- 1The Applied Marine Biology and Ecology Research Laboratory, Department of Maritime Civilizations, Leon Charney School of Marine Sciences, University of Haifa, Haifa, Israel
- 2Recanati Institute for Maritime Studies, University of Haifa, Haifa, Israel
This study examined the ability of a Mediterranean demosponge Chondrosia reniformis to oxidize exogenous ammonium, simulating N-rich conditions that occur near finfish farms. We hypothesized that as the concentration of ammonium increases in the surrounding seawater, nitrification mediated by microbes associated with C. reniformis will lead to enhancement of ammonium uptake, nitrate excretion and oxygen consumption by the sponge holobiont. To test this hypothesis, we conducted laboratory experiments with C. reniformis explants exposed to ammonium enrichments (300–6667 nM) and to ambient seawater (45–1511 nM ammonium). We analyzed inhaled (IN) and exhaled (EX) water samples for dissolved oxygen, ammonium, nitrates and retention of picoplankton cells. We observed ammonium uptake in nearly half the cases and excretion of nitrate in most experimental outcomes. Yet, the consumption of ammonium and oxygen, as well as the excretion of nitrate by C. reniformis were not related to the concentration of inhaled ammonium, which suggests that the nitrification activity of sponge-associated microbes is not necessarily related to the concentration of ammonium in the surrounding seawater. Further research is required to reveal the sources of nitrate released from sponges and the fate of this nitrate in natural and manipulated ecosystems.
Introduction
Marine sponges feed on microorganisms and host dense and diverse microbial communities in their bodies (Taylor et al., 2007; Fan, 2012; Webster and Taylor, 2012; Pita et al., 2018). Owing to the importance of microbe-mediated processes in the sponge's life, the term “holobiont,” i.e., “whole organism,” is used to emphasize that microbial consortia are integral parts of the sponge (Hentschel et al., 2003). Microbial associates contribute to sponge's metabolism and ecological functioning in various ways, such as the processing of dissolved inorganic nitrogen (DIN), which is the subject of the present study.
Sponges produce ammonium () as a metabolic waste (endogenous ammonium), which makes the sponge body an attractive niche for microorganisms in nitrogen-limited marine environments (Han et al., 2013). Nitrification is an exclusively prokaryotic process whereby ammonium is oxidized to nitrite () and subsequently to nitrate (), and it is utilized to obtain energy by different groups of bacteria and archaea associated with sponges (Kowalchuk and Stephen, 2001; Schläppy et al., 2010; Ribes et al., 2012; Pita et al., 2018). Several sponge species were found to consume exogenous ammonium while excreting nitrate, thereby resembling microbe-mediated nitrification (Corredor et al., 1988; Diaz and Ward, 1997; Jiménez and Ribes, 2007; Schläppy et al., 2010; Ribes et al., 2012).
The present study examines the potential of a Mediterranean high microbial abundance (HMA) sponge Chondrosia reniformis (Nardo, 1847) to act as a sink for ammonium under conditions of elevated concentrations of ammonium in seawater. Such conditions are expected near marine finfish farms, where the concentrations of ammonium are elevated because of fish metabolic wastes (Hargrave et al., 1993; Pitta et al., 2006; Korzen et al., 2016). By doing so, we tested the possible functioning of C. reniformis as an ammonium sink in Integrated Multi Trophic Aquaculture (IMTA), as suggested by Pronzato (1999), Chopin (2012), and Gökalp et al. (2019).
The working hypothesis of this study, hereafter referred to as the “ammonium-induced nitrification,” predicted elevated uptake of ammonium, elevated excretion of nitrate and elevated consumption of oxygen by C. reniformis as the concentration of ammonium increases in seawater, due to the oxidation of ammonium to nitrate (nitrification) by the sponge-associated microbes. The “induced nitrification” hypothesis stems from the evidence of nitrification in C. reniformis obtained by Jiménez and Ribes (2007), Bayer et al. (2008), and Schläppy et al. (2010). Ammonia-oxidizing prokaryotes (bacteria and archaea) and functional genes for nitrification (ammonia monooxygenase, amoA/amoB) are usually found in HMA sponges including C. reniformis (Han et al., 2013; Bayer et al., 2014; Gantt et al., 2019). Hence, we chose to perform a practical analysis of nitrification in C. reniformis, especially regarding its possible role as an ammonium sink in human-impacted marine ecosystems such as aquaculture facilities.
Here we present simultaneous measurements of all nitrification components: ammonium, nitrite, nitrate, and oxygen by non-intrusive methods which require no physical contact with the sponge. We tested the “induced nitrification” hypothesis in laboratory aquaria enriched with ammonium, and the results were used to predict if C. reniformis would act as a sink for ammonium in integrated aquaculture.
Materials and Methods
Sponge Collection and Handling
Live sponges were collected by SCUBA diving from 2 to 4 m depths at a rocky reef in the Eastern Mediterranean Sea (32°29′24.71″N, 34°53′9.29″E) in July 2014. Specimens covering roughly 10 cm2 each were cut from different individuals, such that the larger part of the sponge was not removed to allow for its regeneration. The excised specimens, from here on referred to as “explants,” were inserted underwater into sealed plastic bags and brought to the laboratory within 2 h after collection while constantly immersed in seawater to prevent possible damage to sponges from exposure to air.
