- 1Shimoda Marine Research Center, University of Tsukuba, Shimoda, Japan
- 2Molecular and Biological Agricultural Sciences Program, Taiwan International Graduate Program, National Chung Hsing University and Academia Sinica, Taipei, Taiwan
- 3Graduate Institute of Biotechnology, National Chung Hsing University, Taichung, Taiwan
- 4Biodiversity Research Center, Academia Sinica, Taipei, Taiwan
- 5Graduate School of Engineering and Science, University of the Ryukyus, Okinawa, Japan
- 6Tropical Biosphere Research Center, University of the Ryukyus, Okinawa, Japan
- 7Institute of Fisheries Science, National Taiwan University, Taipei, Taiwan
Endolithic microbes in coral reefs may act as a nutrient source for their coral hosts. Increasing atmospheric CO2 concentrations are causing ocean acidification (OA), which may affect marine organisms and ecosystems, especially calcifying organisms such as reef-building corals. However, knowledge of how OA affects marine microbes remains limited, and little research has been done on how coral endolithic communities respond to shifting environmental baselines. In this study, the endolithic communities of two common shallow water coral species, Isopora palifera and Porites lobata, were examined to investigate the microbial community dynamics under OA treatments. The colonies were placed in an environment with a partial pressure of carbon dioxide (pCO2) of 1,000 or 400 ppm (control) for 2 months. Several I. palifera colonies bleached and died at 1,000 ppm pCO2, but the P. lobata colonies remained unaffected. Inversely, the endolithic community in P. lobata skeletons showed significant changes after OA treatment, whereas no significant dynamics were observed among the I. palifera endoliths. Our findings suggest that the skeletal structures of different coral species may play a key role in corals host and endoliths under future high-OA scenarios.
Introduction
Endolithic communities in the coral skeleton are a group of microorganisms including cyanobacteria, fungi, algae, and bacteria that live in harsh, dim-light environments; they face drastic diurnal fluctuations in pH and oxygen and play an important role in the biogeochemical cycles of the coral reef ecosystem (Chazottes et al., 1995; Radtke et al., 1996; Ghirardelli, 2002; De Los Ríos et al., 2005). Culture-independent methods have identified various anaerobic endolithic bacteria in oxygen- and light-limited zones of the skeleton (Yang et al., 2016). For example, anaerobic photoautotrophic bacteria—like green sulfur bacteria (GSB) and sulfur reducing bacteria—were dominant and formed a visible green band in the skeleton of the coral Isopora palifera. These endolithic communities might be indispensable for nitrogen cycling and provide numerous primary products for coral (Shashar et al., 1994; Yang et al., 2019; Pernice et al., 2020).
Atmospheric CO2 concentrations are rising each year due to human activities. According to the State of the Climate in 2019, the annual average of global atmospheric CO2 concentration increased from 0.6 ± 0.1 ppm year–1 in the 1960s to 2.3 ppm year–1 in 2009–2018, and reached 2.5 ± 0.1 ppm in 2018–2019, an estimated overall increase of 95 ppm (from 315 to 410 ppm) (Dunn et al., 2020). Current predictions suggest that CO2 concentrations will rise to 680 ± 50 ppm by the year 2050 and the partial pressure of carbon dioxide (pCO2) will reach 900 ± 50 ppm by 2100 (IPCC RCP 6.0 projections, Pachauri et al., 2015). Rising global CO2 emissions in the atmosphere lead to increases in seawater pCO2, which reduces oceanic pH and carbonate ion concentrations, a process known as ocean acidification (OA) (Caldeira and Wickett, 2003).
Ocean acidification may have serious effects on marine organisms and ecosystems, especially calcifying organisms such as reef-building corals (Hofmann et al., 2010; Pandolfi et al., 2011; Kroeker et al., 2013). A recent study showed that lower pH increases porosity in the coral skeleton, thus reducing the skeleton’s density (Tambutté et al., 2015). This increased porosity may change the concentration of oxygen in the skeletal micro-environment, potentially shifting endolithic microbial composition and function. Recent evidence indicated that elevated pCO2 temperature scenarios influenced endolithic algae (Ostreobium spp.) in coral skeletons (Reyes-Nivia et al., 2013). Ostreobium spp. have been commonly reported in the pronounced green layer of various coral species across different geographical regions (del Campo et al., 2017). These species have been hypothesized to be an important endolithic group that supports coral host tissue undergoing bleaching (Fine and Loya, 2002). Nevertheless, the green layer in Isopora palifera skeletons from Green Island, Taiwan (Yang et al., 2016, 2019) were dominated by GSB and not Ostreobium. Whether this is due to the coral species or geographical factors remains uncertain, but the finding does provide an opportunity to understand the roles of other bacterial communities in the coral skeleton. In addition, little is known about the specific impacts of OA on the bacterial composition in coral skeletons, such as whether it changes the endolithic anaerobic bacterial composition. Here, two coral species, Isopora palifera and Porites lobata, with visible endolithic green bands in their skeletons were used to investigate the bacterial composition dynamics under OA treatment.
Materials and Methods
Three colonies of Isopora palifera and Porites lobata were collected from a reef flat (2–3 m deep) off of Sesoko Island, Japan in January 2018. The coral samples were obtained with permission from Okinawa prefecture (Permit No. 30–22). After the collection, the corals were put in an outdoor flow-through seawater tank under natural sunlight with a shade cloth for 16 days to acclimate at Sesoko Station (26°38′N, 127°51′E), University of the Ryukyus. Each colony was divided in two with a hammer and sterilized chisel—one I. palifera colony broke into three pieces, so it was added to the acidification set. In total, three and four I. palifera samples and three P. lobata samples were used in the control and acidification treatments. The corals were then acclimated to artificial light conditions (metal-halide lamp, Funnel-2, Kamihata, Japan, 150 W, and 150 μmol/m2/s) (Iguchi et al., 2014) for 6 days in two flow-through seawater indoor tanks with 21°C seawater. Then the pCO2 was adjusted to 1,000 ppm in the OA treatment tank and 400 ppm in the control tank (Iguchi et al., 2014). Due to the requirement of large colony size needed to proceed for time-serious sampling and avoid contamination, the experiment was limited to only two tanks. Sampling time points were conducted on Days 28 (T1) and 56 (T2) using a sterilized chisel to collect fragments from the incubated coral. The first visible green layer zone below the tissue of each fragment was sampled in two parts for sequencing replication.
