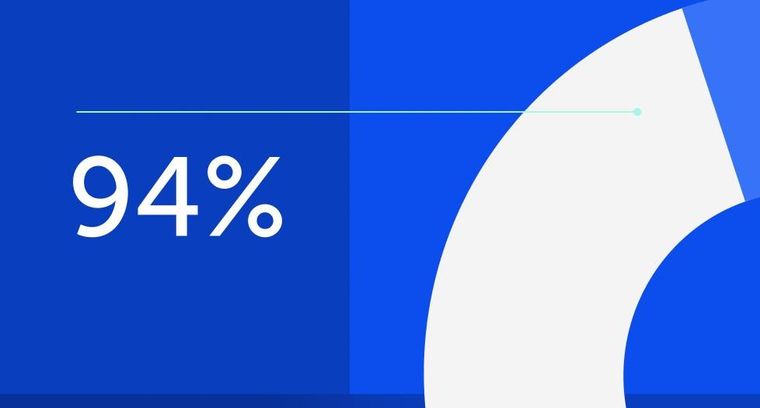
94% of researchers rate our articles as excellent or good
Learn more about the work of our research integrity team to safeguard the quality of each article we publish.
Find out more
ORIGINAL RESEARCH article
Front. Mar. Sci., 14 January 2021
Sec. Marine Ecosystem Ecology
Volume 7 - 2020 | https://doi.org/10.3389/fmars.2020.598132
Mangroves sustain high soil accretion and carbon sequestration rates, yet it is still unknown if they can keep pace with increasing sea level rise (SLR) across a wider range of coastal geomorphic settings. Because accretion rates are controlled by mineral sediment inputs and organic matter accumulation, it is paramount to assess the relative contribution of root productivity to soil formation. Here, we evaluated root biomass, production, and turnover in three mangrove ecotypes to evaluate the role of soil nutrient limitation, stressors, and hydroperiod in controlling root dynamics in San Andres Island (SAI), a karstic oceanic island in the Caribbean Sea. Root production was modulated by soil stress conditions and not by nutrient availability as it has been reported for other karstic environments. The lowest root biomass allocation, and both production and turnover of fine roots were measured under low flooding duration, and low salinity (<20 PSU) and sulfide concentrations (0.84 ± 0.4 mM). Yet, when soil stress conditions increased during high flooding duration (6207 h y–1) and low oxygen conditions (Eh), root tissues reached the highest biomass and production values, including a relative fast turnover of fine roots (<2 mm; 0.75 y–1). Our results follow the predictions of the plant root longevity cost-benefit hypothesis where plants maintain roots only until the efficiency of resource acquisition is maximized by water and nutrient acquisition. Because of the importance of groundwater in controlling porewater salinity and mangrove root productivity in karstic oceanic islands such as SAI, water use and coastal development should be regulated in the short term to avoid the loss of mangrove area and concomitant ecosystem services.
The provision of ecosystem services by coastal wetlands is recognized worldwide, yet most of these services are under increasing threat due to the combined impacts of natural and human disturbances and accelerating sea level rise (SLR) as result of climate change (McLeod et al., 2011; Murdiyarso et al., 2015; Lovelock et al., 2017). Mangrove extension in tropical and subtropical latitudes has declined especially in areas undergoing urban, aquaculture and agricultural expansion, deforestation, and landscape level hydrological alterations (Kairo et al., 2008; Friess and Webb, 2014; Thomas et al., 2017). Among the ecosystem services under major risks are carbon storage and sequestration, which contribute to soil formation, consolidation, and stability (Chmura et al., 2003; McKee et al., 2007; Walters et al., 2008; Donato et al., 2011). The mitigation of greenhouse gas emissions through carbon storage and sequestration in vegetation (above- and belowground) and soil has also been recognized as an important ecosystem service for mangroves (McLeod et al., 2011; Murdiyarso et al., 2015). Consequently, determining ecosystem services both ecological and economic value to mitigate future negative impacts is a major priority (Jerath et al., 2016). However, any economic assessment requires a comprehensive and accurate evaluation, not only of the mangrove extension, but also estimation of above- and belowground (total) mangrove biomass and production across different environmental settings (Woodroffe et al., 2016; Atwood et al., 2018; Twilley et al., 2018). Unfortunately, total biomass and productivity data are available in few mangrove ecotypes (i.e., riverine, fringe, basin, scrub; sensu Lugo and Snedaker, 1974) and generally limited to the quantification of aboveground biomass and litterfall production (Alongi, 2002; Bouillon et al., 2008; Castañeda-Moya et al., 2013; Rivera-Monroy et al., 2013; Rovai et al., 2016; Rivera-Monroy et al., 2019). Although the quantification of mangrove belowground biomass and productivity have advanced during the last 20 years, there is still a lack of understanding about the magnitude of this production and root dynamics across ecogeomorphic settings and latitudinal gradients (Saintilan, 1997; Komiyama et al., 2000; Gleason and Ewel, 2002; McKee et al., 2007; Tamooh et al., 2008; Castañeda-Moya et al., 2011; Adame et al., 2014; Cormier et al., 2015). This is the case of oceanic islands (Cormier et al., 2015) and semi-arid and arid coastal regions (Ochoa-Gomez et al., 2019; Saderne et al., 2019) in the Atlantic East Pacific (AEP) mangrove biogeographic region (Duke, 2017; Rivera-Monroy et al., 2017a).
Mangrove biomass and productivity vary not only across diverse ecogeomorphic settings at regional scales (> 1 km2), but also within regions at the local scale (< 1 ha) (Rivera-Monroy et al., 2013, 2017b; Rovai et al., 2016; Twilley et al., 2017; Simard et al., 2019). Environmental factors that explain differences in mangrove productivity and forest structure at this scale have been grouped into three operational categories: soil regulators (e.g., salinity, sulfide concentrations, oxidation-reduction potential (Eh/mV) (Koch et al., 1990; Pezeshki and Delaune, 2012), resources (e.g., nitrogen, N; phosphorus, P), and hydroperiod (frequency, duration, and depth of flooding) (Twilley and Rivera-Monroy, 2005, 2009; Woodroffe et al., 2016). The magnitudes and interactions among these factors may result in either promoting forest productivity and vegetation expansion or causing physiological stress to the plant that limit tree growth and development (Twilley and Rivera-Monroy, 2005; Kristensen et al., 2017; Osland et al., 2018; Castañeda-Moya et al., 2020; He et al., in press). Indeed, nutrient limitation in terrestrial and wetland ecosystems can result in vegetation allocating more biomass to belowground roots relative to aboveground, maximizing the efficiency for capturing the limited resources and increasing root:shoot ratios (Grime, 1977; Chapin, 1980; Nadelhoffer et al., 1985; Chapin et al., 1987; Gleeson and Tilman, 1992; Castañeda-Moya et al., 2011; Cormier et al., 2015). However, the few studies partially assessing the interaction among resources, regulators, and hydroperiod in mangroves shown complex and incomplete patterns (Berger et al., 2008; Krauss et al., 2008; Atwood et al., 2018; Castañeda-Moya et al., 2020).
Although porewater salinity is recognized as one key regulator exerting the greatest impact on plant growth rates, mangrove species composition/zonation and production, particularly in dry environments (Cintron et al., 1978; Ball, 1988, 1998; Medina and Francisco, 1997; Castañeda-Moya et al., 2006; Jayatissa et al., 2008; Ochoa-Gomez et al., 2019), it is not clear how root productivity is controlled by salinity (Wang et al., 2011; Krauss and Ball, 2013), sulfide or hydroperiod across ecotypes within different environmental settings (e.g., karstic, deltaic) (Woodroffe et al., 2016). While greenhouse experiments have shown that salinity promotes an increase in root biomass compared to aboveground (Ball, 1998; Cardona-Olarte et al., 2006), field studies have not directly partition the salinity effect on resource allocation to root growth (Downton, 1982; Burchett et al., 1984; Naidoo, 1987, 1989, 1990; Ball and Pidsley, 1995). Similarly, sulfide (H2S)—a strong phytotoxin in wetlands controlled by O2 availability and soil oxidation-reduction conditions (Pezeshki and Delaune, 2012)— is known to negatively impact nutrient uptake and plant growth across different species. Thus, H2S is considered a major factor controlling the spatial distribution of mangrove species, especially in areas where flooding duration is long (Nickerson and Thibodeau, 1985; Thibodeau and Nickerson, 1986; McKee, 1993, 1995; Lamers et al., 2013).
Hydroperiod is a critical variable controlling mangrove forest structural and productivity spatiotemporal patterns (Castañeda-Moya et al., 2013; Rivera-Monroy et al., 2017b; Cameron et al., 2019; Zhao et al., 2020), yet it has been difficult to make generalizations about its relative contribution in natural settings given the close interaction with other hydrological and climate variables that influence the frequency, duration, and depth of inundation (Mitsch and Gosselink, 2015). Although some studies have concluded that hydroperiod is the main driver of the spatial pattern of dominance in mangrove communities (e.g., Crase et al., 2013), other reports caution about making generalizations given the multi-factorial and inter-correlated nature of abiotic and biotic variables in mangroves, particularly when using statistical models (e.g., Clarke, 2014). Mangrove species responses to hydroperiod in greenhouse studies indicate that distinct root biomass allocation patterns are driven by species-specific relationships. In the case of the species Avicennia germinans, for example, an increase in flooding duration was directly related with an increase in root biomass. In contrast, root biomass decreased with increasing frequency of inundation when Rhizophora mangle and Laguncularia racemosa co-existed (e.g., Krauss et al., 2006). Studies in natural environments show that low frequency and high duration of flooding affect mangrove root production and the magnitude of energy in root biomass allocation (McKee, 2011). This allocation pattern has also been observed in karstic environments (i.e., Florida Everglades; Castañeda-Moya et al., 2011), although the response was attributed to lower root production linked to a close interaction between lower flooding frequency and high soil total phosphorus concentrations, instead of a longer flooding duration. Conversely, high root biomass allocation was observed in scrub mangroves flooded for long periods (∼8600 h y–1), but when salinity was < 35 PSU (Castañeda-Moya et al., 2011). Few field studies have evaluated the relative role and interaction of the most critical environmental factors limiting belowground productivity, especially in karstic geomorphic settings. This type of setting is dominant in the AEP northern latitudes throughout the extensive Caribbean region (2,754,000 km2) where human impacts are increasing during the last four decades and directly threating mangrove resources and ecosystem services due to deforestation and freshwater demands.
The objective of this study was to evaluate the interaction and relative effect of regulators (salinity, Eh, and sulfide), resources (soil N and P), and hydroperiod (frequency, duration, and depth of flooding) on mangrove forest belowground biomass and production in an oligotrophic karstic oceanic island in the Caribbean region. We hypothesized that an increase in root biomass, productivity and root turnover was directly associated with an increase in soil stress conditions. Here we considered a stressful condition for mangrove trees when soil total N (< 3 mg cm–3) and P (< 0.3 mg cm–3) concentrations are low under both high soil porewater salinity (> 50 PSU) and sulfide concentrations (> 0.5 mM), which are modulated by local hydroperiod regimes (McKee, 1993; Suarez and Medina, 2006; Castañeda-Moya et al., 2011; Lamers et al., 2013). Specifically, we addressed the following questions: (1) What are the spatiotemporal patterns of belowground biomass and productivity in response to differences in flooding duration and frequency in different ecotypes across the island? and (2) How do fine root productivity and root turnover vary in response to salinity, soil reducing conditions and nutrient gradients?
This study was conducted in the San Andrés Island (hereafter, SAI) (12° 29′ and 12° 36′ N, 81° 41′ and 81° 43′ W), an oceanic karstic island in the Caribbean Sea, located approximately 750 km northwest from the Colombian coast. This island is part of the Sea Flower International Biosphere Reserve and the largest of an island group forming the San Andrés, Providencia and Santa Catalina Archipelago (Figure 1). SAI is ∼13 km in length and has an area of 27 km2 representing 60% of the total Archipelago area. This karstic island originates from Tertiary calcareous rocks and recent deposits of the Quaternary (Vargas, 2004). The climate is humid and dry with average annual precipitation of 1973 mm with three distinct periods (Figure 2A, mean period 1960–2015; Figure 2B, 2013): a rainy season (80% of the precipitation) from June to November, a dry season from January to April, and two transition months (May and December; Gavio et al., 2010). Mean annual air temperature is 27°C and ranges from 25 to 30°C (Howard et al., 2012).
Figure 1. Location of mangrove forest, study sites and plots in the San Andrés Island, Colombia. Old Point Mangrove Regional Park (OPMRP); fringe forest (F1–F5); basin forest (B1, B2, B3); Smith Channel Inland forest (I1–I6). Mangrove areas: Cocoplum Bay (CB), Sound Bay (SB), Salt Creek (SCR), Smith Chanel (SC), OPMRP, Cove (C).
