- 1Fisheries College, Ocean University of China, Qingdao, China
- 2Marine Botany, Faculty Biology/Chemistry, University of Bremen, Bremen, Germany
- 3Molecular Plant Genetics, Institute of Plant Science and Microbiology, University of Hamburg, Hamburg, Germany
- 4Alfred-Wegener-Institute, Helmholtz Centre for Polar and Marine Research, Bremerhaven, Germany
- 5Helmholtz Institute for Functional Marine Biodiversity at the University of Oldenburg (HIFMB), Oldenburg, Germany
- 6Institute of Biochemistry I, Medical Faculty, University Hospital Cologne, University of Cologne, Cologne, Germany
- 7Station Biologique de Roscoff, Plateforme ABiMS, CNRS: FR 2424, Sorbonne Université, Roscoff, France
Kelps in the Arctic region are facing challenging natural conditions. They experience over 120 days of darkness during the polar night surviving on storage compounds without conducting photosynthesis. Furthermore, the Arctic is experiencing continuous warming as a consequence of climate change. Such temperature increase may enhance the metabolic activity of kelps, using up storage compounds faster. As the survival strategy of kelps during darkness in the warming Arctic is poorly understood, we studied the physiological and transcriptomic responses of Saccharina latissima, one of the most common kelp species in the Arctic, after a 2-week dark exposure at two temperatures (0 and 4°C) versus the same temperatures under low light conditions. Growth rates were decreased in darkness but remained stable at two temperatures. Pigments had higher values in darkness and at 4°C. Darkness had a greater impact on the transcriptomic performance of S. latissima than increased temperature according to the high numbers of differentially expressed genes between dark and light treatments. Darkness generally repressed the expression of genes coding for glycolysis and metabolite biosynthesis, as well as some energy-demanding processes, such as synthesis of photosynthetic components and transporters. Moreover, increased temperature enhanced these repressions, while the expression of some genes encoding components of the lipid and laminaran catabolism, glyoxylate cycle and signaling were enhanced in darkness. Our study helps to understand the survival strategy of kelp in the early polar night and its potential resilience to the warming Arctic.
Introduction
Kelps are perennial macroalgae with high economic and ecological significance. They are important primary producers and provide food and shelter for numerous organisms in the marine ecosystem (Bischof et al., 2019). Kelps show a broad geographical distribution from cold-temperate to Arctic coastlines. Compared to the kelps in temperate regions, kelps in the Arctic face a harsher physical environment due to the high latitude. The Arctic features strong, seasonal fluctuating solar radiation. In Kongsfjorden, Svalbard (78°55′N, 11°55′E), the polar night lasts for 129 days, from the end of October to mid-February (Cohen et al., 2015). Due to sea ice and snow cover, kelps in the inner part of Kongsfjorden can be exposed to extended darkness until April–July when the sea ice breaks up (Svendsen et al., 2002). To some kelps originating from cold-temperate regions (e.g., Saccharina latissima), the low water temperature in the Arctic represents a suboptimal growth environment (Fortes and Lüning, 1980; Hurd et al., 2014). However, such environments are changing due to climate change. The Arctic has warmed at more than twice as fast as the global mean air temperature increase since the mid-1990s (Overland et al., 2019). In Kongsfjorden, the surface water temperature has increased to around 2°C in winter and early spring from 2007 (Hegseth et al., 2019). It might continue to increase since the winter air temperature in the Arctic is predicted to increase by over 7°C at the end of the 21st century (IPCC, 2007; MacDonald, 2010). Meanwhile, the warming climate largely prevents the formation of sea ice in the inner part of Kongsfjorden since 2006 (Pavlova et al., 2019) and consequently shortens the period of darkness. Hence, it is urgent to understand the survival mechanisms of kelps during the polar night.
Some species of seaweeds in the Arctic may potentially benefit from the increase of water temperature if their optimum temperature for growth is above 5°C (Gómez et al., 2009). However, the metabolic activity of organisms increases with temperature rise, potentially leading to shorter survival times of organisms during the polar night. For example, Schaub et al. (2017) demonstrated that the Arctic benthic diatom Navicula perminuta utilized lipid resources significantly faster at 7°C than 0°C during an 8-week dark exposure period. The magnitude of temperature increase also affects the survival period of organisms. The Antarctic sea-ice diatom Fragilariopsis cylindrus showed only a 7-day maximum dark survival time at 10°C, in contrast with a maximum survival time of 60 days at −2 and 4°C (Reeves et al., 2011).
Historically, studies on algae exposed to prolonged dark periods were mainly focused on the growth patterns (Lüning, 1969; Smayda and Mitchell-Innes, 1974; Chapman and Lindley, 1980; Dunton, 1985), physiological performances and biochemical mechanisms (Weykam et al., 1997; Lüder et al., 2002; Karsten et al., 2019). Mcminn and Martin (2013) concluded several strategies for algae to survive long periods of darkness. One of them is changing physiological activities, including the reduction of metabolic rate (Palmisano and Sullivan, 1982; Jochem, 1999) and utilization of energy storage products (e.g., laminarans and lipids) to maintain basic metabolism during prolonged darkness (Manoharan et al., 1999; Schaub et al., 2017; Scheschonk et al., 2019). The pigments and photosynthetic performances of algae in darkness have been widely investigated (Wulff et al., 2008; Martin et al., 2012; Naik and Anil, 2018). Darkness showed a varying influence on the photophysiology of algae depending on species and exposure time. Several laboratory studies demonstrated chlorophylls remained constant while the potential of photochemistry was decreased during the exposure to darkness (Montechiaro and Giordano, 2006; Reeves et al., 2011; Lacour et al., 2019). A field study showed that Arctic kelps can preserve their photosynthetic capability throughout the 4-month polar night reflected by the stable chlorophyll a fluorescence and pigment content (Scheschonk et al., 2019). Recently, the molecular acclimation processes of microalgae to long-term darkness have been examined using transcriptomics (Nymark et al., 2013; Mock et al., 2017; Mundt et al., 2019) and proteomics (Bai et al., 2016; Kennedy et al., 2019). However, the above studies were mainly focused on the molecular responses of diatoms. Up to now, there is no study investigating molecular regulations of macroalgae in prolonged darkness, and the mechanisms of molecular acclimation for macroalgae in darkness are still unknown.
Peters and Thomas (1996) showed that algae change most of their physiological performance during the first days of dark exposure and then remain relatively stable after 20 days. Furthermore, the transcriptomic response is usually more sensitive than the physiological reaction (Heinrich et al., 2012b, 2015). Hence, in the present study, we analyzed the growth rate, photochemistry and transcriptomic responses by RNA-sequencing (RNA-Seq) of S. latissima after a 2-week dark exposure at two temperatures (0 and 4°C). We aim to detect the gene regulations of S. latissima combined with its physiological performance under darkness, and subsequently discuss the survival strategy of Arctic S. latissima during the early polar night.
Materials and Methods
Algal Material
Stock gametophyte cultures of S. latissima, which were established from spores of fertile sporophytes collected in Kongsfjorden (78°58′N; 11°30′E; Svalbard, Norway), were used to raise young sporophytes and kept at the Alfred-Wegener-Institute Helmholtz Centre for Polar and Marine Research (Bremerhaven, Germany; culture number: 3123, 3124). Male and female gametophytes were fragmented and pooled together using sterile mortar and pestle, then transferred to Petri dishes filled with sterile Provasoli enriched seawater (PES; Starr and Zeikus, 1993) at 8 ± 1°C. After 2 weeks growing sporophytes were transferred to aerated 5l glass bottles and grown in PES at 8 ± 1°C. During fertilization and pre-cultivation, the light conditions were 20 μmol photons m2 s–1 (Mitras Lightbar Daylight 150, GHL) at 18:6 h light: dark period. The medium was changed twice per week. Due to the limitation of culture rooms, the sporophytes were then transferred and cultured at 12 ± 1°C for nearly 5 months before the experiment. Hence, the sporophytes need to be acclimated from 12 to 4°C and 0°C gradually. The acclimation procedure was as follows: sporophytes were transferred to temperature-controlled incubators where the temperature was reduced by 2 ± 1°C every 2 or 3 days from 12 ± 1°C to 4 ± 1°C. When the temperature was reduced to 4 ± 1°C, the sporophytes were kept at 4 ± 1°C for 1-week of acclimation. Then parts of sporophytes were chosen for the 4°C treatments, and the rest of the sporophytes were kept in the same condition due to the limitation of incubators. Hence the 4°C treatments had 19 days pre-acclimation. After the experiment of 4°C, the temperature of incubators was reduced gradually to 0 ± 1°C and sporophytes were kept at 0 ± 1°C for 1-week before the dark experiment. Thus the 0°C treatments had 47 days of pre-acclimation.
Temperature and Dark Treatments
The experiment included two light conditions (light: 20 μmol photons m2 s–1, 18:6 h light: dark; dark: continuous dark) combined with two temperatures (0 and 4 ± 1°C), hereafter referred as 0°C_Light, 0°C_Dark, 4°C_Light and 4°C_Dark. The experiment lasted for 14 days. Each treatment consisted of four aerated 5l culture bottles (n = 4 true replicates) containing five uniparental sporophytes (one spot-labeled by a Pasteur pipette for growth and fluorometric measurement, one for pigment measurement, one for RNA-seq and others as backup), and was conducted in different incubators. For dark treatments, the bottles were wrapped in aluminum foil. The bottles were filled with sterile PES at the beginning without further water change during the experiment. After 14 days, sporophytes for RNA extraction and pigment analysis were randomly chosen from each bottle and immediately frozen in liquid nitrogen, then stored at -80°C until further processing. The spot-labeled sporophytes were firstly measured by an Imaging-PAM, then subjected to the fresh weight measurements.
Relative Growth Rate
The fresh weights and morphological characters (n = 4) were measured using the sporophytes with spot-label prior to the experiment and at the end of the experiment. Sporophytes were gently dried from surplus water using paper tissue and weighed by a digital scale (Precisa 40SM-200A). Relative growth rates (RGRs, day–1) of fresh weights were calculated between day 0 and 14 according to the formula of Lüning et al. (1990): RGR (day–1) = 100 × ln(W1/W2)/(T1-T2), where W1 is the fresh weight (g) in time 1 (T1), W2 is the fresh weight (g) in time 2 (T2), T1 and T2 are the time in days.
Pigment and Chlorophyll a Fluorescence
Chlorophyll a (Chl a), chlorophyll c2 (Chl c2) and fucoxanthin (n = 4) were extracted and analyzed according to the method described in Koch et al. (2015). Briefly, lyophilized and pulverized samples were extracted in 1 mL Acetone (90%, v/v) at 4°C in darkness for 24 h. The extracted pigments were analyzed by a high-performance liquid chromatography (HPLC) and quantified by co-chromatography with standards for Chl a, Chl c2, and fucoxanthin. Hence, though Phaeophyceae possess Chl c1 and c2 (Hurd et al., 2014), we only analyzed Chl c2 in this study. Pigment contents were calculated as μg g–1 dry weight (DW). The accessory pigment pool (Acc) was calculated by the sum of Chl c2 and fucoxanthin.
The maximum quantum yield of photosystem II (Fv/Fm, n = 4) was measured by an Imaging-PAM chlorophyll fluorometer (Heinz Walz GmbH, Germany) after 10 min dark acclimation. All measurements were processed in a dark room at ambient temperature. Fv/Fm was calculated by the following formula: Fv/Fm = (Fm-F0)/Fm, where Fm is the maximum fluorescence measured after exposure to a saturating pulse, and F0 is the minimum fluorescence measured in the dark with measuring light.
Statistical Analysis
SPSS software version 25 (IBM) was used for statistical analysis. For the RGRs of fresh weights, one replicate in 4°C_Dark was excluded due to a technically erroneous measurement (hence the total N = 15, n = 3 in the treatment of 4°C_Dark and n = 4 in the other three treatments). For the other parameters, each treatment had four biological replicates. A two-way ANOVA was used to test the effects of temperature and light conditions on growth and photochemistry. Prior to analysis, all data were tested for the normality by the Shapiro–Wilk test and the homogeneity of variances by the Levene’s test. Since the data of RGRs failed to comply with the homogeneity of variances, a non-parametric test (Mann-Whitney U test) was used to test the effects of temperature and light conditions, respectively. The level of significance was set at α = 0.05. All data are given as mean ± standard deviation unless otherwise stated.