The collected samples were washed twice with filtered seawater and stored at −20°C until DNA extraction. The extraction procedure followed the established method in Yang et al. (2016) using a hand drill (Dremel 2050-15, Mt. Prospect, IL, United States). Slurry samples from the green layers of the coral skeleton were transferred into clean 1.5 mL tubes with 1X TE buffer. Total genomic DNA was extracted for each sample using the PowerSoil DNA Isolation Kit (MoBio, Solana Beach, CA, United States). Following Yang et al. (2016), PCR was performed using two bacterial universal primers: 968F (5′-AGAGTTTGATCMTGGCTCAG-3′) and 1391R (5′-CTGCTGCCTCCCGTAGG-3′), designed for the bacterial V6–V8 hypervariable regions in the 16S ribosomal RNA gene. The PCR conditions followed the method described in Yang et al. (2016).
Each PCR product was tagged using DNA tagging PCR (Chen et al., 2011) and then sequenced by the Illumina Miseq 250 bp paired-end System. Reads from Illumina sequencing were merged and quality-filtered using Mothur v1.36.1 (Schloss et al., 2009). Chimeric reads were detected and removed by USEARCH v8.1.1.1861 (Edgar, 2013) in reference mode (3% minimum divergence). Operational taxonomic units (OTUs) were grouped by 97% identity using the UPARSE pipeline (Edgar, 2013) and classified using the Silva v128 database (Quast et al., 2013; Yilmaz et al., 2014) with a pseudo-bootstrap threshold of 80%. All samples with replicates were analyzed using OTU levels and plotted in Non-metric multidimensional scaling (nMDS) by PRIMER6 (Clarke and Gorley, 2005) first to check the similarity between sample replicates (Supplementary Figure 1). Then the duplicate sequences from each sample OTU were combined for nMDS and ANOSIM analyses. OTU abundance was rarefied using the minimum sequencing depth before processing for the alpha (Shannon diversity and the Kruskal–Wallis test) and beta diversity (PERMANOVA, weighted unifrac) analyses in QIIME2 (Bolyen et al., 2019). The R1 (R Core Team, 2017) and ggplot2 packages (Wickham, 2009) were used to plot the Shannon diversity and relative abundances of the assigned taxa at different taxonomic levels. The Venn diagrams of OTU and family level were plotted using website developed by the Van de Peer lab2. The sequences were deposited into GenBank (PRJNA656865)3.
Results
The two coral species responded differently to acidification. Two of the I. palifera colonies bleached and died under the acidified conditions. One colony (Figure 1, T1I1A and T2I1A) started bleaching after 2 weeks and the whole colony died one-week later, and the other (Figure 1, T1I2A, and T2I2A) started bleaching 5 days after the first sampling and died 3 days later.
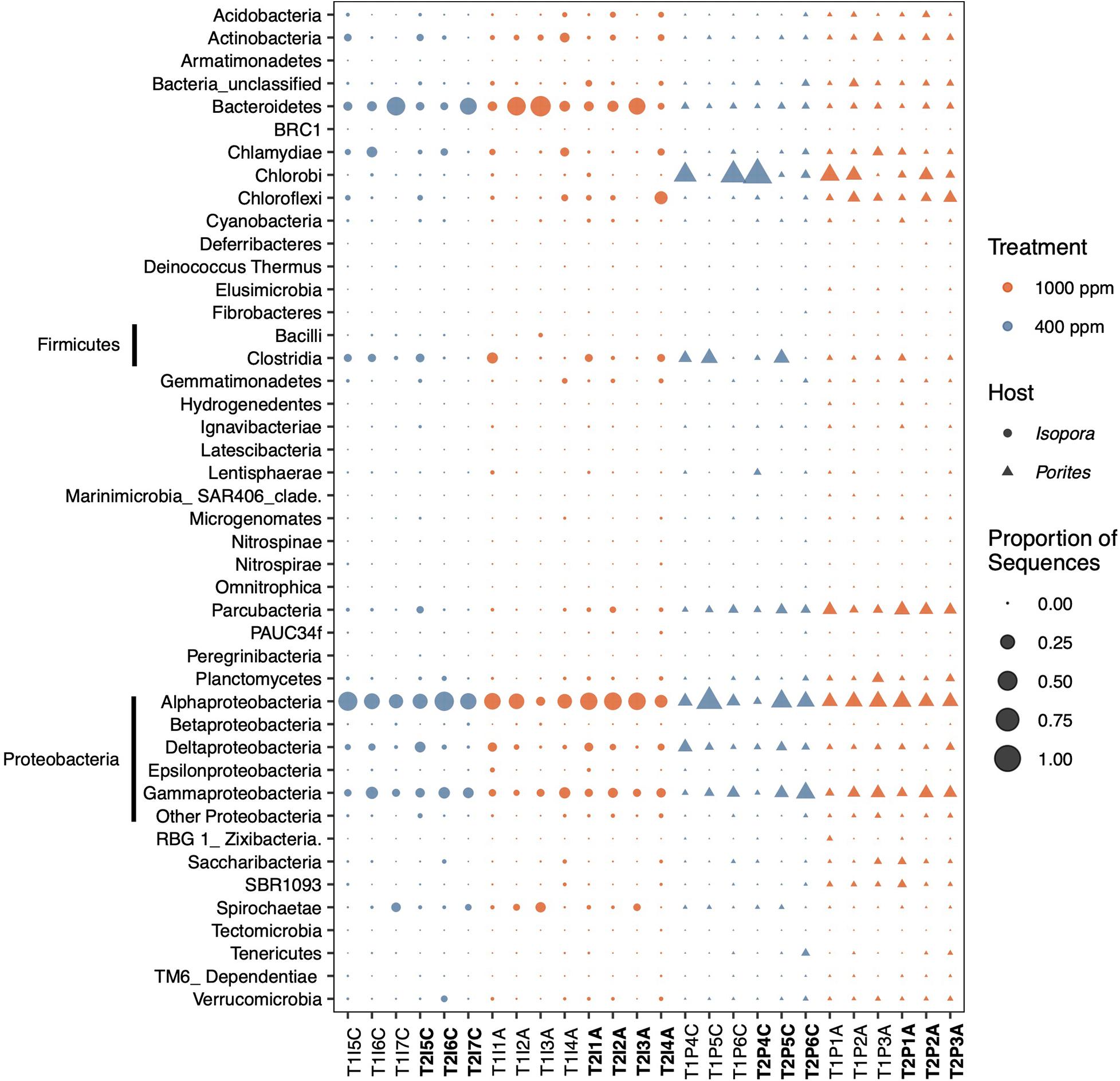
Figure 1. Profiles of the 38 most abundant phyla of endolithic bacteria in coral skeletons under pCO2 treatments of 1,000 ppm and 400 ppm. The phyla Firmicutes and Proteobacteria are divided at the class level. The sample name in bold is from the second sampling point.
