- Simon F.S. Li Marine Science Laboratory, School of Life Sciences, The Chinese University of Hong Kong, Hong Kong, China
Biological fitness relies on processes acting at various levels of organization, all of which can be modified by environmental change. Application of synthesis frameworks, such as the Adverse Outcome Pathway (AOP), can enhance our understanding of the responses to stressors identified in studies at each level, as well as the links among them. However, the use of such frameworks is often limited by a lack of data. In order to identify contexts with sufficient understanding to apply the AOP framework, we conducted a meta-analysis of studies considering ocean acidification effects on calcifying mollusks. Our meta-analysis identified that most studies considered the adult life history stage, bivalve taxonomic group, individual-level changes, and growth- and metabolism-related responses. Given the characteristics of the published literature, we constructed an AOP for the effects of ocean acidification on calcification in an adult bivalve, specifically the Pacific oyster (Magallana gigas). By structuring results within the AOP framework, we identify that, at present, the supported pathways by which ocean acidification affects oyster calcification are via the downregulation of cavortin and arginine kinase transcription. Such changes at the molecular level can prompt changes in cellular and organ responses, including altered enzyme activities, lipid peroxidation, and regulation of acid–base status, which have impacts on organism level metabolic rate and, therefore, calcification. Altered calcification may then impact organism mortality and population sizes. We propose that when developed and incorporated in future studies, the AOP framework could be used to investigate sources of complexity including varying susceptibility within and among species, feedback mechanisms, exposure duration and magnitude, and species interactions. Such applications of the AOP framework will allow more effective reflections of the consequences of environmental change, such as ocean acidification, on all levels of biological organization.
Introduction
Organism fitness depends on processes acting at various levels of biological organization, all of which are affected by abiotic conditions (Prosser, 1955). The response of organisms to changing environmental conditions is, therefore, governed by cumulative effects across multiple biological levels, from molecular pathways to whole-organism processes. Ultimately, changes in organism fitness will affect population composition, ecosystem structure, and evolutionary potential (Munday et al., 2013; Queirós et al., 2014).
Marine systems around the world are experiencing unprecedented rates of abiotic change, with ocean acidification associated with rapid alterations in environmental conditions (Burrows et al., 2011). It is estimated that the oceans have absorbed almost half of the carbon dioxide emitted globally almost the past two decades (Sabine et al., 2004). Such uptake has resulted in the average oceanic pH declining by 0.1 units compared to preindustrial levels, in a process termed ocean acidification (Caldeira, 2005). In addition to reduced pH, ocean acidification also incorporates other alterations to seawater chemistry, including reduction in the calcium carbonate saturation state and alteration of the global carbon cycle (Zeebe and Wolf-Gladrow, 2001). In the future, seawater pH is predicted to undergo a 0.3–0.4 unit decrease by 2100 (Caldeira and Wickett, 2003; IPCC, 2013). As pH is measured on the logarithmic scale, this corresponds to a doubling in acidity by the end of the century and represents a profound change for future oceans.
Ocean acidification is recognized to negatively impact many calcifying organisms, including mollusks (see reviews by Kroeker et al., 2010; Gazeau et al., 2013 and references therein). Such impacts are of concern given that mollusks fulfill numerous ecological roles (Gazeau et al., 2013), provide an abundance of ecosystem services (Lemasson et al., 2017), and represent an aquaculture industry that was worth US $19 billion globally in 2016 (FAO, 2016). The effects of ocean acidification on calcifying mollusks have been suggested to result largely from impacts on the development of calcified structures, as well as their maintenance via enhanced erosion and dissolution due to the increased porosity of the shells' internal microstructure (e.g., Marshall et al., 2008; Nienhuis et al., 2010; Rodolfo-Metalpa et al., 2011; Fitzer et al., 2015, 2016, 2019; Meng et al., 2018; Byrne and Fitzer, 2019; Leung et al., 2020a). Although calcification represents one of the physiological processes most susceptible to ocean acidification (Kroeker et al., 2010), studies have only relatively recently started to unravel the complex drivers of such effects (e.g., Meng et al., 2018; Clark et al., 2020; Leung et al., 2020b). Currently, the key mechanism impairing calcification under ocean acidification is considered to be the exacerbation of the energetic costs of maintaining internal pH homeostasis under the increased concentration of protons in the surrounding seawater (Barry et al., 2011; Stumpp et al., 2011; Waldbusser et al., 2015; Cyronak et al., 2016; Fitzer et al., 2016). The required reallocation of metabolic resources to maintain calcified structures appears to occur at the detriment of other physiological processes including growth, reproduction, and immune system function (Wood et al., 2008; Scanes et al., 2014; Garilli et al., 2015; Harvey and Moore, 2017; Leung et al., 2017; Telesca et la., 2019). Promisingly, some calcifying mollusks can buffer the effects of acidification through energy reallocation (Leung et al., 2020b), adjusting internal pH (Zhao et al., 2018), and adjusting the mineralogical composition of their shells (Leung et al., 2017, 2020a). It is important to note, however, that such adaptive capacities may be considerably reduced when acidity is modified to more extreme levels, indicating that processes associated with calcification may lack the capacity to overcome the challenges of future acidification (Leung et al., 2020a). Of particular concern are changes occurring in oysters, which are a group of considerable economic and ecological importance (see Lemasson et al., 2017 and references therein). For oysters, acidification is considered to reduce individual fitness by generating energetically costly effects in early life history stages that are then carried over to later stages (Hettinger et al., 2013). Specifically, acidification can decrease larval metamorphosis by up to 30% (Talmage and Gobler, 2009), as it alters the expression of proteins involved in energy production and calcification (Dineshram et al., 2016). Such changes can lead to later life stages experiencing abnormal development, decreased body size, and altered shell properties (Parker et al., 2009; Hettinger et al., 2012; Gazeau et al., 2013).
While a general understanding of the pathways by which ocean acidification influences marine calcifiers is being developed, confidence may be increased where more rigorous synthesis approaches are used. That is, the above description of effects of ocean acidification on calcification is based on a “qualitative literature review” approach, where we selected the portion of evidence we felt best described the process (Falkenberg et al., 2018). A more objective approach could be the application of a conceptual framework that synthesizes the sequential cascade of responses initiated by environmental stressors, specifically the Adverse Outcome Pathway (AOP) framework (Ankley et al., 2010). AOPs were first developed in 1992 to bring together key information on the sequential consequences of toxicants across biological processes (Ankley et al., 2010). The subsequent adoption of AOPs in toxicology has gained momentum (Leist et al., 2017), leading to the design of online databases gathering information on a myriad of stressors and their impacts (Society for Advancement of AOPs, 2020).
To date, the AOP framework has yet to be used to address ocean acidification, with few examples of its application to global change parameters (but see Hooper et al., 2013). Nonetheless, the effects of human-driven global change (e.g., increased temperatures, hypoxia, or ocean acidification) are akin to human-derived toxicants, as they represent potentially lethal threats to organisms. Successful application of the AOP framework within the context of global change will depend on sufficient data availability for different: (a) life history stages, (b) taxonomic groups, (c) biological responses, and (d) response variables. Where the required data exist, application of synthesis frameworks, such as the AOP, can enhance our understanding of the generality of responses identified in studies of each level, as well as the links between those observed from molecular, to physiological, and population levels. Thus, AOPs would provide a unique and comprehensive tool to address climate-related impacts on ecosystems and the species within them (Figure 1; Hooper et al., 2013; Falkenberg et al., 2018).