Figure 2. Average monthly precipitation (mm) in the San Andrés island. (A) Period: 1960–2015. (B) Mean precipitation in 2013. Source: meteorological station of the Institute of Hydrology, meteorology, and environmental studies (IDEAM, 2017).
Mangroves in SAI are distributed within six main areas. Cocoplum Bay (CB), Sound Bay (SB), Salt Creek (SCR), and Smith Chanel (SC) areas are dominated by tall developed riverine mangroves, while Old Point Mangrove Regional Park (OPMRP) and the Cove (C) areas, located on the western side of the island have been classified as fringe forests (Urrego et al., 2009). The study was conducted in OPMRP and SC, located in the island’s north and southeast regions, respectively (Figure 1). At OPMRP, two mangrove forest ecotypes (fringe and basin; sensu Lugo and Snedaker, 1974) were selected as study sites. This mangrove area includes the only basin forest on the island and encompasses ∼90% of the total island’s fringe forests. The fringe mangrove forest is dominated by R. mangle representing 79% of total tree density, followed by A. germinans (15%), and L. racemosa (6%), with an average canopy height of 6.6 ± 0.1 m, and a diameter at breast height (DBH) of 7 ± 0.1 cm (Sánchez-Nuñez and Mancera-Pineda, 2011; Medina-Calderón, 2016). Additionally, individuals of Conocarpus erectus, a mangrove associate, occur in the forest interior zone, primarily at higher elevations. Soil elevation in this site ranges from 0.23 to 0.3 m (mean sea level, MSL). Mangroves in this area are influenced by rainfall and semi-diurnal tides with a mean amplitude of ∼32 cm (IDEAM, 2017), which regulate porewater salinity (<35 PSU; Urrego et al., 2009; Sánchez-Nuñez and Mancera-Pineda, 2011; Medina-Calderón, 2016; Rodriguez-Rodriguez et al., 2018). The basin forest in OPMRP is dominated by A. germinans (58%), with a smaller contribution by L. racemosa (23%) and R. mangle (20%). The forest is characterized by an average tree height of 7.6 ± 0.2 m and a DBH of 8.9 ± 0.3 cm (Sánchez-Nuñez and Mancera-Pineda, 2011; Medina-Calderón, 2016). Salinity values in this forest (> 50 PSU) are influenced by rainfall and semi diurnal tides (Sánchez-Nuñez and Mancera-Pineda, 2011; Medina-Calderón, 2016). Soil elevation in this forest type is lower compared to the fringe site, decreasing from 0.20 m MSL in the north side to −0.20 m MSL in the south side of the forest. In addition, the presence of a ridge formation with higher elevation in the southeast side of the forest restricts water exchange with the ocean. Thus, poor draining and permanent flooding conditions are observed in this site during spring tides.
A third site classified in this study as an inland forest was selected within the SC mangrove area (18.1 ha). R. mangle and L. racemosa co-occur in this site with an average canopy height of 21.7 ± 0.7 m (and maximum in the island) and a DBH of 30 ± 1.2 cm (Sánchez-Nuñez and Mancera-Pineda, 2011; Medina-Calderón, 2016) representing the highest forest development within the island (Howard et al., 2012). The mangrove forest extends ∼120 m from the shoreline and lacks direct water exchange with streams, rivers, or tidal channels (Figure 1). The presence of a sand dune restricts direct water exchange with the sea (Medina-Calderón, 2016). Soil elevation ranges from 0 to 2.5 m relative to MSL in less than 20 m from the shoreline due to the presence of the dune and decreases to 0.7 m MSL toward the interior of the forest (Medina-Calderón, 2016). Freshwater inputs to the inland mangrove forest include both rainfall and groundwater flow, which maintain porewater salinity at < 10 PSU throughout the year (Urrego et al., 2009; Rodriguez-Rodriguez et al., 2018). During the rainy season, the forest remains flooded and water loss is due to evaporation. This mangrove area is comparable to other karstic coastal wetland ecosystems in the AEP region such as the Florida Everglades (tree islands; Sklar and van der Valk, 2002) and the northern Yucatan Peninsula (i.e., “Petenes”; López-Portillo et al., 1989), where groundwater is the main freshwater source to forest ecosystems and urban/agriculture areas (Rivera-Monroy et al., 2020). Mangroves in the inland study site thrive above an extensive natural aquifer (1310 ha) that comprises 48% of the island area and supplies freshwater to the local villages (Guzmán and Hernandez, 2012).
We established a total of 14 plots in three mangrove sites (fringe, basin, inland). The plot (20 × 20 m) location was determined by the forest spatial extension and distribution and usually followed a northeast/southwest direction (Figure 1). The number of plots varied due to the extension of each mangrove ecotype and to capture the potential spatial variation in forest structure within each site (Medina-Calderón, 2016), as implemented in other studies (e.g., Ochoa-Gomez et al., 2019; Rivera-Monroy et al., 2019). Six plots were established perpendicular to the shoreline (separated ∼50 m each one) in the inland mangrove forest. Five plots parallel to the shoreline were established in the fringe mangrove forest, 5 m from the mangrove edge; three in the north side and two in the south side (also separated 50 m each one). In the basin forest, three plots were established 50 m apart (Figure 1). All vegetation plots were considered as experimental units and treated as replicates within each forest ecotype.
For the estimation of in situ root biomass, six soil cores were collected in each of the 14 plots using a PVC coring device (10.2 cm in diameter × 45 cm length; Castañeda-Moya et al., 2011) in January 2013. All cores were maintained at low temperature (∼4°C) until further processing in the laboratory. Samples were processed separately and initially washed with fresh water to separate soil particles using a 1 mm sieve. Live roots were separated manually considering their buoyancy, turgor and color. Root samples were then sorted by diameter into three size classes: fine (< 2 mm), small (2–5 mm), and coarse (5–20 mm) roots (Castañeda-Moya et al., 2011). Roots greater than 20 mm in diameter were not included in the analysis due to sampling limitations (i.e., core area). All root samples were oven-dried at 60°C for 72 h until constant weight and weighed using an analytical scale (± 0.0001 g). Root estimates were expressed in g m–2 of dry mass.
Root production was evaluated using the ingrowth core soil implant technique (Flower-Ellis and Persson, 1980; Persson, 1983). Ingrowth cores (10 cm in diameter x 45 cm length) were made of a synthetic material (3 mm mesh) and filled with commercial root-free soil. This material had similar soil properties to mangrove peat in our study sites, including bulk density (0.63 mg cm–3), organic matter (OM) content (18%), and total carbon (120 mg cm–3). Nine ingrowth cores were implanted in the same holes from which we sampled root biomass at each of the 14 experimental plots. Duplicate or triplicate root cores were harvested at 12, 18, and 24 months and processed individually following the same methodology used for biomass estimation. Originally, we deployed 12 ingrown cores to measure production after 36 months, but we lost up to three initial cores due to vandalism. Thus, the number cores for initial root biomass measurements were adjusted accordingly. Total root production (dry biomass g m–2 y–1) represents the accumulation in biomass of fine, small, and coarse roots within an ingrowth bag during a specific time interval. Root turnover rate was calculated by dividing root production by the in situ biomass (Eissenstat and Yanai, 1997). Root longevity (y–1) was calculated as the inverse of root turnover (Castañeda-Moya et al., 2011).
We evaluated soil properties in all three mangrove sites from July 2012 to February 2014. At each site, porewater samples were collected at 30 cm depth at four permanent sampling stations established within each plot using a 60 ml syringe attached to a stopcock valve and a rigid tubing probe (3/16″ OD; McKee et al., 1988). Soil porewater sulfide (H2S) concentrations and soil oxidation-reduction potential (i.e., redox, Eh; Pezeshki and Delaune, 2012) were sampled in 2013 (Eh: wet and dry seasons; H2S: wet season) and 2014 (Eh and H2S: dry season). Soil porewater salinity and pH were also measured in the same years/seasons and in 2012 (wet season). Water temperature and salinity were measured with a handheld Schott conductivity meter (model LF-12). A second porewater sample was added to an equal volume of antioxidant buffer (pH 12) in the field to preserve the sample, and sulfide concentrations were determined within 12 h of collection using a silver/sulfide electrode (Model 9616 BN Orion; McKee et al., 1988). Eh was measured in situ at 0, 10, and 45 cm depth using multiple-depth platinum probes (Hargis and Twilley, 1994).
Duplicate soil cores (45 cm depth) were randomly collected once at each plot using a Russian Peat Corer to determine soil bulk density, OM content, and total N and P concentrations in all sites. Soil cores were sectioned into 15 cm intervals, refrigerated at 4°C in the field, and transported to the laboratory for further analyses. Samples were oven-dried at 60°C to a constant mass and weighed to determine bulk density (grams of dry mass per unit volume of wet soil). Samples were ground with a Wiley Mill (250 μm mesh screen). OM content was determined by combustion for 2 h at 550°C (Davies, 1974). Total N was determined by dry combustion on duplicate analytical replicates using an ECS 4010 elemental analyzer (Costech Analytical Technologies, Inc., Valencia, California). Total P concentrations were analyzed by ignition of soil subsamples at 550°C and subsequent digestion with 1 N HCl for 16 h at 150 rpm (Aspilla et al., 1976). Nutrient (N and P) data were expressed on a volume basis (mg cm–3) using bulk density values.
Continuous water levels at 1 h intervals were measured using sonic water level recorders (model 220, Infinities USA Inc., Port Orange, Florida) installed in the interior at each mangrove site and approximately 40–50 m from the forest edge. Instruments were installed on top of a PVC pipe (3″ OD) that was exposed 1.5 m above the soil surface and buried 1 m below the soil surface. Flooding duration, frequency, and water depth data were analyzed for the year of 2013. Water levels are reported relative to the soil surface at each site and are not referenced to the North America Vertical Datum of 1988 (NAVD88).
Differences in root biomass and turnover rates were tested among sites and root-size classes using a two-way analysis of variance (ANOVA). We used repeated measures ANOVA to evaluate differences in root production among sites, root size classes, and harvests (12, 18, and 24 months), with harvest as the repeated measure. Variation in soil properties (salinity, sulfides, Eh) was tested separately for differences among sites and seasons using a two-way split-plot repeated measures ANOVA; sites were considered as the main plot and season as the subplot. Plots (experimental unit) were nested within each site and considered random effects. All main effects (site and season) were considered fixed and interactions were included for all analyses. The Kenward-Roger procedure was used to adjust the degrees of freedom of the F-test statistics when the design was unbalanced or when an unequal variance model was significant (Kenward and Roger, 1997; SAS Institute, Cary, North Carolina, United States). Post hoc pairwise comparisons were performed with Fisher’s Least Significant Differences (LSD) when significant differences (P < 0.05) were observed within a main effect or interaction. Root biomass and production data were transformed [ln (x + 1)] to meet ANOVA assumptions (Zar, 2010). Porewater sulfide data were log-transformed [ln (x + 1)] prior to analysis to achieve normality and homoscedasticity (Zar, 2010). All statistical analyses were performed using PROC MIXED (Sas, 2013). Unless otherwise stated, data presented are means (± 1 SE) of untransformed data. Soil properties data in all mangrove plots were used for regression analyses with root biomass, productivity, and turnover.
Hydroperiod showed contrasting spatial and seasonal patterns among mangrove sites (Figure 3 and Table 1). Water levels followed a distinct gradient where the highest water level (∼33 cm) was observed during the rainy season in the inland mangrove forest (Figure 3C) followed by the fringe (∼25 cm; Figure 3A) and basin forests (< 22 cm; Figure 3B). The highest water level registered in the inland forest site underscored the contribution of precipitation (Figure 2) to the water level/budget in this location. As a result of both the higher relative soil elevation in this site and the presence of a dune and a road between the forest and the coastline, there is a hydrological decoupling of subsurface flow to adjacent coastal waters. This temporal decoupling is registered by the tidal signal in groundwater levels registered from February to the beginning of the rainy season (May) (Figures 2A,B, 3C). Similar to the inland site, the basin forest site also showed a low groundwater level (−60 cm; Figure 3B); although low water levels in this forest were mostly measured in the dry season, i.e., from February to the end of April when mean values were ∼30 cm below the soil surface. These monthly water level patterns are typical in basin forests due to their distance inland from the water edge.
Figure 3. Annual hydrographs (2013) in different mangrove forest ecotypes: (A) Fringe, (B) Basin, (C) Inland in San Andrés Island, Colombia. The zero mark (gray line) represents the soil surface.
Table 1. Statistical results of organic matter, bulk density, soil nutrients, and N:P atomic ratio, measured in mangrove forest in San Andres island, Colombia.