The sequencing generate a total of 6,378,998 sequence from 52 sequencing samples. After a quality check and chimera removal, a total of 874,328 sequences and 4,619 OTUs were identified and used for subsequent analyses. Detailed information is in the supplementary OTU table. There were 1,158 OTUs (50 families) found only in I. palifera and 1,650 (48 families) only in P. lobata, and they share 1,811 OTUs (306 families) (Supplementary Figure 2). In both OTUs and family levels, the unique groups increased in both species under OA (Supplementary Figure 2).
Proteobacteria was the dominant endolithic bacterium (Figure 1) in the skeleton, accounting for 30–70% of the community in both I. palifera and P. lobata, the majority of which was Alphaproteobacteria, then Gammaproteobacteria (Figure 1). In I. palifera, Bacteroidetes was the sub-dominant group, followed by Firmicutes, Chloroflexi, Spirochaetae, and Chlamydiae. Porites lobata contained a greater variety of taxa in different colonies, the sub-dominant group of which was Chlorobi, followed by Firmicutes and Parcubacteria. The bacterial community compositions in the control colonies of I. palifera and P. lobata were significantly different (PERMANOVA, weighted unifrac, F = 5.06, and p < 0.005).
Under the OA, there was on average a slight increase in Actinobacteria (2%), Bacteroidetes (5%), and Chloroflexi (3%) compositions, and a 10% decrease in Proteobacteria in I. palifera (Figure 1). In Proteobacteria, Alphaproteobacteria decreased around 6% and Gammaproteobacteria decreased by 3% under OA. Within Alphaproteobacteria, order Rhodobacterales was dominant (28%) in the control set, but it dropped by 24% under OA; Rhizobiales and Rhodospirillales increased by 4% each and became dominant (Supplementary Figure 3A). In Gammaproteobacteria, orders Xanthomonadales, Vibrionales, and Legionellales had higher abundances in the control; Xanthomonadales and Oceanospirillales increased under the OA, but Vibrionales decreased (Supplementary Figure 3B). Despite these changes, the difference between control and acidified samples was not significant (Figure 2A, ANOSIM, R = 0.047, p = 0.265). Some genera fluctuated over the sampling times (samples marked with T1 and T2 in Figure 1); the most pronounced example of this was Bacteroidetes, which on average dropped to ∼5% in the control and 16% under the OA treatment. However, sampling time still had no significant effects on the endolithic community under control or acidification conditions (Supplementary Figure 4A, Shannon, Kruskal–Wallis test, p = 0.512 and 0.386, respectively). The same was true for the two dead colonies—there were no significant changes, even when after the colonies died.
