. Data derived from 16S and 18S rDNA analyses (n = 4). The measured values used to generate the box-plots are shown in gray.
Discussion
The oligotrophic status of the SEMS is derived, among others, by the relatively low input of external nutrients to its coastal zone. The main external nutrients source to the SEMS, the Nile River, was dammed in the late 1960s, which effectively stopped most of the water inflow and its associated nutrients into the Mediterranean Sea. Most other surface water discharge to the SEMS are considered as “seasonal streams” (i.e., flow only during wintertime) that supply relatively low amounts of nutrients to coastal waters (Poulos, 2020). We argue that the significant differences in NOx (and silica) concentrations between Achziv and Tel-Shikmona are the result of relatively intense SGD rather than sporadic inflow of coastal streams or nutrient-rich effluents discharge (e.g., Rahav and Bar-Zeev, 2017; Rahav et al., 2018a). This conclusion is based on the inverse linear relationship between salinity and NOx (Figure 3A), the direct relationship between NOx and alkalinity (Figure 3B), as well as by the pronounced and constant (year round, including summertime) enrichment of NOx in Achziv relative to Tel-Shikmona between 2011 and 2019 (Figure 2A). We are aware that other approaches are often used to identify, locate, and quantify SGD in coastal environments, including measurements of natural tracers such as radon (Rn) and radium (Ra) and even methane (Burnett et al., 2008; Paytan et al., 2015). Nevertheless, our results clearly indicate that the coastal waters in Achziv were highly influenced by SGD as indicated by the salinity and trace element measurements (Figures 3, 4). Samples collected from underwater seeps at Achziv show contribution of submarine groundwater diluting the seawater as indicated from the freshwater-seawater mixing line established by Sivan et al. (2005) and the overall high concentrations of trace-elements measured (Figure 4). Specifically, the high Ba concentrations are a typical indication of SGD (Moore, 1996), and the Sr concentrations lying above the mixing line between fresh water and seawater indicate water-rock interaction in the coastal aquifer (Sivan et al., 2005), where the fresher water discharge from.
In turn, the discharge of nutrient-rich groundwater may supply a substantial input to coastal waters of dissolved inorganic nitrogen and silica, and to a lesser extent phosphate (Slomp and Van Cappellen, 2004; Wang et al., 2018), often leading to an increase in algal biomass (Paerl, 1997; Hwang et al., 2005; Lecher, 2017) and in some cases to the development of harmful algal blooms (Laroche et al., 1997; Liefer et al., 2009; Lecher et al., 2015). Similarly, several studies found a direct-linear relationship between Chl a and Rn, which is a natural tracer for SGD (Valiela et al., 1992; Su et al., 2014). Our in situ observations concur with the abovementioned studies, showing that the high nutrient levels in Achziv resulted in an increased phytoplankton biomass (most pronounced are the cyanobacteria, see below discussion), activity and changes in diversity (Figures 5, 6).
The response of phytoplankton to SGD may vary as a function of the initial physicochemical characteristics of the coastal environment (e.g., light levels, daily/seasonal temperature amplitudes, and salinity), the microbial species present (e.g., small-size cyanobacteria vs. large-size phytoplankton), the nutrient composition and content of the groundwater (e.g., absolute concentrations and N:P ratio), and the groundwater flux (Blanco et al., 2008; Lecher, 2017; Sugimoto et al., 2017). In many cases, diatoms are the main phytoplankton group to benefit from SGD. Diatoms have silica-based frustules (Hildebrand, 2003), and the high Si content in SGD which derives from rock–water interactions in the aquifer (Anschutz et al., 2009), along with N, often induce diatom blooms (Lecher et al., 2015). In this study, diatom biomass (estimated via the fucoxanthin/Chl a ratio) was similar in both the Achziv and Tel-Shikmona sites (Figure 5E), despite the significantly higher nitrate and silica concentrations at the Achziv site year round (Figure 2). While diatoms belonging to the genera Chaetoceros, Thalassiosira, Rhizosolenia, Pseudo-nitzschia, and Leptocylindrus are routinely found along the Israeli coast (using taxonomy-based approaches), their biomass is usually low (<1,000 cells L–1), even in sites exposed to local anthropogenic eutrophication (Rahav and Bar-Zeev, 2017). Even though the multi annual average of monthly NOx measurements at Achziv exceeded the medium threshold defined for eutrophication (>0.1 mg L–1) (Bricker et al., 2003), and for “good environmental status” of Israeli Mediterranean shallow coastal waters (Kress et al., 2019), our algal biomass measurements derived from Chl a were <1 μg L–1 and usually did not exceed the eutrophication threshold recently defined for the SEMS coastal waters (∼0.2–0.8 μg L–1, depending on the area along the Israeli coast; Kress et al., 2019). We surmise that the “severe” oligotrophic characteristics of the SEMS, even at the Achziv site (Chl a < 1 μg L–1, Figure 5A), preclude diatoms from forming large blooms. This may be because diatoms are outcompeted by small cyanobacteria (e.g., Synechococcus; Figure 5B) with more efficient nutrient uptake and utilization rates due to their high surface-area to volume ratio (Snoeijs et al., 2002). Moreover, diatoms at these sites may be outcompeted by mixotrophic phytoplankton (e.g., dinoflagellates), or may be limited by other factors that were not considered here (e.g., dissolved organic phosphorus; Põder et al., 2003). It is also possible that the diatoms grazing pressure was weaker at Tel-Shikmona than in Achziv. A previous study in the NW Mediterranean showed that zooplankton exert tighter control on microphytoplankton in oligotrophic environments than in productive systems (Calbet, 2001). To the best of our knowledge, no recent zooplankton surveys were carried out along the Israeli coast, and therefore we cannot estimate if indeed such top-down control occurs.
