- 1Department of Atmospheric Sciences, Texas A&M University, College Station, TX, United States
- 2Department of Oceanography, Texas A&M University, College Station, TX, United States
- 3National Aeronautics and Space Administration (NASA) Langley Research Center, Hampton, VA, United States
- 4Department of Ecology, Evolution, and Marine Biology, University of California, Santa Barbara, Santa Barbara, CA, United States
To date, the relative contribution of primary marine organic matter to the subset of atmospheric particles that nucleate cloud droplets is highly uncertain. Here, cloud condensation nuclei (CCN) measurements were conducted on aerosolized sea surface microlayer (SML) samples collected from the North Atlantic Ocean during the NASA North Atlantic Aerosols and Marine Ecosystems Study (NAAMES), κ values were predicted for three representative high molecular weight (HMW) organic components of marine aerosol: 6-glucose, humic acid, and ribulose-1,5-bisphosphate carboxylase/oxygenase (RuBisCO). The predicted κ values for pure organic aerosols varied by only ±0.01 across all of the organics chosen. For the desalted SML samples, calculations assuming an organic composition of entirely RuBisCO provided the closest predicted κ values for the desalted SML samples with a mean κ value of 0.53 ± 0.10. These results indicate that it is the sea salt in the SML which drives the cloud formation potential of marine aerosols. While the presence of organic material from the ocean surface waters may increase aerosol mass due to enrichment processes, cloud formation potential of mixed organic/salt primary marine aerosols will be slightly weakened or unchanged compared to sea spray aerosol.
Introduction
Marine aerosol-cloud interactions represent one of the largest uncertainties in understanding climate change (Carslaw et al., 2013; IPCC, 2014; Brooks and Thornton, 2018). Some aerosols act as cloud condensation nuclei (CCN) by providing sites onto which cloud droplets form. Natural events such as phytoplankton blooms may influence aerosol production and consequently have a large impact on climate. Increasing ocean temperatures, predicted to warm by +1.3 to +2.8°C globally over the twenty-first century (IPCC, 2014), are likely to increase water vapor resulting from evaporation (Held and Soden, 2000) adding further uncertainty to predictions of aerosol-cloud interactions. The warming of the ocean has the potential to change the biogenic sources of CCN as the subtropical gyres expand and phytoplankton distributions change (Rees, 2012; IPCC, 2014; Käse and Geuer, 2018). Through bubble bursting, aerosols containing large surfactants may enter the atmosphere and act as CCN to form clouds (Quinn et al., 2015; Brooks and Thornton, 2018). In addition, CCN production can occur through secondary aerosol formation resulting from chemical reactions involving gas phase marine emissions (O'Dowd et al., 2004, 2015; Sanchez et al., 2018).
Over the oceans, aerosol populations are comprised of marine aerosol and transported continental aerosol. When comparing the cloud forming potential of different aerosol populations, it is common to calculate the apparent hygroscopicity parameter, κ, of each population (Petters and Kreidenweis, 2007). Calculating κ values provides a simple way of parameterizing cloud formation which has been widely used in modeling cloud formation (Rose et al., 2010; Markelj et al., 2017). An aerosol with a low κ value requires a high supersaturation for CCN activation while an aerosol with a high κ value needs a lower supersaturation for CCN activation. Typical κ values for continental and marine regions are 0.27 ± 0.21 and 0.72 ± 0.24, respectively (Pringle et al., 2010). Variations in κ values arise due to dependence on aerosol source, composition, and aging. Table 1 shows a summary of κ values from various field campaigns and laboratory experiments.
Results from a laboratory study that measured the CCN activity changes of sodium chloride or artificial seawater mixed with surfactants estimated there would be less than a 3% change in the CCN number concentrations over the remote boundary layer under simulated “bloom” conditions (Moore M. J. K. et al., 2011). The inclusion of organic matter during bubble bursting and sea spray aerosol (SSA) formation had little effect on CCN activity suggesting that phytoplankton derived organic matter had negligible effects on CCN formation. The influence of the organics was largely masked by the influence of salts on cloud formation. In a previous study, the organic concentration measured in both fresh and aged SSA indicated that the organic content did not correlate with the CCN activation of the SSA (Herich et al., 2009; Modini et al., 2010), further suggesting that the changes in the amounts of organics present had no effect on the particles propensity to activate as CCN. Oppositely, studies have shown that the enriched organic component in ambient aerosol and could have a larger impact of the CCN activation. A laboratory experiment by Rastelli et al. (2017) found that the generation of sea spray aerosol through bubble bursting produced aerosols enriched in each organic component analyzed which included lipids (enrichment up to 140,000), carbohydrates (enrichment up to 100,000), and proteins (enrichment up to 120,000). Van Pinxteren et al. (2017) found that organic carbon was enriched in the SML relative to bulk seawater on average by a factor of two and further enriched in submicron ambient aerosol by a factor of 103-104 during periods of low chlorophyll a and 105 during periods of high chlorophyll a. Another study measured the concentration of individual saccharides relative to sodium and found enrichment of 14–1,314-fold in fine SSA, 3–138-fold in coarse SSA, and 1.0–16.2-fold in SML compared to bulk water (Jayarathne et al., 2016). Schill et al. (2015) studied internal mixtures with sea salt: galactose over a range of mass mixing ratios. These results show that for an increasing concentration of organic matter within a mixture the κ values decrease. Fuentes et al. (2011) observed that the presence of marine organic matter caused a reduction of 5–24% in the cloud condensation activity of the primary marine aerosol compared to seawater not containing any marine organics. In a series of mesocosm experiments, Schwier et al. (2017) found there were little differences in the properties of submicron aerosol generated from seawaters of the control mesocosm compared to the primary marine aerosol (PMA) generated from the enriched mesocosms, indicating that artificial blooms did not strongly affect the physical or chemical properties of PMA. In summary, there is a wide range of reported marine κ values ranging from a low κ value of <0.30 to a high κ value >0.90. A clear explanation of the causes of the variations remains unknown in part because the connection between the properties of primary marine aerosol and its organic content and composition are poorly understood.
The ability of an aerosol to activate as a CCN depends on its chemical composition as well as its size. At one point it was thought that the major source of marine CCN originated from secondary sulfate aerosol (Charlson et al., 1987). It has since been recognized that the ocean also emits primary organic aerosols which may contribute to the marine CCN population (Quinn and Bates, 2011). Microorganisms (including phytoplankton) release dimethylsulfoniopropionate (DMSP) into the water. DMSP is lysed by enzymes to produce dimethyl sulfide (DMS) and its volatile nature results in a flux to the atmosphere. After a series of oxidation reactions lasting 1–2 days in the troposphere, DMS produces sulfate aerosol (Andreae, 1990; Chen et al., 2018). A recent study quantified DMS-derived CCN concentrations from primary production and reported an increase in CCN concentrations during the biologically active late-spring compared to late-autumn when biological activity was low (Sanchez et al., 2018). Contrary to these results, Bates et al. (2020) showed that the physiological state of marine phytoplankton ecosystems have little effect on SSA and hypothesized that the major source of organic SSA is the pool of background dissolved organic carbon (DOC) available in the ocean (Quinn et al., 2014). Primary marine aerosol contributions to CCN have not been quantified with the same rigor as DMS contributions to CCN. This is due in part to the lack of measurements that constrain the amount, chemical composition, and potential sources of organic matter in primary marine aerosol (Brooks and Thornton, 2018). The wide variety of marine κ values reported across experimental and field campaigns suggest that organics may be responsible for this variability.
Sea spray aerosol composed predominantly of salt enters the marine boundary layer at an estimated rate of 2,000–10,000 Tg yr−1 (Gantt and Meskhidze, 2013). SSA also contains 10 ± 5 Tg yr−1 of organic material. While this is a relatively small amount, it may have significant impacts on the cloud forming properties of aerosols. The composition of seawater, contains 35 g/L salts and 0.002 g/L organics (Mackinnon, 1981; Hansell and Carlson, 2014). These organics are mainly contributed by the marine dissolved organic carbon (DOC) reservoir which, at 662 Pg (Hansell et al., 2009) is the largest pool of reduced carbon in the ocean. DOC is operationally defined as organic matter that passes through a 0.70 μm filter and is comprised of myriad of compounds that span from dissolved solutes, to colloidal material to small cells and cell parts (Carlson and Hansell, 2015). DOC is often grouped into low molecular weight (LMW) and high molecular weight (HMW) pools, which are <1,000 atomic mass units (amu) and >1,000 amu in size, respectively (Benner and Amon, 2015). HMW DOC is typically 25–40% of the DOC pool in the surface ocean (Benner, 2002; Kaiser and Benner, 2009). Injections of fresh DOC into the atmosphere can contribute to changes in SSA composition (Miyazaki et al., 2018).
In a series of field experiments in clean regions (with minimal anthropogenic influences) of the North Atlantic and Arctic, the majority of organic mass in aerosols was classified as carbohydrate-like compounds containing hydroxyl groups from primary marine emissions (Russell et al., 2010). Similarly, another study found that chemical interactions between soluble saccharides and surface-active molecules could provide a saccharide-like composition of the SSA (Burrows et al., 2016) through a proposed mechanism of ion-mediated co-adsorption (Schill et al., 2018). Conversely, Lawler et al. (2020) determined that the alcohol functional group was a major contributor to primary sea spray organic mass and that polysaccharides represent some fraction of the alcohol group mass but do not appear to be the main contributor. Changes in molecular diversity in seawater have been shown to modulate the CCN activity of SSA (Cochran et al., 2017). However, relating the composition of SSA to organic matter in the water column is a challenge given the molecular diversity of dissolved organic material (DOM) is an estimated 1012-1015 compounds (Hedges, 2002). During a phytoplankton bloom, the composition of organic matter in the water column will depend on community composition of the phytoplankton and associated bacterioplankton, their relative abundances, production, and physiological strategy as they respond to the dynamic physical and chemical environment (Williams, 1995; Carlson et al., 1998; Kujawinski, 2011; Thornton, 2014). Consequently, the molecular diversity of marine aerosols is affected by the composition and productivity of microorganisms in the water (Cochran et al., 2017). In a mesocosm experiment it was found that only 32% of the submicron aerosol contained long-chain fatty acids at the beginning of a phytoplankton bloom (Cochran et al., 2017). Other compounds included polysaccharides (14%), siliceous material (13%), and a mixture of free saccharides and short-chain fatty acids (17%). However, at the later phases of the bloom the fraction of long-chain fatty acid particles increased significantly (up to 75%). High concentrations of CCN were measured in sea spray aerosol enriched in organic matter, suggesting this phenomenon was related to the enrichment of marine hydrogels in the SSA which contributed to the CCN population (Ovadnevaite et al., 2011). Gel-forming properties similar to those in marine hydrogels are thought to play a role in the observed dichotomous behavior of organic rich sea spray particles (Ovadnevaite et al., 2011). Primary aerosols are injected into the atmosphere through bubble bursting, in which bubbles in the ocean rise to the surface where they pass through the sea-air interface, known as the sea surface microlayer (SML). The SML is operationally defined as the uppermost 1–1,000 μm of the ocean (Liss and Duce, 1997). It is enriched in surface-active organic compounds, such as proteins, lipids, polysaccharides, and amino acids, relative to the subsurface waters (Kuznetsova and Lee, 2002; Reinthaler et al., 2008; Cunliffe et al., 2013; Thornton et al., 2016; Aller et al., 2017). Enriched organics present in the SML can be in the form of gel-like particles such as Coomassie Stainable Particles (CSP) (which contain protein) and Transparent Exopolymer Particles (TEP) (which contain acidic polysaccharides) (Alldredge et al., 1993; Passow, 2002; Wurl and Holmes, 2008; Thornton et al., 2016; Engel et al., 2017). Surface active compounds (surfactants) are emitted to the atmosphere as a major component of primary marine aerosol (Cochran et al., 2016; Chingin et al., 2018; Frossard et al., 2019) through bubble bursting (Facchini et al., 2008; Frossard et al., 2014, 2019). While bubble bursting through the SML is a source of primary marine aerosol (Russell et al., 2010; Brooks and Thornton, 2018), previous work suggests that surface active compounds are primarily emitted from the ocean directly by bubble plumes, which scavenge organics as they rise to the surface (Chingin et al., 2018; Beaupre et al., 2019; Frossard et al., 2019).