The frequency and duration of inundation were determined by the interaction between precipitation and tidal inundation across seasons (Figures 2B, 3). This interaction was dominated by the effect of the tidal cycle in the fringe forest where the number of tides (231 tides y–1) was higher than in the basin forest (145 tides y–1) (Figures 3A,B). In contrast, the inland mangrove forest showed the lowest frequency of inundation (33 floods y–1) as a result of lower tidal exchange. Yet, the forest was permanently inundated from October to November (Figure 3C; average water levels > 25 cm) due to precipitation indicating the importance of this freshwater input (Figure 2B). Even considering the peak flooding during those 2 months, the inland forest remained flooded only 37% of the year (i.e., 3245 h y–1; Figure 3C). The low topography of the basin forest facilitated a much longer (6207 h y–1) duration of inundation throughout the year (70%) than in the fringe (57%, 4966 h y–1) and inland forest. Moreover, the frequency of inundation in the basin forest was controlled by spring tides when the duration of the flood is comparatively greater (Figure 3B), while in the fringe forest the frequency of inundation was higher and more periodic (Figure 3A).
OM content was significantly [F(2, 11.1) = 37.4, P < 0.0001] higher in the inland mangrove forest (88.5 ± 0.7%) compared to the fringe (64.7 ± 1.6%) and basin (64.1 ± 1.6%) sites. Soil bulk density followed a similar trend with lower values in the inland forest (0.12 ± 0.01 g cm–3) relative to the fringe and basin sites (0.16 ± 0.01 and 0.17 ± 0.01 g cm–3), respectively (Table 1). There were no significant differences in nutrient concentrations: N [F(2, 11.1) = 2.32, P = 0.14] and P [F(2, 11) = 0.06, P = 0.94] among sites. Mean total P concentrations ranged between 0.08 ± 0.02 mg cm–3 (inland mangrove forest) and 0.09 ± 0.01 mg cm–3 (fringe and basin forests). Total N ranged from 1.67 ± 0.2 mg cm–3 (fringe) to 2.27 ± 0.2 mg cm–3 (inland). Soil N:P was lower in the fringe forest (46.6 ± 10.4) compared to the basin (69.5 ± 13.5) and inland (71.4 ± 9.6) sites, although these site differences were not significant [F(2, 11.1) = 1.72, P = 0.22] (Table 1).
Porewater salinity was significantly different among sites (F2, 11.1 = 175.8, P < 0.001) and seasons [F(1, 912) = 11.75, P = 0.0007; Table 2]. The highest salinity (61.6 ± 2.3 PSU) was measured in the basin forest while the fringe site showed intermediate values (37.8 ± 1.8 PSU) and the inland site the lowest values (9.7 ± 1.6 PSU; Table 2). Salinity was relatively lower during the wet season compared to the dry season across all sites (Table 2). Sulfide concentrations (mM) were higher in the fringe forest (2.01 ± 0.4 mM) than in the inland (0.84 ± 0.4 mM) and basin (0.16 ± 0.6 mM) mangrove sites; when comparing seasons, sulfide was significantly higher in the wet season (1.1 ± 0.3 mM; [F(1, 325) = 5.49, P < 0.001] relative to the dry season (0.86 ± 0.3 mM). There was a significant interaction [F(2, 325) = 16.7, P = 0.001] among sites and seasons indicating that the variation in sulfide concentrations between seasons is dependent on-site differences (Table 2). The fringe site showed the highest concentrations during the wet season (2.6 ± 0.4 mM) compared to the other sites (range: 0.1 ± 0.5 mM (i.e., wet, basin) to 1.4 ± 0.5 mM (i.e., dry, fringe). Soil redox potential (Eh) varied significantly among sites [F(2, 10.9) = 35.6, P < 0.001], seasons [F(2, 2637) = 368, P < 0.001], and depths [F(2, 2631) = 277, P < 0.001], with a significant site, season, and depth interaction [F(4, 2631) = 10.52, P < 0.001; Table 2]. Soil Eh decreased significantly with depth at all sites during both seasons, except during the wet season at the basin forest (Table 2). Eh values were higher in the dry season (Table 2) than in the wet season and higher at 0 cm compared between 10 and 45 cm depths across all sites (Table 2).
Table 2. Statistical results of salinity (PSU), sulfide (mM), pH, and Eh (mV) of porewater in mangrove forest of San Andrés Island, Colombia.
Total root biomass varied significantly among sites [F(2, 12.2) = 9.75, P = 0.003; Table 3], with higher biomass allocation in the fringe (1482 ± 120 g m–2) and basin (1293 ± 177 g m–2) forests compared to the inland site (650 ± 88 g m–2; Figure 4A). Significant difference in root biomass were also found among root size classes [F(2, 157) = 75.9, P < 0.001], with higher biomass of coarse roots (716 ± 39 g m–2) relative to fine (230 ± 38 g m–2) and small (227 ± 38 g m–2) root size classes at all sites. There was no significant interaction [F(4, 157) = 1.97, P = ns] between sites and root size classes, indicating that the variation in root biomass among classes is independent of site differences (Table 3). On average, coarse roots represented the largest (60%) fraction of total live root biomass at all sites, whereas fine and small roots contributed with ∼20% to the total value (Figure 4B). Total root biomass showed a positive linear relationship with the frequency and duration of inundation (Figures 5A–C) as was the case between fine root biomass and porewater salinity (Figures 5D,E). In contrast, a negative linear relationship was observed between total root biomass and Eh (Figure 5F). Root biomass did not show any significant relationship with soil TP (r2 = 0.01, P = 0.64) and TN (r2 = 0.24, P = 0.07) or sulfides (r2 = 0.18, P = 0.13).
Table 3. Statistical results of root biomass (g m–2), productivity (g m–2 y–1), turnover rate (y–1).
Figure 4. Root and biomass and productivity among mangrove ecotypes: (A) Mangrove total root biomass (g m– 2); (B) root size class biomass contribution (%); (C) total root productivity (g m– 2 y– 1); (D) root size productivity contribution. San Andrés island. Capital letters denote significant differences (p < 0.05; mean ± 1SE).
Figure 5. Regression models between environmental variables and root biomass: (A,C) flooding duration; (B) frequency of inundation; (D,E) porewater salinity, and (F) redox potential (Eh). Notice that the X-axis values (Eh) range from −50 to 250.
Root productivity rates were not significantly different among harvest periods (12, 18, and 24 months; [F(2, 61.9) = 1.73, P = 0.18; Table 3] due in part to high sample variability across sites. Thus, we used data from harvest one (12 months) to evaluate differences among sites and root size classes. Total root production was significantly higher in the fringe forest (714 ± 172 g m–2 y–1; [F(2, 11.3) = 7.69, P = 0.007] compared to the inland (183 ± 53 g m–2 y–1) and basin (105 ± 41 g m–2 y–1) forests (Figure 4C and Table 3). Mean root production did vary among size classes, with higher production of coarse roots (267 ± 73 g m–2 y–1) relative to fine (100 ± 30 g m–2 y–1) and small root size classes (73 ± 35 g m–2 y–1; [F(2, 46.9) = 3.39, P = 0.04; Table 3]. There was no significant interaction among sites and size classes [F(4, 45.9) = 0.4, P = 0.8]. Overall, coarse roots contributed between 28 and 43% of the total productivity in all sites, while small and fine roots accounted for 25 and 36% of the total productivity across all sites, respectively (Figure 4D). Although no relationships between root productivity and soil nutrients or regulators (i.e., salinity, sulfides, Eh) were detected (data no shown), there was a significant linear relationship between root productivity and frequency of inundation (r2 = 0.39, P = 0.01; Figure 6A).
Figure 6. (A) Regression between frequency of inundation and total root productivity; (B) mean root turnover rate (Mean ± 1SE) per size class in difference mangrove forest ecotype. San Andrés Island, Colombia.
Root turnover was not significantly different among mangrove sites [F(2, 45.9) = 1.29, P = 0.28] and root size classes [F(2, 57.3) = 0.09, P = 0.91; Table 3]. However, mean root turnover decreased as the root size class increased from less than 2 mm (fine) to greater than 5 mm (coarse) for the fringe and inland forests (fine = 0.37 ± 0.11 y–1; small = 0.35 ± 0.08 y–1; coarse 0.33 ± 0.15 y–1). In contrast, in the basin forest the turnover of roots had an opposite trend with size classes (Figure 6B). Overall, mean root turnover ranged from 0.1 ± 0.06 y–1 (basin) to 0.73 ± 0.26 y–1 (fringe) for fine roots; from 0.16 ± 0.11 y–1 (basin) to 0.46 ± 0.08 y–1 (fringe) for small roots; and from 0.33 ± 0.01 y–1 (inland) to 0.60 y–1 for coarse roots (Figure 6B). Root longevity estimates were wide for fine (range: 1.3–9.9 years), small (2.1–5.5 years), and coarse roots (1.6–2.9 years) (Supplementary Table 1). Root turnover did not show any significant linear relationship with soil nutrients, regulators (salinity, sulfides, Eh) or hydroperiod.
Vegetation in terrestrial and wetland ecosystems allocate more resources to belowground root biomass and growth to acquire limiting resources under nutrient limitation (Grime, 1977; Chapin, 1980; Gleeson and Tilman, 1992). This strategy restricts aboveground growth and increases root:shoot ratios leading to a favorable carbon:nutrient balance in nutrient-poor soils (Chapin, 1980). Thus, we hypothesized that high soil stress conditions because of low soil nutrient concentrations (TN, < 3 mg cm–3; TP, < 0.3 mg cm–3) interacting with high porewater salinity (> 50 PSU) and high sulfide concentrations (> 0.5 mM) will promote higher root biomass accumulation, productivity and root turnover in SAI mangroves. This pattern was expected given the SAI’s karstic oligotrophic conditions. However, our results show that the spatiotemporal differences in root dynamics in different mangrove ecotypes were not explained solely by soil nutrient concentrations as generally found in terrestrial forests (Vogt et al., 1987, 1998; Cuevas and Medina, 1988; Nadelhoffer and Raich, 1992) and other mangroves in karstic settings (Castañeda-Moya et al., 2011; Adame et al., 2014; Cormier et al., 2015). In fact, root dynamics in SAI were not associated to potential P availability as reported for other oligotrophic karstic-dominated regions in northern AEP latitudes (e.g., Celestun–Gulf of Mexico, Everglades-Florida: Castañeda-Moya et al., 2011; Adame et al., 2014).
These results indicate that in addition to nutrient gradients, other variables stimulated or limited root biomass accumulation and growth rates in our study sites. Our analysis revealed that root biomass allocation in SAI mangroves was related to the interaction between hydroperiod (frequency and duration) and soil regulators (i.e., salinity, sulfides, Eh). This interaction is underscored by the strong covariation between flooding duration and porewater salinity (r2 = 96%, P < 0.001) in all three sites where the lowest porewater salinity was registered in the inland forest (< 20 PSU) and the highest in the basin forest (50–70 PSU) (Supplementary Figure 1). In the basin forest, the longest flooding duration (6207 h y–1) and lower frequency of flooding (145 tides y–1) cause the accumulation of salts as indicated by the high porewater salinity (61.6 ± 2.3 PSU) due to evaporation of stagnant seawater. In contrast, the higher frequency of flooding (231 tides y–1) and shorter flooding duration (4966 h y–1) registered in the fringe forest promote a higher tidal exchange and lower porewater salinity values (37.8 ± 1.8 PSU). This salinity difference among ecotypes is common as hydro-edaphic conditions drive soil spatiotemporal patterns (Castañeda-Moya et al., 2006; Larcher et al., 2016; Naidoo, 2016; Zhao et al., 2020). Moreover, the inland forest is a type of wetland (i.e., “Peten” forest; López-Portillo et al., 1989; Islebe et al., 2015) located in higher elevation areas relative to sea level and where groundwater inputs control the hydroperiod due to its development on top of limestone platforms across the Caribbean and the Gulf of Mexico (Fleury et al., 2007; Bauer-Gottwein et al., 2011; Twilley et al., 2018). In contrast to other mangrove ecotypes, a Peten can be found up to 10 km inland from the coastline where water infiltrates the karstic/carbonate ground and flows from inland point-sources to the coastline where salinity values are low (< 15 PSU) (e.g., Zaldivar-Jimenez et al., 2010; Stalker et al., 2014). This inland location reduces tidal flooding exchange resulting in very low frequency (33 tides y–1) of inundation and flooding duration (3245 h y–1), especially in the dry season and during sharp seasonal transitions in precipitation, as is the case in the SAI (Figure 3C). This interaction between inundation and soil properties (i.e., high soil OM; low BD values) in the inland and fringe mangrove sites facilitates higher oxygen diffusion rates into the soil that drive the negative linear relationship (87%) between high redox potential (Eh) and frequency of inundation (Supplementary Figure 1).
