- 1Department of Environmental Biology, Sapienza University of Rome, Rome, Italy
- 2CoNISMa, Rome, Italy
Determining food web architecture and its seasonal cycles is a precondition for making predictions about Antarctic marine biodiversity under varying climate change scenarios. However, few scientific data concerning Antarctic food web structure, the species playing key roles in web stability and the community responses to changes in sea-ice dynamics are available. Based on C and N stable isotope analysis, we describe Antarctic benthic food webs and the diet of species occurring in shallow waters (Tethys Bay, Ross Sea) before and after seasonal sea-ice break-up. We hypothesized that the increased availability of primary producers (sympagic algae) following sea-ice break-up affects the diet of species and thus food web architecture. Basal resources had distinct isotopic signatures that did not change after sea-ice break-up, enabling a robust description of consumer diets based on Bayesian mixing models. Sympagic algae had the highest δ13C (∼−14‰) and red macroalgae the lowest (∼−37‰). Consumer isotopic niches and signatures changed after sea-ice break-up, reflecting the values of sympagic algae. Differences in food web topology were also observed. The number of taxa and the number of links per taxon were higher before the thaw than after it. After sea-ice break-up, sympagic inputs allowed consumers to specialize on abundant resources at lower trophic levels. Foraging optimization by consumers led to a simpler food web, with lower potential competition and shorter food chains. However, basal resources and Antarctic species such as the bivalve Adamussium colbecki and the sea-urchin Sterechinus neumayeri were central and highly connected both before and after the sea-ice break-up, thus playing key roles in interconnecting species and compartments in the web. Any disturbance affecting these species is expected to have cascading effects on the entire food web. The seasonal break-up of sea ice in Antarctica ensures the availability of resources that are limiting for coastal communities for the rest of the year. Identification of species playing a key role in regulating food web structure in relation to seasonal sea-ice dynamics, which are expected to change with global warming, is central to understanding how these communities will respond to climate change.
Introduction
The persistence of biodiversity in seasonally variable environments is linked to the ability of populations to adapt to variable and/or temporally constrained resource inputs (McMeans et al., 2015). This ability is directly dependent on their trophic plasticity, a key functional trait that allows populations to persist in complex food webs (Rossi et al., 2019).
Among other factors, seasonality is a major force structuring ecological communities (Thrush and Cummings, 2011; Clark et al., 2013). Nevertheless, our knowledge of how temporal variations in the trophic niches of populations affect food web structures in real ecosystems is scarce. This limits our understanding of stability mechanisms in temporally forced ecosystems (sensu McMeans et al., 2015), thus impairing their management and conservation.
The effects of seasonality are expected to increase with latitude, with tremendous changes observed in polar areas (Clark et al., 2013; Chown et al., 2015; Ingels et al., 2020), where light and sea-ice dynamics drive the structure and functioning of aquatic food webs (Poloczanska et al., 2016; Wing et al., 2018). Sea-ice influences key ecological processes in polar ecosystems, such as phytoplankton blooms, physical disturbance of the seabed due to iceberg scouring (Gutt and Piepenburg, 2003; Arrigo, 2014), reproductive cycles, recruitment, and trophic interactions among a wide range of species (Constable et al., 2014). Specifically, sea-ice shrinking during spring, together with sea-ice break-up in summer, allows a massive increase in ice-bound primary production (sympagic production), which is subsequently released into the water column, fuelling both pelagic and benthic communities (Pusceddu et al., 1999; Lizotte, 2001; Leu et al., 2015). Under these conditions, sympagic algae represent the main direct (via vertical flux) and indirect (after sedimentation) food input for a large part of Antarctic marine diversity (Lizotte, 2001; Michel et al., 2019; Rossi et al., 2019).
The sympagic algae that live inside or on the lower surface of the sea-ice produce at least 20% of the primary biomass of the Arctic and Antarctic Oceans (Stoecker et al., 2000; Arrigo, 2014). The seasonal availability of this high-quality energy source favors trophic specialization among a large number of taxa in both pelagic and benthic communities, which are specifically adapted to the physical and biological conditions characteristic of polar environments (Knox, 2006; McMahon et al., 2006). In contrast, short but intense phytoplankton blooms occur mostly during open-sea periods (Pusceddu et al., 1999; Lizotte, 2001; Leu et al., 2015). The increased availability of basal resources after sea-ice break-up allows consumers to exploit a range of carbon sources in both the water column and on the bottom, reinforcing benthic-pelagic coupling (Cattaneo-Vietti et al., 1999; Kaehler et al., 2000; Dunton, 2001; Knox, 2006). The seasonal supply of sympagic algae thus becomes crucial to energy exchanges between ecosystem compartments, also affecting the structure and stability of communities (Hobson et al., 1995; Søreide et al., 2010; Leu et al., 2015; McMeans et al., 2015; Michel et al., 2019).
Expected changes in seasonal light and sea-ice dynamics due to climate change could directly and indirectly compromise biodiversity persistence (Clark et al., 2013; Rossi et al., 2019). These changes, together with ocean warming, could also favor the entry and success of new faster-moving and more generalist species, one of the main causes of biodiversity loss worldwide (Aronson et al., 2007; Morley et al., 2019; Hughes et al., 2020). This in turn could affect the structure and function of polar communities by altering the interactions between populations and the energy pathways between benthic and pelagic compartments (Fossheim et al., 2015; Hughes et al., 2020). Biodiversity management and conservation efforts have generally focused on the study of individual species (Jordán, 2009; McDonald-Madden et al., 2016). However, failure to consider interactions with all the other species within the community and with the physical environment can lead to ineffective management and conservation measures in the medium and long term (Trebilco et al., 2020).
In the Ross Sea (Antarctica), a biodiversity hotspot and the most pristine marine ecosystem on our planet (Rossi et al., 2019; Leihy et al., 2020), seasonal changes in sea-ice coverage are particularly marked (Wing et al., 2018). Expected changes in sea-ice dynamics due to climate change will modify the extent and intensity of algal blooms, with possible effects on benthic food webs and pelagic top predators, thus altering biodiversity architecture (Clark et al., 2013; Poloczanska et al., 2016; Mangoni et al., 2019; Hughes et al., 2020). Although our knowledge of biodiversity in the region is increasing (Chown et al., 2015), little is known about species’ functional roles in the food web, particularly in the benthic habitat, where the majority of Antarctic biodiversity lies (Rossi et al., 2019). This represents a major gap, limiting our ability to conserve biodiversity and anticipate the effects of changes in sea-ice dynamics on Antarctic food webs.
Recently, application of stable isotope analysis to Antarctic benthic communities has proved useful for describing space-time variations in nutrient fluxes associated with sea-ice dynamics (Michel et al., 2019; Rossi et al., 2019). Studies in the Ross Sea have demonstrated that sympagic production is readily incorporated into benthic biomass following sea-ice break-up (Norkko et al., 2007; Calizza et al., 2018), supporting between 30 and 80% of benthic secondary production (Wing et al., 2018). Accordingly, pulsed sympagic inputs during summer (Pusceddu et al., 2000, 2009) produce significant changes in the diet and trophic position of benthic taxa (Norkko et al., 2007; Calizza et al., 2018; Wing et al., 2018; Rossi et al., 2019), with cascade effects on predators at higher trophic levels (Leu et al., 2015; Poloczanska et al., 2016) and contaminant redistribution throughout the food web (Signa et al., 2019).
At present, quantitative data on feeding and competition links in Antarctic benthos are extremely scarce, and quantitative descriptions of the complex food web structures and their dependence on sea-ice coverage are even scarcer (Rossi et al., 2019). However, key ecological properties including stability, energy flux, vulnerability to biodiversity loss and invasibility are closely related to the complexity of food webs and the distribution of links among species (Aronson et al., 2007; Carscallen and Romanuk, 2012; Rossi et al., 2015; Calizza et al., 2019; Trebilco et al., 2020). While challenging, the quantitative analysis of food webs thus represents a necessary step toward understanding the persistence mechanisms of Antarctic communities and improving our ability to conserve Antarctic biodiversity during climate change.
In our study, for the first time we had the opportunity to track seasonal changes at the same site in both the trophic niches of populations and food web structure in the species-rich benthic Antarctic community. By means of C and N stable isotope analysis and Bayesian mixing models, we quantified (i) the isotopic niche metrics of populations, (ii) the strength of feeding and competitive interactions, and (iii) the food web properties of shallow-water benthic communities both before (hereafter BEFORE) and after (hereafter AFTER) sea-ice break-up in Tethys Bay, an inlet inside Terra Nova Bay in the Ross Sea. We sought to understand how the expected increase in resource availability following summer sea-ice break-up (Pusceddu et al., 1999; Lizotte, 2001; Leu et al., 2015) affects the trophic niches of populations and how this results in a rewiring of feeding links in the food web.
For this purpose, we measured variations in the food web structure of the shallow-water Antarctic benthic community under two different sets of sea-ice coverage conditions. Since the number of species in shallow-water Antarctic benthic communities is subject to natural seasonal variation (Gutt and Piepenburg, 2003; Thrush and Cummings, 2011; Clark et al., 2013; Ingels et al., 2020), to avoid any bias arising from species number in the interpretation of niche and food web metrics, we also considered taxa common to both study periods.
Lastly, based on food web structure analysis, we sought to quantify food web vulnerability to biodiversity loss, identifying those taxa that occupied the most critical positions in the food web and quantifying their sensitivity to changes in resource availability associated with sea-ice dynamics. In accordance with optimal foraging theory (Pyke et al., 1977; Rossi et al., 2015), we hypothesized that changes in the trophic niches of populations resulting from the increased availability of primary producers following sea-ice break-up drive the simplification of food web architecture, with effects on its stability and vulnerability to species loss.
Materials and Methods
Study Area and Sampling Activities
The study was carried out in Tethys Bay (74° 41′8″S, 164° 04′8″E), an inlet inside Terra Nova Bay in the Ross Sea (Figure 1), during the 32nd expedition of the PNRA (Italian National Antarctic Research Program).
