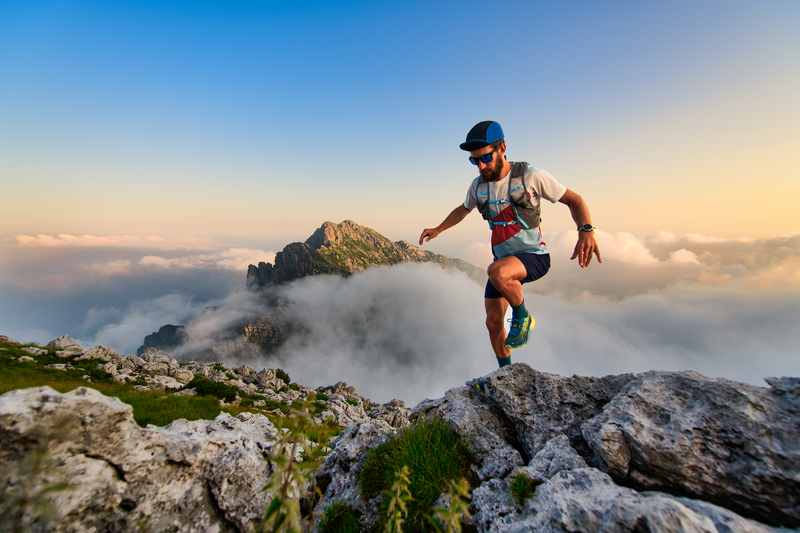
95% of researchers rate our articles as excellent or good
Learn more about the work of our research integrity team to safeguard the quality of each article we publish.
Find out more
ORIGINAL RESEARCH article
Front. Mar. Sci. , 10 December 2020
Sec. Marine Fisheries, Aquaculture and Living Resources
Volume 7 - 2020 | https://doi.org/10.3389/fmars.2020.595682
This article is part of the Research Topic Fish Nutrition, Metabolism and Physiology View all 20 articles
Lysine is known to be a functional nutrient from yeast to human beings. However, the mechanisms for lysine to regulate body growth and metabolism in fish species are far from fully illuminated. In order to elucidate the molecular effects of lysine on growth performance and metabolism, largemouth bass (Micropterus salmoides), which is extremely sensitive to protein composition, was chosen as the experimental model. Juvenile largemouth bass (4.16 ± 0.02 g) were fed with the lysine control diet (LC diet), the lysine supplementation diet (LS diet), and the low lysine diet (LL diet) for 8 weeks. The results showed that the LS diet group significantly increased fish growth, feed efficiency, and nutrient retention compared with those of the LL diet group in largemouth bass. Moreover, compared to the LL diet, lysine supplementation also elevated the plasma total protein, total EAA, total AA, and some individual AA concentrations. Mechanistically, lysine supplementation had a significant effect to decrease the mRNA expression levels of peptide and AA transporters induced by lysine restriction. Lysine supplementation also had an important impact on regulating the target of rapamycin (TOR) signaling pathway. More importantly, the key regulators in the amino acid response (AAR) signaling pathway were also down-regulated by lysine supplementation. Our results provide a clear elucidation of how dietary lysine affected growth performance, physiological and biochemical responses, and signaling responses and represent a sound foundation for using lysine to improve the nutrient utilization of poultry by-product meal in largemouth bass.
Beyond acting as building blocks for proteins, amino acids (AA) also have diverse physiological functions, such as regulating body growth and metabolism, cell signaling, immunity, appetite stimulation, stress responses, and reproduction (Li et al., 2009; Wu et al., 2014). In 2010, Wu (2010) defined the AA that played a vital role in regulating key metabolic pathways as a new concept of “functional amino acids” (FAA). A restriction in an FAA leading to AA imbalance not only impairs protein synthesis but also disrupts whole body metabolism homeostasis (Wu, 2010). Consequently, the ideal balances of dietary AA will promote optimal growth, production, and health performance (Reeds, 2000).
As an important FAA in both human beings and other animals, lysine exerts extraordinary effects on body growth and metabolism (Li et al., 2009). More surprisingly, data on the AA composition of the ingredients used in animal feeds show that lysine is one of most often limiting AA, especially in plant protein sources (Mai et al., 2006). Therefore, lysine supplementation represents a crucial way to improve the nutrient utilization of low-lysine ingredients. Increasing evidences in mammals had shown that dietary lysine concentration is the top regulator of the metabolism in piglets (Shikata et al., 2007). Consistent with mammals, studies on fish also revealed that lysine restriction caused not only growth restriction and metabolic obstruction (Marine et al., 2011) but also scoliosis, lordosis, and immune problems (Khan and Abidi, 2015). Until now, a large number of studies have only explored the effect of dietary lysine on the phenotypic changes in the growth performance of fish species, but the underlying molecular mechanism by which lysine affects growth and metabolism still need to be fully revealed.