Dinoflagellates were more abundant in the nutrient-poor waters at Tel-Shikmona than at Achziv (Figure 5D), in contrast to studies in which ammonium-rich SGD induces dinoflagellates blooms (Gobler and Boneillo, 2003; Troccoli-Ghinaglia et al., 2010). Here, however, ammonium concentrations were similar in both sites (Table 1), while the ratio between NOx and NH4 was 0.2–1:1 in Tel-Shikmona and ∼2–15:1 in Achziv, suggesting unknown nutrient-dependent interactions. The factors governing nano/micro-phytoplankton species (e.g., diatoms and dinoflagellates) interactions and dynamics in the SEMS are still unclear and should be further investigated.
Interestingly, unlike many coastal environments affected by GSD where microphytoplankton species benefit the most from the nutrients inputs (abovementioned references), here cyanobacteria exhibited the biggest differences change, with higher abundances at Achziv compared to Tel-Shikmona (Figure 5B). We surmise that the reason why cyanobacterial biomass increased while diatoms remained overall the same relates to the ultra-oligotrophic nature of the SEMS that dictate the dominance of small-size microorganisms capable to rapidly utilize nutrients from their surroundings (specifically N as the initial Si(OH)4 levels in the water, even without groundwater input, was not considered limiting for diatoms). This was also reflected in the relative cyanobacterial OTU abundances (Figure 6A). It is to be noted, though, that information retrieved from DNA sequencing should be interpreted with caution, and may not necessarily be comparable to direct phytoplankton quantification measures (e.g., HPLC, flow cytometry, and microscopic taxonomy), since the copy number of rRNA per cell could vary depending on the taxa, as demonstrated for microphytoplankton (Zhu et al., 2005; Galluzzi et al., 2010) and bacteria (Valdivia-Anistro et al., 2016).
In accordance with the increase in cyanobacteria at Achziv over Tel-Shikmona, previous microcosm and mesocosm studies from the SEMS showed that cyanobacterial biomass is stimulated by additions of nitrate or nitrate + silica + phosphorus (Rahav et al., 2018b; Raveh et al., 2019). Furthermore, a recent study, also in the SEMS, showed that sewage discharges may lead to a short-term bloom of the cyanobacterium Trichodesmium (Rahav and Bar-Zeev, 2017), which may be harmful/toxic to local plankton communities (Detoni et al., 2016). Therefore, a chronic input of N (as well as silica and possibly other elements) by SGD is likely to support cyanobacterial proliferation and boost primary productivity. These observations are in agreement with other studies showing an increase in cyanobacterial abundance due to nutrient spikes at SGD sites (Blanco et al., 2008; Su et al., 2014).
The coastal SEMS harbors a variety of potentially toxic cyanobacteria (e.g., Microcystis sp.), diatoms (e.g., Pseudo-nitzschia multiseries) and dinoflagellates (e.g., Prorocentrum minimum, Ostreopsis siamensis, and Akashiwo sanguinea), especially near coastal river estuaries (Herut et al., 2020). Due to its oligotrophic characteristics (Table 1; Kress et al., 2019), natural or human-induced eutrophication in the SEMS may potentially lead to increased harmful algal biomass, as recently demonstrated in mesocosm experiments following N + Si-rich well-amelioration brine enrichments (Raveh et al., 2019) or in the past in Haifa Bay (Kress and Herut, 1998). An SGD discharge into the Gulf of Mexico triggered a Pseudo-nitzschia bloom (Macintyre et al., 2011) and a similar event occurred in an Alabama lagoon (Liefer et al., 2009). Moreover, SGD stimulated eutrophication in Bangdy Bay (Hwang et al., 2005) and in Lake Kasumigaura, Japan (Nakayama and Watanabe, 2008).
The Chl a and PP rates measured in the SGD-enriched site at Achziv are not consistent with the south-north gradient routinely found along the Israeli coast, which is attributed to the general longshore current in the same direction (Rosentraub and Brenner, 2007) and to point-sources of anthropogenic pollution (Frank et al., 2019; Kress et al., 2019; Raveh et al., 2019). The southern Israeli coastal waters usually have higher Chl a (∼0.5–1.0 μg L–1) and PP (∼4–9 μg C L–1 d–1) levels, whereas lower values are often measured in the northern region (0.05–0.5 μg L–1 and 1–4 μg C L–1 d–1, respectively) (Rahav et al., 2018b). Although it is located at the northern end of the Israeli coast, the Achziv site displayed similar characteristics to those found in the southern stations which have higher algal biomass. This highlights the potential of nutrient-rich SGD to relieve nutrient limitation of phytoplankton along the Israeli coast. In support of this observation, Raveh et al. (2019) showed that co-addition of nitrate and silica in the form of well-amelioration brines stimulates phytoplankton growth and enhances PP in oligotrophic Israeli coastal waters. Moreover, Sugimoto et al. (2017) showed that SGD leads to higher PP rates when compared to anthropogenic pollution discharges in Japanese waters, suggesting that SGD may cause eutrophication even in non-oligotrophic systems.