We also observed a conspicuous hydroperiod gradient among sites that was positively correlated with root dynamics. There was a positive relationship between flooding duration (r2 = 0.51; P = 0.0004) and frequency of inundation (r2 = 0.71, P = 0.001) with total root biomass (Figures 5A,B) and with total root productivity (Figure 6A). As expected, the relationship between total biomass and the range of Eh values in our sites was negative and characterized by high redox potential and high sulfide concentrations, which are considered phytotoxic when concentrations are > 0.5 mM in most wetlands (Lamers et al., 2013; Table 2 and Figure 5F). Similar relationships between fine root biomass and these environmental variables have been reported for other mangroves areas in the Gulf of Mexico (e.g., Everglades, Florida; Castañeda-Moya et al., 2011). The positive relationship between total and fine root biomass and salinity (Figures 5D,E) is also similar in other mangroves throughout the AEP region (e.g., Sherman et al., 2003; Giraldo-Sanchéz, 2005) where this soil stressor limits both stem and root growth (Krauss and Ball, 2013). Low redox values (−50 to −150 Eh mV) in the SAI basin and fringe mangrove forests seem to promote root biomass accumulation most probable as a mechanism to offset limiting oxygen diffusion when higher flooding duration occurs (Figure 5F). However, this pattern is reversed in other sites when the lowest root biomass accumulation occurs under the lowest redox potential (e.g., Sapwalap-Micronesia; Cormier et al., 2015). A combination of field and multispecies controlled-mesocosm studies will further help to quantify the regulatory effect of redox potential on root production.
Although we partitioned the interactions among drivers (hydroperiod vs. salinity) and root biomass and productivity, it is apparent that this interaction is complex. Our results show that under minimum stress conditions caused by regulators (e.g., salinity) and hydroperiod (low frequency and duration of inundation), root biomass and production rates in the inland mangrove forest are very low compared to the fringe and basin forests. This lower allocation of carbon to root biomass suggests that this inland forest invests more energy in other physiological functions such as tree growth (DBH = 30 ± 1.2 cm), as indirectly observed by the canopy tree height (21.6 ± 0.7 m); the highest in the SAI when compared to canopy height in the basin (7.6 ± 0.2 m) and fringe (6.6 ± 0.1 m) forests (Sánchez-Nuñez and Mancera-Pineda, 2011; Medina-Calderón, 2016; Rodriguez-Rodriguez et al., 2018). In contrast, when stress conditions increase due to the interaction of stressors (e.g., high salinity and sulfides) and hydroperiod (e.g., moderate/high flooding duration), root tissue reached the highest biomass and production values, including a relative fast turnover rate of fine roots (0.75 y–1) in the fringe mangrove forest. Yet, the highest stress condition occurs in the basin forest where we measured the highest flooding duration (70%; 6207 h y–1) and highest soil porewater salinity (61.6 ± 0.4 PSU); under these conditions, root productivity was the lowest (Figure 4C) and the turnover rate of fine roots decreased.
Roots dynamics per size classes also showed wide variability across sites. Biomass root allocation in the inland and fringe mangrove forests was inversely correlated with root size classes, while turnover rates increase up to five times (Supplementary Table 1, inland mangrove forest). Similarly, root longevity was greater in coarse roots (i.e., 5–20 mm; 2.84–2.97 years) compared to smaller (2–5 mm; 2.11–2.78 years), and fine roots (1.33–2.66 years) in both the inland and fringe mangrove forests (Supplementary Table 1). These trends are comparable to other mangrove areas in karstic-dominated environments (Supplementary Table 1), as well as in terrestrial forests (Gaudinski et al., 2001; Matamala et al., 2003; Baddeley and Watson, 2005). Fine roots have lower longevity due to their high metabolic activity and usually grow faster than coarse roots according to the cost-benefit hypothesis; it proposes that plants promote fine roots growth due to its significant contribution to water and nutrient acquisition (Ruess et al., 2006; Wang et al., 2019) and relatively low amount of energy required for their maintenance (Eissenstat and Yanai, 1997; Norby and Jackson, 2000). However, if environmental conditions constrain plant growth, fine roots can also increase their longevity (Eissenstat et al., 2000; Guswa, 2008). Depending on specific adaptations, water uptake capacity in mangrove species may display a trade-off between xylem characteristics that ensure safety against cavitation and efficiency in sapflow (Sobrado, 2001; López-Portillo et al., 2005; Medina et al., 2015). When soil salinity increases, the density of the vessels increases while their diameter decreases. Smaller diameter vessels reduce hydraulic conductivity and the likelihood of embolism but can also limit the ability to transport water and nutrients from the root system (Stuart et al., 2007; Reef and Lovelock, 2015; Mendez-Alonzo et al., 2016). Therefore, the hydraulic characteristics that are required for safety during high salinity conditions come at the cost of lower growth rates and modifications in tree allometry (Vovides et al., 2014; Nguyen et al., 2015).
This appears to be the case in the SAI basin forest dominated by A. germinans where hydroperiod and soil regulators impose a relatively higher stress condition; as a result, mangroves produce long-lived roots instead of building new tissue. Since the cost-benefit ratio of keeping living tissue longer is more advantageous than allocating energy for new tissue, the longevity of coarse roots increases, and coarse root biomass remains high in SAI mangroves (Supplementary Table 1). These tradeoffs in root biomass allocation by trees reflect a local ecophysiological adaptation and phenotypical plasticity to soil stress conditions at the expense of aboveground development (Reef and Lovelock, 2015; Lovelock et al., 2016; Vovides et al., 2018). Given the importance of water availability in the SAI controlling mangrove trees photosynthetic water use efficiency (Rodriguez-Rodriguez et al., 2018), it is possible to find local-scale differences in resource allocation, even by the same species, within environmentally distinct areas; especially, in the case of A. germinans and L. racemosa—two mangrove species with a wide ecophysiological plasticity (Yanez-Espinosa et al., 2004; Mendez-Alonzo et al., 2008; Vovides et al., 2014; Deslauriers et al., 2017; Osland et al., 2018; Wang et al., 2018; Pascoalini et al., 2019).
Despite the critical contribution of root biomass and productivity to soil formation in mangroves, there is a limited number of studies across different geomorphic settings and ecotypes (Supplementary Table 2). Among these studies, few simultaneously assessed fine root biomass and productivity (Supplementary Table 2). Mean fine root biomass (< 2 mm) values and range (650–1482 g m–2) observed in SAI mangrove forests are within the range reported for similar mangrove ecotypes. This root size class is not only a major contributor to carbon cycling, but also a good indicator of stress as plants under soil nutrient-poor conditions increase root production (Eissenstat et al., 2000; Finer et al., 2011; Wang et al., 2019). Root biomass values estimated in the inland mangrove site were relatively low (650 ± 88 g m–2), yet biomass estimates were comparable to other mangrove sites across different latitudinal ranges including: Cuba (1080–1710 g m–2; Fiala and Hernandez, 1993), Mexico (947–3040 g m–2; Adame et al., 2014), United States (3198–4,389 g m–2; Castañeda-Moya et al., 2011), Micronesia (449–2640 g m–2; Cormier et al., 2015), and Australia (1150–5,580 g m–2; Alongi, 2009). Although fine root values in SAI are in the lower range when compared to other ecogeomorphic settings (e.g., Golley et al., 1962; Golley, 1975; Briggs, 1977; Komiyama et al., 1988; Mackey, 1993; Alongi et al., 2000; Tamooh et al., 2008; Abohassan et al., 2012; Muhammad-Nor et al., 2019; Supplementary Table 2), root biomass and production in SAI’s karstic environment still play a key role in soil formation by maintaining soil vertical accretion offsetting an increasing SLR rate in the Caribbean region (Breithaupt et al., 2017; Lamont et al., 2020); this rate is currently estimated at 2.8 mm y–1 and projected to increase to > 4.0 mm y–1 by year 2050 (Ruiz-Ramirez et al., 2019). Further, the range of mean fine (< 2 mm) root belowground productivity rates in our study sites (48–219 g m–2 y–1) (Supplementary Table 2) were comparatively similar to other karstic-dominated sites in the AEP region including Belize (43–197 g m–2 y–1, McKee et al., 2007), Honduras (171–199 g m–2 y–1, Cahoon et al., 2003), and Florida (140–280 g m–2 y–1; McKee and Faulkner, 2000; 307–378 g m–2 y–1, Giraldo-Sanchéz, 2005; 130–206 g m–2 y–1, Castañeda-Moya et al., 2011). Unfortunately, not all these root production studies related fine root productivity to the set of specific hydroperiod components (duration, frequency, depth), thus limiting a comparative analysis.
When comparing total root productivity values among geomorphic settings, we found that estuarine environments in oceanic volcanic areas in Micronesia (i.e., 46–119 g m–2 y–1; Cormier et al., 2015) were overall lower than our rates (105–714 g m–2 y–1) in the SAI (Supplementary Table 2). In contrast to the SAI location, where soil P was not different among sites, high soil N:P ratios in Micronesia mangroves indicate low P availability. This local environmental variability driving root production and accumulation in mangrove soils needs to be considered when attempting to identify sources contributing to soil total organic carbon budgets at regional and global spatial scales. Globally, more that 80% of the organic carbon storage by mangrove forest is in the soil as determined by the interpolation and upscaling of values obtained in situ using soil cores and statistical modeling (Donato et al., 2011; Jardine and Siikamaki, 2014; Atwood et al., 2018). Yet, it is still difficult to establish a functional connectivity between below- and aboveground carbon production to explain ecological differences in carbon storage across temperature and precipitation latitudinal ranges (Sanders et al., 2016; Simard et al., 2019). One reason is the need to consider the temporal differences of the annual/decadal ecological (e.g., root production per size class and accumulation) vs. geological (e.g., soil accumulation/formation) processes involved in the long-term organic carbon accumulation in mangrove soils (Kristensen et al., 2017; Rivera-Monroy et al., 2017b; Rovai et al., 2018). Another reason for this uncertainty is the use of aboveground forest allometric equations to estimate root organic carbon contribution when root data are not available. In this case, structural variables (e.g., aboveground biomass, tree density and diameter at breast height-DBH) are used as proxy of root biomass thus creating large uncertainties (range: 40–100%; Adame et al., 2017) when estimating these values due to major differences in environmental variables generally found across mangrove ecotypes (Rivera-Monroy et al., 2017b). To avoid large error propagation when estimating regional and global soil organic carbon budgets, it is recommended to use actual in situ values that include at least two root size classes (i.e., < 2 m and > 2 mm) and dead and live roots (Adame et al., 2017). These spatiotemporal discrepancies between statistical estimates and direct root biomass measurements in mangrove soils are beginning to be recognized when attempting to build robust mangrove carbon budgets to inform mangrove conservation and management programs (Atwood et al., 2018; Sanderman et al., 2018; Simard et al., 2019).
Because SAI is an oceanic island lacking rivers and located ∼230 km from the continent, there is a limited source of allochthonous inorganic sediment inputs from the interior of the island to the coastal zone. Hence, below- (e.g., roots) and aboveground biomass storage and productivity in mangrove forests represent the major source of organic material for soil formation and maintaining accretion and soil elevation in this karstic environment. Aboveground productivity, for example, in the inland mangrove forest (i.e., “Peten”) is one the highest recorded in the AEP region (mean litterfall: 21.4 ± 0.09 Mg ha–1 year–1, Medina-Calderón, 2016) and other type of forests (Zhang et al., 2014). Low decomposition rates in this forest control the accumulation of soil OM (e.g., senescent leaves, mean −kt = 19.55 ± 1.04 week–1; Sierra-Rozo et al., 2009); this seasonal OM input and the soil surface/subsurface remains dry from February-August (Figure 3C) until the beginning of the rainy season when humidity promotes mineralization. This OM accumulation mechanism contrasts with other forests where the frequency of inundation is higher and litterfall can be exported by most frequent tidal exchange (Figures 3A,B). Although accretion rates have not been directly measured in SAI, the presence of mangroves in the island dates to the mid-Holocene (∼5000 years) period (Ellison, 1996; González et al., 2010), indicating that these wetlands have been able to keep up with SLR (Saintilan et al., 2020). The distinct mangrove peat-dominated shallow soil layer in SAI functions as a substrate for maintaining limited, but constant, mangrove growth rates and relative elevation as groundwater regulates salinity and nutrient availability. Mangrove forests growing in areas with limited nutrient inputs, but on top of large aquifers and subsurface water flow, such as oceanic islands (McKee et al., 2007; McKee, 2011), coastal karstic environments (Adame et al., 2014; Rivera-Monroy et al., 2019; Castañeda-Moya et al., 2020), and semi-arid coastal regions (Ezcurra et al., 2016; Ochoa-Gomez et al., 2019) have the capacity of storing significant amounts of organic carbon in shallow soil layers (e.g., 100–239 Mg C ha–1 at 45 cm; Ochoa-Gomez et al., 2019) that contribute to soil elevation gain relative to increasing SLR rates.