Nutrient-sensing signaling pathway activation is the core mechanism for AA to regulate body growth and metabolism (Kimball, 2007). Target of rapamycin (TOR) and amino acid response (AAR) are two complementary nutrient-sensing signaling pathways that control body anabolism and catabolism (Guo and Cavener, 2007; Trevino et al., 2008). A well-balanced AA profile diet could activate the TOR signaling pathway to promote protein synthesis and intermediary metabolism (Ma and Blenis, 2009). In contrast, deficiency in any individual AA will enhance the abundance of uncharged tRNAs, resulting in the activation of general control non-derepressible 2 kinase (GCN2), a key regulator involved in the AAR signaling pathway, and the subsequent inhibition of total protein (TP) translation (Guo and Cavener, 2007). Furthermore, peptide and AA transporters, as the bridge connecting the internal and exterior cell milieu, are the AA sensing mechanisms that lie upstream of the TOR and AAR pathways (Castagna et al., 1997; Russell et al., 2003). In recent years, peptide and AA transporters have been considered as the first step for AA sensing and are increasingly thought to play a pivotal role in body nutrient sensing (Bröer, 2008; Song et al., 2017). In mammals, numerous reports have revealed the regulatory mechanism of dietary lysine levels on nutrient-sensing signaling pathways (Sato et al., 2015). However, little is known about how lysine regulates body growth and metabolism through nutrient-sensing pathways in fish species.
In the present study, lysine supplementation to low lysine diet was hypothesized to promote the body growth and metabolism by affecting nutrient-sensing signaling pathways. To test this hypothesis, largemouth bass, a carnivorous fish species, was chosen as the experimental model to explore the changes in body growth performance, nutrient accumulation, plasma AA concentration levels, peptide and AA transporters expressions, and TOR and AAR signaling pathways after feeding different lysine level diets. This trial should provide an improved mechanistic understanding of the inner links among lysine, nutrient-sensing, and body growth performance.
The experimental procedures strictly complied with the regulations of the University Animal Care and Use Committee of the South China Normal University (approval reference number 1002019-02-0016).
All the components in the experimental diets are presented in Table 1. Three isonitrogenous (approximately 54.8% crude protein) and isolipidic (approximately 12.5% crude lipid) diets were formulated using fishmeal (FM), poultry by-product meal (PBM), and L-crystalline AA with different levels of lysine. The lysine control diet (LC diet) contained 65% FM, the low lysine diet (LL diet) contained 65% PBM, and supplementation with all the EAA except lysine to match the EAA profile of the LC diet, and the lysine supplementation diet (LS) contained 65% PBM and supplementation with all the EAA to match the EAA profile of the LC diet. The ingredients used in this experiment were finely grounded into powder through a 320-μm screen. After that, all the compositions used in the diets were individually blended in the mixer and further homogenized after oil and water added. The pellets were wet-extruded by using a pelletizer (F-26, South China University of Technology, Guangzhou, China). All the experimental diets were dried at 45°C for 12 h and stored at −20°C until used. The AA compositions of the experimental diets are presented in Table 2.
Juvenile largemouth bass were purchased from Zengcheng Fish Farm (Guangdong, China). Before the experiment, all fish were fed commercial feed twice per day for 2 weeks to apply experimental conditions. Afterward, the largemouth bass were fasted for 24 h and weighed to obtain the initial body weight. Fish with an initial weight of 4.16 ± 0.02 g were randomly assigned into tanks with 300 L of freshwater. Each diet was allocated in triplicate tanks, and each tank contained 30 fish. Fish were fed twice (07:00 and 17:00) daily to apparent satiation for 8 weeks. During the trial, the water temperature ranged from 24°C to 27°C, NH4–N ranged from 0.07 to 0.10 mg/L, and dissolved oxygen ranged from 6.0 to 7.0 mg/L.
At the beginning of the trial, 20 fish were randomly sampled to obtain the initial whole body compositions. After 8 weeks of feeding, the experimental fish were weighed and counted after 24 h of fasting, and four fish from each tank were randomly selected for final biochemical analysis. Meanwhile, 12 largemouth bass (4 per tank) from each treatment were quickly collected. First, all the fish were euthanized with 100 mg/L eugenol (Shanghai Medical Co., Ltd, Shanghai, China). The caudal venipuncture method was used to obtain the blood samples. Blood samples were centrifuged at 3,000 g for 5 min at 4°C immediately after the blood was drawn. The supernatant plasma was transferred into the new tubes and immediately placed into liquid nitrogen and then kept at −80°C for analysis. The livers and visceral mass of four fish from each tank were weighed, and the ratios were expressed as a percentage of body weight. At the same time, the liver, intestine, and dorsolateral white muscle were dissected and pooled into RNase-free tubes (Axygen), frozen in liquid N2 and then stored at −80°C until analysis.
Proximate composition analyses of the ingredients, diets, and whole fish were performed following previous methods (Liu et al., 2014). Whole fish were dried at 105°C to a constant weight to calculate the water content. Crude protein contents were determined by the Kjeldahl method (Kjeltec TM 8400, FOSS, Sweden) to measure nitrogen and then multiply a coefficient, 6.25. SoxtecTM 2055 extraction by using petroleum ether (B.P. 30–60°C for 3 h) was used for lipid content measurement. Moreover, fish samples were put into a muffle furnace (FO610C, Yamato Scientific Co., Ltd., Tokyo, Japan) at 550°C for 8 h to analyze the ash content. For AA concentrations, all the ingredients and diets were dried by a freezer dryer (ALPHA1-2 LD plus, Christ Co., Ltd, Germany). After digesting with 6 M HCl for 22 h, all the AA composition in ingredients and diets were measured by an L-8900 AA analyzer (Hitachi, Japan; Blankenship et al., 1989).
The concentration of plasma TP was measured by using an Automatic Biochemical Analyzer (CHEMIX- 800, Sysmex Corporation, Kobe, Japan). The concentrations of free AA in the plasma were determined using the method described before (Mente et al., 2003). Briefly, an ion-exchange AA analyzer (L8900; Hitachi, Tokyo, Japan) was used to measure the concentration of free AA; 1.2 ml of 10% sulfosalicylic acid solution and 400 μl of each plasma sample were mixed well. After incubation for 5 min at 4°C, all samples were centrifuged at 13,000 rpm for 15 min at 4°C. Supernatants were filtered through 0.22-μm filters for free AA concentration analysis.