Eutrophication from SGD is a well-known phenomenon, observed both in field surveys and in laboratory manipulations (De Sieyes et al., 2008; Garcés et al., 2011; Lecher et al., 2015; Kobayashi et al., 2017; Sugimoto et al., 2017). The differences in Chl a and PP levels in Achziv compared to Tel-Shikmona (Figure 2) are in agreement with these studies (e.g., response relative to unamended control or unaffected area), both in magnitude (e.g., % effect) and direction (e.g., a positive increase) (Figure 7). Additions of NOx or silica alone resulted in lower increases in Chl a and PP in the southeastern Mediterranean shelf waters, while well-amelioration brine amendments which contain both high N and Si resulted in similar increases as in the Achziv site (Figure 7). This suggests that phytoplankton along the Mediterranean Israeli coast are N + Si limited, as opposed to the offshore waters that are P or N and P co-limited (Zohary et al., 2005; Tanaka et al., 2011; Tsiola et al., 2016). Given that the coastal waters often intrude into offshore waters (Barale et al., 2008; Efrati et al., 2013), enrichment by SGD may not only be a local coastal phenomenon but may also influence the pelagic microbial populations offshore and therefore higher trophic levels.
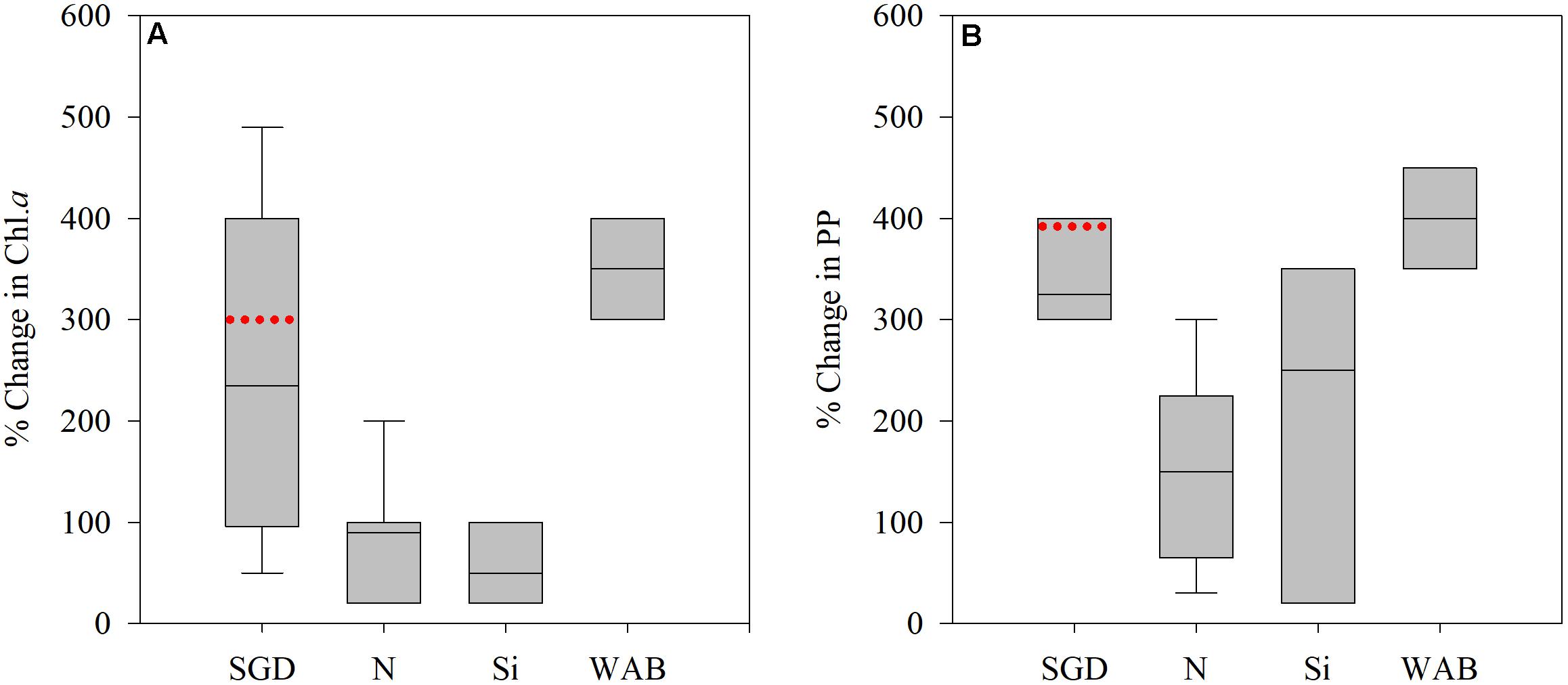
Figure 7. Literature compilation showing the% change in chlorophyll a (Chl a) (A) primary productivity (PP) (B) attributed to SGD, NO3 (N), Si(OH)4 (Si), or well-amelioration brines (WAB, containing mostly high levels of nitrate and silica). Data taken from De Sieyes et al. (2008), Garcés et al. (2011), Lecher et al. (2015), Kobayashi et al. (2017), Sugimoto et al. (2017), Rahav et al. (2018b) and Raveh et al. (2019). Box shows the interquartile range (25th to 75th percentile) and median value (horizontal line within the box). Red line indicates the median change found between Achziv and Tel-Shikmona.
Lastly, in Europe, NO3 eutrophication from agricultural sources to groundwater is addressed by the “Nitrates-Directive” (#91/676/EEC) under the Water Framework Directive (Directive 2000/60/EC). It is aimed to attain “good ecological and chemical status” of Europe’s fresh and coastal waters, where rivers and groundwater containing NO3 > 25 mg L–1 (∼5.6 mg NO3-N L–1) reflect a threshold of concern. While we did not measure the NO3 levels in groundwater directly at Achziv (i.e., from a drilling site at the aquifer), nearly 70 mg NO3-N L–1 (∼15.7 mg NO3-N L–1) were previously found near the discharge area (source: The Israeli Water Authority). In the context of anthropogenic contamination of groundwater, and the flux to the sea of nutrients via SGD, we recommend that further studies assessing the influences of this mechanism should be conducted, especially in oligotrophic marine areas such as the SEMS for the proper determination and implementation of “good ecological and chemical status.”