Organic carbon can be enriched by more than 100% from the subsurface waters to the SML (Van Pinxteren et al., 2017). By comparison, the enrichment of salt in the SML does not typically exceed 3% compared to the bulk water (Liss and Duce, 1997). Organic compounds common in the SML have been identified in primary marine aerosol, including HMW surface active DOM, such as polymers incorporated into microgels, TEP, and CSP (Kuznetsova et al., 2005; Orellana et al., 2011; Aller et al., 2017). HMW species are of interest because they tend to be largely surface active organics (Engel and Händel, 2011; Engel and Galgani, 2016). As such, it has been proposed that their potential role in improving CCN efficiency is high even when they are present in low concentrations, with organic mass fraction as small as 0.20 (Sorjamaa et al., 2004; Lowe et al., 2019). In addition, HMW DOM is more closely coupled to local biological activity than LMW DOM as it is generally more recently produced. Additionally, freshly produced LMW compounds are also the most labile compounds, thus the flux and associated turnover of fresh LMW can be very high resulting in little fresh LMW DOM accumulation. In contrast, LMW DOM contains more recalcitrant material that reflects the “background” DOM but can also be coupled to local biological activity (Amon and Benner, 1996).
To date, the connection between HMW organic surfactants concentrated in the SML and cloud formation is uncertain. Since HMW organic species tends to be surface active, their presence even in relatively small quantities may reduce the equilibrium vapor pressure over drops promoting the activation and growth of cloud droplets (Lohmann et al., 2016; Kubicki et al., 2019). It has been shown that after marine primary aerosol are formed they can become coated with organic surfactants, such as long-chain hydrocarbons (Djikaev and Ruckenstein, 2014; Luo et al., 2019). In this study, SML samples were collected in the North Atlantic Ocean to quantify the relative importance of the salt and organic constituents of aerosols emitted from the surface waters. Analyzing SML samples rather than directly collected SSA is a clear caveat in this study. However, previous studies have also relied on SML samples (Rasmussen et al., 2017; Irish et al., 2019; Christiansen et al., 2020; Ickes et al., 2020; Wolf et al., 2020) and even on surface water samples (Mason et al., 2016; Irish et al., 2017; Wilbourn et al., 2020) to understand the warm and ice cloud nucleating behavior of materials contained in aerosol. Christiansen et al. (2020) observed that the CCN activity of SML samples collected in the North Atlantic and Arctic Ocean was the same as artificial seawater containing only salt, within experimental error. Although seawater rather than SML samples were analyzed, Rasmussen et al. (2017) did not observe significant changes in CCN properties due to marine organics. In our study, a subset of the SML samples were desalted to remove all sea salt components leaving only the organic constituents behind. Next, both SML and desalted SML samples were aerosolized and their CCN properties were measured. Modified Köhler theory, along with supporting measurements of SML composition and surface tension, was used to evaluate the contribution of primary organic aerosols to the overall cloud formation process. The results from this modeling study determine how mixtures of marine organics and salts influence κ values. Modeled results were compared to the observed CCN properties to provide insight on which SML components drive the cloud formation potential of marine aerosols.
Experimental Details
The National Aeronautics and Space Administration (NASA) North Atlantic Aerosols and Marine Ecosystems Study (NAAMES) focused on the effects of phytoplankton blooms and associated ecosystem processes on the formation of marine aerosols and their effect on boundary layer cloud properties (Behrenfeld et al., 2019). NAAMES consisted of four separate research cruises on the R/V Atlantis over a 4-year span. Within the context of NAAMES, the objective of this study was to characterize the cloud-forming potential of organic compounds in marine aerosols generated by the SML, in comparison to the cloud forming potential of salts. SML samples were collected during the third and fourth campaigns, August-September 2017 and March-April 2018, respectively. Composition analysis included ion chromatography (IC), total organic carbon (TOC) measurements, and DOC measurements.
Sample Collection and Storage
SML samples were collected during cruises in August–September 2017 (NAAMES 3) and March–April 2018 (NAAMES 4) at each of the stations indicated in Figure 1. At each station, profiles of hydrographic variables including TOC, DOC, and chlorophyll a were collected in the surface 500 m as described in Behrenfeld et al. (2019) and Baetge et al. (2020). These were later compared against corresponding measurements of SML samples to determine which components were enriched in the SML. To collect a SML sample, a Garrett screen (57.5 cm2) was attached to a nylon rope and lowered over the starboard side of the ship (Garrett, 1965; Galachyants et al., 2016; Dreshchinskii and Engel, 2017; Sabbaghzadeh et al., 2017; Drozdowska et al., 2018) until it lightly touched the surface of the ocean and then it was pulled back up to the deck. During times of SML sampling, the ship was in a stationary position and the screen was lowered down to the ocean surface close to the hull of the ship. The Garrett screen was held at an angle to allow the microlayer sample to run off the screen through an acid-cleaned glass funnel and into an acid-cleaned polycarbonate bottle. Sampling was repeated until a volume of ~1.5 L was collected. Milli-Q water was used to clean the screen, funnel, and polycarbonate bottle between sampling periods. Samples were then subsampled for CCN property measurements into acid-washed storage bottles and immediately placed into a −80°C freezer to preserve the samples until they could be analyzed in laboratories at Texas A&M University and NASA Langley Research Center (LaRC). Samples were also subsampled for TOC and DOC into pre-combusted (4 h at 450C) 40 mL borosilicate glass EPA vials, fixed immediately with DOC-free 4N HCl to a pH of <3, and stored at ~14°C in an environmental chamber free of volatile organics until processing at the University of California, Santa Barbara.
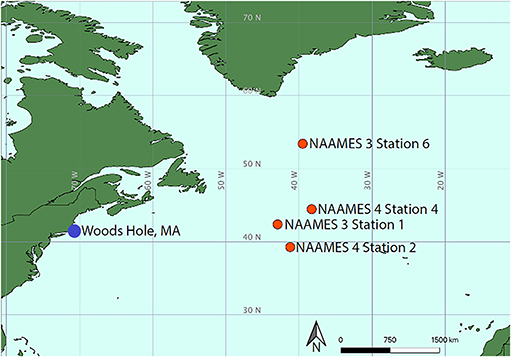
Figure 1. Subset of NAAMES 3 and NAAMES 4 sampling stations. Sampling stations are indicated with red markers. The blue marker indicates the start and end point for NAAMES 3 and the end point for NAAMES 4.
Desalting Process
Prior to measuring the cloud activation potential of the organics contained in the microlayer, it was necessary to first remove the non-organic salt components from the samples. Chlorophyll a concentrations were measured using HPLC. From each field campaign, two samples were chosen from the stations with the highest and lowest chlorophyll a concentration (Table 2). To begin the desalting process, the samples were removed from the −80°C storage freezer at Texas A&M University and allowed to thaw at room temperature. Next, the salinity of each sample was determined using a portable refractometer. The samples were not filtered prior to the desalting process.
The desalting process was conducted using Spectra/Por 7 regenerated cellulose membrane (Spectrum Chemical) tubing with a volume to length ratio of 4.6 ml/cm. The inside and the outside of the tubing was rinsed with ultra-high purity (UHP) water to remove any associated organic or inorganic compounds. A clamp was placed on one end of the tubing and then the tubing was filled with 10 ml of sample. The other end was clamped closed and the bag was placed in a container filled with UHP water. The water in the container was stirred every 2 h and the salinity of the samples was measured after 6 h using a portable refractometer. After 6 h the water in the container was replaced with fresh UHP water and the final salinity of the samples was measured again after 24 h. The samples were poured from the dialysis tubing into VWR sterile 15 ml centrifuge tubes and frozen at −80°C until further analysis could be performed.
Dialysis membranes are characterized by their molecular weight cut-off (MWCO), which is defined by the manufacturer as the smallest solute at which 90% (by weight) is retained by the membrane. The Spectra/Por 7 membrane had a molecular weight cut-off 1,000 amu. Additionally, the efficiency of the Spectra/Por 7 membrane was determined in previous work (Thornton et al., 2007). For compounds with sizes below the MWCO, the removal efficiency will be increased. The membrane removed salts in this study by >99% as confirmed by the refractometer and ion chromatography measurements. Conversely, the representative HMW organic compounds evaluated in this study, 6-glucose (a stable oligosaccharide composed of 6 glucose units), humic acid, and RuBisCO, are each larger than the membrane MWCO. Based on the manufacturer specifications, at least 90% of the mass of these organic compounds was retained. Since it was not feasible to measure the organic composition in the desalted samples, a more accurate value cannot be reported. Fortunately, the CCN measurement procedure employed in this study is not sensitive to the total mass of material, only to the relative contributions to the mass of solutes in the aerosolized solution. It should be noted that any smaller organic compounds, i.e., the LMW organic compounds, will be largely removed during the desalting process. Since the desalting process largely removed LMW compounds, measurements after desalting reflect the HMW organic compounds.
Characterizing Organic and Salt Contributions to Cloud Formation Potential
The SML and desalted SML (DSML) samples were shipped in an insulated container with dry ice to the NASA LaRC for further analysis, including the abundance and size distribution of aerosols generated from the samples in the laboratory, the CCN activity of the aerosols, ion chromatography (IC), and surface tension.
Cloud Condensation Nucleation
The setup for CCN measurements in the NASA LaRC laboratory is shown in Figure 2. A custom built nebulizer was used to create aerosols from the SML and DSML samples. While the nebulizer generated particles, the CCN measurements in this study were not sensitive to the total mass of material, only to the relative concentrations of the mass of solutes in the aerosolized solutions. The aerosols were then passed through a desiccant drier to a differential mobility analyzer (DMA, TSI Inc. Model 3082) for size selection. The size range was set to measure aerosol sizes from 10 to 520 nm. Exiting the DMA, the flow of aerosols was split between a condensation particle counter (CPC, TSI Inc. Model 3776) and a cloud condensation nuclei counter (CCN counter, Droplet Measurement Technologies, Inc. CCNC-100). The use of the nebulizer in this study may have reduced the organic fraction in the aerosol to some degree. Other studies have reported that using the water fall method produces aerosols with more realistic marine aerosol compositions than other generation methods (Fuentes et al., 2010; Prather et al., 2013; Stokes et al., 2013; Collins et al., 2014; Lee et al., 2015; McCluskey et al., 2017; Christiansen et al., 2019).
Using the LaRC setup shown in Figure 2, a supersaturation was selected on the CCN counter. Then a series of diameters were scanned by the DMA to allow a range of particle sizes to enter the CCN counter (Moore et al., 2010). The activated fraction, which is the ratio of the concentration of particles which activate at a given supersaturation as CCN (measured by the CCN counter) to the total concentration of particles counted (measured by the CPC) was determined as a function of diameter.
The critical diameter of an aerosol is defined as the diameter an aerosol must reach for spontaneous uptake of water and cloud droplet formation to ensue. Operationally, the critical diameter was determined by plotting activated fraction against dry diameter and determining the diameter at which 50% of the particles activated as CCN (Rose et al., 2008; Ma et al., 2013). The critical supersaturation, defined as the supersaturation when the critical diameter is achieved and spontaneous growth occurs, was also determined. An example of an activated fraction vs. diameter plot is provided in Supplementary Figure 1.
Prior to the experiment, CCN instrument calibrations were performed following the procedure of Moore et al. (2010). Using data from an ammonium sulfate calibration, the critical supersaturation of ammonium sulfate determined using Köhler Theory was plotted against the temperature gradient measured within the CCN instrument. Model I regression was used to calculate an adjusted supersaturation and hence, the critical supersaturation. In the Model I regression the temperature gradient was considered the independent variable and the percentage supersaturation was considered the dependent variable.
Seawater Organic Carbon Measurements
Replicate TOC and DOC concentrations (μmol C L-1) were determined by the high temperature combustion technique using Shimadzu TOC-V or TOC-L analyzers (Carlson et al., 2010). Each batch analysis was calibrated using glucose solutions of 25–100 μmol CL-1 in low carbon blank water. Data quality was assessed by measuring surface and deep seawater references [sourced from the Santa Barbara Channel (SBC)] after every 6–8 samples. Precision for DOC analysis is ~1 μmol L-1 or a coefficient of variation (CV) of ~2%. SBC reference waters were calibrated with DOC consensus reference material provided by Hansell (2005).
For the subsurface waters TOC samples were taken directly from the shallowest Niskin bottles (5 m), and DOC samples were filtered through 0.7 μm GF/F filters from the Niskin bottles. From the SML samples, a total of six corresponding TOC and DOC samples were taken. In those samples, the mean difference between the two measurements was 3.94% (Supplementary Figure 2). All other SML samples were only subsampled for either TOC or DOC. To be able to compare subsurface samples to SML samples, the mean difference between SML TOC and DOC was applied as a correction factor to TOC samples, such that DOC in those samples equaled: TOC - (TOC × 0.0394).
Salt Concentration Measurements
To obtain measurements of the salt concentrations before and after desalting, a portable refractometer and ion chromatography (IC) were used. Ion chromatography (Dionex ICS-3000, ThermoFisher Scientific) measured the concentrations of chloride, bromide, nitrate, sulfate, sodium, ammonium, potassium, magnesium, and calcium. Two parallel systems were used to analyze anions and cations using potassium hydroxide and methanesulfonic acid eluents, respectively. Ion peaks were identified using conductometric detection and quantified against inorganic standards (Dionex combined seven anion standard II, Dionex combined six cation standard, ThermoFisher Scientific). Then, using the IC data and the stoichiometry of the most abundant salts in seawater (Morcos, 1970), the relative concentration of salts was determined by pairing cations with anions to predict concentrations of sodium chloride, magnesium chloride, magnesium sulfate, and sodium sulfate. While other compounds found in seawater were not considered, these four compounds comprised at least 95% of the mass of the salts in each SML sample. No IC data available for NAAMES 3 Station 6 sample so the composition was assumed to be the same as the NAAMES 3 Station 1 sample. For reference, the ion chromatography data is reported in Supplementary Table 1.