In the laboratory, the explants were placed on porcelain tiles to provide them with substrate for attachment and kept in individual 6 L aquaria immersed in a sealed wooden table filled with seawater to maintain equal temperature among the aquaria. The aquaria were supplied with a constant flow of sand-filtered seawater that was pumped from 150 m off the coast at 2 m depth. Prior to initiation of experiments the sponges were checked for: (1) attachment to the substrate; (2) opening of osculae; (3) natural color; and (4) pumping of water by spreading fluorescein dye near the sponge and observing the efflux of the dye through the osculae.
The experiments were conducted in two seasons during 2014: summer (July–August) and autumn (November–December). The water temperature in experimental aquaria ranged between 27–28°C in the summer and between 18–21°C C in the autumn.
Experimental Design
To evaluate the influence of sponge filtration on the concentrations of ammonium, nitrite, and nitrate, we used direct sampling of water that is inhaled and exhaled by the sponge, IN-EX, sensu Yahel et al. (2005). We calculated the differences in concentrations of ammonium, nitrate and nitrite between the inhaled (IN) and the exhaled (EX) water samples. We combined the differences in concentrations of nitrite and nitrate as ΔNOx and addressed these as the difference in nitrate concentrations. To evaluate the oxygen consumption by sponge explants during the sampling, we measured the concentrations of dissolved oxygen at IN and EX sites by needle-type optode sensors (Fire-Sting®, Pyro-Science GmbH Germany, Supplementary Figure 1) and calculated the differences between the IN and EX oxygen concentrations as ΔO2. The working hypothesis predicted that an increase in concentration of ammonium inhaled by the sponge (NH4IN) would result in elevated uptake of ammonium (), elevated excretion of nitrate (ΔNOx), and elevated oxygen consumption (ΔO2).
We used a split-plot design to test the effect of inhaled ammonium concentration (NH4IN) on three response variables: ΔNH4, ΔNOx, and ΔO2. We applied two treatments, “ambient” and “enrichment,” to all explants as a whole-plot factor. For the ambient treatment, the concentration of ammonium in aquaria was not augmented artificially and thus represented natural concentrations. For the enrichment treatment, we added ammonium chloride (Sigma-Aldrich PN 254134) into a 10 L container with seawater, and from that container to an aquarium with a sponge in a flow-through manner. The ranges of ammonium concentrations were 0.045–1.511 μM and 0.344–6.667 μM for the ambient and the enrichment treatments, respectively. To account for the variance in responses to elevated ammonium concentration between the explants, we used each “explant” as a split-plot factor and applied both treatments to each explant alternately.
To verify that sponges were filtering the water, all IN-EX samples were examined by flow cytometry for retention of picoplankton. To control for the variability in analyte concentrations which are not caused by sponge activity, three pairs of samples from an aquarium without a sponge were collected under two ambient and two enrichment treatments and analyzed in the same way as the samples obtained from sponges.
Sampling Procedure
The IN-EX samples were collected by siphoning through two identical Teflon hoses of 1 m length and internal diameter of 0.5 mm equipped with glass capillary tips of 300 μm internal diameter (Supplementary Figure 1). The samples of inhaled water (IN) were collected at a distance of 5 mm from the sponge's surface and at least 1 cm away from an osculum, and the samples of exhaled water (EX) were collected in front of the sponge's osculum by placing the sampling gear as close as possible to the osculum while not touching the sponge. The samples were collected into sterile test tubes and kept in a dark styrofoam box filled with ice in order to slow down the microbial transformations of DIN during the collection and handling. All sampling equipment was cleaned with a 10% HCl solution and rinsed with double-distilled water between the experiments.
Analytical Procedures
From each sample of an IN-EX pair (30 ml), 2 ml were analyzed for ammonium immediately after collection, 10 ml were filtered through 0.45 μm membranes (Millex PVDF, Millipore) and stored at 4°C until the analyses of nitrite and nitrate, and 1.5 ml were preserved with 10% glutaraldehyde (Sigma-Aldrich PN G7651) and stored at −80°C for flow cytometry analysis.
Ammonium
The concentration of ammonium () was determined by the fluorometric method amended from Holmes et al. (1999), as described in Appendix S2 (Supplementary Material). Fluorescence was measured by a Trilogy fluorometer (Turner Designs, USA) with excitation at 350–380 nm and emission at 410–450 nm (ammonium kit 7200-041). The accuracy of ammonium assay was tested against a certified reference material (Fluka QC3179) and the precision between duplicate analyses of sample splits was <50 nM.
Nitrite and Nitrate
The analyses of nitrate () and nitrite () were conducted using a flow injection autoanalyzer (Lachat Instruments, QuikChem 8000) following the method of Hansen and Koroleff (1999). The analyses were fully automated and peak areas were calibrated using 0–5 μM standards prepared in nutrient-depleted filtered seawater (Meeder et al., 2012). The precision between duplicate analyses of sample splits was ±0.02 and ±0.05 μM for nitrite and nitrate, respectively.
