- 1Strathclyde Institute of Pharmacy and Biomedical Sciences, University of Strathclyde, Glasgow, United Kingdom
- 2Sea Mammal Research Unit, Scottish Oceans Institute, University of St Andrews, St Andrews, United Kingdom
- 3Institute of Biodiversity, Animal Health & Comparative Medicine, College of Medical, Veterinary and Life Sciences, University of Glasgow, Glasgow, United Kingdom
Mothers of the Atlantic grey seal, Halichoerus grypus, lactate for about 20 days, during which they do not feed and may have no access to water. Following weaning, they depart to sea leaving their pups unattended and unfed for up to another 40 days. We are interested in how this lactation strategy supports the pups’ rapid growth and development while also preparing them for their long fast before independently going to sea. We report a broad spectrum metabolomic analysis of whole milks of these seals that reveals continuous changes in key metabolites from birth to weaning. Certain components exhibit abbreviated appearances at the onset of lactation, followed by continuous rises or falls in others until weaning. Riboflavin/Vitamin B2, hormone-related sterol sulfates, lactose, and complex oligosaccharides all appear in milk briefly after birth then disappear. Lipids associated with cellular signaling and brain development occur at highest levels shortly after birth, then diminish. In contrast, other lipids and Vitamin B6/pyridoxine steadily increase as weaning approaches. Overall, these findings may indicate an early transition from carbohydrate to fat-based energy metabolism and establishment of gut microbiomes in pups, followed by provisioning for post-weaning development and fasting.
Introduction
Milks are a critical source of nutrition and passive immune protection for mammalian neonates. Milks of eutherian (“placental”) mammals change dramatically in composition during the immediate post-partum period and then usually resolve into stable, “mature” phases (Langer, 2009). The nature of these changes reflects the type of placenta that a species exhibits and the developmental stage of offspring at birth (Langer, 2008, 2009; Wooding and Burton, 2008). Eutherian neonates vary from altricial (relatively undeveloped, may be blind, deaf, hairless, immobile, and unable to thermoregulate) to precocious (well-developed, mobile, sense organs fully functional, fully haired) (Schulz and Bowen, 2004).
Oceanic marine mammals produce precocial neonates that are invested with large fat reserves that provide insulation against hypothermia when birthed in water, on land in exposed coastal environments, or on ice (Berta et al., 2015a). Those that birth on land or ice are the pinnipeds (sea lions and eared seals; Ottariidae), the walruses (Odobenidae), and the true, “earless” seals (Phocidae). The true seals present a particularly extreme lactation strategy among all mammal groups in that they have the shortest lactation periods in proportion to maternal body mass, ranging from 5 to 7 weeks in Weddell seals to a mere 3–5 days in hooded seals (Schulz and Bowen, 2004), the only real outlier to this pattern being Mediterranean monk seals that nurse for about 18 week (Pastor and Aguilar, 2003; Aguilar et al., 2007). In about half of seal species, the mother remains with her pup and does not feed before abruptly ending lactation and returning to sea, leaving the weaned pup to fend for itself without any period of mixed feeding (Schulz and Bowen, 2004). These seals are therefore capital breeders whose brief, intense lactations are thought to have evolved in response to exploitation of transient pack ice for pup rearing, reduction in total energy costs of hunting and feeding vs. lactation, and predation (Schulz and Bowen, 2004, 2005; Berta et al., 2015b).
Mobilization of fat reserves in lactating seals is remarkable in the rate and scale of the loss in body mass exhibited by the mothers during nursing—for example, Atlantic grey seal, Halichoerus grypus, mothers lose 39% of their initial mass during lactation (Pomeroy et al., 1999). The rate of gain in their offspring is equally dramatic—in hooded seals, for example, a 24 kg pup at birth doubles its body weight in 4 days (Lydersen and Kovacs, 1999). Atlantic grey seals come ashore, give birth, nurse for about 15–20 days during which they do not feed, rarely if ever drink, then leave their pups abruptly and return to sea (Schulz and Bowen, 2004). The pups then stay on land for up to about 40 days before they have matured sufficiently to go to sea (Bennett et al., 2010; Hanson et al., 2019). So, not only must mothers provide for the dramatic weight gain of their pups, but must also supply them with sufficient resources to survive the post-weaning extra period of development without further nutritional intake. Moreover, the pups need also to retain sufficient blubber to fund energy metabolism and maintain euthermia while they learn to fish at sea. Energy resource management of an Atlantic grey seal mother, therefore, exhibits a fine balance between the success of her pup against her own survival.
In Atlantic grey seals we previously reported that the change in milk from colostrum to mature phase lactation occurs faster than has been reported for any other group of mammals (Lowe et al., 2017). The rapidity of the effect was clearly apparent for proteins, but there were indications that the small molecule metabolome changed more slowly and that there were indicators of metabolic strain as the mothers’ fasts progressed toward weaning (Lowe et al., 2017). We now report a broad spectrum metabolomic study of grey seal milk revealing continuous changes from birth to weaning, punctuated by abrupt changes in the presence of some vitamins and other essential nutrients during the first few days after birth. There is therefore, no period that may be described as a stable, mature phase of lactation. Some of these changes may indicate an early switch from carbohydrate to fat metabolism in pups, and provisioning of pups beyond their immediate needs (“stockpiling”) for their post-weaning fast (Watson et al., 2020). These new findings emphasize the dramatic adaptations, strains, and refinements in the evolution of lactation by Atlantic grey and other true seals as capital breeding, hypercarnivorous mammals that have moved from land to cold and frozen aquatic environments.
Materials and Methods
Milk Collection, Storage, and Processing
Samples were collected from 21 mother-pup pairs in the Atlantic grey seal breeding colony on the Isle of May, Scotland (56.1°N, 2.55°W), in October and November 2013. Regular surveys of the colony identified known animals and their pupping dates as part of a wider demographic study (Pomeroy et al., 2000). Five of these mothers provided samples on four occasions between giving birth and just before the mothers were expected to wean pups and return to sea. The earliest samplings were during day 2 post-partum to allow mother-pup bonds to form, with subsequent samplings on days 7 and 13, followed by days 17, 18, or 19 (for ease of presentation, samples taken 17, 18, or 19 days after birth are presented as day 18 in graphs). Females were chemically immobilized with a mass-adjusted dose of ®Zoletil 100 (Virbac, Bury St Edmunds, Suffolk, United Kingdom). The bodyweight of the animal to be immobilized was estimated, and the dose of drug delivered using a pressurized projectile syringe delivered by a blowpipe to the posterior lumbar muscles, all as described (Langton et al., 2011). The darted animals were kept under observation from a distance for any difficulties for 10 min before approach (Langton et al., 2011). Oxytocin was administered intravenously to stimulate milk let-down and an intramuscular prophylactic dose of tetracycline was given, as reported previously (Watson et al., 2020). Milks were frozen at -20°C and stored at -20 to -25°C until aliquoted for analysis. Whole milk samples were used here, without removal of the fat layer, unlike in our previous report in which milk had been cold-centrifuged and the fat layer discarded (Lowe et al., 2017).
Metabolomics
Ammonium carbonate, high performance liquid chromatography (HPLC) grade acetonitrile, and methanol were purchased from Sigma-Aldrich, United Kingdom. HPLC grade water was produced by a Direct-Q 3 Ultrapure Water System from Millipore, United Kingdom. The milk samples were analyzed by mixing 200 μl of the whole milk with 800 μl of isopropanol. The solution was mixed thoroughly and the emulsion centrifuged for 10 min at 15,000 rpm at 4°C (Eppendorf 5424 R, maximum RCF = 21.130 g). The supernatant was transferred to an HPLC vial for liquid chromatography-mass spectrometry (LC-MS) analysis.
Hydrophilic interaction liquid chromatography- high resolution mass spectrometry (HILIC–HRMS) and multiple tandem HRMS analysis and data processing was carried out on an Accela 600 HPLC system combined with an Exactive (Orbitrap) mass spectrometer (Thermo Fisher Scientific, United Kingdom). An aliquot of each sample solution (10 μL) was injected onto a ZIC-pHILIC column (150 × 4.6 mm, 5 μm; HiChrom, Reading, United Kingdom) with mobile phase A: 20 mM ammonium carbonate in HPLC grade water (pH 9.2), and B: HPLC grade acetonitrile. The gradient program was as follows: 80% B (0 min) 20% B (30 min) 5% B (36 min) 80% B (37 min) 80% B (45 min). Peak extraction and alignment were carried out by using MZMine 2.14 software, as previously described (Lowe et al., 2017). Metabolites were identified by searching against an in-house metabolite database in house data base drawn from the Human Metabolome Database1, KEGG2, and Lipid Maps3. The data was transferred to Excel and P-values and peak area ratios for the identified metabolites were determined. In addition, retention times of metabolites were matched against those of standard mixtures of 200 metabolites run at the same time as the samples (Howe et al., 2018). Otherwise metabolite matches were based on accurate masses <3 ppm and identified to MSI levels 1, where the retention time matched that of a standard or to level 2 (Sumner et al., 2007). Further analysis of the extracted data was carried out by using Metaboanalyst 4.0 to obtain ANOVA with Tukey honestly significant difference (HSD) test and multivariate principal components analysis (PCA) plots (Chong et al., 2019). The raw metabolomics data and the statistical analysis of these are available in Supplementary Materials B, C, respectively.
Results and Discussion
Continuous Changes in Grey Seal Milk Metabolome With Time After Birth
We first screened whole milk samples for overall changes in metabolites from soon after parturition to near weaning in five Atlantic grey seals. Figure 1 shows a PCA analysis indicating that, while the overall composition is relatively stable, there was a progressive change over successive sampling times that culminated in a separation between the beginning and end phases of lactation. Also apparent was the individuality of the mothers, which could be due to a combination of different parities, indeterminacies in establishing precise times of parturition under field conditions, recent suckling histories, and intrinsic diversity among mothers in the composition of milks.