Surface Tension
The surface tension was measured for each SML and DSML sample using a pendant drop tensiometer (Biolin Scientific Model Attension Theta). The measured surface tension values of the SML and DSML samples were used in the predictions of the hygroscopicity parameter, κ, as discussed below. In CCN calculations, the surface tension is often assumed to be that of pure water (Ovadnevaite et al., 2017; Bzdek et al., 2020; Lin et al., 2020). However, that assumption may be inaccurate in the case of marine samples because surface active microgels, carbohydrates, proteins, and lipids can reduce surface tension and allow CCN to activate at lower supersaturations (Moore et al., 2008; Brooks and Thornton, 2018; Jenkinson et al., 2018). It has been suggested that for the surface tension of aerosols composed purely of surfactants to reach its equilibrium requires significant time (as much as 100 s or more) (Noziere et al., 2014). Fortunately, the inclusion of salts, such as in marine aerosol, reduces the equilibrium time. Therefore, in the present study, the surface tension and CCN measurements may have had sufficient time to each equilibrium, after all. Nevertheless, this should be addressed in future research.
Calculations
Calculating κ Values From CCN Measurements
Traditional Köhler Theory describes an aerosol's ability to activate as a CCN and form cloud droplets based upon the aerosol's physical and chemical properties (Köhler, 1936). The aerosol's size and composition will affect the aerosol's cloud-forming efficiency by changing the critical diameter and critical supersaturation. Petters and Kreidenweis (2007) reformulated the Köhler equation as κ-Köhler Theory to provide a single parameter, κ, to predict cloud activation based on particle hygroscopicity. Values of κ can be derived directly from CCN measurement or predicted based on physicochemical properties of the aerosols available to act as CCN.
The flow chart in Figure 3 shows the inputs required to calculate κ values directly from CCN measurements and to predict κ values based on physiochemical properties and auxiliary measurements. The critical diameter (D) and critical supersaturation (Sc) were determined experimentally as described above. Next, a κ value was determined using Equations (1) and (2) (Petters and Kreidenweis, 2007). The surface tension of SML and DSML samples was measured and used as an input in Equation (2) (also used in Equation 12) to predict the critical diameter of a droplet at thermodynamic equilibrium. In these equations, σ is the surface tension of the solution (SML or DSML sample measured in this study), MWwater is the molecular weight of water, ρwater is the density of water, R is the ideal gas constant, T is the temperature in Kelvin, D is the dry diameter of the particle, and Sc is the critical saturation ratio. The terms within the variable A are based on the physical properties of pure water, except for σ which is based on the solution.
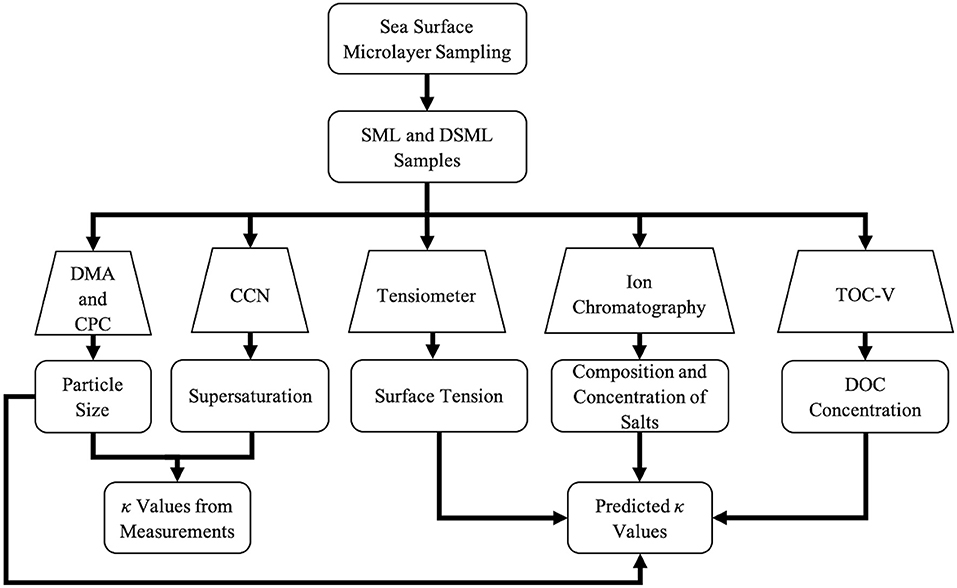
Figure 3. Flow chart for analysis to determine κ values from measurements and predicted κ values. The trapezoids and rectangles indicate the instrumentation or analysis technique used and the information collected from the specified methods, respectively.
κ Predictions Based on Organic Compounds in the SML
To predict κ values based on physiochemical properties, the measured organic and inorganic compositions and surface tension were used as inputs in combination with choices of representative organic compounds in the SML (Figure 3). The primary purpose of these predictions was to quantify how the total organic mass in marine aerosols influenced the aerosol's ability to act as a cloud forming droplet. Secondly, predicted κ values were compared to experimentally obtained κ values to evaluate which possible organic composition best matches the observations.
The SML predicted κ values were calculated using the quantity of salts (from ion chromatography) and measured DOC concentrations. According to the ion chromatography, the DSML salt concentration was reduced by >99% from the desalting process. For the predicted κ values for the DSML samples, the organic concentrations were assumed to be the same as the concentrations in the SML samples. Measured DOC concentrations were converted from to based on the molecular formula of the representative organic species.
Three different HMW organic compounds, 6-glucose, humic acid, and RuBisCO, were chosen to represent the marine organic compounds in the Atlantic Ocean. Properties for each of the HMW organic compounds are shown in Table 3. The theoretical κ values included in the table were calculated using modified Köhler theory, as discussed below. Six-glucose is an oligomer of glucose. Glucose is a major component of DOM (Skoog and Benner, 1997; Goldberg et al., 2011) and a main building block of polysaccharides. Carbohydrates make up about 20% of marine DOM, with the bulk being polysaccharides (Benner et al., 1992). Humic acid is known to contribute to marine surfactants, both can be enriched in the SML (Drozdowska et al., 2017). RuBisCO is a key enzyme in carbon fixation in plants, algae, cyanobacteria, and phototrophic and chemoautotrophic bacteria, and is responsible for carbon fixation during 95% of marine photosynthesis (Orellana and Hansell, 2012; Dai et al., 2018; Bar-On and Milo, 2019). RuBisCO is the most abundant protein on Earth (Ellis, 1979).
As described above, the desalting process removed ~90% of any LMW compounds which may have been present in the initial samples, while leaving at least 90% of the HMW compounds in the samples. Therefore, a direct comparison between the desalted field samples and calculations for LMW compounds cannot be made. Nonetheless, several LMW compounds are included in Table 3 to provide insight on how cloud properties vary with organic composition. Along with humic acid, fulvic acid contributes to DOM (Drozdowska et al., 2017). Triolein was chosen because in unpolluted surface microlayers 40–65% of the lipids are fatty acids and triacylglycerols (Arts, 1999). Triolein is a triglyceride formed from oleic acid which has been measured in the North Atlantic Ocean waters and also from aerosol measurements sampled over the North Atlantic Ocean (Duce et al., 1983). The photosynthetic pigment chlorophyll a was chosen because of its ubiquitous distribution and relatively high concentrations in the surface ocean, and because it is used as an indicator of phytoplankton biomass.
To predict a κ value for the SML and DSML samples, the concentration of salts and organics within the aerosol must be considered (Rogers and Yau, 1989). First, the salts were considered independently from the organics. The individual salt ions measured by the IC were paired together to form ionic compounds (sodium chloride, sodium sulfate, magnesium sulfate, magnesium chloride, and calcium sulfate) that are typically found in seawater (Supplementary Table 1). Next, the number of moles and mole fraction of each compound in the seawater were determined. The mole fraction (mole fractionsalts) and the molecular weight (molecular weight) of each compound were used to find the weighted molecular weight of the salts (MWsalts), shown in Equation (3). Using the mole fraction and the van't Hoff factor, the weighted van't Hoff factor for each compound was determined. The van't Hoff factor is a ratio between the actual concentration of dissolved ions produced when the substance is dissolved and the molar concentration of the substance. The weighted density of the salts (ρsalts), is shown in Equation (4), which includes the volume fraction of the salts (volume fractionsalts) and the density (density). We refer to the mixture of ionic salt compounds present in the microlayer samples as salts.
For samples containing organics and salts, the salt concentration was determined as a weighted average as above, and for simplicity we assumed the molar C equivalent of the SML DOC concentration was composed of a single organic compound. We recognize that this is an oversimplification of the complexity of naturally occurring DOC, but it is useful as a first order evaluation of whether a compositional shift in DOC had an impact on κ values. The measured DOC concentration was converted from , which is the number of μmoles of carbon per liter, to g/L of the single representative organic compound. This conversion is shown in Equation (5) where N is the number of carbon atoms in the chosen organic compound and MWorg is the molecular weight of the chosen organic compound. Since the number of carbon atoms in the molecules varies between samples, the term N is used to normalize the organic concentration between the different organic compounds considered.
Since the SML samples contain both salts and organics, the next step was to determine the volume fraction for the salts and the organics in the SML aerosols. In calculating the volume fractions, it was assumed that the volume of the solute plus the volume of water equals the total volume (volume additivity assumption), which uses the pure water density to determine the partial molar volume of the water. One volume fraction was used to determine the concentration of salts present in the aerosol and another volume fraction was used to determine the concentration of organics present in the aerosol. In these equations Wsalts is the volume fraction for the salts, Worg is the volume fraction for the chosen organic compound, ρsalts is the weighted density of salts, organic concentration is determined using Equation (5), and ρorg is the density of the chosen organic compound.
The density of the particle was determined as a volume-weight average of the pure component densities. In this equation ρparticle is the density of the particle, Wsalts is the volume fraction for salts, ρsalts is the weighted density of salts, Worg is the volume fraction for organics, and ρorg is the density of the chosen organic compound.
Similarly, the mass of the organic in the SML aerosol was determined using the density of the organic and the volume of the particle. The volume of the particle was determined assuming the particle was a sphere. In these equations D is the critical diameter, massorganic is the mass of the organic, ρorg is the density of the organic, and V is the volume of the particle.
Once the density of the particle was known, the mass of the mixture within the aerosol generated from the SML was calculated. In this equation massmixture is the mass of the mixture, ρparticle is the density of the particle determined using Equation (8), and V is the volume of the particle determined using Equation (9).
Since the SML aerosol was a mixture of salts and organics, the factors to calculate a κ for the aerosol must be weighted based on the concentration of salts and organics present in the aerosol. These variables include a weighted van't Hoff factor, iweighted, and a weighted molecular weight, MWweighted. Using Equations (6) and (7) above, a weighted van't Hoff factor and a weighted molecular weight were calculated for the mixture of salts and organics within the particle. For the weighted van't Hoff factor, a van't Hoff factor of 1 was used for each organic composition assuming the organic was unlikely to dissociate in water.
Using Equation (12) (Rogers and Yau, 1989), the critical supersaturation was calculated.
In Equation (13), σ is surface tension of the solution measured in the experiments, ρwater is the density of water, R is the ideal gas constant, and T is the temperature in Kelvin. In Equation (14), i is the van't Hoff factor, masss is the mass of the solute, MWwater is the molecular weight of water, ρwater is the density of water, MWs is the molecular weight of the solute.
In calculating the parameter c for an internally mixed composition, the weighted factors calculated above are needed. For the SML aerosols containing both salts and organics, the weighted factors adjust the calculations to reflect the concentrations of salts and organics present in the mixed aerosol. When determining κ for the aerosols generated by the desalted SML samples, the weighted factors were also needed, due to the fact that a reduced concentration of salt remained in the samples following the desalting process. These remaining salts were quantified using IC data. Therefore, the aerosols generated by the DSML samples must also be treated as a mixture of salts and organics but the concentration of salts has been greatly reduced. When the aerosol was treated as organic only, the κ values predicted were too low and did not match the κ values derived from the CCN measurements. Equations (14a) and (14b) include chemical composition data that is needed to calculate a κ value for the aerosols generated by the DSML and SML samples. Equations (14a) and (14b) show how the equation is modified depending on if the aerosol is considered pure organic or mixed (salts and organics). In these equations MWwater is the molecular weight of water, ρwater is the density of water, co is a parameter for organic composition only, iorg is the van't Hoff factor for the organic, massorganic is the mass of the organic, MWorg is the molecular weight of the chosen organic compound, cm is a parameter for a mixture of organics and salts, iweighted is the weighted van't Hoff factor, massmixture is the mass of the mixture, and MWweighted is the weighted molecular weight. The mixture mass is the organics plus the salts measured before or after desalting.