Oxygen
Oxygen optodes underwent two-point calibration using 100% oxygen-saturated seawater and oxygen-depleted seawater made anoxic by addition of sodium sulfite. Dissolved oxygen readings were automatically adjusted to the water temperature that was recorded in situ by the instrument's temperature sensor (Supplementary Figure 1).
Flow Cytometry
Concentrations of picoplankton cells in the IN-EX samples were measured using an LSR II flow cytometer (Becton, Dickinson and Co., USA) and using the FCS Express v.5 software for data analysis. The specifications of the flow cytometer were adjusted to the concentrations of cells in the Eastern Mediterranean water and 1 μm beads (Invitrogen) were used for size reference (Appendix S3 in Supplementary Material).
Phytoplankton cells with red and orange fluorescence were classified as picoeukaryotes (Euk), prokaryotic Prochlorococcus-like (Pro) or Synechococcus-like (Syn) populations, and detected using the autofluorescence, size and shape characteristics, according to Marie et al. (2001). The non-photosynthetic heterotrophic bacteria (Het) were detected using SYBR Green (SG) staining for DNA as in Perea-Blázquez et al. (2012).
The efficiency of cell retention by the sponge (RE) was calculated as:
RE [%] = (([IN] – [EX])/[IN])*100%, where [IN], [EX] – are the concentrations of a given cell type (cells/ml) in the inhaled and the exhaled samples, respectively.
Negative retention rates resulting from cell concentrations in exhaled samples being higher than the concentrations in inhaled samples were interpreted as no retention of picoplankton and consequently changed to zero.
Statistical Analyses
We collected 87 IN-EX pairs of water samples from 10 C. reniformis explants. Nineteen IN-EX pairs were excluded from analyses due to missing results, low retention rates (<50% for all picoplankton groups) or suspicion of artifacts. The data selected for statistical analyses included 68 IN-EX pairs of DIN, oxygen, and picoplankton retention readings obtained from 10 different explants (Supplementary Material, Data Sheet 1).
The effects of inhaled ammonium concentration (NH4IN) on the differences in ammonium, nitrites, and oxygen between the inhaled and the exhaled water (Δ, ΔNOx, and ΔO2, respectively) were analyzed by the Generalized Linear Mixed Model (GLIMMIX) procedure of SAS version 9.4. We used explant as a random blocking variable and treatment (ambient vs. enrichment), season (summer vs. autumn), and inhaled ammonium (NH4IN) as fixed effects. For each of the response variables (ΔNH4, ΔNOx, and ΔO2) a full-factorial model of fixed effects and their interactions was tested first, and after removing the non-significant interactions (p ≥ 0.05) by backward elimination, the model was re-run.
Results
During the experiments the explants maintained natural color, opened osculae, and pumped water (Supplementary Figure 2). Water pumping through the sponge was verified by spreading a small amount of fluorescein near sponge's surface and observing the efflux of fluorescein from the osculae.
The changes in DIN and oxygen concentrations between the inhaled (IN) and the exhaled (EX) water samples collected from sponge explants were considerably larger than the changes obtained from the control aquarium without a sponge (Figure 1). Positive differences between the inhaled and the exhaled concentrations of ammonium (ammonium uptake) were detected in 36 out of 68 IN-EX pairs. The differences in NOx concentrations between the inhaled and the exhaled samples were negative in most cases (63 out of 68 IN-EX pairs), i.e., nitrates were excreted by the sponge (Supplementary Material, Data Sheet 1).
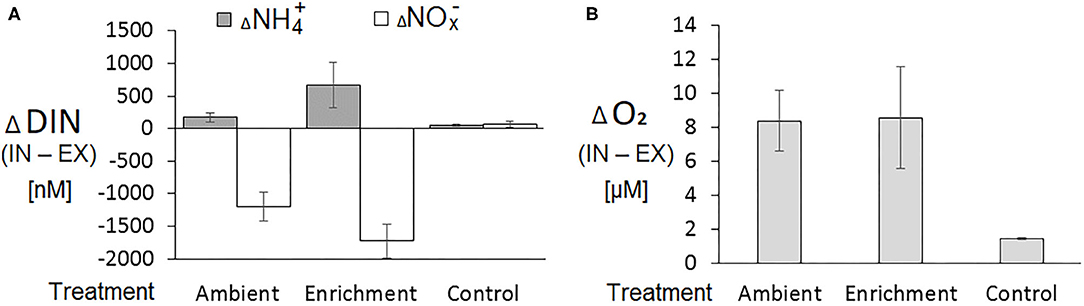
Figure 1. Changes in dissolved inorganic nitrogen and dissolved oxygen concentrations between IN-EX samples collected from sponge and control (no sponge) aquaria. (A) Dissolved inorganic nitrogen (DIN) as ammonium () and nitrates ( + = NOx); (B) Dissolved oxygen. Averages ± SD. n = 39, 32, and 6 for ambient, enrichment and control treatments, respectively.