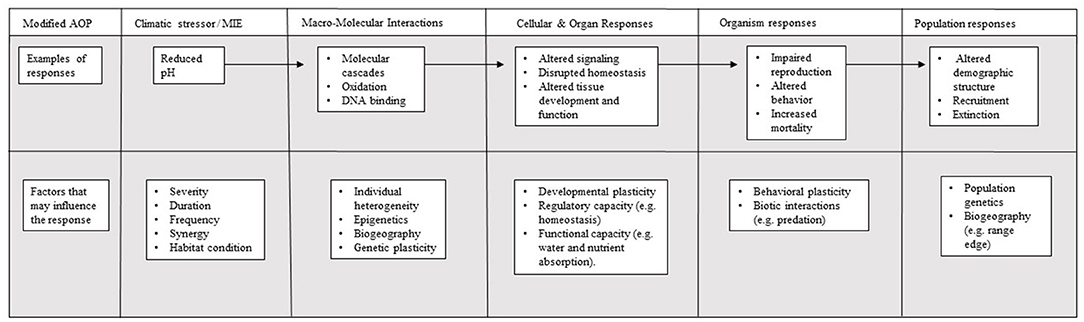
Figure 1. Conceptual diagram of the Adverse Outcome Pathway (AOP) linking the effect of the toxicant, considered to be ocean acidification, with the mechanistic toxicity pathway, and adverse outcomes (based on Ankley et al., 2010).
Here, we explore the potential for developing more accurate predictions of ocean acidification effects on organism fitness based on empirical data using the AOP framework. To identify the contexts in which there would be sufficient understanding to apply the AOP framework, we conducted a meta-analysis of studies considering the effects of ocean acidification on calcifying mollusks. Findings were organized to recognize our understanding of acidification across: (a) life history stages, (b) taxonomic groups, (c) biological levels, and (d) response variables. We then used the identified literature to construct an AOP for calcification in an organism and life stage we found to be well-studied. Where the AOP approach is developed, advanced, and informed by a greater number of studies, it will ultimately enhance our ability to relate findings across levels of biological organization.
Methods
Literature Search and Suitability Criteria
The meta-analysis followed Preferred Reporting Items for Systematic Reviews and Meta-Analyses (PRISMA) guidelines, which provide an evidence-based set of requirements for conducting and reporting meta-analyses. First, a standardized search for peer-reviewed research articles about the effects of ocean acidification on marine mollusks was conducted on Web of Science, Scopus, and Science Direct in October 2019. The search string used was TS = [(“ocean acidification” OR “marine acidification” OR “seawater acidification”) AND (“mollusc” OR “mollusk”)], the language was restricted to English, and no constraints were placed on the publication year.
The initial search identified 5,850 potentially relevant papers, which were then screened to determine their eligibility. Specifically, studies were narrowed to peer-reviewed research relating to contemporary climate change. Papers included in our analysis are those that considered the effect of a control “ambient” acidification treatment and at least one “future” acidification treatment as based on the author's opinion of “ambient” and “future” levels of ocean acidification. These treatments needed to be achieved using commonly accepted methods, with studies that manipulated carbonate chemistry with acid addition excluded (following criteria outlined by Harvey et al., 2013). All the abstracts were then read from the retained papers, and the full manuscripts obtained for those studies that indicated in their title, abstract, or keywords that relevant data could be collected (n = 517 papers). These papers were then read, and 267 papers were included.
For each of the included papers, we extracted information on the considered: (a) life history stages, (b) taxonomic groups, (c) biological levels, and (d) response variable groups. For studies that included more than one life history stage, taxonomic group, biological level, or response variable in the reported experiment(s), all separate life history stages, taxonomic groups, biological levels, and response variables were included as long as they met our overall criteria for inclusion. Response variables were grouped into the most commonly considered metrics (i.e., calcification, survival, growth, reproduction, development, and metabolism) as employed in previous meta-analyses (e.g., Gazeau et al., 2013; Harvey et al., 2013). Additionally, counts of the numbers of life history stages, taxonomic groups, biological levels, and response variables groups were made to determine the number of levels of each characteristic considered in each study.
Statistical Analysis
Chi-squared tests were used to examine differences in the frequency of (a) life history stages, (b) taxonomic groups, (c) biological levels, and (d) response variable groups considered in each study, as well as the frequency of the number of factors listed above (1, 2, or >2 factors). All tests were done in GraphPad Prism v 8.42.
AOP Framework Development
Studies considering the adult Pacific oyster, Magallana gigas, were then used to develop an AOP addressing the cascading mechanisms resulting in effects on calcification in response to acidification in adults of this species. This case study was selected as the focal life history stage (adult), taxonomic group (bivalve), biological level (individual), and response variable of interest (calcification) were well-represented in our meta-analysis relative to other levels. To develop the AOP, results from our meta-analysis were grouped according to the biological level of organization and organized into the major outcomes of studies, termed key events (KEs), caused by acidification. The KEs were then organized into a causal sequence starting with the molecular initiating event (MIE) (i.e., the reduction in external pH), progressing through the hierarchical levels of organization, and culminating in the outcomes (referred to as adverse outcomes, AOs, under the AOP terminology) at the organism and population level. Each KE is connected by key event relationships (KERs), which describe how alterations in upstream events (lower levels of organization) relate to the downstream modifications (higher levels of organization). The number of studies supporting each KE for M. gigas in the present meta-analysis is indicated. If certain KEs were not supported by these studies, plausible linkages with further evidence from other bivalve species were added. Further information on existing guidelines for constructing AOPs is available on the publicly accessible knowledgebase (Society for Advancement of AOPs, 2020).
Results
There were significant differences in the proportion of studies across: (a) life history stages [ = 273.371, p < 0.0001; Figure 2A], (b) taxonomic groups [ = 479.696, p < 0.0001; Figure 2B], (c) biological levels [ = 475.324, p < 0.0001; Figure 2C], and (d) response variable groups [ = 160.139, p < 0.001; Figure 2D). In terms of life history stages, there was a higher proportion of studies considering adults (60%) than each of the other stages (i.e., embryos, larvae, and juveniles were all <20%; Figure 2A). While less well-studied than adults, the effects of acidification on earlier life stages is relatively well-understood, as 16% of studies in our review consider larvae and another 15% consider juvenile life stages, comprising 117 studies total (60 and 57 studies, respectively). Across taxonomic groups, almost three-quarters of the studies examined effects on bivalve species (70%), with the next most commonly considered group gastropods (22%) (Figure 2B). The majority of studies considered individual level responses (65%; Figure 2C), primarily focusing on response variable groups relating to growth (27%), metabolism (26%), survival (16%), and calcification (13%; Figure 2D).
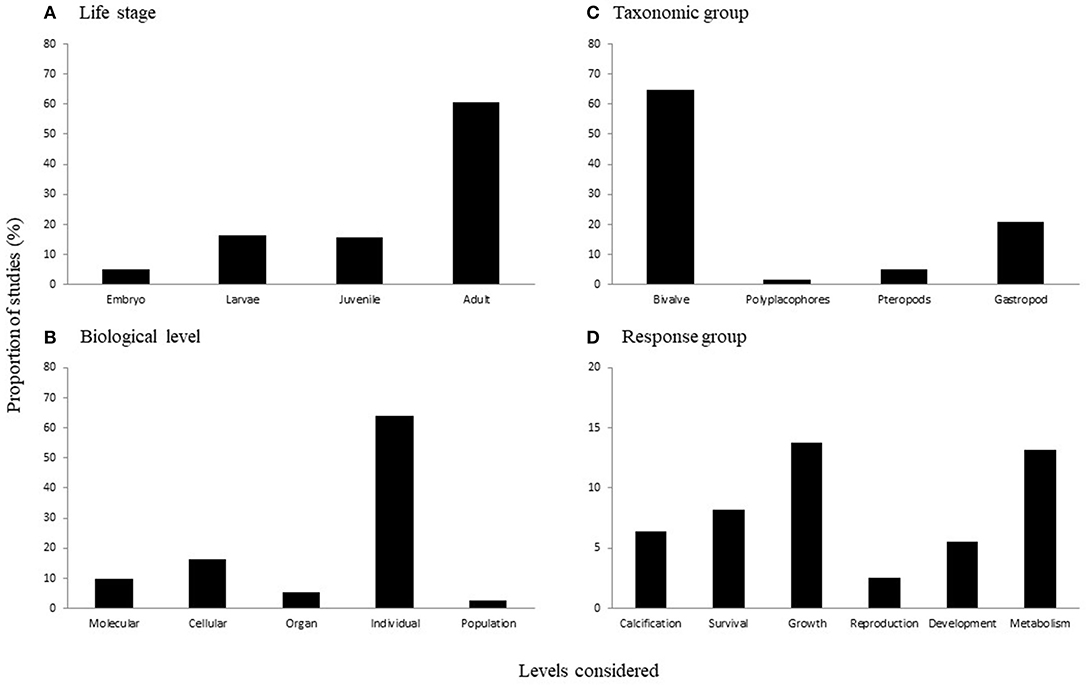
Figure 2. The proportion of studies considering different levels of (A) life stages, (B) taxonomic groups, (C) biological levels, and (D) response variable groups. Note the difference in scale on the y-axis of (D).