RNA-Seq and Data Processing
One sporophyte per bottle (n = 3) in each treatment was randomly chosen for RNA analysis. Total RNA extraction followed the protocol of Heinrich et al. (2012a). Briefly, each sporophyte was ground in liquid nitrogen respectively. Afterward, the sample was transferred into a 2.0 mL Eppendorf tube and mixed with 1 mL extraction buffer (2% CTAB, 1 M NaCl, 100 mM Tris pH 8, 50 mM EDTA, pH 8) and 10 μL β-mercaptoethanol, followed by 15 min incubation at 45°C. Then 1 mL chloroform was added into the tube. After 10 min mixing, the sample was centrifuged for 20 min at 20°C. 750 μL of supernatant was transferred into a new tube and mixed with 250 μL ethanol and 1 mL chloroform. The tube was conducted again with 10 min mixing and 20 min centrifugation. Subsequently, 500 μL of supernatant was transferred into a new tube. The following RNA extraction was conducted using a Qiagen Plant Mini Kit (Qiagen, Germany) according to the manufacturer’s protocol. The RNA quality was evaluated by a NanoDrop ND-100 UV-Vis Spectrophotometer and Agilent 2100 Bioanalyzer (Agilent Technologies, Germany). cDNA libraries were constructed using an Illumina TruSeq RNA Library Prep Kit (Illumina, United States). The libraries were sequenced by an Illumina 2500 and 75 bp paired reads were clipped using the default values of Illumina software. The quality of raw data was checked by FastQC v. 0. 11. 5.1 Low-quality data was trimmed by Trimmomatic v. 0.36 (Bolger et al., 2014) with the following parameters: leading 3, trailing 3, sliding window 4: 15, minlen 30. Remaining data were pseudo-aligned against a de novo transcriptomic reference established from cDNA libraries of S. latissima from Roscoff, France (Machado Monteiro et al., 2019) using Salmon (Patro et al., 2017). One replicate of the 0°C_light treatment was excluded as outlier after analyzing the relationship between sample replicates using a principal component analysis (PCA). Differentially expressed genes (DEGs) were obtained from the comparisons between 0°C_Light vs. 0°C_Dark, 0°C_Light vs. 4°C_Light, 4°C_Light vs. 4°C_Dark, and 0°C_Dark vs. 4°C_Dark, using DESeq 2 (Love et al., 2014). Trinity contigs (hereafter referred as genes) with an adjusted p-value of ≤ 0.001 and a log2 fold change (FC) of at least two were accepted as significantly differential expressed. The applied tools were the Trinity package v. 2.4.0 (Grabherr et al., 2011). Functional annotation was performed through the Trinotate functional annotation pipeline.2 Functional annotation included searches against the databases of UniProtKB/Swiss-Prot and UniRef90 with the BLASTX and BLASTP algorithms with the default e-value setting to 1e–6, annotation of the biochemical pathway in Kyoto Encyclopedia of Genes and Genome (KEGG), prediction of protein functions based on known orthologous gene products in non-supervised Orthologous Groups (eggNOG), identification of protein domains in Pfam database with HMMER, prediction of signal peptides with SignalP, prediction of transmembrane helices with TMHMM, and prediction of gene ontology (GO) terms (based on the BLAST and Pfam annotation). All DEGs were further annotated using diamond local aligner (Buchfink et al., 2015) against the National Center for Biotechnology Information (NCBI) non-redundant protein sequence (Nr) database (cut-off 1e–9). GOseq (Young et al., 2010) was used to perform the GO enrichments (p-value < 0.05). To further detect the gene regulations of S. latissima in darkness, the comparisons between 0°C_Light vs. 0°C_Dark and 4°C_Light vs. 4°C_Dark were additionally analyzed by following procedures: the Venn diagrams of DEGs were produced using the online tool.3 Enriched GO terms were summarized by REVIGO (Supek et al., 2011) with an allowed similarity of 0.5. The biological process GO terms after redundancy reduction were displayed in the form of scatterplots produced by REVIGO. The heatmap of expression (log10 TPM) of DEGs related to photosynthesis was generated by the OmicShare tools.4 To explore the constitutively expressed transcripts within the control (the control refers to 0°C_Light in the comparison between 0°C_Light vs. 0°C_Dark, and 4°C_Light in the comparison between 4°C_Light vs. 4°C_Dark), normalized read counts, given as transcripts per million (TPM), were analyzed as described by Iñiguez et al. (2017).
Results
Growth and Photochemistry
Light conditions significantly affected RGRs of fresh weights after 14 days of exposure (p = 0.001, Mann-Whitney U Test, Supplementary Table S1). The averaged RGR significantly decreased from 5.067 ± 0.681 day–1 in the light treatments to 0.641 ± 0.672 day–1 in the dark treatments (Table 1). The averaged RGR at 4°C (3.435 ± 2.587 day–1) was slightly higher than RGR at 0°C (2.623 ± 2.282 day–1), but there was no significant difference between two temperatures (p = 0.298, Mann-Whitney U Test). Sporophytes in the dark treatments did not show the apparent decomposition of pigment or algal tissue at the end of the experiment (Supplementary Figure S1). Chl a and Acc shared similar patterns, which were significantly affected by temperature and light conditions respectively. Both contents at 4°C were higher than contents at 0°C. Meanwhile, contents in dark treatments were higher than contents in light treatments. Fv/Fm did not differ among temperature and light treatments.
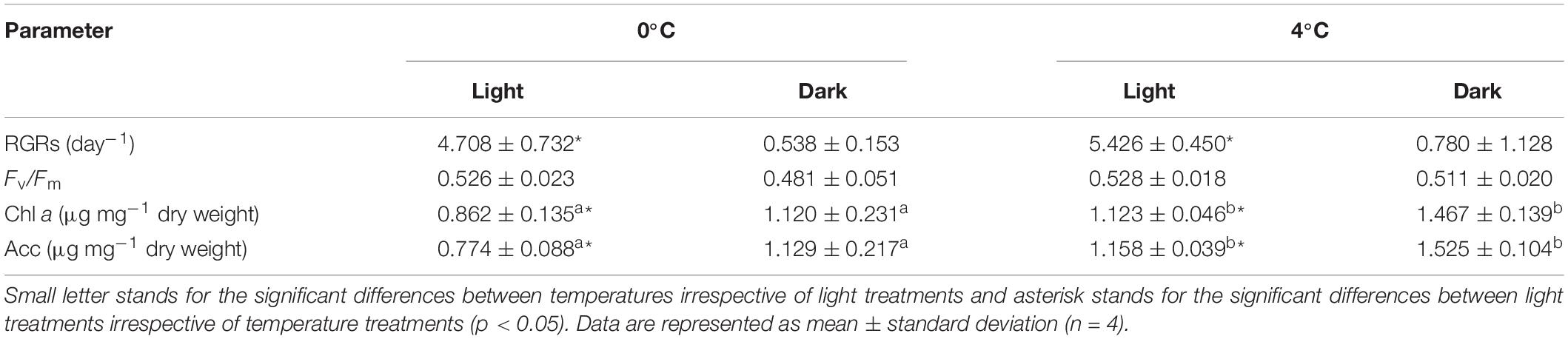
Table 1. Physiological parameters (relative growth rates [RGRs], maximum quantum yield of Photosystem II [Fv/Fm], contents of chlorophyll a [Chl a] and accessory pigment pool [Acc]) in Saccharina latissima among four treatments.
Gene Expression and GO Enrichment
The number of reads per complementary DNA library ranged from 19.9 to 38.1 million with an average of 31.3 million reads. The averaged remapping rate to the de novo library was 87.57%. Four comparisons yielded 3, 284 DEGs, accounting for 2.42% of 135,959 total de novo assembled genes (Supplementary Table S2). The number of contigs was more affected by light conditions than temperature. Namely, there were 1,184 DEGs between 0°C_Light and 0°C_Dark, and 1,735 DEGs between 4°C_Light and 4°C_Dark. In contrast, the two temperatures featured 148 DEGs and 217 DEGs respectively in light treatments and dark treatments. The averaged annotation rate was 8.2% for DEGs from the UniprotKB/Swiss-Prot database (Supplementary Table S2). To investigate the transcriptomic response for S. latissima to darkness, we focused on the comparisons between 4°C_Dark and 4°C_Light, as well as 0°C_Dark and 0°C_Light. 0°C_Dark (compared to 0°C_Light) featured more upregulated genes (658) than downregulated genes (526), whereas 4°C_Dark (compared to 4°C_Light) had more downregulated genes (1, 179) than upregulated genes (556). The Venn diagram (Figure 1) shows that 0°C_Dark and 4°C_Dark shared 163 upregulated genes and 296 downregulated genes.
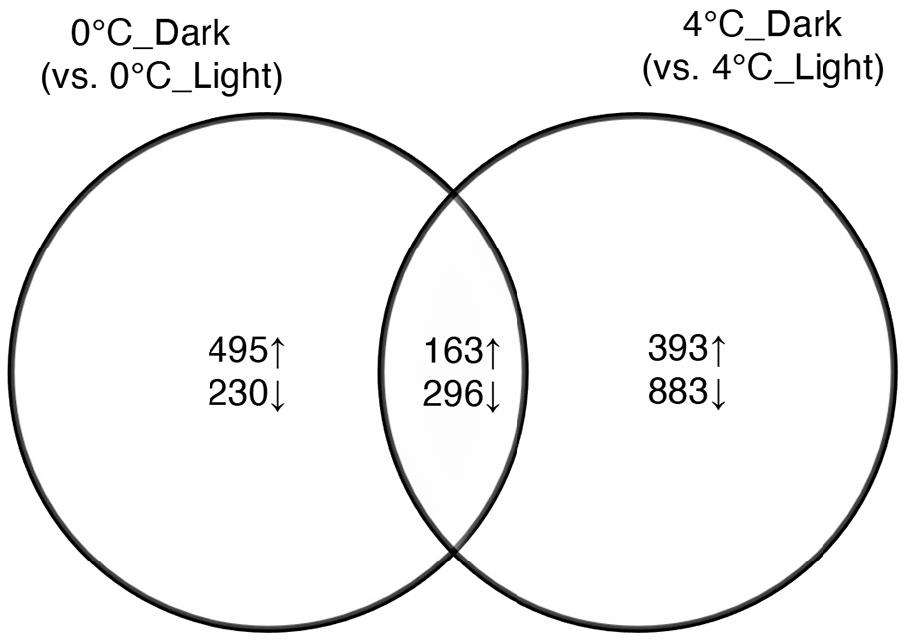
Figure 1. Venn diagram of significantly upregulated (↑) and downregulated (↓) genes after dark exposure at two temperatures (0 and 4°C) versus the same temperatures in the light (p ≤ 0.001, |log2 FC| ≥ 2).
The enriched GO terms were summarized using REVIGO (Supek et al., 2011) and the complete list is available in Supplementary Table S3. The GO terms for biological processes in 0°C_Dark and 4°C_Dark were visualized in Figure 2. Generally, the downregulated genes showed more enriched GO terms than the upregulated genes. In 0°C_Dark and 4°C_Dark, the enriched GO terms of downregulated genes mainly included carbohydrate metabolism, inorganic anion transport, chloroplast, photosystem and many others, while upregulated genes involved functions such as regulation of acute inflammatory response and glyoxysome.
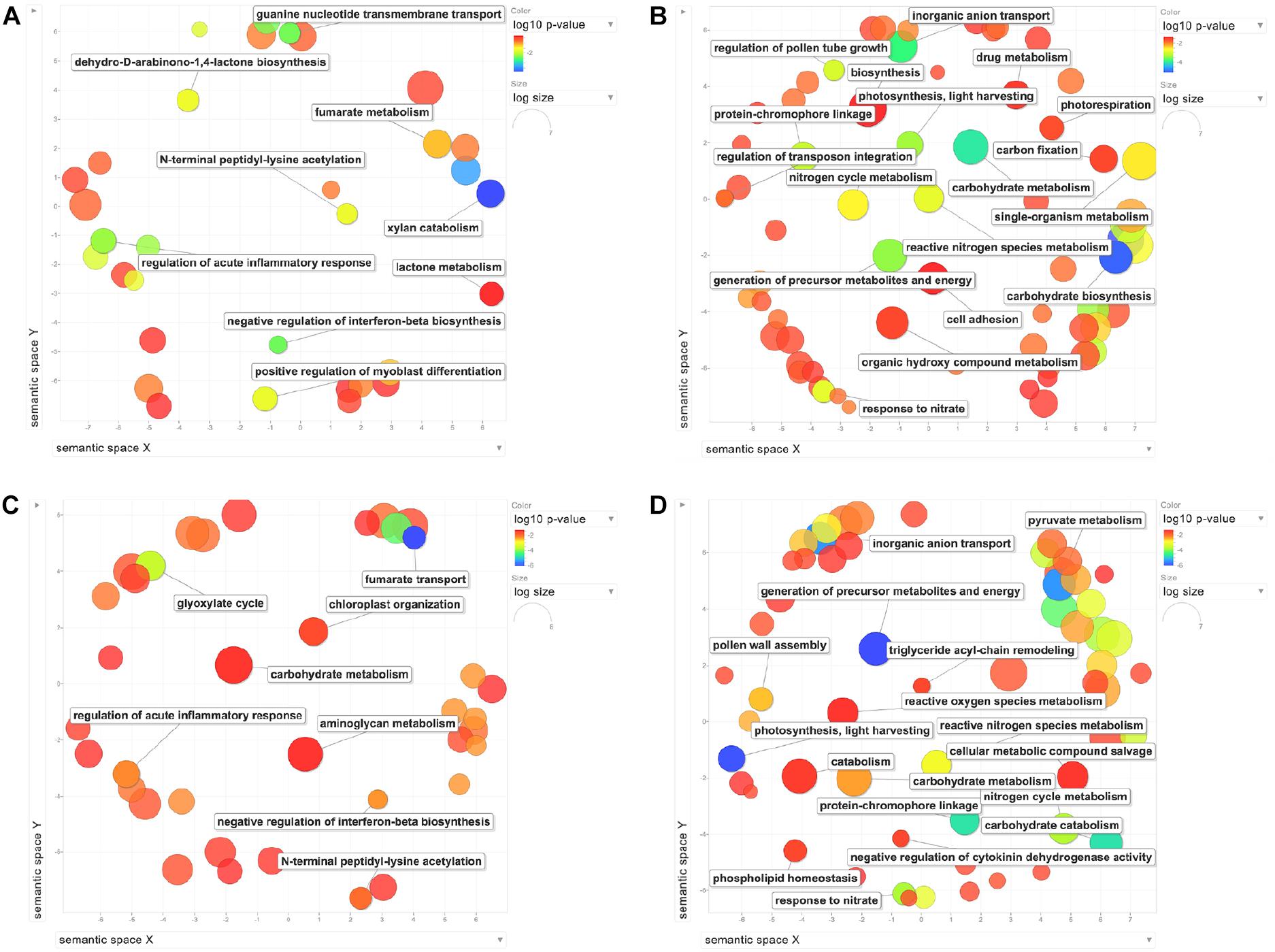
Figure 2. REVIGO scatterplots of the biological process enriched GO terms after the redundancy reduction. (A) Upregulated genes in 0°C_Dark. (B) Downregulated genes in 0°C_Dark. (C) Upregulated genes in 4°C_Dark. (D) Downregulated genes in 4°C_Dark. Bubble color indicates the p-value; bubble size indicates the frequency of the GO terms in the underlying Gene Ontology Annotation (GOA) database (larger bubbles represent more general terms). The legends of GO terms are displayed in the scatterplot when their dispensability is below 0.145.