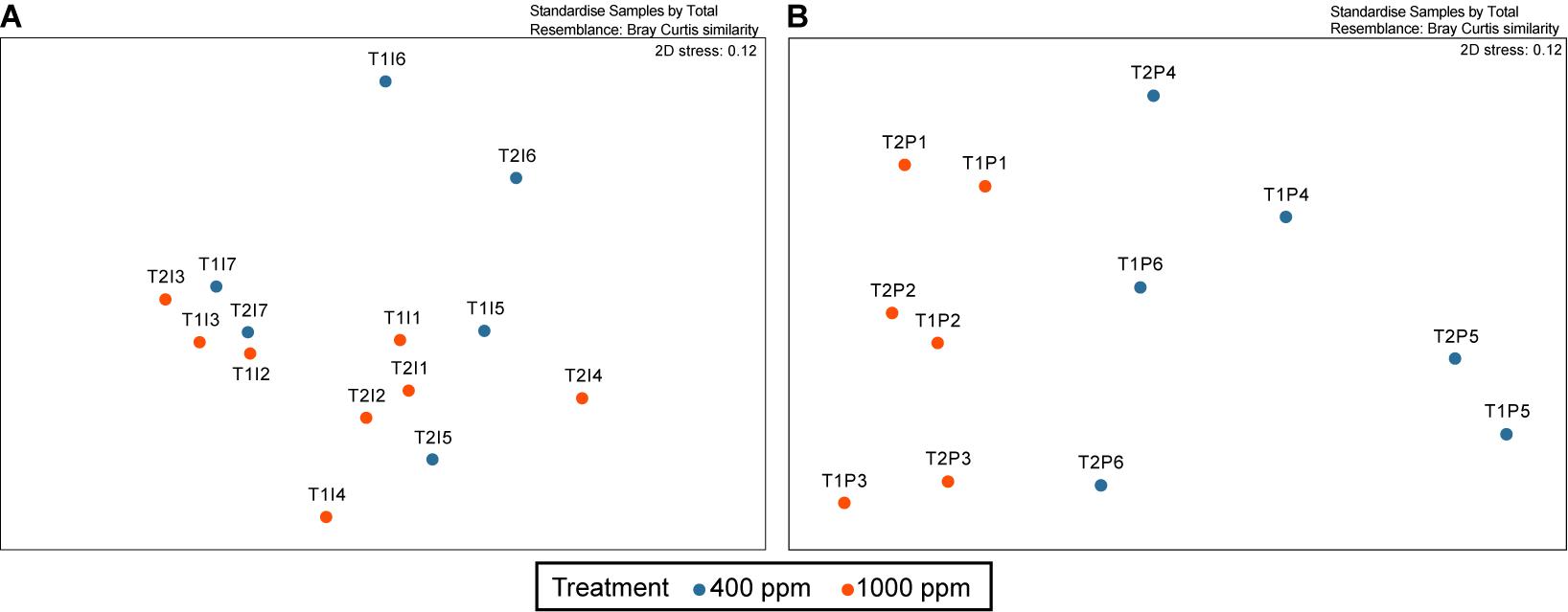
Figure 2. Non-metric multidimensional scaling (nMDS) analysis of the changing endolithic bacterial community in the (A) Isopora palifera and (B) Porites lobata colonies. The blue indicates coral colonies under 400 ppm pCO2 (control) and the orange indicates colonies under 1,000 ppm pCO2. The samples marked as T1 and T2 are represent the first and second sampling times, respectively.
The endolithic community in P. lobata changed significantly under OA treatment (Figure 2B, ANOSIM, R = 0.552, p < 0.005) compared with the control; on average, there was a reduction in Chlorobi (by 17%), Firmicutes (by 7%), and Proteobacteria (by 5%) and an increase in Parcubacteria (by 6%), Chloroflexi (by 7%), Actinobacteria (by 3%), Chlamydiae (by 3%), Planctomycetes (by 3%), Saccharibacteria (by 2%) and the group SBR1093 (by 2%) (Figure 1). Within Proteobacteria, the reductions were mainly in Deltaproteobacteria (by 3%) and Gammaproteobacteria (by 2%). Although there seemed to be no change in Alphaproteobacteria, there were differences at the order level: Parvularculales (10%) and Rhodospirillales (5%) were dominant in the control, whereas Rhizobiales (8%) and Rhodobacterales (5%) were dominant under the OA condition (Supplementary Figure 2A) Desulfobacterales is the major order in Deltaproteobacteria, and it decreased under OA (4% decrease). Myxococcales, however, increased by 1% under OA (Supplementary Figure 3C). In Gammaproteobacteria, Xanthomonadales decreased from 10% to 1% under OA (Supplementary Figure 3B). The incubation time also had no effects on the community diversity for either treatment (Supplementary Figure 4B, Shannon, Kruskal–Wallis test, p = 0.275 and 0.827 for control and acidification, respectively). Nevertheless, the proportion of Chlorobi dropped by 6% and 8% in the control and acidification treatments, respectively, and Firmicutes dropped by 5% in the control group (Figure 1).
According to the bacterial family compositions in Isopora and Porites (Supplementary Figure 5), both corals in the control had clear dominant bacterial families (Rhodobacteraceae in Isopora and Chlorobiaceae in Porites). However, after OA treatment, the relative abundance of the dominant families decreased in both corals (Supplementary Figure 5). The same pattern was found at the OTU level. The endolithic diversity varied among I. palifera samples (Figure 3A and Supplementary Figure 4A). The diversity in the T2 acidification set (Supplementary Figure 4A) was slightly higher than in other sets, but it was not significantly different among treatments (Supplementary Figure 3A, Shannon, Kruskal–Wallis test, to T1IA, p = 0.386; to T1IC, p = 0.479, to T2IC, p = 0.723). The endolithic community of P. lobata changed significantly under the acidified condition (Figure 3B, Shannon, Kruskal–Wallis test, p < 0.005). The control set in the second sampling shows a high variation in diversity among colonies (Supplementary Figure 4B) that is not significantly different from the diversities in the other sets. Under acidification conditions, however, the diversity was high in both sets and significantly different from the first control sampling (Shannon, Kruskal–Wallis test, p < 0.005).