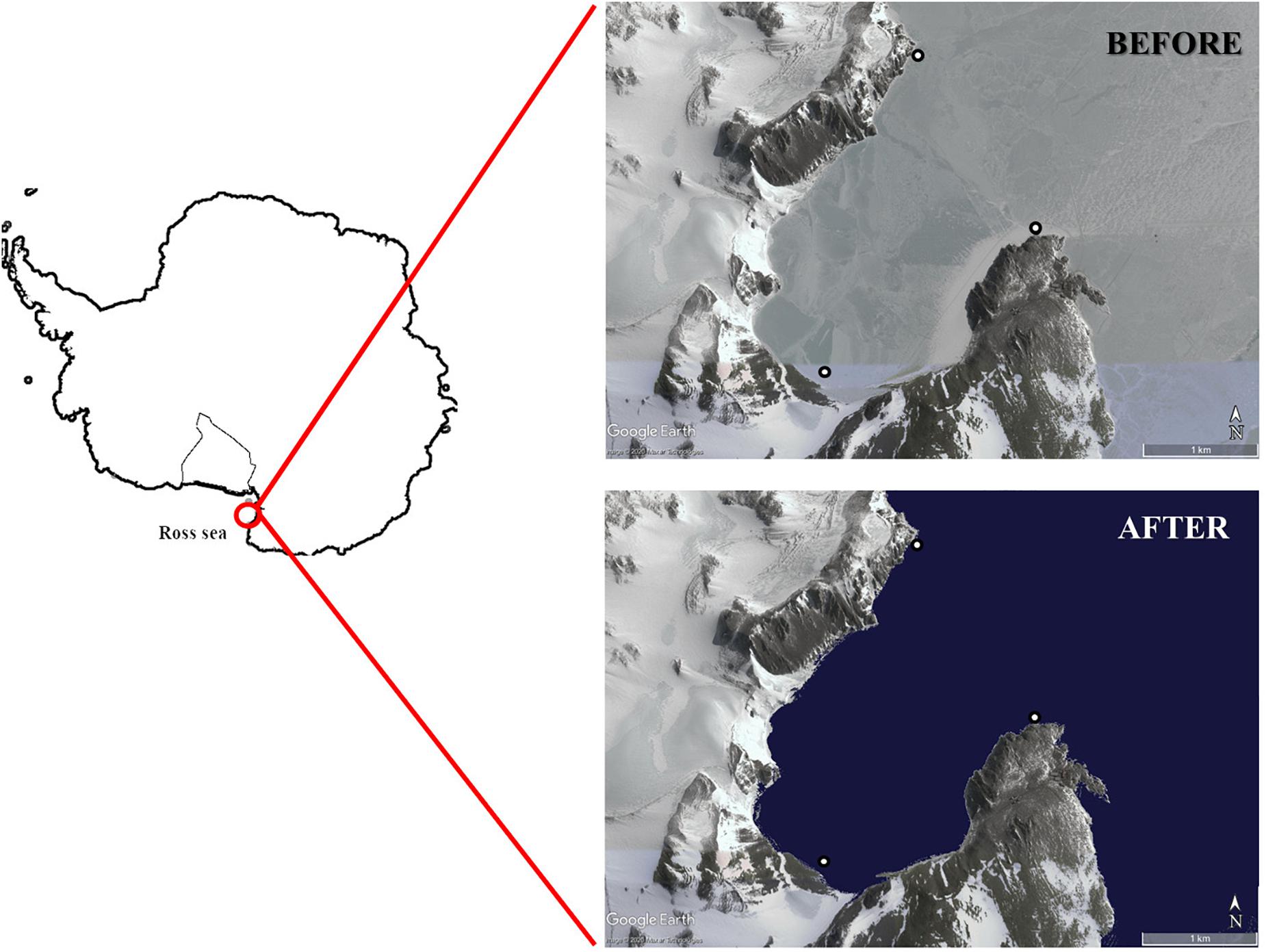
Figure 1. Sampling sites in Tethys Bay (Terra Nova Bay, Ross Sea). The background image of Tethys Bay was obtained from Google Earth.
Tethys Bay is characterized by marked seasonality in sea-ice dynamics, with coverage generally persisting until mid-December, leaving the area ice-free during the austral summer. This produces marked spatio-temporal variations in the availability of food for benthic communities (Pusceddu et al., 1999; Lizotte, 2001; Leu et al., 2015; Calizza et al., 2018). The chlorophyll-a concentrations in this area generally range between 0.1 and 4 mg m–3, with even higher values observed between January and February in association with phytoplankton blooms (Lazzara et al., 1997; Pusceddu et al., 2009; Mangoni et al., 2019). This trend was also observed in Aqua MODIS satellite images generated during our sampling periods (sources: worldview.earthdata.nasa.gov). Further information on the study area can be found in Calizza et al. (2018) and Rossi et al. (2019) and the literature cited therein.
Samplings were performed before and after sea-ice break-up, which started around 18th December 2016, leading to ice-free open water around 10th January 2017. The first round of sampling (i.e., BEFORE) was performed from 14th November to 15th December 2016. During this period, the whole study area was characterized by dark and non-turbid waters. The second round of sampling (i.e., AFTER) was performed from 19th January to 7th February 2017. During this period, icebergs flowed into and around the bay (personal observation), as usually observed in the area (Melchiori and Cincotti, 2018). The number of days elapsing between sea-ice break-up and sampling time were quantified by means of direct observations on the ground coupled with reference to satellite images (Landsat 8, target paths: 61, target row: 113).
Three sampling stations in shallow waters were chosen (Figure 1), characterized by mixed rocky and soft bottoms and varying slopes in order to take full account of the environmental heterogeneity of this area (Cattaneo-Vietti et al., 1999). All possible invertebrate organisms and basal resources were collected both BEFORE and AFTER. The collection and analysis of samples was carried out by the same field and laboratory research team in both sampling periods using similar sampling methods.
Benthic invertebrates, detritus (organic matter in coarse, fine and ultra-fine sediments) and macroalgae (Iridaea cordata and Phyllophora antarctica) with associated epiphytes were collected by SCUBA divers at a depth of 10–30 m. Small invertebrates, such as polychaetes and amphipods, were collected by carefully separating them from sediments or other sampled benthic specimens, mainly sponges.
Sediment samples were sieved and grouped on the basis of particle diameter into Coarse (>1 mm), Fine (between 1 and 0.56 mm) and Ultra-fine (<0.56 mm) fractions in accordance with Calizza et al. (2018).
Sympagic algae (diatoms and filamentous algal aggregates present in the ice core and at the ice-water interface) were collected by coring the ice-pack three times at each sampling site. Three replicates of sympagic algae per ice-core (i.e., a total of 27 samples) were collected. The filamentous and diatom fractions were separated from each other by sieving and filtering through Whatman GF/F filters. The sympagic diatoms and filamentous algal aggregates in Tethys Bay are mainly composed of species belonging to the genera Amphiprora and Nitzschia, respectively (Lazzara et al., 1995; Saggiomo et al., 2017 for further details).
Phytoplankton and zooplankton were collected by vertically sampling the water column at a depth of 20 m with a plankton net (20-μm mesh size) from sea-ice holes BEFORE and in open waters AFTER. Each zooplankton specimen was carefully separated from the bulk sample under a stereoscope. Phytoplankton was collected by filtering the remaining water sample at 100 μm and then collected on pre-combusted Whatman GF/F filters. In our study area, the phytoplankton assemblages are dominated by Phaeocystis antarctica (Fabiano et al., 1997; Mangoni et al., 2019).
Since coprophagy is a common strategy among Antarctic benthic invertebrates (McClintock, 1994; Norkko et al., 2007), samples of seal feces from a colony of Weddell seals (Leptonychotes weddellii, Lesson, 1826) were also collected. The seal colony consisted of around 80–100 specimens usually stationed in the sampling area. Basal resources (including the upper 5 cm layer of sediments and seal feces) were collected within the first week of sampling activities in both sampling periods. They were analyzed in order to measure differences between BEFORE and AFTER in the isotopic baseline of consumer diets and food web architecture.
All collected samples were sorted, counted, identified to the finest possible taxonomic level and transported to Italy to be properly processed for the stable isotope analysis.
Laboratory Procedures and Stable Isotope Analysis
Samples were individually stored at −80°C and then freeze-dried for 24 h before Stable Isotope Analysis (SIA).
Specific soft tissues were taken from the large invertebrates (Norkko et al., 2007; Careddu et al., 2017; Calizza et al., 2018; Rossi et al., 2019). For small invertebrates (such as amphipods and polychaetes), the whole body was used. These samples were individually analyzed. In addition, samples of very small organisms (e.g., nematodes) were grouped in order to obtain sufficient biomass for stable isotope analyses (Hobson et al., 1995; Rossi et al., 2015). The dry sample was homogenized to a fine powder using an agate mortar and pestle or a ball mill (Mini-Mill Fritsch Pulverisette 23: Fritsch Instruments, Idar-Oberstein, Germany). Where necessary, samples were pre-acidified using 1M HCl drop-by-drop (Hobson et al., 1995) in order to eliminate inorganic carbon, which can interfere with the δ13C signature, and were then re-dried at 60°C for 72 h. δ15N was measured in non-acidified powders to avoid possible interference of HCl with the nitrogen signatures (Calizza et al., 2018; Rossi et al., 2019).
Aliquots of 0.50 ± 0.10 mg for animals and 4.00 ± 1.00 mg for basal resources were placed in tin capsules for the carbon and nitrogen SIA. Samples were then analyzed in two replicates using a continuous flow mass spectrometer (IsoPrime100, Isoprime Ltd., Cheadle Hulme, United Kingdom) coupled with an elemental analyzer (Elementar Vario Micro-Cube, Elementar Analysensysteme GmbH, Germany). Carbon (C) and Nitrogen (N) isotopic signatures were expressed in δ units (δ13C and δ15N, respectively) as parts per thousand (‰) deviations from international standards (atmospheric N2 for N; Vienna-PDB for C). The isotope ratios were calculated relative to the respective standard following the equation: δX (‰) = [(Rsample − Rstandard)/Rstandard] × 103 (McKinney et al., 1950; Vander Zanden and Rasmussen, 1996), where X is 13C or 15N and R is the corresponding ratio of heavy to light isotope for the element (13C/12C or 15N/14N). Caffeine IAEA-600 (C8H10N4O2) was used as the internal laboratory standard. Measurement errors associated with the linearity and stability of the mass spectrometer were typically smaller than 0.05‰, while the standard deviation of repeated measurements of lab standard material (one replicate every 10 analyses) was typically ± 0.1‰ for δ13C and ± 0.2‰ for δ15N.
Isotopic Niche Metrics
The isotopic niche metrics of populations at both sampling times were calculated in accordance with Layman et al. (2007) and Jackson et al. (2011). These metrics, originally proposed for application at community level, can also be used at population level to obtain information on trophic diversity within a single population (Layman et al., 2007; Jackson et al., 2011; Careddu et al., 2017; Sporta Caputi et al., 2020). Carbon Range (CR) and Nitrogen Range (NR) were measured as the difference between the lowest and highest δ13C and δ15N values, respectively. CR provides information about the variety of food sources exploited by the population and hence its degree of trophic generalism. NR quantifies the degree of omnivory of the population, accounting for inter-specimen variation in trophic position.
The isotopic niche width was measured as the total area (TA) of the convex hull encompassing all the isotopic values of individuals within each population. It is expressed as the isotopic area (‰2) and allows comparison of isotopic niches between populations. The overlap between the isotopic niches of populations was calculated and expressed both as a percentage of TA and as the number of overlaps for each taxon (R software ver. 3.5.3, SIBER analysis package, Jackson et al., 2011). Both TA and isotopic niche overlap were measured for populations common to BEFORE and AFTER with a sample size greater than or equal to 4 individuals (18 animal taxa in total). Although low, this sample size allowed us to include naturally scarce taxa in the comparison of isotopic niche metrics between study periods. The number was also less limiting for species diet reconstruction based on Bayesian Mixing Models.
The overall competitive effect was expressed as the diffuse overlap (sensu MacArthur, 1972 and Pianka, 1974), measured as the average overlapping TA multiplied by the average number of overlapping taxa.
Food Webs
The diet of each taxon was estimated by means of Bayesian Mixing Models (SIMMr package, R software ver. 3.5.3, Parnell et al., 2010; Parnell and Inger, 2016). The inputs of the model were: (i) the isotopic signatures of the target consumer, (ii) the mean isotopic signatures and relative standard deviation of potential food sources, and (iii) the Trophic Enrichment Factor (TEF) of the consumer. The TEFs between consumers and their potential food sources (expressed as the mean ± standard deviation) were 0.4 ± 0.2‰ for δ13C and 2.3 ± 0.4‰ for δ15N (Calizza et al., 2018; Costantini et al., 2018; Rossi et al., 2019). The models return the probability distribution of the proportional contribution of each food source to the consumer’s diet, taking account of the uncertainty in the source and Trophic Enrichment Factor (TEF) values (Parnell et al., 2010; Jackson et al., 2011; Parnell and Inger, 2016; Careddu et al., 2017; Costantini et al., 2018; Rossi et al., 2019). The set of initial potential food sources for each consumer was assigned based on literature data and/or its position in the bi-dimensional isotopic space with respect to that of the target consumer (Costantini et al., 2018; Rossi et al., 2019). Since a high number of resources can reduce the discriminatory ability of mixing models and thus the effectiveness of Stable Isotope Analysis in food web reconstruction, for each taxon, a multiple-step procedure, in accordance with Costantini et al. (2018), was applied. Based on the output of the Bayesian isotopic Mixing Models, the pool of real (i.e., likely to be assimilated) food sources for each consumer was then selected in accordance with Costantini et al. (2018) and Rossi et al. (2019). At each step in the mixing model, a food source was maintained if either (i) its modal contribution was at least 5% and a contribution of 0% did not lie within the 75 or 95% confidence intervals or (ii) the food source was necessary in order to obtain the mixing space that enclosed the consumer.