The common feature among our study sites is the patchy spatial distribution of mangroves along low topographic gradients associated with shallow groundwater levels and closness to water bodies. Due to the functional role of groundwater hydrology in controlling soil porewater salinity and mangrove productivity across the SAI, it is paramount that the recharge rate (0.04 m3 s–1 m–2) of the San Luis Aquifer—a critical water reservoir in the SAI—would not be impacted by human activities in the long term (Bedoya et al., 2010; Fabian et al., 2018). Presently, it is not clear how the current urban expansion (Medina-Calderón and Toro, 2018a,b) within the island will alter groundwater availability and storage across the elevation gradient where mangrove habitat is extensive and coastal deforestation and drinking water demands can compound these threats. This vulnerability is in addition to potential changes in local and regional precipitation patterns due to climate change in the extensive Caribbean region (McLean et al., 2015; Taylor et al., 2018). It is expected that a precipitation deficit could alter the SAI hydrology and directly impact the sustainability of mangroves and their capacity to provide key ecosystem services in the long term.
This study revealed that the interaction between hydroperiod and salinity have a major control on root productivity in different mangrove ecotypes in the oceanic island of San Andres, Colombia. Our findings contrast to previous studies in karstic-dominated environments where mangrove forests are usually limited by inorganic P and N availability. Under conditions of minimum stress (i.e., low salinity and sulfide, high redox potential, and short flooding duration), root biomass allocation, production, turnover rate, and longevity of fine roots were low, indicating that both hydroperiod and soil regulators impose higher stress on plant physiological traits, particularly in areas where duration of inundation is high. Since the cost-benefit ratio of keeping living tissue longer is more advantageous than allocating energy to build new tissue, the longevity of coarse roots (> 5 mm) increased as indicated by high biomass in this root size class; this greatly contributes to soil formation. Inorganic allochthonous sediment inputs that potentially can contribute to soil formation and accretion are absent since the SAI is an oceanic island (∼230 km from the continent) where rivers are absent, thus limiting any transport of sediment and organic material from the interior of the island into the coastal zone. Due to the relative importance of groundwater hydrology in controlling soil porewater salinity, and consequently mangroves root productivity across the SAI, it is paramount that the San Luis Aquifer is not negatively impacted by human activities (e.g., water extraction, eutrophication) in the long term. Increasing deforestation and urban development, both inland and in the coastal zone, will cause significant hydrological change thus modulating the sustainability of mangroves, including their role in keeping the island coastline above increasing SLR.
The raw data supporting the conclusions of this article will be made available by the authors, without undue reservation, to any qualified researcher.
JM-C, JM-P, and VR-M conceived and designed the study. JM-P and VR-M wrote the first manuscript draft, evaluated results, and contributed to final analysis. JM-C and EC-M performed the statistical analyses. All authors helped to wrote the final version of the manuscript.
Partial funding to EC-M and VR-M was provided by the Florida Coastal Everglades Long-Term Ecological Research (FCE-LTER) program funded by the National Science Foundation (Grants #DEB-1832229 and #DEB-1237517). The Department of the Interior South-Central Climate Adaptation Science Center (Cooperative Agreement #G12AC00002) also provided funding for VR-M’s participation.
The authors declare that the research was conducted in the absence of any commercial or financial relationships that could be construed as a potential conflict of interest.
We want to thank Professor José Teodecto Castaño and his students at the SAI school “Luis Amigó” for providing invaluable assistance in the laboratory and the field including Jabber Hooker, Marbel Steel, Trisha Forbes, Laura Aguas, and Anthony Rojas. We would like to thank Robert R. Twilley and Tommy Blanchard (LSU) for facilitating equipment and the use of laboratory facilities during sample analyses. This paper is based on a Ph.D. dissertation submitted to Sede Caribe de la Universidad Nacional de Colombia by JM-C. Evaluación de Servicios Ecosistémicos asociados al ecosistema de manglar en bosques con régimen hídrico constrastantes en una isla oceóanica de la Reserva de Biósfera Seaflower, Código Hermes 39416. We want to thank two reviewers for constructive comments on an earlier version of this manuscript. This is CECIMAR contribution 510 (Programa de Posgrado en Biología– línea Biología Marina) and contribution number 998 from the Southeast Environmental Research Center in the Institute of Environment at Florida International University.
The Supplementary Material for this article can be found online at: https://www.frontiersin.org/articles/10.3389/fmars.2020.598132/full#supplementary-material
Abohassan, R. A. A., Okia, C. A., Agea, J. G., Kimondo, J. M., and McDonald, M. M. (2012). Perennial biomass production in arid mangrove systems on the Red sea coast of Saudi Arabia. Environ. Res. J. 6, 22–31.
Adame, M. F., Cherian, S., Reef, R., and Stewart-Koster, B. (2017). Mangrove root biomass and the uncertainty of belowground carbon estimations. For. Ecol. Manag. 403, 52–60. doi: 10.1016/j.foreco.2017.08.016
Adame, M. F., Teutli, C., Santini, N. S., Caamal, J. P., Zaldivar-Jimenez, A., Hernandez, R., et al. (2014). Root biomass and production of mangroves surrounding a Karstic Oligotrophic coastal lagoon. Wetlands 34, 479–488. doi: 10.1007/s13157-014-0514-5
Alongi, D. M. (2002). Present state and future of the world’s mangrove forests. Environ. Conserv. 29, 331–349. doi: 10.1017/s0376892902000231
Alongi, D. M., Tirendi, E., and Clough, B. F. (2000). Below-ground decomposition of organic matter in forests of the mangroves Rhizophora stylosa and Avicennia marina along the arid coast of Western Australia. Aquat. Bot. 68, 97–122. doi: 10.1016/s0304-3770(00)00110-8
Aspilla, K. I., Agemian, H., and Chau, A. S. Y. (1976). A semi-automated method for determination of inorganic, organic, and total phosphate in sediments. Analyst 101, 187–197. doi: 10.1039/an9760100187
Atwood, T. B., Connolly, R. M., Almahasheer, H., Carnell, P. E., Duarte, C. M., Lewis, C. J. E., et al. (2018). Global patterns in mangrove soil carbon stocks and losses. Nat. Clim. Change 8, 257–257. doi: 10.1038/s41558-017-0019-3
Baddeley, J. A., and Watson, C. A. (2005). Influences of root diameter, tree age, soil depth and season on fine root survivorship in Prunus avium. Plant Soil 276, 15–22. doi: 10.1007/s11104-005-0263-6
Ball, M. C. (1998). Mangrove species richness in relation to salinity and waterlogging: a case study along the Adelaide River floodplain, northern Australia. Glob. Ecol. Biogeogr. Lett. 7, 73–82. doi: 10.2307/2997699
Ball, M. C., and Pidsley, S. M. (1995). Growth-responses to salinity in relation to distribution of 2 mangrove species, Sonneratia-alba and S-lanceolata, in Northern Australia. Funct. Ecol. 9, 77–85. doi: 10.2307/2390093
Bauer-Gottwein, P., Gondwe, B. R. N., Charvet, G., Marin, L. E., Rebolledo-Vieyra, M., and Merediz-Alonso, G. (2011). Review: the Yucatan Peninsula karst aquifer, Mexico. Hydrogeol. J. 19, 507–524. doi: 10.1007/s10040-010-0699-5
Bedoya, J. M., Carmona, J. A., Vélez, M. V., and Bedoya-Soto, J. M. (2010). “Modelo numérico del acuífero de la Isla de San Andrés, Colombia,” in Proceedings of the XXIV Congreso Latinoamericano de Hidráulica, Punta del Este.
Berger, U., Rivera-Monroy, V. H., Doyle, T. W., Dahdouh-Guebas, F., Duke, N. C., Fontalvo-Herazo, M. L., et al. (2008). Advances and limitations of individual-based models to analyze and predict dynamics of mangrove forests: a review. Aquat. Bot. 89, 260–274. doi: 10.1016/j.aquabot.2007.12.015
Bouillon, S., Borges, A. V., Castaneda-Moya, E., Diele, K., Dittmar, T., Duke, N. C., et al. (2008). Mangrove production and carbon sinks: a revision of global budget estimates. Glob. Biogeochem. Cycl. 22, 1–12. doi: 10.1029/2007gb003052
Breithaupt, J. L., Smoak, J. M., Rivera-Monroy, V. H., Castaneda-Moya, E., Moyer, R. P., Simard, M., et al. (2017). Partitioning the relative contributions of organic matter and mineral sediment to accretion rates in carbonate platform mangrove soils. Mar. Geol. 390, 170–180. doi: 10.1016/j.margeo.2017.07.002
Briggs, S. V. (1977). Estimates of biomass in a temperate mangrove community. Austr. J. Ecol. 2, 369–373. doi: 10.1111/j.1442-9993.1977.tb01151.x
Burchett, M. D., Field, C. D., and Pulkownik, A. (1984). Salinity, growth and root respiration in the grey mangrove, Avicennia-marina. Physiol. Plant. 60, 113–118. doi: 10.1111/j.1399-3054.1984.tb04549.x
Cahoon, D. R., Hensel, P., Rybczyk, J., McKee, K. L., Proffitt, C. E., and Perez, B. C. (2003). Mass tree mortality leads to mangrove peat collapse at Bay Islands, Honduras after Hurricane Mitch. J. Ecol. 91, 1093–1105. doi: 10.1046/j.1365-2745.2003.00841.x
Cameron, C., Hutley, L. B., Friess, D. A., and Munksgaard, N. C. (2019). Hydroperiod, soil moisture and bioturbation are critical drivers of greenhouse gas fluxes and vary as a function of landuse change in mangroves of Sulawesi, Indonesia. Sci. Total Environ. 654, 365–377. doi: 10.1016/j.scitotenv.2018.11.092
Cardona-Olarte, P., Twilley, R. R., Krauss, K. W., and Rivera-Monroy, V. (2006). Responses of neotropical mangrove seedlings grown in monoculture and mixed culture under treatments of hydroperiod and salinity. Hydrobiologia 569, 325–341. doi: 10.1007/s10750-006-0140-1
Castañeda-Moya, E., Rivera-Monroy, V. H., Chambers, R. M., Zhao, X. C., Lamb-Wotton, L., Gorsky, A., et al. (2020). Hurricanes fertilize mangrove forests in the Gulf of Mexico (Florida Everglades, USA). Proc. Natl. Acad. Sci. U.S.A. 117, 4831–4841. doi: 10.1073/pnas.1908597117
Castañeda-Moya, E., Rivera-Monroy, V. H., and Twilley, R. R. (2006). Mangrove zonation in the dry life zone of the Gulf of Fonseca, Honduras. Estuar. Coasts 29, 751–764. doi: 10.1007/bf02786526
Castañeda-Moya, E., Twilley, R. R., and Rivera-Monroy, V. H. (2013). Allocation of biomass and net primary productivity of mangrove forests along environmental gradients in the Florida Coastal Everglades, USA. For. Ecol. Manag. 307, 226–241. doi: 10.1016/j.foreco.2013.07.011
Castañeda-Moya, E., Twilley, R. R., Rivera-Monroy, V. H., Marx, B. D., Coronado-Molina, C., and Ewe, S. M. L. (2011). Patterns of Root Dynamics in Mangrove Forests Along Environmental Gradients in the Florida Coastal Everglades, USA. Ecosystems 14, 1178–1195. doi: 10.1007/s10021-011-9473-3
Chapin, F. S. (1980). The mineral nutrition of wild plants. Annu. Rev. Ecol. Syst. 11, 233–260. doi: 10.1146/annurev.es.11.110180.001313
Chapin, F. S., Bloom, A. J., Field, C. B., and Waring, R. H. (1987). Plant-responses to multiple environmental-factors. Bioscience 37, 49–57. doi: 10.2307/1310177
Chmura, G. L., Anisfeld, S. C., Cahoon, D. R., and Lynch, J. C. (2003). Global carbon sequestration in tidal, saline wetland soils. Glob. Biogeochem. Cycl. 17, 1–12. doi: 10.1029/2002gb001917
Cintron, G., Lugo, A. E., Pool, D. J., and Morris, G. (1978). mangrove in arid envvironments in Puerto Rico and adjacent Islands. Biotropica 10, 110–121. doi: 10.2307/2388013
Clarke, P. J. (2014). Seeking global generality: a critique for mangrove modellers. Mar. Freshwater Res. 65, 930–933. doi: 10.1071/mf13326
Cormier, N., Twilley, R. R., Ewel, K. C., and Krauss, K. W. (2015). Fine root productivity varies along nitrogen and phosphorus gradients in high-rainfall mangrove forests of Micronesia. Hydrobiologia 750, 69–87. doi: 10.1007/s10750-015-2178-4
Crase, B., Liedloff, A., Vesk, P. A., Burgman, M. A., and Wintle, B. A. (2013). Hydroperiod is the main driver of the spatial pattern of dominance in mangrove communities. Glob. Ecol. Biogeogr. 22, 806–817. doi: 10.1111/geb.12063
Cuevas, E., and Medina, E. (1988). Nutrient dynamics within amazonian forests. Oecologia 76, 222–235. doi: 10.1007/BF00379956
Davies, B. E. (1974). Loss on ignition as an estimate of soil organic matter. Soil Sci. Soc. Am. Proc. 38, 150–151. doi: 10.2136/sssaj1974.03615995003800010046x
Deslauriers, A., Fonti, P., Rossi, S., Rathgeber, C. B. K., and Grièar, J. (2017). “Ecophysiology and Plasticity of Wood and Phloem Formation,” in Dendroecology: Tree-Ring Analyses Applied to Ecological Studies, eds M. M. Amoroso, L. D. Daniels, P. J. Baker, and J. J. Camarero (Cham: Springer International Publishing), 13–33. doi: 10.1007/978-3-319-61669-8_2
Donato, D. C., Kauffman, J. B., Murdiyarso, D., Kurnianto, S., Stidham, M., and Kanninen, M. (2011). Mangroves among the most carbon-rich forests in the tropics. Nat. Geosci. 4, 293–297. doi: 10.1038/ngeo1123
Downton, W. J. S. (1982). Growth and osmotic relations of the mangrove Avicennia-marina, as influenced by salinity. Australian J. Plant Physiol. 9, 519–528. doi: 10.1071/pp9820519
Duke, N. C. (2017). “Mangrove Floristics and Biogeography Revisited: Further Deductions from Biodiversity Hot Spots, Ancestral Discontinuities, and Common Evolutionary Processes,” in Mangrove Ecosystems: A Global Biogeographic Perspective: Structure, Function, and Services, eds V. H. Rivera-Monroy, S. Y. Lee, E. Kristensen, and R. R. Twilley (Cham: Springer International Publishing), 17–53. doi: 10.1007/978-3-319-62206-4_2
Eissenstat, D. M., Wells, C. E., Yanai, R. D., and Whitbeck, J. L. (2000). Building roots in a changing environment: implications for root longevity. New Phytol. 147, 33–42. doi: 10.1046/j.1469-8137.2000.00686.x
Eissenstat, D. M., and Yanai, R. D. (1997). “The ecology of root lifespan,” in Advances in Ecological Research, Vol. 27, eds M. Begon and A. H. Fitter (Cambridge, MA: Academic Press), 1–60. doi: 10.1016/s0065-2504(08)60005-7
Ellison, J. C. (1996). Pollen evidence of late holocene mangrove development in bermuda. Glob. Ecol. Biogeogr. Lett. 5, 315–326. doi: 10.2307/2997587
Ezcurra, P., Ezcurra, E., Garcillan, P. P., Costa, M. T., and Aburto-Oropeza, O. (2016). Coastal landforms and accumulation of mangrove peat increase carbon sequestration and storage. Proc. Natl. Acad. Sci. U.S.A. 113, 4404–4409. doi: 10.1073/pnas.1519774113
Fabian, C. L., Ibañez, J. W., Prieto, F. S., and Caro Camargo, C. (2018). Groundwater Sustainability Assessment in Small Islands: the case study of san andres in the caribbean sea. Preprints 2018:2018070449. doi: 10.20944/preprints201807.0449.v1
Fiala, K., and Hernandez, L. (1993). Root biomass of a mangrove forest in Southwestern Cuba (Majana). Ekol. Bratislava 12, 15–30.