Total RNA samples of different tissues were extracted using TRIzol reagent (Vazyme Biotech Co., Ltd, China) following the manufacturer’s instructions. The quality and quantity of isolated RNA were detected by using a 1.2% denaturing agarose column and a NanoDrop 2000 spectrophotometer, respectively. Intact RNA (1 μg) was then reverse transcribed into cDNA by a Prime Script RT reagent Kit (Vazyme Biotech Co., Ltd, China).
All the primers used in this study are listed in Table 3. There were no changes in the expression of β-actin, elongation factor 1α (EF1α), and glyceraldehyde-3-phosphate dehydrogenase (GAPDH) in the corresponding tissues among the treatments. Therefore, the geometric means of these three reference genes were used for the normalization of the samples. The Quantitative Real-Time PCR (qRT-PCR) was performed in an ABI 7500 real-time PCR machine (Applied Biosystems, United States) using Hiff® qPCR SYBR Green Master Mix (Yeasen, Shanghai, China) according to the manufacturer’s instructions. The PCR efficiency was determined by the slope of a standard curve using four serial dilutions of cDNA. The qRT-PCR program was as follows: 95°C for 2 min and then 40 cycles at 95°C for 15 s, 60°C for 30 s, and 72°C for 20 s. At the end of each PCR, melting curve analysis was performed to confirm that only single amplified product was present. Comparative CT method (2–ΔΔCt method) was used to calculate the relative expression level. Gene expressions were represented as the fold change related to the LC diet group.
The expression level of protein kinase B (AKT), phosphor-AKT, TOR, phosphor-TOR, ribosomal protein S6 (S6), phosphor-S6, eukaryotic initiation factor 4E binding protein-1 (4E-BP1), phosphor-4E-BP1, activating transcription factor 4 (ATF4), eukaryotic initiation factor 2α (eIF2α), phosphor-eIF2α, and β-tubulin were measured by using Western blot as previous described (Song et al., 2016). After homogenization on ice, muscle and liver were lysed in RIPA buffer (50 mM Tris–HCl, 1 mM EDTA, 150 mM NaCl, 0.1% SDS, 0.5% NP-40, and pH 7.5) with phosphatase inhibitor and protease cocktails (Roche, United States) for 1 h. The protein concentrations of the samples were measured by using the BCA protein assay kit (Beyotime Biotechnology). Samples were separated by sodium dodecyl sulfate-polyacrylamide gels (SDS-PAGE) and transferred to 0.45-μm PVDF membranes (Millipore). After blocking with 5% non-fat milk in TBST buffer (20 mM Tris–HCl, 500 mM NaCl, and 0.1% Tween-20) for 1 h, the membranes were incubated with primary antibody (Cell Signaling Technology, United States, and Santa Cruz Biotechnology Inc., United States) overnight at 4°C. Then, the membranes were incubated in horseradish peroxidase-labeled secondary antibodies for 1 h and visualized using ECL reagents (Beyotime Biotechnology). The Western blot result bands were quantified with the NIH Image 1.63 software. The antibodies used in present study were presented as follows: phospho-Akt (Ser473, 9271), Akt (9272), phospho-TOR (Ser2448, 2971), TOR (2972), phospho-S6 (Ser235/236, 4856), S6 (2217), phospho-4E-BP1 (Thr37/46, 9459), 4E-BP1 (9452), ATF4 (sc-200), phosphor-eIF2α (Ser51, 3597), eIF2α (9722), and β-tubulin (2146). All the antibodies in present study were confirmed to be conserved and successfully used in fish species (Song et al., 2016; Xu et al., 2016).
Growth parameters were calculated as follows:
Specific growth rate (SGR,%/day) = 100 × (Ln Wt-Ln W0)/t;
Weight gain rate (WGR,%) = 100 × (Wt-W0)/W0;
Feed efficiency ratio (FER) = (Wt-W0)/(dry feed intake);
Protein efficiency ratio (PER) = (Wt-W0)/(dry feed intake × protein percent in dry diet);
Nutrient retention (%) = 100 × (final body weight × final carcass nutrient content-initial body weight × initial carcass nutrient content)/nutrient intake;
Survival rate (SR,%) = 100 × final amount of fish/initial amount of fish;
Condition factor (CF, g/cm3) = 100 × (final body weight/body length (cm)3);
Viscerosomatic index (VSI,%) = 100 × (viscera wet weight/body wet weight);
Hepatosomatic indices (HSI,%) = 100 × (liver weight/whole body weight).
W0 and Wt represented the body weight (g) of initial fish and final fish, respectively, while t represented the rearing days of this experiment.
Data were expressed as means ± SEM. All the statistical evaluations were analyzed using one-way analysis of variance (ANOVA) followed by Tukey’s multiple range test with the SPSS 19.0 software. Differences were considered as significant when P < 0.05.
As shown in Table 4, after 8 weeks of feeding, the fish fed the LS diet gained significantly more body weight (26.9 ± 0.14 g) than those fed LL diet (24.1 ± 0.32 g), but less body weight than those the fed LC diet (29.2 ± 0.21 g). Compared with the LL diet, the LS diet significantly increased the SGR, WGR, FER, PER, protein retention, fat retention and body protein, and lipid contents (P < 0.05). However, compared with the LC diet, the LS diet significantly decreased the SGR, WGR, FER, PER, protein retention, and fat retention (P < 0.05). Moreover, the LL diet markedly increased the VSI compared with the LC diet (P < 0.05). Dietary lysine levels did not affect the SR, CF, HSI, and the body compositions, including moisture and ash content (P > 0.05).