Finally, following the assignment of all the trophic links, the structures and the respective metrics of the food webs were obtained using the software (Cytoscape 3.7.2 and R-Statistical 3.5.3). Although a generally accepted classification of Antarctic organisms into trophic guilds is limited by their marked trophic generalism and omnivory, purely in order to improve food web representation, taxa were grouped into broad “feeding modes” based on bibliographical information (McClintock, 1994; Dunton, 2001; Gillies et al., 2013). These included: “suspension feeder,” “deposit feeder,” “omnivorous/grazer,” “omnivorous/predator,” and “predator.”
Food Web Metrics and Data Analysis
The food web metrics of the BEFORE and AFTER communities were calculated and compared using the Cheddar and NetworkExtinction packages in R-software (Hudson et al., 2013; Corcoran et al., 2019) and Cytoscape 3.7.2.
In the food web, the nodes are the taxa (S) and the links (L) are the feeding links from resources to consumers. The Linkage density (L/S) was measured as the average number of feeding links per taxon present in the food web. The minimum Connectance (Cmin) was calculated as: Cmin = 2L/S2. The trophic position (TP) of each taxon (i) was calculated in accordance with the formula: TPi = 1 + Σj (TPj × fij), where TPj is the trophic position of food item j and f is the proportional consumption of food item j by consumer i (Odum and Heald, 1975). TP = 1 was assigned to basal food sources. The proportional similarity of diet among taxa was measured by the Bray–Curtis similarity index. The index is based on the contribution of each resource to the diet of each consumer and it ranges from 0, when no common resources are found for the compared groups, to 1, when the compared groups have the same resources in the same proportions (Calizza et al., 2018; Costantini et al., 2018; Sporta Caputi et al., 2020).
The potential competition for resources was measured in accordance with Levins’ (1968) equation:
in which the competition strength αi,j is expressed as the effect of species j on species i, where pik and pjk are the proportional contributions of any given resource k to species i and j, respectively.
Topological food web metrics were also measured. These included Betweenness Centrality (BC), Neighborhood Connectivity (NC) and food web robustness to taxon (i.e., node) loss. BC is a measure of the centrality of a taxon in the food web. This parameter indicates the importance of a taxon in terms of the role it plays in connecting other nodes in the network (Freeman, 1977). Taxa with BC > 0 play a central role in connecting others within the food web (Martín González et al., 2010). Neighborhood Connectivity (NC) indicates the average connectivity of each taxon in the food web and provides a measure of interconnection (direct and indirect) among all taxa in the food web. Food web robustness to loss of taxa was estimated by simulating primary extinctions (sensu Dunne et al., 2002). Taxa were sequentially removed from the most to the least connected, in accordance with Calizza et al. (2012) and Rossi et al. (2019). Secondary extinction occurs when a taxon loses all of its food items.
Statistics
If not specified otherwise, the results are reported as mean ± standard error (SE). The Shapiro–Wilk normality test was applied to each dataset to verify that it followed a Gaussian distribution.
Differences between sampling periods were tested using the Student’s t-test for variables that fulfilled the normality assumption (similarity of diet, competition strength, number of competitors) and the Mann-Whitney test when normality was not observed (mean trophic position and food chain, Neighborhood Connectivity). The same tests were also used to test differences in the contribution of resources to the diet of BEFORE and AFTER communities.
Two-way PERMANOVA and associated post hoc comparisons were used to test for the effect of taxon and period (i.e., BEFORE and AFTER) on the isotopic signatures of basal resources and consumers. A Mantel test was performed in order to assess the correlation between the matrices of isotopic Euclidean distances and the Bray-Curtis diet similarity (Signa et al., 2019).
Finally, the difference between BEFORE and AFTER in terms of the proportion of the total number of links in the web accounted for by feeding links between resources and consumers was assessed using Chi-square (χ2) tests, while ANCOVA was used to test the difference in total extinction distribution. In order to avoid the potential confounding effects of differences in taxonomic composition between the BEFORE and AFTER communities, further comparisons of isotopic and food web metrics included only consumers common to both sampling periods and were performed using paired t-tests and Wilcoxon paired tests for inter-period comparison of variables with normal and non-normal distribution, respectively.
Results
Isotopic Niche and Species Diet
A total of 1732 specimens belonging to 61 animal taxa were sampled, 34 of which were common to the BEFORE and AFTER samples (Table 1 and Figure 2). Sediments, sympagic algae and animal-derived food (live prey or carrion) were the resource items that most contributed to the diet of benthic organisms in both sampling periods (Figure 3).
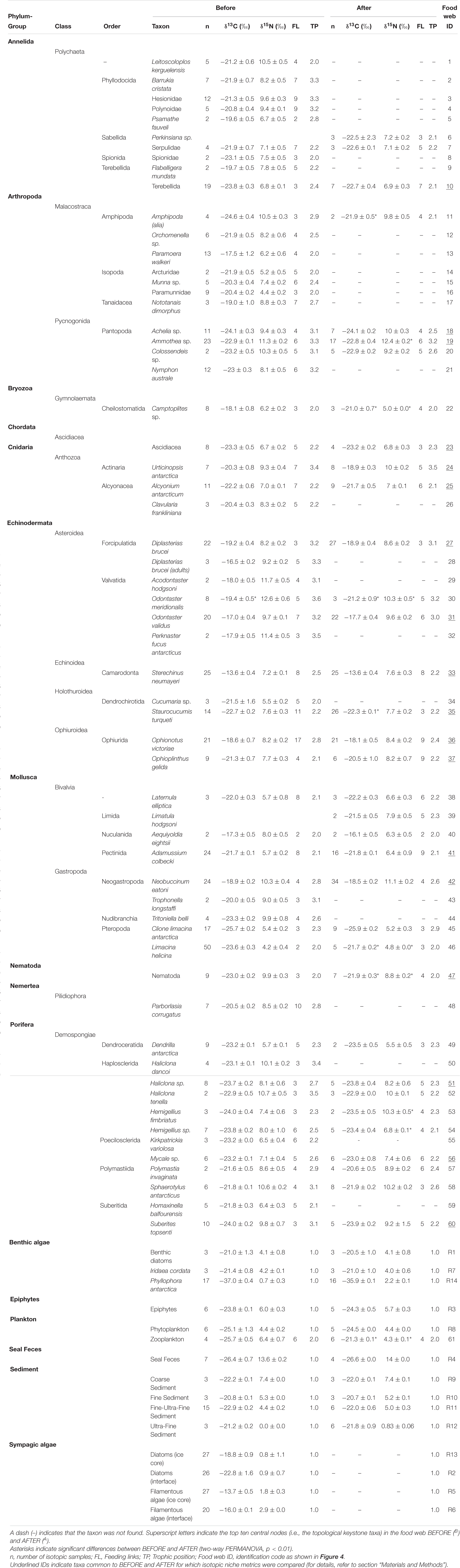
Table 1. Community carbon (δ13C) and nitrogen (δ15N) isotopic signatures (mean ± SE) BEFORE and AFTER.
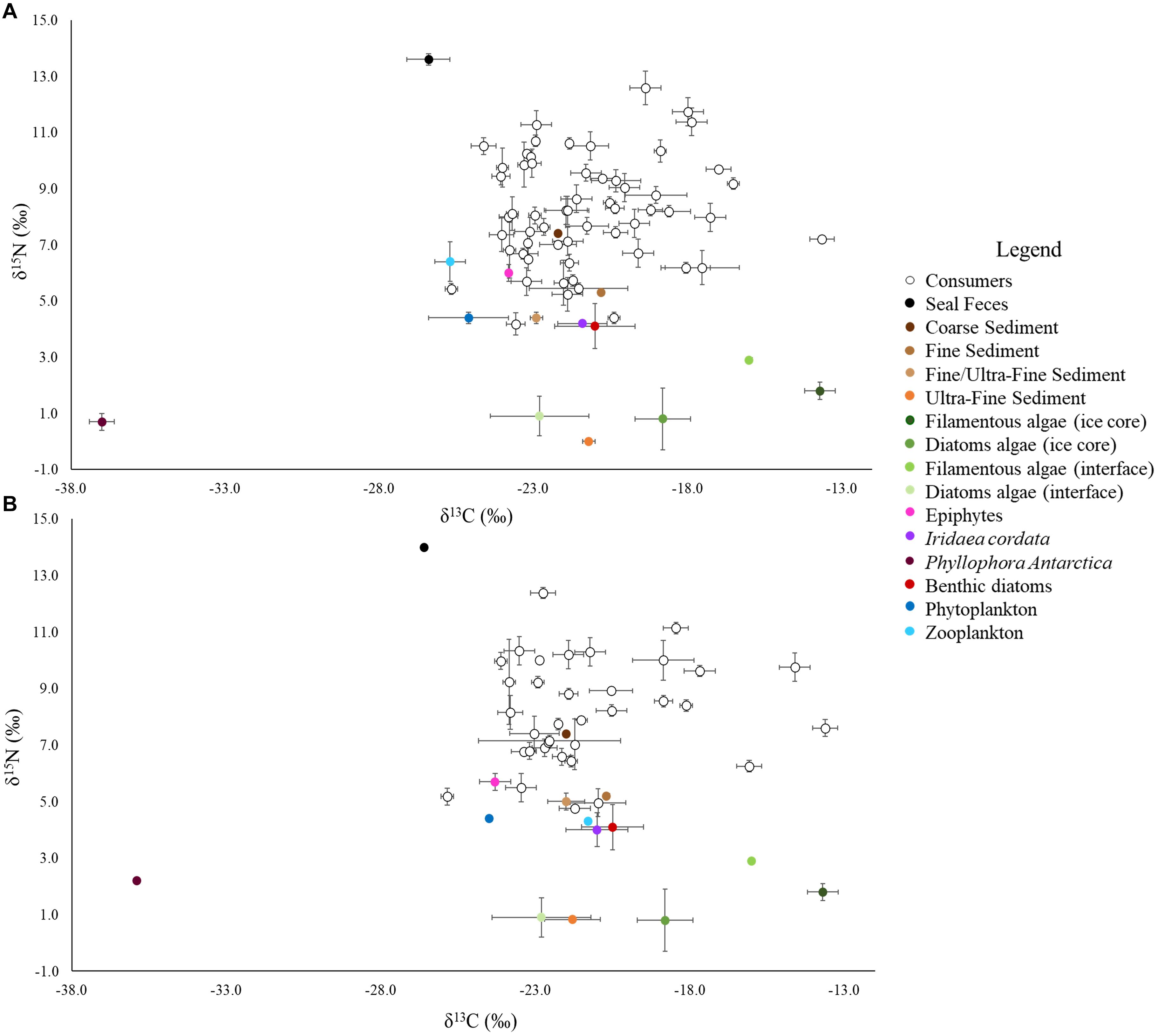
Figure 2. Isotopic biplots of the BEFORE (A) and AFTER (B) communities. Each point represents a taxon. Isotopic signatures were expressed as the mean ± SE. The legend shows the respective colors of consumers and basal resources.