Finer, L., Ohashi, M., Noguchi, K., and Hirano, Y. (2011). Fine root production and turnover in forest ecosystems in relation to stand and environmental characteristics. For. Ecol. Manag. 262, 2008–2023. doi: 10.1016/j.foreco.2011.08.042
Fleury, P., Bakalowicz, M., and de Marsily, G. (2007). Submarine springs and coastal karst aquifers: a review. J. Hydrol. 339, 79–92. doi: 10.1016/j.jhydrol.2007.03.009
Flower-Ellis, J. G. K., and Persson, H. (1980). Investigation of structural preoperties and dynamics of Scots pine stands. Ecol. Bull. 32, 125–138.
Friess, D. A., and Webb, E. L. (2014). Variability in mangrove change estimates and implications for the assessment of ecosystem service provision. Glob. Ecol. Biogeogr. 23, 715–725. doi: 10.1111/geb.12140
Gaudinski, J. B., Trumbore, S. E., Davidson, E. A., Cook, A. C., Markewitz, D., and Richter, D. D. (2001). The age of fine-root carbon in three forests of the eastern United States measured by radiocarbon. Oecologia 129, 420–429. doi: 10.1007/s004420100746
Gavio, B., Palmer-Cantillo, S., and Mancera, J. E. (2010). Historical analysis (2000-2005) of the coastal water quality in San Andrés Island, SeaFlower Biosphere Reserve, Caribbean Colombia. Mar. Pollut. Bull. 60, 1018–1030. doi: 10.1016/j.marpolbul.2010.01.025
Giraldo-Sanchéz, B. (2005). Belowground Productivity of Mangrove Forests in Southwest Florida. PhD Dissertation, Louisiana State University, Baton Rouge.
Gleason, S. M., and Ewel, K. C. (2002). Organic matter dynamics on the forest floor of a Micronesian mangrove forest: an investigation of species composition shifts. Biotropica 34, 190–198. doi: 10.1111/j.1744-7429.2002.tb00530.x
Gleeson, S. K., and Tilman, D. (1992). Plant allocation and the multiple limitation hypothesis. Am. Natural. 139, 1322–1343. doi: 10.1086/285389
Golley, F., Odum, H. T., and Wilson, R. F. (1962). The structure and metabolism of a puerto rican red mangrove forest in May. Ecology 43, 9–19. doi: 10.2307/1932034
Golley, F. B. (1975). Mineral Cycling in a Tropical Moist Forest Ecosystem. Athens: University of Georgia Press.
González, C., Urrego, L. E., Martínez, J. I., Polanía, J., and Yokoyama, Y. (2010). Mangrove dynamics in the southwestern Caribbean since the ‘Little Ice Age’: a history of human and natural disturbances. Holocene 20, 849–861. doi: 10.1177/0959683610365941
Grime, J. P. (1977). Evidence for the existence of three primary strategies in plants and its relevance to ecological and evolutionary theory. Am. Natural. 111, 1169–1194. doi: 10.1086/283244
Guswa, A. J. (2008). The influence of climate on root depth: a carbon cost-benefit analysis. Water Resources Res. 44, 1–11. doi: 10.1029/2007wr006384
Guzmán, O. G., and Hernandez, P. O. (2012). “Proyecto hidrogeológico del acuífero de San Andrés y recuperación del campo de pozos: Cartografía geológica de la isla de San Andrés,” in Proceedings of the Informe interno, (Bogota: IInstituto Nacional de Investigaciones Geológico Mineras).
Hargis, T. G., and Twilley, R. R. (1994). A multi-depth probe for measuring Oxidation-Reduction (REDOX) potential in wetlands soils. J. Sediment. Res. Sec. Sediment. Petrol. Process. 64, 684–685. doi: 10.1306/d4267e6a-2b26-11d7-8648000102c1865d
He, D., Rivera-Monroy, V. H., Jaffé, R., and Zhao, X. (in press). Mangrove leaf species-specific isotopic signatures along a salinity and phosphorus soil fertility gradients in a subtropical estuary. Estuar. Coast. Shelf Sci.
Howard, A. A., Bolaños, N., Machacon, I., Lasso, J., Gómez, D. I., and Ward, V. (2012). “Actualización del conocimiento de los ecosistemas marinos en la Reserva de Biósfera Seaflower, con énfasis en las islas de San Andrés y Providencia,” in Atlas de la Reserva de Biósfera Seaflower. Archipiélago de San Andrés, Providencia y Santa Catalina, eds D. I. Gómez-López, C. Segura-Quintero, P. C. Sierra-Correa, and J. Garay-Tinoco (Santa Marta: Instituto de Investigaciones Marinas y Costeras “José Benito Vives De Andréis” -INVEMAR- y Corporación para el Desarrollo Sostenible del Archipiélago de San Andrés, Providencia y Santa Catalina - CORALINA).
IDEAM (2017). Servicio Mareográfico Pronóstico de Pleamares y bajamares en la costa Caribe Colombiana. Colombia: Instituto de Hidrología, Meteorología y Estudios Ambientales.
Islebe, G. A., Sanchez-Sanchez, O. O., Valdez-Hernandez, M., and Weissenberger, H. (2015). “Distribution of Vegetation Types,” in Chapter 3. Biodiversity and Conservation of the Yucatán Peninsula, eds G. A. Islebe, S. Calmé, J. L. Leon-Cortes, and B. C. Schmook (Switzerland: Springer), 39–53. doi: 10.1007/978-3-319-06529-8_3
Jardine, S. L., and Siikamaki, J. V. (2014). A global predictive model of carbon in mangrove soils. Environ. Res. Lett. 9:104013. doi: 10.1088/1748-9326/9/10/104013
Jayatissa, L. P., Wickramasinghe, W. A. A. D. L., Dahdouh-Guebas, F., and Huxham, M. (2008). Interspecific variations in responses of mangrove seedlings to two contrasting salinities. Int. Rev. Hydrobiol. 93, 700–710. doi: 10.1002/Iroh.200711017
Jerath, M., Bhat, M., Rivera-Monroy, V. H., Castaneda-Moya, E., Simard, M., and Twilley, R. R. (2016). The role of economic, policy, and ecological factors in estimating the value of carbon stocks in Everglades mangrove forests. South Florida, USA. Environ. Sci. Pol. 66, 160–169. doi: 10.1016/j.envsci.2016.09.005
Kairo, J. G., Lang’at, J. K. S., Dahdouh-Guebas, F., Bosire, J., and Karachi, M. (2008). Structural development and productivity of replanted mangrove plantations in Kenya. For. Ecol. Manag. 255, 2670–2677. doi: 10.1016/J.Foreco.2008.01.031
Kenward, M. G., and Roger, J. H. (1997). Small sample inference for fixed effects from restricted maximum likelihood. Biometrics 53, 983–997. doi: 10.2307/2533558
Koch, M. S., Mendelssohn, I. A., and McKee, K. L. (1990). Mechanism for the hydrogen sulfide-induced growth limitation in wetland macrophytes. Limnol. Oceanogr. 35, 399–408. doi: 10.4319/lo.1990.35.2.0399
Komiyama, A., Havanond, S., Srisawatt, W., Mochida, Y., Fujimoto, K., Ohnishi, T., et al. (2000). Top/root biomass ratio of a secondary mangrove (Ceriops tagal (Perr.) CB Rob.) forest. For. Ecol. Manag. 139, 127–134. doi: 10.1016/s0378-1127(99)00339-4
Komiyama, A., Moriya, H., Suhardjono, P., Toma, T., and Ogino, K. (1988). “Forest as an ecosystem, its structure and function,” in Biological System of Mangroves, eds K. Ogino and M. Chihara (Ehime: Ehime University), 85–151.
Krauss, K. W., and Ball, M. C. (2013). On the halophytic nature of mangroves. Trees Struct. Funct. 27, 7–11. doi: 10.1007/s00468-012-0767-7
Krauss, K. W., Doyle, T. W., Twilley, R. R., Rivera-Monroy, V. H., and Sullivan, J. K. (2006). Evaluating the relative contributions of hydroperiod and soil fertility on growth of south Florida mangroves. Hydrobiologia 569, 311–324. doi: 10.1007/s10750-006-0139-7
Krauss, K. W., Lovelock, C. E., McKee, K. L., Lopez-Hoffman, L., Ewe, S. M. L., and Sousa, W. P. (2008). Environmental drivers in mangrove establishment and early development: a review. Aquat. Bot. 89, 105–127. doi: 10.1016/j.aquabot.2007.12.014
Kristensen, E., Connolly, R. M., Otero, X. L., Marchand, M., Ferreira, T. O., and Rivera-Monroy, V. H. (2017). “Chapter 11. Biogeochemical cycles: Global approaches and perspectives,” in Mangrove Ecosystems: A Global Biogeographic Perspective Structure, Function and Ecosystem Services, eds V. H. Rivera-Monroy, S. Lee, Y. E. Kristensen, and R. R. Twilley (New York, NY: Springer), 163–219.