Conclusion
Our results indicate that SGD can be an important source of nutrients at the oligotrophic SEMS coastal water. While diatoms usually benefit from SGD due to the addition of both N and Si in groundwater, here cyanobacteria increased the most while diatoms abundance remained overall unchanged. This unusual phenomenon is a further indication for the ultra-oligotrophic nature of the SEMS where small-size cyanobacteria (as well as other prokaryotic microorganisms) likely outcompete larger-size microphytoplankton for limiting nutrients, specifically for nitrogen.
Data Availability Statement
The datasets presented in this study can be found in online repositories. The names of the repository/repositories and accession number(s) can be found below: NCBI BioProject [accession: PRJNA671735].
Author Contributions
ER, OR, and DA conceived and designed the experiments. ER and OR performed the samplings. ER, OR, NB, KY-G, YK, BH, JS, and DA analyzed the data. NB, PA, NT, MM, ER, OR, and JS run analyses. ER and YK contributed to reagents, materials, and analysis tools. ER, BH, JS, KY-G, and DA wrote the manuscript. All authors contributed to the article and approved the submitted version.
Funding
This work was supported by the Israeli National Monitoring Program and by grants awarded to ER by the Ministry of Energy (grant number 3-11519) and to ER, JS, and BH by the Ministry of Environmental Protection (grant number 145–1–2).
Conflict of Interest
The authors declare that the research was conducted in the absence of any commercial or financial relationships that could be construed as a potential conflict of interest.
Acknowledgments
We would like to express our gratitude to the Sir Maurice and Lady Irene Hatter Scholarship and the MERCI scholarship awarded to OR.
Supplementary Material
The Supplementary Material for this article can be found online at: https://www.frontiersin.org/articles/10.3389/fmars.2020.611497/full#supplementary-material
Supplementary Figure 1 | Multi-annual monthly averages of NOx (A), Si(OH)4 (B), PO4 (C), and total alkalinity normalized to a constant salinity of 39.234 PSU (D) in the surface water at the edge of the erosion platforms in Achziv (green) and Tel Shikmona (white) during the period 2011–2019. The error bars represent the standard deviations of 6–9 measurements made at each site during each month throughout the monitoring period.
Footnotes
References
Anschutz, P., Smith, T., Mouret, A., Deborde, J., Bujan, S., Poirier, D., et al. (2009). Tidal sands as biogeochemical reactors. Estuar. Coast. Shelf Sci. 84, 84–90. doi: 10.1016/j.ecss.2009.06.015
Barale, V., Jaquet, J. M., and Ndiaye, M. (2008). Algal blooming patterns and anomalies in the Mediterranean Sea as derived from the SeaWiFS data set (1998-2003). Remote Sens. Environ. 112, 3300–3313. doi: 10.1016/j.rse.2007.10.014
Belkin, N., Rahav, E., Elifantz, H., Kress, N., and Berman-Frank, I. (2017). The effect of coagulants and antiscalants discharged with seawater desalination brines on coastal microbial communities: a laboratory and in situ study from the southeastern Mediterranean. Water Res. 110, 321–331. doi: 10.1016/j.watres.2016.12.013
Berman-Frank, I., and Rahav, E. (2012). “Dinitrogen fixation as a source for new production in the Mediterranean Sea: a review,” in Life in the Mediterranean Sea: A Look at Habitat Changes, ed. N. E. Stambler (New York, NY: Nova Science Publishers), 199–226.
Blanco, A. C., Nadaoka, K., and Yamamoto, T. (2008). Planktonic and benthic microalgal community composition as indicators of terrestrial influence on a fringing reef in Ishigaki Island, Southwest Japan. Mar. Environ. Res. 66, 520–535. doi: 10.1016/j.marenvres.2008.08.005
Bricker, S. B., Ferreira, J. G., and Simas, T. (2003). An integrated methodology for assessment of estuarine trophic status. Ecol. Modell. 169, 39–60. doi: 10.1016/S0304-3800(03)00199-6
Burnett, W. C., Peterson, R., Moore, W. S., and de Oliveira, J. (2008). Radon and radium isotopes as tracers of submarine groundwater discharge - results from the Ubatuba, Brazil SGD assessment intercomparison. Estuar. Coast. Shelf Sci. 76, 501–511. doi: 10.1016/j.ecss.2007.07.027
Calbet, A. (2001). Annual zooplankton succession in coastal NW Mediterranean waters: the importance of the smaller size fractions. J. Plankton Res. 23, 319–331. doi: 10.1093/plankt/23.3.319
Caporaso, J. G., Lauber, C. L., Walters, W. A., Berg-Lyons, D., Lozupone, C. A., Turnbaugh, P. J., et al. (2011). Global patterns of 16S rRNA diversity at a depth of millions of sequences per sample. Proc. Natl. Acad. Sci. U.S.A. 108, 4516–4522. doi: 10.1073/pnas.1000080107