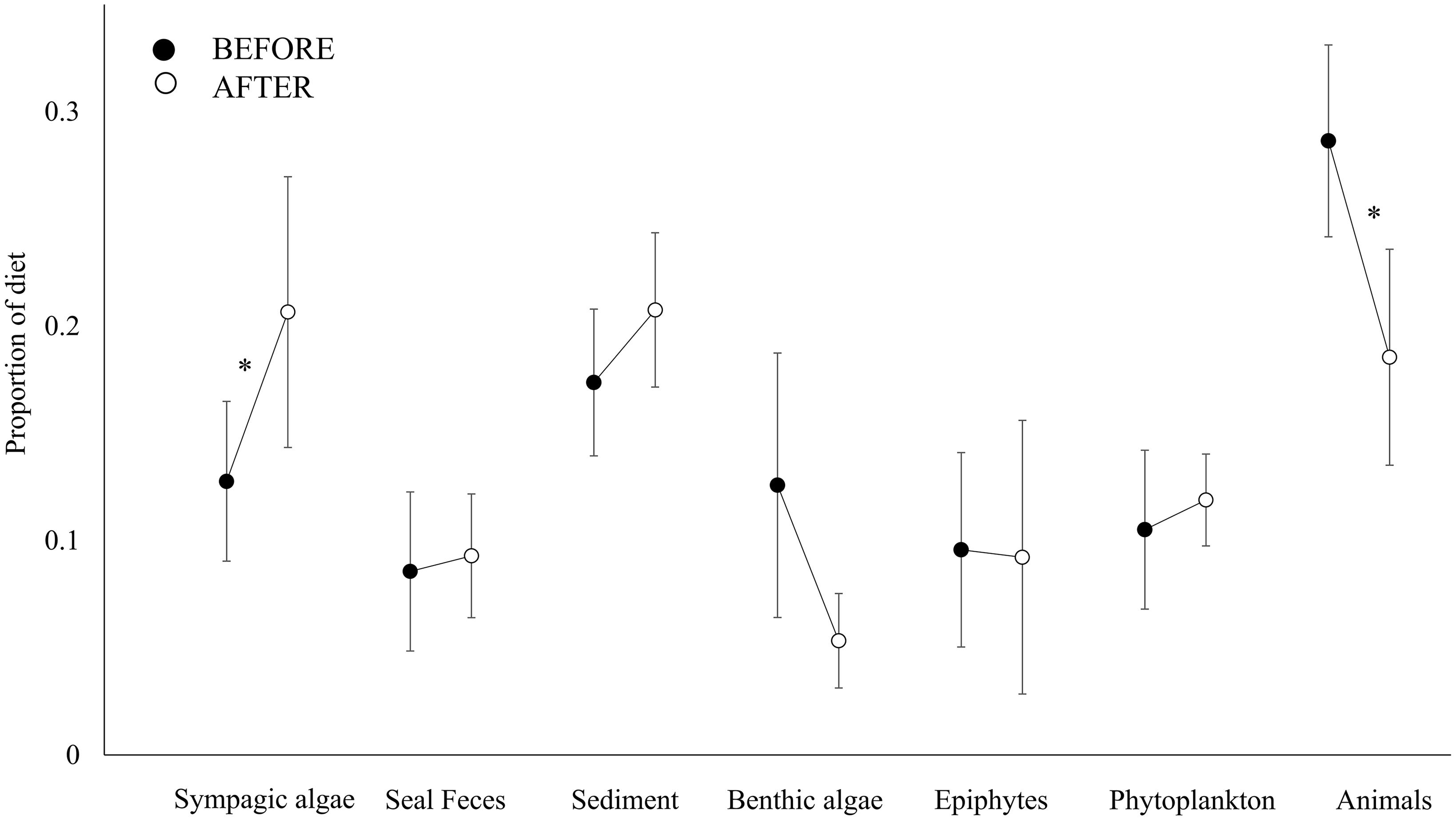
Figure 3. Contribution of basal resources (Sympagic algae, Seal feces, Sediment, Benthic algae, Epiphytes and Phytoplankton) and animal-derived resources (Animals) to the diet BEFORE (full symbols) and AFTER (empty symbols). The proportional contribution of each item is reported as the mean ± SE. Superscript symbols (*) indicate a significant difference between periods (see also section “Results”).
Basal resources were isotopically distinct from each other (although interface diatoms had similar values to the ultra-fine fraction), and their BEFORE and AFTER values did not differ (Figure 2; Two-way PERMANOVA, Fresource = 99.17, p < 0.0001; Ftime = 2.32 and Finteraction = −2.20, n.s.; pairwise post hoc comparisons). Among the basal food sources, the red macroalga Phyllophora antarctica and sympagic algae (especially filamentous algae) had the lowest and highest δ13C values (respectively, −36.7 ± 0.3‰ and −13.7 ± 0.5‰), while the ultra-fine sediment fraction and seal feces had the lowest and the highest δ15N values (respectively, −0.04 ± 0.02‰ and 13.6 ± 0.1‰; Figure 2).
While no differences between sampling periods were found in the δ13C distribution within the community as a whole, the δ13C values of taxa common to the two periods were higher in the AFTER sample (Wilcoxon paired test, z = 2.2, p < 0.05), with values closer to those typical of sympagic algae (Table 1 and Figure 2). Yet differences between groups were also observed. Zooplankton and Amphipoda were the most 13C-depleted consumers BEFORE, with values denoting dependence on phytoplankton and detritus chains. Amphipoda, which occupied the outermost area of the isotopic niche space close to the benthic compartment resources, had more enriched δ13C values, similar to those of sympagic algae, AFTER. The sea urchin Sterechinus neumayeri had the highest δ13C among consumers, showing dependence on sympagic algae in both periods, although this dependence was more pronounced AFTER. Other Antarctic key species such as the predaceous sea star Odontaster meridionalis and the anemone Urticinopsis antarctica occupied a central position in the isotopic space, with intermediate δ13C and high δ15N values, indicating consumption of materials mainly of animal origin, both BEFORE and AFTER (Table 1 and Figure 2).
The mean isotopic niche width (Total Area) of taxa common to both periods decreased from 7.60 ± 1.3‰2 to 6.05 ± 1.3‰2 (Table 2), and the Carbon Range decreased from 5.2 ± 0.8‰ to 3.5 ± 0.5‰ (paired t-test, t: 1.78, p < 0.01), but individual taxa displayed distinctive trends. While the brittle star Ophionotus victoriae, the sea-star Diplasterias brucei and the anemone Urticinopsis antarctica mirrored the general trends, with decreasing Total Area and CR after the sea-ice break-up, the scallop Adamussium colbecki, the brittle star Ophioplinthus gelida and the sea-urchin Sterechinus neumayeri increased their Total Area along with NR.
The closer the taxa in the isotopic niche space, the more similar their diet according to the Bayesian mixing models (Mantel test, Correlation R: 0.5, p < 0.001). Within trophic guilds, the isotopic signatures of taxa were rather heterogeneous (e.g., the two congeneric seastars Odontaster meridionalis and O. validus and the two bivalves Aequiyoldia eightsii and Adamussium colbecki, Table 1). Consistent with the changes in the isotopic distribution of animal taxa, the contribution of sympagic algae to their diet increased after the sea-ice break-up (t-test, t: 2.49, p < 0.05), while that of animal-derived food decreased (Mann-Whitney test, U: 466, p < 0.01, Figure 3). As a result, the diet composition of the various taxa became more similar (Bray-Curtis: 15.07 ± 0.86% and 22.58 ± 1.86%; t-test, p < 0.01). Among other resources, the contribution of seal feces to consumers’ diets was low in both BEFORE and AFTER.
The mean potential competition strength based on isotopic niches increased but the number of competitor taxa decreased (t-test, t at least: 2.37, p < 0.01, Tables 1, 3). The lower number of competitors, together with the generally narrower isotopic niche in AFTER (Table 2), was reflected in a lower diffuse niche overlap between taxa (from 235.9 ± 36.4% to 196.1 ± 33.6%; paired t-test, t: 2.3, p < 0.05.
Food Web Structures
The two periods were characterized by different food web topology and metrics (Table 3). Both the total number of feeding links and the number of food chains in the web were higher BEFORE than AFTER (Figures 4, 5A and Table 3). Specifically, the feeding links between consumers and basal food sources increased from 52% to 66% of the total (Table 1, χ2-test, χ2:10, p < 0.01) and Compartmentalization slightly increased from 0.10 to 0.13 (Table 3). Furthermore, although a similarly, high degree of omnivory was found in the BEFORE and AFTER food webs, the food chains were shorter on average (Figures 4, 5A) and the mean trophic position was thus lower AFTER than BEFORE (Table 1 and Figure 4; Mann-Whitney test, U:1323.5, p < 0.05).
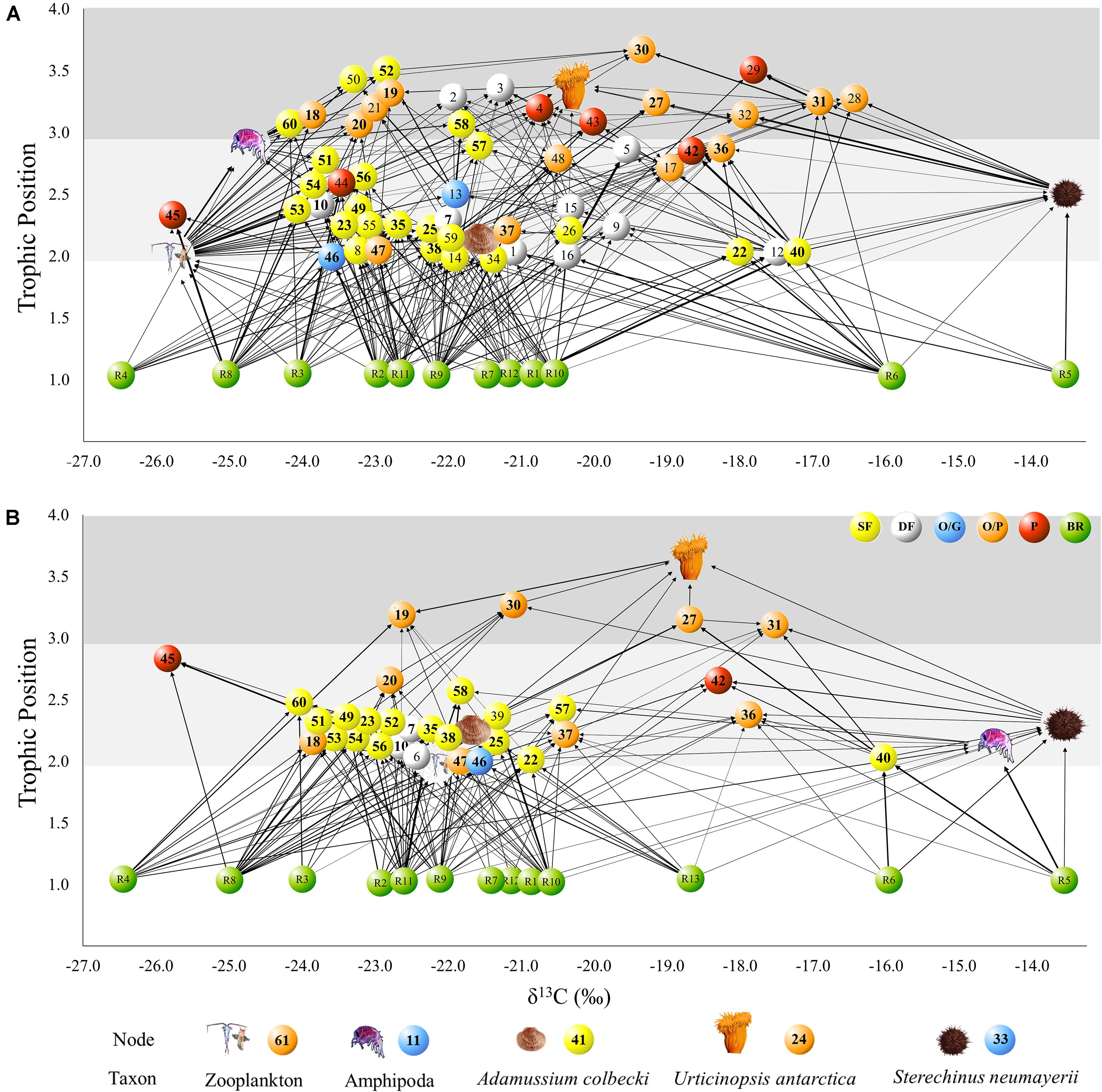
Figure 4. Benthic food web structure in Tethys Bay, Terra Nova Bay (Ross Sea): BEFORE (A) and AFTER (B). Each node represents one taxon in the community. Arrows point from a resource to its consumer and their thickness is proportional to the linkage strength. The position of each node represents its δ13C and Trophic Position (TP). Colors indicate the dominant feeding mode of each taxon: yellow: suspension feeder; white: deposit feeder; blue: omnivorous/grazer; orange: omnivorous/predator; red: predator. Basal resources are shown in green. The food web graphs were developed using Cytoscape software. Bold IDs indicate taxa sampled in both study periods. For the list of taxa please refer to Table 1.