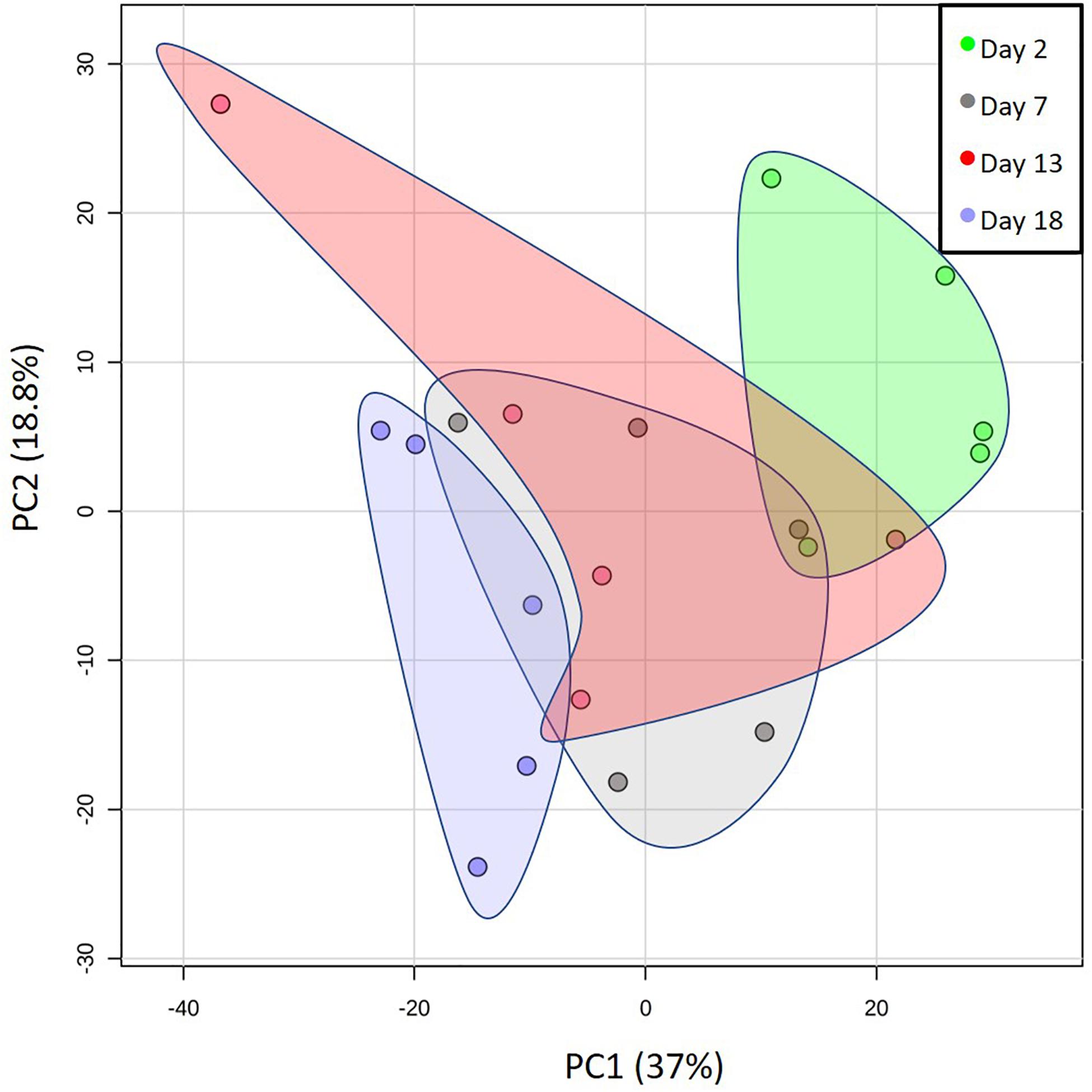
Figure 1. Changes in the metabolomes of the milks of five mother grey seals with time after parturition. Principal component (PC) analysis plot showing the overall changes in the milk metabolome from shortly after the birth of pups to weaning. The days indicated are the estimated times after birth observed in the wild. Here, and for all subsequent graphs, the data spots labeled for day 18 came from days 17, 18, or 19 of an approximately 20-day lactation. Each spot represents the metabolomes of a single mother grey seal. The shaded areas group the PC values for the five mother seals sampled per day. The % values indicate the % of the variation in the data explained by the two components.
The sections below focus on selected metabolite groups that exhibited statistically significant differences in abundance with time (see Supplementary C), or that show other potentially informative changes, as lactation proceeded from parturition to weaning.
Lactose and Oligosaccharides
Saccharides in mammalian milks have four main functions—fuel for energy metabolism, osmotic regulation of water content, anti-microbial activities, and to establish and consolidate a gut microbiome appropriate for a milk diet in the neonate (Zivkovic et al., 2011; Oftedal et al., 2014; James et al., 2019). Oligosaccharides can comprise the main carbohydrates in milks, and, excepting lactose, they may not be digested or absorbed for energy metabolism (Gnoth et al., 2000; German et al., 2008; Zivkovic et al., 2011). Some have anti-microbial activities, such as through competitive inhibition of carbohydrate-binding (lectin) adhesion molecules that prevent the adherence of pathogenic microbes to gut mucus and epithelial cells (Peterson et al., 2013; ten Bruggencate et al., 2014; Hoeflinger et al., 2015; Maldonado-Gomez et al., 2015; Pacheco et al., 2015; Kim et al., 2019). Some milk oligosaccharides are metabolized by gut microorganisms, such as Bifidobacterium spp., that are enriched in the gut of naturally nursed animals and humans (Zivkovic et al., 2011; Musilova et al., 2014; James et al., 2019).
We found lactose in milk only on Day 2 after the onset of lactation in grey seals, and by Day 5 it was undetectable (Figure 2). Whether it would be higher immediately after birth before our first samples were collected is worthy of investigation. Lactose is the primary sugar for energy metabolism in the milks of many species of mammals, but not in pinnipeds. Otariids and odobenids appear to have lost (though phocids have retained) functional genes encoding α-lactalbumin that is necessary for enhanced production of lactose (Reich and Arnould, 2007). This loss has been hypothesized to be an advantage to marine mammals because lactose is involved in the osmotic drawing of water into milk—close regulation of physiologic water balance without persistent lactose-based osmosis might be advantageous to marine animals, especially during long lactations that are interrupted while mothers leave their pups to forage at sea (Schulz and Bowen, 2004; Sharp et al., 2007). Also, the milks of marine mammals are so fat-rich that energy supplies are not dependent on lactose.
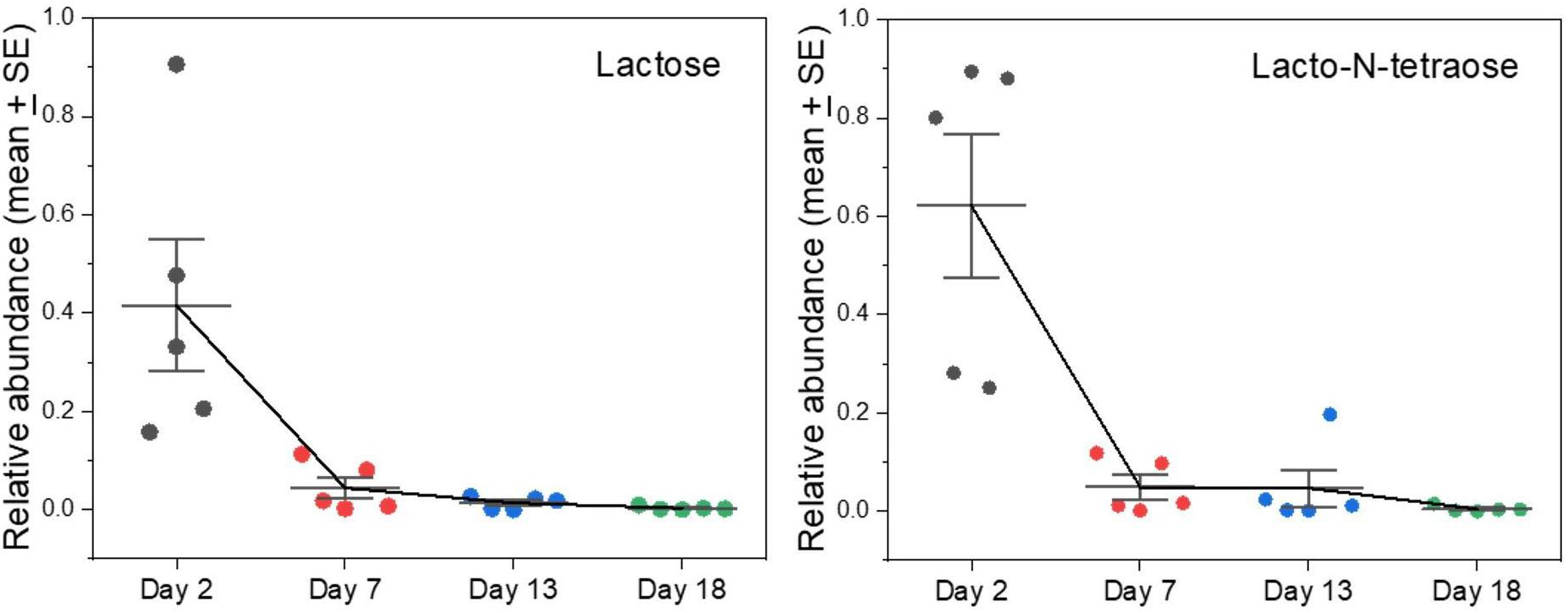
Figure 2. Changes in levels of lactose and oligosaccharides of grey seal mothers’ milks with time after parturition. The abundances are expressed in arbitrary units adjusted to a maximum of approximately 1. Error bar center lines are at the mean, and the whiskers are the standard errors of the mean. See Supplementary A Figure S2 for similar changes observed in other complex oligosaccharides found. All of these and other graphs are reproduced in Supplementary A Figure S2 with values from cow and goat milks added for comparison included.
Our observations suggest the possibility that lactose temporarily supplies energy at the start of lactation during the switch-over from glucose-supported energy metabolism in utero to lipid-dependent energy metabolism in the developing pup. This early supply of lactose may be to ensure a supply of glucose for essential functions such as brain activity, which is totally dependent of glucose (Vannucci and Vannucci, 2000; Steiner, 2020), particularly for phocid neonates that have unusually large brains (Eisert et al., 2014). Alternatively, it may obviate a need for wasteful gluconeogenesis from proteins and fats until the neonatal digestive system matures sufficiently to cope with fat-rich milk (Eisert et al., 2013). There may, for instance, be a time lag to await sufficient production of bile acid detergents, and secretion of fat-digesting enzymes such as bile salt-activated lipase can be delayed in neonates (Lombardo, 2001; Lindquist and Hernell, 2010). This still begs the question of how otariid and odobenid neonates manage without a lactose-rich transitional milk.
Phocid pups are totally reliant on milk for their water, yet the mothers of species that remain on land to nurse have limited or no access to water. The water content of phocid milks can be highest immediately after birth (Eisert et al., 2013; Zhang et al., 2014), so osmotic dilution of milk may result in immediate post-birth hydration of pups before maternal water supply becomes limited or zero, and a degree of dehydration has been observed in fasting grey seal pups (Nordoy et al., 1992). Otariids and odobenids, in contrast, forage at sea during lactation so the problem of water supply does not present itself (Berta et al., 2015b).