The number of levels of each characteristic considered in a study varied across the categories of interest. That is, the majority of studies considered just one life history stage [90%; = 397.011, p < 0.0001; Figure 3A], taxonomic group [94%; = 448.472, p < 0.0001; Figure 3B], or biological level [73%, = 209.598, p < 0.0001; Figure 3C]. In contrast, the number of response variable groups more often considered two or more levels, although there was a significant difference in the number of levels considered [ = 11.048, p < 0.01; Figure 3D].
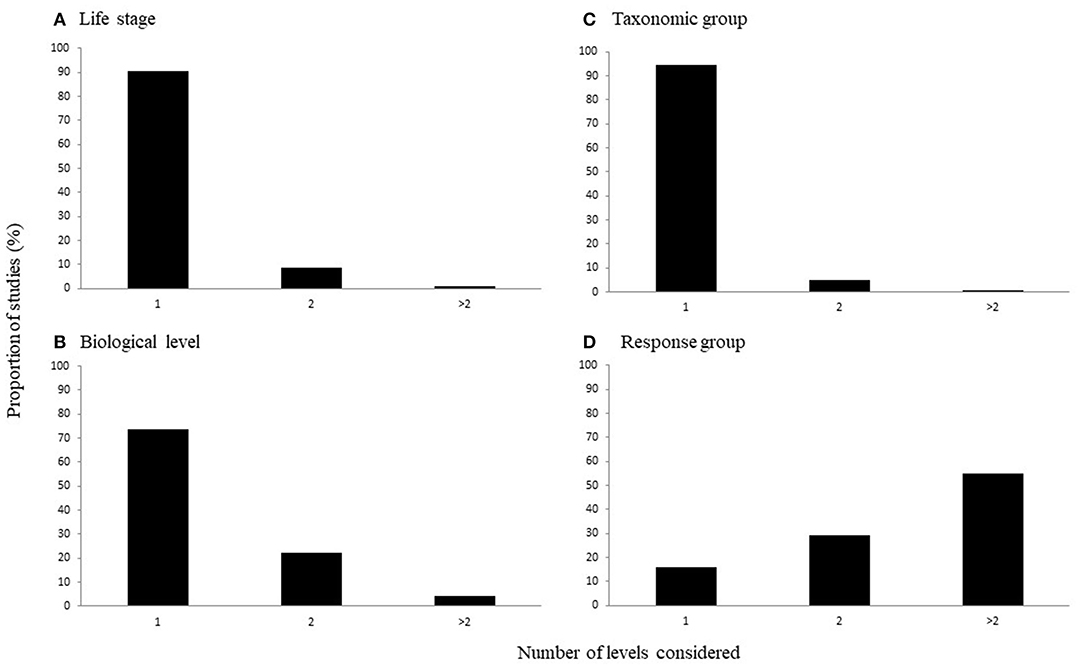
Figure 3. The proportion of studies considering different numbers of levels of (A) life stages, (B) taxonomic groups, (C) biological levels, and (D) response variable groups.
The AOP that links the molecular initiating event of reduced seawater pH to the adverse effect of altered calcification and effects at higher levels highlights what is currently understood regarding the related pathways for M. gigas (Figure 4). A direct pathway supported by the literature on M. gigas is via the molecular downregulation of cavortin transcription. A second supported pathway to changes in calcification is via the molecular level change of downregulated genes encoding metabolic pathways, including kinase transcription. These changes lead to the cellular- and organ-level responses of altered enzyme activities and increased lipid peroxidation, which can lead to a depressed metabolic rate that is, in turn, linked with altered calcification. Similarly, the downregulation of genes encoding metabolic pathways can also lead to the deregulation of acid–base status, decreased metabolic rates, and altered calcification rates (although there is no M. gigas-specific support for this deregulation). The consequences of altered calcification are also considered in the AOP; altered calcification can potentially prompt organism-level changes in shell thickness that lead to increased mortality and ultimately population level declines.
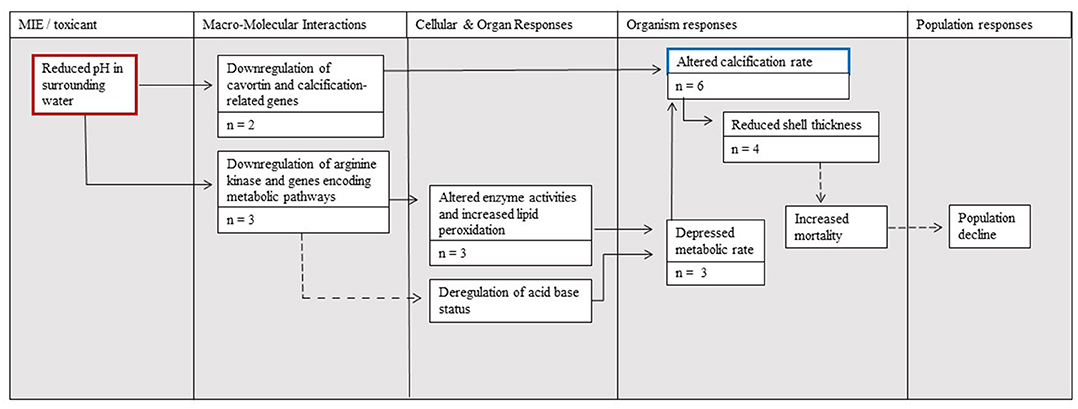
Figure 4. The Adverse Outcome Pathway (AOP) linking the effects of ocean acidification (red border) to calcification (blue border) and its effects on Magallana gigas as supported by the studies assembled in the present meta-analysis. The number of studies supporting each step is denoted by n = X. Solid arrow lines represent established linkages with quantitative data documented for M. gigas, whereas dotted arrow lines represent linkages supported with data considering other bivalves.
Discussion
Forecasting the effects of ocean acidification on calcifying organisms, and the associated ecosystems and human societies, will benefit from the use of structured frameworks informed by sufficient experimental evidence. Here, we identified that such an approach may be more suitable for certain groups; in the context of marine mollusks, greater evidence is available for particular life history stages (adults), biological levels (individuals), taxonomic groups (bivalves), and response variables (metabolism, growth, survival, and calcification). Promisingly, there is potential that the existing evidence can be used to inform structured frameworks, such as the Adverse Outcome Pathway. Here, we consider the impact of ocean acidification on a well-represented life stage, biological level, taxonomic group, and response variable, specifically the calcification of individual adult oysters, M. gigas. The resulting AOP reveals the extent of repercussions across biological levels as a result of simulated acidification treatments. It allows us to conclude that, at present, the pathways by which ocean acidification is known to impact calcification in M. gigas is via the downregulation of cavortin and kinase transcription. As gaps in the existing literature are filled, more complex AOPs will be able to be developed.
Characteristics of Existing Literature Regarding Effects of Ocean Acidification on Mollusks and Their Implications for AOP Development
Characteristics of the existing literature identified in meta-analyses will determine our ability to develop well-informed frameworks. Promisingly, here we identified that a range of response variables were relatively well-represented in the current literature (i.e., growth, metabolism, and survival; Figure 2D). Moreover, the number of response variables considered in each study was typically two or above, indicating that we are developing an understanding of how responses relate to each other across groups (Figure 3D). That is, where a number of response variables are considered, particularly within a single study, this provides key information for subsequent AOPs in terms of how processes affect one another both within, and across, levels of organization.