The absolute concentrations of nitrite did not exceed 20% of the total NOx (NO2 + NO3) and the changes in the concentration of nitrite between the IN and EX samples were much smaller than those of nitrate, usually by an order of magnitude (results not shown). Therefore, we combined the differences in concentrations of nitrite and nitrate as ΔNOx and addressed these as the difference in nitrate concentrations.
The effect of inhaled ammonium concentration (NH4IN) alone on any of the response variables (, ΔNOx, and ΔO2) was not statistically significant. The effect of treatment was of borderline statistical significance on ΔNOx; and the effects of season and of the interaction NH4IN*season on ΔO2 were statistically significant (Table 1). The retention of picoplankton by C. reniformis was similar under both treatments, with highest retention for Synechococcus-like particles (Table 2).
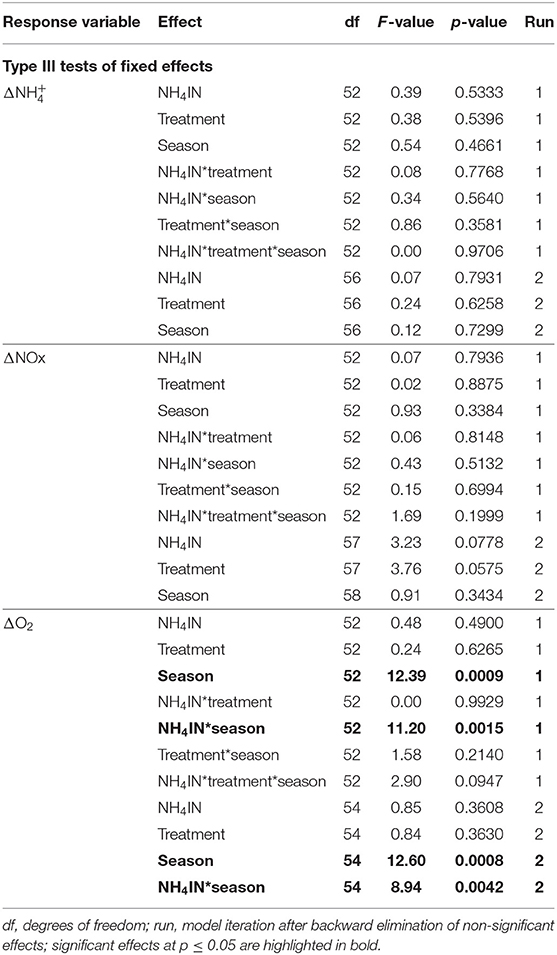
Table 1. Results of GLIMMIX analysis of the effects of inhaled ammonium, treatment, and season on the outcomes of nitrification.
Discussion
The present study examined the effect of ammonium enrichment on the microbe-mediated nitrification in an HMA sponge Chondrosia reniformis. The augmentation of water with ammonium simulated the cultivation of C. reniformis next to fish farms where the water is enriched with ammonium. Based on the previous findings of Jiménez and Ribes (2007), Bayer et al. (2008), Schläppy et al. (2010), and Ribes et al. (2012), we assumed that the microbe-mediated nitrification in C. reniformis would be enhanced by elevating the concentrations of ammonium in the water inhaled by the sponge. Although we observed ammonium uptake in nearly half of experimental outcomes and excretion of nitrate in most IN-EX pairs, the oxidation of exogenous ammonium (the ammonium-induced nitrification) seems not to be the source for nitrate excretion, since ammonium and oxygen consumptions were not linked to nitrate excretion, as discussed below.
Nitrification and Feeding
The analysis of IN-EX results (Table 1) suggests that the fluxes of ammonium and NOx through C. reniformis are not related to the concentration of ammonium in the surrounding seawater. Fiore et al. (2013) observed in a field study both positive and negative fluxes of ammonium and NOx through the tropical HMA sponge Xestospongia muta. In a laboratory study of Bayer et al. (2008), the Mediterranean HMA sponge Aplysina aerophoba acted as a sink or a source of ammonium alternately, depending on the water temperature. In the present work, the flux of ammonium through C. reniformis was not affected by temperature: uptake and release of ammonium were observed at similar frequencies in summer and in autumn. Taken together, the findings of Bayer et al. (2008), Fiore et al. (2013), and the present work suggest that HMA sponges do not always act as a sink for ammonium.
It is noteworthy that while HMA sponges such as C. reniformis are usually positive for nitrate excretion (Jiménez and Ribes, 2007; Bayer et al., 2008; Hoffmann et al., 2009; Ribes et al., 2012; present study), sponges of the LMA group do not excrete nitrate or do so at much reduced rates (Jiménez and Ribes, 2007; Yahel et al., 2007; Ribes et al., 2012). Yet, there is evidence for aerobic nitrification and for anaerobic processes such as denitrification and anammox in both HMA and LMA sponges (Hoffmann et al., 2009; Mohamed et al., 2010; Schläppy et al., 2010). It is reasonable to assume that in the present study we observed the outcome of coexisting or even competing aerobic and anaerobic microbial processes co-occurring in the same sponge (C. reniformis), which might explain the variations in the excretion of nitrate. Further research is needed to decipher the specific contributions of co-occurring microbial processes to the net outcome of nitrite and nitrate excretion.