The concentration of TP and AA in plasma after feeding different diets was assessed. As shown in Table 5, crystalline lysine addition significantly elevated TP concentration in plasma compared with the LL diet (P < 0.05). Meanwhile, lysine supplementation also increased the concentration levels of lysine in plasma. The concentrations of total EAA, total AA, and individual AA, including threonine, methionine, leucine, cysteine, and tyrosine, were significantly higher in fish fed the LS diet than in fish fed the LL diet (P < 0.05). However, supplementation with lysine to LC diet level still did not increase the total EAA, total NEAA, total AA, threonine, methionine, phenylalanine, glycine, and alanine concentration levels to those of the LC diet group (P < 0.05). Moreover, compared to the LC diet group, lysine supplementation had no significant effect on increasing the plasma total NEAA concentration (P > 0.05).
Table 5. Total protein and FAA concentration in the plasma after largemouth bass intake different diets.
The mRNA expression levels of peptide and AA transporters were examined in both muscle (Figure 1A) and intestine (Figure 1B). In muscle, fish fed the LL diet showed significantly enhanced mRNA expression levels of the peptide transporter solute carrier family 15 member 2 (SLC15A2, PepT2) and AA transporters, including solute carrier family 7 member 5 (SLC7A5, LAT1), solute carrier family 7 member 8 (SLC7A8, LAT2), and solute carrier family 38 member 2 (SLC38A2, SNAT2), compared to the fish fed the other two diets (P < 0.05). Compared with the LL diet, lysine supplementation markedly reduced the mRNA expression levels of these four transporters, which had no significant differences with the LC diet group (P < 0.05). The AA transporters in the intestine displayed various mRNA expression patterns after the fish fed different diets. Compared with the fish fed the LC diet, the fish fed the LL diet significantly increased the mRNA expression levels of SLC15A2, SLC7A5, and SLC38A2 (P < 0.05). Lysine addition had a significant effect to decrease the mRNA expression levels of SLC15A2 and SLC38A2 to the LC diet group level (P < 0.05). No significant differences were observed in SLC7A8 expression level among the three groups (P > 0.05).
Figure 1. Effects of the LC diet, LL diet supplemented with dietary lysine (LS diet) and the LL diet on peptide and AA transporters mRNA expression in muscle (A) and intestine (B). Different superscript letter “a, b” indicated statistical significant differences (P < 0.05; mean ± SEM, n=6).
The mRNA expression levels of key regulators involved in the TOR signaling pathway were measured in both the muscle (Figure 2A) and liver (Figure 2B). There is no doubt that among the three different treatments, the gene expression level of the selected key regulators in the TOR signaling pathway showed significantly high expression in the LC diet regardless of muscle and liver (P < 0.05). Compared with the fish fed LL diet, the fish fed the LS diet elevated the mRNA expression of TOR, S6, and 4E-BP1 in muscle and S6 and 4E-BP1 in liver (P < 0.05). No significant differences were detected in muscle and liver AKT expressions between the LL and LS diet groups (P > 0.05).
Figure 2. Effects of the LC diet, LL diet supplemented with dietary lysine (LS diet) and the LL diet on muscle (A) and liver (B) mRNA expression levels of key regulators involved in TOR signaling pathway. Different superscript letters “a, b” indicated statistical significant differences (P < 0.05; mean ± SEM, n = 6).
Moreover, the TP and corresponding phosphorylation levels of key regulators involved in the TOR signaling pathway were also evaluated in muscle and liver (Figure 3). Consistent with the mRNA expression profile, the LL diet group had significantly lower phosphorylation levels of AKT, TOR, S6, and 4E-BP1 than the LC diet group in both muscle and liver (P < 0.05). Lysine supplementation had a significant effect to increase the phosphorylation levels of S6 in liver and AKT, TOR, S6, and 4E-BP1 in muscle (P < 0.05).
Figure 3. Effects of the LC diet, LL diet supplemented with dietary lysine (LS diet) and the LL diet on muscle (A) and liver (B) protein phosphorylation levels of key regulators involved in TOR signaling pathway. Different superscript letters “a, b, c” indicated statistical significant differences (P < 0.05; mean ± SEM, n= 3).
The data describing the relative expression of key regulators in the AAR signaling pathway are presented in Figure 4. The LL diet activated the AAR signaling pathway in both the muscle and liver (P < 0.05). Lysine supplementation significantly decreased the gene expression level of key regulators including eIF2α, ATF4, and regulated in development and DNA damage responses 1 (REDD1) to the expression levels observed with the LC diet in muscle (P < 0.05). In the liver, lysine supplementation significantly reduced the mRNA expression levels of ATF4 and REDD1 induced by low lysine level (P < 0.05). No significant differences were observed in the expression level of eIF2α, ATF4, CHOP, and REDD1 between the LS and LC diet groups (P > 0.05).
Figure 4. Effects of the LC diet, LL diet supplemented with dietary lysine (LS diet) and the LL diet on muscle (A) and liver (B) mRNA expression levels of key regulators involved in AAR signaling pathway. Different superscript letters “a, b” indicated statistical significant differences (P < 0.05; mean ± SEM, n= 6).