After the critical supersaturation has been calculated, it is used in variations of Equations (1) and (2) to determine the κ values.
Results and Discussion
CCN Measurements
The critical diameters and κ values derived from CCN measurements of aerosolized SML and DSML samples are shown in Figures 4A,B, respectively. As expected, the mean critical diameter for SML samples decreased with an increase in supersaturation (SS), ranging from 48.6 ± 0.5 nm at 0.32% SS to 20.3 ± 0.3 nm at 1.24% SS. Rasmussen et al. (2017) determined the critical diameters of aerosolized SML to be 70.9 nm at 0.2% SS and 30.6 nm at 0.76% SS. Christiansen et al. (2020) found the critical diameters of aerosolized SML to be 100 nm at 0.11% SS and 50 nm at 0.32% SS. The DSML critical diameters ranged from 73.9 ± 5.7 nm at 0.32% SS to 27.9 ± 1.0 nm at 1.24% SS. At every supersaturation, a t-test was performed to compare the SML and DSML critical diameters. In all cases, the critical diameter was different at the 5% significance level. The mean κ value for SML samples ranged from 0.83 ± 0.09 at 0.63% SS to 1.1 ± 0.03 at 0.32% SS. The mean κ values for DSML samples ranged from 0.32 ± 0.07 at 0.32% SS to 0.39 ± 0.04 at 1.24% SS. From station to station the SML κ value observed at each supersaturation varied at most by ±0.09 and the DSML κ value varied at most by ±0.07. These data suggested that the desalting process affected κ values more than the variation in organic matter between stations.
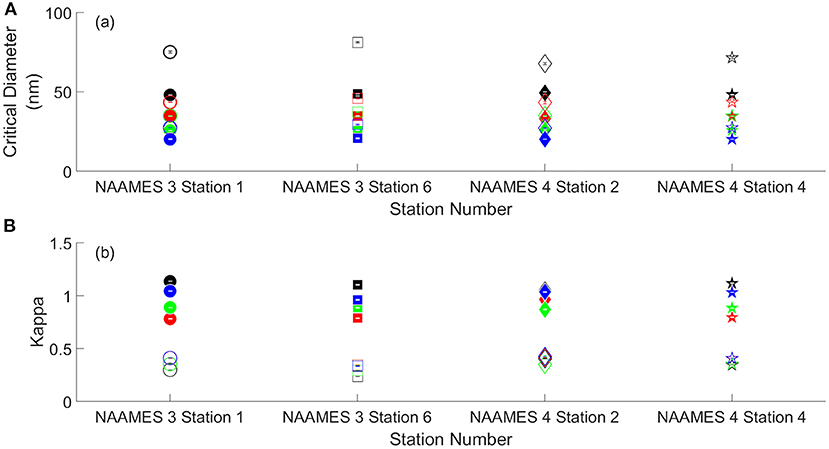
Figure 4. (A) Measured critical diameters of aerosols generated from SML and DSML samples. (B) Hygroscopicity parameter, κ, values derived from CCN measurements for aerosols generated by SML and DSML samples. The black, red, green, and blue symbols indicate measurements at 0.32% 0.63% at 0.93%, and 1.24% supersaturation, respectively. The open symbols indicate DSML samples and the solid symbols indicate SML samples. Error bars show error calculated using error propagation analysis.
The results suggest that aerosols containing relatively more HMW organic material and less salt require more initial aerosol mass for spontaneous droplet growth to occur. In previous studies, indirect evidence has suggested that HMW marine organic compounds are surface active and efficient CCN (O'Dowd et al., 2004; Facchini et al., 2008; Ovadnevaite et al., 2011). However, the results indicate that the concentration of organics present in this study weaken rather than strengthen the cloud-nucleating properties of sea spray aerosol, in that the organic compounds required growth to larger critical diameters prior to activation. Amongst the DSML samples, the highest critical diameter, 81.2 nm at 0.32% SS was observed for the NAAMES 3 Station 6 sample, compared to a lower critical diameter of 67.9 nm at the same SS at NAAMES 4 Station 2. The reason for variations from station to station could not be determined.
SML aerosols were more hygroscopic with κ values of 0.96 ± 0.12 compared to the DSML aerosols which had κ values of 0.36 ± 0.05 (Figure 4B). In the DSML samples, the κ values were significantly lower than those from the SML samples. As above, a t-test was performed on the κ values from the SML and DSML samples at the 5% significance level which concluded that the SML κ values were statistically higher than the DMSL values. Collins et al. (2016) found global marine κ values ranged from 0.70 to 1.4 with an average of 0.95 ± 0.15. The κ values from the present study are higher than those observed along the coast of Southern California which averaged 0.22 ± 0.12; however, this study reported that pollution and other anthropogenic sources led to possible decreases in κ values (Gaston et al., 2018). To our knowledge, this is the first study to determine κ values directly from aerosols generated by SML samples. Broadly speaking, the κ values in this study were in good agreement with a modeling study which estimated global mean κ for marine regions of 0.72 ± 0.24 (Pringle et al., 2010). However, their modeling results exhibited a narrower standard deviation than the range of values in our study. The current study is novel in that the aerosolized SML samples were analyzed to determine κ values directly from aerosols generated by SML samples, and it must be noted that the difference in techniques may contribute to different values of κ. To our knowledge, the only previous study on κ values of SML samples was that by Christiansen et al. (2020). They report κ values of 1.03–1.16, though these were taken in the Arctic Ocean unlike our own.
Comparison of Variables Between SML and Water Column
The station averaged DOC concentrations in the SML samples are shown in Figure 5. These concentrations were used to calculate the total mass of organics present in the aerosols generated by the SML and DSML samples. In all cases, the SML had a higher concentration of DOC than the subsurface water, which was sampled at 5 m using the conductivity, temperature, depth (CTD) rosette. The enrichment factor for DOC in the SML ranged 1.2–3.2 compared to the subsurface water. The SML chlorophyll a concentrations at each station are also shown in Figure 5. SML DOC concentrations were not directly coupled to phytoplankton biomass, indicated by the fact that the lowest SML chlorophyll a concentration was associated with the highest SML DOC concentration. The lack of correlation between SML DOC and chlorophyll a concentrations is unsurprising given that DOC is comprised of a myriad of compounds of varying lability and turnover times that is dominated by large and old refractory background pool (Carlson, 2002; Hansell, 2013).
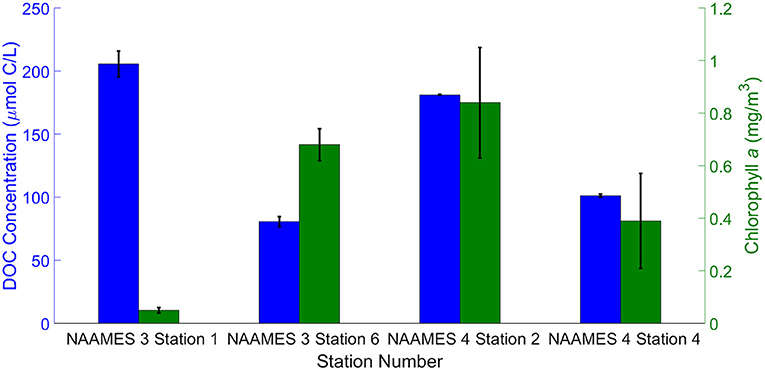
Figure 5. Station averaged dissolved organic carbon (DOC) and chlorophyll a concentrations in SML samples. The blue and green bars represent the DOC concentrations and chlorophyll a concentrations, respectively. The black lines on each blue and green bar represent the standard deviation across measurements of DOC and chlorophyll a at each station.
Salt Concentration
A comparison between the salt concentrations measured using portable refractometer and ion chromatography (IC) data is included in Table 2. The portable refractometer was used to monitor the salinity of the samples during the desalting by dialysis. The limit of detection for the portable refractometer was 1.7 parts per thousand by volume (pptv). The refractometer was used as an initial measurement technique to determine if the desalting process would be effective at decreasing the concentration of salts in the SML samples. Since the refractometer is not a high resolution instrument, IC was used to determine the concentration of the individual salts in the samples. The IC data was used to calculate the weighted molecular weight, weighted van't Hoff factor, and the weighted density of the total salts present in the samples. By comparing the concentrations of inorganics measured before and after the desalting, it was clear that dialysis was effective (Table 2). Desalting removed >99% of the mass of salts from the SML samples.
Surface Tension
The mean surface tension of the SML and DSML samples were 69.4 ± 1.8 mN/m and 70.4 ± 2.0 mN/m, respectively, which are indistinguishable from one another at the 5% significance level. Overall, the surface tension measurements of the SML and DSML samples were small variations from the surface tension of pure water (72.8 mN/m). This result was in contrast to other studies which showed that surface active microgels, carbohydrates, proteins, and lipids can reduce the surface tension and allow CCN to activate at lower supersaturations (Moore et al., 2008; Brooks and Thornton, 2018; Jenkinson et al., 2018). However, the surface tension measured and discussed in this study was performed using the SML and DSML samples. The surface tension of the aerosol generated from the SML and DSML samples was not evaluated. Nevertheless, for accuracy in the calculations, the surface tension measured from the SML and DSML samples (Figure 6) was used in the calculations of κ. The measured surface tension values were utilized in Equations (1), (2), (12), and (13) to determine the critical saturation ratio used when predicting κ values for the aerosols generated by the SML and DSML samples.
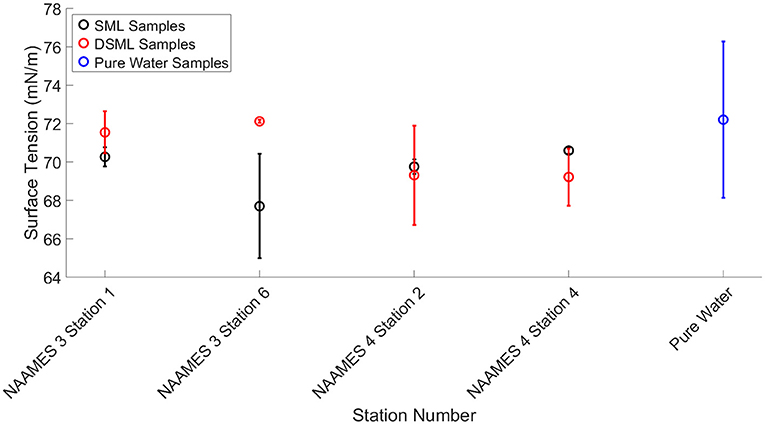
Figure 6. Surface tension measurements of SML samples, DSML samples, and pure water. Black, red, and blue symbols indicate measurements from SML samples, DSML samples, and pure water, respectively. Error bars represent the standard deviation (n = 21, 16, and 7 for SML samples, DSML samples, and pure water respectively) of the measurements taken.
CCN Modeling Variation in Organic Composition and Resulting κ Values
Figures 7A–C show the κ values from the CCN measurements compared to the κ values from predictions based on specific HMW organic composition within the SML samples. The composition and concentration of salt used in κ value predictions was based on the IC data of the SML samples. The concentration of the organic compound was based on the DOC measurements. No IC data was available for the NAAMES 3 Station 6 sample so the relative composition was assumed to be the same as the NAAMES 3 Station 1 sample. Adjusting the selected HMW compound used in predicting the SML κ values resulted in no significant difference in κ (5% significance level) (Figures 7A–C). The predicted κ values varied, at most, by ±0.01 across all of the organics chosen. The predicted κ values assuming each organic composition are shown in Table 4 along with the error calculated using error propagation analysis for the κ value prediction. The small variation between different HMW organic compositions was due to large concentration of salt present in the SML samples. The relatively small concentration of organic matter in these samples did not make a significant contribution due to the large concentration of salt.