Besides nitrite and nitrate, nitrogen monoxide also known as nitric oxide (NO) is a DIN compound of considerable interest. NO is produced by the enzyme nitric oxide synthase (NOS), which converts arginine into NO and citrulline while consuming molecular oxygen (Alderton et al., 2001). NOS activity was described in diverse metazoan phyla: Cnidaria, Echinodermata, Annelida, Mollusca, Arthropoda, Urocordata, and Porifera (Jacklet, 1997; Giovine et al., 2001; Palumbo, 2005; Ueda et al., 2016) and in microbial communities (Schreiber, 2006). Since NO is a signaling molecule rather than a primary metabolite, we did not measure its production by the C. reniformis holobiont in the present study. Nonetheless, in light of the evidence that their exposure to high concentrations of ammonia induces NOS activity in fish (Choudhury and Saha, 2012), further research is needed on NOS activity and NO concentrations in sponges exposed to high environmental concentrations of ammonia.
Sponges feed on picoplankton cells in the size range of 0.2–2 μm with almost 100% efficiency (Ribes et al., 1999; Topçu et al., 2010; Jimenez, 2011). In the present work, the efficiencies of picoplankton retention by C. reniformis (Table 2) were lower than those reported by Jimenez (2011) for the same species from the Western Mediterranean Sea. We observed 16% retention of Prochlorococcus-like population and 45% retention of heterotrophic bacteria, whereas Jimenez (2011) observed 93 and 98% retention of the corresponding populations. In our study, Synechococcus-like cells were retained with the highest efficiency (96%) as in the work of Jimenez (2011). Topçu et al. (2010) and Perea-Blázquez et al. (2012) also found that Synechococcus-like cells are the preferred food source for sponges, which suggests that in our study C. reniformis explants were feeding normally during the experiments.
Oxygen Consumption
The consumption of oxygen by C. reniformis explants was more affected by season than by the concentration of inhaled ammonium (Table 1). These results suggest that the contribution of microbe-mediated nitrification to the oxygen consumption by C. reniformis is minor. Moreover, although we verified that the sponges were feeding (Table 2), we did not account for possible changes in the sponge's other oxygen-consuming functions, such as water pumping. A sponge may use about 25% of its oxygen consumption to pump water (Hadas et al., 2008; Leys et al., 2011). Therefore, future studies of oxygen consumption and nutrient processing by sponges should account for seasonality and include measurements of pumping rates.
Conclusions and Suggestions for Further Research
Our findings suggest that we cannot generalize about HMA sponges serving as a source or a sink of ammonium. Particularly, this study shows that C. reniformis should not be considered as a sink for ammonium in models of integrated aquaculture.
Further research is needed into the possible effects of ammonium on the activity of nitric oxide synthase (NOS) enzymes in sponges. It is likely that elevated concentrations of exogenous ammonium may affect the arginine-NO-citrulline pathway, which is shared by the sponge host and the sponge-associated microbes (Song et al., 2020). We suggest further research into the roles of nitric oxide in the flux of DIN through the sponge and in regulating sponge's regeneration.
A recent study showed that the relative abundances of ammonia-oxidizing microbial taxa increase when sponges exhibit higher levels of ammonium consumption (Gantt et al., 2019). In order to elucidate the mechanisms underlying the variability in sponge-mediated DIN fluxes, we suggest combining physiological experiments as performed in the present work with sampling for microbial community composition as performed by Gantt et al. (2019). By knowing what the sponge-associated microbes are and what these microbes do in different conditions we can better predict the sponge-mediated DIN fluxes in natural and in human-impacted ecosystems and use these predictions in planning aquaculture or other applications.
Data Availability Statement
The datasets generated for this study can be found in online repositories. The names of the repository/repositories and accession number(s) can be found in the article/Supplementary Material.
Author Contributions
PN and DA contributed conception and design of the study. PN analyzed the data and wrote the first draft of the manuscript. All authors contributed to manuscript revision, read, and approved the submitted version.
Funding
The research was partially funded by the EU FP7 grant to DA Increasing Industrial Resource Efficiency in European Mariculture (IDREEM, project number 30857, web-site: www.idreem.eu). Financial support to PN was provided by the Hatter Fellowship for Maritime Studies, the Department of Maritime Civilizations, University of Haifa; by the Rotary Branch in Haifa, by the Rieger Foundation and by the Lev Lemaan Israel Fund. Fieldwork and sponge maintenance equipment were supported by Ramot Yam regional school and Ruppin Academic College in Mikhmoret, Israel; and by the Lev Yam Fish Mariculture Ltd. Israel. Laboratory analyses of flow cytometry were supported by the LSE Infrastructure Unit, Technion, Israel; the analyses of Nitrite and Nitrate were supported by the Inter University Institute of Marine Sciences, Eilat, Israel.
Conflict of Interest
The authors declare that the research was conducted in the absence of any commercial or financial relationships that could be construed as a potential conflict of interest.