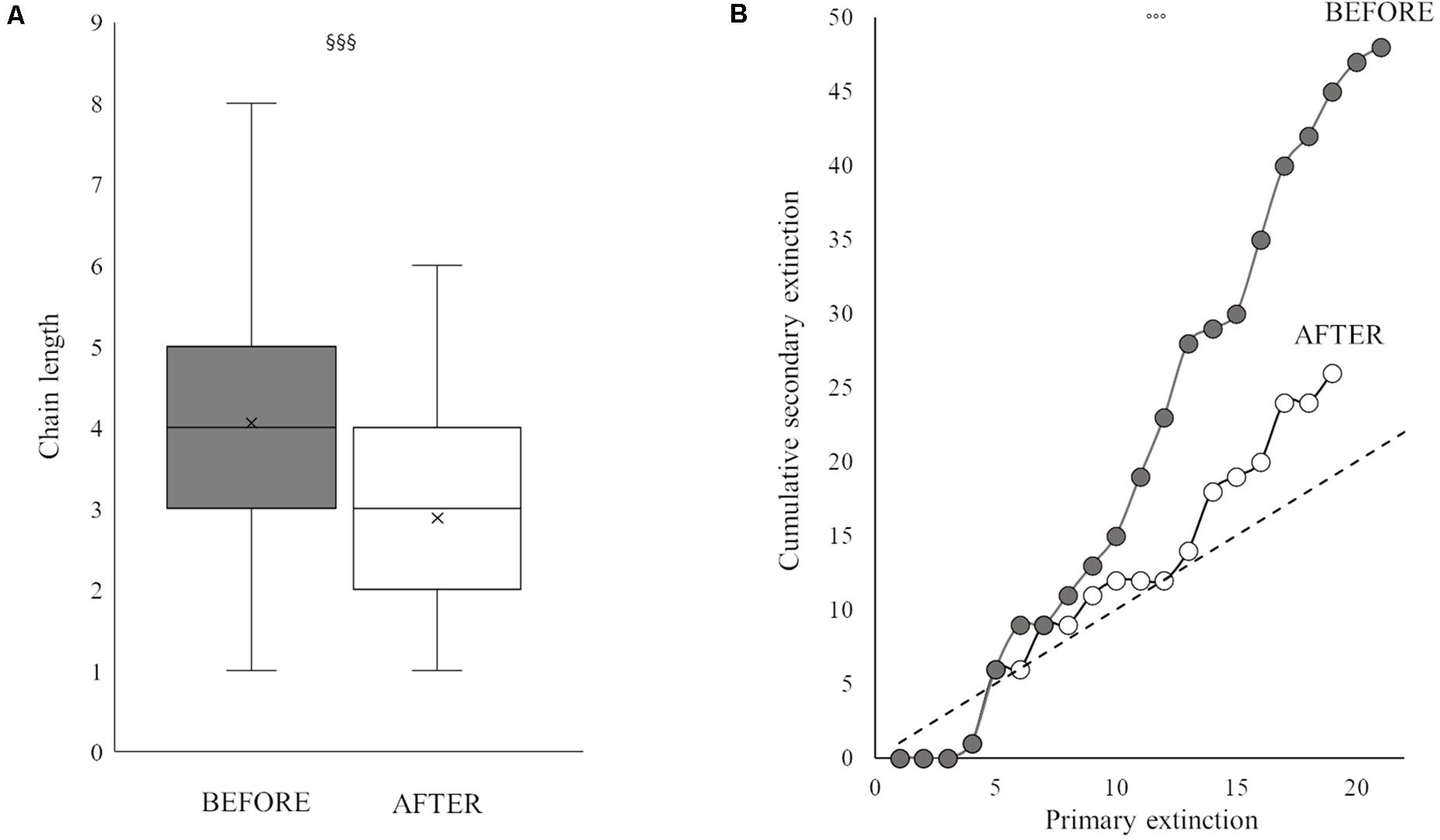
Figure 5. Box plot of mean chain length (A) and robustness trend (primary extinction against cumulative secondary extinction) (B) BEFORE (gray) and AFTER (white). Superscript symbols (§ and ∘) indicate a significant difference between periods (see also “Results” section). One, two and three superscript symbols indicate p values of <0.05, <0.01, and <0.001, respectively.
The trophic position of consumers varied between 2.0 and 3.6 (Figure 4). Urticinopsis antarctica, Odontaster meridionalis, Ammothea sp., D. brucei, and O. validus had a high trophic position, equal to or greater than 3, in both sampling periods. Another 14 taxa had a similarly, high trophic position BEFORE but, of those species present in both periods, the sea-spiders Colossendeis sp. and Achelia sp. and the sponges Sphaerotylus antarcticus, Haliclona tenella, and Suberites topsenti were found at lower trophic positions AFTER (Table 1). More generally, at the guild level, the common suspension feeders, including sponges, had significantly lower trophic positions AFTER (Table 1 and Figure 4; Wilcoxon test, W: 263, p < 0.01).
As observed for the community as a whole (Table 3), the taxa common to both sampling periods were less connected (both directly and indirectly) with others in the AFTER food web (NC: 14.37 ± 0.73 vs. 11.39 ± 0.60, Wilcoxon test, W: 1009, p < 0.001). The mean linkage density decreased (Table 2, Wilcoxon test, W: 659, p < 0.01), together with both the number of food items in the diet and potential competitors (Tables 1, 2; Wilcoxon test, W: 594, and W: 638, p < 0.0001, respectively). Specifically, the most generalist taxa BEFORE saw the greatest reductions in the number of consumed resources AFTER (e.g., O. victoriae and the sea cucumber Staurocucumis turqueti), while a small number of specialist taxa had a higher number of trophic links AFTER (e.g., Terebellidae and the brittle star Ophioplinthus gelida).
These differences influenced the topology of the food web and its robustness to the loss of highly connected nodes, which was higher in the AFTER food web (Table 3 and Figure 5B, ANCOVA test, F: 125.1, p < 0.001). Highly connected nodes BEFORE included zooplankton and Amphipoda (including Paramoera walkeri), whereas AFTER they included Ophioplintus gelidae, Aequiyoldia eightsii, Terebellidae and D. brucei. Basal resources and S. neumayeri were highly connected in both periods. Except for A. eightsii and basal resources, these taxa also ranked among the 10 most central nodes in the food web structure in terms of their Betweenness Centrality (Figure 4), with much higher consumption of sympagic algae AFTER.
Discussion
Antarctic benthic organisms are markedly adapted to their extreme environment, which is characterized by long-term low availability of food resources, which are closely linked to seasonal sea-ice dynamics (Pusceddu et al., 2000; Clark et al., 2013; Calizza et al., 2018; Rossi et al., 2019). Thus, changes in sea-ice spatio-temporal dynamics, which are expected in all climate change scenarios (Schofield et al., 2010; Constable et al., 2014), will determine changes in resource inputs, affecting biodiversity persistence and ecosystem structure and functioning (Pusceddu et al., 2000; Norkko et al., 2007; Clark et al., 2013; Constable et al., 2014; Calizza et al., 2018; Michel et al., 2019; Rossi et al., 2019).
In this study, community isotopic characterization, coupled with diet and food web reconstruction, provided crucial support for understanding and describing the response of Antarctic coastal benthic communities to the availability of basal resources under two different sea-ice coverage conditions (Calizza et al., 2015; Rossi et al., 2015, 2019). The results highlighted significant changes in the trophic niches of Antarctic benthic populations dwelling in shallow waters, as well as in food web architecture, after the sea-ice break-up and its associated input of sympagic algae. In addition, they allowed us to identify the main carbon pathways that support the benthic populations, including taxa that play a key role in the organization of these complex communities.
The isotopic signatures of basal resources were clearly distinct and highly conserved over time. This allowed a robust description of consumers’ diets based on isotopic Bayesian Mixing Models. In addition, values were similar to what has been observed in other study areas (Kaehler et al., 2000; Gillies et al., 2013) and in Tethys Bay in different seasons and years and at a range of depths (Norkko et al., 2007; Calizza et al., 2018; Rossi et al., 2019), demonstrating the slow pace of biochemical transformation. The observed isotopic similarity of phytoplankton over time suggests that samples collected in dark waters before the sea-ice break-up are the result of horizontal transport to the coast from open waters, as already reported for the study area (Pusceddu et al., 1999; Norkko et al., 2007). The isotopic signature of the ultra-fine sediment fraction (particle diameter <0.56 mm) did not differ significantly from that of sympagic diatoms. Other studies have found isotopic similarity between sediments and sympagic algae, suggesting that these algae are available to benthic organisms both as fresh material when released into the water column and as a small but constant supply of organic matter in the sediment (Lizotte, 2001; Wing et al., 2018). The latter constitutes an important food bank in the unproductive season (Mincks et al., 2008), consistent with several studies carried out in the study area (Cattaneo-Vietti et al., 1999; Pusceddu et al., 2000). Biochemical studies show that the organic matter on the bottom in the neritic zone of the area was largely undegraded (Fabiano and Pusceddu, 1998; Pusceddu et al., 1999). The isotopic similarity suggests negligible biogeochemical transformations of organic carbon and nitrogen following production, and thus minimal change in food quality, which might impact the proportions of carbon and nitrogen sources and their apparent incorporation in benthic communities over time. By comparing the short- and long-term diets of the sea urchin Sterechinus neumayeri, Calizza et al. (2018) demonstrated the rapid availability of sympagic material for the benthos within a few days of sea-ice break-up. Similarly, Wing et al. (2018) observed that the contribution of sympagic algae to the secondary productivity of Antarctic benthos increased near the edge of the sea ice soon after the summer break-up. However, this may not represent a general pattern in polar areas (Gibson et al., 1999; Lovvorn et al., 2005). Biochemical processes in sediments associated with the short- and long-term deposition of sympagic and pelagic matter after sea-ice break-up thus require further studies in order to improve our understanding of carbon and nitrogen pathways and recycling in the Antarctic, especially in the light of climate change.
Overall, sediments, sympagic algae and animal-derived matter contributed most to the diet of organisms, both before and after the sea-ice break-up. This is consistent with what is generally observed in Antarctic benthic communities (Dunton, 2001; Norkko et al., 2007; Smale et al., 2007; Gillies et al., 2012; Michel et al., 2019), including in our study area but at a greater depth (Rossi et al., 2019). The change in diet following the sea-ice break-up was taxon-dependent and was reflected in changes in both the carbon (δ13C) and nitrogen (δ15N) signatures and in the trophic niche of the populations, regardless of their feeding mode (ranging from filter feeders to predators). Indeed, while isotopic similarity among taxa predicted similarity in their diet, taxa that are generally grouped within broad trophic guilds in the classification of Antarctic benthos were not always isotopically similar (i.e., the composition of their diet differed), as in the case of the two omnivorous predators Odontaster meridionalis and O. validus and the two suspension feeders Aequiyoldia eightsii and Adamussium colbecki.