In addition to its support for energy metabolism, lactose can also be a precursor for oligosaccharides that are non-nutritive but control potentially pathogenic microbes or nourish beneficial ones (Thurl et al., 2010; Jantscher-Krenn et al., 2012; Yu et al., 2013). Lacto-N-tetraose, for example, is a tetrasaccharide that can be abundant in human milk and is known to differ in concentration between mothers in relation to parity and as nursing progresses (Jantscher-Krenn et al., 2012; Hoeflinger et al., 2015). It and related oligosaccharides are known to confer relative resistance against certain gut bacterial pathogens (Thurl et al., 2010; Jantscher-Krenn et al., 2012). As with lactose, lacto-N-tetraose was present in day 2 seal milk samples and then virtually disappeared (Figure 2). We previously noted the presence and then rapid loss of defensive oligosaccharides such as sialyllactose and fucosyllactose in grey seal milk (Lowe et al., 2017), and we now find that this pattern is a common theme for several different species of complex saccharides (Supplementary A Figure S2). If these sugars are important for control and regulation of the gut microbiome in pups, then their rapid disappearance from milk is puzzling unless it relates to a change in microbiome that becomes adapted to a fat- rather than a sugar-based energy metabolism, or that the absence of sugars limits colonization of inappropriate microbes.
Not only do the milks of mammals exhibit a high diversity of oligosaccharide types (Urashima et al., 2000, 2009, 2013; Messer and Urashima, 2002; Fischer-Tlustos et al., 2020; Weinborn et al., 2020), but they can also be highly polymorphic between individuals within the same species. This is particularly true of humans, in which there are many oligosaccharide types present (Gabrielli et al., 2011; Urashima et al., 2012; ten Bruggencate et al., 2014), which can differ dramatically between individual women (Newburg et al., 2004; Zivkovic et al., 2011). Moreover, this variability between mothers can influence the susceptibility of infants to intestinal bacterial infections (Newburg, 1999; Newburg et al., 2002; Ruiz-Palacios et al., 2003; Morrow et al., 2004; Hoeflinger et al., 2015; Pacheco et al., 2015; James et al., 2019; Kim et al., 2019). If this diversity were also true in seals then it would contribute to the range of abundances observed between individual mothers (Figure 2 and Supplementary A Figure S2). Remarkably, not only do several types of oligosaccharide appear only briefly in the seal milks, but most of them are not found in cow or goat milks (see below and Supplementary A Figure S2).
Vitamins and Other Key Nutrients
Several metabolites that cannot be synthesized by mammals and therefore must derive from the mothers’ diets before they fast were found to have opposing time courses in grey seal milk. This applies notably to riboflavin (vitamin B2) and pyridoxine (vitamin B6). Riboflavin appeared in samples taken soon after birth but then fell rapidly to barely detectable levels (Figure 3). Flavins and their derivatives have a wide range of biochemical activities as enzyme cofactors, notably in the citric acid cycle and the electron transport chain. It may be no coincidence, therefore, that riboflavin’s presence in grey seal milk was contemporaneous with lactose, again consistent with early dependence by pups on carbohydrate metabolism before a change to reliance on lipids for energy metabolism and glucose supply. Riboflavin is a component in FAD which is an essential in the terminal respiratory chain accepting a proton from NADH. Thus, FAD is linked to metabolism of both carbohydrates and fatty acids although not directly involved in β-oxidation of fatty acids as is NAD. The early pulse of riboflavin after birth may, therefore, allow sufficient incorporation of flavins into these systems such that any additional supply need only be minimal. Ingestion of excess riboflavin usually results in its excretion, although fasting has been found in rats and humans to lead to an increase in its excretion for a short time before a decline (Windmueller et al., 1964; Fukuwatari et al., 2010; Moriya et al., 2012, 2013). If this also occurs in mother seals then the appearance of riboflavin in their milk shortly after the onset of fasting and birth could be the result of release of this water-soluble B vitamin into their circulation and leakage into their milk.
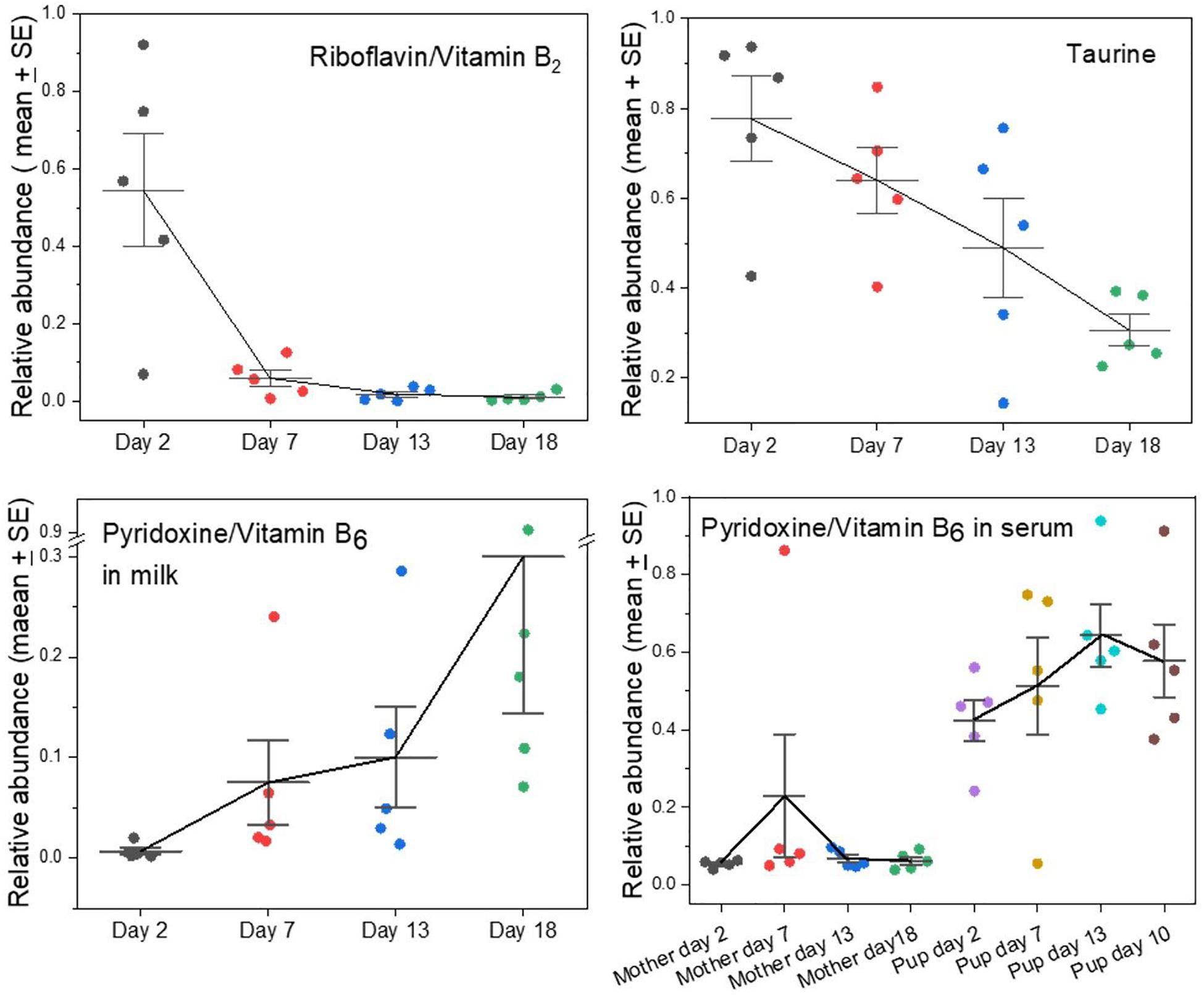
Figure 3. Changes in the levels of key nutrients and vitamins in the milks of grey seal mothers with time after birth. The fall in taurine levels has been reported before (Lowe et al., 2017) but is clearer here in which whole, unseparated milk was analyzed. For pyridoxine, the levels in both milk (note the scale break) and mother and pup serum are given; for serum data see ref. Watson et al. (2020). See Supplementary A Figure S3 for comparison with cow and goat milks.
Pyridoxine/Vitamin B6 is also an essential nutrient and has the opposite time course to riboflavin’s. Its levels were relatively low in early milks and rose steadily toward the termination of lactation (Figure 3). It is required for a wide range of synthetic processes such as for amino acids, carbohydrates, lipids, and heme, the pups’ need for all of which may arise later during growth and development. We had previously found that the serum of seal pups accumulates higher concentrations of pyridoxine than are present in their mothers, leading to the suggestion that pups accumulate a stockpile of this metabolite to satisfy a dependency on it during their subsequent long fast (Figure 3; Watson et al., 2020).
We had found before that levels of taurine decline steadily with time after birth in grey seal milk (Lowe et al., 2017), and this is even clearer in the current analysis (Figure 3). Carnivory provides a plentiful and continuous supply of taurine, which is known or suspected to be an essential dietary nutrient in some hypercarnivores (Hedberg et al., 2007, 2011; Chesney et al., 2009). Inability to synthesize taurine would explain its decrease in grey seal milk level as maternal stores are depleted during the lactational fast.
Signaling and Other Lipids
Certain polyunsaturated fatty acids are essential dietary components because they cannot be synthesized by mammals. Examples include the plant-derived alpha-linolenic acid (an Ω-3 fatty acid) and linoleic acid (an Ω-6 fatty acid) that are precursors of developmentally and physiologically important lipids that carnivores would obtain from their prey. A case in point is docosahexaenoic acid (DHA), an Ω-3 fatty acid that is a crucial component of membranes of the brain, nervous, and visual systems (Singh, 2005; Carlson et al., 2013; Gharami et al., 2015; Lauritzen et al., 2016). DHA can be synthesized to some degree from another essential fatty acid, eicosapentaenoic acid (EPA) that is itself an important dietary constituent and a precursor for pharmacologically active lipids such as prostaglandins, leukotrienes, and thromboxanes (Das, 2006; Farooqui et al., 2007; Hadley et al., 2016). Levels of DHA rose steadily in grey seal milk during lactation (Figure 4), potentially to facilitate development of the nervous systems of pups and may represent stockpiling in preparation for weaning, and may also support the stratification of blubber layers associated with thermoregulation (Tverin et al., 2019). Curiously, however, EPA does not exhibit a similar rise to DHA, but is present at relatively consistent levels throughout (Figure 4).