Acknowledgments
We are grateful to the Leon H. Charney School of Marine Sciences and the Leon Recanati Institute of Maritime Studies, University of Haifa; Ramot Yam regional school and Ruppin Academic Center, Mikhmoret Israel. We thank the following people for their help in various ways: Gitai Yahel, Reuben Rosenblat, Rafi Yavetz, David Halfon, Zeev Glauber, Arik Weinberger, Gal Bogolowsky, Zafrir Kuplik, Tal Shomrat, Dori Edelist, Udi Arkin, Laura Cohen, Dina Zvieli, JJ Gottlieb, Mia Elasar, Dafna Israel, and Tanya Ukrainsky.
Supplementary Material
The Supplementary Material for this article can be found online at: https://www.frontiersin.org/articles/10.3389/fmars.2020.607979/full#supplementary-material
Supplementary Figure 1. Schematic sketch and photographs of the system used for water sampling and for measuring the concentration of dissolved oxygen. (A) Schematic sketch of the system, not to scale. 1 – Oxygen module with a PC (2) showing (3) the in situ oxygen readings by IN (green) and EX (red) optodes; 4 – temperature sensor; 5, 6 – water sampling sets and optodes; 7 – sponge explant attached to the substrate; 8 – tubes for collection of water samples; 9 – sub-samples for subsequent analyses. (B) Photograph of the sponge in aquarium with the sampling sets held by the GorillaPod manipulator, and the temperature sensor attached to the aquarium wall on the right; (C) close-up photograph of the sampling set, with an additional close-up (D) showing the tip of the oxygen sensor (top) and the tip of the water sampling capillary (bottom).
Supplementary Figure 2. Photographs of the sponge Chondrosia reniformis during and after the experiment. (A) a sponge at the beginning of the experiment (July 2014), the arrow points to attachment of the sponge to a porcelain tile. (B) The same sponge after 4 months of handling in aquarium (October 2014), arrows point to the open osculae. (C) The same sponge after the experiment (May 2015). The scale bar under the sponge number is 1 cm.
References
Alderton, W. K., Cooper, C. E., and Knowles, R. G. (2001). Nitric oxide synthases: structure, function and inhibition. Biochem. J. 357, 593–615. doi: 10.1042/bj3570593
Bayer, K., Moitinho-Silva, L., Brümmer, F., Cannistraci, C. V., Ravasi, T., and Hentschel, U. (2014). GeoChip-based insights into the microbial functional gene repertoire of marine sponges (high microbial abundance, low microbial abundance) and seawater. FEMS Microbiol. Ecol. 90, 832–843. doi: 10.1111/1574-6941.12441
Bayer, K., Schmitt, S., and Hentschel, U. (2008). Physiology, phylogeny and in situ evidence for bacterial and archaeal nitrifiers in the marine sponge Aplysina aerophoba. Environ. Microbiol. 10, 2942–2955. doi: 10.1111/j.1462-2920.2008.01582.x
Chopin, T. (2012). Aquaculture, integrated multi-trophic (IMTA). Encycl. Sustain. Sci. Technol. 542–564. doi: 10.1007/978-1-4419-0851-3_173
Choudhury, M. G., and Saha, N. (2012). Influence of environmental ammonia on the production of nitric oxide and expression of inducible nitric oxide synthase in the freshwater air-breathing catfish (Heteropneustes fossilis). Aquat. Toxicol. 116, 43–53. doi: 10.1016/j.aquatox.2012.03.006
Corredor, J. E., Wilkinson, C. R., Vicente, V. P., Morell, J. M., and Otero, E. (1988). Nitrate release by Caribbean reef sponges. Limnol. Oceanogr. 33, 114–120. doi: 10.4319/lo.1988.33.1.0114
Diaz, M. C., and Ward, B. B. (1997). Sponge-mediated nitrification in tropical benthic communities. Mar. Ecol. Prog. Ser. 156, 97–107. doi: 10.3354/meps156097
Fan, L. (2012). Phylogenetic diversity, functional convergence, and stress response of the symbiotic system between sponges and microorganisms. Proc. Natl. Acad. Sci. U.S.A. 109, E1878–E1887. doi: 10.1073/pnas.1203287109
Fiore, C. L., Baker, D. M., and Lesser, M. P. (2013). Nitrogen biogeochemistry in the Caribbean sponge Xestospongia muta: a source or sink of dissolved inorganic nitrogen? PLoS ONE 8:e72961. doi: 10.1371/journal.pone.0072961
Gantt, S. E., McMurray, S. E., Stubler, A. D., Finelli, C. M., Pawlik, J. R., and Erwin, P. M. (2019). Testing the relationship between microbiome composition and flux of carbon and nutrients in Caribbean coral reef sponges. Microbiome 7:124. doi: 10.1186/s40168-019-0739-x
Giovine, M., Pozzolini, M., Favre, A., Bavestrello, G., Cerrano, C., Ottaviani, F., et al. (2001). Heat stress-activated, calcium-dependent nitric oxide synthase in sponges. Nitric Oxide Biol. Chem. 5, 427–431. doi: 10.1006/niox.2001.0366
Gökalp, M., Wijgerde, T., Sarà, A., de Goeij, J., and Osinga, R. (2019). Development of an integrated mariculture for the collagen-rich sponge Chondrosia reniformis. Mar. Drugs 17:29. doi: 10.3390/md17010029
Hadas, E., Ilan, M., and Shpigel, M. (2008). Oxygen consumption by a coral reef sponge. J. Exp. Biol. 211, 2185–2190. doi: 10.1242/jeb.015420
Han, M., Li, Z., and Zhang, F. (2013). The ammonia oxidizing and denitrifying prokaryotes associated with sponges from different sea areas. Microb. Ecol. 66, 427–436. doi: 10.1007/s00248-013-0197-0
Hansen, H. P., and Koroleff, F. (1999). “Determination of nutrients,” in Methods of Seawater Analysis, eds K. Grasshoff, M. Ehrhardt, and K. Kremling (Weinheim: Verlag Chemie), 180–186.