Manual Inspection of DEGs
To further study the transcriptomic responses of S. latissima in darkness, we manually analyzed and classified DEGs with annotations (UniProtKB/Swiss-Prot database as the main source, NCBI Nr database as a supplement; Figure 3). A full list of DEGs with annotations can be found in Supplementary Table S4. In summary, the number of downregulated genes in darkness at both temperatures was higher than the number of upregulated genes, and increased temperature (4°C) exacerbated the repression of gene expression in darkness compared to 0°C. In contrast, 4°C_Dark and 0°C_Dark showed similar numbers of upregulated genes. The summary highlights the distinguished up- and downregulated metabolisms: first, darkness induced some genes involved in the glyoxylate cycle, stress- and signaling-related activities. Meanwhile, 0°C_Dark significantly upregulated enzymes catabolizing lipid. Second, darkness widely repressed photosynthesis, glycolysis, transport, and main metabolite biosynthesis (e.g., carbohydrate, amino acid, and lipids).
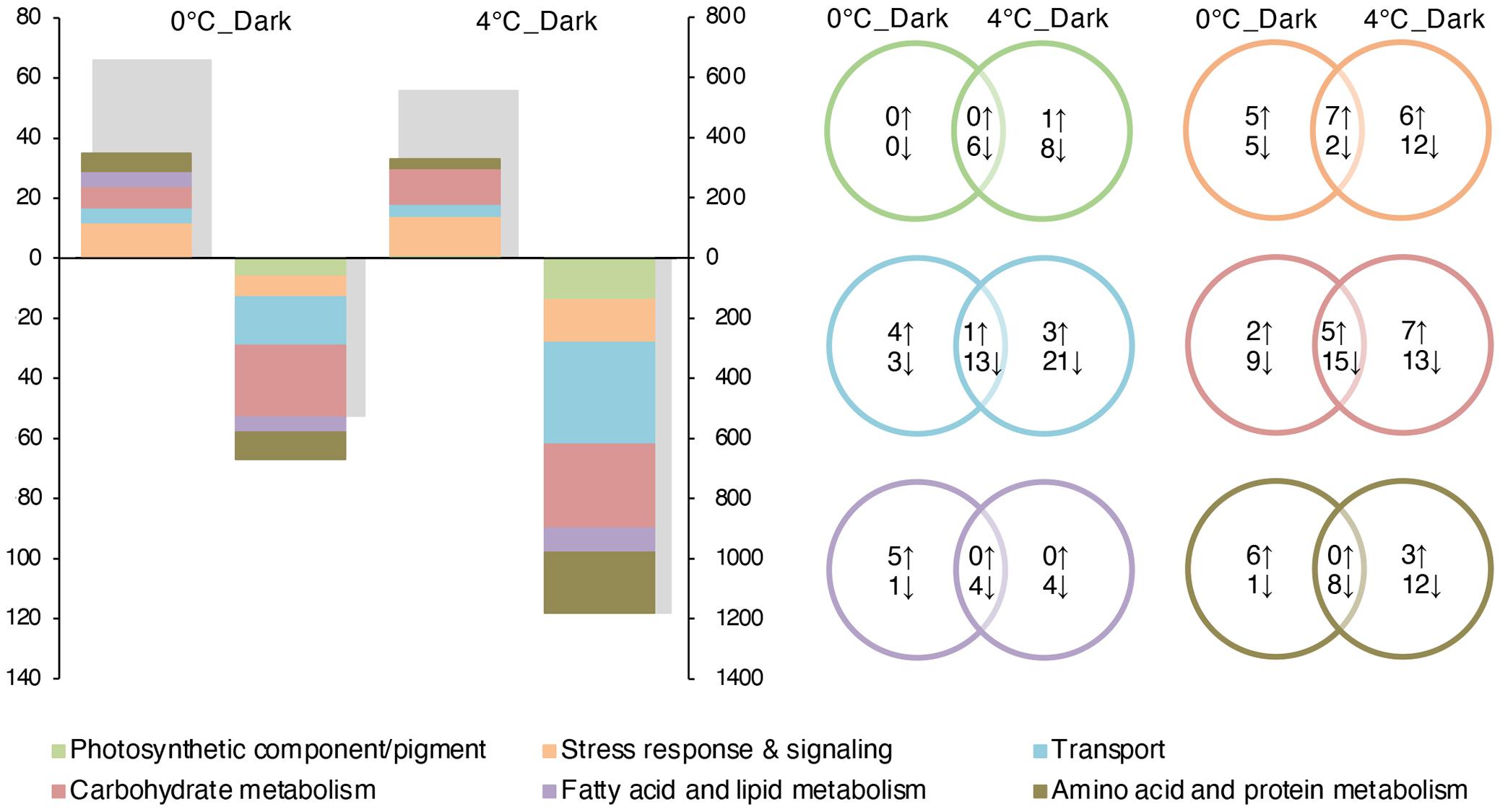
Figure 3. Number of differentially expressed genes (DEGs) in 0°C_Dark (compared to 0°C_Light) and 4°C_Dark (compared to 4°C_Light; p ≤ 0.001; | log2 FC| ≥ 2). On the (left) graph, the colored bars represent the number of up- and downregulated DEGs according to manually functional categories of interest corresponding to the (left) axis, the gray bars represent the total number of up- and downregulated DEGs corresponding the (right) axis. The (upper) part of the graph displays upregulated DEGs; the (lower) part displays downregulated DEGs. On the (right) graph, the Venn diagrams reflect the number of DEGs in each category.
Photosynthetic Components and Pigments
All DEGs encoding components of the light-harvesting complex (LHC) were downregulated after dark exposure at both 0 and 4°C with large log2 - fold changes (Figure 4). The highest downregulation of LHC reached 7- and 8- log2 - fold change at 0 and 4°C respectively. In addition, 4°C_Dark repressed more genes (8) encoding LHC than 0°C_Dark (4). A few DEGs involved in pigment metabolism were detected. For carotenoid biosynthesis, one gene coding for chloroplastic prolycopene isomerase was downregulated in 0°C_Dark and 4°C_Dark. 4°C_Dark solely repressed another gene encoding zeta-carotene desaturase. Pheophorbide a oxygenase, responsible for chlorophyll degradation, was induced in 4°C_Dark. In addition, 4°C_Dark repressed one gene encoding ferredoxin-NADP reductase, which is involved in photosynthetic electron transport.
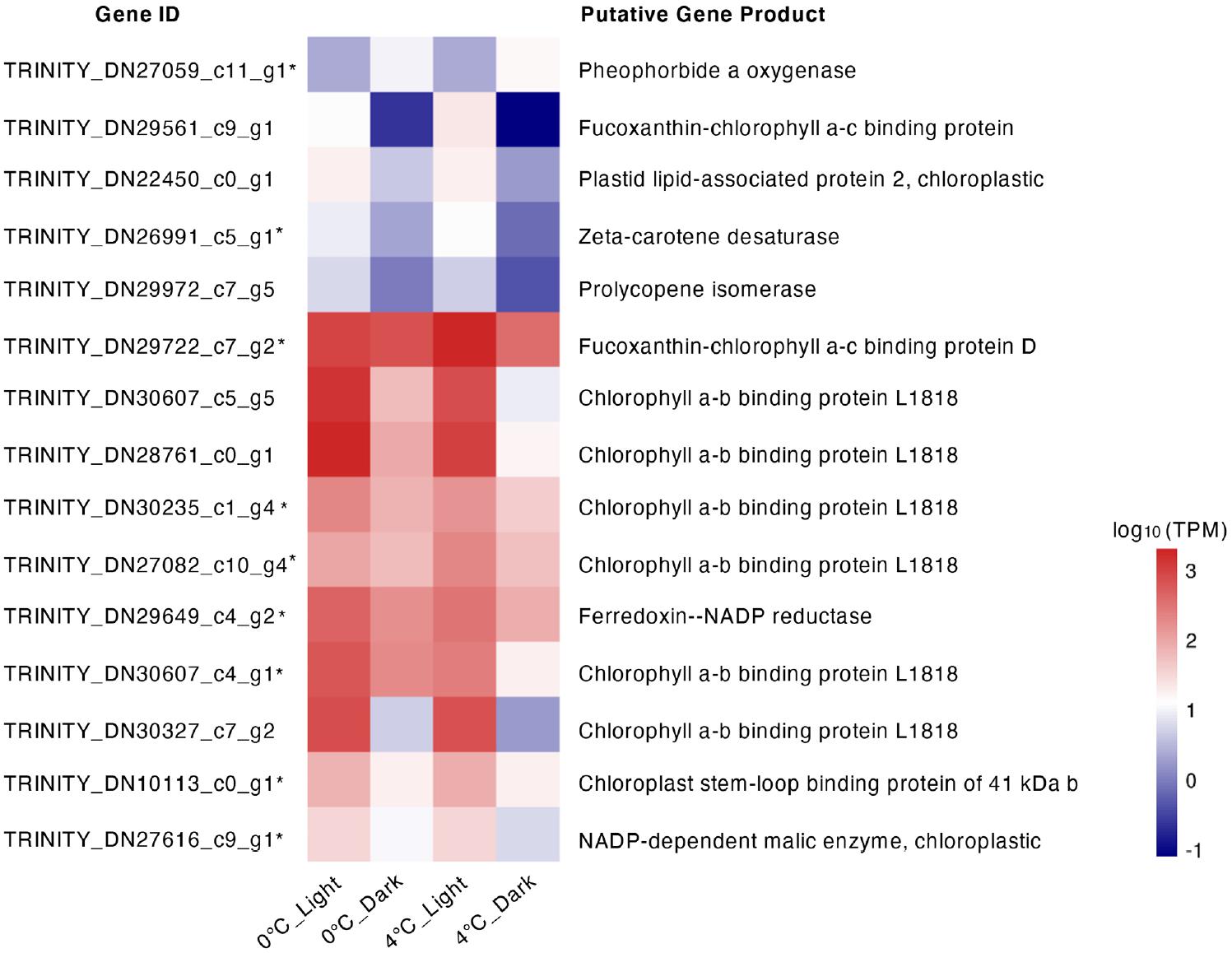
Figure 4. Heat map of gene expression for differentially expressed genes (DEGs) with functions in regulating photosynthetic components and pigment metabolism. Color stands for the log10 [transcripts per million (TPM) value] of each gene. *means that the DEG only occurred in 4°C_Dark (compared to 4°C_Light).
Transport
A total of 34 genes in 4°C_Dark and 16 genes in 0°C_Dark and related to the transport were downregulated after darkness exposure. These repressions included different metabolites and ion transporters, such as zinc, chromate, and nitrate (Table 2). The downregulation changes were around 2- to 4- log2 - fold. Genes encoding nitrate and ammonium transporters were widely repressed, four genes in 0°C_Dark and seven genes in 4°C_Dark, respectively. A small number of transporters were upregulated in both darkness treatments. Namely one gene encoding ammonium transporter 1 member 1 was upregulated in both 0°C_Dark and 4°C_Dark. 4°C_Dark induced one gene coding for succinate/fumarate mitochondrial transporter.