Our meta-analysis also revealed traits of the existing literature that could be strengthened to enhance the development of future AOPs. In this context, it is worth noting that an emphasis has been placed on considering a single life history stage within each study, typically the adults (Figures 2A, 3A). Such a focus on the adult life stage, and the omission of others, limits our capacity to understand how effects differ across life stages and also how effects on early life stages, such as the embryo, can influence later life stages. For example, studies in our meta-analysis considering early life history stages identified that larvae experienced negative responses in metamorphosis and development (Scanes et al., 2014; Dineshram et al., 2016; Wang et al., 2017) likely due to changes in the expression of proteins related to energy production (Dineshram et al., 2012, 2015, 2016). Similarly, a certain taxonomic group, specifically the bivalves, was the focus of the majority of studies (Figures 2B, 3B), considering that a broader range of taxonomic groups will be important given the potential for varying responses to manifest, even among closely related groups (reviewed by Doney et al., 2009). Moreover, we identified that fewer studies consider “lower” level processes (i.e., molecular or cellular), with the majority focused on those that manifest at the individual level (e.g., growth, respiration; Figure 2C). In addition, the majority of studies considered one biological level (Figure 3C). Altogether, these traits of the existing literature confer challenges to scaling effects and understanding how responses are connected. For instance, connecting across levels will allow us to understand how responses at the molecular level, such as altered gene expression patterns, may generate outcomes for processes operating at the cellular or organ levels (Harvey et al., 2013). Overall, consideration of the less commonly studied groups identified here—be they life history stages, taxonomic groups, or biological levels—will improve our understanding regarding the generality of responses and, therefore, AOP development.
Considering the Effects of Ocean Acidification Using the AOP Framework
The AOP constructed here for the calcification of the oyster M. gigas indicates biological pathways impacted by ocean acidification (Figure 4). In this application of the AOP framework, the reduction in external pH is considered to be the molecular initiating event (MIE). The changes in external pH associated with ocean acidification can then be detected by the oysters through several mechanisms, including signaling peptides (Roggatz et al., 2016), ingestion of acidified water (Jaeckle, 2017), or direct contact (O'Donnell et al., 2013). Once detected, the associated molecular signal is then transmitted and received.
When the chemical signal associated with ocean acidification is received, effects can be observed at the molecular level. Anticipated molecular level changes include the decreased expression of genes involved in the calcification process, such as the transcription of cavortin, a gene involved in shell deposition (Itoh et al., 2011; Wei et al., 2015). In addition, the MIE can also lead to molecular changes that result in decreased expression of arginine kinase (AK) and adenosine triphosphate (ATP), which are fundamental components in metabolic pathways (Dineshram et al., 2012; Wei et al., 2015; Evans et al., 2017).
The changes at the molecular level can then generate changes at the cellular and organ level. Here, we anticipate that the downregulation of genes encoding metabolic pathways would lead to changes in enzyme activities, increases in lipid peroxidation, and deregulation of the acid–base status (e.g., extracellular acidosis). These changes subsequently alter the individual level rates of energetic expenditure and metabolism (Michaelidis et al., 2005; Wang et al., 2017), which are linked with decreased calcification rates.
Impacts on the individual level process of calcification can then scale up to affect “higher” levels of organization. That is, our meta-analysis and subsequent AOP highlight that altered calcification can lead to impaired shells in oysters; for example, ocean acidification has led to a drop in shell hardness and stiffness in the oyster Magallana angulata (Meng et al., 2018). Such changes have been shown to result in increased mortality in other bivalves. That is, thinner shells are weaker, which directly increases the prevalence of shell microfractures and reduces shell resistance (Dickinson et al., 2011), increasing the susceptibility of individuals to predation (Fitzer et al., 2016). Indirect effects can, however, alter the direct effect of ocean acidification on shell structure. That is, where energy-rich food is available under ocean acidification, calcifiers can build shells that are thicker, more crystalline, and more mechanically resilient (Leung et al., 2019). Where such resources are not available, these responses not possible, and shells impaired, the increased mortality at the individual level can then lead to a decline in the size of the overall population.
In addition to highlighting what is known regarding the effects of ocean acidification, constructing this AOP also highlighted areas of uncertainty. That is, while the AOP reveals links from the chemical changes driven by ocean acidification through the various levels of biological organization to the population responses, there is still uncertainty regarding many key steps and their effects. This uncertainty occurs despite M. gigas representing a relatively well-studied species. Such uncertainty results as the existing experimental literature makes few connections across levels of biological hierarchy, with extensive variations identified where such connections have been made (e.g., Dineshram et al., 2012; Wei et al., 2015). The strength of links from lower to higher biological levels of organization may also vary, which is not taken into account under the AOP framework. For example, an impact with strong links across levels would be reduced antioxidant activity, which lowers immune system function and directly impacts individual survival (Wang et al., 2017). In contrast, an impact with weaker or less direct links across levels would be a reduction in metabolic efficiency, which represents a malleable response with largely indirect effects on individual fitness through energy reallocation (Cao et al., 2018). Connections across biological levels, therefore, require further consideration as, despite our limited understanding, they are recognized to play a key influence in the overall response of mollusks (Queirós et al., 2014) and other calcifying taxa to environmental change (Calosi et al., 2013; Palumbi et al., 2014).
Recommendations for Future AOP Development
The development of AOPs considering the impacts of environmental stressors associated with global change, such as ocean acidification, provides an opportunity to explore the impacts of more complex scenarios. Some key aspects of complexity that could be considered in future iterations of this framework include varying susceptibility within and among species; feedback mechanisms; stressor duration, magnitude, and co-occurrence; as well as species interactions.
Varying Susceptibility Within and Among Species
In the future, the AOP framework could be used to consider mechanisms underlying varying responses that have been found, for example, between populations across large spatial areas (e.g., Parker et al., 2010; Ginger et al., 2013; Kraft et al., 2014; Falkenberg et al., 2019; Telesca et al., 2019; Clark et al., 2020), among distinct populations of the same species (e.g., Stapp et al., 2016), among closely related species (Vihtakari et al., 2016), and across different taxonomic groups (e.g., Kroeker et al., 2010). For example, recent studies considering a range of ectotherms from different taxonomic groups have suggested that Nab/Kb ATPase, a major cellular ATP consumer (Wieser and Krumschnabel, 2001), may be broadly susceptible to the effects of acidification (Pörtner et al., 2004; Lannig et al., 2010). If AOPs were to be constructed for a range of organisms representing diverse taxonomic groups, and all the pathways included impacts on the Nab/Kb ATPase, it would indicate the general importance of this pathway. In contrast, if the AOPs did not reveal this expected pattern, it would highlight that there are greater differences among taxonomic groups than anticipated. In addition to providing insight into conserved mechanisms across species, AOPs also have the potential to reveal divergent processes that lead to varied responses. For example, the mechanisms that cephalopods and some echinoderms use to maintain internal pH under acidification contrast with the processes used by bivalves. That is, cephalopods and some echinoderms can partially or fully restore their internal pH through the use of extracellular respiratory pigments and accumulation of bicarbonate (Gutowska et al., 2010); in contrast bivalves, which lack these pigments, are unable to adjust their internal pH in this way (Collard et al., 2013). Such differences would be revealed in the AOPs constructed for species of these groups.
Feedback Mechanisms—Between and Within Biological Levels
Despite the broad outcomes of ocean acidification being relatively well-documented, the implications of processes acting between and within biological levels require further consideration (Figure 5). Of particular interest are feedbacks moving between biological levels from “higher” to “lower” levels (e.g., compromised immune system affecting metabolic pathways; Figure 5C) and within-level feedback cycles (e.g., reduced individual growth affecting energy expenditure affecting reduced individual growth, etc.; Figure 5B). Feedback mechanisms between biological levels could be explored using the AOP framework where outcomes at higher levels of biological organization are linked to their consequences for processes at lower levels. An example of such feedbacks includes the effect of reduced organism level metabolic rate altering cellular- and organ-level processes, specifically enzyme activity and increasing lipid peroxidation (Wei et al., 2015; Wang et al., 2017). The AOP framework also provides an opportunity to explore within-level impacts. For instance, at the level of the organism, changes in the process of calcification and development may subsequently affect metabolic rates (e.g., in sea urchin; Stumpp et al., 2011).