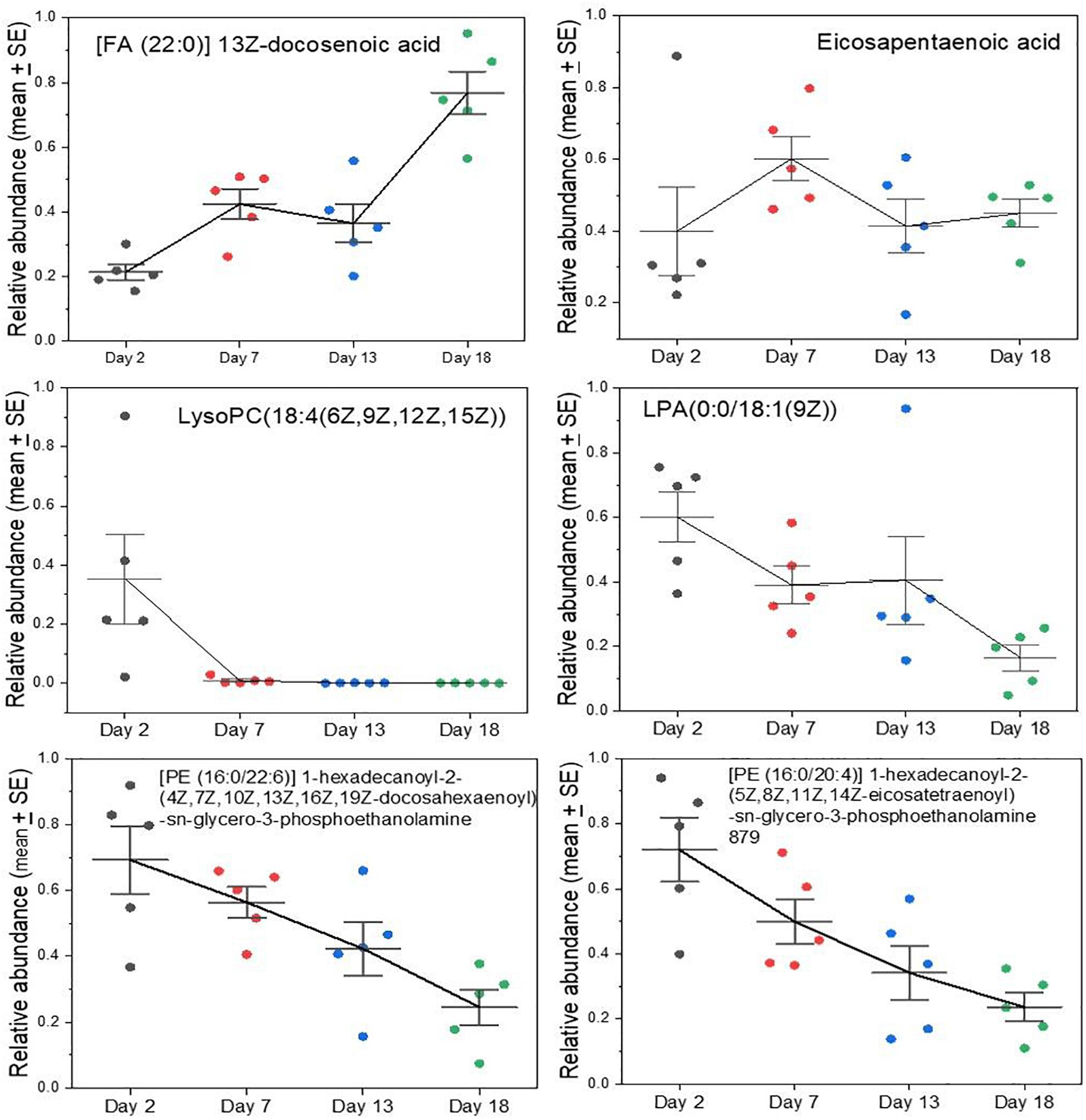
Figure 4. Changes in key lipids in grey seal milk with time after birth. Some of these lipids (DHA and EHA) are derived from dietary essential lipids in mammals. Lyso lipids can be involved in cellular signaling, and all those with polyunsaturated tails may be important in the development of the brain and visual systems. See Supplementary A Figure S4 for other examples and comparisons with cow and goat milks for all the lipids examined here.
Lysolipids, such as lysophosphatidlycholine (LysoPC) and lysophospatidylethanolamine (LysoPE) have a wide range of functions relevant to cellular signaling, inflammation and development (Makide et al., 2009, 2014; Frasch and Bratton, 2012; Grzelczyk and Gendaszewska-Darmach, 2013; Barnes et al., 2015; Kamat et al., 2015; Nishikawa et al., 2015) and appear in highest relative concentrations in early seal milk, but then gradually fall (Figure 4). The same is true of more complex diglycerides with polyunsaturated tails, such as the two shown in Figure 4. If the rise in DHA reflects developmental needs, such as for the brain and nervous system, then it is difficult to explain that these lysolipids and other complex lipids decrease—precipitously in the case of the lysoPC shown in Figure 4.
The total lipid and fat content of grey seal milk is known to rise with time after birth (Lydersen and Kovacs, 1999; Arriola et al., 2013). Our analysis shows that this rise comprises a complex dynamic of changes in lipid types that are presumably relevant to progressive changes in the metabolism and developmental needs of the pups both during lactation and thereafter.
Sterols
Cholesterol sulfate is yet another major component that appeared in early milks and then virtually disappeared (Figure 5). It is the most important sterol sulfate in human plasma, and is involved in a wide range of processes such as stabilizing cell membranes, protecting erythrocytes from osmotic lysis, involvement in platelet adhesion, and in regulating a range of enzymes involved in clotting (Strott and Higashi, 2003). It has further emerged as having important regulatory effects on protein kinase and cellular differentiation processes (Strott and Higashi, 2003; Hanyu et al., 2012; Neunzig and Bernhardt, 2018). In humans it is increased modestly in maternal circulation during pregnancy and is synthesized in the placenta (Strott and Higashi, 2003). The placenta of humans is different from those of Carnivora (Wooding and Burton, 2008), perhaps including a difference in synthetic activities or permeabilities to maternal supply. The amount of cholesterol sulfate in human plasma is similar to that of dehydroepiandrosterone sulfate (syn. 3β-hydroxyandrost-5-en-17-one 3-sulfate; DHEA-S), which we also find in seal milk only in early samples (Supplementary A Figure S5).
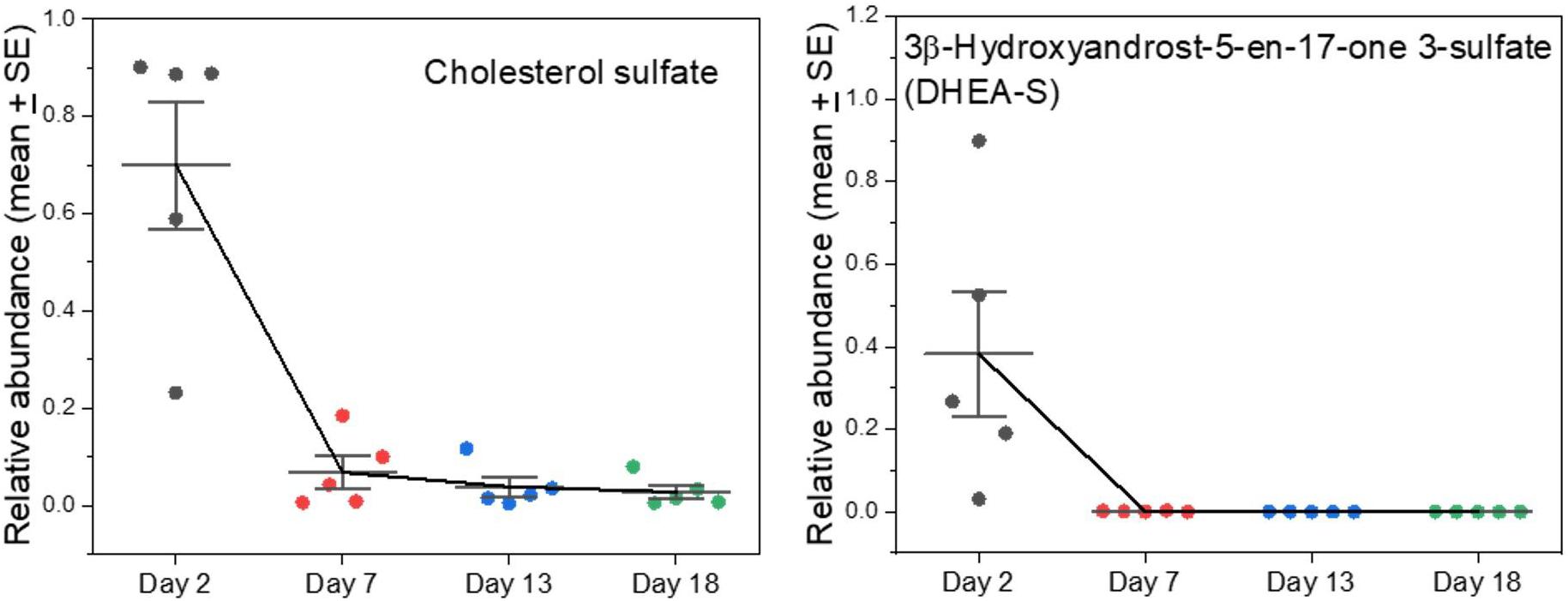
Figure 5. Changes in sulfated sterols in grey seal milk with time after birth. 3β-hydroxyandrost-5-en-17-one 3-sulfate (DHEA-S) is also known as dehydroepiandrosterone. See Supplementary A Figure S5 for more examples of sulfated sterols in the milks, all with high values soon after birth that fall dramatically thereafter, and comparisons with cow and goat milks.
Sulfated steroids do not bind to steroid receptors but can act as reservoirs and desulfated as necessary (Sanchez-Guijo et al., 2016). Reversible sulfation occurs primarily in the adrenals, the liver, and small intestine (Strott and Higashi, 2003; Sanchez-Guijo et al., 2016). Orally ingested DHEA is converted into its sulfate form when passing through the intestines and liver, and the adrenals also carry out the conversion (Tashiro et al., 2000; Neunzig and Bernhardt, 2018). It is the precursor of androstenedione, which can undergo further conversion to produce the androgen testosterone and the estrogens estrone and estradiol (Idkowiak et al., 2016). Serum DHEA sulfate is also a marker for adrenarche, and subsequently for the individual hormonal milieu in humans (Idkowiak et al., 2016). We found other sulfated sterols with similar time courses to cholesterol sulfate and DHEA in the seal milks, though their functions are not known (Supplementary A Figure S5). Some sulfated steroids are proposed to be active neurosteroids and could be involved in brain remodeling/programming which could be pertinent to rapid changes after birth (Strott and Higashi, 2003; Sanchez-Guijo et al., 2016).