The phosphorylation levels of key regulators involved in the AAR signaling pathway were also assessed in the present study (Figure 5). Compared with the fish fed with the LC diet, the fish fed with LL diet had significantly higher ATF4 expression level in muscle, liver, and higher eIF2α phosphorylation level in liver (P < 0.05). Lysine addition had a marked effect to decrease the TP expression of ATF4 in both muscle and liver. Furthermore, in liver, lysine supplementation had a different effect to decrease the phosphorylation level of eIF2α in liver (P < 0.05).
Figure 5. Effects of the LC diet, LL diet supplemented with dietary lysine (LS diet) and the LL diet on muscle (A) and liver (B) protein phosphorylation levels of key regulators involved in AAR signaling pathway. Different superscript letters “a, b” indicated statistical significant differences (P < 0.05; mean ± SEM, n= 3).
Fishmeal replacement is an urgent and complicated problem that directly affects the development of aquaculture (Gatlin et al., 2007). Up to now, a large number of studies have been conducted to improve the utilization of non-fishmeal protein sources in order to achieve the fishmeal replacement (Hardy, 2010). Available evidences have shown that improving feed efficiency by supplementing AA is becoming increasingly important for all species, including mammals, poultry, and fish (Wu et al., 2014; Regmi et al., 2017). Lysine as an EAA is often limited in ingredients used for aquafeeds. Therefore, lysine supplementation is becoming a pivotal method to improve the effects of non-fishmeal protein source substitution in aquaculture (Li et al., 2009; Huang et al., 2020). So far, many studies have been tested and suggested that compared with other protein sources, PBM is one of the most promising ingredients widely used in aquafeeds industry due to its higher protein content and good apparent digestibility (Zapata et al., 2016; Sabbagh et al., 2019). However, the high percentage of PBM substituted with FM often reduced body growth performance. The main problem leading to this phenomenon is PBM, compared with FM, contained lower levels of lysine and other AA. AA imbalance, especially lysine restriction, has become one of the main limiting factors of using PBM to replace FM. Previous studies showed that the replacement of FM with PBM caused growth restrictions of more than 50% in humpback grouper (Cromileptes altivelis; Shapawi et al., 2007) and over 80% in white shrimp (Litopenaeus vannamei; Cruzsuárez et al., 2007). Consistent with these studies, our trial showed that the complete replacement of FM with PBM reduced growth performance, nutrient retention, and feed efficiency. Lysine supplementation could ameliorate these problems caused by PBM replacement in largemouth bass. These results further confirmed the effect of lysine in improving growth performance in fish species. However, the underlying mechanism for this phenomenon is still not fully revealed in fish species. In the present study, the nutrient-sensing system was evaluated to figure out how dietary lysine regulates growth performance and nutrient metabolism.
The free AA concentration in the metabolic pool is considered as the primary driving force to determine anabolism or catabolism. It is noteworthy that the concentrations of free AA in plasma were easily influenced by nutritional state, developmental stage, and endocrine status (Bos et al., 2003; Wu, 2013). Our previous study in turbot revealed that crystalline AA addition could significantly increase the plasma free AA concentration (Song et al., 2017). Consistent with the turbot study, the present study showed that largemouth bass fed the LL diet for 8 weeks presented significantly lower concentration of total EAA, TAA, and individual AA than did fish fed the LS diet. Lysine supplementation had a significant effect to elevate these AA’s concentrations. Lysine restriction created a nutritional environment in which other AA could not successfully transport into plasma. Lysine supplementation balanced the AA composition of the PBM and further promoted the transport and absorption of various AA in plasma. Elevated free AA increased the “building blocks” for protein synthesis and ultimately promoted growth performance (Wu, 2018).
Peptide and AA transporters, as sensors of intracellular and extracellular, play an important role in the absorption of AA in both intestine and other tissues (Russell et al., 2003; Taylor, 2013). To determine the underlying mechanisms by which dietary lysine level changed the composition of free AA pools, the cellular transporters in both intestine and muscle were measured in this study. SLC15A2 is the major peptide transporter widely expressed in intestinal and extraintestinal tissues (Gilbert et al., 2008). We observed that SLC15A2 had significantly higher expression in the LL diet group than in the LS diet group. Fish fed the LS diet had decreased mRNA expression of SLC15A2, but not to the level of fish fed the LC diet. These results were in accordance with studies in blunt snout bream (Megalobrama amblycephala; Ahmed et al., 2019) and turbot (Scophthalmus maximus L.; Xu et al., 2017). The SLC7A5, SLC7A8, and SLC38A2, acting as the intermediates to regulate FAA transportation, were also identified in fish species (Xu et al., 2016, 2017). In both the muscle and intestine, lysine supplementation down-regulated the mRNA expression levels of AA transporters except SLC7A8 in intestine, but in muscle, the AA transporter expression levels were still higher than those in the LC diet. By combining the free AA concentrations results, we could find a link between the gene expression of peptide and AA transporters and free AA concentrations. Lysine restriction reduced the free AA concentration in plasma; a lower AA concentration stimulated transporters’ expression to transport as many AA to the free AA pool as possible. This adaptation mechanism could ensure the optimal growth when the body is exposed to different nutritional environments.