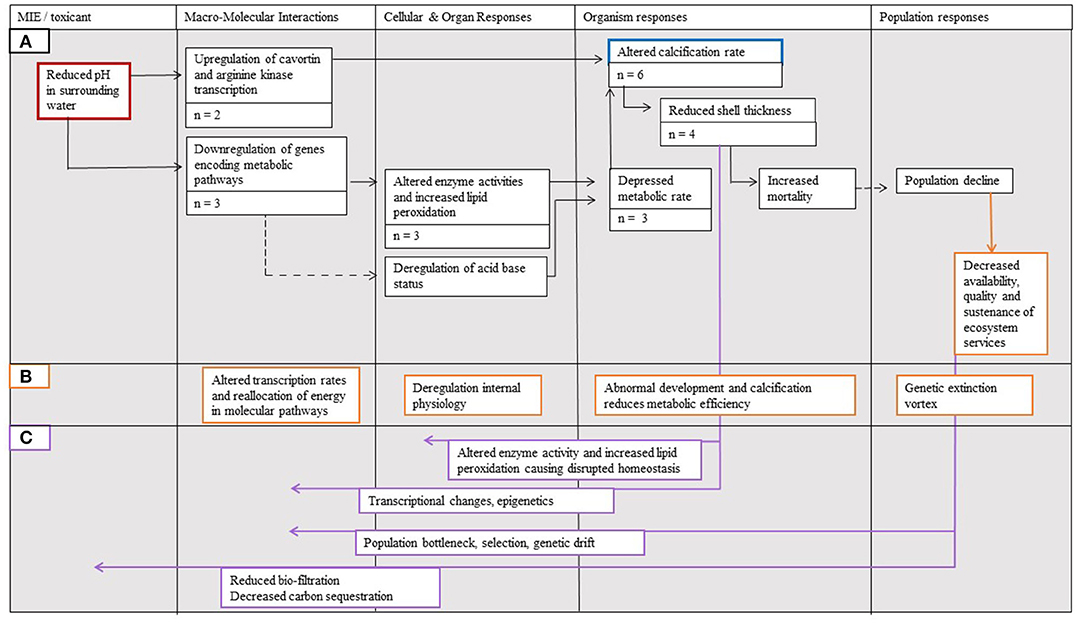
Figure 5. The Adverse Outcome Pathway (AOP) linking the effects of (A) ocean acidification (red border) to calcification (blue border) and its effects on Magallana gigas, amended to incorporate (B) within-level feedback mechanisms (orange borders indicate added examples of responses and arrow lines indicate added pathways), as well as (C) interaction effects from higher to lower levels of biological organization (purple arrow lines indicate added pathways). The number of studies supporting each step is denoted n = X. Solid arrow lines represent established linkages with quantitative data documented for M. gigas, whereas dotted arrow lines represent linkages supported with data considering other bivalves.
Stressor Duration, Magnitude, and Co-occurrence
Developing AOPs with recognition of the features of treatments used, such as the duration, magnitude, and co-occurrence of stressor exposure, will allow for more nuanced understanding of anticipated effects. In terms of duration, it would be useful to incorporate information on whether each of the observed outcomes occurred in a matter of hours, days, weeks, or years, potentially developing separate AOPs for each of these timescales. Where information on the longer timescales is available, this could facilitate inclusion of the evolutionary responses to climate change in AOPs (see Munday et al., 2013 and references therein). Long-term responses to stressors spanning several generations are gaining increased interest, which could allow incorporation of the role of epigenetics (Turner, 2009) and carry over effects (Parker et al., 2010). Another important development will be the incorporation of information regarding the relationship between dose dependency and expected outcomes, an issue that has only relatively recently been addressed (e.g., Hooper et al., 2013). The level of the stressor considered may play a key role in the responses observed as biological responses may be characterized by distinct “thresholds” or sensitivities (e.g., May, 1977). Moreover, the combination of stressor exposure duration and magnitude will be important to consider; exposure to shorter and stronger treatments may lead to considerably different responses than longer and weaker exposures (Kroeker et al., 2010, 2019). Finally, environmental stressors will occur in combination, and this could modify their effects. For instance, the effects of ocean acidification can be altered where this condition interacts with higher temperature (Leung et al., 2020b) and salinity (Przeslawski et al., 2015) to affect organisms, including those of particular concern, such as oysters (Thiyagarajan and Ko, 2012; Ginger et al., 2014). The effect can also be dependent on other factors, such as life history stage considered, with acidification and temperature found to have positive effects on larval growth but lead to an increase in overall mortality (Parker et al., 2017). Stressor identity is also likely to be influential. When acidification is combined with increased salinity, it has negative effects on attachment and metamorphosis of developing oysters, but such effects were not observed when combined with elevated temperature (Thiyagarajan and Ko, 2012; Ginger et al., 2014). Consequently, an important role of AOPs will be to combine independent AOPs created for each stressor to identify common effects overlapping across separate pathways. In this way, AOPs may be used to better understand the complexity of responses including the duration magnitude and synergy of stressors.
Multiple Species and Their Interactions
The AOPs developed for individual species could be assembled together to form large networks that depict the impacts of stressors on whole ecosystems. Where these links are made, it could allow for species interactions to be incorporated into studies applying the AOP framework. For instance, such a network approach could allow representation of the impacts that result from the degradation of shallow-water oyster reefs to reduce biodiversity and eventually result in profound shifts in composition from a calcareous-dominated system to one dominated by non-calcareous species (Christen et al., 2012; Rossoll et al., 2012). Where such networks are created for a range of sites characterized by similar ecosystems, this could allow improved understanding of the similarities or differences in responses to ocean acidification and the underlying mechanisms. In particular, comparisons of AOPs developed for species and communities in naturally acidified conditions such as volcanic seeps, areas of upwelling, lagoons, and estuaries may be of particular interest for predicting the impacts of future ocean conditions (see studies by Tunnicliffe et al., 2009; Thomsen et al., 2013; Basso et al., 2015).
Conclusions
Here, we highlight how the AOP framework can be applied to consider the effects of a climate stressor, specifically ocean acidification, on a process of interest in a key organism, specifically oyster calcification. This application highlights that such an approach can effectively bring together key findings from existing literature to demonstrate how responses may manifest. Looking forward, the AOP framework may provide a novel approach to consider mechanisms by which ocean acidification, and other climate drivers, have an effect and alter organism function, ecosystem structure, and benefits provided to human societies.
Data Availability Statement
The raw data supporting the conclusions of this article will be made available by the authors, without undue reservation.
Author Contributions
JD and LF contributed to the conception and design of this meta-analysis, AOP, manuscript, and manuscript revision. JD performed the meta-analysis, did the statistical analysis, created the AOP, and produced the figures with guidance from LF. JD prepared the first draft of the manuscript. All authors contributed to the article and approved the submitted version.
Conflict of Interest
The authors declare that the research was conducted in the absence of any commercial or financial relationships that could be construed as a potential conflict of interest.
Acknowledgments
We would like to thank Jodie Kei Yu Ip and Cindy B. Tong for their assistance in reviewing papers for the meta-analysis.
References
Ankley, G. T., Bennett, R. S., Erickson, R. J., Hoff, D. J., Hornung, M. W., Johnson, R. D., et al. (2010). Adverse outcome pathways: a conceptual framework to support ecotoxicology research and risk assessment. Environ. Toxicol. Chem. 29, 730–741. doi: 10.1002/etc.34
Barry, J. P., Widdicombe, S., and Hall-Spencer, J. M. (2011). “Effects of ocean acidification on marine biodiversity and ecosystem function,” in Ocean Acidification, eds J. P. Gattuso and L. Lansson (Oxford: Oxford University Press), 192–209.