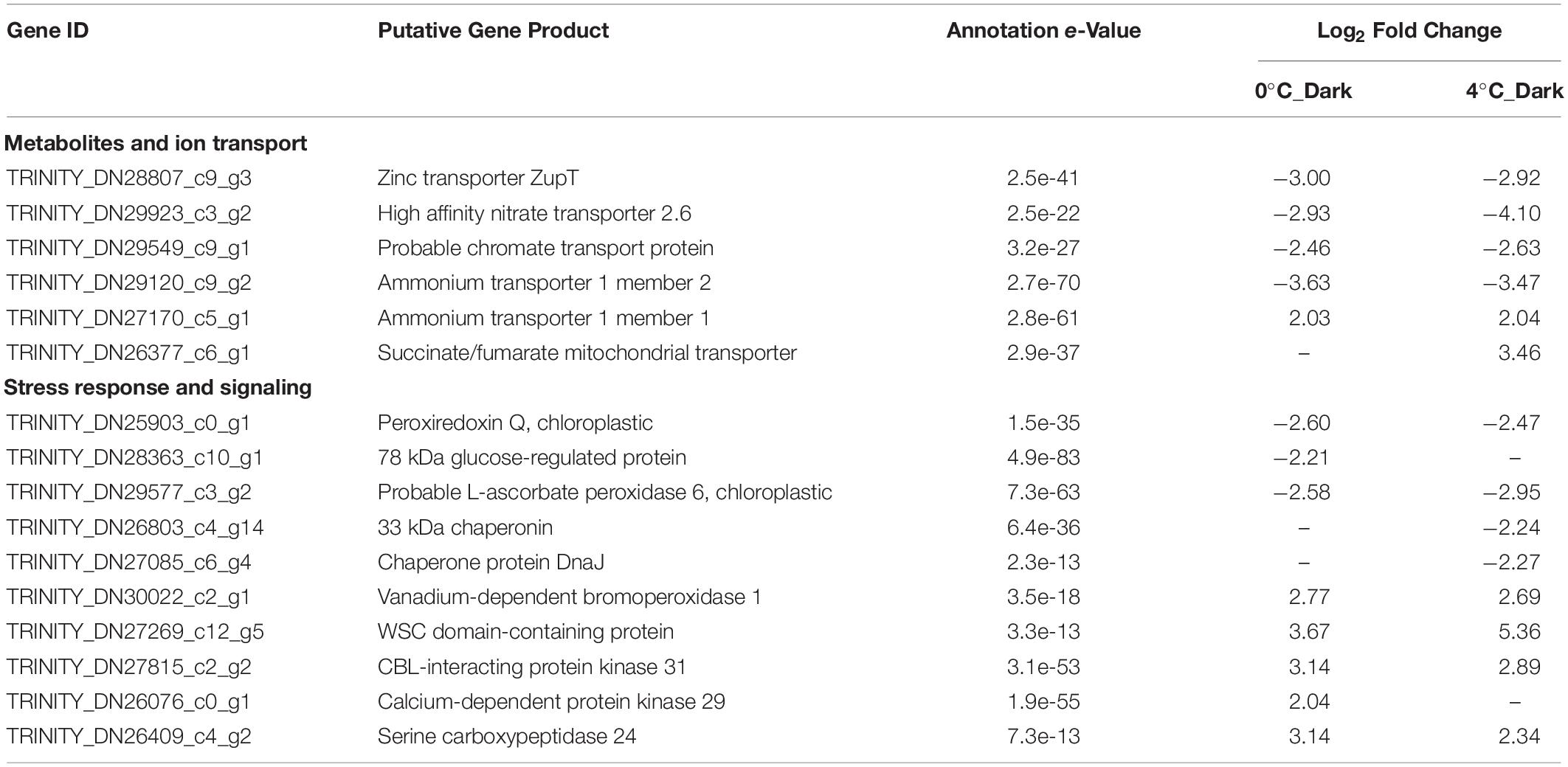
Table 2. Selected significant differentially expressed genes (DEGs) with functions in transporters, stress response and signaling after dark exposure at two temperatures (0 and 4°C) versus the same temperature in the light (p ≤ 0.001, | log2 FC| ≥ 2).
Stress Response and Signaling
Genes encoding antioxidative enzymes and chaperones were downregulated in the dark compared to the light (Table 2). On the contrary, genes encoding vanadium-dependent bromoperoxidase were induced in dark treatments, two in 0°C_Dark and three in 4°C_Dark, respectively. Besides, several genes encoding WSC domain-containing protein, responsible for cell wall integrity (CWI) and stress response, were 2- to 5- log2 - fold induced in dark treatments. For signaling, several enzymes involved in different signaling pathways were upregulated in darkness. Namely, serine carboxypeptidase 24 and CBL-interacting protein kinase 31 were induced in both 0°C_Dark and 4°C_Dark.
Carbohydrate Metabolism
Carbon Assimilation
Darkness did not cause obvious changes for genes correlated to photosynthetic carbon fixation. 0°C_Dark and 4°C_Dark only downregulated one gene coding for phosphoglycerate kinase (Table 3). For light independent carbon fixation (LICF), phosphoenolpyruvate carboxylase (PEPC) featured around 2- log2 - fold downregulation in both dark treatments. Another important LICF enzyme phosphoenolpyruvate carboxykinase (PEPCK) was not detected in our reference library.
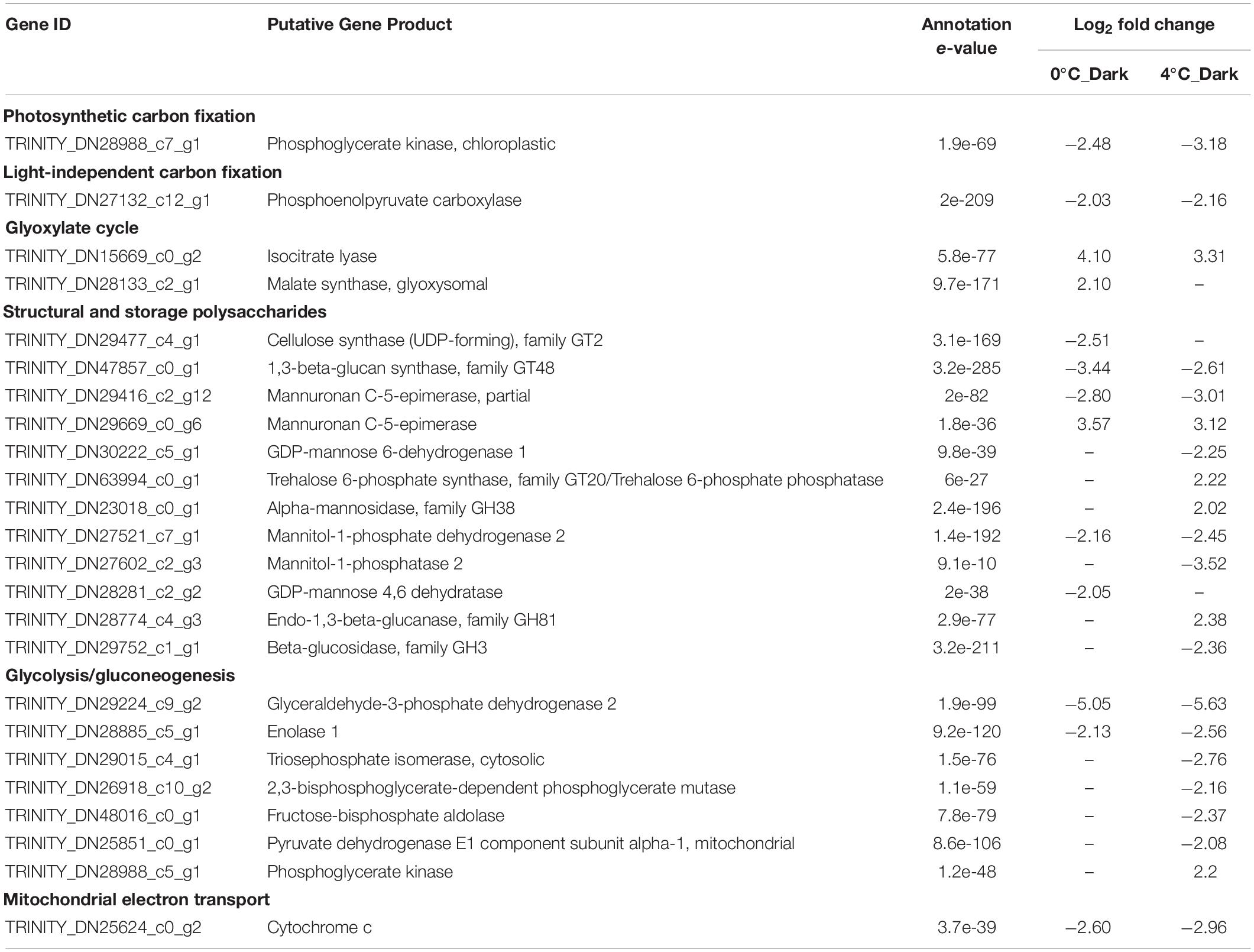
Table 3. Selected significant differentially expressed genes (DEGs) with functions in carbohydrate metabolism after dark exposure at two temperatures (0 and 4°C) versus the same temperature in the light (p ≤ 0.001, | log2 FC| ≥ 2).
Glyoxylate Cycle
Two key enzymes in the glyoxylate cycle, isocitrate lyase and malate synthase, were over-expressed in response to darkness. 4°C_Dark upregulated two genes encoding isocitrate lyase with an average 3.47- log2 - fold change. 0°C_Dark upregulated one gene encoding isocitrate lyase with a 4.1- log2 -fold change, and one gene encoding glyoxysomal malate synthase with a 2.1- log2 - fold change.
Structural and Storage Polysaccharides
Darkness repressed most of the genes responsible for the biosynthesis of polysaccharides at 0 and 4°C. For the biosynthesis of alginate, one important component of cell wall polysaccharides, 0°C_Dark showed seven downregulated genes encoding mannuronan C-5-epimerase. 4°C_Dark repressed two genes encoding mannuronan C-5-epimerase and two genes encoding GDP-mannose 6-dehydrogenase. However, both 0°C_Dark and 4°C_Dark showed several upregulated genes encoding mannuronan C-5-epimerase. Apart from the alginate, 0°C_Dark solely repressed two genes for the biosynthesis of cell wall polysaccharides, i.e., cellulose synthase for cellulose and GDP-mannose 4,6-dehydratase for fucan. 0°C_Dark and 4°C_Dark also downregulated genes related to the biosynthesis of storage polysaccharides (mannitol and laminaran). Four genes encoding 1,3-beta-glucan synthase, involved in the biosynthesis of laminaran, were downregulated in both 0°C_Dark and 4°C_Dark. Furthermore, 0°C_Dark showed higher regulated changes (from 3.18- to 3.88- log2 - fold change) than 4°C_Dark (from 2.49- to 2.71- log2 - fold change). For the biosynthesis of mannitol, 0°C_Dark and 4°C_Dark jointly repressed one gene encoding mannitol-1-phosphate dehydrogenase, while 4°C_Dark individually repressed another gene encoding mannitol-1-phosphatase 2. For the degradation of laminaran, 4°C_Dark downregulated two genes encoding beta-glucosidase, family GH3 and upregulated one gene encoding endo-1,3-beta-glucanase, family GH81.
Glycolysis
Darkness widely repressed the expression of genes related to glycolysis, and 4°C_Dark downregulated more genes than 0°C_Dark. Glycolysis has two phases: the preparatory phase and the payoff phase (Nelson and Cox, 2005). In the preparatory phase, two genes were downregulated in 4°C_Dark, encoding triosephosphate isomerase, cytosolic and fructose-bisphosphate aldolase. In the payoff phase, several DEGs encoding glyceraldehyde-3-phosphate dehydrogenase were downregulated in both 0°C_Dark and 4°C_Dark, with transcriptional changes between 2.98- and 6.38- log2 - fold. One DEG coding for enolase 1 was repressed after darkness treatment at both temperatures. Besides, one gene encoding phosphoglycerate mutase was solely repressed in 4°C_Dark. Since the pyruvate produced by glycolysis is further oxidized by cellular respiration to conduct the complete catabolism of carbohydrates, we also checked DEGs for cellular respiration, i.e., the production of acetyl-CoA, citric acid cycle, electron transfer and oxidative phosphorylation. Pyruvate dehydrogenase E1 component subunit alpha-1 belonging to the pyruvate dehydrogenase complex, responsible for the convention of pyruvate to acetyl-CoA, was downregulated in 4°C_Dark. However, there were no DEGs directly participating in the tricarboxylic acid (TCA) cycle. One gene encoding cytochrome c, involved in the mitochondrial electron transport chain (ETC), was repressed in 0°C_Dark and 4°C_Dark.
Amino Acid and Protein Metabolism
For amino acid and protein metabolism, 4°C_Dark downregulated more genes (20) than 0°C_Dark (9; Figure 2). Eight genes were downregulated in both 0°C_Dark and 4°C_Dark, including functions for nitrogen assimilation, biosynthesis of amino acid and protein modification (Table 4). Besides, 4°C_Dark solely repressed several genes about protein ubiquitination and proteolysis (e.g., E3 ubiquitin-protein ligase pub3) and one gene about urea cycle (arginase).