Lamers, L. P. M., Govers, L. L., Janssen, I. C. J. M., Geurts, J. J. M., Van der Welle, M. E. W., Van Katwijk, M. M., et al. (2013). Sulfide as a soil phytotoxin-a review. Front. Plant Sci. 4:268. doi: 10.3389/fpls.2013.00268
Lamont, K., Saintilan, N., Kelleway, J. J., Mazumder, D., and Zawadzki, A. (2020). Thirty-year repeat measures of mangrove above- and below-ground biomass reveals unexpectedly high carbon sequestration. Ecosystems 23, 370–382. doi: 10.1007/s10021-019-00408-3
Larcher, L., Boeger, M. R. T., and Sternberg, L. D. S. L. O. R. (2016). Gas exchange and isotopic signature of mangrove species in Southern Brazil. Aquat. Bot. 133, 62–69. doi: 10.1016/j.aquabot.2016.06.001
López-Portillo, J., Ewers, F. W., and Angeles, G. (2005). Sap salinity effects on xylem conductivity in two mangrove species. Plant Cell Environ. 28, 1285–1292. doi: 10.1111/j.1365-3040.2005.01366.x
López-Portillo, J., Ezcurra, E., and Mass, J. M. (1989). Los petenes de Sian Ka’an. Quintana Roo y su relación con gradientes de presión hídrica. Acta Bot. Mexic. 5, 19–29. doi: 10.21829/abm5.1989.575
Lovelock, C. E., Atwood, T., Baldock, J., Duarte, C. M., Hickey, S., Lavery, P. S., et al. (2017). Assessing the risk of carbon dioxide emissions from blue carbon ecosystems. Front. Ecol. Environ. 15:257–265. doi: 10.1002/fee.1491
Lovelock, C. E., Krauss, K. W., Osland, M. J., Reef, R., and Ball, M. C. (2016). “The physiology of mangrove trees with changing climate,” in Tropical Tree Physiology: Adaptations and Responses in a Changing Environment, eds G. Goldstein and L. S. Santiago (New York, NY: Springer), 149–179. doi: 10.1007/978-3-319-27422-5_7
Mackey, A. P. (1993). Biomass of the mangrove Avicennia-marina (forsk) vierh near Brisbane, South-Eastern Queensland. Austr. J. Mar. Freshwater Res. 44, 721–725. doi: 10.1071/mf9930721
Matamala, R., Gonzalez-Meler, M. A., Jastrow, J. D., Norby, R. J., and Schlesinger, W. H. (2003). Impacts of fine root turnover on forest NPP and soil C sequestration potential. Science 302, 1385–1387. doi: 10.1126/science.1089543
McKee, K. L. (1993). Soil physicochemical patterns and mangrove species distribution-reciprocal effects? J. Ecol. 81, 477–487. doi: 10.2307/2261526
McKee, K. L. (1995). Seedling recruitment patterns in a Belizean mangrove forest: effects of establishment ability and physico-chemical factors. Oecologia 101, 448–460. doi: 10.1007/bf00329423
McKee, K. L. (2011). Biophysical controls on accretion and elevation change in Caribbean mangrove ecosystems. Estuar. Coast. Shelf Sci. 91, 475–483. doi: 10.1016/j.ecss.2010.05.001
McKee, K. L., Cahoon, D. R., and Feller, I. C. (2007). Caribbean mangroves adjust to rising sea level through biotic controls on change in soil elevation. Glob. Ecol. Biogeogr. 16, 545–556. doi: 10.1111/j.1466-8238.2007.00317.x
McKee, K. L., and Faulkner, P. L. (2000). Restoration of biogeochemical function in mangrove forests. Restorat. Ecol. 8, 247–259. doi: 10.1046/j.1526-100x.2000.80036.x
McKee, K. L., Mendelsshon, I. A., and Hester, M. W. (1988). Reexamination of pore water sulfide concentrations and redox potentials near the aerial roots of Rhizophora mangle and Avicennia germinnans. Am. J. Bot. 75, 1352–1359. doi: 10.1002/j.1537-2197.1988.tb14196.x
McLean, N. M., Stephenson, T. S., Taylor, M. A., and Campbell, J. D. (2015). Characterization of future caribbean rainfall and temperature extremes across rainfall zones. Adv. Meteorol. 2015:425987. doi: 10.1155/2015/425987
McLeod, E., Chmura, G. L., Bouillon, S., Salm, R., Bjork, M., Duarte, C. M., et al. (2011). A blueprint for blue carbon: toward an improved understanding of the role of vegetated coastal habitats in sequestering CO2. Front. Ecol. Environ. 9:552–560. doi: 10.1890/110004
Medina, E., Fernandez, W., and Barboza, F. (2015). Element uptake, accumulation, and resorption in leaves of mangrove species with different mechanisms of salt regulation. Web Ecol. 15, 3–13. doi: 10.5194/we-15-3-2015
Medina, E., and Francisco, M. (1997). Osmolality and delta C-13 of leaf tissues of mangrove species from environments of contrasting rainfall and salinity. Estuar. Coast. Shelf Sci. 45, 337–344. doi: 10.1006/ecss.1996.0188
Medina-Calderón, J. H. (2016). Estructura, biomasa y producción primaria neta de bosques de manglar en ambientes kársticos de islas oceánicas. PhD Thesis Dissertation, Universidad Nacional de Colombia sede Caribe, San Andrés.
Medina-Calderón, J. H., and Toro, C. (2018a). Caracterización de Calidad del Agua Subterránea por Unidades de Planificación Insular en San Andrés Isla. San Andrés: Universidad Nacional de Colombia- Sede Caribe.
Medina-Calderón, J. H., and Toro, C. (2018b). Caracterización de la Demanda, Presiones por Uso y Tensores Contaminantes de la Explotación Doméstica de Agua Subterránea en San Andrés por Unidad de Planificación Insular. San Andres: Universidad Nacional de Colombia- Sede Caribe.
Mendez-Alonzo, R., Lopez-Portillo, J., Moctezuma, C., Bartlett, M. K., and Sack, L. (2016). Osmotic and hydraulic adjustment of mangrove saplings to extreme salinity. Tree Physiol. 36, 1562–1572. doi: 10.1093/treephys/tpw073
Mendez-Alonzo, R., Lopez-Portillo, J., and Rivera-Monroy, V. H. (2008). Latitudinal variation in leaf and tree traits of the mangrove Avicennia germinans (Avicenniaceae) in the central region of the Gulf of Mexico. Biotropica 40, 449–456. doi: 10.1111/j.1744-7429.2008.00397.x
Muhammad-Nor, S. M., Huxham, M., Salmon, Y., Duddy, S. J., Mazars-Simon, A., Mencuccini, M., et al. (2019). Exceptionally high mangrove root production rates in the Kelantan Delta. Malaysia; An experimental and comparative study. For. Ecol. Manag. 444, 214–224. doi: 10.1016/j.foreco.2019.04.026
Murdiyarso, D., Purbopuspito, J., Kauffman, J. B., Warren, M. W., Sasmito, S. D., Donato, D. C., et al. (2015). The potential of Indonesian mangrove forests for global climate change mitigation. Nat. Clim. Change 5, 1089–1092. doi: 10.1038/nclimate2734
Nadelhoffer, K. J., Aber, J. D., and Melillo, A. J. (1985). Fine roots, net primary produciton, and soil nitrogen availability: a new hypothesis. Ecology 66, 1377–1390. doi: 10.2307/1939190
Nadelhoffer, K. J., and Raich, J. W. (1992). Fine root production estimates and belowground carbon allocation in forest ecosystems. Ecology 73, 1139–1147. doi: 10.2307/1940664
Naidoo, G. (1987). Effects of Salinity and Nitrogen on Growth and Water Relations in the Mangrove, Avicennia Marina (Forsk.) Vierh. New Phytol 107, 317–325. doi: 10.1111/j.1469-8137.1987.tb00183.x
Naidoo, G. (1989). Seasonal plant water relations in a South African mangrove swamp. Aquat. Bot. 33, 87–100. doi: 10.1016/0304-3770(89)90022-3
Naidoo, G. (1990). Effects of nitrate, ammonium and salinity on growth of the mangrove Bruguiera-gymnorrhiza (l) lam. Aquat. Bot. 38, 209–219. doi: 10.1016/0304-3770(90)90006-7
Naidoo, G. (2016). The mangroves of South Africa: an ecophysiological review. South Afr. J. Bot. 107, 101–113. doi: 10.1016/j.sajb.2016.04.014
Nguyen, H. T., Stanton, D. E., Schmitz, N., Farquhar, G. D., and Ball, M. C. (2015). Growth responses of the mangrove Avicennia marina to salinity: development and function of shoot hydraulic systems require saline conditions. Ann. Bot. 115, 397–407. doi: 10.1093/aob/mcu257
Nickerson, N. H., and Thibodeau, F. R. (1985). Association between pore water sulfide concentrations and the distribution of mangroves. Biogeochemistry 1, 183–192. doi: 10.1007/BF02185041
Norby, R. J., and Jackson, R. B. (2000). Root dynamics and global change: seeking an ecosystem perspective. New Phytol. 147, 3–12. doi: 10.1046/j.1469-8137.2000.00676.x
Ochoa-Gomez, J. G., Lluch-Cota, S. E., Rivera-Monroy, V. H., Lluch-Cota, D. B., Troyo-Dieguez, E., Oechel, W., et al. (2019). Mangrove wetland productivity and carbon stocks in an arid zone of the Gulf of California (La Paz Bay. Mexico). For. Ecol. Manag. 442, 135–147. doi: 10.1016/j.foreco.2019.03.059
Osland, M. J., Feher, L. C., Lopez-Portillo, J., Day, R. H., Suman, D. O., Menendez, J. M. G., et al. (2018). Mangrove forests in a rapidly changing world: global change impacts and conservation opportunities along the Gulf of Mexico coast. Estuar. Coast. Shelf Sci. 214, 120–140. doi: 10.1016/j.ecss.2018.09.006
Pascoalini, S. S., Pereira Tognella, M. M., Oliveira Lima, K. O. D., and Ralph Falquetto, A. (2019). Structural plasticity and species distribution in a peri-urban mangrove of Southeastern Brazil. Sci. Res. Essays 14, 129–144. doi: 10.5897/SRE2018.6645
Persson, H. A. (1983). The distribution and productivity of fine roots in Boreal forests. Plant Soil 71, 87–101. doi: 10.1007/bf02182644
Pezeshki, S. R., and Delaune, R. D. (2012). Soil oxidation-reduction in wetlands and its impact on plant functioning. Biology 1, 196–221. doi: 10.3390/biology1020196
Reef, R., and Lovelock, C. E. (2015). Regulation of water balance in mangroves. Ann. Bot. 115, 385–395. doi: 10.1093/aob/mcu174
Rivera-Monroy, V. H., Castañeda-Moya, E., Barr, J. G., Engel, V., Fuentes, J. D., Troxler, T. G., et al. (2013). “Current methods to evaluate net primary production and carbon budgets in mangrove forests,” in Methods in Biogeochemistry of Wetlands, eds R. D. DeLaune, K. R. Reddy, P. Megonigal, and C. Richardson (Madison WI: Soil Science Society of America Book Series), 243–288. doi: 10.2136/sssabookser10.c14
Rivera-Monroy, V. H., Danielson, T. M., Castaneda-Moya, E., Marx, B. D., Travieso, R., Zhao, X. C., et al. (2019). Long-term demography and stem productivity of Everglades mangrove forests (Florida. USA): resistance to hurricane disturbance. For. Ecol. Manag. 440, 79–91. doi: 10.1016/j.foreco.2019.02.036
Rivera-Monroy, V. H., Farfan, L. M., Brito-Castillo, L., Cortes-Ramos, J., Gonzalez-Rodriguez, E., D’Sa, E. J., et al. (2020). Tropical Cyclone Landfall Frequency and Large-Scale Environmental Impacts along Karstic Coastal Regions (Yucatan Peninsula, Mexico). Appl. Sci. Basel 10:5815. doi: 10.3390/app10175815
Rivera-Monroy, V. H., Lee, S. Y., Kristensen, E., and Twilley, R. R. (2017a). Mangrove Ecosystems: A Global Biogeographic Perspective. New York, NY: Springer.
Rivera-Monroy, V. H., Osland, M. J., Day, J. W., Ray, S., Rovai, A. S., Day, R. H., et al. (2017b). “Advancing Mangrove Macroecology,” in Mangrove Ecosystems: A Global Biogeographic Perspective: Structure, Function and Ecosystem Services, eds V. H. Rivera-Monroy, E. Kristensen, S. Y. Lee, and R. R. Twilley (New York, NY: Springer), 347–371. doi: 10.1007/978-3-319-62206-4_11
Rodriguez-Rodriguez, J. A., Pineda, J. E. M., Melgarejo, L. M., and Calderon, J. H. M. (2018). Functional traits of leaves and forest structure of neotropical mangroves under different salinity and nitrogen regimes. Flora 239, 52–61. doi: 10.1016/j.flora.2017.11.004
Rovai, A. S., Riul, P., Twilley, R. R., Castaneda-Moya, E., Rivera-Monroy, V. H., Williams, A. A., et al. (2016). Scaling mangrove aboveground biomass from site-level to continental-scale. Glob. Ecol. Biogeogr. 25, 286–298. doi: 10.1111/geb.12409
Rovai, A. S., Twilley, R. R., Castaneda-Moya, E., Riul, P., Cifuentes-Jara, M., Manrow-Villalobos, M., et al. (2018). Global controls on carbon storage in mangrove soils. Nat. Clim. Change 8:534. doi: 10.1038/s41558-018-0162-5
Ruess, R. W., Hendrick, R. L., Vogel, J. C., and Sveinbjornsson, B. (2006). “Chapter 12. The Role of Fine Roots in the Functioning of Alaskan boreal Forests,” in Alaska’s Changing Boreal Forest, eds F. S. Chapin, M. W. Oswood, K. B. V. Cleve, L. A. Viereck, and D. L. Verbyla (New York, NY: Oxford University Press), 189–210.