De Sieyes, N. R., Yamahara, K. M., Layton, B. A., Joyce, E. H., and Boehm, A. B. (2008). Submarine discharge of nutrient-enriched fresh groundwater at Stinson Beach, California is enhanced during neap tides. Limnol. Oceanogr. 53, 1434–1445. doi: 10.4319/lo.2008.53.4.1434
Detoni, A. M. S., Luiza, D. F. C., Pacheco Abrão, L., and Yunes, J. S. (2016). Toxic Trichodesmium bloom occurrence in the southwestern South Atlantic Ocean. Toxicon 110, 51–55. doi: 10.1016/j.toxicon.2015.12.003
Dickson, A. G., Afghan, J. D., and Anderson, G. C. (2003). Reference materials for oceanic CO2 analysis: a method for the certification of total alkalinity. Mar. Chem. 80, 185–197. doi: 10.1016/S0304-4203(02)00133-0
Efrati, S., Lehahn, Y., Rahav, E., Kress, N., Herut, B., Gertman, I., et al. (2013). Intrusion of coastal waters into the pelagic eastern Mediterranean: in situ and satellite-based characterization. Biogeosciences 10, 3349–3357. doi: 10.5194/bg-10-3349-2013
Frank, H., Fussmann, K. E., Rahav, E., and Zeev, E. B. (2019). Chronic effects of brine discharge form large-scale seawater reverse osmosis desalination facilities on benthic bacteria. Water Res. 151, 478–487. doi: 10.1016/j.watres.2018.12.046
Galluzzi, L., Bertozzini, E., Penna, A., Perini, F., Garcés, E., and Magnani, M. (2010). Analysis of rRNA gene content in the mediterranean dinoflagellate Alexandrium catenella and Alexandrium taylori: implications for the quantitative real-time PCR-based monitoring methods. J. Appl. Phycol. 22, 1–9. doi: 10.1007/s10811-009-9411-3
Garcés, E., Basterretxea, G., and Tovar-Sánchez, A. (2011). Changes in microbial communities in response to submarine groundwater input. Mar. Ecol. Prog. Ser. 438, 47–58. doi: 10.3354/meps09311
Gobler, C. J., and Boneillo, G. E. (2003). Impacts of anthropogenically influenced groundwater seepage on water chemistry and phytoplankton dynamics within a coastal marine system. Mar. Ecol. Prog. Ser. 255, 101–114. doi: 10.3354/meps255101
Hazan, O., Silverman, J., Sisma-Ventura, G., Ozer, T., Gertman, I., Shoham-Frider, E., et al. (2018). Mesopelagic prokaryotes alter surface phytoplankton production during simulated deep mixing experiments in Eastern Mediterranean Sea waters. Front. Mar. Sci. 5:1. doi: 10.3389/fmars.2018.00001
Herut, B., Almogi-Labin, A., Jannink, N., and Gertman, I. (2000a). The seasonal dynamics of nutrient and chlorophyll a concentrations on the SE Mediterranean shelf-slope. Oceanol. Acta 23, 771–782. doi: 10.1016/S0399-1784(00)01118-X
Herut, B., Kress, N., and Hornung, H. (2000b). Nutrient pollution at the lower reaches of Mediterranean coastal rivers in Israel. Water Sci. Technol. 42, 147–152. doi: 10.2166/wst.2000.0306
Herut, B., Segal, Y., Gertner, Y., Tibor, G., and Silverman, J. (2020). Israel Monitoring Program in the Southeastern Mediterranean Sea (in Hebrew) H07/2020. Haifa∗.
Hildebrand, M. (2003). Biological processing of nanostructured silica in diatoms. Prog. Org. Coatings 47, 256–266. doi: 10.1016/S0300-9440(03)00142-5
Hwang, D. W., Lee, Y. W., and Kim, G. (2005). Large submarine groundwater discharge and benthic eutrophication in Bangdu Bay on volcanic Jeju Island. Korea. Limnol. Oceanogr. 50, 1393–1403. doi: 10.4319/lo.2005.50.5.1393
Jeffrey, S. W., Mantoura, R. F. C., and Wright, S. W. (1997). “Phytoplankton pigments in oceanography: guidlines to modern methods,” in Monographs on Oceanographic Methodology, 1st Edn, eds S. Jeffery, R. F. C. Mantoura, and S. W. Wright (Paris: UNESCO).
Kobayashi, S., Sugimoto, R., Honda, H., Miyata, Y., Tahara, D., Tominaga, O., et al. (2017). High-resolution mapping and time-series measurements of 222 Rn concentrations and biogeochemical properties related to submarine groundwater discharge along the coast of Obama Bay, a semi-enclosed sea in Japan. Prog. Earth Planet. Sci. 4:6. doi: 10.1186/s40645-017-0124-y
Kress, N., Frede Thingstad, T., Pitta, P., Psarra, S., Tanaka, T., Zohary, T., et al. (2005). Effect of P and N addition to oligotrophic Eastern Mediterranean waters influenced by near-shore waters: a microcosm experiment. Deep. Res. Part II Top. Stud. Oceanogr. 52, 3054–3073. doi: 10.1016/j.dsr2.2005.08.013
Kress, N., and Herut, B. (1998). Hypernutrification in the oligotrophic Eastern Mediterranean: a study in Haifa Bay (Israel). Estuar. Coast. Shelf Sci. 46, 645–656. doi: 10.1006/ecss.1997.0302
Kress, N., and Herut, B. (2001). Spatial and seasonal evolution of dissolved oxygen and nutrients in the Southern Levantine Basin (Eastern Mediterranean Sea): chemical characterization of the water masses and inferences on the N : P ratios. Deep Sea Res. Part I Oceanogr. Res. Pap. 48, 2347–2372. doi: 10.1016/s0967-0637(01)00022-x