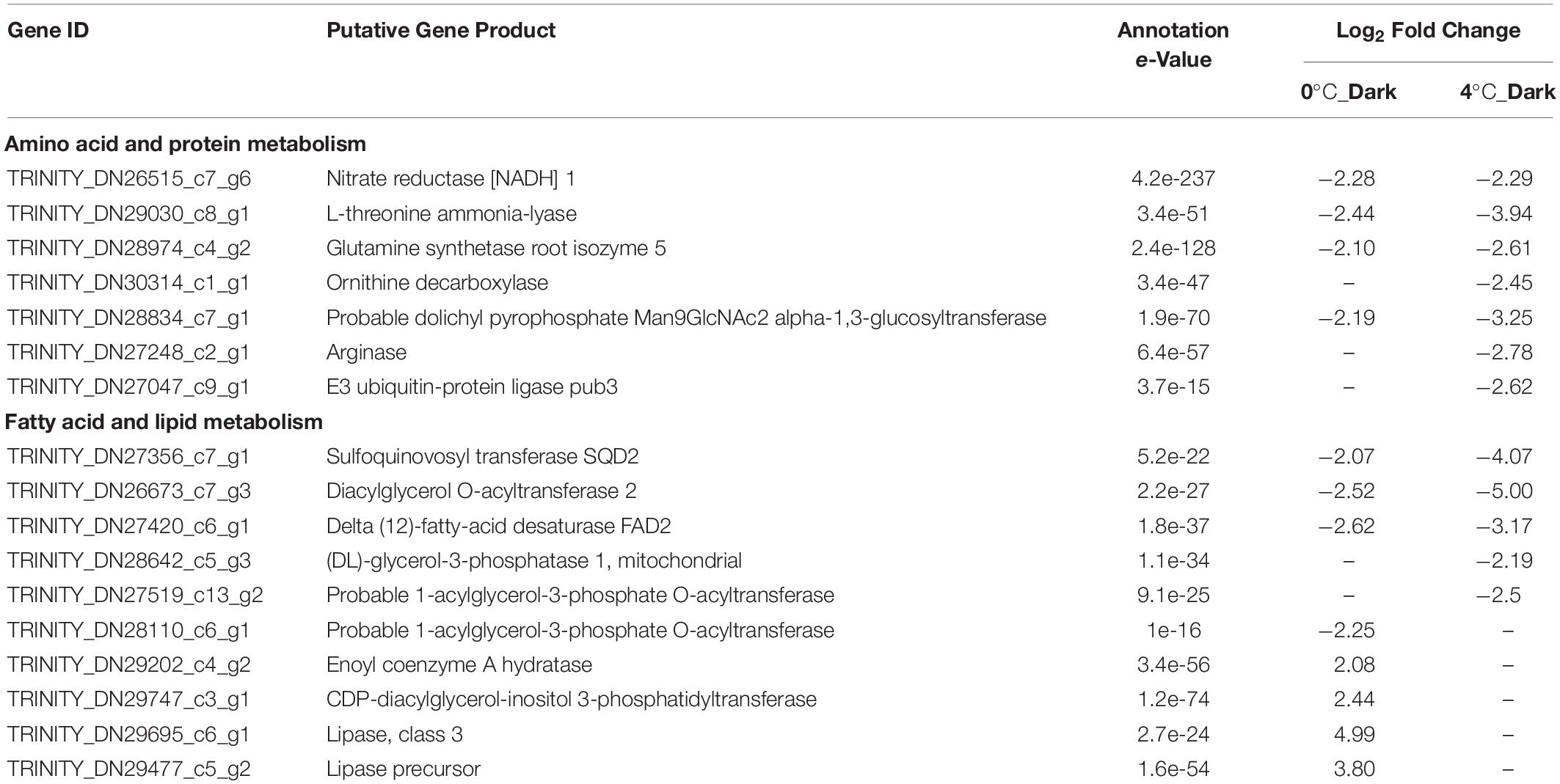
Table 4. Selected significant differentially expressed genes (DEGs) with functions in amino acid and protein metabolism, fatty acid and lipid metabolism after dark exposure at two temperatures (0 and 4°C) versus the same temperature in the light (p ≤ 0.001, | log2 FC| ≥ 2).
Fatty Acid and Lipid Metabolism
0°C_Dark and 4°C_Dark jointly repressed four genes about the biosynthesis of unsaturated fatty acid, triacylglycerol and glycolipid. 4°C_Dark generally had higher fold changes than 0°C_Dark (Table 4). Two different genes encoding probable 1-acylglycerol-3-phosphate O-acyltransferase, function in phosphatidic acid biosynthesis, were found downregulated in 0°C_Dark and 4°C_Dark, respectively. In contrast to the wide repression of fatty acid and lipid biosynthesis, some enzymes about lipid catabolism and fatty acid beta-oxidation were induced in 0°C_Dark, namely three genes encoding lipase and one gene encoding enoyl coenzyme A hydratase.
Discussion
Our study investigated the transcriptomic responses of S. latissima to a 2-week dark exposure by RNA-Seq, combined with physiological performance. Here, we tried to understand the implications of transcriptomic regulations to the survival strategy of kelp during the early polar night.
Photosynthetic Responses
The marked repression of genes encoding components of the LHC induced by darkness suggests that S. latissima reduces the energy costs for encoding photosynthetic components during the prolonged darkness. This transcriptomic response is in accordance with the studies of Nymark et al. (2013) and Mock et al. (2017), who observed that diatoms downregulated genes encoding light-harvesting and photosynthetic components after dark exposure. The repression of LHCs induced by darkness was also observed in diatoms on the proteomic level (Bai et al., 2016; Kennedy et al., 2019). Apart from the repression of genes correlated to LHCs, we did not detect DEGs coding for photosystems and parts of the electron transport chain except one downregulated gene encoding ferredoxin–NADP reductase in 4°C_Dark. After checking the TPM of genes in the light treatments, over 20 genes at 0 and 4°C encoding LHCs had TPM value above the averaged TPM value (20.47 in 0°C_Light and 20.04 in 4°C_Light, Supplementary Table S6). LHC, as a light receptor, captures and delivers excitation energy to photosystems. The abundance of LHC genes might enable S. latissima to adjust its photosynthetic efficiency more flexibly when facing adverse abiotic stress. DEGs related to light-dependent carbon fixation were also limited, which is in accordance with a previous study showing the maintenance of Rubisco content in polar diatoms after 1-month dark exposure (Lacour et al., 2019). In our study, Fv/Fm did not change among treatments. Fv/Fm represents the maximum capacity for absorbed photons conversion into electron transport in photosystem II, and, hence, reflects the overall status of the photochemical machinery (Hanelt, 2018). Scheschonk et al. (2019) also found that field S. latissima can keep stable Fv/Fm during the polar night. In contrast, more laboratory studies observed the decrease of photochemistry potential in algae under darkness with indicators such as Fv/Fm and maximum photosystem II electron transport rate (ETRmax; Lüder et al., 2002; Wulff et al., 2008; Reeves et al., 2011). Lacour et al. (2019) inferred that the decrease of photochemistry is a regulated response to darkness rather than reflecting damage or degradation of photosystem II. Indeed, regardless of the reduced potential for photochemistry in darkness, most of the studies showed that algae can increase fluorescence performances quickly after re-illumination (Lüder et al., 2002; Nymark et al., 2013; Kennedy et al., 2019). Hence, we infer that S. latissima downregulates the expression of some LHCs to reduce any unnecessary energy loss during darkness, while it still keeps the constant expression of photosystem, ETC, carbon fixation, as well as part of LHCs so that it can restore photosynthesis right after the light comes back at the end of the polar night.
The small number of DEGs related to pigment metabolism, combined with the higher contents of Chl a and Acc in dark treatments proved that S. latissima can synthesize pigments in darkness. Several studies also observed that pigments can rise at the first period of darkness (Bird et al., 1982; Lüder et al., 2002; Reeves et al., 2011), and more laboratory studies found that chlorophylls kept stable under 1 to 6-months dark exposure (Weykam et al., 1997; Montechiaro and Giordano, 2006; Naik and Anil, 2018). Sheath et al. (1977) demonstrated that the red alga Porphyra leucosticta can synthesize Chl a in the darkness at rates comparable with those in the light. In the current climate scenario, field investigations showed that S. latissima can maintain pigment content during 4 months of polar night, as shown for the contents of Chl a and antenna pigments (Scheschonk et al., 2019). The small variation of DEGs related to pigment metabolisms in our study is in agreement with above observations, indicating that S. latissima is well adapted to the low light and keeps the pigment metabolism in darkness to preserve the photosynthetic apparatus. This ability also helps algae to resume photosynthesis quickly after re-illumination (Weykam et al., 1997).
Repression of Metabolite Biosynthesis
The biosynthesis of polysaccharides (both structure and storage polysaccharides) were widely inhibited in darkness, likely accounting for the low RGRs in darkness. For storage polysaccharides, brown algae feature a unique carbohydrate metabolism that in the outflow of Calvin cycle they use fructose-6-phosphate to produce mannitol instead of sucrose in higher plants (Michel et al., 2010a). Meanwhile, brown algae possess unique carbon storage polysaccharides laminaran (Percival and Ross, 1951). All genes related to the biosynthesis of mannitol and laminaran were downregulated in both treatments, 0°C_Dark and 4°C_Dark. For the function of structure, alginates, fucoidans, and cellulose are three main polysaccharides in the cell wall of brown algae (Michel et al., 2010b). 0°C_Dark showed 9 repressed genes involved in the metabolism of these three polysaccharides, whereas 4°C_Dark only repressed 4 genes connected to alginates biosynthesis, suggesting that low temperature inhibited the biosynthesis of cell wall polysaccharides. Several mannuronan C-5-epimerases, catalyzing mannuronan to alginate (Michel et al., 2010b), were also upregulated in both darkness treatments. These differential regulations of mannuronan C-5-epimerases might highlight the multi-functions of mannuronan C-5-epimerases in S. latissima during the acclimation to darkness.
Decreased biosynthesis of metabolites was also reflected by the regulation of nitrogen assimilation. Firstly, several nitrate and ammonium transports were repressed, indicating S. latissima reduced its investment for very costly absorbing nitrogen from environments in darkness. Subsequently, the nitrate reductase [NADH], responsible for the reduction of nitrate to nitrite, was also repressed in darkness. The downregulated genes related to amino acids and amine biosynthesis, such as threonine synthase, suggested that S. latissima reduced the biosynthesis of amino acid in darkness, which is in accordance with the low RGR in darkness. Such a decrease of nitrogen assimilation under darkness is also found by Bai et al. (2016), who observed nitrate reductase and glutamine synthetase were downregulated in marine diatom Thalassiosira pseudonana after 4 days dark stress. Similarly, the decrease of fatty acids, as well as the storage and membrane lipids biosynthesis might also contribute to the low RGRs in darkness.
Glycolysis and Respiration
Organisms obtain energy (ATP) from glycolysis and respiration to support their metabolic demands (Nelson and Cox, 2005). The repression of several glycolysis-related genes suggests that S. latissima tends to reduce the consumption of carbohydrates to save energy under prolonged darkness. Mundt et al. (2019) also found that genes encoding for key components of glycolysis were mainly repressed in Cosmarium crenatum after 1-week dark exposure. Additionally, 4°C_Dark exposure repressed more genes related to glycolysis compared to 0°C_Dark, suggesting that increased temperature exaggerates the repression of glycolysis in darkness. Cellular respiration includes three main stages: the production of acetyl-CoA, the oxidation of acetyl-CoA via citric acid cycle, the electron transfer and oxidative phosphorylation (Nelson and Cox, 2005). In our detection of DEGs related to respiration, only one gene encoding cytochrome c was downregulated in both dark treatments. Cytochrome c is the component of the electron transport chain in mitochondria, transferring electrons from complex III to complex IV and reducing O2 to H2O (Nelson and Cox, 2005). The downregulation of cytochrome c, which is a key regulating step in mitochondrial respiration, could suggest a decrease in the respiration rate in darkness compared to light conditions, although this might be corroborated with physiological measurements. Mundt et al. (2019) further found that genes encoding other enzymes and membrane complexes involved in oxidative phosphorylation were downregulated after 1-week in darkness. In contrast, Kennedy et al. (2019) observed that proteins involved in the citric acid cycle and mitochondrial electron transfer chain were generally upregulated in the sea-ice diatom F. cylindrus during 120-day of dark exposure. Such different observations illustrate that the response of respiration to darkness might be different due to the period of dark exposure, species and methodology, and therefore need to be carefully evaluated. Physiological measurements also showed that algae have different respiration rates in darkness (Henley and Dunton, 1997; Weykam et al., 1997; Borum et al., 2002; Montechiaro and Giordano, 2006). For example, the meristem of Laminaria solidungula increased respiration rate while S. latissima kept stable oxygen consumption at the end of the polar night (Scheschonk et al., 2019). Back to our results, the limited DEGs related to respiration versus the reduction of glycolysis suggest that the source of acetyl-CoA for respiration might be changed during the dark acclimation. A possible source for compensation might be from lipids as reflected by the upregulation of enzymes about lipid catabolism and fatty acid β-oxidation in 0°C_Dark.