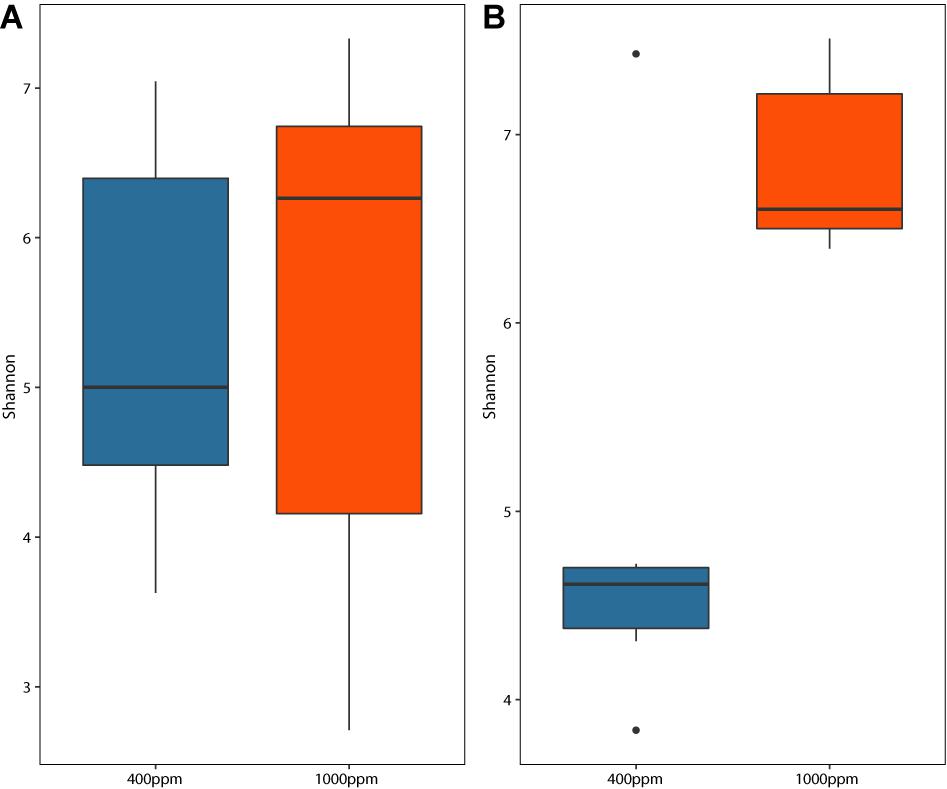
Figure 3. Shannon diversity boxplot of the endolithic bacterial communities in the (A) Isopora palifera and (B) Porites lobata colonies under two pCO2 treatments. Blue indicates the control (400 ppm pCO2) and orange indicates the acidification treatment (1,000 ppm pCO2).
Discussion
The highly synergistic relationship between coral hosts and their endosymbiotic zooxanthellae is considered the main contributor to primary production in the coral reef system; however, the endolithic community also plays an important role [reviewed in Pernice et al. (2020)]. The endolithic community may contribute a large amount of nutrients to the coral tissue through the skeletal pore-water (Ferrer and Szmant, 1988; Sangsawang et al., 2017). Previous studies on the endolithic community predominantly focused on the aerobic algae Ostreobium, cyanobacteria, and fungi, which may contribute photoassimilates (Fine and Loya, 2002; Tribollet et al., 2006) to the coral host. This contribution may provide the host with vital sustenance during bleaching (Fine and Loya, 2002). Recently, the endolithic bacterial community was found to contribute to nitrogen fixation (Yang et al., 2016), and anaerobic photoautotrophic green sulfur bacteria (GSB) under anoxygenic conditions were shown to act as a carbon source (Yang et al., 2019). The endolithic microbial functioning in the coral skeleton therefore may change under OA conditions through changes in the host-Symbiodiniaceae physiology and dissolution of carbonate substrates.
Porites lobata and I. palifera are two shallow water species that are highly affected by ocean acidification. In this study, the OA treatment had a greater effect on I. palifera tissue than did Porites lobata tissue. Porites has been found around naturally high pCO2 conditions (Marcelino et al., 2017; O’Brien et al., 2018; Jury et al., 2019) and is one of the popular genera for OA studies. As for Isopora, there is not much information on the host’s health conditions, but Iguchi et al. (2014) reported on I. palifera colony mortality under high pCO2 treatment and suggests that I. palifera hosts are susceptible to acidification. OA can affect photosynthetic capacity (Crawley et al., 2010), leading to coral bleaching and reducing host productivity and calcification rate (Anthony et al., 2008). Anthony et al. (2008) found that half of their crustose coralline algae and Acropora (a closely-related genus of Isopora) colonies bleached under the eight-week OA treatment, but only 10% of the Porites colonies bleached. The bleaching also allows more light to penetrate deeper into the skeleton and favor more photosynthetic groups in the green layer (Fine and Loya, 2002). Therefore, the condition of the coral host under OA treatment may result in the bacterial dynamics in coral skeleton. Our results suggest that the endolithic bacterial community was vaguely affected in I. palifera, but the increase in the abundance of photosynthetic groups (Chloroflexi and Rhodospirillales) under OA could be due to the bleaching and death of the host.
Skeleton formation differs between Isopora and Porites, and the differences further determine the sensitivities of each group to bioerosion/dissolution caused by microorganisms and photosynthetic microbes. The skeletal structure hence may be the major factor dictating how endolithic communities form and change. This study found that the endolithic community was minorly affected in I. palifera, but shifted remarkably in P. lobata, indicating that the skeleton’s structure and porous permeability determine the exchange and diffusion of nutrients and microbes between the host’s tissue and skeleton. Isopora palifera has a higher calcification rate and density than Porites (Razak et al., 2017; Yang et al., 2019). Structurally, Isopora has a sinuous axial corallite and irregular coenosteum structure and its growth is not aligned (Humblet et al., 2015). Porites has small and crowded polyps and shares a thin wall between corallites, the skeleton grows a mesh-like network constructed by vertical trabecular rods perpendicular to parallel short bars (Humblet et al., 2015); for P. lobata, the tissue perforates deep into the skeleton (Yost et al., 2013).
The skeletal traits have prominent effects on bioerosion. Reyes-Nivia et al. (2013) used freshly isolated coral skeleton (no tissue) to simulate bleaching conditions and observe the synergistic effects of warming and OA conditions on the microbioerosion of the coral skeleton. Their results showed that the biomass of endolithic algae increased under a high OA and warming treatment in both Isopora cuesta and Porites cylindrica, and the biomass in I. cuesta was higher than in P. cylindrica, but the skeleton dissolution rate of P. cylindrica was nearly twofold higher than in I. cuesta. The dense and poor porosity of the Isopora skeleton creates an enclosed environment for the endolithic community, which may restrain the space and nutrient exchange from the endolithic community to the tissue under acidification conditions. The skeleton of P. lobata, on the other hand, is less dense and more porous, so the tissue can extend down to the skeleton, which contains zooxanthellae (Yost et al., 2013), creating changes in the micro-environment (e.g., pH, oxygen) that might prominently affect the endolithic community. Several studies also showed that the skeleton of Porites is more sensitive to OA (Tribollet et al., 2009; Jury et al., 2019).