Basso, L., Hendriks, I. E., Rodríguez-Navarro, A. B., Gambi, M. C., and Duarte, C. M. (2015). Extreme pH conditions at a natural CO2 vent system (Italy) affect growth, and survival of juvenile pen shells (Pinna nobilis). Estuaries Coast 38, 1986–1999. doi: 10.1007/s12237-014-9936-9
Burrows, M. T., Schoeman, D. S., Buckley, L. B., Moore, P., Poloczanska, E. S., Brander, K. M., et al. (2011). The pace of shifting climate in marine and terrestrial ecosystems. Science 334, 652–655. doi: 10.1126/science.1210288
Byrne, M., and Fitzer, S. (2019). The impact of environmental acidification on the microstructure and mechanical integrity of marine invertebrate skeletons. Conserv. Physiol. 7:coz062. doi: 10.1093/conphys/coz062
Caldeira, K. (2005). Ocean model predictions of chemistry changes from carbon dioxide emissions to the atmosphere and ocean. J. Geophys. Res. 110:C9. doi: 10.1029/2004JC002671
Caldeira, K., and Wickett, M. E. (2003). Anthropogenic carbon and ocean pH. Nature 425:365. doi: 10.1038/425365a
Calosi, P., Rastrick, S. P., Lombardi, C., Guzman, H. J., Davidson, L., Jahnke, M., et al. (2013). Adaptation and acclimatization to ocean acidification in marine ectotherms: an in situ transplant experiment with polychaetes at a shallow CO2 vent system. Philos. T. R. Soc. B. 368:20120444. doi: 10.1098/rstb.2012.0444
Cao, R., Liu, Y., Wang, Q., Zhang, Q., Yang, D., Liu, H., et al. (2018). The impact of ocean acidification and cadmium on the immune responses of Pacific oyster, Crassostrea gigas. Fish. Shell. Immun. 81, 456–462. doi: 10.1016/j.fsi.2018.07.055
Christen, N., Calosi, P., McNeill, C. L., and Widdicombe, S. (2012). Structural and functional vulnerability to elevated pCO2 in marine benthic communities. Mar. Biol. 160, 2113–2128. doi: 10.1007/s00227-012-2097-0
Clark, M. S., Peck, L. S., Arivalagan, J., Backeljau, T., Berland, S., Cardoso, J. C. R., et al. (2020). Deciphering mollusc shell production: the roles of genetic mechanisms through to ecology, aquaculture and biomimetics. Biol. Rev. doi: 10.1111/brv.12640
Collard, M., Laitat, K., Moulin, L., Catarino, A. I., Grosjean, P., and Dubois, P. (2013). Buffer capacity of the coelomic fluid in echinoderms. Comp. Biochem. Physiol. A Mol. Int. Physiol. 166, 199–206. doi: 10.1016/j.cbpa.2013.06.002
Cyronak, T., Schulz, K. G., and Jokiel, P. L. (2016). The Omega myth: what really drives lower calcification rates in an acidifying ocean. ICES J. Mar. Sci. 73, 558–562. doi: 10.1093/icesjms/fsv075
Dickinson, G. H., Ivanina, A. V., Matoo, O. B., Pörtner, H. O., Lannig, G., Bock, C., et al. (2011). Interactive effects of salinity and elevated CO2 levels on juvenile eastern oysters, Crassostrea virginica. J. Exp. Biol. 215, 29–43. doi: 10.1242/jeb.061481
Dineshram, R., Chandramouli, K., Ko, G. W., Zhang, H., Qian, P., Ravasi, T., and Thiyagarajan, V. (2016). Quantitative analysis of oyster larval proteome provides new insights into the effects of multiple climate change stressors. Glob. Chang. Biol. 22, 2054–2068. doi: 10.1111/gcb.13249
Dineshram, R. Q. Q., Sharma, R., Chandramouli, K., Yalamanchili, H. K., et al. (2015). Comparative and quantitative proteomics reveal the adaptive strategies of oyster larvae to ocean acidification. Proteom 15, 4120–4134. doi: 10.1002/pmic.201500198
Dineshram, R., Wong, K. K., Xiao, S., Yu, Z., Qian, P. Y., and Thiyagarajan, V. (2012). Analysis of Pacific oyster larval proteome and its response to high-CO2. Mar. Pollut. Bull. 64, 2160–2167. doi: 10.1016/j.marpolbul.2012.07.043
Doney, S. C., Fabry, V. J., Feely, R. A., and Kleypas, J. A. (2009). Ocean acidification: the other CO2 problem. Ann. Rev. Mar. Sci. 1, 169–192. doi: 10.1146/annurev.marine.010908.163834
Evans, T. G., Pespeni, M. H., Hofmann, G. E., Palumbi, S. R., and Sanford, E. (2017). Transcriptomic responses to seawater acidification among sea urchin populations inhabiting a natural pH mosaic. Mol. Ecol. Resour. 26, 2257–2275. doi: 10.1111/mec.14038
Falkenberg, L. J., Dupont, S., and Bellerby, R. G. (2018). Approaches to reconsider literature on physiological effects of environmental change: examples from ocean acidification research. Front. Mar. Sci. 5:453. doi: 10.3389/fmars.2018.00453
Falkenberg, L. J., Styan, C. A., and Havenhand, J. N. (2019). Sperm motility of oysters from distinct populations differs in response to ocean acidification and freshening. Sci. Rep. 9:7970. doi: 10.1038/s41598-019-44321-0
FAO (2016). The State of World Fisheries and Aquaculture. Meeting the Sustainable Development Goals. Rome.
Fitzer, S. C., Chan, V. B. S., Meng, Y., Rajan, K. C., Suzuki, M., Not, C., et al. (2019). “Established and emerging techniques for characterizing the formation, structure and performance of calcified structures under ocean acidification,” in Oceanography and Marine Biology: An Annual Review, eds S. J. Hawkins, A. L. Allcock, A. E. Bates, L. B. Firth, I. P. Smith, S. E. Swearer, and P. A. Todd (Boca Raton; London; New York, NY: CRC Press, Taylor & Francis Group), 57, 89–125.
Fitzer, S. C., Chung, P., Maccherozzi, F., Dhesi, S. S., Kamenos, N. A., Phoenix, V. R., and Cusack, M. (2016). Biomineral shell formation under ocean acidification: a shift from order to chaos. Sci. Rep. 6:21076. doi: 10.1038/srep21076
Fitzer, S. C., Zhu, W., Tanner, K. E., Phoenix, V. R., Kamenos, N. A., and Cusack, M. (2015). Ocean acidification alters the material properties of Mytilus edulis shells. J. R. Soc. Interface 12:20141227. doi: 10.1098/rsif.2014.1227
Garilli, V., Rodolfo-Metalpa, R., Scuderi, D., Brusca, L., Parrinello, D., Rastrick, S. P., et al. (2015). Physiological advantages of dwarfing in surviving extinctions in high-CO2 oceans. Nat. Clim. Chang. 5, 678–682. doi: 10.1038/nclimate2616
Gazeau, F., Parker, L. M., Comeau, S., Gattuso, J., O'Connor, W. A., Martin, S., et al. (2013). Impacts of ocean acidification on marine shelled mollusks. Mar. Biol. 160, 2207–2245. doi: 10.1007/s00227-013-2219-3
Ginger, K. W. K., Dineshram, R., Campanati, C., Chan, V. B. S., Havenhand, J., and Thiyagarajan, V. (2014). Interactive effects of ocean acidification, elevated temperature, and reduced salinity on early-life stages of the Pacific oyster. Environ. Sci. Tech. 48, 10079–10088. doi: 10.1021/es501611u
Ginger, K. W. K., Vera, C. B. S., Dennis, C. K. S., Adela, L. J., Yu, Z., Thiyagarajan, V., et al. (2013). Larval and post-larval stages of Pacific oyster (Crassostrea gigas) are resistant to elevated CO2. PLoS. ONE 8:e34147. doi: 10.1371/journal.pone.0064147
Gutowska, M. A., Melzner, F., Langenbuch, M., Bock, C., Claireaux, G., and Pörtner, H. O. (2010). Acid–base regulatory ability of the cephalopod (Sepia officinalis) in response to environmental hypercapnia. J. Comp. Physiol. 180, 323–335. doi: 10.1007/s00360-009-0412-y
Harvey, B., and Moore, P. (2017). Ocean warming and acidification prevent compensatory response in a predator to reduced prey quality. Mar. Ecol. Prog. Ser. 563, 111–122. doi: 10.3354/meps11956
Harvey, B. P., Gwynn-Jones, D., and Moore, P. J. (2013). Meta-analysis reveals complex marine biological responses to the interactive effects of ocean acidification and warming. Ecol. Evol. 3, 1016–1030. doi: 10.1002/ece3.516
Hettinger, A., Sanford, E., Hill, T. M., Lenz, E. A., Russell, A. D., and Gaylord, B. (2013). Larval carry-over effects from ocean acidification persist in the natural environment. Glob. Chang. Biol. 19, 3317–3326. doi: 10.1111/gcb.12307
Hettinger, A., Sanford, E., Hill, T. M., Russell, A. D., Sato, K. N. S., Hoey, J., et al. (2012). Persistent carry-over effects of planktonic exposure to ocean acidification in the Olympia oyster. Ecology 93, 2758–2768. doi: 10.1890/12-0567.1
Hooper, M. J., Ankley, G. T., Cristol, D. A., Maryoung, L. A., Noyes, P. D., and Pinkerton, K. E. (2013). Interactions between chemical and climate stressors: a role for mechanistic toxicology in assessing climate change risks. Environ. Toxicol. Chem. 32, 32–48. doi: 10.1002/etc.2043
IPCC (2013). Climate Change 2013 - The Physical Science Basis: Working Group I Contribution to the Fifth Assessment Report of the IPCC. Cambridge: Cambridge University Press.