Stress Responses and Signaling
Organisms produce enhanced reactive oxygen species (ROS) production when they are under abiotic stress (Dring, 2005). Excessive ROS, if their accumulation is exceeding the antioxidant capacity of cells, inhibit photosynthesis and destruct cell components by oxidizing lipids, proteins and nucleic acids (Bartsch et al., 2008). Hence the ability of scavenging ROS plays a crucial role for organisms to adapt to different environments. Peroxiredoxin is prevalent as thioredoxin- or glutaredoxin-dependent peroxidase and functions in reducing organic and inorganic hydroperoxides (Rouhier et al., 2004). L-ascorbate peroxidase can detoxify hydrogen peroxide in the ascorbate-glutathione cycle (Noctor and Foyer, 1998). Both enzymes are important for ROS scavenging, and their downregulation might be due to the fact that photosynthesis, a source of ROS (Mittler, 2002), cannot be conducted in S. latissima during darkness. Consequently, S. latissima suffers less from ROS stress under darkness and saves the energy for synthesizing these two enzymes. Another possible reason might be that the bromoperoxidases play the main role in scavenging ROS in S. latissima, reflected by the upregulation of genes encoding vanadium-dependent bromoperoxidase (vBPO) in both darkness treatments. Haloperoxidases catalyze H2O2 to oxidize halide ions, forming hypohalous acids (Wang et al., 2014). They are regarded as alternative key enzymes for managing oxidative stress in kelps (Bischof and Rautenberger, 2012) and found to be induced in response to different physiological stress, e.g., osmotic (Teo et al., 2009) and temperature stress (Mehrtens and Laturnus, 1997).
Signal transduction is a complex network and important for organisms when facing abiotic stress, since it perceives environmental signals and transmits the signals to the cellular machinery to initiate adaptive reactions (Xiong et al., 2002). In our study, several WSC-domain proteins were upregulated in darkness. The CWI and stress response component (WSC) domain was originally identified in the WSC-family protein of the yeast Saccharomyces cerevisiae (Oide et al., 2019), which has been found in proteins of multiple origins, such as fungi, metazoans, and mammals. In brown algae, the genome of Ectocarpus siliculosus showed that the WSC domain family is one of the largest protein domain families, with 115 genes containing at least one WSC module (Cock et al., 2010). WSC-domain containing proteins are the cell-surface sensors of CWI signaling pathway which detects and responds to cell wall stress induced by various environmental challenges (Levin, 2011). As we discussed above, darkness induced the differential expressions of cell wall polysaccharides, such fluctuations might affect the CWI. Hence, we infer that the WSC-domain proteins might have an important role in sensing the light conditions and transfer the signal to the downstream signaling pathway in S. latissima. Additionally, both 0°C_Dark and 4°C_Dark upregulated a gene encoding CBL-interacting protein kinase. Calcineurin B-like protein (CBL) – interacting protein kinase (CIPK) decodes calcium signals and occupies a vital regulated position of plants in response to different abiotic stress (Kim et al., 2003; Mao et al., 2016). Previous studies found that CBL-CIPK works as the regulator of membrane channel and nutrient transporters to regulate the ion homeostasis, such as sodium (Na+; Shi et al., 2002) and nitrate (NO3–; Ho et al., 2009; Léran et al., 2015). In conclusion, above responses of S. latissima highlight the possible signaling pathway for kelps to adapt to prolonged darkness.
Energy Source and Carbon Replenishment
Mannitol and laminaran are main storage carbohydrate in brown algae, which can be interconverted to each other (Yamaguchi et al., 1966). As summarized by Gómez and Huovinen (2012), mannitol, released from laminaran in distal regions, can be transported to meristematic parts and fueled the kelps’ growth. Previous studies showed that kelps depend on the storage polysaccharide laminaran to maintain basic metabolism during the polar night (Dunton and Schell, 1986; Henley and Dunton, 1995). A field study showed that laminaran was reduced by 96% and mannitol was reduced by 55% in S. latissima after 4 months of the polar night, indicating laminaran is a crucial energy source for kelp in darkness (Scheschonk et al., 2019). The degradation of laminaran is catalyzed by endo-1,3-beta-glucanase, then the laminaran oligosaccharide can be further hydrolyzed by beta-glucosidase (Michel et al., 2010a). In our study, even though 4°C_Dark downregulated two genes encoding beta-glucosidase, their TPM values were generally low in four treatments (Supplementary Table S5). In contrast, the upregulated gene encoding endo-1,3-beta-glucanase in 4°C_Dark had a relatively high TPM value (443.32), which might suggest that S. latissima utilized laminaran as an energy source during the darkness. Mannitol 2-dehydrogenase, responsible for the degradation of mannitol (Michel et al., 2010a), was also expressed but without significant difference. The TPM values showed that mannitol 2-dehydrogenase featured a relatively high TPM which is above the averaged TPM in the light treatments (Supplementary Table S5).
Three genes encoding lipase or the lipase precursor, and one gene encoding enoyl coenzyme A hydratase were induced in 0°C_Dark. Since no upregulated genes connected to lipid catabolism were detected in 4°C_Dark, we checked the TPM values of these four induced DEGs in 0°C_Dark among four treatments (Supplementary Table S5). Lipases are the enzymes that catalyze the hydrolysis of stored triacylglycerols (TAGs), releasing fatty acids for export to sites where they are required as fuel (Nelson and Cox, 2005). Enoyl coenzyme A hydratase is involved in the fatty acid β-oxidation (Poirier et al., 2006). Hence, the upregulation of these enzymes indicates that lipids might be another energy source in S. latissima under prolonged darkness. Mundt et al. (2019) also observed the inductions of lipases after 1-week darkness exposure in C. crenatum. The biochemical study further supports our findings, as Schaub et al. (2017) detected that Navicula cf. perminuta utilized 32% of its TAG at 0°C during 8 weeks of darkness.
Mannitol, as the main product of photosynthesis and basic substrate of carbon metabolism in brown algae (Yamaguchi et al., 1966), provides carbon substrates to synthesize amino acids, nucleotides and other essential metabolites through intermediates produced by pentose phosphate pathway and TCA cycle (Nelson and Cox, 2005). Since photosynthesis cannot be conducted in darkness, S. latissima need to replenish carbon intermediates to complete the basic metabolic activity. In brown algae, LICF is a way to replenish carbon intermediates (Gómez and Huovinen, 2012). PEPC and PEPCK are two key enzymes in LICF. However, we did not find PEPCK in our expression library, and PEPC was downregulated in 4°C_Dark. On the contrary, the upregulation of isocitrate lyase and malate synthase, as well as the lipid catabolism in 0°C_Dark might indicate that S. latissima utilized storage lipids to remedy the carbon shortage via pathways of glyoxylate cycle and gluconeogenesis. The glyoxylate cycle participates in the conversion of lipids to carbohydrates, serving as a primary nutrient source and providing the biosynthetic precursors prior to the commencement of photosynthesis in plant seedlings (Eastmond et al., 2000). Isocitrate lyase and malate synthase are two unique enzymes in the glyoxylate cycle (Dunn et al., 2009). Isocitrate lyase cleaves isocitrate to glyoxylate (Smith and Gunsalus, 1954) and succinate, and malate synthase converts acetyl-CoA and glyoxylate to malate (Kornberg, 1966). In organisms, the production of fructose 6-phosphate from stored lipids is integrated by three reaction sequences, which are glyoxylate cycle in glyoxysomes, TCA cycle in mitochondria, and gluconeogenesis in the cytosol (Nelson and Cox, 2005). The succinate produced by the glyoxylate cycle needs to pass from glyoxysome into mitochondria, then to be converted to oxaloacetate for the following sequences. Consequently, the upregulations of succinate/fumarate mitochondrial transporter in 4°C_Dark might also suggest that darkness induced the glyoxylate cycle in S. latissima. Another evidence for the increased gluconeogenesis is that two genes encoding phosphoglycerate kinase were upregulated in 4°C_Dark. Phosphoglycerate kinase has different isoforms involved in different pathways, i.e., glycolysis, gluconeogenesis, and Calvin cycle (Rosa-Téllez et al., 2018); the upregulated phosphoglycerate kinases were most likely involved in the pathway of gluconeogenesis as another two pathways are supposed to be downregulated under darkness.
Ecological Implication
Arctic kelps are facing increased temperature conditions during the polar night. Hence the adaptive capacity of kelps to enhanced temperature under prolonged darkness is critical to survive in the future scenarios of climate change. Previous studies suggest that Arctic and Antarctic phytoplankton can grow at maximum 6°C above natural environmental conditions and become severely impaired at higher temperatures (Reeves et al., 2011; Martin et al., 2012). Our study also shows that the increased temperature from 0 to 4°C had no significant effect on the RGRs. It should be clarified that, prior to the experiment, the sporophytes were cultured at 12°C for nearly 5 months. Although we have conducted a sequential temperature-acclimation to minimize its effect, this relatively high temperature cultivation could potentially affect the performances of sporophytes and their comparisons between 0 and 4°C. Hence the non-significance of RGRs between 0 and 4°C, as well as the limited DEGs between two temperatures should be carefully evaluated. In addition, due to the longer acclimation period of 0°C treatments, the sporophytes at 0°C were older than sporophytes at 4°C, specifically with wider and less colored thalli (Supplementary Figure S1), which might potentially lead to the higher pigment contents at 4°C than at 0°C. At the transcriptomic level, 4°C_Dark (compared to 4°C_Light) induced more downregulation of genes than 0°C_Dark (compared to 0°C_Light). When comparing 4°C_Dark directly to 0°C_Dark, 4°C_Dark also had more downregulated genes (143) than upregulated genes (74). On one hand, this indicates that the transcriptomic responses are more sensitive than the physiological responses, which is in accordance with the observation of Heinrich et al. (2015). On the other hand, S. latissima might repress transcriptomic activities to reduce enhanced metabolism stimulated by temperature increase, so that it can reduce energy consumption as much as possible in darkness. In fact, current Arctic sea temperature is below the optimum growth temperature of S. latissima, which is from 10 to 15°C (Fortes and Lüning, 1980). In our study, 0°C_Dark upregulated three genes related to stress response (i.e., vanadium-dependent bromoperoxidase and glutathione S-transferase) compared to 4°C_Dark, suggesting that S. latissima might face more severely oxidative stress at 0°C than at 4°C. Hence the moderate temperature increase could possibly help kelp to reduce energy consumption to restore the adverse effects of chilling temperature. At the end of 21st century, the ocean surface warming is projected to vary from about 0.5°C to more than 3°C upon different emission scenarios (IPCC, 2013), which is also below the favor temperature of S. latissima. Li et al. (2020) showed that S. latissima originating from Kongsfjorden grew better at 15 and 8°C than at 0°C. Hence, the increased temperature can help the perennial kelp S. latissima to produce and accumulate more storage compounds during the light season, but in turn accelerates the consumption of compounds during the polar night. As a result, maintaining a positive balance between accumulation and consumption of organic compounds can help kelp survive in the Arctic. Our data indicates that kelp reduce the energetic cost by downregulation of transcriptomes in darkness at 4°C and gives insight into the survival strategy of kelp during the dark acclimation (Figure 5). Even though 2-week dark exposure represents one-tenth of the period of the polar night, our results clearly illustrate the adaptive responses in kelp at the onset of the polar night. A longer dark exposure would presumably help to intensify the effect of increased temperature in kelp performance during the polar night. The investigation of organic carbon metabolism throughout the year could also help to study the final carbon balance of S. latissima. Moreover, Saccharina latissima, as a season responder, grows mostly during a brief period in early spring, when light and temperature increase, and nutrients are not yet depleted by phytoplankton blooms (Bischof et al., 2019). Therefore, the survival of sporophytes under warming conditions would be much clearer if the sporophytes were subjected to a light recovery after the dark incubation.