Kress, N., Rahav, E., Silverman, J., and Herut, B. (2019). Environmental status of Israel’s Mediterranean coastal waters: setting reference conditions and thresholds for nutrients, chlorophyll-a and suspended particulate matter. Mar. Pollut. Bull. 141, 612–620. doi: 10.1016/j.marpolbul.2019.02.070
Krom, M. D., Kress, N., Berman-Frank, I., and Rahav, E. (2014). “Past, present and future patterns in the nutrient chemistry of the Eastern Mediterranean,” in The Mediterranean Sea: Its History and Present Challenges, eds S. Goffredo, and Z. Dubinsky (Netherlands: Springer), 49–68. doi: 10.1007/978-94-007-6704-1_4
Laroche, J., Nuzzi, R., Waters, R., Wyman, K., Falkowski, P., and Wallace, D. (1997). Brown tide blooms in long Island’s coastal waters linked to interannual variability in groundwater flow. Glob. Chang. Biol. 3, 397–410. doi: 10.1046/j.1365-2486.1997.00117.x
Lecher, A. L. (2017). Groundwater discharge in the arctic: a review of studies and implications for biogeochemistry. Hydrology 4:41. doi: 10.3390/hydrology4030041
Lecher, A. L., Mackey, K., Kudela, R., Ryan, J., Fisher, A., Murray, J., et al. (2015). Nutrient loading through submarine groundwater discharge and phytoplankton growth in Monterey bay, CA. Environ. Sci. Technol. 49, 6665–6673. doi: 10.1021/acs.est.5b00909
Lecher, A. L., and Mackey, K. R. M. (2018). Synthesizing the effects of submarine groundwater discharge on Marine Biota. Hydrology 5, 1–21. doi: 10.3390/hydrology5040060
Liefer, J. D., MacIntyre, H. L., Novoveská, L., Smith, W. L., and Dorsey, C. P. (2009). Temporal and spatial variability in Pseudo-nitzschia spp. in Alabama coastal waters: a “hot spot” linked to submarine groundwater discharge? Harmful Algae 8, 706–714. doi: 10.1016/j.hal.2009.02.003
Macintyre, H. L., Stutes, A. L., Smith, W. L., Dorsey, C. P., Annabraham, A., and Dickey, R. W. (2011). Environmental correlates of community composition and toxicity during a bloom of Pseudo-nitzschia spp. in the northern Gulf of Mexico. J. Plankton Res. 33, 273–295. doi: 10.1093/plankt/fbq146
Marie, D., Partensky, F., Jacquet, S., and Vaulot, D. (1997). Enumeration and cell cycle analysis of natural populations of marine picoplankton by flow cytometry using the nucleic acid stain SYBR Green I. Appl. Environ. Microbiol. 63, 186–193. doi: 10.1128/aem.63.1.186-193.1997
Medlin, L., Elwood, H. J., Stickel, S., and Sogin, M. L. (1988). The characterization of enzymatically amplified eukaryotic 16S-like rRNA-coding regions. Gene 71, 491–499. doi: 10.1016/0378-1119(88)90066-2
Mella-Flores, D., Mazard, S., Humily, F., Partensky, F., Mahe, F., Bariat, L., et al. (2011). Is the distribution of Prochlorococcus and Synechococcus ecotypes in the Mediterranean Sea affected by global warming? Biogeosciences 8, 2785–2804. doi: 10.5194/bg-8-2785-2011
Moore, W. S. (1996). Large groundwater inputs to coastal waters revealed by 226 Ra enrichments. Nature 380, 612–614. doi: 10.1038/380612a0
Nakayama, T., and Watanabe, M. (2008). Missing role of groundwater in water and nutrient cycles in the shallow eutrophic Lake Kasumigaura. Japan. Hydrol. Process. 22, 1150–1172. doi: 10.1002/hyp.6684
Paerl, H. W. (1997). Coastal eutrophication and harmful algal blooms: importance of atmospheric deposition and groundwater as “new” nitrogen and other nutrient sources. Limnol. Oceanogr. 42, 1154–1165. doi: 10.4319/lo.1997.42.5_part_2.1154
Paldor, A., Shalev, E., Katz, O., and Aharonov, E. (2019). Dynamics of saltwater intrusion and submarine groundwater discharge in confined coastal aquifers: a case study in northern Israel. Hydrogeol. J. 27, 1611–1625. doi: 10.1007/s10040-019-01958-5
Paytan, A., Lecher, A. L., Dimova, N., Sparrow, K. J., Garcia-Tigreros Kodovska, F., Murray, J., et al. (2015). Methane transport from the active layer to lakes in the Arctic using Toolik Lake, Alaska, as a case study. Proc. Natl. Acad. Sci. U.S.A. 112, 3636–3640. doi: 10.1073/pnas.1417392112
Põder, T., Maestrini, S. Y., Balode, M., Lips, U., Bechmin, C., Andrushaitis, A., et al. (2003). The role of inorganic and organic nutrients on the development of phytoplankton along a transect from the Daugava River mouth to the Open Baltic, in spring and summer 1999. ICES J. Mar. Sci. 60, 827–835. doi: 10.1016/s1054-3139(03)00069-9
Poulos, S. E. (2020). The Mediterranean and black sea marine system: an overview of its physico-geographic and oceanographic characteristics. Earth-Science Rev. 200:103004. doi: 10.1016/j.earscirev.2019.103004
Psarra, S., Tselepides, A., and Ignatiades, L. (2000). Primary productivity in the oligotrophic Cretan Sea (NE Mediterranean): seasonal and interannual variability. Prog. Oceanogr. 46, 187–204. doi: 10.1016/s0079-6611(00)00018-5