The endolithic community in I. palifera from Taiwan is diverse but dominated by anaerobic groups. The phyla that Yang et al. (2019) found to be dominance—Chlorobi, Chloroflexi, and Firmicutes—actually had low abundance in the present study. The main difference between these two studies is the morphology of I. palifera: Yang et al. (2019) used encrusting type samples and the present study used columnar type ones. Their main phyla are all anaerobic bacteria, which could be because the thick skeleton encrusts the benthic substrate to form an anaerobic environment. A columnar structure, on the other hand, is surrounded by host tissue that might be full of oxygen, and this study found that the resident bacteria had a variety of oxygen requirements. Since there is no information associated with I. palifera in other locations or with different morphologies, further studies are needed.
The P. lobata in this study under OA showed a major decrease in the relative abundances of the anaerobic bacteria Chlorobi and Firmicutes (the majority of which are in class Clostridia), and a minor decrease in order Desulfobacterales; on the other hand, facultative anaerobic groups like Chloroflexi, Actinobacteria, Chlamydiae, and Planctomycetes increased in abundance under OA, which might have led the skeleton to become a more aerobic environment. The bacterial community further diversified after a long incubation period. The observations in this study were different from those in a naturally high pCO2 seep environment (Marcelino et al., 2017; O’Brien et al., 2018). These previous surveys on endolithic (Marcelino et al., 2017) and whole coral bacterial (O’Brien et al., 2018) communities of the massive Porites spp. from two pCO2 seeps and the nearby control sites in Papua New Guinea found no significant difference between the controls and pCO2 seeps cites. However, the bacterial community differed highly between locations and had a higher diversity under one of the seep sites, regardless of the pH of the seep areas (O’Brien et al., 2018). This may imply that the main composition of the bacterial community is location-driven, and would therefore respond differently to OA. In both studies, the dominant phylum was Proteobacteria, but samples were sub-dominated by Bacteroidetes and Parvarchaeota, followed by Planctomycetes and Actinobacteria, but were low in Chlorobi and Firmicutes. Nevertheless, the porous skeleton could be a key factor making Porites stress-tolerant, as it makes the nutrients supplied by the endolithic community easily accessible to the tissue (Fine and Loya, 2002).
The coral skeleton is a habitat for numerous marine organisms and act as an essential carbon source/sink in the coral reef ecosystems. As OA increases, these organisms’ impacts on coral skeletons will be critical for coral reef ecological functions. Coral endolithic bacterial communities, especially their ecological functions to coral tissue, have been poorly studied in coral reef research compared with microbes in coral tissues. In addition, more studies are needed to determine whether the condition of the coral tissue will influence the endolithic community composition under OA (e.g., coral tissue thickness, energy reserves, and the associated Symbiodiniaceae). The results of the present study shed light on the endolithic associations in different coral species and their responses to future OA scenarios. In addition, the endolithic community composition is different from those found in Yang et al. (2019) and Marcelino et al. (2017), suggesting that location does play an important role on the endolithic community. Further investigations of other geographical regions with other microeukaryotes that have high biomass and could influence the nutrient cycle, will yield a more comprehensive understanding of the coral endolithic community dynamics.
Data Availability Statement
The datasets presented in this study can be found in online repositories. The names of the repository/repositories and accession number(s) can be found in the article/Supplementary Material.
Author Contributions
S-HY and S-LT conceived of the idea. HY, KS, and S-HY designed the research. HY and RD performed the coral sampling. RD and S-HY performed the experiment. S-YY, C-YL, and S-HY analyzed the data and wrote the manuscript. All authors edited and approved the manuscript.
Funding
We greatly thank the Ministry of Education, Taiwan, R.O.C., under Grant number MOST 109-2611-M-001-002. We also thank National Taiwan University, under Grant number 109L2033-70.
Conflict of Interest
The authors declare that the research was conducted in the absence of any commercial or financial relationships that could be construed as a potential conflict of interest.
Acknowledgments
We thank reviewers’ efforts toward improving this manuscript. We appreciate Sylvain Agostini and Ben Harvey for their technical suggestions. We also thank the support from Cristin Manullang, Kshitij Tandon for his analysis support and Noah Last of Third Draft Editing for editing the manuscript’s language. The experiment was supported by Academia Sinica, Taipei, Taiwan and the University of the Ryukyus, Okinawa, Japan.
Supplementary Material
The Supplementary Material for this article can be found online at: https://www.frontiersin.org/articles/10.3389/fmars.2020.603293/full#supplementary-material
Supplementary Figure 1 | Non-metric multidimensional scaling (nMDS) analysis of the changing endolithic bacterial community in the (A) Isopora palifera and (B) Porites lobata colonies. The gray indicates coral colonies under 400 ppm pCO2 (control) and the pink indicates colonies under 1,000 ppm pCO2. The triangle and circles represent the first and second sampling times, respectively. All the sample replicates are plotted.
Supplementary Figure 2 | The Venn diagram of OTU (up) and family (down) for each treatment group.
Supplementary Figure 3 | Order level of the relatively abundant of (A) Alphaproteobacteria, (B) Gammaproteobacteria, and (C) Deltaproteobacteria in each treatment. IC, I. palifera control treatment; IA, I. palifera acidification treatment; PC, P. lobata control treatment; PA, P. lobata acidification treatment.
Supplementary Figure 4 | Shannon diversity boxplot of the endolithic bacterial communities in the (A) Isopora palifera and (B) Porites lobata colonies under two pCO2 treatments and two sampling points. Green indicates the control treatment and orange indicates the acidification treatment.
Supplementary Figure 5 | The relatively abundant in family level for all treatments.