Hargrave, B. T., Duplisea, D. E., Pfeiffer, E., and Wildish, D. J. (1993). Seasonal changes in benthic fluxes of dissolved oxygen and ammonium associated with marine cultured Atlantic salmon. Mar. Ecol. Prog. Ser. 96, 249–257. doi: 10.3354/meps096249
Hentschel, U., Fieseler, L., Wehrl, M., Gernert, C., Steinert, M., Hacker, J., et al. (2003). “Microbial diversity of marine sponges,” in Sponges (Porifera), eds W. E. G. Müller (Berlin; Heidelberg: Springer), 59–88. doi: 10.1007/978-3-642-55519-0_3
Hoffmann, F., Radax, R., Woebken, D., Holtappels, M., Lavik, G., Rapp, H. T., et al. (2009). Complex nitrogen cycling in the sponge Geodia barretti. Environ. Microbiol. 11, 2228–2243. doi: 10.1111/j.1462-2920.2009.01944.x
Holmes, R. M., Aminot, A., Kérouel, R., Hooker, B. A., and Peterson, B. J. (1999). A simple and precise method for measuring ammonium in marine and freshwater ecosystems. Can. J. Fish. Aquat. Sci. 56, 1801–1808. doi: 10.1139/f99-128
Jacklet, J. W. (1997). Nitric oxide signaling in invertebrates. Invertebr. Neurosci. 3, 1–14. doi: 10.1007/BF02481710
Jimenez, E. (2011). Nutrient fluxes in marine sponges: methodology, geographical variability and the role of associated microorganisms (Ph.D. thesis), Universitat Politècnica de Catalunya, Barcelona, Spain, 140.
Jiménez, E., and Ribes, M. (2007). Sponges as a source of dissolved inorganic nitrogen: nitrification mediated by temperate sponges. Limnol. Oceanogr. 52, 948–958. doi: 10.4319/lo.2007.52.3.0948
Korzen, L., Abelson, A., and Israel, A. (2016). Growth, protein and carbohydrate contents in Ulva rigida and Gracilaria bursa-pastoris integrated with an offshore fish farm. J. Appl. Phycol. 28, 1835–1845. doi: 10.1007/s10811-015-0691-5
Kowalchuk, G. A., and Stephen, J. R. (2001). Ammonia-oxidizing bacteria: a model for molecular microbial ecology. Annu. Rev. Microbiol. 55, 485–529. doi: 10.1146/annurev.micro.55.1.485
Leys, S. P., Yahel, G., Reidenbach, M. A., Tunnicliffe, V., Shavit, U., and Reiswig, H. M. (2011). The sponge pump: the role of current induced flow in the design of the sponge body plan. PLoS ONE 6:e27787. doi: 10.1371/journal.pone.0027787
Marie, D., Partensky, F., Vaulot, D., and Brussaard, C. (2001). Enumeration of phytoplankton, bacteria, and viruses in marine samples. Curr. Protoc. Cytom. 10, 11–15. doi: 10.1002/0471142956.cy1111s10
Meeder, E., Mackey, K. R. M., Paytan, A., Shaked, Y., Iluz, D., Stambler, N., et al. (2012). Nitrite dynamics in the open ocean — clues from seasonal and diurnal variations. Mar. Ecol. Prog. Ser. 453, 11–26. doi: 10.3354/meps09525
Mohamed, N. M., Saito, K., Tal, Y., and Hill, R. T. (2010). Diversity of aerobic and anaerobic ammonia-oxidizing bacteria in marine sponges. ISME J. 4, 38–48. doi: 10.1038/ismej.2009.84
Nardo, G. D. (1847). “Prospetto della fauna marina volgare del Veneto Estuario con cenni sulle principali specie commestibili dell'Adriatico,sulle venete pesche, sulle valli, ecc,” in Venezia e le sue lagune, 113–156.