Target of rapamycin and AAR are two distinct but complementary pathways responsible for sensing AA concentration at the cellular level (Peichuan et al., 2002; Anne et al., 2003). Increasing evidences from yeast to mammals demonstrated that aside from being the unit for protein synthesis, lysine also acts as a signaling molecule that has a profound effect on regulating the cellular nutrient sensing system (Dann and Thomas, 2006; Wu, 2010). Moreover, our previous studies in turbot also demonstrated that dietary AA availability regulated body growth and metabolism through the TOR and AAR signaling pathways (Song et al., 2016; Xu et al., 2016). The phosphorylation site of Ser2448 for TOR, Ser473 for AKT, Thr37/46 for 4E-BP1, Ser235/236 for S6, and Ser51 for eIF2α were more sensitive to dietary nutrient composition (Seiliez et al., 2011; Dai et al., 2013). The present study showed that low lysine level diet activated the AAR pathway and inhibited the activity of the TOR signaling pathway. These results are consistent with previous studies that focused on the relationship between AA and nutrient-sensing pathways (Jordan et al., 2013). Moreover, our results demonstrated that in the TOR signaling pathway, the phosphorylation site of Ser235/236 for S6 could be the most sensitive regulator for dietary lysine availability. Muscle had a stronger response to crystalline lysine addition. Dietary lysine supplementation had a limited effect on regulating both mRNA and protein expression of the key regulators involved in the TOR signaling pathway. On the contrary, the limitation of individual AA could activate the AAR pathway to repress global protein synthesis but increased special protein translation including some EAA, AA transporters, and so on (Kilberg et al., 2005; Guo and Cavener, 2007). So, it was inferred that lysine restriction is a powerful signaling method to activate the AAR signaling pathway, which then leads to the shutdown of protein anabolism and an increase in the mRNA expression levels of peptide and AA transporters to maintain body homeostasis in largemouth bass. These results showed a noticeable correlation between the AAR pathway and AA transporter activity in fish species.
Taken together, the present study indicated that lysine supplementation in FM totally replaced by PBM diet improved growth performance and body nutrient content not only by balancing the AA profile of the diet but also by supplying a signaling molecule to regulate the nutrient-sensing pathways. A well-balanced AA composition of the diet improved the free AA concentration and then inhibited the AAR signaling and activated TOR pathway. Hypoactivated AAR signaling status then decreased the mRNA expression of AA transporters in turn to regulate free AA availability in fish. Our observations confirmed that crystalline lysine supplementation was an extremely effective method that could, through a nutrient-sensing system, maintain the balance of the AA profile to improve the utilization of PBM in largemouth bass.
The raw data supporting the conclusions of this article will be made available by the authors, without undue reservation.
The animal study was reviewed and approved by University Animal Care and Use Committee of the South China Normal University.
FS, SC, and KM designed the study. WW, YX, and PY performed the study. YX and WW analyzed the data. FS wrote the manuscript. All authors read and approved the final manuscript.
This research was supported by the Foundation and Applied Basic Research Fund project of Guangdong Province (2019A1515110606), the Research and Development Fund for Young Teachers of South China Normal University (19KJ03), and the Research Start-Up Fund of South China Normal University.
The authors declare that the research was conducted in the absence of any commercial or financial relationships that could be construed as a potential conflict of interest.
We thank the Ocean University of China for helping with free amino acid concentration measurement and Y.H. Chen and Z.Z. Sun for their help during the experiment.
Ahmed, M., Liang, H., Kasiya, H. C., Ji, K., Ge, X., Ren, M., et al. (2019). Complete replacement of fish meal by plant protein ingredients with dietary essential amino acids supplementation for juvenile blunt snout bream (Megalobrama amblycephala). Aquac. Nutr. 25, 205–214. doi: 10.1111/anu.12844
Anne, B., Tee, A. R., Taylor, P. M., and Proud, C. G. (2003). Regulation of targets of mTOR (mammalian target of rapamycin) signalling by intracellular amino acid availability. Biochem. J. 372, 555–566. doi: 10.1042/bj20021266
Blankenship, D. T., Krivanek, M. A., Ackermann, B. L., and Cardin, A. D. (1989). High-sensitivity amino acid analysis by derivatization with O-phthalaldehyde and 9-fluorenylmethyl chloroformate using fluorescence detection: application in protein structure determination. Anal. Biochem. 178, 227–232. doi: 10.1016/0003-2697(89)90629-5
Bos, C., Metges, C., Gaudichon, C., Petzke, K. J., Pueyo, M. E., Morens, C., et al. (2003). Postprandial kinetics of dietary amino acids are the main determinant of their metabolism after soy or milk protein ingestion in humans. J. Nutr. 133, 1308–1315. doi: 10.1093/jn/133.5.1308
Bröer, S. (2008). Amino acid transport across mammalian intestinal and renal epithelia. Physiol. Rev. 88, 249–286. doi: 10.1152/physrev00018.2006
Castagna, M., Shayakul, C., Trotti, D., Sacchi, V. F., Harvey, W. R., and Hediger, M. A. (1997). Molecular characteristics of mammalian and insect amino acid transporters: implications for amino acid homeostasis. J. Exp. Biol. 200, 269–286.