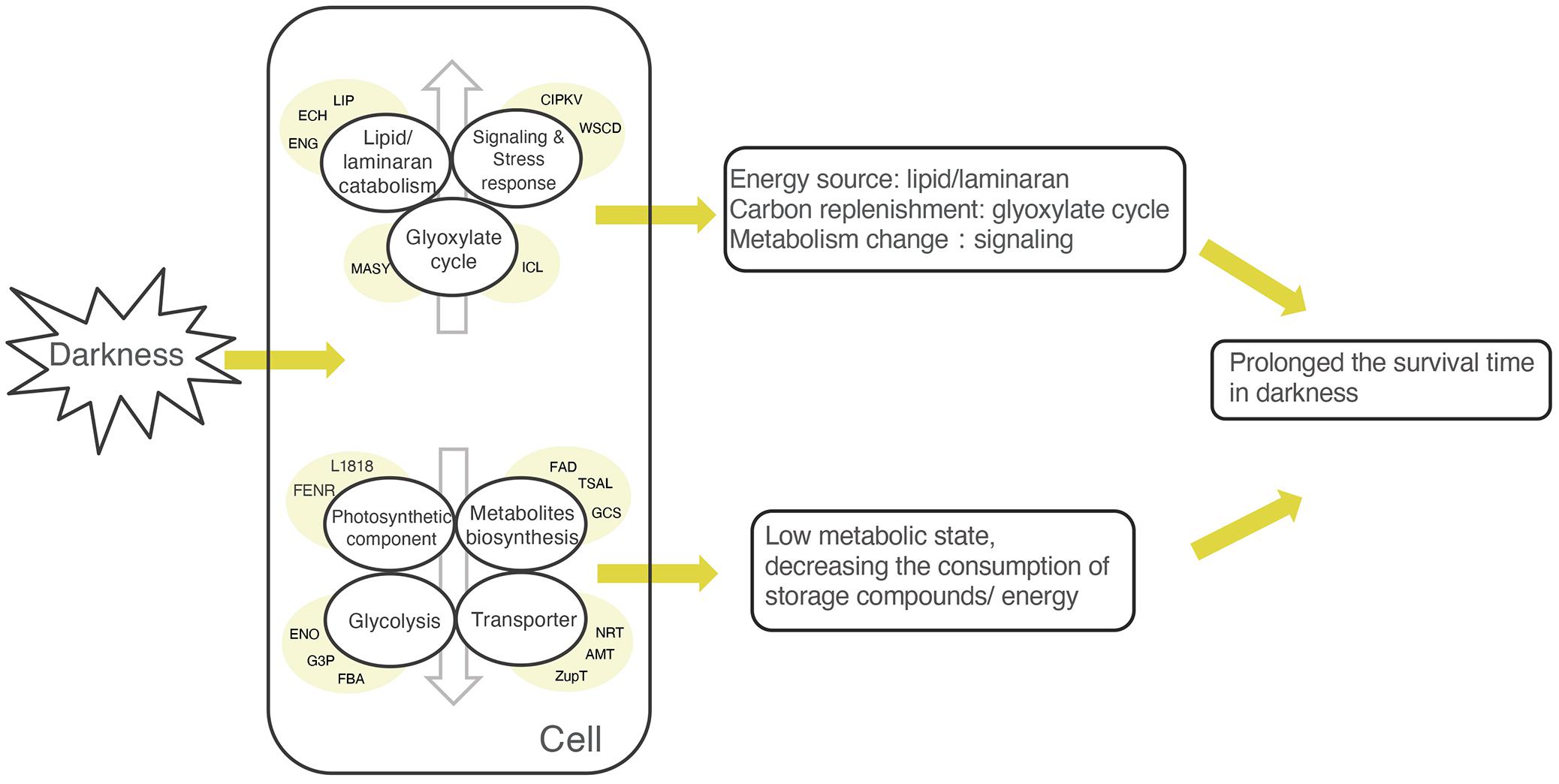
Figure 5. Simplified model explaining the possible survival strategy of Saccharina latissima in darkness. The lowered expression of photosynthetic components, transporter, glycolysis, and metabolite biosynthesis indicates that S. latissima maintained a low metabolic state to decrease the consumption of storage compounds and energy. The upregulation of genes related to lipid/laminaran catabolism and the glyoxylate cycle suggests that S. latissima might use lipid/laminaran as energy sources and replenish carbon substrates via the glyoxylate cycle. Meanwhile, some stress- and signal- related proteins were activated, reflecting that the metabolic states were changing during the acclimation to prolonged darkness. The abbreviations in the yellow circle represent typical differentially expressed genes (DEGs) in each category. Lipid/laminaran catabolism: ECH, enoyl coenzyme A hydratase; EGN, endo-1,3-beta-glucanase, family GH81; LIP, lipase; Signaling and stress responses: CIPKV, CBL-interacting protein kinase; WSCD, WSC domain-containing protein; Glyoxylate cycle: ICL, isocitrate lyase; MASY, malate synthase; Photosynthetic component: FENR, ferredoxin-NADP reductase; L1818, chlorophyll a-b binding protein L1818; Metabolites biosynthesis: FAD, delta (12)-fatty-acid desaturase; GCS, 1,3-beta-glucan synthase; TSAL, L-threonine ammonia-lyase; Glycolysis: ENO, enolase; FBA, fructose-bisphosphate aldolase; G3P, glyceraldehyde-3-phosphate dehydrogenase; Transporter: AMT, ammonium transporter 1 member 2; NRT, high affinity nitrate transporter; ZupT, zinc transporter ZupT.
Data Availability Statement
The datasets generated in this study can be found in online repositories. The names of the repository/repositories and accession number(s) can be found below: NCBI BioProject (accession: PRJNA564197).
Author Contributions
HL, LS, SH, KB, and KV designed the experiment. HL and LS conducted the experiment and carried out the physiological measurements. HL extracted RNA, analyzed the data, and wrote the manuscript. LS contributed to the discussion and draft review. SH and KB supervised the transcriptomic data interpretation and discussion, as well as reviewed the draft. GG carried out the RNA sequencing. EC assembled the de novo transcriptome. LH performed the bioinformatics analysis of RNA-Seq data. KB, SH, GG, and KV supervised the project and helped with data interpretation. All authors reviewed and approved the manuscript.
Funding
The project was funded by the German Research Foundation within the ERA-Net Cofund BiodivERsA 3 program MARFOR (ANR-16-EBI3-0005-01). Further funding was provided by the Alfred-Wegener-Institute Helmholtz Centre for Polar and Marine Research (Bremerhaven, Germany). HL was supported by the Chinese Scholarship Council (No. 201606330045).
Conflict of Interest
The authors declare that the research was conducted in the absence of any commercial or financial relationships that could be construed as a potential conflict of interest.
Acknowledgments
We would like to thank Inka Bartsch for providing the algae cultures. We also thank Britta Meyer-Schlosser and Andreas Wagner for their supports in laboratory work. Cologne Center for Genomics generated the RNA-Seq data.
Supplementary Material
The Supplementary Material for this article can be found online at: https://www.frontiersin.org/articles/10.3389/fmars.2020.592033/full#supplementary-material
Supplementary Figure 1 | Photograph of the spot-labeled sporophytes under different treatments prior to the experiment (day 0) and at the end of the experiment (day 14).
Supplementary Table 1 | Results of the two-way ANOVA for effects of temperature and light treatments on physiological data. The non-parametric test (Mann-Whitney U test) was used for relative growth rates since the homogeneity of variances was violated. Statistically significant values are indicated by asterisks (p < 0.05).
Supplementary Table 2 | Number of differentially expressed genes (DEGs) for the comparisons between four treatments and their annotation rates (p ≤ 0.001, | log2 FC| ≥ 2).
Supplementary Table 3 | Full list of REVIGO summarized GO enrichments of differentially expressed genes (DEGs) from the comparisons of 0°C_Light vs. 0°C_Dark and 4°C_Light vs. 4°C_Dark.
Supplementary Table 4 | Full list of differentially expressed genes (DEGs) from the comparisons of 0°C_Light vs. 0°C_Dark, 0°C_Light vs. 4°C_Light, 4°C_Light vs. 4°C_Dark and 0°C_Dark vs. 4°C_Dark (p ≤ 0.001, | log2 FC| ≥ 2).
Supplementary Table 5 | Transcripts per million (TPM) counts of Trinity genes in four treatments involved in the degradation of laminaran and mannitol, and four significantly upregulated genes about the degradation of lipid and fatty acid in 0°C_Dark compared to 0°C_Light (p ≤ 0.001, —log2 FC— ≥ 2).
Supplementary Table 6 | Transcripts per million (TPM) counts of Trinity genes (above the average TPM value) in 0°C_Light and 4°C_Light encoding the light-harvesting complex.
Footnotes
- ^ http://www.bioinformatics.babraham.ac.uk/projects/fastqc/
- ^ https://trinotate.github.io/
- ^ http://bioinformatics.psb.ugent.be/webtools/Venn/
- ^ http://www.omicshare.com/tools
References
Bai, X., Song, H., Lavoie, M., Zhu, K., Su, Y., Ye, H., et al. (2016). Proteomic analyses bring new insights into the effect of a dark stress on lipid biosynthesis in Phaeodactylum tricornutum. Sci. Rep. 6:25494. doi: 10.1038/srep25494
Bartsch, I., Wiencke, C., Bischof, K., Buchholz, C. M., Buck, B. H., Eggert, A., et al. (2008). The genus Laminaria sensu lato: recent insights and developments. Eur. J. Phycol. 43, 1–86. doi: 10.1080/09670260701711376
Bird, K. T., Habig, C., and DeBusk, T. (1982). Nitrogen allocation and storage patterns in Gracilaria tikvahiae (Rhodophyta). J. Phycol. 18, 344–348. doi: 10.1111/j.1529-8817.1982.tb03194.x
Bischof, K., Buschbaum, C., Fredriksen, S., Gordillo, F. J. L., Heinrich, S., Jimeìnez, C., et al. (2019). “Kelps and environmental changes in kongsfjorden: stress perception and responses,” in The Ecosystem of Kongsfjorden, Svalbard. Advances in Polar Ecology, eds H. Hop and C. Wiencke (Cham: Springer), 373–422. doi: 10.1007/978-3-319-46425-1_10
Bischof, K., and Rautenberger, R. (2012). “Seaweed responses to environmental stress: reactive oxygen and antioxidative strategies,” in Seaweed Biology - Novel Insights into Ecophysiology, Ecology and Utilization, eds C. Wiencke and K. Bischof (Berlin: Springer), 109–132. doi: 10.1007/978-3-642-28451-9
Bolger, A. M., Lohse, M., and Usadel, B. (2014). Trimmonmatic: a flexible trimmer for Illumina sequence data. Bioinformatics 30, 2114–2120. doi: 10.1093/bioinformatics/btu170
Borum, J., Pedersen, M. F., Krause-Jensen, D., Christensen, P. B., and Nielsen, K. (2002). Biomass, photosynthesis and growth of Laminaria saccharina in a high-arctic fjord. NE Greenland. Mar. Biol. 141, 11–19. doi: 10.1007/s00227-002-0806-9
Buchfink, B., Xie, C., and Huson, D. H. (2015). Fast and sensitive protein alignment using DIAMOND. Nat. Methods 12, 59–60. doi: 10.1038/nmeth.3176
Chapman, A. R. O., and Lindley, J. E. (1980). Seasonal growth of Laminaria solidungula in the Canadian high arctic in relation to irradiance and dissolved nutrient concentrations. Mar. Biol. 57, 1–5. doi: 10.1007/BF00420961
Cock, J. M., Sterck, L., Rouzé, P., Scornet, D., Allen, A. E., Amoutzias, G., et al. (2010). The Ectocarpus genome and the independent evolution of multicellularity in brown algae. Nature 465, 617–621. doi: 10.1038/nature09016
Cohen, J. H., Berge, J., Moline, M. A., Asgeir, J. S., Last, K., Falk-Petersen, S., et al. (2015). Is ambient light during the high Arctic polar night sufficient to act as a visual cue for zooplankton? PLoS One 10:e0126247. doi: 10.1371/journal.pone.0126247
Dring, M. J. (2005). Stress resistance and disease resistance in seaweeds: the role of reactive oxygen metabolism. Adv. Bot. Res. 43, 175–207. doi: 10.1016/S0065-2296(05)43004-9
Dunn, M. F., Ramírez-Trujillo, J. A., and Hernández-Lucas, I. (2009). Major roles of isocitrate lyase and malate synthase in bacterial and fungal pathogenesis. Microbiology 155, 3166–3175. doi: 10.1099/mic.0.030858-0
Dunton, K. H. (1985). Growth of dark-exposed Laminaria saccharina (L.) lamour. and Laminaria solidungula J. Ag. (Laminariales: Phaeophyta) in the Alaskan Beaufort Sea. J. Exp. Mar. Biol. Ecol. 94, 181–189. doi: 10.1016/0022-0981(85)90057-7
Dunton, K. H., and Schell, D. M. (1986). Seasonal carbon budget and growth of Laminaria solidungula in the Alaskan High Arctic. Mar. Ecol. Prog. Ser. 31, 57–66. doi: 10.3354/meps031057
Eastmond, P. J., Germain, V., Lange, P. R., Bryce, J. H., Smith, S. M., and Graham, I. A. (2000). Postgerminative growth and lipid catabolism in oilseeds lacking the glyoxylate cycle. Proc. Natl. Acad. Sci. U S A. 97, 5669–5674. doi: 10.1073/pnas.97.10.5669
Fortes, M. D., and Lüning, K. (1980). Growth rates of North Sea macroalgae in relation to temperature, irradiance and photoperiod. Helgolander Meeresunters 34, 15–29. doi: 10.1007/BF01983538
Gómez, I., and Huovinen, P. (2012). “Morpho-functionality of carbon metabolism in seaweeds,” in Seaweed Biology - Novel Insights into Ecophysiology, Ecology and Utilization, eds C. Wiencke and K. Bischof (Berlin: Springer), 25–46. doi: 10.1007/978-3-642-28451-9
Gómez, I., Wulff, A., Roleda, M. Y., Huovinen, P., Karsten, U., Quartino, M. L., et al. (2009). Light and temperature demands of marine benthic microalgae and seaweeds in polar regions. Bot. Mar. 52, 593–608. doi: 10.1515/BOT.2009.073
Grabherr, M. G., Haas, B. J., Yassour, M., Levin, J. Z., Thompson, D. A., Amit, I., et al. (2011). Full-length transcriptome assembly from RNA-Seq data without a reference genome. Nat. Biotechnol. 29, 644–652. doi: 10.1038/nbt.1883
Hanelt, D. (2018). “Photosynthesis assessed by chlorophyll fluorescence,” in Bioassays, eds D.-P. Häder and G. S. Erzinger (Amsterdam: Elsevier), 169–198. doi: 10.1016/B978-0-12-811861-0.00009-7
Hegseth, E. N., Assmy, P., Wiktor, J. M., Wiktor, J. Jr., Kristiansen, S., Leu, E., et al. (2019). “Phytoplankton seasonal dynamics in kongsfjorden, svalbard and the adjacent shelf,” in The Ecosystem of Kongsfjorden, Svalbard. Advances in Polar Ecology, eds H. Hop and C. Wiencke (Cham: Springer), 173–227. doi: 10.1007/978-3-319-46425-1_6
Heinrich, S., Frickenhaus, S., Glöckner, G., and Valentin, K. (2012a). A comprehensive cDNA library of light- and temperature-stressed Saccharina latissima (Phaeophyceae). Eur. J. Phycol. 47, 83–94. doi: 10.1080/09670262.2012.660639
Heinrich, S., Valentin, K., Frickenhaus, S., John, U., and Wiencke, C. (2012b). Transcriptomic analysis of acclimation to temperature and light stress in Saccharina latissima (Phaeophyceae). PLoS One 7:e44342. doi: 10.1371/journal.pone.0044342
Heinrich, S., Valentin, K., Frickenhaus, S., and Wiencke, C. (2015). Temperature and light interactively modulate gene expression in Saccharina latissima (Phaeophyceae). J. Phycol. 51, 93–108. doi: 10.1111/jpy.12255
Henley, W. J., and Dunton, K. H. (1995). A seasonal comparison of carbon, nitrogen, and pigment content in Laminaria solidungula and L. saccharina (Phaeophyta) in the Alaskan Arctic. J. Phycol. 31, 325–331. doi: 10.1111/j.0022-3646.1995.00325.x
Henley, W. J., and Dunton, K. H. (1997). Effects of nitrogen supply and continuous darkness on growth and photosynthesis of the Arctic kelp Laminaria solidungula. Limnol. Oceanogr. 42, 209–216. doi: 10.4319/lo.1997.42.2.0209
Ho, C. H., Lin, S. H., Hu, H. C., and Tsay, Y. F. (2009). CHL1 functions as a nitrate sensor in plants. Cell 138, 1184–1194. doi: 10.1016/j.cell.2009.07.004
Hurd, C. L., Harrison, P. J., Bischof, K., and Lobban, C. S. (2014). Seaweed Ecology and Physiology, 2nd Edn. Cambridge: Cambridge University Press.