Indicators of Metabolic Stress and Change
We had noted previously that there are metabolites in grey seal milk that indicate changes, or even strains, in the metabolism of mothers (Lowe et al., 2017). This is now affirmed by the analysis of whole milks in which levels of nicotinamide and N1-methyl-2-pyridone-5-carboxamide are shown to increase with time until the end of lactation, while O-acetylcarnitine decreases (Supplementary A Figure S6), as does another acylated carnitine, tetradecanoylcarnitine (Figure 6). The carnitines serve to transport fatty acids into mitochondria to undergo β-oxidation, and they remove fatty acids from mitochondria to maintain a balance of free coenzyme A. The carnitines may leak into milk from the maternal circulation, and their progressive decline might reflect a decrease in blubber fat mobilization in the mothers. Interestingly, however, the fall of O-acetylcarnitine in milk occurred at the same time as its rise in mothers’ sera, while the levels in their pups’ sera are low and relatively consistent (Figure 6).
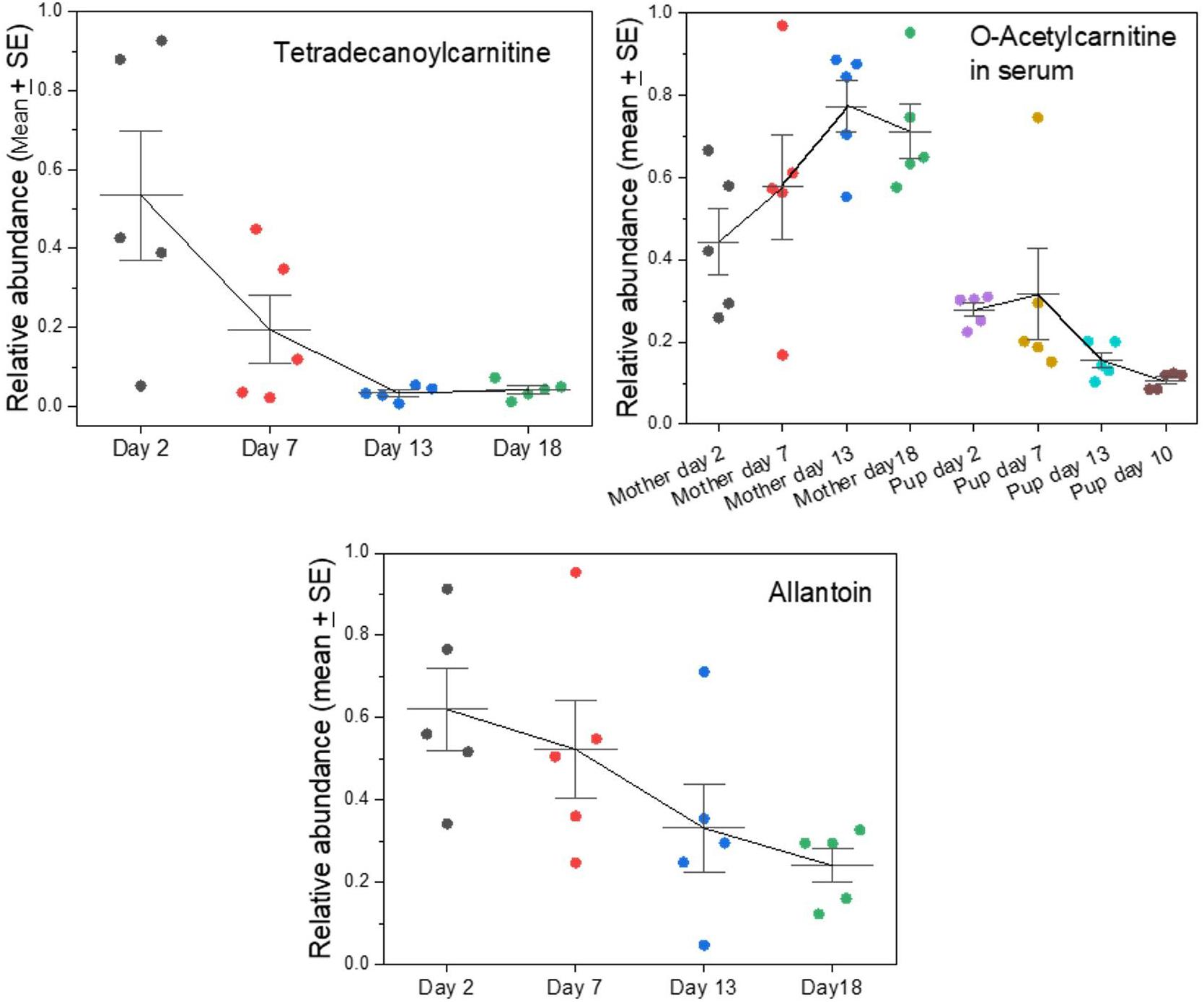
Figure 6. Changes in key indicators of maternal fat and purine metabolism appearing in grey seal milk with time after birth. See Supplementary A Figure S6 for further metabolism-related metabolites and comparison with cow and goat milks. For the data on serum levels of O-acetylcarnitine, in mothers and their pups please see Watson et al. (2020).
One metabolite that is almost certainly a waste product that leaks into milk is allantoin, which is the uricase/urate oxidase-mediated product from uric acid, which is in turn a product of purine metabolism. In the grey seal milks, allantoin decreased with time, potentially indicating a decrease in the mothers’ purine metabolism or enhancement of purine recycling such as is known in fasting post-weaning elephant seal pups (Sonanez-Organis et al., 2012).
Comparison of Phocine Lactation With That of Land Animals With Precocial Neonates and No Maternal Fasting
Lactation in true seals is distinctive in its brevity, extraordinarily fat-rich milks, and maternal anorexia. But what other features of seal milks exist may distinguish them among mammals and that may tell us more about how their milks reflect their adaptations to their particular reproductive strategy? To begin to address this question, we compared our grey seal milk samples with milks from two species of land mammal (cow and goat) whose neonates are also precocial and similar to grey seals in relative body mass at birth. Cows and goats have lower-fat milks, long lactations, and no maternal fasting. We analyzed samples from commercially produced cow and goat milk (during the mature lactation phase) in parallel with the seal milk samples. The PCA comparison in Figure 7 illustrates the dramatic differences between these milks relative to the seals’. A pilot analysis that included camel milk revealed patterns closely similar to those of cows and goats (not shown). Supplementary A reproduces all of the graphs of individual metabolites given in Figures 2–6 here and includes values for cow and goat milk.
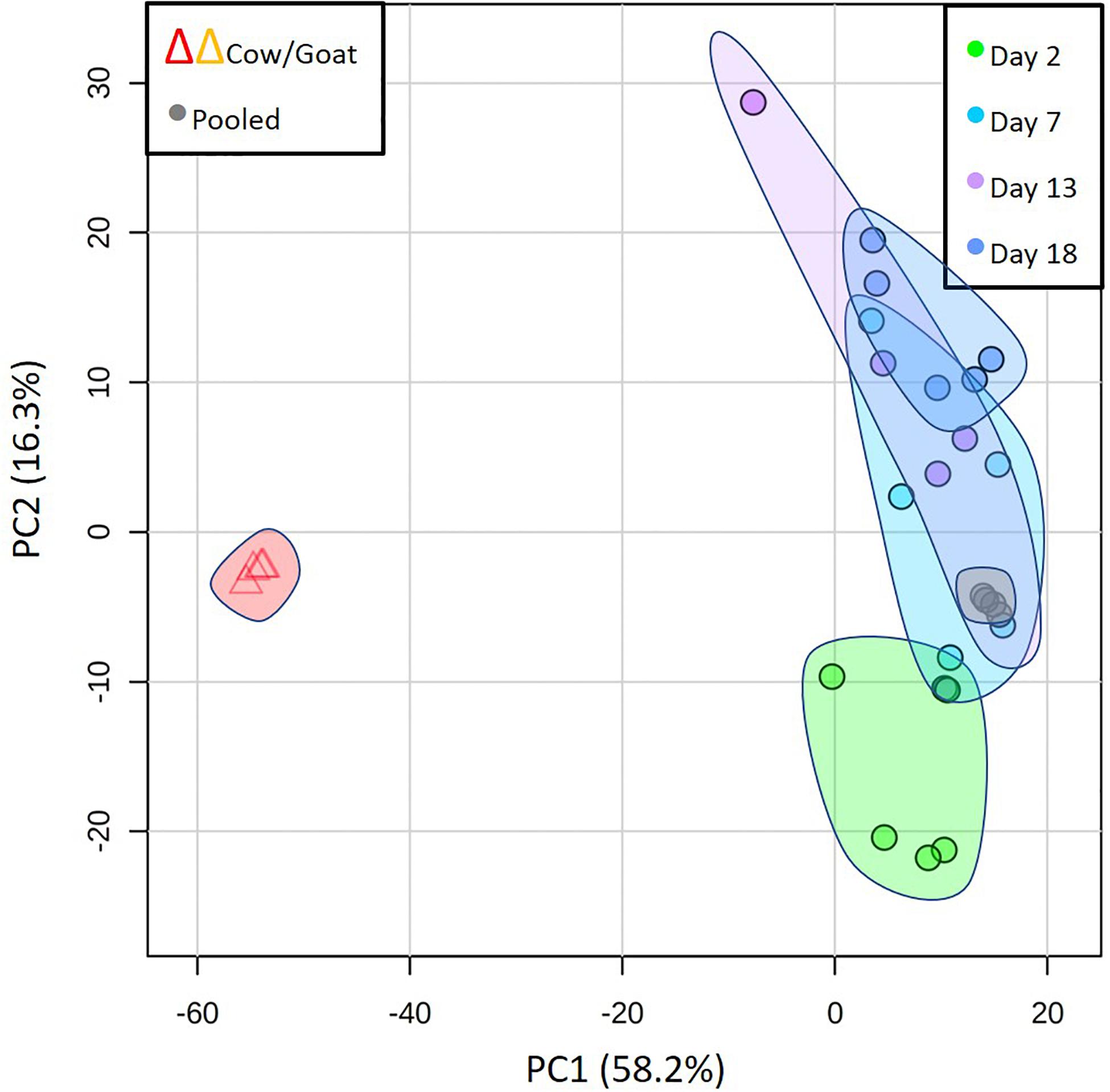
Figure 7. Principal component analysis to compare the overall metabolome of seal milks with time after parturition with cow and goat milk, emphasizing the dramatic differences between the milks of seals and those land mammals. Also included here are the results of repeated analysis of pooled seal milk samples that are routinely included to check on instrument consistency (“Pooled”).
Cow and goat milks are substantially richer in lactose than grey seal milk, and the oligosaccharides present in seal milk were either absent or differed in quantity between the two land mammals (Supplementary A Figure S2). In the case of riboflavin, cholesterol sulfate, and taurine, cow and goat milks have low or undetectable levels, although, as with the seals, this may be time-dependent. Pyridoxine, which rises dramatically in seal milk as weaning approaches, was not detected at all in cow and goat milk (Supplementary A Figure S3). The polyunsaturated fatty acids, lysolipids, and more complex lipids found in seal milk were essentially absent in cow and goat milks (Supplementary A Figure S4), as were sulfated steroids (Supplementary A Figure S5). Finally, the compounds that are mechanistically associated with fat mobilization, namely nicotinamide and N1-methyl-2-pyridone-5-carboxamide, were at very low relative levels in the cow and goat milks, though carnitines and allantoin were at similar levels (Supplementary A Figure S6).