Cruzsuárez, L. E., Nietolópez, M., Guajardobarbosa, C., Tapiasalazar, M., Scholz, U., and Ricquemarie, D. (2007). Replacement of fish meal with poultry by-product meal in practical diets for Litopenaeus Vannamei, and digestibility of the tested ingredients and diets. Aquaculture 272, 466–476. doi: 10.1016/j.aquaculture.2007.04.084
Dai, W., Panserat, S., Mennigen, J. A., Terrier, F., Dias, K., Seiliez, I., et al. (2013). Post-prandial regulation of hepatic glucokinase and lipogenesis requires the activation of TORC1 signalling in rainbow trout (Oncorhynchus mykiss). J. Exp. Biol. 216, 4483–4492. doi: 10.1242/jeb.091157
Dann, S. G., and Thomas, G. (2006). The amino acid sensitive TOR pathway from yeast to mammals. FEBS Lett. 580, 2821–2829. doi: 10.1016/j.febslet.2006.04.068
Gatlin, D. M., Barrows, F. T., Brown, P., Dabrowski, K., Gaylord, T. G., Hardy, R. W., et al. (2007). Expanding the utilization of sustainable plant products in aquafeeds: a review. Aquac. Res. 38, 551–579. doi: 10.1111/j.1365-2109.2007.01704.x
Gilbert, E. R., Wong, E. A., and Webb, K. E. (2008). Board-invited review: peptide absorption and utilization: implications for animal nutrition and health. J. Anim. Sci. 86, 2135–2155. doi: 10.2527/jas.2007-0826
Guo, F., and Cavener, D. R. (2007). The GCN2 eIF2α kinase regulates fatty-acid homeostasis in the liver during deprivation of an essential amino acid. Cell Metabol. 5, 103–114. doi: 10.1016/j.cmet.2007.01.001
Hardy, R. W. (2010). Utilization of plant proteins in fish diets: effects of global demand and supplies of fishmeal. Aquac. Res. 41, 770–776. doi: 10.1111/j.1365-2109.2009.02349.x
Huang, D., Liang, H., Ren, M., Ge, X., Ji, K., Yu, H., et al. (2020). Effects of dietary lysine levels on growth performance, whole body composition and gene expression related to glycometabolism and lipid metabolism in grass carp, Ctenopharyngodon idellus fry. Aquaculture 530:735806. doi: 10.1016/j.aquaculture.2020.735806
Jordan, G., Eylul, H., and Mitchell, J. R. (2013). Amino acid sensing in dietary-restriction-mediated longevity: roles of signal-transducing kinases GCN2 and TOR. Biochem. J. 449, 1–10. doi: 10.1042/BJ20121098
Khan, M. A., and Abidi, S. F. (2015). Effect of dietary L-lysine levels on growth, feed conversion, lysine retention efficiency and haematological indices of Heteropneustes fossilis (Bloch) fry. Aquac. Nutr. 17, 657–667. doi: 10.1111/j.1365-2095.2010.00815.x
Kilberg, M. S., Pan, Y. X., Chen, H., and Leungpineda, V. (2005). Nutritional control of gene expression: how mammalian cells respond to amino acid limitation. Annu. Rev. Nutr. 25, 59–85. doi: 10.1146/annurev.nutr.24.012003.132145
Kimball, S. R. (2007). The role of nutrition in stimulating muscle protein accretion at the molecular level. Biochem. Soc. Trans. 35, 1298–1301. doi: 10.1042/BST0351298
Li, P., Mai, K., Trushenski, J., and Wu, G. (2009). New developments in fish amino acid nutrition: towards functional and environmentally oriented aquafeeds. Amino Acids 37, 43–53. doi: 10.1007/s00726-008-0171-1
Liu, Y., He, G., Wang, Q., Mai, K., Wei, X., and Zhou, H. (2014). Hydroxyproline supplementation on the performances of high plant protein source based diets in turbot (Scophthalmus maximus L.). Aquaculture 433, 476–480. doi: 10.1016/j.aquaculture.2014.07.002
Ma, X. M., and Blenis, J. (2009). Molecular mechanisms of mTOR-mediated translational control. Nat. Rev. Mol. Cell Biol. 10, 307–318. doi: 10.1038/nrm2672
Mai, K., Zhang, L., Ai, Q., Duan, Q., Zhang, C., Li, H., et al. (2006). Dietary lysine requirement of juvenile Japanese seabass, Lateolabrax japonicus. Aquaculture 258, 535–542. doi: 10.1016/j.aquaculture.2006.04.043
Marine, L., Stéphane, P., Elisabeth, P. J., Karine, D., Iban, S., and Sandrine, S. C. (2011). L-leucine, L-methionine, and L-lysine are involved in the regulation of intermediary metabolism-related gene expression in rainbow trout hepatocytes. J. Nutr. 141, 75–80. doi: 10.3945/jn.110.124511
Mente, E., Deguara, S., Santos, M. B., and Houlihan, D. (2003). White muscle free amino acid concentrations following feeding a maize gluten dietary protein in Atlantic salmon (Salmo salar L.). Aquaculture 225, 133–147. doi: 10.1016/s0044-8486(03)00285-0
Peichuan, Z., Mcgrath, B. C., Jamie, R., Olsen, D. A. S., Li, L., Sangeeta, G., et al. (2002). The GCN2 eIF2alpha kinase is required for adaptation to amino acid deprivation in mice. Mol. Cell. Biol. 22, 6681–6688. doi: 10.1128/MCB.22.19.6681-6688.2002
Reeds, P. J. (2000). Dispensable and indispensable amino acids for humans. J. Nutr. 130, 1835S–1840S. doi: 10.1093/jn/130.7.1835S
Regmi, N., Wang, T., Crenshaw, M., Rude, B., and Liao, S. (2017). Effects of dietary lysine levels on the concentrations of selected nutrient metabolites in blood plasma of late-stage finishing pigs. J. Anim. Physiol. Anim. Nutr. 102, 403–409. doi: 10.1111/jpn.12714
Russell, H., Taylor, P. M., and Hundal, H. S. (2003). Amino acid transporters: roles in amino acid sensing and signalling in animal cells. Biochem. J. 373, 1–18. doi: 10.1042/bj20030405
Sabbagh, M., Schiavone, R., Brizzi, G., Sicuro, B., Zilli, L., and Vilella, S. (2019). Poultry by-product meal as an alternative to fish meal in the juvenile gilthead seabream (Sparus aurata) diet. Aquaculture 511, 1–10. doi: 10.1016/jaquaculture.2019.734220
Sato, T., Ito, Y., and Nagasawa, T. (2015). Dietary L-Lysine suppresses autophagic proteolysis and stimulates Akt/mTOR signaling in the skeletal muscle of rats fed a low-protein diet. J. Agric. Food Chem. 63, 8192–8198. doi: 10.1021/acs.jafc.5b03811
Seiliez, I., Panserat, S., Lansard, M., Polakof, M., and Skiba-Cassy, S. (2011). Dietary carbohydrate-to-protein ratio affects TOR signaling and metabolism-related gene expression in the liver and muscle of rainbow trout after a single meal. Am. J. Physiol. Regul. Integr. Comp. Physiol. 300, 733–743.