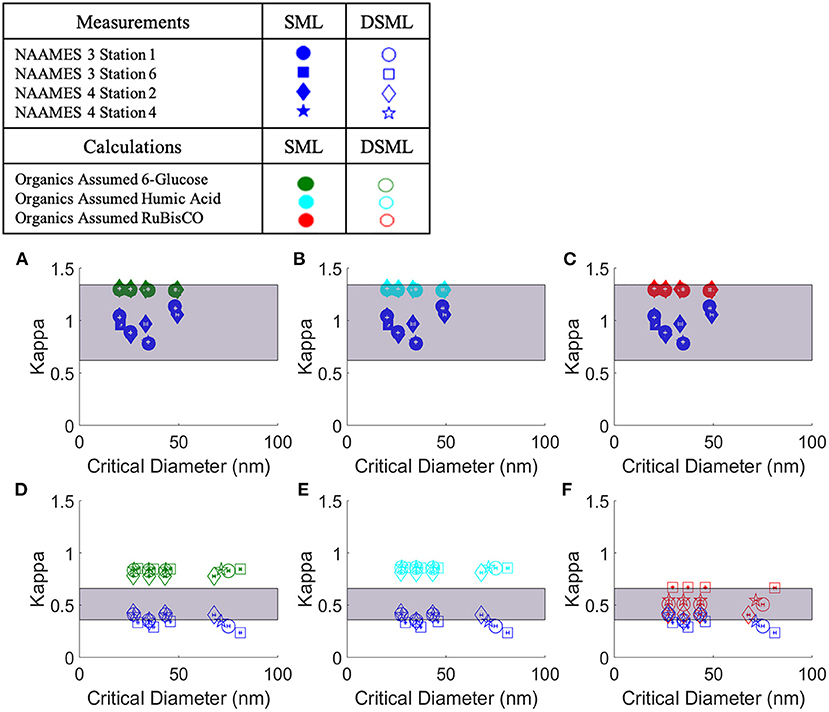
Figure 7. Measured and predicted κ values for SML and DSML samples using IC data for composition of salts. The different symbols represent the SML and DSML samples collected at different stations. The dark blue symbols indicate the κ values calculated from direct CCN measurements. κ values predicted by assuming a single organic composition of 6-glucose, humic acid, and RuBisCO are presented in green, light blue, and red symbols, respectively. (A–C) show the predicted κ values and the κ values from CCN measurements for the SML samples. The predicted κ values are also provided in Table 4. The white lines on each symbol show the error associated with that measurement which was determined using error propagation analysis. The gray box presents the uncertainty in the κ value for seawater salts, represented by NaCl (upper boundary, 1.34) and calcium sulfate (lower boundary, 0.62), both of which are components in seawater. (D–F) show the predicted κ values and the κ values from CCN measurements for the DSML samples. These κ values include the small amount of salt remaining after dialysis. Error bars were calculated using error propagation analysis. The purple box indicates a range of κ values calculated for the organics assuming the organic molecular weight, density, and number of carbons may independently vary by +50% relative to RuBisCO, as described in Table 5 (Cases 2–7).
When discussing aerosols generated in a marine environment, the κ values for the aerosol are often compared to the κ values of salts. The κ values for salts contained in a seawater mixture are shown in Table 3. The highest κ value for these compounds is sodium chloride (1.34) while the lowest κ value is for calcium sulfate (0.62). The range of κ values calculated for inorganics between sodium chloride and calcium sulfate are shown on Figures 7A–C in the purple shaded area. This provides a range of κ values that are reasonable for samples containing a high concentration of sea salts. The κ value for artificial seawater, 1.1, determined in one lab experiment is also reported (Zieger et al., 2017). By comparison, the κ values from the SML samples (0.96 ± 0.12) fall below sodium chloride and only slightly below the artificial seawater κ values but are above the calcium sulfate κ values. In a comparison of field and laboratory measurements, Collins et al. (2016) determined a similar mean κ value (0.95 ± 0.15), whereas Atwood et al. (2017) estimated a much lower marine κ value of 0.65 for the ambient atmosphere. The SML κ values calculated based on measurements in the present study were comparable to the uncertainty range of global marine κ values stated by the modeling study of Pringle et al. (2010) of 0.72 ± 0.24.
Figures 7D–F show the κ values calculated directly from the CCN measurements and the predicted κ values for the DSML samples. Error bars for each measurement are included on Figures 7D–F. The error was calculated using error propagation analysis which included error for different measurement and analysis techniques used in the κ value predictions. Compared to the untreated SML samples, DSML samples were less effective at forming clouds, with a mean κ value of 0.36 ± 0.05. The HMW organic composition which predicted κ values close to the measured DSML κ values was RuBisCO.
While the modeling results found the most representative single organic compound to be RuBisCO, a caveat of this study is the treatment of the organic material as a single compound. In the microlayer there are many different organic species present. In particular, there are many HMW organic compounds present in the ocean that may have similar characteristics to RuBisCO. Next, a thought experiment was conducted to evaluate the sensitivity of predicted κ values to changes in the organic's properties including molecular weight, density, and number of carbon atoms in the organic compound (Table 5). The reported κ values were calculated assuming that each parameter (molecular weight, density, number of carbon atoms) was varied independently, while the unchanged parameters in each test were assumed to have RuBisCO values. Therefore, the actual range in these κ values could be even larger. Decreasing the density by 50%, Case 4 in Table 5, or decreasing the number of carbon atoms in the organic compound by 50%, Case 6 in Table 5, produces predicted κ values that fall within the standard deviation of the κ values derived from CCN measurements. Case 8 used the properties of RuBisCO from Control Case 1 (same molecular weight, density, and number of carbon atoms) but assumed an enrichment in organic concentration from the SML. The original organic concentration measured in the SML sample was increased by a factor of 104 based on observations that DOC in aerosols can be enriched by as much as factor of 104 relative to the SML, even in low chlorophyll a conditions (Van Pinxteren et al., 2017). Case 8 shows how dramatically the organic concentration can influence the marine κ values if the organic concentration is high enough. Table 5 provides a range of κ values for potential organics that could improve the predicted κ values for the DSML samples.
The intercomparison between measured and predicted κ values in this study focused on HMW organic compounds. However, the impact of the estimated 10% of the LMW organic mass that remained within the desalted sampled could be substantial, particularly if its cloud forming potential is vastly different than the HMW material. However, marine LMW organic compounds including glucose, fulvic acid, triolein, and chlorophyll a all exhibit low κ values similar to the HMW organic compounds studied, and much lower than the salts (Table 3). While future work will consider additional marine organic compounds and internal mixtures of multiple organic compounds, the results of this study do not contain any evidence that the presence of organic materials enhances the cloud forming potential of marine sea spray.
Conclusions
The potential for organic surfactants produced in the ocean's surface waters to modulate the formation and properties of low-lying marine clouds has been suggested many times. Nevertheless, since ocean waters also contain high concentrations of salts, quantifying the influence of the organics on cloud-forming aerosol production has remained elusive. In this study, the cloud forming potential of aerosolized SML samples were evaluated before and after salts were removed from the samples to untangle the cloud forming properties of salts from those of organics.
By comparing the CCN activity of SML samples to that of DSML samples, the influence of organics on marine aerosol CCN activation was determined. The diameter a droplet must reach prior to becoming large enough to activate spontaneously as a cloud drop increased from a mean value of 48.6 ± 0.5 nm to 73.9 ± 5.7 nm and 20.3 ± 0.3 nm to 27.9 ± 1.0 nm at 0.32% SS and 1.24% SS respectively, following the desalting procedure in the SML and DSML samples, respectively. Removal of the salts reduced the κ values calculated using the CCN measurements by 62.5% ± 15.6%. The remaining, predominately organic samples, had low κ values consistent with weakly hygroscopic compositions. In contrast to some previous studies, the present study indicates that organics present in the samples will not enhance cloud droplet formation but rather suggest that aerosols with higher concentrations of organic materials relative to salts will have reduced ability to form cloud droplets.
Theoretical predictions of κ, which employed composition and surface tension as inputs, were largely driven by the concentration of salts. The predicted κ values for SML samples varied only from 1.29 ± 0.01, depending on the assumed molecular formula of the organic material. In all cases, these κ values predicted for the SML samples were higher than the κ values calculated from the CCN measurements. Since the organics contributed such a small proportion of the aerosol mass, their effect was negligible and therefore, variation in the composition of the organic matter in the aerosols did not make a significant difference in estimates of the SML κ values.
In contrast to the untreated SML, once the salts were removed, variations in cloud potential were sensitive to the different organic compounds assumed. Assuming the organic material was RuBisCO resulted in predicted the κ values (0.53 ± 0.10) most closely matched to the measured DSML results. The κ values calculated for the DSML samples using the CCN measurements were 0.36 ± 0.05. Even in the DSML samples, which had a reduced salt concentration of >99%, the concentration of salt remaining after the desalting process exhibited a strong influence on the κ values for the aerosols generated by the DSML samples. The κ for pure RuBisCO assuming no other salts or organics are present is 5.16E-05 (Table 3). This is significantly smaller than the predicted κ values for the DSML samples containing the organic RuBisCO and the remaining salt after desalting, 0.53 ± 0.10, indicating the salt concentration is capable of raising the κ value significantly. While using RuBisCO as the representative organic provided the closest predicted κ values compared to the κ values calculated using CCN data, the actual organic composition in the microlayer would contain multiple types of organics. Selecting a singular organic compound is an oversimplification of the microlayer organic matter composition used to evaluate how organic matter characteristics can potentially alter κ values for marine CCN. This study revealed that salt overwhelmingly controls the κ values of marine clouds. Additionally, the organic matter composition in marine CCN serves to reduce cloud forming potential of marine aerosols.
Data Availability Statement
The datasets generated for this study can be found in online repositories. The names of the repository/repositories and accession number(s) can be found at: NASA Atmospheric Science Data Center (ASDC; https://doi.org/10.5067/Suborbital/NAAMES/DATA001) SeaWiFS Bio-Optical Archive and Storage System (SeaBASS; https://doi.org/10.5067/SeaBASS/NAAMES/DATA001).
Author Contributions
BH and SB designed the project and led the writing of the manuscript. SML sample collection was performed by BH, JM, AA, NB, and CC. The desalting process of the SML samples was performed with the guidance of DCOT in the Department of Oceanography at Texas A&M University. Measurements of the SML were conducted at the NASA Langley Research Center with help from RM, EC, and LZ. All authors contributed to the article and approved the submitted version.
Funding
Funding for this project was provided by the NSF Atmospheric Chemistry Program, Award #1539881 to SB and DCOT. Funding for sample collection was provided by NASA Earth Venture Suborbital-2 (EVS-2) Award #NNX15AE68G and NSF OCE-157943 to CC.
Conflict of Interest
The authors declare that the research was conducted in the absence of any commercial or financial relationships that could be construed as a potential conflict of interest.
Acknowledgments
Thanks to Thomas Bell at the Plymouth Marine Laboratory for providing the SML sampling screen, and thanks to Thomas Bell and Brandon Stephens at the University of California, Santa Barbara for assisting in SML sample collection.
Supplementary Material
The Supplementary Material for this article can be found online at: https://www.frontiersin.org/articles/10.3389/fmars.2020.596225/full#supplementary-material
References
Alldredge, A. L., Passow, U., and Logan, B. E. (1993). The abundance and significance of a class of large, transparent organice particles in the ocean. Deep Sea Res. Part I Oceanogr. Res. Papers 40, 1131–1140. doi: 10.1016/0967-0637(93)90129-Q
Aller, J. Y., Radway, J. C., Kilthau, W. P., Bothe, D. W., Wilson, T. W., Vaillancourt, R. D., et al. (2017). Size-resolved characterization of the polysaccharidic and proteinaceous components of sea spray aerosol. Atmos. Environ. 154, 331–347. doi: 10.1016/j.atmosenv.2017.01.053
Amon, R. M. W., and Benner, R. (1996). Bacterial utilization of different size classes of dissolved organic matter. Limnol. Oceanogr. 41, 41–51. doi: 10.4319/lo.1996.41.1.0041
Andreae, M. O. (1990). Ocean-atmosphere interactions in the global biogeochemical sulfur cycle. Mar. Chem. 30, 1–29. doi: 10.1016/0304-4203(90)90059-L
Atwood, S. A., Reid, J. S., Kreidenweis, S. M., Blake, D. R., Jonsson, H. H., Lagrosas, N. D., et al. (2017). Size-resolved aerosol and cloud condensation nuclei (CCN) properties in the remote marine South China Sea - Part 1: Observations and source classification. Atmos. Chem. Phys. 17, 1105–1123. doi: 10.5194/acp-17-1105-2017
Baetge, N., Graff, J. R., Behrenfeld, M. J., and Carlson, C. A. (2020). Net community production, dissolved organic carbon accumulation, and vertical export in the Western North Atlantic. Front. Marine Sci. 7:16. doi: 10.3389/fmars.2020.00227
Bar-On, Y. M., and Milo, R. (2019). The global mass and average rate of rubisco. Proc. Natl. Acad. Sci. U.S.A. 116, 4738–4743. doi: 10.1073/pnas.1816654116
Bates, T. S., Quinn, P. K., Coffman, D. J., Johnson, J. E., Upchurch, L., Saliba, G., et al. (2020). Variability in marine plankton ecosystems are not observed in freshly emitted sea spray aerosol over the North Atlantic Ocean. Geophys. Res. Lett. 47:e2019GL085938. doi: 10.1029/2019GL085938
Beaupre, S. R., Kieber, D. J., Keene, W. C., Long, M. S., Maben, J. R., Lu, X., et al. (2019). Oceanic efflux of ancient marine dissolved organic carbon in primary marine aerosol. Sci. Adv. 5:9. doi: 10.1126/sciadv.aax6535
Behrenfeld, M. J., Moore, R. H., Hostetler, C. A., Graff, J., Gaube, P., Russell, L. M., et al. (2019). The North Atlantic Aerosol and Marine Ecosystem Study (NAAMES): science motive and mission overview. Front. Marine Sci. 6:122. doi: 10.3389/fmars.2019.00122
Benner, R. (2002). “Chemical composition and reactivity,” in Biogeochemistry of Marine Dissolved Organic Matter, eds D. A. Hansell, and C. A. Carlson (San Diego, CA: Academic Press), 59–90. doi: 10.1016/B978-012323841-2/50005-1
Benner, R., and Amon, R. M. W. (2015). “The size-reactivity continuum of major bioelements in the ocean,” in Annual Review of Marine Science, Vol. 7, eds C. A. Carlson and S. J. Giovannoni (Palo Alto, CA: Annual Reviews), 185–205.