Conclusion
There are clear, continuous, sometimes rapid, changes in the small molecule components in Atlantic grey seal milk from onset to cessation of lactation. The initial rapid changes end sometime between 2 and 5 days postpartum and there is no subsequent period that could be described as stable or consistent. The distinctiveness of the early phase might indicate currently unsuspected changes in the digestion and metabolism of pups. After that there is a continuum of modifications that may indicate changes in the developmental needs of pups as well as their need to build reserves for the post-weaning requirements. At the same time, these progressive changes reflect a depletion of maternal reserves and the metabolic stress of lactation without intake of food or water. The indicators of this are metabolites that are involved in mobilization and catabolism of fat reserves as the mothers move from surplus to deficit. The indicative metabolites include cofactors that may be involved in blubber fat metabolism and mobilization that escape into the mothers’ circulations and adventitiously leak into milk, or they may derive from the lipid-handling cells of the mammary gland. Alternatively, the mothers may be donating these cofactors to their offspring to assist the pups’ lipid-based energy metabolisms and accumulation of fat reserves before imminent weaning. That thought also arose from our findings on how metabolites change in concentration in the serum of mothers and pups with time (Watson et al., 2020). If so, then one would expect it not to occur, or to occur to a lesser extent, in those species of seal, otariids, and walrus that forage during lactation. It may, however, apply to those cetaceans in which the females migrate to calving grounds where they give birth and begin to nurse without feeding (Pitman et al., 2020).
Within the eutherian Order Carnivora, the Infraorder Arctoidea (canids, ursids, pinnipeds, mustelids) comprises species group whose members differ dramatically in the degree of development of their offspring at birth and how their milks change through lactation. It is clear that, in terms of altriciality vs. precocity, and changes in lactation with time after birth, bears and true seals, respectively, represent unrivaled extremes (Loeffler et al., 2006; Griffiths et al., 2015). The dramatic differences between bears and seals exist despite their relatively close phylogenetic relatedness and likely similarities in placental structure (Elliot and Crespi, 2009; Diessler et al., 2015). The differences are unlikely, therefore, to lay in absolute limitations in placental form and function, but rather in their contrasting reproductive strategies and lifestyles.
Data Availability Statement
The original contributions presented in the study are included in the article/Supplementary Material, further inquiries can be directed to the corresponding author/s.
Ethics Statement
Work involving animals in this study was licensed under the UK Home Office project 60/4009 or preceding versions and conformed to the UK Animals (Scientific Procedures) Act, 1986. Research was approved by the University of St Andrews Animal Welfare and Ethics Committee.
Author Contributions
MK, PP, and DW conceived and developed the project and wrote the manuscript. DW carried out the analyses. DW and MK analyzed the data and created the figures. All authors contributed to the article and approved the submitted version.
Funding
The work was funded from core support given to the Sea Mammal Research Unit, Scottish Oceans Institute, from the National Environmental Research Council (United Kingdom), and separately by the Universities of Glasgow and Strathclyde. The funding of mass spectrometry equipment for metabolomics was provided by the Scottish Life Sciences Alliance.
Conflict of Interest
The authors declare that the research was conducted in the absence of any commercial or financial relationships that could be construed as a potential conflict of interest.
Acknowledgments
We are grateful to all involved in fieldwork, particularly Simon Moss and to Scottish National Heritage for permitting our time and sampling on the Isle of May. Thanks also go to Neil Evans for advice on hormone-related sterols, to Sami Bawazeer for expertly carrying out pilot analyses, and also to Kati Loeffler for constructive comments on a draft.
Supplementary Material
The Supplementary Material for this article can be found online at: https://www.frontiersin.org/articles/10.3389/fmars.2020.596904/full#supplementary-material
Supplementary Data Sheet 1 | Supplementary A, including Figures S1–S6.
Supplementary Data Sheet 2 | Supplementary B, containing the raw metabolomics data.
Supplementary Data Sheet 3 | Supplementary C, containing the statistical analysis of the raw metabolomics data.
Abbreviations
ANOVA with HSD, One-way analysis of variance with post-hoc Tukey’s honestly significant difference test; DHEA, dehydroepiandrosterone; FA, fatty acid; FAD, flavin adenine dinucleotide; FMN, flavin mononucleotide; GC or LC, gas or liquid chromatography; HCA, Hierarchical Cluster Analysis; HILIC-HMRS, Hydrophilic Interaction Liquid Chromatography-High Resolution Mass Spectrometry; LPE or lysoPE, lysophosphatidylethanolamine; LPC or lysoPC, lysophosphatidylcholine; MS, mass spectrometry; NAD, nicotinamide adenine dinucleotide; OPLS-DA, Orthogonal Partial Least Squares Discriminant Analysis; PCA, multivariate principal components analysis.
Footnotes
References
Aguilar, A., Cappozzo, L. H., Gazo, M., Pastor, T., Forcada, J., and Grau, E. (2007). Lactation and mother-pup behaviour in the Mediterranean monk seal Monachus monachus: an unusual pattern for a phocid. J. Mar. Biol. Assoc. U. K. 87, 93–99. doi: 10.1017/s0025315407056147
Arriola, A., Biuw, M., Walton, M., Moss, S., and Pomeroy, P. (2013). Selective blubber fatty acid mobilization in lactating gray seals (Halichoerus grypus). Physiol. Biochem. Zool. 86, 441–450. doi: 10.1086/671446
Barnes, M. J., Li, C.-M., Xu, Y., An, J., Huang, Y., and Cyster, J. G. (2015). The lysophosphatidylserine receptor GPR174 constrains regulatory T cell development and function. J. Exp. Med. 212, 1011–1020. doi: 10.1084/jem.20141827
Bennett, K. A., McConnell, B. J., Moss, S. E. W., Speakman, J. R., Pomeroy, P. P., and Fedak, M. A. (2010). Effects of age and body mass on development of diving capabilities of gray seal pups: costs and benefits of the postweaning fast. Physiol. Biochem. Zool. 83, 911–923. doi: 10.1086/656925
Berta, A., Sumich, J. L., and Kovacs, K. M. (2015a). “Energetics,” in Marine Mammals: Evolutionary Biology, 3rd Edn, eds A. Berta, J. L. Sumich, and K. M. Kovacs (Amsterdam: Academic Press), 269–297.
Berta, A., Sumich, J. L., and Kovacs, K. M. (2015b). “Reproductive structures, strategies, and patterns,” in Marine Mammals: Evolutionary Biology, 3rd Edn, eds A. Berta, J. L. Sumich, and K. M. Kovacs (Amsterdam: Academic Press), 465–532. doi: 10.1016/b978-0-12-397002-2.00013-2
Carlson, S. J., Fallon, E. M., Kalish, B. T., Gura, K. M., and Puder, M. (2013). The role of the omega-3 fatty acid DHA in the human life cycle. J. Parenter. Enteral Nutr. 37, 15–22. doi: 10.1177/0148607112467821
Chesney, R. W., Hedberg, G. E., Rogers, Q. R., Dierenfeld, E. S., Hollis, B. E., Derocher, A., et al. (2009). “Does taurine deficiency cause metabolic bone disease and rickets in polar bear cubs raised in captivity?,” in Taurine 7 Advances in Experimental Medicine and Biology, Vol. 643, eds J. Azuma, S. W. Schaffer, and T. Ito (New York, NY: Springer), 325–331. doi: 10.1007/978-0-387-75681-3_33
Chong, J., Wishart, D. S., and Xia, J. (2019). Using metaboanalyst 4.0 for comprehensive and integrative metabolomics data analysis. Curr. Protoc. Bioinform. 68:e86.