Rahav, E., and Bar-Zeev, E. (2017). Sewage outburst triggers Trichodesmium bloom and enhance N2 fixation rates. Sci. Rep. 7:4367. doi: 10.1038/s41598-017-04622-8
Rahav, E., Belkin, N., Paytan, A., and Herut, B. (2018a). Bacterioplankton response to desert dust deposition in the coastal waters of the southeastern Mediterranean Sea; a four year in-situ survey. Atmos 9:305. doi: 10.3390/atmos9080305
Rahav, E., Raveh, O., Hazan, O., Gordon, N., Kress, N., Silverman, J., et al. (2018b). Impact of nutrient enrichment on productivity of coastal water along the SE Mediterranean shore of Israel - a bioassay approach. Mar. Pollut. Bull. 127, 559–567. doi: 10.1016/j.marpolbul.2017.12.048
Rahav, E., Giannetto, M., and Bar-Zeev, E. (2016a). Contribution of mono and polysaccharides to heterotrophic N2 fixation at the eastern Mediterranean coastline. Sci. Rep. 6:27858. doi: 10.1038/srep27858
Rahav, E., Paytan, A., Chien, C., Ovadia, G., Katz, T., and Herut, B. (2016b). The impact of atmospheric dry deposition associated microbes on the southeastern Mediterranean Sea surface water following an intense dust storme. Front. Mar. Sci. 3:127. doi: 10.3389/fmars.2016.00127
Rahav, E., Herut, B., Levi Adi, Mulholland, M. R., and Berman-Frank, I. (2013). Springtime contribution of dinitrogen fixation to primary production across the Mediterranean Sea. Ocean Sci. 9, 489–498. doi: 10.5194/os-9-489-2013
Rahav, E., Silverman, J., Raveh, O., Hazan, O., Rubin-Blum, M., Zeri, C., et al. (2019). The deep water of Eastern Mediterranean Sea is a hotspot for bacterial activity. Deep Sea Res. Part II Top. Stud. Oceanogr. 164, 135–143. doi: 10.1016/j.dsr2.2019.03.004
Raveh, O., Angel, D. L., Astrahan, P., Belkin, N., Bar-Zeev, E., and Rahav, E. (2019). Phytoplankton response to N-rich well amelioration brines: a mesocosm study from the southeastern Mediterranean Sea. Mar. Pollut. Bull. 146, 355–365. doi: 10.1016/j.marpolbul.2019.06.067
Raveh, O., David, N., Rilov, G., and Rahav, E. (2015). The temporal dynamics of coastal phytoplankton and bacterioplankton in the eastern mediterranean sea. PLoS One 10:e0140690. doi: 10.1371/journal.pone.0140690
Rilov, G. (2016). Multi-species collapses at the warm edge of a warming sea. Sci. Rep. 6:36897. doi: 10.1038/srep36897
Rodellas, V., Garcia-Orellana, J., Masqué, P., Feldman, M., Weinstein, Y., and Boyle, E. A. (2015). Submarine groundwater discharge as a major source of nutrients to the Mediterranean Sea. Proc. Natl. Acad. Sci. U.S.A. 112, 3926–3930. doi: 10.1073/pnas.1419049112
Rosentraub, Z., and Brenner, S. (2007). Circulation over the southeastern continental shelf and slope of the Mediterranean Sea: direct current measurements, winds, and numerical model simulations. J. Geophys. Res. Ocean. 112:C11001. doi: 10.1029/2006JC003775
Sass, E., and Ben-Yaakov, S. (1977). The carbonate system in hypersaline solutions: dead sea brines. Mar. Chem. 5, 183–199. doi: 10.1016/0304-4203(77)90006-8
Siokou-Frangou, I., Christaki, U., Mazzocchi, M. G., Montresor, M., Ribera d’Alcalá, M., et al. (2010). Plankton in the open Mediterranean Sea: a review. Biogeosciences 7, 1543–1586. doi: 10.5194/bg-7-1543-2010
Sisma-Ventura, G., and Rahav, E. (2019). DOP stimulates heterotrophic bacterial production in the oligotrophic southeastern mediterranean coastal waters. Front. Microbiol. 10:1913. doi: 10.3389/fmicb.2019.01913
Sivan, O., Yechieli, Y., Herut, B., and Lazar, B. (2005). Geochemical evolution and timescale of seawater intrusion into the coastal aquifer of Israel. Geochim. Cosmochim. Acta 69, 579–592. doi: 10.1016/j.gca.2004.07.023
Slomp, C. P., and Van Cappellen, P. (2004). Nutrient inputs to the coastal ocean through submarine groundwater discharge: controls and potential impact. J. Hydrol. 295, 64–86. doi: 10.1016/j.jhydrol.2004.02.018
Snoeijs, P., Busse, S., and Potapova, M. (2002). The importance of diatom cell size in community analysis. J. Phycol. 38, 265–272. doi: 10.1046/j.1529-8817.2002.01105.x
Steemann-Nielsen, E. (1952). The use of radioactive carbon (14C) for measuring organic production in the sea. J. Cons. Perm. Int. Explor. Mer. 18, 117–140. doi: 10.1093/icesjms/18.2.117
Su, N., Burnett, W. C., MacIntyre, H. L., Liefer, J. D., Peterson, R. N., and Viso, R. (2014). Natural radon and radium isotopes for assessing groundwater discharge into little lagoon, AL: implications for harmful algal blooms. Estuaries and Coasts 37, 893–910. doi: 10.1007/s12237-013-9734-9
Sugimoto, R., Kitagawa, K., Nishi, S., Honda, H., Yamada, M., Kobayashi, S., et al. (2017). Phytoplankton primary productivity around submarine groundwater discharge in nearshore coasts. Mar. Ecol. Prog. Ser. 563, 25–33. doi: 10.3354/meps11980