Benner, R., Pakulski, J. D., McCarthy, M., Hedges, J. I., and Hatcher, P. G. (1992). Bulk chemical characteristics of dissolved organic-matter in the ocean. Science 255, 1561–1564. doi: 10.1126/science.255.5051.1561
Brooks, S. D., and Thornton, D. C. O. (2018). Marine aerosols and clouds. Ann. Rev. Mar. Sci 10, 289–313. doi: 10.1146/annurev-marine-121916-063148
Burrows, S. M., Gobrogge, E., Fu, L., Link, K., Elliott, S. M., Wang, H. F., et al. (2016). OCEANFILMS-2: representing coadsorption of saccharides in marine films and potential impacts on modeled marine aerosol chemistry. Geophys. Res. Lett. 43, 8306–8313. doi: 10.1002/2016GL069070
Bzdek, B. R., Reid, J. P., Malila, J., and Prisle, N. L. (2020). The surface tension of surfactant-containing, finite volume droplets. Proc. Natl. Acad. Sci. U.S.A. 117, 8335–8343. doi: 10.1073/pnas.1915660117
Carlson, C. A. (2002). “Chapter 4 - Production and removal processes,” in Biogeochemistry of Marine Dissolved Organic Matter, eds D. A. Hansell and C. A. Carlson (San Diego, CA: Academic Press), 91–151.
Carlson, C. A., Ducklow, H. W., Hansell, D. A., and Smith, W. O. (1998). Organic carbon partitioning during spring phytoplankton blooms in the Ross Sea polynya and the Sargasso Sea. Limnol. Oceanogr. 43, 375–386. doi: 10.4319/lo.1998.43.3.0375
Carlson, C. A., and Hansell, D. A. (2015). “Chapter 3 - DOM sources, sinks, reactivity, and budgets,” in Biogeochemistry of Marine Dissolved Organic Matter, 2nd Edn., eds D. A. Hansell and C. A. Carlson (Boston, MA: Academic Press), 65–126.
Carlson, C. A., Hansell, D. A., Nelson, N. B., Siegel, D. A., Smethie, W. M., Khatiwala, S., et al. (2010). Dissolved organic carbon export and subsequent remineralization in the mesopelagic and bathypelagic realms of the North Atlantic basin. Deep Sea Res. Part II Top. Stud. Oceanogr. 57, 1433–1445. doi: 10.1016/j.dsr2.2010.02.013
Carslaw, K. S., Lee, L. A., Reddington, C. L., Pringle, K. J., Rap, A., Forster, P. M., et al. (2013). Large contribution of natural aerosols to uncertainty in indirect forcing. Nature 503:67. doi: 10.1038/nature12674
Charlson, R. J., Lovelock, J. E., Andreae, M. O., and Warren, S. G. (1987). Oceanic phytoplankton, atmospheric sulfur, cloud albedo and climate. Nature 326, 655–661. doi: 10.1038/326655a0
Chen, Q. J., Sherwen, T., Evans, M., and Alexander, B. (2018). DMS oxidation and sulfur aerosol formation in the marine troposphere: a focus on reactive halogen and multiphase chemistry. Atmos. Chem. Phys. 18, 13617–13637. doi: 10.5194/acp-18-13617-2018
Chingin, K., Yan, R. H., Zhong, D. C., and Chen, H. W. (2018). Enrichment of surface-active compounds in bursting bubble aerosols. Acs Omega 3, 8709–8717. doi: 10.1021/acsomega.8b01157
Christiansen, S., Ickes, L., Bulatovic, I., Leck, C., Murray, B. J., Bertram, A. K., et al. (2020). Influence of arctic microlayers and algal cultures on sea spray hygroscopicity and the possible implications for mixed-phase clouds. J. Geophys. Res. Atmos. 125:16. doi: 10.1029/2020JD032808
Christiansen, S., Salter, M. E., Gorokhova, E., Nguyen, Q. T., and Bilde, M. (2019). Sea spray aerosol formation: laboratory results on the role of air entrainment, water temperature, and phytoplankton biomass. Environ. Sci. Technol. 53, 13107–13116. doi: 10.1021/acs.est.9b04078
Cochran, R. E., Laskina, O., Jayarathne, T., Laskin, A., Laskin, J., Lin, P., et al. (2016). Analysis of organic anionic surfactants in fine and coarse fractions of freshly emitted sea spray aerosol. Environ. Sci. Technol. 50, 2477–2486. doi: 10.1021/acs.est.5b04053
Cochran, R. E., Laskina, O., Trueblood, J. V., Estillore, A. D., Morris, H. S., Jayarathne, T., et al. (2017). Molecular diversity of sea spray aerosol particles: impact of ocean biology on particle composition and hygroscopicity. Chem 2, 655–667. doi: 10.1016/j.chempr.2017.03.007
Collins, D., Zhao, D., Ruppel, M., Laskina, O., Grandquist, J., Modini, R., et al. (2014). Direct aerosol chemical composition measurements to evaluate the physicochemical differences between controlled sea spray aerosol generation schemes. Atmos. Measure. Tech. 7, 3667–3683. doi: 10.5194/amt-7-3667-2014
Collins, D. B., Bertram, T. H., Sultana, C. M., Lee, C., Axson, J. L., and Prather, K. A. (2016). Phytoplankton blooms weakly influence the cloud forming ability of sea spray aerosol. Geophys. Res. Lett. 43, 9975–9983. doi: 10.1002/2016GL069922
Cunliffe, M., Engel, A., Frka, S., Gasparovic, B., Guitart, C., Murrell, J. C., et al. (2013). Sea surface microlayers: a unified physicochemical and biological perspective of the air-ocean interface. Prog. Oceanogr. 109, 104–116. doi: 10.1016/j.pocean.2012.08.004
Dai, W., Chen, M. Y., Myers, C., Ludtke, S. J., Pettitt, B. M., King, J. A., et al. (2018). Visualizing individual RuBisCO and its assembly into carboxysomes in marine cyanobacteria by cryo-electron tomography. J. Mol. Biol. 430, 4156–4167. doi: 10.1016/j.jmb.2018.08.013
Djikaev, Y. S., and Ruckenstein, E. (2014). Thermodynamics of water condensation on a primary marine aerosol coated by surfactant organic molecules. J. Phys. Chem. A 118, 9879–9889. doi: 10.1021/jp505578a
Dreshchinskii, A., and Engel, A. (2017). Seasonal variations of the sea surface microlayer at the Boknis Eck Times Series Station (Baltic Sea). J. Plankton Res. 39, 943–961. doi: 10.1093/plankt/fbx055
Drozdowska, V., Kowalczuk, P., Konik, M., and Dzietzbicka-Glowacka, L. (2018). Study on different fractions of organic molecules in the baltic sea surface microlayer by spectrophoto- and spectrofluorimetric methods. Front. Marine Sci. 5:12. doi: 10.3389/fmars.2018.00456
Drozdowska, V., Wrobel, I., Markuszewski, P., Makuch, P., Raczkowska, A., and Kowalczuk, P. (2017). Study on organic matter fractions in the surface microlayer in the Baltic Sea by spectrophotometric and spectrofluorometric methods. Ocean Sci. 13, 633–647. doi: 10.5194/os-13-633-2017
Duce, R. A., Mohnen, V. A., Zimmerman, P. R., Grosjean, D., Cautreels, W., Chatfield, R., et al. (1983). Organic material in the global troposphere. Rev. Geophys. 21, 921–952. doi: 10.1029/RG021i004p00921
Ellis, R. J. (1979). Most abundant protein in the world. Trends Biochem. Sci. 4, 241–244. doi: 10.1016/0968-0004(79)90212-3
Engel, A., Bange, H. W., Cunliffe, M., Burrows, S. M., Friedrichs, G., Galgani, L., et al. (2017). The ocean's vital skin: toward an integrated understanding of the sea surface microlayer. Front. Marine Sci. 4:165. doi: 10.3389/fmars.2017.00165
Engel, A., and Galgani, L. (2016). The organic sea-surface microlayer in the upwelling region off the coast of Peru and potential implications for air-sea exchange processes. Biogeosciences 13, 989–1007. doi: 10.5194/bg-13-989-2016
Engel, A., and Händel, N. (2011). A novel protocol for determining the concentration and composition of sugars in particulate and in high molecular weight dissolved organic matter (HMW-DOM) in seawater. Mar. Chem. 127, 180–191. doi: 10.1016/j.marchem.2011.09.004
Facchini, M. C., Rinaldi, M., Decesari, S., Carbone, C., Finessi, E., Mircea, M., et al. (2008). Primary submicron marine aerosol dominated by insoluble organic colloids and aggregates. Geophys. Res. Lett. 35:5. doi: 10.1029/2008GL034210
Frossard, A. A., Gerard, V., Duplessis, P., Kinsey, J. D., Lu, X., Zhu, Y. T., et al. (2019). Properties of seawater surfactants associated with primary marine aerosol particles produced by bursting bubbles at a model air-sea interface. Environ. Sci. Technol. 53, 9407–9417. doi: 10.1021/acs.est.9b02637
Frossard, A. A., Russell, L. M., Burrows, S. M., Elliott, S. M., Bates, T. S., and Quinn, P. K. (2014). Sources and composition of submicron organic mass in marine aerosol particles. J. Geophys. Res. Atmos. 119, 12977–13003. doi: 10.1002/2014JD021913
Fuentes, E., Coe, H., Green, D., Leeuw, G. D., and McFiggans, G. (2010). Laboratory-generated primary marine aerosol via bubble-bursting and atomization. Atmos. Measure. Tech. 3, 141–162. doi: 10.5194/amt-3-141-2010
Fuentes, E., Coe, H., Green, D., and McFiggans, G. (2011). On the impacts of phytoplankton-derived organic matter on the properties of the primary marine aerosol - Part 2: composition, hygroscopicity and cloud condensation activity. Atmos. Chem. Phys. 11, 2585–2602. doi: 10.5194/acp-11-2585-2011
Galachyants, A. D., Bel'kova, N. L., Sukhanova, E. V., Blinov, V. V., and Parfenova, V. V. (2016). Methods of neuston sampling for the quantitative characteristic of microbial communities of Lake Baikal. Inland Water Biol. 9, 329–336. doi: 10.1134/S1995082916030068
Gantt, B., and Meskhidze, N. (2013). The physical and chemical characteristics of marine primary organic aerosol: a review. Atmos. Chem. Phys. 13, 3979–3996. doi: 10.5194/acp-13-3979-2013
Garrett, W. D. (1965). Collection of slick-forming materials from the sea surface. Limnol. Oceanogr. 10, 602–605. doi: 10.4319/lo.1965.10.4.0602
Gaston, C. J., Cahill, J. F., Collins, D. B., Suski, K. J., Ge, J. Y., Barkley, A. E., et al. (2018). The cloud nucleating properties and mixing state of marine aerosols sampled along the Southern California Coast. Atmosphere 9:16. doi: 10.3390/atmos9020052
Goldberg, S. J., Carlson, C. A., Brzezinski, M., Nelson, N. B., and Siegel, D. A. (2011). Systematic removal of neutral sugars within dissolved organic matter across ocean basins. Geophys. Res. Lett. 38:7. doi: 10.1029/2011GL048620
Gross, S., Freudenthaler, V., Schepanski, K., Toledano, C., Schafler, A., Ansmann, A., et al. (2015). Optical properties of long-range transported Saharan dust over Barbados as measured by dual-wavelength depolarization Raman lidar measurements. Atmos. Chem. Phys. 15, 11067–11080. doi: 10.5194/acp-15-11067-2015
Hansell, D. A. (2005). Dissolved organic carbon reference material program. Eos Trans. Am. Geophys. Union 86, 318–318. doi: 10.1029/2005EO350003
Hansell, D. A. (2013). “Recalcitrant dissolved organic carbon fractions,” in Annual Review of Marine Science, Vol. 5, eds C. A. Carlson and S. J. Giovannoni (Palo Alto, CA: Annual Reviews), 421–445.