Before the sea-ice break-up, consumers showed 13C-depleted values typical of benthic resources (Norkko et al., 2007; Calizza et al., 2018; Michel et al., 2019; Rossi et al., 2019). Their wide isotopic niches and Carbon Ranges suggested high trophic generalism within the community in this period (Layman et al., 2007; Jackson et al., 2011; Careddu et al., 2017; Sporta Caputi et al., 2020). After the sea-ice break-up, the increased availability of sympagic algae allowed consumers to specialize on this carbon source, making the coupling of distinct energy channels less marked (Kaehler et al., 2000; Dunton, 2001; Knox, 2006). Specifically, the opportunistic brittle star Ophionotus victoriae and sea-star Diplasterias brucei, the micro-predators/suspension feeders Alcyonium antarcticum (soft coral) and Ascidiacea, and the predator/scavenger anemone Urticinopsis antarctica are characterized by the highest trophic plasticity among Antarctic invertebrates (McClintock, 1994; Norkko et al., 2007; Marina et al., 2018). These taxa had a narrower range of resources in their diet after the sea-ice break-up than before. On the other hand, the trophic niches of the sea-star Odontaster validus and the sea-urchin Sterechinus neumayeri widened after the sea-ice break-up, and their δ13C indicated that sympagic algae made an important contribution to their diets in both sampling periods, in accordance with previous observations (Corbisier et al., 2004; Jacob et al., 2006; Norkko et al., 2007; Rossi et al., 2019).
During the Antarctic winter, benthic communities in shallow waters obtain large quantities of carbon from benthic algae, detritus accumulated in sediment (Kaehler et al., 2000; Dunton, 2001; Mincks et al., 2008; McMeans et al., 2015) and animal-derived matter (McClintock, 1994; Gillies et al., 2012). Predation and necrophagy, which are common in these communities, are considered successful strategies above all when basal resource inputs are limiting and in the presence of disturbance caused by ice-scouring (McClintock, 1994; Smale et al., 2007; Dunlop et al., 2014). The latter reduces the diversity and abundance of benthic animals and algae (McClintock, 1994; Smale et al., 2007; Dunlop et al., 2014; Ingels et al., 2020), with a substantial impact on the local availability of food sources. The incidence of ice-scouring is generally known to be higher in shallow than in medium-depth waters (Gutt and Piepenburg, 2003), although the availability of sympagic algae is greater, and can explain the lower number of taxa found after than before the sea-ice break-up. Both factors can result in lower competitive pressure and greater stability of the community in shallow waters. Benthic consumers, mainly Antarctic echinoderms, may opportunistically feed on seal feces when this resource is available (McClintock, 1994 and literature cited therein). Considering that Weddell seals forage mainly in medium-depth waters (more than 100 m) (Burns et al., 1998), we hypothesize that the observed low consumption of seal feces was related to their low availability for shallow-water benthic consumers in our study area. The low consumption of feces was also evident from the distinct isotopic signatures of this resource compared to those of consumers both before and after sea-ice break-up. However, further studies of the seasonal availability of this resource for Antarctic benthic organisms are necessary.
Compared to other basal resources for polar benthic organisms, sympagic algae are higher quality (McMahon et al., 2006; Pusceddu et al., 2009). The higher consumption of sympagic algae led to a reduction in both the overall trophic position and the length of food chains in the web, as also observed in other studies based on stable isotope analyses (Gillies et al., 2012; Michel et al., 2019; Rossi et al., 2019). This is consistent with expectations from theory (Kondoh and Ninomiya, 2009), observations from temperate aquatic systems (Calizza et al., 2012) and previous observations in our study area (Norkko et al., 2007; Rossi et al., 2019), and may be related to increased community resilience, theoretically associated with shorter food chains (Pimm and Lawton, 1977).
Sympagic algae are both released in small quantities from sea ice during their growth (i.e., before sea-ice break-up), and in a strong pulse for a short time during and soon after the melting of sea ice (Clarke, 1988; Cattaneo-Vietti et al., 1999; Pusceddu et al., 2009; Leu et al., 2015). After the ice melt, this abundant food source quickly becomes available to consumers (in a few hours in shallow waters and in a few days in medium-depth waters), which then adopt a more specialized diet and increase feeding (Calizza et al., 2018; Michel et al., 2019; Rossi et al., 2019) and reproductive activities (Pearse et al., 1991; Knox, 2006). This was observed both at the level of the entire food web and in species common to the two study periods. Generalist taxa reduced their trophic niches and the number of links after the sea-ice break-up, when the availability and consumption of basal resources increased. However, the fact that certain more specialist taxa increased the number of trophic links after the sea-ice break-up suggests that the lower number of taxa did not generate bias in the linkage density measurements.
Changes in foraging behavior following the ice melt affected the entire food web, leading to its simplification. Specifically, before the sea-ice break-up, the community was composed of a high number of densely linked species. The mean numbers of trophic positions and potential competitors per taxon were found to be high, in accordance with the literature (McMeans et al., 2015; Michel et al., 2019). Web simplification observed after the sea-ice break-up is consistent with the findings of Rossi et al. (2019). In their “space for time” study of the effects of sea-ice dynamics on the medium-depth benthic communities, the food webs were based on isotopic trophospecies, or Isotopic-Trophic Units (i.e., groups of individuals that share a similar position in the food web). After the sea-ice break-up, linkage density decreased and the trophic diversity within populations increased, lowering competitive interactions but increasing the vulnerability to the loss of highly connected ITUs. In contrast, in our study, the lower connectivity between species observed when resources were less limiting made food webs less vulnerable to the loss of taxa (Elton, 1927; Pimm and Lawton, 1977; Dunne et al., 2002; Kondoh and Ninomiya, 2009). Indeed, lower connectivity can reduce the risk of disturbance propagation (Calizza et al., 2019). In this context, highly interconnected populations could accelerate disturbance propagation along food chains via both direct effects, such as predation, and indirect effects, such as competition (Estrada, 2007; Lai et al., 2012; Marina et al., 2018). Specifically, when simulating a scenario of species loss from the most to the least connected species (Dunne et al., 2002; Staniczenko et al., 2010), secondary extinctions were boosted by the loss of central nodes in the food web. This suggested that the topological position of the species in the food web plays a greater role in the propagation of disturbance and hence in maintaining the stability of the community than the number of trophic links (Allesina and Pascual, 2009; Staniczenko et al., 2010). Keystone species, which generally dominate the Antarctic benthic communities (sea urchins, scallops, anemones, and amphipods) were key topological nodes in our food webs. Disturbance arising from changes in seasonal sea-ice dynamics directly and indirectly affecting these topological keystone populations can spread rapidly via trophic cascades, leading to food web collapse or abrupt rewiring (Estrada, 2007).
Bayesian mixing models proved to be a useful tool for tracking changes in food web structure based on shifts in species’ diets. However, the presence of a large number of possible food sources linked to the high degree of generalism and trophic plasticity of Antarctic benthic species (Norkko et al., 2007; Carscallen and Romanuk, 2012; Dunlop et al., 2014) may limit the models’ power of discrimination and thus the reconstruction of food webs in complex communities (Rossi et al., 2019). Our previous knowledge of the studied system, along with the results of the multi-step mixing models and the rigorous selection of each resource based on the probability of its contribution to a consumer’s diet, made it possible to disentangle the diets of species belonging to this complex community. Nevertheless, the combination of stable isotopes with other biochemical tracers (e.g., fatty acids) (North et al., 2019) and the isotopic analysis of limiting amino-acids (Larsen et al., 2013) would further improve our ability to discern and understand the contribution of the various carbon sources to Antarctic food webs and their vulnerability in the face of changes in sea-ice coverage.
Concluding Remarks
In conclusion, the marked seasonality of sea-ice dynamics in Antarctica ensures the summer availability of resources that are limiting for the rest of the year (Chown et al., 2015; Rossi et al., 2019). Spatio-temporal changes in sea-ice dynamics can affect the trophic choices of species populations within the community, with cascade effects on the entire food web (Pusceddu et al., 2000; Leu et al., 2015; Poloczanska et al., 2016; Calizza et al., 2018; Rossi et al., 2019). Coastal marine Antarctic ecosystems, which are biodiversity hotspots closely associated with sea-ice, are seeing significant changes in physical and environmental conditions as a result of ongoing climate change (Michel et al., 2019; Leihy et al., 2020; Rogers et al., 2020). In these complex ecosystems, identifying key species in terms of community stability under a range of sea-ice coverage conditions requires the isotopic characterization of the community and the quantification of intra- and interspecific links between benthic populations, providing useful information for the management and conservation of biodiversity. Our study location (Tethys Bay, in Terra Nova Bay) is in the middle of the recently established Ross Sea marine protected area, and it is an Antarctic biodiversity hotspot. Our comprehensive dataset, in which several taxa were characterized by analyzing a substantial number of specimens, enabled a very robust estimation of species’ isotopic niches and diet composition, useful for the reconstruction of the food web. This dataset includes taxa spanning multiple feeding modes and trophic levels, as well as abundant circumpolar species playing key ecological roles in Antarctic coastal ecosystems.
In addition, the study of pristine biodiverse communities in the Ross Sea also represents a unique opportunity to explore stability mechanisms in seasonally forced food webs that are not affected by human pressure (Norkko et al., 2007; Chown et al., 2015; Cummings et al., 2018; Wing et al., 2018; Rossi et al., 2019; Leihy et al., 2020). Some genera and families included in this study are not limited to polar areas, being also found at lower latitudes and in anthropised coastal areas. Hence, studying them in the Ross Sea makes it possible to observe mechanisms of trophic plasticity and adaptation to temporal variations in food sources under undisturbed conditions.
Data Availability Statement
The raw data supporting the conclusions of this article will be made available by the authors, without undue reservation.
Author Contributions
LR and MLC: conceptualization, supervision, and funding acquisition. EC and GC: field sampling. SSC, EC, GC, FF, and DM: investigation. SSC and GC: formal analysis and visualization. LR, MLC, and SSC: writing – original draft preparation. All authors contributed to the writing – review and editing.
Funding
This work was supported by PNRA-2015/AZ1.01 (MLC) and PNRA16_00291 (LR) granted by MIUR-PNRA and the APC was funded by Progetti di Ricerca di Ateneo-RM11916B88AD5D75 (EC).
Conflict of Interest
The authors declare that the research was conducted in the absence of any commercial or financial relationships that could be construed as a potential conflict of interest.
Acknowledgments
We thank Mr. George Metcalf for revising the English text and two Reviewers for their comments, which improved the manuscript. We also thank Simone D’Alessandro, Lorenzo Maria Iozia, and Flaminia Tito for the help with the preliminary processing of the samples.
References
Allesina, S., and Pascual, M. (2009). Googling food webs: can an eigenvector measure species’ importance for coextinctions? PLoS Comput. Biol. 5:e1000494. doi: 10.1371/journal.pcbi.1000494
Aronson, R. B., Thatje, S., Clarke, A., Peck, L. S., Blake, D. B., Wilga, C. D., et al. (2007). Climate Change and Invasibility of the Antarctic Benthos. Annu. Rev. Ecol. Evol. Syst. 38, 129–154. doi: 10.1146/annurev.ecolsys.38.091206.095525
Arrigo, K. R. (2014). Sea ice ecosystems. Annu. Rev. Mar. Sci. 6, 439–467. doi: 10.1146/annurev-marine-010213-135103
Burns, J. M., Trumble, S. J., Castellini, M. A., and Testa, J. W. (1998). The diet of Weddell seals in McMurdo Sound, Antarctica as determined from scat collections and stable isotope analysis. Polar Biol. 19, 272–282. doi: 10.1007/s003000050245
Calizza, E., Careddu, G., Caputi, S. S., Rossi, L., and Costantini, M. L. (2018). Time- and depth-wise trophic niche shifts in Antarctic benthos. PLoS One 13:e0194796. doi: 10.1371/journal.pone.0194796
Calizza, E., Costantini, M. L., and Rossi, L. (2015). Effect of multiple disturbances on food web vulnerability to biodiversity loss in detritus-based systems. Ecosphere 6, 1–20. doi: 10.1890/ES14-00489.1
Calizza, E., Costantini, M. L., Rossi, D., Carlino, P., and Rossi, L. (2012). Effects of disturbance on an urban river food web. Freshw. Biol. 57, 2613–2628. doi: 10.1111/fwb.12033
Calizza, E., Rossi, L., Careddu, G., Sporta Caputi, S., and Costantini, M. L. (2019). Species richness and vulnerability to disturbance propagation in real food webs. Sci. Rep. 9:19331. doi: 10.1038/s41598-019-55960-8
Careddu, G., Calizza, E., Costantini, M. L., and Rossi, L. (2017). Isotopic determination of the trophic ecology of a ubiquitous key species – The crab Liocarcinus depurator (Brachyura: Portunidae). Estuar. Coast. Shelf Sci. 191, 106–114. doi: 10.1016/j.ecss.2017.04.013
Carscallen, W. M. A., and Romanuk, T. N. (2012). Structure and robustness to species loss in Arctic and Antarctic ice-shelf meta-ecosystem webs. Ecol. Model. 245, 208–218. doi: 10.1016/j.ecolmodel.2012.03.027
Cattaneo-Vietti, R., Chiantore, M., Misic, C., Povero, P., and Fabiano, M. (1999). The role of pelagic-benthic coupling in structuring littoral benthic communities at Terra Nova Bay (Ross Sea) and in the straits of magellan. Sci. Mar. 63, 113–121. doi: 10.3989/scimar.1999.63s1113
Chown, S. L., Clarke, A., Fraser, C. I., Cary, S. C., Moon, K. L., and McGeoch, M. A. (2015). The changing form of Antarctic biodiversity. Nature 522, 431–438. doi: 10.1038/nature14505
Clark, G. F., Stark, J. S., Johnston, E. L., Runcie, J. W., Goldsworthy, P. M., Raymond, B., et al. (2013). Light-driven tipping points in polar ecosystems. Glob. Change Biol. 19, 3749–3761. doi: 10.1111/gcb.12337
Clarke, A. (1988). Seasonality in the Antarctic marine environment. Comp. Physiol. Biochem. 90B, 89–99.