Das, U. N. (2006). Essential fatty acids – a review. Curr. Pharm. Biotechnol. 7, 467–482. doi: 10.2174/138920106779116856
Diessler, M. E., Migliorisi, L., Marquez, M. E. I., Negrete, J., Mennucci, J. A., Zanuzzi, C. N., et al. (2015). Microscopic features of the placenta of Mirounga leonina (Carnivora, Pinnipedia, Phocidae). Placenta 36, 514–515. doi: 10.1016/j.placenta.2015.01.534
Eisert, R., Oftedal, O. T., and Barrell, G. K. (2013). Milk composition in the weddell seal Leptonychotes weddellii: evidence for a functional role of milk carbohydrates in pinnipeds. Physiol. Biochem. Zool. 86, 159–175. doi: 10.1086/669036
Eisert, R., Potter, C. W., and Oftedal, O. T. (2014). Brain size in neonatal and adult weddell seals: costs and consequences of having a large brain. Mar. Mamm. Sci. 30, 184–205. doi: 10.1111/mms.12033
Elliot, M. G., and Crespi, B. J. (2009). Phylogenetic evidence for early hemochorial placentation in Eutheria. Placenta 30, 949–967. doi: 10.1016/j.placenta.2009.08.004
Farooqui, A. A., Horrocks, L. A., and Farooqui, T. (2007). Modulation of inflammation in brain: a matter of fat. J. Neurochem. 101, 577–599. doi: 10.1111/j.1471-4159.2006.04371.x
Fischer-Tlustos, A. J., Hertogs, K., van Niekerk, J. K., Nagorske, M., Haines, D. M., and Steele, M. A. (2020). Oligosaccharide concentrations in colostrum, transition milk, and mature milk of primi- and multiparous Holstein cows during the first week of lactation. J. Dairy Sci. 103, 3683–3695. doi: 10.3168/jds.2019-17357
Frasch, S. C., and Bratton, D. L. (2012). Emerging roles for lysophosphatidylserine in resolution of inflammation. Prog. Lipid Res. 51, 199–207. doi: 10.1016/j.plipres.2012.03.001
Fukuwatari, T., Yoshida, E., Takahashi, K., and Shibata, K. (2010). Effect of fasting on the urinary excretion of water-soluble vitamins in humans and rats. J. Nutr. Sci. Vitaminol. 56, 19–26. doi: 10.3177/jnsv.56.19
Gabrielli, O., Zampini, L., Galeazzi, T., Padella, L., Santoro, L., Peila, C., et al. (2011). Preterm milk oligosaccharides during the first month of lactation. Pediatrics 128, E1520–E1531. doi: 10.1542/peds.2011-1206
German, J. B., Freeman, S. L., Lebrilla, C. B., and Mills, D. A. (2008). Human milk oligosaccharides: evolution, structures and bioselectivity as substrates for intestinal bacteria. Nestle Nutr. Works. Ser. Pediatr. Program. 62, 205–222. doi: 10.1159/000146322
Gharami, K., Das, M., and Das, S. (2015). Essential role of docosahexaenoic acid towards development of a smarter brain. Neurochem. Int. 89, 51–62. doi: 10.1016/j.neuint.2015.08.014
Gnoth, M. J., Kunz, C., Kinne-Saffran, E., and Rudloff, S. (2000). Human milk oligosaccharides are minimally digested in vitro. J. Nutr. 130, 3014–3020. doi: 10.1093/jn/130.12.3014
Griffiths, K., Hou, R., Wang, H., Zhang, Z., Zhang, L., Zhang, T., et al. (2015). Prolonged transition time between colostrum and mature milk in a bear, the giant panda, Ailuropoda melanoleuca. R. Soc. Open Sci. 2:150395. doi: 10.1098/rsos.150395
Grzelczyk, A., and Gendaszewska-Darmach, E. (2013). Novel bioactive glycerol-based lysophospholipids: new data – new insight into their function. Biochimie 95, 667–679. doi: 10.1016/j.biochi.2012.10.009
Hadley, K. B., Ryan, A. S., Forsyth, S., Gautier, S., and Salem, N. (2016). The essentiality of arachidonic acid in infant development. Nutrients 8:216. doi: 10.3390/nu8040216
Hanson, N., Smout, S., Moss, S., and Pomeroy, P. (2019). Colony-specific differences in decadal longitudinal body composition of a capital-breeding marine top predator. Aquat. Conserv. Mar. Freshwater Ecosyst. 29, 131–143. doi: 10.1002/aqc.3093
Hanyu, O., Nakae, H., Miida, T., Higashi, Y., Fuda, H., Endo, M., et al. (2012). Cholesterol sulfate induces expression of the skin barrier protein filaggrin in normal human epidermal keratinocytes through induction of ROR alpha. Biochem. Biophys. Res. Commun. 428, 99–104. doi: 10.1016/j.bbrc.2012.10.013
Hedberg, G. E., Derocher, A. E., Andersen, M., Rogers, Q. R., DePeters, E. J., Loennerdal, B., et al. (2011). Milk composition in free-ranging polar bears (Ursus maritimus) as a model for captive rearing milk formula. Zoo Biol. 30, 550–565. doi: 10.1002/zoo.20375
Hedberg, G. E., Dierenfeld, E. S., and Rogers, Q. R. (2007). Taurine and zoo felids: considerations of dietary and biological tissue concentrations. Zoo Biol. 26, 517–531. doi: 10.1002/zoo.20158
Hoeflinger, J. L., Davis, S. R., Chow, J., and Miller, M. J. (2015). In vitro impact of human milk oligosaccharides on Enterobacteriaceae growth. J. Agric. Food Chem. 63, 3295–3302. doi: 10.1021/jf505721p
Howe, C. C. F., Alshehri, A., Muggeridge, D., Mullen, A. B., Boyd, M., Spendiff, O., et al. (2018). Untargeted metabolomics profiling of an 80.5 km simulated treadmill ultramarathon. Metabolites 8:14. doi: 10.3390/metabo8010014
Idkowiak, J., Taylor, A. E., Subtil, S., O’Neil, D. M., Vijzelaar, R., Dias, R. P., et al. (2016). Steroid sulfatase deficiency and androgen activation before and after puberty. J. Clin. Endocrinol. Metabol. 101, 2545–2553. doi: 10.1210/jc.2015-4101
James, K., Bottacini, F., Contreras, J. I. S., Vigoureux, M., Egan, M., Motherway, M. O., et al. (2019). Metabolism of the predominant human milk oligosaccharide fucosyllactose by an infant gut commensal. Sci. Rep. 9:15427. doi: 10.1038/s41598-019-51901-7
Jantscher-Krenn, E., Lauwaet, T., Bliss, L. A., Reed, S. L., Gillin, F. D., and Bode, L. (2012). Human milk oligosaccharides reduce Entamoeba histolytica attachment and cytotoxicity in vitro. Br. J. Nutr. 108, 1839–1846. doi: 10.1017/s0007114511007392
Kamat, S. S., Camara, K., Parsons, W. H., Chen, D.-H., Dix, M. M., Bird, T. D., et al. (2015). Immunomodulatory lysophosphatidylserines are regulated by ABHD16A and ABHD12 interplay. Nat. Chem. Biol. 11, 164–U116. doi: 10.1038/nchembio.1721
Kim, J., Kim, Y. J., and Kim, J. W. (2019). Bacterial clearance is enhanced by alpha 2,3-and alpha 2,6-sialyllactose via receptor-mediated endocytosis and phagocytosis. Infect. Immun. 87:e00694-18. doi: 10.1128/iai.00694-18
Langer, P. (2008). The phases of maternal investment in eutherian mammals. Zoology 111, 148–162. doi: 10.1016/j.zool.2007.06.007
Langer, P. (2009). Differences in the composition of colostrum and milk in eutherians reflect differences in immunoglobulin transfer. J. Mammal 90, 332–339. doi: 10.1644/08-mamm-a-071.1
Langton, S. D., Moss, S. E., Pomeroy, P. P., and Borer, K. E. (2011). Effect of induction dose, lactation stage and body condition on tiletamine-zolazepam anaesthesia in adult female grey seals (Halichoerus grypus) under field conditions. Vet. Rec. 168:457. doi: 10.1136/vr.d1047
Lauritzen, L., Brambilla, P., Mazzocchi, A., Harslof, L. B. S., Ciappolino, V., and Agostoni, C. (2016). DHA effects in brain development and function. Nutrients 8:6. doi: 10.3390/nu8010006
Lindquist, S., and Hernell, O. (2010). Lipid digestion and absorption in early life: an update. Curr. Opin. Clin. Nutr. Metab. Care 13, 314–320. doi: 10.1097/MCO.0b013e328337bbf0
Loeffler, I. K., Montali, R. J., and Rideout, B. A. (2006). “Diseases and pathology of giant pandas,” in Giant Pandas: Biology, Veterinary Medicine and Management, eds D. E. Wildt, A. Zhang, H. Zhang, D. L. Janssen, and S. Ellis (Cambridge: Cambridge University Press), 377–409. doi: 10.1017/cbo9780511542244.017
Lombardo, D. (2001). Bile salt-dependent lipase: its pathophysiological implications. Biochim. Biophys. Acta Mol. Cell Biol. Lipids 1533, 1–28. doi: 10.1016/s1388-1981(01)00130-5
Lowe, A. D., Bawazeer, S., Watson, D. G., McGill, S., Burchmore, R. J. S., Pomeroy, P. P., et al. (2017). Rapid changes in Atlantic grey seal milk from birth to weaning – immune factors and indicators of metabolic strain. Sci. Rep. 7:16093. doi: 10.1038/s41598-017-16187-7
Lydersen, C., and Kovacs, K. M. (1999). Behaviour and energetics of ice-breeding, North Atlantic phocid seals during the lactation period. Mar. Ecol. Prog. Ser. 187, 265–281. doi: 10.3354/meps187265
Makide, K., Kitamura, H., Sato, Y., Okutani, M., and Aoki, J. (2009). Emerging lysophospholipid mediators, lysophosphatidylserine, lysophosphatidylthreonine, lysophosphatidylethanolamine and lysophosphatidylglycerol. Prostaglandins Other Lipid Mediat. 89, 135–139. doi: 10.1016/j.prostaglandins.2009.04.009
Makide, K., Uwamizu, A., Shinjo, Y., Ishiguro, J., Okutani, M., Inoue, A., et al. (2014). Novel lysophosphoplipid receptors: their structure and function. J. Lipid Res. 55, 1986–1995. doi: 10.1194/jlr.R046920
Maldonado-Gomez, M. X., Lee, H., Barile, D., Lu, M., and Hutkins, R. W. (2015). Adherence inhibition of enteric pathogens to epithelial cells by bovine colostrum fractions. Int. Dairy J. 40, 24–32. doi: 10.1016/j.idairyj.2014.08.014
Messer, M., and Urashima, T. (2002). Evolution of milk oligosacharides and lactose. Trends Glycosci. Glycotechnol. 14, 153–176. doi: 10.4052/tigg.14.153
Moriya, A., Fukuwatari, T., Sano, M., and Shibata, K. (2012). Different variations of tissue B-group vitamin concentrations in short- and long-term starved rats. Br. J. Nutr. 107, 52–60. doi: 10.1017/s0007114511002339
Moriya, A., Fukuwatari, T., and Shibata, K. (2013). Excess vitamin intake before starvation does not affect body mass, organ mass, or blood variables but affects urinary excretion of riboflavin in starving rats. Nutr. Metab. Insights 6, 23–28. doi: 10.4137/nmi.S12060
Morrow, A. L., Ruiz-Palacios, G. M., Altaye, M., Jiang, X., Guerrero, M. L., Meinzen-Derr, J. K., et al. (2004). Human milk oligosaccharides are associated with protection against diarrhea in breast-fed infants. J. Pediatr. 145, 297–303. doi: 10.1016/j.jpeds.2004.04.054
Musilova, S., Rada, V., Vlkova, E., and Bunesova, V. (2014). Beneficial effects of human milk oligosaccharides on gut microbiota. Benef. Microbes 5, 273–283. doi: 10.3920/bm2013.0080
Neunzig, J., and Bernhardt, R. (2018). Effect of sulfonated steroids on steroidogenic cytochrome P450-dependent steroid hydroxylases. J. Steroid Biochem. Mol. Biol. 179, 3–7. doi: 10.1016/j.jsbmb.2017.07.004
Newburg, D. S. (1999). Human milk glycoconjugates that inhibit pathogens. Curr. Med. Chem. 6, 117–127.
Newburg, D. S., Chaturvedi, P., Ruiz-Palacios, G. M., Altaye, M., Guerrero, M. D., Meinzen-Derr, J. K., et al. (2002). High ratios of alpha 1,2-linked to alpha 1,3/4-linked fucosyloligosaccharides in human milk are associated with protection of breast-fed infants against diarrhea. Faseb J. 16:A608.