Hansell, D. A., and Carlson, C. A. (2014). Biogeochemistry of Marine Dissolved Organic Matter. San Diego, CA: Academic Press.
Hansell, D. A., Carlson, C. A., Repeta, D. J., and Schlitzer, R. (2009). Dissolved organic matter in the ocean a controversy stimulates new insights. Oceanography 22, 202–211. doi: 10.5670/oceanog.2009.109
Hedges, J. I. (2002). “Chapter 1 - Why dissolved organics matter,” in Biogeochemistry of Marine Dissolved Organic Matter, eds D. A. Hansell and C. A. Carlson (San Diego, CA: Academic Press), 1–33.
Held, I. M., and Soden, B. J. (2000). Water vapor feedback and global warming. Annu. Rev. Energy Environ. 25, 441–475. doi: 10.1146/annurev.energy.25.1.441
Herich, H., Kammermann, L., Friedman, B., Gross, D. S., Weingartner, E., Lohmann, U., et al. (2009). Subarctic atmospheric aerosol composition: 2. Hygroscopic growth properties. J. Geophys. Res. Atmos. 114:14. doi: 10.1029/2008JD011574
Ickes, L., Porter, G. C. E., Wagner, R., Adams, M. P., Bierbauer, S., Bertram, A. K., et al. (2020). The ice-nucleating activity of Arctic sea surface microlayer samples and marine algal cultures. Atmos. Chem. Phys. 20, 11089–11117. doi: 10.5194/acp-20-11089-2020
IPCC (2014). IPCC, 2014: Climate Change 2014: Synthesis Report. Contribution of Working Groups I, II and III to the Fifth Assessment Report of the Intergovernmental Panel on Climate Change, ed R. K. P. a. L. A. Meyer. Geneva.
Irish, V. E., Elizondo, P., Chen, J., Chou, C., Charette, J., Lizotte, M., et al. (2017). Ice-nucleating particles in Canadian Arctic sea-surface microlayer and bulk seawater. Atmos. Chem. Phys. 17, 10583–10595. doi: 10.5194/acp-17-10583-2017
Irish, V. E., Hanna, S. J., Yu, X., Boyer, M., Polishchuk, E., Ahmed, M., et al. (2019). Revisiting properties and concentrations of ice-nucleating particles in the sea surface microlayer and bulk seawater in the Canadian Arctic during summer. Atmos. Chem. Phys. 19, 7775–7787. doi: 10.5194/acp-19-7775-2019
Jayarathne, T., Sultana, C. M., Lee, C., Malfatti, F., Cox, J. L., Pendergraft, M. A., et al. (2016). Enrichment of saccharides and divalent cations in sea spray aerosol during two phytoplankton blooms. Environ. Sci. Technol. 50, 11511–11520. doi: 10.1021/acs.est.6b02988
Jenkinson, I. R., Seuront, L., Ding, H. B., and Elias, F. (2018). Biological modification of mechanical properties of the sea surface microlayer, influencing waves, ripples, foam and air-sea fluxes. Elementa Sci. Anthropocene 6:32. doi: 10.1525/elementa.283
Kaiser, K., and Benner, R. (2009). Biochemical composition and size distribution of organic matter at the Pacific and Atlantic time-series stations. Mar. Chem. 113, 63–77. doi: 10.1016/j.marchem.2008.12.004
Käse, L., and Geuer, J. K. (2018). Phytoplankton Responses to Marine Climate Change – An Introduction. Cham: Springer International Publishing.
Köhler, H. (1936). The nucleus in and the growth of hygroscopic droplets. Trans. Faraday Soc. 32, 1152–1161. doi: 10.1039/TF9363201152
Kristensen, T. B., Muller, T., Kandler, K., Benker, N., Hartmann, M., Prospero, J. M., et al. (2016). Properties of cloud condensation nuclei (CCN) in the trade wind marine boundary layer of the western North Atlantic. Atmos. Chem. Phys. 16, 2675–2688. doi: 10.5194/acp-16-2675-2016
Kubicki, S., Bollinger, A., Katzke, N., Jaeger, K. E., Loeschcke, A., and Thies, S. (2019). Marine biosurfactants: biosynthesis, structural diversity and biotechnological applications. Mar. Drugs 17:30. doi: 10.3390/md17070408
Kujawinski, E. B. (2011). “The impact of microbial metabolism on marine dissolved organic matter,” in Annual Review of Marine Science, Vol. 3, eds C. A. Carlson and S. J. Giovannoni (Palo Alto, CA: Annual Reviews), 567–599.
Kuznetsova, M., and Lee, C. (2002). Dissolved free and combined amino acids in nearshore seawater, sea surface microlayers and foams: influence of extracellular hydrolysis. Aquat. Sci 64, 252–268. doi: 10.1007/s00027-002-8070-0
Kuznetsova, M., Lee, C., and Aller, J. (2005). Characterization of the proteinaceous matter in marine aerosols. Mar. Chem. 96, 359–377. doi: 10.1016/j.marchem.2005.03.007
Lawler, M. J., Lewis, S. L., Russell, L. M., Quinn, P. K., Bates, T. S., Coffman, D. J., et al. (2020). North Atlantic marine organic aerosol characterized by novel offline thermal desorption mass spectrometry approach: polysaccharides, recalcitrant material, secondary organics. Atmos. Chem. Phys. Discuss. 2020, 1–28. doi: 10.5194/acp-2020-562-supplement
Lee, C., Sultana, C. M., Collins, D. B., Santander, M. V., Axson, J. L., Malfatti, F., et al. (2015). Advancing model systems for fundamental laboratory studies of sea spray aerosol using the microbial loop. J. Phys. Chem. A 119, 8860–8870. doi: 10.1021/acs.jpca.5b03488
Lin, L. J. J., Kristensen, T. B. B., Calderon, S. M. M., Malila, J., and Prisle, N. L. (2020). Effects of surface tension time-evolution for CCN activation of a complex organic surfactant. Environ. Sci. Processes Impacts 22, 271–284. doi: 10.1039/C9EM00426B
Liss, P., and Duce, R. (eds.). (1997). The Sea Surface and Global Change. Cambridge: Cambridge University Press. doi: 10.1017/CBO9780511525025
Lohmann, U., Lüönd, F., and Mahrt, F. (2016). An Introduction to Clouds: From the Microscale to Climate. Cambridge: Cambridge University Press.
Lowe, S. J., Partridge, D. G., Davies, J. F., Wilson, K. R., Topping, D., and Riipinen, I. (2019). Key drivers of cloud response to surface-active organics. Nat. Commun. 10:5214. doi: 10.1038/s41467-019-12982-0
Luo, M., Dommer, A. C., Schiffer, J. M., Rez, D. J., Mitchell, A. R., Amaro, R. E., et al. (2019). Surfactant charge modulates structure and stability of lipase-embedded monolayers at marine-relevant aerosol surfaces. Langmuir 35, 9050–9060. doi: 10.1021/acs.langmuir.9b00689
Ma, Y., Brooks, S. D., Vidaurre, G., Khalizov, A. F., Wang, L., and Zhang, R. Y. (2013). Rapid modification of cloud-nucleating ability of aerosols by biogenic emissions. Geophys. Res. Lett. 40, 6293–6297. doi: 10.1002/2013GL057895
Mackinnon, M. D. (1981). “Chapter 14: The measurement of organic carbon in sea Water11NRCC No. 16920,” in Elsevier Oceanography Series, eds E. K. Duursma and R. Dawson (New York, NY: Elsevier), 415–443.
Markelj, J., Madronich, S., and Pompe, M. (2017). Modeling of hygroscopicity parameter kappa of organic aerosols using quantitative structure-property relationships. J. Atmos. Chem. 74, 357–376. doi: 10.1007/s10874-016-9347-3
Mason, R., Si, M., Chou, C., Irish, V., Dickie, R., Elizondo, P., et al. (2016). Size-resolved measurements of ice-nucleating particles at six locations in North America and one in Europe. Atmos. Chem. Phys. 16:1637. doi: 10.5194/acp-16-1637-2016
McCluskey, C. S., Hill, T. C., Malfatti, F., Sultana, C. M., Lee, C., Santander, M. V., et al. (2017). A dynamic link between ice nucleating particles released in nascent sea spray aerosol and oceanic biological activity during two mesocosm experiments. J. Atmos. Sci. 74, 151–166. doi: 10.1175/JAS-D-16-0087.1
Miyazaki, Y., Yamashita, Y., Kawana, K., Tachibana, E., Kagami, S., Mochida, M., et al. (2018). Chemical transfer of dissolved organic matter from surface seawater to sea spray water-soluble organic aerosol in the marine atmosphere. Sci. Rep. 8:10. doi: 10.1038/s41598-018-32864-7
Modini, R. L., Harris, B., and Ristovski, Z. D. (2010). The organic fraction of bubble-generated, accumulation mode Sea Spray Aerosol (SSA). Atmos. Chem. Phys. 10, 2867–2877. doi: 10.5194/acp-10-2867-2010
Moore, M. J. K., Furutani, H., Roberts, G. C., Moffet, R. C., Gilles, M. K., Palenik, B., et al. (2011). Effect of organic compounds on cloud condensation nuclei (CCN) activity of sea spray aerosol produced by bubble bursting. Atmos. Environ. 45, 7462–7469. doi: 10.1016/j.atmosenv.2011.04.034
Moore, R. H., Bahreini, R., Brock, C. A., Froyd, K. D., Cozic, J., Holloway, J. S., et al. (2011). Hygroscopicity and composition of Alaskan Arctic CCN during April 2008. Atmos. Chem. Phys. 11, 11807–11825. doi: 10.5194/acp-11-11807-2011
Moore, R. H., Cerully, K., Bahreini, R., Brock, C. A., Middlebrook, A. M., and Nenes, A. (2012). Hygroscopicity and composition of California CCN during summer 2010. J. Geophys. Res. Atmos. 117:14. doi: 10.1029/2011JD017352
Moore, R. H., Ingall, E. D., Sorooshian, A., and Nenes, A. (2008). Molar mass, surface tension, and droplet growth kinetics of marine organics from measurements of CCN activity. Geophys. Res. Lett. 35:5. doi: 10.1029/2008GL033350
Moore, R. H., Nenes, A., and Medina, J. (2010). Scanning mobility CCN analysis-A method for fast measurements of size-resolved CCN distributions and activation kinetics. Aerosol Sci. Technol. 44, 861–871. doi: 10.1080/02786826.2010.498715
Morcos, S. A. (1970). Chemical composition of seawater and the variation of calcium and alkalinity. ICES J. Marine Sci. 33, 126–133. doi: 10.1093/icesjms/33.2.126
Noziere, B., Baduel, C., and Jaffrezo, J.-L. (2014). The dynamic surface tension of atmospheric aerosol surfactants reveals new aspects of cloud activation. Nat. Commun. 5, 2–3. doi: 10.1038/ncomms4335
O'Dowd, C., Ceburnis, D., Ovadnevaite, J., Bialek, J., Stengel, D. B., Zacharias, M., et al. (2015). Connecting marine productivity to sea-spray via nanoscale biological processes: phytoplankton Dance or Death Disco? Sci. Rep. 5:11. doi: 10.1038/srep14883
O'Dowd, C. D., Facchini, M. C., Cavalli, F., Ceburnis, D., Mircea, M., Decesari, S., et al. (2004). Biogenically driven organic contribution to marine aerosol. Nature 431, 676–680. doi: 10.1038/nature02959
Orellana, M. V., and Hansell, D. A. (2012). Ribulose-1,5-bisphosphate carboxylase/oxygenase (RuBisCO): a long-lived protein in the deep ocean. Limnol. Oceanogr. 57, 826–834. doi: 10.4319/lo.2012.57.3.0826
Orellana, M. V., Matrai, P. A., Leck, C., Rauschenberg, C. D., Lee, A. M., and Coz, E. (2011). Marine microgels as a source of cloud condensation nuclei in the high Arctic. Proc. Natl. Acad. Sci. U.S.A. 108, 13612–13617. doi: 10.1073/pnas.1102457108
Ovadnevaite, J., Ceburnis, D., Martucci, G., Bialek, J., Monahan, C., Rinaldi, M., et al. (2011). Primary marine organic aerosol: a dichotomy of low hygroscopicity and high CCN activity. Geophys. Res. Lett. 38:5. doi: 10.1029/2011GL048869
Ovadnevaite, J., Zuend, A., Laaksonen, A., Sanchez, K. J., Roberts, G., Ceburnis, D., et al. (2017). Surface tension prevails over solute effect in organic-influenced cloud droplet activation. Nature 546, 637–641. doi: 10.1038/nature22806
Passow, U. (2002). Transparent exopolymer particles (TEP) in aquatic environments. Prog. Oceanogr. 55, 287–333. doi: 10.1016/S0079-6611(02)00138-6
Petters, M. D., and Kreidenweis, S. M. (2007). A single parameter representation of hygroscopic growth and cloud condensation nucleus activity. Atmos. Chem. Phys. 7, 1961–1971. doi: 10.5194/acp-7-1961-2007
Phillips, B. N., Royalty, T. M., Dawson, K. W., Reed, R., Petters, M. D., and Meskhidze, N. (2018). Hygroscopicity- and size-resolved measurements of submicron aerosol on the east coast of the United States. J. Geophys. Res. Atmos. 123, 1826–1839. doi: 10.1002/2017JD027702
Prather, K. A., Bertram, T. H., Grassian, V. H., Deane, G. B., Stokes, M. D., DeMott, P. J., et al. (2013). Bringing the ocean into the laboratory to probe the chemical complexity of sea spray aerosol. Proc. Natl. Acad. Sci. U.S.A. 110, 7550–7555. doi: 10.1073/pnas.1300262110
Pringle, K. J., Tost, H., Pozzer, A., Poschl, U., and Lelieveld, J. (2010). Global distribution of the effective aerosol hygroscopicity parameter for CCN activation. Atmos. Chem. Phys. 10, 5241–5255. doi: 10.5194/acp-10-5241-2010
Quinn, P. K., and Bates, T. S. (2011). The case against climate regulation via oceanic phytoplankton sulphur emissions. Nature 480, 51–56. doi: 10.1038/nature10580
Quinn, P. K., Bates, T. S., Schulz, K. S., Coffman, D. J., Frossard, A. A., Russell, L. M., et al. (2014). Contribution of sea surface carbon pool to organic matter enrichment in sea spray aerosol. Nat. Geosci 7, 228–232. doi: 10.1038/ngeo2092
Quinn, P. K., Collins, D. B., Grassian, V. H., Prather, K. A., and Bates, T. S. (2015). Chemistry and related properties of freshly emitted sea spray aerosol. Chem. Rev. 115, 4383–4399. doi: 10.1021/cr500713g
Rasmussen, B. B., Nguyen, Q. T., Kristensen, K., Nielsen, L. S., and Bilde, M. (2017). What controls volatility of sea spray aerosol? Results from laboratory studies using artificial and real seawater samples. J. Aerosol Sci. 107, 134–141. doi: 10.1016/j.jaerosci.2017.02.002
Rastelli, E., Corinaldesi, C., Dell'Anno, A., Martire, M. L., Greco, S., Facchini, M. C., et al. (2017). Transfer of labile organic matter and microbes from the ocean surface to the marine aerosol: an experimental approach. Sci. Rep. 7, 1–10. doi: 10.1038/s41598-017-10563-z
Rees, A. P. (2012). Pressures on the marine environment and the changing climate of ocean biogeochemistry. Philos. Trans. R. Soc. A Math. Phys. Eng. Sci. 370, 5613–5635. doi: 10.1098/rsta.2012.0399
Reinthaler, T., Sintes, E., and Herndl, G. J. (2008). Dissolved organic matter and bacterial production and respiration in the sea-surface microlayer of the open Atlantic and the western Mediterranean Sea. Limnol. Oceanogr. 53, 122–136. doi: 10.4319/lo.2008.53.1.0122
Rogers, R. R., and Yau, M. K. (1989). A Short Course in Cloud Physics. Burlington, MA: Butterworth Heinemann.