Constable, A. J., Melbourne-Thomas, J., Corney, S. P., Arrigo, K. R., Barbraud, C., Barnes, D. K. A., et al. (2014). Climate change and Southern Ocean ecosystems I: how changes in physical habitats directly affect marine biota. Glob. Change Biol. 20, 3004–3025. doi: 10.1111/gcb.12623
Corbisier, T. N., Petti, M. A. V., Skowronski, R. S. P., and Brito, T. A. S. (2004). Trophic relationships in the nearshore zone of Martel Inlet (King George Island, Antarctica): δ13C stable-isotope analysis. Polar Biol. 27, 75–82. doi: 10.1007/s00300-003-0567-z
Corcoran, D., Ávila-Thieme, M. I., Valdovinos, F. S., Navarrete, S. A., and Marquet, P. A. (2019). NetworkExtinction v0. 1.1. Available online at: https://CRAN.R-project.org/package = NetworkExtinction (accessed March 19, 2020).
Costantini, M. L., Carlino, P., Calizza, E., Careddu, G., Cicala, D., Sporta Caputi, S., et al. (2018). The role of alien fish (the centrarchid Micropterus salmoides) in lake food webs highlighted by stable isotope analysis. Freshw. Biol. 63, 1130–1142. doi: 10.1111/fwb.13122
Cummings, V. J., Hewitt, J. E., Thrush, S. F., Marriott, P. M., Halliday, N. J., and Norkko, A. (2018). Linking ross sea coastal benthic communities to environmental conditions: documenting baselines in a spatially variable and changing world. Front. Mar. Sci. 5:232. doi: 10.3389/fmars.2018.00232
Dunlop, K. M., Barnes, D. K. A., and Bailey, D. M. (2014). Variation of scavenger richness and abundance between sites of high and low iceberg scour frequency in Ryder Bay, west Antarctic Peninsula. Polar Biol. 37, 1741–1754. doi: 10.1007/s00300-014-1558-y
Dunne, J. A., Williams, R. J., and Martinez, N. D. (2002). Food-web structure and network theory: the role of connectance and size. PNAS 99, 12917–12922. doi: 10.1073/pnas.192407699
Dunton, K. H. (2001). δ15N and δ13C measurements of antarctic peninsula fauna: trophic relationships and assimilation of benthic seaweeds. Integr. Comp. Biol. 41, 99–112. doi: 10.1093/icb/41.1.99
Estrada, E. (2007). Characterization of topological keystone species: local, global and “meso-scale” centralities in food webs. Ecol. Complexity 4, 48–57. doi: 10.1016/j.ecocom.2007.02.018
Fabiano, M., Povero, P., Cattaneo-Vietti, R., Pusceddu, A., Misic, C., and Albertelli, G. (1997). Short-term variations in particulate matter flux in Terra Nova Bay, Ross Sea. Antarctic Sci. 9, 143–149. doi: 10.1017/S0954102097000187
Fabiano, M., and Pusceddu, A. (1998). Total and hydrolizable particulate organic matter (carbohydrates, proteins and lipids) at a coastal station in Terra Nova Bay (Ross Sea, Antarctica). Polar Biol. 19, 125–132. doi: 10.1007/s003000050223
Fossheim, M., Primicerio, R., Johannesen, E., Ingvaldsen, R. B., Aschan, M. M., and Dolgov, A. V. (2015). Recent warming leads to a rapid borealization of fish communities in the Arctic. Nat. Clim. Change 5, 673–677. doi: 10.1038/nclimate2647
Freeman, L. C. (1977). A set of measures of centrality based on betweenness. Sociometry 40, 35–41. doi: 10.2307/3033543
Gibson, J. A. E., Trull, T., Nichols, P. D., Summons, R. E., and McMinn, A. (1999). Sedimentation of 13C-rich organic matter from Antarctic sea-ice algae: a potential indicator of past sea-ice extent. Geology 27, 331–334. doi: 10.1130/0091-7613(1999)027<0331:socrom>2.3.co;2
Gillies, C. L., Stark, J. S., Johnstone, G. J., and Smith, S. D. A. (2012). Carbon flow and trophic structure of an Antarctic coastal benthic community as determined by δ13C and δ15N. Estuar. Coast. Shelf Sci. 97, 44–57. doi: 10.1016/j.ecss.2011.11.003
Gillies, C. L., Stark, J. S., Johnstone, G. J., and Smith, S. D. A. (2013). Establishing a food web model for coastal Antarctic benthic communities: a case study from the Vestfold Hills. Mar. Ecol. Prog. Ser. 478, 27–41. doi: 10.3354/meps10214
Gutt, J., and Piepenburg, D. (2003). Scale-dependent impact on diversity of Antarctic benthos caused by grounding of icebergs. Mar. Ecol. Prog. Ser. 253, 77–83. doi: 10.3354/meps253077
Hobson, K. A., Ambrose, W. G., and Renaud, P. E. (1995). Sources of primary production, benthic-pelagic coupling, and trophic relationships within the Northeast Water Polynya: insights from δ 13 C and δ 15 N analysis. Mar. Ecol. Prog. Ser. 128, 1–10. doi: 10.3354/meps128001
Hudson, L. N., Emerson, R., Jenkins, G. B., Layer, K., Ledger, M. E., Pichler, D. E., et al. (2013). Cheddar: analysis and visualisation of ecological communities in R. Methods Ecol. Evol. 4, 99–104. doi: 10.1111/2041-210X.12005
Hughes, K. A., Pescott, O. L., Peyton, J., Adriaens, T., Cottier-Cook, E. J., Key, G., et al. (2020). Invasive non-native species likely to threaten biodiversity and ecosystems in the Antarctic Peninsula region. Glob. Change Biol. 26, 2702–2716. doi: 10.1111/gcb.14938
Ingels, J., Aronson, R. B., Smith, C. R., Baco, A., Bik, H. M., Blake, J. A., et al. (2020). Antarctic ecosystem responses following ice-shelf collapse and iceberg calving: science review and future research. WIREs Clim. Change n/a:e682. doi: 10.1002/wcc.682
Jackson, A. L., Inger, R., Parnell, A. C., and Bearhop, S. (2011). Comparing isotopic niche widths among and within communities: SIBER – stable isotope bayesian ellipses in R. J. Anim. Ecol. 80, 595–602. doi: 10.1111/j.1365-2656.2011.01806.x
Jacob, U., Brey, T., Fetzer, I., Kaehler, S., Mintenbeck, K., Dunton, K., et al. (2006). Towards the trophic structure of the Bouvet Island marine ecosystem. Polar Biol. 29, 106–113. doi: 10.1007/s00300-005-0071-8
Jordán, F. (2009). Keystone species and food webs. Philos. Trans. R. Soc. B Biol. Sci. 364, 1733–1741. doi: 10.1098/rstb.2008.0335
Kaehler, S., Pakhomov, E. A., and McQuaid, C. D. (2000). Trophic structure of the marine food web at the Prince Edward Islands (Southern Ocean) determined by δ13C and δ15N analysis. Mar. Ecol. Prog. Ser. 208, 13–20. doi: 10.3354/meps208013
Kondoh, M., and Ninomiya, K. (2009). Food-chain length and adaptive foraging. Proc. R. Soc. B Biol. Sci. 276, 3113–3121. doi: 10.1098/rspb.2009.0482
Lai, S.-M., Liu, W.-C., and Jordán, F. (2012). On the centrality and uniqueness of species from the network perspective. Biol. Lett. 8, 570–573. doi: 10.1098/rsbl.2011.1167
Larsen, T., Ventura, M., Andersen, N., O’Brien, D. M., Piatkowski, U., and McCarthy, M. D. (2013). Tracing carbon sources through aquatic and terrestrial food webs using amino acid stable isotope fingerprinting. PLoS One 8:e73441. doi: 10.1371/journal.pone.0073441
Layman, C. A., Arrington, D. A., Montaña, C. G., and Post, D. M. (2007). Can stable isotope ratios provide for community-wide measures of trophic structure? Ecology 88, 42–48. doi: 10.1890/0012-9658(2007)88[42:csirpf]2.0.co;2
Lazzara, L., Massi, L., Nuccio, C., and Biondi, N. (1997). “Phytoplankton ecology: irradiance, particles, gilvin, pigments, absorption, fluorescence, production and species density in Terra Nova Bay, Ross Sea,” in ROSSMIZE 93-95, eds F.M. Faranda, L. Guglielmo, and P. Povero (Alexandria: National Program of Antarctic), 229–279.
Lazzara, L., Nuccio, C., Massi, L., and Innamorati, M. (1995). Le microalghe simpagiche di Baia Terra Nova (Antartide), nell’estate 1994/95. Giornale Bot. Ital. 129, 425–425. doi: 10.1080/11263509509436156
Leihy, R. I., Coetzee, B. W. T., Morgan, F., Raymond, B., Shaw, J. D., Terauds, A., et al. (2020). Antarctica’s wilderness fails to capture continent’s biodiversity. Nature 583, 567–571. doi: 10.1038/s41586-020-2506-3
Leu, E., Mundy, C. J., Assmy, P., Campbell, K., Gabrielsen, T. M., Gosselin, M., et al. (2015). Arctic spring awakening – Steering principles behind the phenology of vernal ice algal blooms. Prog. Oceanogr. 139, 151–170. doi: 10.1016/j.pocean.2015.07.012
Levins, R. (1968). Evolution in Changing Environments: Some Theoretical Explorations. Princeton, NJ: Princeton University Press.