Itoh, N., Xue, Q., Schey, K. L., Li, Y., Cooper, R. K., and Peyre, J. F. (2011). Characterization of the major plasma protein of the Eastern Oyster, Crassostrea virginica, and a proposed role in host defense. Comp. Biochem. Physiol. B Biochem. Mol. Biol. 158, 9–22. doi: 10.1016/j.cbpb.2010.06.006
Jaeckle, W. (2017). “Chapter 9: Physiology of Larval Feeding” in Evolutionary Ecology of Marine Invertebrate Larvae (Oxford: Oxford Scholarship Online), 124–141. doi: 10.1093/oso/9780198786962.003.0009
Kraft, N. J. B., Adler, P. B., Godoy, O., James, E. C., Fuller, S., and Levine, J. M. (2014). Community assembly, coexistence and the environmental filtering metaphor. Funct. Ecol. 29, 592–599. doi: 10.1111/1365-2435.12345
Kroeker, K. J., Bell, L. E., Donham, E. M., Hoshijima, U., Lummis, S., Toy, J. A., and Willis-Norton, E. (2019). Ecological change in dynamic environments: accounting for temporal environmental variability in studies of ocean change biology. Glob. Chang. Biol. 26, 54–67. doi: 10.1111/gcb.14868
Kroeker, K. J., Kordas, R. L., Crim, R. N., and Singh, G. G. (2010). Meta-analysis reveals negative yet variable effects of ocean acidification on marine organisms. Ecol. Lett. 13, 1419–1434. doi: 10.1111/j.1461-0248.2010.01518.x
Lannig, G., Eilers, S., Pörtner, H. O., Sokolova, I. M., and Bock, C. (2010). Impact of ocean acidification on energy metabolism of oyster, Crassostrea gigas – changes in metabolic pathways and thermal response. Mar. Drugs 8, 2318–2339. doi: 10.3390/md8082318
Leist, M., Ghallab, A., Graepel, R., Marchan, R., Hassan, R., Bennekou, S. H., et al. (2017). Adverse outcome pathways: opportunities, limitations and open questions. Arch. Toxicol. 91, 3477–3505. doi: 10.1007/s00204-017-2045-3
Lemasson, A. J., Fletcher, S., Hall-Spencer, J. M., and Knights, A. M. (2017). Linking the biological impacts of ocean acidification on oysters to changes in ecosystem services: a review. J. Exp. Mar. Biol. Ecol. 492, 49–62. doi: 10.1016/j.jembe.2017.01.019
Leung, J. Y. S., Chen, Y., Nagelkerken, I., Zhang, S., Xie, Z., and Connell, S. D. (2020a). Calcifiers can adjust shell building at the nanoscale to resist ocean acidification. Small 16:2003186. doi: 10.1002/smll.202003186
Leung, J. Y. S., Doubleday, Z. A., Nagelkerken, I., Chen, Y., Xie, Z., and Connell, S. D. (2019). How calorie-rich food could help marine calcifiers in a CO2-rich future. Proc. R Soc. B 286:20190757. doi: 10.1098/rspb.2019.0757
Leung, J. Y. S., Russell, B. D., and Connell, S. D. (2017). Mineralogical plasticity acts as a compensatory mechanism to the impacts of ocean acidification. Environ. Sci. Technol. 51, 2652–2659. doi: 10.1021/acs.est.6b04709
Leung, J. Y. S., Russell, B. D., and Connell, S. D. (2020b). Linking energy budget to physiological adaptation: how a calcifying gastropod adjusts or succumbs to ocean acidification and warming. Sci. Total. Environ. 715:136939. doi: 10.1016/j.scitotenv.2020.136939
Marshall, D. J., Santos, J. H., Leung, K. M., and Chak, W. H. (2008). Correlations between gastropod shell dissolution and water chemical properties in a tropical estuary. Mar. Environ. Res. 66, 422–429. doi: 10.1016/j.marenvres.2008.07.003
May, R. M. (1977). Thresholds and breakpoints in ecosystems with a multiplicity of stable states. Nature 269, 471–477. doi: 10.1038/269471a0
Meng, Y., Guo, Z., Fitzer, S. C., Upadhyay, A., Chan, V. B. S., Li, C., et al. (2018). Ocean acidification reduces hardness and stiffness of the Portuguese oyster shell with impaired microstructure: a hierarchical analysis. Biogeosciences 15, 6833–6846. doi: 10.5194/bg-15-6833-2018
Michaelidis, B., Ouzounis, C., Paleras, A., and Pörtner, H. (2005). Effects of long-term moderate hypercapnia on acid-base balance and growth rate in marine mussels Mytilus galloprovincialis. Mar. Ecol. Prog. Ser. 293, 109–118. doi: 10.3354/meps293109
Munday, P. L., Warner, R. R., Monro, K., Pandolfi, J. M., and Marshall, D. J. (2013). Predicting evolutionary responses to climate change in the sea. Ecol. Lett. 16, 1488–1500. doi: 10.1111/ele.12185
Nienhuis, S., Palmer, A. R., and Harley, C. D. (2010). Elevated CO2 affects shell dissolution rate but not calcification rate in a marine snail. Proc. R Soc. B 277, 2553–2558. doi: 10.1098/rspb.2010.0206
O'Donnell, M. J., George, M. N., and Carrington, E. (2013). Mussel byssus attachment weakened by ocean acidification. Nat. Clim. Change. 3, 587–590. doi: 10.1038/nclimate1846
Palumbi, S. R., Barshis, D. J., Traylor-Knowles, N., and Bay, R. A. (2014). Mechanisms of reef coral resistance to future climate change. Science 344, 895–898. doi: 10.1126/science.1251336
Parker, L. M., O'Connor, W. A., Byrne, M., Coleman, R. A., Virtue, P., Dove, M., et al. (2017). Adult exposure to ocean acidification is maladaptive for larvae of the Sydney rock oyster Saccostrea glomerata in the presence of multiple stressors. Biol. Lett. 13:20160798. doi: 10.1098/rsbl.2016.0798
Parker, L. M., Ross, P. M., and O'Connor, W. A. (2009). The effect of ocean acidification and temperature on the fertilization and embryonic development of the Sydney rock oyster Saccostrea glomerata (Gould, 1850). Glob. Chang. Biol. 15, 2123–2136. doi: 10.1111/j.1365-2486.2009.01895.x
Parker, L. M., Ross, P. M., and O'Connor, W. A. (2010). Comparing the effect of elevated pCO2 and temperature on the fertilization and early development of two species of oysters. Mar. Biol. 157, 2435–2452. doi: 10.1007/s00227-010-1508-3
Pörtner, H. O., Langenbuch, M., and Reipschläger, A. (2004). Biological impact of elevated ocean CO2 concentrations: lessons from animal physiology and earth history. J. Oceanogr. 60, 705–718. doi: 10.1007/s10872-004-5763-0
Prosser, C. L. (1955). Physiological variation in animals. Biol. Rev. 30, 229–261. doi: 10.1111/j.1469-185X.1955.tb01208.x
Przeslawski, R., Byrne, M., and Mellin, C. (2015). A review and meta-analysis of the effects of multiple abiotic stressors on marine embryos and larvae. Glob. Chang. Biol. 21, 2122–2140. doi: 10.1111/gcb.12833
Queirós, A. M., Fernandes, J. A., Faulwetter, S., Nunes, J., Rastrick, S. P., Mieszkowska, N., et al. (2014). Scaling up experimental ocean acidification and warming research: from individuals to the ecosystem. Glob. Chang. Biol. 21, 130–143. doi: 10.1111/gcb.12675