Newburg, D. S., Ruiz-Palacios, G. M., Altaye, M., Chaturvedi, P., Meinzen-Derr, J., Guerrero, M. D., et al. (2004). Innate protection conferred by fucosylated oligosaccharides of human milk against diarrhea in breastfed infants. Glycobiology 14, 253–263. doi: 10.1093/glycob/cwh020
Nishikawa, M., Kurano, M., Ikeda, H., Aoki, J., and Yatomi, Y. (2015). Lysophosphatidylserine has bilateral effects on macrophages in the pathogenesis of atherosclerosis. J. Atheroscler. Thromb. 22, 518–526. doi: 10.5551/jat.25650
Nordoy, E. S., Stijfhoorn, D. E., Raheim, A., and Blix, A. S. (1992). Water flux and early signs of entrance into Phase-III of fasting in gray seal pups. Acta Physiol. Scand. 144, 477–482. doi: 10.1111/j.1748-1716.1992.tb09324.x
Oftedal, O. T., Nicol, S. C., Davies, N. W., Sekii, N., Taufik, E., Fukuda, K., et al. (2014). Can an ancestral condition for milk oligosaccharides be determined? Evidence from the Tasmanian echidna (Tachyglossus aculeatus setosus). Glycobiology 24, 826–839. doi: 10.1093/glycob/cwu041
Pacheco, A. R., Barile, D., Underwood, M. A., and Mills, D. A. (2015). The Impact of the milk glycobiome on the neonate gut microbiota. Annu. Rev. Anim. Biosci. 3, 419–445. doi: 10.1146/annurev-animal-022114-111112
Pastor, T., and Aguilar, A. (2003). Reproductive cycle of the female Mediterranean monk seal in the western Sahara. Mar. Mammal Sci. 19, 318–330. doi: 10.1111/j.1748-7692.2003.tb01111.x
Peterson, R., Cheah, W. Y., Grinyer, J., and Packer, N. (2013). Glycoconjugates in human milk: protecting infants from disease. Glycobiology 23, 1425–1438. doi: 10.1093/glycob/cwt072
Pitman, R. L., Durban, J. W., Joyce, T., Fearnbach, H., Panigada, S., and Lauriano, G. (2020). Skin in the game: epidermal molt as a driver of long-distance migration in whales. Mar. Mammal Sci. 36, 565–594. doi: 10.1111/mms.12661
Pomeroy, P. P., Fedak, M. A., Rothery, P., and Anderson, S. (1999). Consequences of maternal size for reproductive expenditure and pupping success of grey seals at North Rona, Scotland. J. Anim. Ecol. 68, 235–253. doi: 10.1046/j.1365-2656.1999.00281.x
Pomeroy, P. P., Twiss, S. D., and Duck, C. D. (2000). Expansion of a grey seal (Halichoerus grypus) breeding colony: changes in pupping site use at the Isle of May, Scotland. J. Zool. 250, 1–12. doi: 10.1111/j.1469-7998.2000.tb00573.x
Reich, C. M., and Arnould, J. P. Y. (2007). Evolution of Pinnipedia lactation strategies: a potential role for alpha-lactalbumin? Biol. Lett. 3, 546–549. doi: 10.1098/rsbl.2007.0265
Ruiz-Palacios, G. M., Cervantes, L. E., Ramos, P., Chavez-Munguia, B., and Newburg, D. S. (2003). Campylobacter jejuni binds intestinal H(O) antigen (Fuc alpha 1, 2Gal beta 1, 4GlcNAc), and fucosyloligosaccharides of human milk inhibit its binding and infection. J. Biol. Chem. 278, 14112–14120. doi: 10.1074/jbc.M207744200
Sanchez-Guijo, A., Neunzig, J., Gerber, A., Oji, V., Hartmann, M. F., Schuppe, H. C., et al. (2016). Role of steroid sulfatase in steroid homeostasis and characterization the sulfated steroid pathway: evidence from steroid sulfatase deficiency. Mol. Cell. Endocrinol. 437, 142–153. doi: 10.1016/j.mce.2016.08.019
Schulz, T. M., and Bowen, W. D. (2004). Pinniped lactation strategies: evaluation of data on maternal and offspring life history traits. Mar. Mammal Sci. 20, 86–114. doi: 10.1111/j.1748-7692.2004.tb01142.x
Schulz, T. M., and Bowen, W. D. (2005). The evolution of lactation strategies in pinnipeds: a phylogenetic analysis. Ecol. Monogr. 75, 159–177. doi: 10.1890/04-0319
Sharp, J. A., Lefevre, C., Brennan, A. J., and Nicholas, K. R. (2007). The fur seal – a model lactation phenotype to explore molecular factors involved in the initiation of apoptosis at involution. J. Mammary Gland Biol. Neoplasia 12, 47–58. doi: 10.1007/s10911-007-9037-5
Singh, M. (2005). Essential fatty acids, DHA and human brain. Indian J. Pediatr. 72, 239–242. doi: 10.1007/bf02859265
Sonanez-Organis, J. G., Vazquez-Medina, J. P., Zenteno-Savin, T., Aguilar, A., Crocker, D. E., and Ortiz, R. M. (2012). Prolonged fasting increases purine recycling in post-weaned northern elephant seals. J. Exp. Biol. 215, 1448–1455. doi: 10.1242/jeb.067173
Steiner, P. (2020). Brain fuel utilization in the developing brain. Ann. Nutr. Metab. 75 (Suppl. 1), 8–18. doi: 10.1159/000508054
Strott, C. A., and Higashi, Y. (2003). Cholesterol sulfate in human physiology: what’s it all about? J. Lipid Res. 44, 1268–1278. doi: 10.1194/jlr.R300005-JLR200
Sumner, L. W., Amberg, A., Barrett, D., Beale, M. H., Beger, R., Daykin, C. A., et al. (2007). Proposed minimum reporting standards for chemical analysis. Metabolomics 3, 211–221. doi: 10.1007/s11306-007-0082-2
Tashiro, A., Sasano, H., Nishikawa, T., Yabuki, N., Muramatsu, Y., Coughtrie, M. W. H., et al. (2000). Expression and activity of dehydroepiandrosterone sulfotransferase in human gastric mucosa. J. Steroid Biochem. Mol. Biol. 72, 149–154. doi: 10.1016/s0960-0760(00)00020-0
ten Bruggencate, S. J. M., Bovee-Oudenhoven, I. M. J., Feitsma, A. L., van Hoffen, E., and Schoterman, M. H. C. (2014). Functional role and mechanisms of sialyllactose and other sialylated milk oligosaccharides. Nutr. Rev. 72, 377–389. doi: 10.1111/Nure.12106
Thurl, S., Munzert, M., Henker, J., Boehm, G., Mueller-Werner, B., Jelinek, J., et al. (2010). Variation of human milk oligosaccharides in relation to milk groups and lactational periods. Br. J. Nutr. 104, 1261–1271. doi: 10.1017/s0007114510002072
Tverin, M., Westberg, M., Kokkonen, I., Tang, P., Lehmann, P., Lundstrom, K., et al. (2019). Factors affecting the degree of vertical stratification of fatty acids in grey seal blubber. Mar. Biol. 166:105. doi: 10.1007/s00227-019-3556-7
Urashima, T., Asakuma, S., Leo, F., Fukuda, K., Messer, M., and Oftedal, O. T. (2012). The predominance of type I oligosaccharides is a feature specific to human breast milk. Adv. Nutr. 3, 473S–482S. doi: 10.3945/an.111.001412
Urashima, T., Odaka, G., Asakuma, S., Uemura, Y., Goto, K., Senda, A., et al. (2009). Chemical characterization of oligosaccharides in chimpanzee, bonobo, gorilla, orangutan, and siamang milk or colostrum. Glycobiology 19, 499–508. doi: 10.1093/glycob/cwp006
Urashima, T., Taufik, E., Fukuda, K., and Asakuma, S. (2013). Recent advances in studies on milk oligosaccharides of cows and other domestic farm animals. Biosci. Biotechnol. Biochem. 77, 455–466. doi: 10.1271/bbb.120810
Urashima, T., Yamashita, T., Nakamura, T., Arai, I., Saito, T., Derocher, A. E., et al. (2000). Chemical characterization of milk oligosaccharides of the polar bear, Ursus maritimus. Biochim. Biophys. Acta 1475, 395–408. doi: 10.1016/s0304-4165(00)00103-3
Vannucci, R. C., and Vannucci, S. J. (2000). Glucose metabolism in the developing brain. Semin. Perinatol. 24, 107–115. doi: 10.1053/sp.2000.6361
Watson, D. G., Pomeroy, P. P., Al-Tannak, N. F., and Kennedy, M. W. (2020). Stockpiling by pups and self-sacrifice by their fasting mothers observed in birth to weaning serum metabolomes of Atlantic grey seals. Sci. Rep. 10:7465. doi: 10.1038/s41598-020-64488-1
Weinborn, V., Li, Y., Shah, I. M., Yu, H., Dallas, D. C., German, J. B., et al. (2020). Production of functional mimics of human milk oligosaccharides by enzymatic glycosylation of bovine milk oligosaccharides. Int. Dairy J. 102:104583. doi: 10.1016/j.idairyj.2019.104583
Windmueller, H. G., Mickelsen, O., and Anderson, A. A. (1964). Elevated riboflavin levels in urine of fasting human subjects. Am. J. Clin. Nutr. 15, 73–76. doi: 10.1093/ajcn/15.2.73
Wooding, F. B. P., and Burton, G. J. (2008). Comparative Placentation: Structures, Functions and Evolution. Berlin: Springer-Verlag.
Yu, Z.-T., Chen, C., and Newburg, D. S. (2013). Utilization of major fucosylated and sialylated human milk oligosaccharides by isolated human gut microbes. Glycobiology 23, 1281–1292. doi: 10.1093/glycob/cwt065
Zhang, P. J., Song, X. R., Han, J. B., Wang, L. M., and Yang, Y. (2014). Milk composition, milk consumption, and growth rate of a captive spotted seal (Phoca largha) pup from Liaodong Bay, China. Can. J. Zool. 92, 449–452. doi: 10.1139/cjz-2013-0295
Keywords: Atlantic grey seal, Halichoerus grypus, milk, vitamins, lactose, oligosaccharides, sterols, metabolome
Citation: Watson DG, Pomeroy PP and Kennedy MW (2021) Atlantic Grey Seal Milk Shows Continuous Changes in Key Metabolites and Indicators of Metabolic Transition in Pups From Birth to Weaning. Front. Mar. Sci. 7:596904. doi: 10.3389/fmars.2020.596904
Received: 20 August 2020; Accepted: 07 December 2020;
Published: 11 January 2021.
Edited by:
Adrian C. Gleiss, Murdoch University, AustraliaReviewed by:
Ursula Siebert, University of Veterinary Medicine Hannover, GermanyJonas Teilmann, Aarhus University, Denmark
Copyright © 2021 Watson, Pomeroy and Kennedy. This is an open-access article distributed under the terms of the Creative Commons Attribution License (CC BY). The use, distribution or reproduction in other forums is permitted, provided the original author(s) and the copyright owner(s) are credited and that the original publication in this journal is cited, in accordance with accepted academic practice. No use, distribution or reproduction is permitted which does not comply with these terms.
*Correspondence: Patrick P. Pomeroy, cHA2QHN0LWFuZHJld3MuYWMudWs=