Tanaka, T., Thingstad, T. F., Christaki, U., Colombet, J., Cornet-Barthaux, V., Courties, C., et al. (2011). Lack of P-limitation of phytoplankton and heterotrophic prokaryotes in surface waters of three anticyclonic eddies in the stratified Mediterranean Sea. Biogeosciences 8, 525–538. doi: 10.5194/bg-8-525-2011
Tanaka, T., Zohary, T., Krom, M. D., Law, C. S., Pitta, P., Psarra, S., et al. (2007). Microbial community structure and function in the levantine basin of the eastern Mediterranean. Deep Sea Res. Part I Oceanogr. Res. Pap. 54, 1721–1743. doi: 10.1016/j.dsr.2007.06.008
Trezzi, G., Garcia-Orellana, J., Rodellas, V., Masqué, P., Garcia-Solsona, E., and Andersson, P. S. (2017). Assessing the role of submarine groundwater discharge as a source of Sr to the Mediterranean Sea. Geochim. Cosmochim. Acta 200, 42–54. doi: 10.1016/j.gca.2016.12.005
Troccoli-Ghinaglia, L., Herrera-Silveira, J. A., Comín, F. A., and Díaz-Ramos, J. R. (2010). Phytoplankton community variations in tropical coastal area affected where submarine groundwater occurs. Cont. Shelf Res. 30, 2082–2091. doi: 10.1016/j.csr.2010.10.009
Tsiola, A., Pitta, P., Fodelianakis, S., Pete, R., Magiopoulos, I., Mara, P., et al. (2016). Nutrient limitation in surface waters of the oligotrophic eastern Mediterranean Sea: an enrichment microcosm experiment. Microb. Ecol. 71, 575–588. doi: 10.1007/s00248-015-0713-715
Valdivia-Anistro, J. A., Eguiarte-Fruns, L. E., Delgado-Sapién, G., Márquez-Zacarías, P., Gasca-Pineda, J., Learned, J., et al. (2016). Variability of rRNA operon copy number and growth rate dynamics of Bacillus isolated from an extremely oligotrophic aquatic ecosystem. Front. Microbiol. 6, 1–15. doi: 10.3389/fmicb.2015.01486
Valiela, I., Foreman, K., LaMontagne, M., Hersh, D., Costa, J., Peckol, P., et al. (1992). Couplings of watersheds and coastal waters: sources and consequences of nutrient enrichment in Waquoit Bay, Massachusetts. Estuaries 15, 443–457. doi: 10.2307/1352389
Wang, X., Li, H., Zheng, C., Yang, J., Zhang, Y., Zhang, M., et al. (2018). Submarine groundwater discharge as an important nutrient source influencing nutrient structure in coastal water of Daya Bay, China. Geochim. Cosmochim. Acta 225, 52–65. doi: 10.1016/j.gca.2018.01.029
Weinstein, Y., Yechieli, Y., Shalem, Y., Burnett, W. C., Swarzenski, P. W., and Herut, B. (2011). What is the role of fresh groundwater and recirculated seawater in conveying nutrients to the coastal ocean? Environ. Sci. Technol. 45, 5195–5200. doi: 10.1021/es104394r
Welschmeyer, N. A. (1994). Fluorometric analysis of chlorophyll a in the presence of chlorophyll b and pheopigments. Limnol. Oceanogr. 39, 1985–1992. doi: 10.4319/lo.1994.39.8.1985
Zapata, M., Rodríguez, F., and Garrido, J. L. (2000). Separation of chlorophylls and carotenoids from marine phytoplankton: a new HPLC method using a reversed phase C8 column and pyridine-containing mobile phases. Mar. Ecol. Prog. Ser. 195, 29–45. doi: 10.3354/meps195029
Zohary, T., Herut, B., Krom, M. D., Fauzi, C., Mantoura, R., Pitta, P., et al. (2005). P-limited bacteria but N and P co-limited phytoplankton in the Eastern Mediterranean - a microcosm experiment. Deep. Res. Part II Top. Stud. Oceanogr. 52, 3011–3023. doi: 10.1016/j.dsr2.2005.08.011
Keywords: submarine groundwater discharge, nitrate enrichment, chlorophyll-a, phytoplankton, primary production, Eastern Mediterranean Sea
Citation: Rahav E, Raveh O, Yanuka-Golub K, Belkin N, Astrahan P, Maayani M, Tsumi N, Kiro Y, Herut B, Silverman J and Angel DL (2020) Nitrate-Enrichment Structures Phytoplankton Communities in the Shallow Eastern Mediterranean Coastal Waters. Front. Mar. Sci. 7:611497. doi: 10.3389/fmars.2020.611497
Received: 29 September 2020; Accepted: 30 November 2020;
Published: 22 December 2020.
Edited by:
Kyung-Hoon Shin, Hanyang University, South KoreaReviewed by:
J. German Rodriguez, Technological Center Expert in Marine and Food Innovation (AZTI), SpainBum Soo Park, University of Texas at Austin, United States
Copyright © 2020 Rahav, Raveh, Yanuka-Golub, Belkin, Astrahan, Maayani, Tsumi, Kiro, Herut, Silverman and Angel. This is an open-access article distributed under the terms of the Creative Commons Attribution License (CC BY). The use, distribution or reproduction in other forums is permitted, provided the original author(s) and the copyright owner(s) are credited and that the original publication in this journal is cited, in accordance with accepted academic practice. No use, distribution or reproduction is permitted which does not comply with these terms.
*Correspondence: Eyal Rahav, ZXlhbC5yYWhhdkBvY2Vhbi5vcmcuaWw=; ZXlhbHJhaGF2QGdtYWlsLmNvbQ==
†These authors have contributed equally to this work