Rodolfo-Metalpa, R., Houlbrèque, F., Tambutt,é, É., Boisson, F., Baggini, C., Patti, F. P., et al. (2011). Coral and mollusc resistance to ocean acidification adversely affected by warming. Nat. Clim. Chang. 1, 308–312. doi: 10.1038/nclimate1200
Roggatz, C. C., Lorch, M., Hardege, J. D., and Benoit, D. M. (2016). Ocean acidification affects marine chemical communication by changing structure and function of peptide signalling molecules. Glob. Chang. Biol. 22, 3914–3926. doi: 10.1111/gcb.13354
Rossoll, D., Bermúdez, R., Hauss, H., Schulz, K. G., Riebesell, U., Sommer, U., and Winder, M. (2012). Ocean acidification-induced food quality deterioration constrains trophic transfer. PLoS ONE 7:e34737. doi: 10.1371/journal.pone.0034737
Sabine, C. L., Feely, R. A., Gruber, N., Key, R. M., Lee, K., Bullister, J. L., et al. (2004). The oceanic sink for anthropogenic CO2. Science 305, 367–371. doi: 10.1126/science.1097403
Scanes, E., Parker, L. M., O'Connor, W. A., and Ross, P. M. (2014). Mixed effects of elevated pCO2 on fertilisation, larval and juvenile development and adult responses in the mobile subtidal scallop Mimachlamys asperrima (Lamarck, 1819). PLoS ONE 9:93649. doi: 10.1371/journal.pone0093649
Society for Advancement of AOPs (2020). Available online at: https://aopwiki.org/ (accessed October 20, 2019).
Stapp, L. S., Thomsen, J., Schade, H., Bock, C., Melzner, F., Pörtner, H. O., et al. (2016). Intra-population variability of ocean acidification impacts on the physiology of baltic blue mussels (Mytilus edulis): integrating tissue and organism response. J. Comp.Phys. B 187, 529–543. doi: 10.1007/s00360-016-1053-6
Stumpp, M., Wren, J., Melzner, F., Thorndyke, M., and Dupont, S. (2011). CO2 induced seawater acidification impacts sea urchin larval development I: elevated metabolic rates decrease scope for growth and induce developmental delay. Comp. Biochem. Phys. A Mol. Integr. Physiol. 160, 331–340. doi: 10.1016/j.cbpa.2011.06.022
Talmage, S. C., and Gobler, C. J. (2009). The effects of elevated carbon dioxide concentrations on the metamorphosis, size, and survival of larval hard clams (Mercenaria mercenaria), bay scallops (Argopecten irradians), and eastern oysters (Crassostrea virginica). Limnol. Oceangr. 54, 2072–2080. doi: 10.4319/lo.2009.54.6.2072
Telesca, L., Peck, L. S., Sanders, T., Thyrring, J., Sejr, M. K., and Harper, E. M. (2019). Biomineralization plasticity and environmental heterogeneity predict geographical resilience patterns of foundation species to future change. Glob. Change Biol. 25, 4179–4193. doi: 10.1111/gcb.14758
Thiyagarajan, V., and Ko, G. W. K. (2012). Larval growth response of the Portuguese oyster (Crassostrea angulata) to multiple climate change stressors. Aquaculture 370–371, 90–95. doi: 10.1016/j.aquaculture.2012.09.025
Thomsen, J., Casties, I., Pansch, C., Körtzinger, A., and Melzner, F. (2013). Food availability outweighs ocean acidification effects in juvenile Mytilus edulis: laboratory and field experiments. Glob. Chang. Biol. 19, 1017–1027. doi: 10.1111/gcb.12109
Tunnicliffe, V., Davies, K. T., Butterfield, D. A., Embley, R. W., Rose, J. M., and Chadwick, W. W. Jr. (2009). Survival of mussels in extremely acidic waters on a submarine volcano. Nat. Geosci. 2, 344–348. doi: 10.1038/ngeo500
Turner, B. M. (2009). Epigenetic responses to environmental change and their evolutionary implications. Philos. Trans. R Soc. Lond. B Biol. Sci. 364, 3403–3418. doi: 10.1098/rstb.2009.0125
Vihtakari, M., Havenhand, J., Renaud, P. E., and Hendriks, I. E. (2016). Variable individual- and population-level responses to ocean acidification. Front. Mar. Sci. 3:51. doi: 10.3389/fmars.2016.00051
Waldbusser, G. G., Hales, B., Langdon, C. J., Haley, B. A., Schrader, P., Brunner, E. L., et al. (2015). Ocean acidification has multiple modes of action on bivalve larvae. PLoS ONE 10:e128376. doi: 10.1371/journal.pone.0128376
Wang, X., Wang, M., Jia, Z., Qiu, L., Wang, L., Zhang, A., et al. (2017). A carbonic anhydrase serves as an important acid-base regulator in Pacific oyster Crassostrea gigas exposed to elevated CO2: implication for physiological responses of mollusk to ocean acidification. Mar. Biotechnol. 19, 22–35. doi: 10.1007/s10126-017-9734-z
Wei, L., Wang, Q., Wu, H., Ji, C., and Zhao, J. (2015). Proteomic and metabolomic responses of Pacific oyster Crassostrea gigas to elevated pCO2 exposure. J. Proteom. 112, 83–94. doi: 10.1016/j.jprot.2014.08.010
Wieser, W., and Krumschnabel, G. (2001). Hierarchies of ATP-consuming processes: direct compared with indirect measurements, and comparative aspects. Biochem. J. 355:389. doi: 10.1042/bj3550389
Wood, H. L., Spicer, J. I., and Widdicombe, S. (2008). Ocean acidification may increase calcification rates, but at a cost. Proc. R Soc. B 275, 1767–1773. doi: 10.1098/rspb.2008.0343
Zeebe, R. E., and Wolf-Gladrow, D. W. (2001). CO2 in Seawater: Equilibrium, Kinetics, Isotopes. The Netherlands: Elsevier. doi: 10.1016/s0422-9894(01)x8001-x
Keywords: ocean acidification, marine mollusks, marine molluscs, Adverse Outcome Pathway, carbon dioxide, Pacific oyster
Citation: Ducker J and Falkenberg LJ (2020) How the Pacific Oyster Responds to Ocean Acidification: Development and Application of a Meta-Analysis Based Adverse Outcome Pathway. Front. Mar. Sci. 7:597441. doi: 10.3389/fmars.2020.597441
Received: 21 August 2020; Accepted: 05 October 2020;
Published: 12 November 2020.
Edited by:
Jonathan Y. S. Leung, University of Adelaide, AustraliaReviewed by:
Qing Wang, Chinese Academy of Sciences (CAS), ChinaVengatesen Thiyagarajan, The University of Hong Kong, Hong Kong
Copyright © 2020 Ducker and Falkenberg. This is an open-access article distributed under the terms of the Creative Commons Attribution License (CC BY). The use, distribution or reproduction in other forums is permitted, provided the original author(s) and the copyright owner(s) are credited and that the original publication in this journal is cited, in accordance with accepted academic practice. No use, distribution or reproduction is permitted which does not comply with these terms.
*Correspondence: Laura J. Falkenberg, bGF1cmFmYWxrZW5iZXJnQGN1aGsuZWR1Lmhr