Footnotes
- ^ http://www.r-project.org/
- ^ http://bioinformatics.psb.ugent.be/webtools/Venn/
- ^ http://ncbi.nlm.nih.gov
References
Anthony, K. R., Kline, D. I., Diaz-Pulido, G., Dove, S., and Hoegh-Guldberg, O. (2008). Ocean acidification causes bleaching and productivity loss in coral reef builders. Proc. Natl. Acad. Sci. 105, 17442–17446. doi: 10.1073/pnas.0804478105
Bolyen, E., Rideout, J. R., Dillon, M. R., Bokulich, N. A., Abnet, C. C., Al-Ghalith, G. A., et al. (2019). Reproducible, interactive, scalable and extensible microbiome data science using QIIME 2. Nat. Biotechnol. 37, 852–857. doi: 10.1038/s41587-019-0209-9
Caldeira, K., and Wickett, M. E. (2003). Anthropogenic carbon and ocean pH. Nature 425, 365–365. doi: 10.1038/425365a
Chazottes, V., Campion-Alsumard, T. L., and Peyrot-Clausade, M. (1995). Bioerosion rates on coral reefs: interactions between macroborers, microborers and grazers (Moorea, French Polynesia). Palaeogeogr. Palaeoclimatol. Palaeoecol. 113, 189–198. doi: 10.1016/0031-0182(95)00043-L
Chen, C.-P., Tseng, C.-H., Chen, C. A., and Tang, S.-L. (2011). The dynamics of microbial partnerships in the coral Isopora palifera. ISME J. 5, 728–740. doi: 10.1038/ismej.2010.151
Clarke, K. R., and Gorley, R. N. (2005). PRIMER: Getting started with v6. Devon: Primer-E Ltd Plymouth UK.
Crawley, A., Kline, D. I., Dunn, S., Anthony, K. E. N., and Dove, S. (2010). The effect of ocean acidification on symbiont photorespiration and productivity in Acropora formosa. Glob.Change Biol. 16, 851–863. doi: 10.1111/j.1365-2486.2009.01943.x
De Los Ríos, A., Wierzchos, J., Sancho, L. G., Green, T. G. A., and Ascaso, C. (2005). Ecology of endolithic lichens colonizing granite in continental Antarctica. Lichenologist 37, 383–395. doi: 10.1017/S0024282905014969
del Campo, J., Pombert, J., and Šlapeta, J. (2017). The ‘other’ coral symbiont: Ostreobium diversity and distribution. ISME J. 11, 296–299. doi: 10.1038/ismej.2016.101
Dunn, R. J. H., Stanitski, D. M., Gobron, N., Willett, K. M., Ades, M., Adler, R., et al. (2020). Global Climate. Bull. Am. Meteorol. Soc. 101, S9–S128. doi: 10.1175/BAMS-D-20-0104.1
Edgar, R. C. (2013). UPARSE: highly accurate OTU sequences from microbial amplicon reads. Nat. Methods 10, 996–998. doi: 10.1038/nmeth.2604
Ferrer, L. M., and Szmant, A. M. (1988). “Nutrient regeneration by the endolithic community in coral skeletons,” in Proc. 6th Intern. Coral Reef Symp, (Australia), 1–4.
Fine, M., and Loya, Y. (2002). Endolithic algae: an alternative source of photoassimilates during coral bleaching. Proc. R. Soc. Lond. B Biol. Sci. 269, 1205–1210. doi: 10.1098/rspb.2002.1983
Ghirardelli, L. A. (2002). Endolithic microorganisms in live and dead thalli of coralline red algae (Corallinales, Rhodophyta) in the Northern Adriatic Sea. Acta geológica hispánica 37:8.
Hofmann, G. E., Barry, J. P., Edmunds, P. J., Gates, R. D., Hutchins, D. A., Klinger, T., et al. (2010). The effect of ocean acidification on calcifying organisms in marine ecosystems: An organism-to-ecosystem perspective. Annu. Rev. Ecol. Evol. Syst. 41, 127–147. doi: 10.1146/annurev.ecolsys.110308.120227
Humblet, M., Hongo, C., and Sugihara, K. (2015). An identification guide to some major Quaternary fossil reef−building coral genera (Acropora, Isopora, Montipora, and Porites). Isl. Arc. 24, 16–30. doi: 10.1111/iar.12077
Iguchi, A., Kumagai, N. H., Nakamura, T., Suzuki, A., Sakai, K., and Nojiri, Y. (2014). Responses of calcification of massive and encrusting corals to past, present, and near-future ocean carbon dioxide concentrations. Mar. Pollut. Bull. 89, 348–355. doi: 10.1016/j.marpolbul.2014.09.037
Jury, C. P., Delano, M. N., and Toonen, R. J. (2019). High heritability of coral calcification rates and evolutionary potential under ocean acidification. Sci. Rep. 9:20419. doi: 10.1038/s41598-019-56313-1
Kroeker, K. J., Kordas, R. L., Crim, R., Hendriks, I. E., Ramajo, L., Singh, G. S., et al. (2013). Impacts of ocean acidification on marine organisms: quantifying sensitivities and interaction with warming. Glob. Change Biol. 19, 1884–1896. doi: 10.1111/gcb.12179
Marcelino, V. R., Morrow, K. M., Oppen, M. J. H., van, Bourne, D. G., and Verbruggen, H. (2017). Diversity and stability of coral endolithic microbial communities at a naturally high pCO2 reef. Mol. Ecol. 26, 5344–5357. doi: 10.1111/mec.14268
O’Brien, P. A., Smith, H. A., Fallon, S., Fabricius, K., Willis, B. L., Morrow, K. M., et al. (2018). Elevated CO2 has little influence on the bacterial communities associated with the pH-tolerant coral, massive Porites spp. Front. Microbiol. 9:2621. doi: 10.3389/fmicb.2018.02621
Pachauri, R. K., and Mayer, L., and Intergovernmental Panel on Climate Change. (2015). Climate change 2014: synthesis report, eds Edn. Geneva: Intergovernmental Panel on Climate Change.