Shapawi, R., Ng, W. K., and Mustafa, S. (2007). Replacement of fish meal with poultry by-product meal in diets formulated for the humpback grouper, Cromileptesaltivelis. Aquaculture 273, 118–126. doi: 10.1016/j.aquaculture.2007.09.014
Shikata, N., Maki, Y., and Noguchi, Y. (2007). Multi-layered network structure of amino acid (AA) metabolism characterized by each essential AA-deficient condition. Amino Acids 33, 113–121. doi: 10.1007/s00726-006-0412-0
Song, F., Xu, D., Mai, K., Zhou, H., Xu, W., and He, G. (2016). Comparative study on the cellular and systemic nutrient sensing and intermediary metabolism after partial replacement of fishmeal by meat and bone meal in the diet of Turbot (Scophthalmus maximus L.). PLoS One 11:e0165708. doi: 10.1371/journal.pone.0165708
Song, F., Xu, D., Zhou, H., Wei, X., Mai, K., and He, G. (2017). The differences in postprandial free amino acid concentrations and the gene expression of PepT1 and amino acid transporters after fishmeal partial replacement by meat and bone meal in juvenile turbot (Scophthalmus maximus L.). Aquac. Res. 48, 3766–3781. doi: 10.1111/are.13203
Taylor, P. M. (2013). Role of amino acid transporters in amino acid sensing. Am. J. Clin. Nutr. 99, 223–230.
Trevino, J. G., George, S. A., Hughes, S. J., and Chellappan, S. P. (2008). An in vivo and in vitro assessment of TOR signaling cascade in rainbow trout (Oncorhynchus mykiss). Am. J. Physiol. 295, 329–335. doi: 10.1152/ajpregu.00146.2008
Wu, G. (2010). Functional amino acids in growth, reproduction, and health. Adv. Nutr. 1, 31–37. doi: 10.3945/an.110.1008
Wu, G., Bazer, F. W., Dai, Z., Li, D., Wang, J., and Wu, Z. (2014). Amino acid nutrition in animals: protein synthesis and beyond. Annu. Rev. Anim. Biosci. 2, 387–417.
Xu, D., He, G., Mai, K., Wang, Q., Li, M., Zhou, H., et al. (2017). Effect of fish meal replacement by plant protein blend on amino acid concentration, transportation and metabolism in juvenile turbot (Scophthalmus maximus L.). Aquac. Nutr. 23, 1169–1178. doi: 10.1111/anu.12486
Xu, D., He, G., Mai, K., Zhou, H., Xu, W., and Song, F. (2016). Postprandial nutrient-sensing and metabolic responses after partial dietary fishmeal replacement by soyabean meal in turbot (Scophthalmus maximus L.). Br. J. Nutr. 115, 379–388. doi: 10.1017/S0007114515004535
Keywords: lysine, growth performance, nutrient-sensing signaling pathways, gene regulation, largemouth bass (Micropterus salmoides)
Citation: Wang W, Xu Y, Chi S, Yang P, Mai K and Song F (2020) Dietary Lysine Regulates Body Growth Performance via the Nutrient-Sensing Signaling Pathways in Largemouth Bass (Micropterus salmoides). Front. Mar. Sci. 7:595682. doi: 10.3389/fmars.2020.595682
Received: 17 August 2020; Accepted: 05 November 2020;
Published: 10 December 2020.
Edited by:
Kang-le Lu, Jimei University, ChinaReviewed by:
Fotini Kokou, Wageningen University and Research, NetherlandsCopyright © 2020 Wang, Xu, Chi, Yang, Mai and Song. This is an open-access article distributed under the terms of the Creative Commons Attribution License (CC BY). The use, distribution or reproduction in other forums is permitted, provided the original author(s) and the copyright owner(s) are credited and that the original publication in this journal is cited, in accordance with accepted academic practice. No use, distribution or reproduction is permitted which does not comply with these terms.
*Correspondence: Fei Song, c29uZ2ZlaUBtLnNjbnUuZWR1LmNu
†These authors have contributed equally to this work
Disclaimer: All claims expressed in this article are solely those of the authors and do not necessarily represent those of their affiliated organizations, or those of the publisher, the editors and the reviewers. Any product that may be evaluated in this article or claim that may be made by its manufacturer is not guaranteed or endorsed by the publisher.
Research integrity at Frontiers
Learn more about the work of our research integrity team to safeguard the quality of each article we publish.