Ruiz-Ramirez, J. D., Euan-Avila, J. I., and Rivera-Monroy, V. H. (2019). Vulnerability of Coastal Resort Cities to Mean Sea Level Rise in the Mexican Caribbean. Coast. Manag. 47, 23–43. doi: 10.1080/08920753.2019.1525260
Saderne, V., Geraldi, N. R., Macreadie, P. I., Maher, D. T., Middelburg, J. J., Serrano, O., et al. (2019). Role of carbonate burial in Blue Carbon budgets. Nat. Commun. 10:1106. doi: 10.1038/s41467-019-08842-6
Saintilan, N. (1997). Above- and below-ground biomasses of two species of mangrove on the hawkesbury river estuary. new south wales. Mar. Freshwater Res. 48, 147–152. doi: 10.1071/mf96079
Saintilan, N., Khan, N. S., Ashe, E., Kelleway, J. J., Rogers, K., Woodroffe, C. D., et al. (2020). Thresholds of mangrove survival under rapid sea level rise. Science 368:1118. doi: 10.1126/science.aba2656
Sánchez-Nuñez, D. A., and Mancera-Pineda, J. E. (2011). Flowering patterns in three neotropical mangrove species: evidence from a Caribbean island. Aquat. Bot. 94, 177–182. doi: 10.1016/j.aquabot.2011.02.005
Sanderman, J., Hengl, T., Fiske, G., Solvik, K., Adame, M. F., Benson, L., et al. (2018). A global map of mangrove forest soil carbon at 30 m spatial resolution. Environ. Res. Lett. 13:055002. doi: 10.1088/1748-9326/aabe1c
Sanders, C. J., Maher, D. T., Tait, D. R., Williams, D., Holloway, C., Sippo, J. Z., et al. (2016). Are global mangrove carbon stocks driven by rainfall? J. Geophys. Res. 121:2600. doi: 10.1002/2016jg003510
Sherman, R. E., Fahey, T. J., and Martinez, P. (2003). Spatial patterns of Biomass and aboveground net primary productivity in a mangrove ecosystem in the Dominican Republic. Ecosystems 6, 384–398. doi: 10.1007/s10021-002-0191-8
Sierra-Rozo, O., Mancera-Pineda, J. E., and Santos-Martínez, A. (2009). Velocidad de descomposición de la hojarasca en diferentes sustratos de manglar durante la época de lluvias en San Andrés isla. Caribe Colomb. Boletín Invest. Mar. 38, 59–84.
Simard, M., Fatoyinbo, L., Smetanka, C., Rivera-Monroy, V. H., Castaneda-Moya, E., Thomas, N., et al. (2019). Mangrove canopy height globally related to precipitation, temperature and cyclone frequency. Nat. Geosci. 12:40. doi: 10.1038/s41561-018-0279-1
Sklar, F., and van der Valk, A. G. (2002). “Tree islands of the Everglades: on overview,” in Tree Islands of the Everglades, eds F. Sklar and A. G. van der Valk (Dortdretch: Kluwer Academic Publishers).
Sobrado, M. A. (2001). Effect of high external NaCl concentration on the osmolality of xylem sap, leaf tissue and leaf glands secretion of the mangrove Avicennia germinans (L.) L. Flora 196, 63–70. doi: 10.1016/s0367-2530(17)30013-0
Stalker, J. C., Price, R. M., Rivera-Monroy, V. H., Herrera-Silveira, J., Morales, S., Benitez, J. A., et al. (2014). Hydrologic Dynamics of a Subtropical Estuary Using Geochemical Tracers, Celestun, Yucatan, Mexico. Estuar. Coasts 37, 1376–1387. doi: 10.1007/s12237-014-9778-5
Stuart, S. A., Choat, B., Martin, K. C., Holbrook, N. M., and Ball, M. C. (2007). The role of freezing in setting the latitudinal limits of mangrove forests. New Phytol. 173, 576–583. doi: 10.1111/j.1469-8137.2006.01938.x
Suarez, N., and Medina, E. (2006). Influence of salinity on Na+ and K+ accumulation, and gas exchange in Avicennia germinans. Photosynthetica 44, 268–274. doi: 10.1007/s11099-006-0018-5
Tamooh, F., Huxham, M., Karachi, M., Mencuccini, M., Kairo, J. G., and Kirui, B. (2008). Below-ground root yield and distribution in natural and replanted mangrove forests at Gazi bay, Kenya. For. Ecol. Manag. 256, 1290–1297. doi: 10.1016/j.foreco.2008.06.026
Taylor, M. A., Clarke, L. A., Centella, A., Bezanilla, A., Stephenson, T. S., Jones, J. J., et al. (2018). Future caribbean climates in a world of rising temperatures: The 1.5 vs 2.0 Dilemma. J. Clim. 31, 2907–2926. doi: 10.1175/jcli-d-17-0074.1
Thibodeau, F. R., and Nickerson, N. H. (1986). Differential oxidation of mangrove substrate by Avicennia-germinans and Rhizophora-mangle. Am. J. Bot. 73, 512–516. doi: 10.2307/2444255
Thomas, N., Lucas, R., Bunting, P., Hardy, A., Rosenqvist, A., and Simard, M. (2017). Distribution and drivers of global mangrove forest change, 1996-2010. PLoS One 12:e0179302. doi: 10.1371/journal.pone.0179302
Twilley, R., Rovai, A. S., and Riul, P. (2018). Coastal morphology explains global blue carbon distributions. Front. Ecol. Environ. 16, 503–508. doi: 10.1002/fee.1937
Twilley, R. R., Castaneda-Moya, E., Rivera-Monroy, V. H., and Rovai, A. S. (2017). “Chapter 5. Productivity and Carbon Dynamics in Mangrove Wetlands,” in Mangrove Ecosystems: A Global Biogeographic Perspective Structure, Function and Ecosystem Services, eds V. H. Rivera-Monroy, S. Lee, Y. E. Kristensen, and R. R. Twlley (New York, NY: Springer), 113–162. doi: 10.1007/978-3-319-62206-4_5
Twilley, R. R., and Rivera-Monroy, V. H. (2005). Developing performance measures of mangrove wetlands using simulation models of hydrology, nutrient biogeochemistry, and community dynamics. J. Coast. Res. 21, 79–93.
Twilley, R. R., and Rivera-Monroy, V. H. (2009). “Ecogeomorphic Models of Nutrient Biogeochemistry for Mangrove Wetlands,” in Coastal Wetlands: An Integrated Ecosystem Approach, eds G. M. Perillo, E. Wolanski, D. R. Cahoon, and M. M. Brinson (Amsterdam: Elsevier), 641–683.
Urrego, L. E., Polania, J., Buitrago, M. F., Cuartas, L. F., and Lema, A. (2009). Distribution of mangroves along environmental gradients on San Andres Island (Colombian Caribbean). Bull. Mar. Sci. 85, 27–43.
Vogt, K. A., Vogt, D. J., and Bloomfield, J. (1998). Analysis of some direct and indirect methods for estimating root biomass and production of forests at an ecosystem level. Plant Soil 2000, 71–89.
Vogt, K. A., Vogt, D. J., Moore, E. E., Fatuga, B. A., Redlin, M. R., and Edmonds, R. L. (1987). Conifer and angiosperm fine-root biomass in relation to stand age and site productivity in Douglas-fir forests. J. Ecol. 75, 857–870. doi: 10.2307/2260210
Vovides, A. G., Berger, U., Grueters, U., Guevara, R., Pommerening, A., Lara-Dominguez, A. L., et al. (2018). Change in drivers of mangrove crown displacement along a salinity stress gradient. Funct. Ecol. 32, 2753–2765. doi: 10.1111/1365-2435.13218
Vovides, A. G., Vogt, J., Kollert, A., Berger, U., Grueters, U., Peters, R., et al. (2014). Morphological plasticity in mangrove trees: salinity-related changes in the allometry of Avicennia germinans. Trees Struct. Funct. 28, 1413–1425. doi: 10.1007/s00468-014-1044-8
Walters, B. B., Ronnback, P., Kovacs, J. M., Crona, B., Hussain, S. A., Badola, R., et al. (2008). Ethnobiology, socio-economics and management of mangrove forests: a review. Aquat. Bot. 89, 220–236. doi: 10.1016/j.aquabot.2008.02.009
Wang, C. G., Brunner, I., Zong, S. W., and Li, M. H. (2019). The dynamics of living and dead fine roots of forest biomes across the northern Hemisphere. Forests 10:953. doi: 10.3390/f10110953
Wang, W. Q., Yan, Z. Z., You, S. Y., Zhang, Y. H., Chen, L. Z., and Lin, G. H. (2011). Mangroves: obligate or facultative halophytes? A review. Trees Struct. Funct. 25, 953–963. doi: 10.1007/s00468-011-0570-x
Wang, X., Zhou, L., and Lu, C. (2018). Do environmental factors affect the male frequency of exotic mangrove species Laguncularia racemosa (Combretaceae) along the southeast coast of China? Aquat. Ecol. 52, 235–244. doi: 10.1007/s10452-018-9658-3
Woodroffe, C. D., Rogers, K., McKee, K. L., Lovelock, C. E., Mendelssohn, I. A., and Saintilan, N. (2016). “Mangrove Sedimentation and Response to Relative Sea-Level Rise,” in Annual Review of Marine Science, Vol. 8, eds C. A. Carlson and S. J. Giovannoni (Palo Alto, CA: Annual Reviews), 243–266. doi: 10.1146/annurev-marine-122414-034025
Yanez-Espinosa, L., Terrazas, T., Lopez-Mata, L., and Valdez-Hernandez, J. I. (2004). Wood variation in Laguncularia racemosa and its effect on fibre quality. Wood Sci. Technol. 38, 217–226. doi: 10.1007/s00226-004-0228-6
Zaldivar-Jimenez, A., Herrera-Silveira, J., Teutli-Hernández, C., Comin, F. A., Andrade, A., Coronado-Molina, C., et al. (2010). Conceptual framework for mangrove restoration in the Yucatán Peninsula. Ecol. Restorat. 28, 333–342. doi: 10.3368/er.28.3.333
Zhang, H. C., Yuan, W. P., Dong, W. J., and Liu, S. G. (2014). Seasonal patterns of litterfall in forest ecosystem worldwide. Ecol. Complex. 20, 240–247. doi: 10.1016/j.ecocom.2014.01.003
Zhao, X. C., Rivera-Monroy, V. H., Wang, H. Q., Xue, Z. G., Tsai, C. F., Willson, C. S., et al. (2020). Modeling soil porewater salinity in mangrove forests (Everglades, Florida, USA) impacted by hydrological restoration and a warming climate. Ecol. Model. 436:109292. doi: 10.1016/j.ecolmodel.2020.109292
Keywords: karstic-platform mangroves, root biomass, root production, root turnover rate, hydroperiod, regulators, resources, seaflower biosphere
Citation: Medina-Calderón JH, Mancera-Pineda JE, Castañeda-Moya E and Rivera-Monroy VH (2021) Hydroperiod and Salinity Interactions Control Mangrove Root Dynamics in a Karstic Oceanic Island in the Caribbean Sea (San Andres, Colombia). Front. Mar. Sci. 7:598132. doi: 10.3389/fmars.2020.598132
Received: 23 August 2020; Accepted: 14 December 2020;
Published: 14 January 2021.
Edited by:
Stelios Katsanevakis, University of the Aegean, GreeceCopyright © 2021 Medina-Calderón, Mancera-Pineda, Castañeda-Moya and Rivera-Monroy. This is an open-access article distributed under the terms of the Creative Commons Attribution License (CC BY). The use, distribution or reproduction in other forums is permitted, provided the original author(s) and the copyright owner(s) are credited and that the original publication in this journal is cited, in accordance with accepted academic practice. No use, distribution or reproduction is permitted which does not comply with these terms.
*Correspondence: Víctor H. Rivera-Monroy, dmhyaXZlcmFAbHN1LmVkdQ==
†ORCID: Jairo Humberto Medina-Calderón, orcid.org/0000-0003-2512-0474; José Ernesto Mancera-Pineda, orcid.org/0000-0003-2948-3387; Edward Castañeda-Moya, orcid.org/0000-0001-7759-4351; Víctor H. Rivera-Monroy, orcid.org/0000-0003-2804-4139
Disclaimer: All claims expressed in this article are solely those of the authors and do not necessarily represent those of their affiliated organizations, or those of the publisher, the editors and the reviewers. Any product that may be evaluated in this article or claim that may be made by its manufacturer is not guaranteed or endorsed by the publisher.
Research integrity at Frontiers
Learn more about the work of our research integrity team to safeguard the quality of each article we publish.