Pandolfi, J. M., Connolly, S. R., Marshall, D. J., and Cohen, A. L. (2011). Projecting coral reef futures under global warming and ocean acidification. Science 333, 418–422. doi: 10.1126/science.1204794
Pernice, M., Raina, J. B., Rädecker, N., Cárdenas, A., Pogoreutz, C., and Voolstra, C. R. (2020). Down to the bone: the role of overlooked endolithic microbiomes in reef coral health. ISME J. 14, 325–334. doi: 10.1038/s41396-019-0548-z
Quast, C., Pruesse, E., Yilmaz, P., Gerken, J., Schweer, T., Yarza, P., et al. (2013). The SILVA ribosomal RNA gene database project: improved data processing and web-based tools. Nucleic Acids Res. 41, D590–D596.
R Core Team. (2017). R: A language and environment for statistical computing. Vienna: R Foundation for Statistical Computing.
Radtke, G., Le Campion-Alsumard, T., and Golubić, S. (1996). Microbial assemblages of the bioerosional. Algol. Stud. Für Hydrobiol. Suppl 1996, 469–482. doi: 10.1127/algol_stud/83/1996/469
Razak, T. B., Mumby, P. J., Nguyen, A. D., Zhao, J.-X., Lough, J. M., Cantin, N. E., et al. (2017). Use of skeletal Sr/Ca ratios to determine growth patterns in a branching coral Isopora palifera. Mar. Biol. 164:96. doi: 10.1007/s00227-017-3099-8
Reyes-Nivia, C., Diaz-Pulido, G., Kline, D., Guldberg, O.-H., and Dove, S. (2013). Ocean acidification and warming scenarios increase microbioerosion of coral skeletons. Glob. Change Biol. 19, 1919–1929. doi: 10.1111/gcb.12158
Sangsawang, L., Casareto, B. E., Ohba, H., Vu, H. M., Meekaew, A., Suzuki, T., et al. (2017). 13C and 15N assimilation and organic matter translocation by the endolithic community in the massive coral Porites lutea. R. Soc. Open Sci. 4:171201. doi: 10.1098/rsos.171201
Schloss, P. D., Westcott, S. L., Ryabin, T., Hall, J. R., Hartmann, M., Hollister, E. B., et al. (2009). Introducing mothur: Open-source, platform-independent, community-supported software for describing and comparing microbial communities. Appl. Environ. Microbiol. 75, 7537–7541. doi: 10.1128/AEM.01541-09
Shashar, N., Cohen, Y., Loya, Y., and Sar, N. (1994). Nitrogen fixation (acetylene reduction) in stony corals: evidence for coral-bacteria interactions. Mar. Ecol. Prog. Ser. 111, 259–264. doi: 10.3354/meps111259
Tambutté, E., Venn, A. A., Holcomb, M., Segonds, N., Techer, N., Zoccola, D., et al. (2015). Morphological plasticity of the coral skeleton under CO2-driven seawater acidification. Nat. Commun. 6:7368. doi: 10.1038/ncomms8368
Tribollet, A., Godinot, C., Atkinson, M., and Langdon, C. (2009). Effects of elevated pCO2 on dissolution of coral carbonates by microbial euendoliths. Glob. Biogeochem. Cycles 23, 1–7. doi: 10.1029/2008GB003286
Tribollet, A., Langdon, C., Golubic, S., and Atkinson, M. (2006). Endolithic microflora are major primary producers in dead carbonate substrates of Hawaiian coral reefs1. J. Phycol. 42, 292–303. doi: 10.1111/j.1529-8817.2006.00198.x
Yang, S.-H., Lee, S. T. M., Huang, C.-R., Tseng, C.-H., Chiang, P.-W., Chen, C.-P., et al. (2016). Prevalence of potential nitrogen-fixing, green sulfur bacteria in the skeleton of reef-building coral Isopora palifera. Limnol. Oceanogr. 61, 1078–1086. doi: 10.1002/lno.10277
Yang, S.-H., Tandon, K., Lu, C.-Y., Wada, N., Shih, C.-J., Hsiao, S. S.-Y., et al. (2019). Metagenomic, phylogenetic, and functional characterization of predominant endolithic green sulfur bacteria in the coral Isopora palifera. Microbiome 7:3. doi: 10.1186/s40168-018-0616-z
Yilmaz, P., Parfrey, L. W., Yarza, P., Gerken, J., Pruesse, E., Quast, C., et al. (2014). The SILVA Database; All-species living tree project (LTP); taxonomic frameworks. Nucleic Acids Res. 42, D643–D648.
Keywords: Isopora palifera, Porites lobata, ocean acidification, endolith, Okinawa
Citation: Yang S-Y, Lu C-Y, Tang S-L, Das RR, Sakai K, Yamashiro H and Yang S-H (2020) Effects of Ocean Acidification on Coral Endolithic Bacterial Communities in Isopora palifera and Porites lobata. Front. Mar. Sci. 7:603293. doi: 10.3389/fmars.2020.603293
Received: 06 September 2020; Accepted: 26 November 2020;
Published: 18 December 2020.
Edited by:
Hajime Kayanne, The University of Tokyo, JapanReviewed by:
Claudia Pogoreutz, University of Konstanz, GermanyAnthony William Larkum, University of Technology Sydney, Australia
Copyright © 2020 Yang, Lu, Tang, Das, Sakai, Yamashiro and Yang. This is an open-access article distributed under the terms of the Creative Commons Attribution License (CC BY). The use, distribution or reproduction in other forums is permitted, provided the original author(s) and the copyright owner(s) are credited and that the original publication in this journal is cited, in accordance with accepted academic practice. No use, distribution or reproduction is permitted which does not comply with these terms.
*Correspondence: Shan-Hua Yang, c2hhbmh1YUBudHUuZWR1LnR3
†These authors share first authorship