Palumbo, A. (2005). Nitric oxide in marine invertebrates: a comparative perspective. Comp. Biochem. Physiol. Part A Mol. Integr. Physiol. 142, 241–248. doi: 10.1016/j.cbpb.2005.05.043
Perea-Blázquez, A., Davy, S. K., and Bell, J. J. (2012). Nutrient utilisation by shallow water temperate sponges in New Zealand. Hydrobiologia 687, 237–250. doi: 10.1007/s10750-011-0798-x
Pita, L., Rix, L., Slaby, B. M., Franke, A., and Hentschel, U. (2018). The sponge holobiont in a changing ocean: from microbes to ecosystems. Microbiome 6:46. doi: 10.1186/s40168-018-0428-1
Pitta, P., Apostolaki, E. T., Tsagaraki, T., Tsapakis, M., and Karakassis, I. (2006). Fish farming effects on chemical and microbial variables of the water column: a spatio-temporal study along the Mediterranean Sea. Hydrobiologia 563, 99–108. doi: 10.1007/s10750-005-1593-3
Pronzato, R. (1999). Sponge-fishing, disease and farming in the Mediterranean Sea. Aquat. Conserv. Mar. Freshw. Ecosyst. 9, 485–493. doi: 10.1002/(SICI)1099-0755(199909/10)9:5<485::AID-AQC362>3.0.CO;2-N
Ribes, M., Coma, R., and Gili, J. M. (1999). Natural diet and grazing rate of the temperate sponge Dysidea avara (Demospongiae, Dendroceratida) throughout an annual cycle. Mar. Ecol. Prog. Ser. 176, 179–190. doi: 10.3354/meps176179
Ribes, M., Jiménez, E., Yahel, G., López-Sendino, P., Diez, B., Massana, R., et al. (2012). Functional convergence of microbes associated with temperate marine sponges. Environ. Microbiol. 14, 1224–1239. doi: 10.1111/j.1462-2920.2012.02701.x
Schläppy, M. L., Schöttner, S. I., Lavik, G., Kuypers, M. M. M., de Beer, D., and Hoffmann, F. (2010). Evidence of nitrification and denitrification in high and low microbial abundance sponges. Mar. Biol. 157, 593–602. doi: 10.1007/s00227-009-1344-5
Schreiber, F. (2006). Detection and function of nitric oxide in microbial communities (Dissertation). University of Bremen, Bremen, Germany.
Song, H., Hewitt, O. H., and Degnan, S. M. (2020). Arginine biosynthesis by a bacterial symbiont enables nitric oxide production and facilitates larval settlement in the marine sponge host. Curr. Biol. 31:1–5. doi: 10.1016/j.cub.2020.10.051
Taylor, M. W., Radax, R., Steger, D., and Wagner, M. (2007). Sponge-associated microorganisms: evolution, ecology, and biotechnological potential. Microbiol. Mol. Biol. Rev. 71, 295–347. doi: 10.1128/MMBR.00040-06
Topçu, N. E., Pérez, T., Grégori, G., and Harmelin-Vivien, M. (2010). In situ investigation of Spongia officinalis (Demospongiae) particle feeding: coupling flow cytometry and stable isotope analysis. J. Exp. Mar. Bio. Ecol. 389, 61–69. doi: 10.1016/j.jembe.2010.03.017
Ueda, N., Richards, G. S., Degnan, B. M., Kranz, A., Adamska, M., Croll, R. P., et al. (2016). An ancient role for nitric oxide in regulating the animal pelagobenthic life cycle: evidence from a marine sponge. Sci. Rep. 6:37546. doi: 10.1038/srep37546
Webster, N. S., and Taylor, M. W. (2012). Marine sponges and their microbial symbionts: love and other relationships. Environ. Microbiol. 14, 335–346. doi: 10.1111/j.1462-2920.2011.02460.x
Yahel, G., Marie, D., and Genin, A. (2005). InEx – a direct in situ method to measure filtration rates, nutrition, and metabolism of active suspension feeders. Limnol. Oceanogr. Methods 3, 46–58. doi: 10.4319/lom.2005.3.46
Keywords: aquaculture, holobiont, marine ecology, nutrient fluxes, nitrification
Citation: Nemoy P, Spanier E and Angel DL (2021) Nitrification Activity of the Sponge Chondrosia reniformis Under Elevated Concentrations of Ammonium. Front. Mar. Sci. 7:607979. doi: 10.3389/fmars.2020.607979
Received: 18 September 2020; Accepted: 08 December 2020;
Published: 07 January 2021.
Edited by:
Enric Gisbert, Institute of Agrifood Research and Technology (IRTA), SpainReviewed by:
Marina Pozzolini, University of Genoa, ItalyLorenzo Gallus, University of Genoa, Italy
Copyright © 2021 Nemoy, Spanier and Angel. This is an open-access article distributed under the terms of the Creative Commons Attribution License (CC BY). The use, distribution or reproduction in other forums is permitted, provided the original author(s) and the copyright owner(s) are credited and that the original publication in this journal is cited, in accordance with accepted academic practice. No use, distribution or reproduction is permitted which does not comply with these terms.
*Correspondence: Dror L. Angel, ZGFuZ2VsQHVuaXYuaGFpZmEuYWMuaWw=