Lizotte, M. P. (2001). The contributions of sea ice algae to antarctic marine primary production. Integr. Comp. Biol. 41, 57–73. doi: 10.1093/icb/41.1.57
Lovvorn, J. R., Cooper, L. W., Brooks, M. L., Ruyck, C. C. D., Bump, J. K., and Grebmeier, J. M. (2005). Organic matter pathways to zooplankton and benthos under pack ice in late winter and open water in late summer in the north-central Bering Sea. Mar. Ecol. Prog. Ser. 291, 135–150. doi: 10.3354/meps291135
Mangoni, O., Saggiomo, M., Bolinesi, F., Castellano, M., Povero, P., Saggiomo, V., et al. (2019). Phaeocystis antarctica unusual summer bloom in stratified antarctic coastal waters (Terra Nova Bay, Ross Sea). Mar. Environ. Res. 151:104733. doi: 10.1016/j.marenvres.2019.05.012
Marina, T. I., Salinas, V., Cordone, G., Campana, G., Moreira, E., Deregibus, D., et al. (2018). The food web of potter cove (Antarctica): complexity, structure and function. Estuar. Coast. Shelf Sci. 200, 141–151. doi: 10.1016/j.ecss.2017.10.015
Martín González, A. M., Dalsgaard, B., and Olesen, J. M. (2010). Centrality measures and the importance of generalist species in pollination networks. Ecol. Complexity 7, 36–43. doi: 10.1016/j.ecocom.2009.03.008
McClintock, J. B. (1994). Trophic biology of antarctic shallow-water echinoderms. Mar. Ecol. Prog. Ser. 111, 191–202. doi: 10.3354/meps111191
McDonald-Madden, E., Sabbadin, R., Game, E. T., Baxter, P. W. J., Chadès, I., and Possingham, H. P. (2016). Using food-web theory to conserve ecosystems. Nat. Commun. 7:10245. doi: 10.1038/ncomms10245
McKinney, C. R., McCrea, J. M., Epstein, S., Allen, H. A., and Urey, H. C. (1950). Improvements in mass spectrometers for the measurement of small differences in isotope abundance ratios. Rev. Sci. Instr. 21, 724–730. doi: 10.1063/1.1745698
McMahon, K. W. Jr., Ambrose, W. G., Johnson, B. J., Sun, M.-Y., Lopez, G. R., Clough, L. M., et al. (2006). Benthic community response to ice algae and phytoplankton in Ny Ålesund, Svalbard. Mar. Ecol. Prog. Ser. 310, 1–14. doi: 10.3354/meps310001
McMeans, B. C., McCann, K. S., Humphries, M., Rooney, N., and Fisk, A. T. (2015). Food web structure in temporally-forced ecosystems. Trends Ecol. Evol. 30, 662–672. doi: 10.1016/j.tree.2015.09.001
Melchiori, V., and Cincotti, V. (2018). Antartide il Report della XXXI e XXXII Spedizione. Available online at: https://iris.enea.it/handle/20.500.12079/5133#.X4rc9NAzZZU (accessed October 1, 2020).
Michel, L. N., Danis, B., Dubois, P., Eleaume, M., Fournier, J., Gallut, C., et al. (2019). Increased sea ice cover alters food web structure in East Antarctica. Sci. Rep. 9:8062. doi: 10.1038/s41598-019-44605-5
Mincks, S. L., Smith, C. R., Jeffreys, R. M., and Sumida, P. Y. G. (2008). Trophic structure on the West Antarctic Peninsula shelf: detritivory and benthic inertia revealed by δ13C and δ15N analysis. Deep Sea Res. II Top. Stud. Oceanogr. 55, 2502–2514. doi: 10.1016/j.dsr2.2008.06.009
Morley, S. A., Barnes, D. K. A., and Dunn, M. J. (2019). Predicting which species succeed in climate-forced Polar Seas. Front. Mar. Sci. 5:507. doi: 10.3389/fmars.2018.00507
Norkko, A., Thrush, S. F., Cummings, V. J., Gibbs, M. M., Andrew, N. L., Norkko, J., et al. (2007). Trophic structure of coastal antarctic food webs associated with changes in sea ice and food supply. Ecology 88, 2810–2820. doi: 10.1890/06-1396.1
North, C. A., Lovvorn, J. R., Kolts, J. M., Cooper, L. W., and Grebmeier, J. M. (2019). Discriminating trophic niches of carnivorous benthic macroinvertebrates with gut contents, stable isotopes, and fatty acids. Mar. Ecol. Prog. Ser. 631, 49–66. doi: 10.3354/meps13161
Odum, W. E., and Heald, E. J. (1975). The detritus-based food web of an. Estuar. Res. Chem. Biol. Estuar. Syst. 1:265.
Parnell, A. C., Inger, R., Bearhop, S., and Jackson, A. L. (2010). Source partitioning using stable isotopes: coping with too much variation. PLoS One 5:e9672. doi: 10.1371/journal.pone.0009672
Pearse, J. S., Mcclintock, J. B., and Bosch, I. (1991). Reproduction of antarctic benthic marine invertebrates: tempos, modes, and timing. Integr. Comp. Biol. 31, 65–80. doi: 10.1093/icb/31.1.65
Pianka, E. R. (1974). Niche overlap and diffuse competition. PNAS 71, 2141–2145. doi: 10.1073/pnas.71.5.2141
Pimm, S. L., and Lawton, J. H. (1977). Number of trophic levels in ecological communities. Nature 268, 329–331. doi: 10.1038/268329a0
Poloczanska, E. S., Burrows, M. T., Brown, C. J., García Molinos, J., Halpern, B. S., Hoegh-Guldberg, O., et al. (2016). Responses of marine organisms to climate change across oceans. Front. Mar. Sci. 3:62. doi: 10.3389/fmars.2016.00062
Pusceddu, A., Cattaneo-Vietti, R., Albertelli, G., and Fabiano, M. (1999). Origin, biochemical composition and vertical flux of particulate organic matter under the pack ice in Terra Nova Bay (Ross Sea, Antarctica) during late summer 1995. Polar Biol. 22, 124–132. doi: 10.1007/s003000050399
Pusceddu, A., Dell’Anno, A., and Fabiano, M. (2000). Organic matter composition in coastal sediments at Terra Nova Bay (Ross Sea) during summer 1995. Polar Biol. 23, 288–293. doi: 10.1007/s003000050446
Pusceddu, A., Dell’Anno, A., Vezzulli, L., Fabiano, M., Saggiomo, V., Cozzi, S., et al. (2009). Microbial loop malfunctioning in the annual sea ice at Terra Nova Bay (Antarctica). Polar Biol. 32, 337–346. doi: 10.1007/s00300-008-0539-4
Pyke, G. H., Pulliam, H. R., and Charnov, E. L. (1977). Optimal foraging: a selective review of theory and tests. Q. Rev. Biol. 52, 137–154. doi: 10.1086/409852
Rogers, A. D., Frinault, B. A. V., Barnes, D. K. A., Bindoff, N. L., Downie, R., Ducklow, H. W., et al. (2020). Antarctic futures: an assessment of climate-driven changes in ecosystem structure, function, and service provisioning in the Southern Ocean. Annu. Rev. Mar. Sci. 12, 87–120. doi: 10.1146/annurev-marine-010419-011028
Rossi, L., di Lascio, A., Carlino, P., Calizza, E., and Costantini, M. L. (2015). Predator and detritivore niche width helps to explain biocomplexity of experimental detritus-based food webs in four aquatic and terrestrial ecosystems. Ecol. Complexity 23, 14–24. doi: 10.1016/j.ecocom.2015.04.005
Rossi, L., Sporta Caputi, S., Calizza, E., Careddu, G., Oliverio, M., Schiaparelli, S., et al. (2019). Antarctic food web architecture under varying dynamics of sea ice cover. Sci. Rep. 9:12454. doi: 10.1038/s41598-019-48245-7
Saggiomo, M., Poulin, M., Mangoni, O., Lazzara, L., De Stefano, M., Sarno, D., et al. (2017). Spring-time dynamics of diatom communities in landfast and underlying platelet ice in Terra Nova Bay, Ross Sea, Antarctica. J. Mar. Syst. 166, 26–36. doi: 10.1016/j.jmarsys.2016.06.007
Schofield, O., Ducklow, H. W., Martinson, D. G., Meredith, M. P., Moline, M. A., and Fraser, W. R. (2010). How do polar marine ecosystems respond to rapid climate change? Science 328, 1520–1523. doi: 10.1126/science.1185779
Signa, G., Calizza, E., Costantini, M. L., Tramati, C., Sporta Caputi, S., Mazzola, A., et al. (2019). Horizontal and vertical food web structure drives trace element trophic transfer in Terra Nova Bay, Antarctica. Environ. Pollut. 246, 772–781. doi: 10.1016/j.envpol.2018.12.071
Smale, D. A., Barnes, D. K. A., and Fraser, K. P. P. (2007). The influence of ice scour on benthic communities at three contrasting sites at Adelaide Island, Antarctica. Austral. Ecol. 32, 878–888. doi: 10.1111/j.1442-9993.2007.01776.x
Søreide, J. E., Leu, E., Berge, J., Graeve, M., and Falk-Petersen, S. (2010). Timing of blooms, algal food quality and Calanus glacialis reproduction and growth in a changing Arctic. Glob. Change Biol. 16, 3154–3163. doi: 10.1111/j.1365-2486.2010.02175.x
Sporta Caputi, S., Careddu, G., Calizza, E., Fiorentino, F., Maccapan, D., Rossi, L., et al. (2020). Changing isotopic food webs of two economically important fish in mediterranean coastal lakes with different trophic status. Appl. Sci. 10:2756. doi: 10.3390/app10082756
Staniczenko, P. P. A., Lewis, O. T., Jones, N. S., and Reed-Tsochas, F. (2010). Structural dynamics and robustness of food webs. Ecol. Lett. 13, 891–899. doi: 10.1111/j.1461-0248.2010.01485.x
Stoecker, D. K., Gustafson, D. E., Baier, C. T., and Black, M. M. D. (2000). Primary production in the upper sea ice. Aquat. Microb. Ecol. 21, 275–287. doi: 10.3354/ame021275
Thrush, S. F., and Cummings, V. J. (2011). Massive icebergs, alteration in primary food resources and change in benthic communities at Cape Evans, Antarctica. Mar. Ecol. 32, 289–299. doi: 10.1111/j.1439-0485.2011.00462.x
Trebilco, R., Melbourne-Thomas, J., and Constable, A. J. (2020). The policy relevance of Southern Ocean food web structure: implications of food web change for fisheries, conservation and carbon sequestration. Mar. Policy 115:103832. doi: 10.1016/j.marpol.2020.103832
Vander Zanden, M. J., and Rasmussen, J. B. (1996). A trophic position model of pelagic food webs: impact on contaminant bioaccumulation in lake trout. Ecol. Monogr. 66, 451–477. doi: 10.2307/2963490
Wing, S. R., Leichter, J. J., Wing, L. C., Stokes, D., Genovese, S. J., McMullin, R. M., et al. (2018). Contribution of sea ice microbial production to Antarctic benthic communities is driven by sea ice dynamics and composition of functional guilds. Glob. Change Biol. 24, 3642–3653. doi: 10.1111/gcb.14291
Keywords: Antarctica, climate change, food webs, keystone species, population-wide metrics, seasonal sea-ice dynamics, stable isotopes, trophic interactions
Citation: Sporta Caputi S, Careddu G, Calizza E, Fiorentino F, Maccapan D, Rossi L and Costantini ML (2020) Seasonal Food Web Dynamics in the Antarctic Benthos of Tethys Bay (Ross Sea): Implications for Biodiversity Persistence Under Different Seasonal Sea-Ice Coverage. Front. Mar. Sci. 7:594454. doi: 10.3389/fmars.2020.594454
Received: 13 August 2020; Accepted: 10 November 2020;
Published: 11 December 2020.
Edited by:
Susana Carvalho, King Abdullah University of Science and Technology, Saudi ArabiaReviewed by:
Ian Hawes, University of Waikato, New ZealandLee W. Cooper, University of Maryland Center for Environmental Science (UMCES), United States
Copyright © 2020 Sporta Caputi, Careddu, Calizza, Fiorentino, Maccapan, Rossi and Costantini. This is an open-access article distributed under the terms of the Creative Commons Attribution License (CC BY). The use, distribution or reproduction in other forums is permitted, provided the original author(s) and the copyright owner(s) are credited and that the original publication in this journal is cited, in accordance with accepted academic practice. No use, distribution or reproduction is permitted which does not comply with these terms.
*Correspondence: Edoardo Calizza, ZWRvYXJkby5jYWxpenphQHVuaXJvbWExLml0