Rose, D., Gunthe, S. S., Mikhailov, E., Frank, G. P., Dusek, U., Andreae, M. O., et al. (2008). Calibration and measurement uncertainties of a continuous-flow cloud condensation nuclei counter (DMT-CCNC): CCN activation of ammonium sulfate and sodium chloride aerosol particles in theory and experiment. Atmos. Chem. Phys. 8, 1153–1179. doi: 10.5194/acp-8-1153-2008
Rose, D., Nowak, A., Achtert, P., Wiedensohler, A., Hu, M., Shao, M., et al. (2010). Cloud condensation nuclei in polluted air and biomass burning smoke near the mega-city Guangzhou, China - Part 1: size-resolved measurements and implications for the modeling of aerosol particle hygroscopicity and CCN activity. Atmos. Chem. Phys. 10, 3365–3383. doi: 10.5194/acp-10-3365-2010
Russell, L. M., Hawkins, L. N., Frossard, A. A., Quinn, P. K., and Bates, T. S. (2010). Carbohydrate-like composition of submicron atmospheric particles and their production from ocean bubble bursting. Proc. Natl. Acad. Sci. U.S.A. 107, 6652–6657. doi: 10.1073/pnas.0908905107
Sabbaghzadeh, B., Upstill-Goddard, R. C., Beale, R., Pereira, R., and Nightingale, P. D. (2017). The Atlantic Ocean surface microlayer from 50 degrees N to 50 degrees S is ubiquitously enriched in surfactants at wind speeds up to 13ms(-1). Geophys. Res. Lett. 44, 2852–2858. doi: 10.1002/2017GL072988
Sanchez, K. J., Chen, C. L., Russell, L. M., Betha, R., Liu, J., Price, D. J., et al. (2018). Substantial seasonal contribution of observed biogenic sulfate particles to cloud condensation nuclei. Sci. Rep. 8:14. doi: 10.1038/s41598-018-21590-9
Schill, S. R., Burrows, S. M., Hasenecz, E. S., Stone, E. A., and Bertram, T. H. (2018). The impact of divalent cations on the enrichment of soluble saccharides in primary sea spray aerosol. Atmosphere 9:17. doi: 10.3390/atmos9120476
Schill, S. R., Collins, D. B., Lee, C., Morris, H. S., Novak, G. A., Prather, K. A., et al. (2015). The impact of aerosol particle mixing state on the hygroscopicity of sea spray aerosol. Acs Central Science 1, 132–141. doi: 10.1021/acscentsci.5b00174
Schwier, A. N., Sellegri, K., Mas, S., Charriere, B., Pey, J., Rose, C., et al. (2017). Primary marine aerosol physical flux and chemical composition during a nutrient enrichment experiment in mesocosms in the Mediterranean Sea. Atmos. Chem. Phys. 17, 14645–14660. doi: 10.5194/acp-17-14645-2017
Skoog, A., and Benner, R. (1997). Aldoses in various size fractions of marine organic matter: Implications for carbon cycling. Limnol. Oceanogr. 42, 1803–1813. doi: 10.4319/lo.1997.42.8.1803
Sorjamaa, R., Svenningsson, B., Raatikainen, T., Henning, S., Bilde, M., and Laaksonen, A. (2004). The role of surfactants in Kohler theory reconsidered. Atmos. Chem. Phys. 4, 2107–2117. doi: 10.5194/acp-4-2107-2004
Stokes, M., Deane, G., Prather, K., Bertram, T., Ruppel, M., Ryder, O., et al. (2013). A Marine Aerosol Reference Tank system as a breaking wave analogue for the production of foam and sea-spray aerosols. Atmos. Measure. Tech. 6:1085. doi: 10.5194/amt-6-1085-2013
Thornton, D. C. O. (2014). Dissolved organic matter (DOM) release by phytoplankton in the contemporary and future ocean. Eur. J. Phycol. 49, 20–46. doi: 10.1080/09670262.2013.875596
Thornton, D. C. O., Brooks, S. D., and Chen, J. (2016). Protein and carbohydrate exopolymer particles in the sea surface microlayer (SML). Front. Marine Sci. 3:14. doi: 10.3389/fmars.2016.00135
Thornton, D. C. O., Fejes, E. M., DiMarco, S. F., and Clancy, K. M. (2007). Measurement of acid polysaccharides in marine and freshwater samples using alcian blue. Limnol. Oceanogr. Methods 5, 73–87. doi: 10.4319/lom.2007.5.73
Van Pinxteren, M., Barthel, S., Fomba, K. W., Müller, K., Von Tümpling, W., and Herrmann, H. (2017). The influence of environmental drivers on the enrichment of organic carbon in the sea surface microlayer and in submicron aerosol particles–measurements from the Atlantic Ocean. Elementa Sci. Anthropocene 5:35. doi: 10.1525/elementa.225
Wilbourn, E. K., Thornton, D. C. O., Ott, C., Graff, J., Quinn, P. K., Bates, T. S., et al. (2020). Ice nucleation by marine aerosols over the north atlantic ocean in late spring. J. Geophys. Res. Atmos. 125:17. doi: 10.1029/2019JD030913
Williams, P. J. L. (1995). Evidence for the seasonal accumulation of carbon-rich dissolved organic material, its scale in comparison with changes in particulate material and the consequential effect on net C/N assimilation ratios. Mar. Chem. 51, 17–29. doi: 10.1016/0304-4203(95)00046-T
Wolf, M. J., Goodell, M., Dong, E., Dove, L. A., Zhang, C., Franco, L. J., et al. (2020). A link between the ice nucleation activity of sea spray aerosol and the biogeochemistry of seawater. Atmos. Chem. Phys. Discuss. 2020, 1–28. doi: 10.5194/acp-2020-416
Wurl, O., and Holmes, M. (2008). The gelatinous nature of the sea-surface microlayer. Mar. Chem. 110, 89–97. doi: 10.1016/j.marchem.2008.02.009
Zieger, P., Vaisanen, O., Corbin, J. C., Partridge, D. G., Bastelberger, S., Mousavi-Fard, M., et al. (2017). Revising the hygroscopicity of inorganic sea salt particles. Nat. Commun. 8:10. doi: 10.1038/ncomms15883
Glossary
CCN, Cloud Condensation Nuclei; CPC, Condensation Particle Counter; CTD, Conductivity, Temperature, Depth; DOC, Dissolved Organic Carbon; DOM, Dissolved Organic Matter; POC, Particulate Organic Carbon; PMA, Primary Marine Aerosol; CTD, Conductivity, Temperature, and Depth; MWCO, Molecular Weight Cut-Off; DMA, Differential Mobility Analyzer; DSML, Desalted Sea Surface Microlayer; HPLC, High Performance Liquid Chromatography; κ, Apparent hygroscopicity parameter; RuBisCO, Ribulose-1,5-bisphosphate carboxylase/oxygenase; SML, Sea Surface Microlayer; SSA, Sea Spray Aerosol; SS, Supersaturation; TOC, Total Organic Carbon; LaRC, Langley Research Center; SBC, Santa Barbara Channel; HMW, High Molecular Weight; LMW, Low Molecular Weight; D, Critical dry particle diameter; Sc, Critical supersaturation; σ, Surface tension of solution; σmeasured, Measured surface tension of samples; MWwater, Molecular weight of water; R, Universal gas constant; T, Absolute temperature; MWsolution, Molecular weight of solution; MWsalts, Molecular weight of salts in artificial seawater; ρsalts, Density of salts in artificial seawater; ρwater, Density of water; ρsolution, Density of solution; MWorg, Molecular weight of organics; ncarbons, Number of carbons in organic molecule; Wsalts, Volume fraction of salts; Worg, Volume fraction of organics; Wsaltsmole, Mole fraction of salts; Worgmole, Mole fraction of organics; ρparticle, Density of the particle; ρorg, Density of the organic; V, Volume of particle; isalts, van't Hoff factor for salts in artificial seawater; i, van't Hoff factor; iorg, van't Hoff factor for the organic; massmixture, Mass of inorganics and organics; massorganic, Mass of organics; b, Parameter; c, Parameter; co, Parameter to calculate κ for organic composition only; cm, Parameter to calculate κ for mixture of inorganics and organics; N, Number of carbon atoms in the organic molecule; Sc, Critical saturation ratio.
Keywords: microlayer, aerosol, cloud condensation nuclei, cloud formation, desalting, organic compounds
Citation: Hendrickson BN, Brooks SD, Thornton DCO, Moore RH, Crosbie E, Ziemba LD, Carlson CA, Baetge N, Mirrielees JA and Alsante AN (2021) Role of Sea Surface Microlayer Properties in Cloud Formation. Front. Mar. Sci. 7:596225. doi: 10.3389/fmars.2020.596225
Received: 19 August 2020; Accepted: 22 December 2020;
Published: 18 January 2021.
Edited by:
Agostino Merico, Leibniz Centre for Tropical Marine Research (LG), GermanyReviewed by:
Douglas Collins, Bucknell University, United StatesJohn Patrick Dunne, Geophysical Fluid Dynamics Laboratory (GFDL), United States
Copyright © 2021 Hendrickson, Brooks, Thornton, Moore, Crosbie, Ziemba, Carlson, Baetge, Mirrielees and Alsante. This is an open-access article distributed under the terms of the Creative Commons Attribution License (CC BY). The use, distribution or reproduction in other forums is permitted, provided the original author(s) and the copyright owner(s) are credited and that the original publication in this journal is cited, in accordance with accepted academic practice. No use, distribution or reproduction is permitted which does not comply with these terms.
*Correspondence: Sarah D. Brooks, c2Jyb29rcyYjeDAwMDQwO3RhbXUuZWR1