Iñiguez, C., Heinrich, S., Harms, L., and Gordillo, F. J. L. (2017). Increased temperature and CO2 alleviate photoinhibition in Desmarestia anceps: from transcriptomics to carbon utilization. J. Exp. Bot. 68, 3971–3984. doi: 10.1093/jxb/erx164
IPCC (2007). Climate Change 2007: The Physical Science Basis. Contribution of Working Group I to the Fourth Assessment Report of the Intergovernmental Panel on Climate Change. Cambridge: Cambridge University Press.
IPCC (2013). Climate Change 2013: The Physical Science Basis. Contribution of Working Group I to the Fifth Assessment Report of the Intergovernmental Panel on Climate Change. Cambridge: Cambridge University Press.
Jochem, F. J. (1999). Dark survival strategies in marine phytoplankton assessed by cytometric measurement of metabolic activity with fluorescein diacetate. Mar. Biol. 135, 721–728. doi: 10.1007/s002270050673
Karsten, U., Schaub, I., Woelfel, J., Sevilgen, D. S., Schlie, C., Becker, B., et al. (2019). “Living on cold substrata: new insights and approaches in the study of microphytobenthos ecophysiology and ecology in Kongsfjorden,” in The Ecosystem of Kongsfjorden, Svalbard. Advances in Polar Ecology, eds H. Hop and C. Wiencke (Cham: Springer), 303–330. doi: 10.1007/978-3-319-46425-1_8
Kennedy, F., Martin, A., Bowman, J. P., Wilson, R., and McMinn, A. (2019). Dark metabolism: a molecular insight into how the Antarctic sea-ice diatom Fragilariopsis cylindrus survives long-term darkness. New Phytol. 223, 675–691. doi: 10.1111/nph.15843
Kim, K. N., Lee, J. S., Han, H., Choi, S. A., Go, S. J., and Yoon, I. S. (2003). Isolation and characterization of a novel rice Ca2 + -regulated protein kinase gene involved in responses to diverse signals including cold, light, cytokinins, sugars and salts. Plant Mol. Biol. 52, 1191–1202. doi: 10.1023/B:PLAN.0000004330.62660.a2
Koch, K., Thiel, M., Tellier, F., Hagen, W., Graeve, M., Tala, F., et al. (2015). Species separation within the Lessonia nigrescens complex (Phaeophyceae, Laminariales) is mirrored by ecophysiological traits. Bot. Mar. 58, 81–92. 10.1515/bot-2014-0086
Kornberg, H. L. (1966). The role and control of the glyoxylate cycle in Escherichia coli. Biochem. J. 99, 1–11. doi: 10.1042/bj0990001
Lacour, T., Morin, P.-I., Sciandra, T., Donaher, N., Campbell, D. A., Ferland, J., et al. (2019). Decoupling light harvesting, electron transport and carbon fixation during prolonged darkness supports rapid revovery upon re-illumination in the Arctic diatom Chaetoceros neogracilis. Polar Biol. 42, 1787–1799. doi: 10.1007/s00300-019-02507-2
Léran, S., Edel, K. H., Pervent, M., Hashimoto, K., Corratgé-Faillie, C., Offenborn, J. N., et al. (2015). Nitrate sensing and uptake in Arabidopsis are enhanced by ABI2, a phosphatase inactivated by the stress hormone abscisic acid. Sci. Signal. 8:ra43. doi: 10.1126/scisignal.aaa4829
Levin, D. E. (2011). Regulation of cell wall biogenesis in Saccharomyces cerevisiae: the cell wall integrity signaling pathway. Genetics 189, 1145–1175. doi: 10.1534/genetics.111.128264
Li, H., Monteiro, C., Heinrich, S., Bartsch, I., Valentin, K., Harms, L., et al. (2020). Responses of the kelp Saccharina latissima (Phaeophyceae) to the warming Arctic: from physiology to transcriptomics. Physiol. Plantarum 168, 5–26. doi: 10.1111/ppl.13009
Love, M. I., Huber, W., and Anders, S. (2014). Moderated estimation of fold change and dispersion for RNA-seq data with DESeq2. Genome Biol. 15:550. doi: 10.1186/s13059-014-0550-8
Lüder, U. H., Wiencke, C., and Knoetzel, J. (2002). Acclimation of photosynthesis and pigments during and after six months of darkness in Palmaria decipiens (Rhodophyta): a study to simulate Antarctic winter sea ice cover. J. Phycol. 38, 904–913. doi: 10.1046/j.1529-8817.2002.t01-1-01071.x
Lüning, K. (1969). Growth of amputated and dark-exposed individuals of the brown alga Laminaria hyperborea. Mar. Biol. 2, 218–223. doi: 10.1007/BF00351143
Lüning, K., Yarish, C., and Kirkman, H. (1990). Seaweeds - Their Environment, Biogeography and Ecophysiology. New York, NY: Wiley-Interscience Publication.
MacDonald, G. M. (2010). Global warming and the Arctic: a new world beyond the reach of the Grinnellian niche? J. Exp. Biol. 213, 855–861. doi: 10.1242/jeb.039511
Machado Monteiro, C. M., Li, H., Bischof, K., Bartsch, I., Valentin, K. U., Corre, E., et al. (2019). Is geographical variation driving the transcriptomic responses to multiple stressors in the kelp Saccharina latissima? BMC Plant Biol. 19:513. doi: 10.1186/s12870-019-2124-0
Manoharan, K., Lee, T. K., Cha, J. M., Kim, J. H., Lee, W. S., Chang, M., et al. (1999). Acclimation of Prorocentrum minimum (Dinophyceae) to prolonged darkness by use of an alternative carbon source from triacylglycerides and galactolipids. J. Phycol. 35, 287–292. doi: 10.1046/j.1529-8817.1999.3520287.x
Mao, J., Manik, S. M. N., Shi, S., Chao, J., Jin, Y., Wang, Q., et al. (2016). Mechanisms and physiological roles of the CBL-CIPK networking system in Arabidopsis thaliana. Genes 7:62. doi: 10.3390/genes7090062
Martin, A., McMinn, A., Heath, M., Hegseth, E. N., and Ryan, K. G. (2012). The physiological response to increased temperature in over-wintering sea ice algae and phytoplankton in McMurdo Sound, Antarctica and Tromaø Sound, Norway. J. Exp. Mar. Biol. Ecol. 428, 57–66. doi: 10.1016/j.jembe.2012.06.006
Mcminn, A., and Martin, A. (2013). Dark survival in a warming world. Proc. R. Soc. B 280:20122909. doi: 10.1098/rspb.2012.2909
Mehrtens, G., and Laturnus, F. (1997). Halogenating activity in an arctic population of brown macroalga Laminaria saccharina (L.) Lamour. Polar Res. 16, 19–26. doi: 10.3402/polar.v16i1.6622
Michel, G., Tonon, T., Scornet, D., Cock, J. M., and Kloareg, B. (2010a). Central and storage carbon metabolism of the brown alga Ectocarpus siliculosus: insights into the origin and evolution of storage carbohydrates in Eukaryotes. New Phytol. 188, 67–81. doi: 10.1111/j.1469-8137.2010.03345.x
Michel, G., Tonon, T., Scornet, D., Cock, J. M., and Kloareg, B. (2010b). The cell wall polysaccharide metabolism of the brown alga Ectocarpus siliculosus. Insights into the evolution of extracellular matrix polysaccharides in Eukaryotes. New Phytol. 188, 82–97. doi: 10.1111/j.1469-8137.2010.03374.x
Mittler, R. (2002). Oxidative stress, antioxidants and stress tolerance. Trends in Plant Sci. 7, 405–410. doi: 10.1016/S1360-1385(02)02312-9
Mock, T., Otillar, R. P., Strauss, J., McMullan, M., Paajanen, P., Schmutz, J., et al. (2017). Evolutionary genomics of the cold-adapted diatom Fragilariopsis cylindrus. Nature 541, 536–540. doi: 10.1038/nature20803
Montechiaro, F., and Giordano, M. (2006). Effect of prolonged dark incubation on pigments and photosynthesis of the cave-dwelling cyanobacterium Phormidium autumnale (Oscillatoriales, Cyanobacteria). Phycologia 45, 704–710. doi: 10.2216/06-15.1
Mundt, F., Hanelt, D., Harms, L., and Heinrich, S. (2019). Darkness-induced effects on gene expression in Cosmarium crenatum (Zygnematophyceae) from a polar habitat. Sci. Rep. 9:10559. doi: 10.1038/s41598-019-47041-7
Naik, S. M., and Anil, A. C. (2018). Influence of darkness on pigments of Tetraselmis indica (Chlorodendrophyceae, Chlorophyta). J. Photochem. Photobiol. B, Biol. 186, 17–22. doi: 10.1016/j.jphotobiol.2018.06.010
Nelson, D. L., and Cox, M. M. (2005). Lehninger Principles of Biochemistry, 7th Edn. New York, NY: W. H. Freeman and Company.
Noctor, G., and Foyer, C. H. (1998). Ascorbate and glutathione: keeping active oxygen under control. Annu. Rev. Plant Physiol. Plant Mol. Biol. 49, 249–279. doi: 10.1146/annurev.arplant.49.1.249
Nymark, M., Valle, K. C., Hancke, K., Winge, P., Andresen, K., Johnsen, G., et al. (2013). Molecular and photosynthetic responses to prolonged darkness and subsequent acclimation to re-illumination in the diatom Phaeodactylum tricornutum. PLoS One 8:e58722. doi: 10.1371/journal.pone.0058722
Oide, S., Tanaka, Y., Watanabe, A., and Inui, M. (2019). Carbohydrate-binding property of a cell wall integrity and stress response component (WSC) domain of an alcohol oxidase from the rice blast pathogen Pyricularia oryzae. Enzyme Microb. Technol. 125, 13–20. doi: 10.1016/j.enzmictec.2019.02.009
Overland, J. E., Hanna, E., Hanssen-Bauer, I., Kim, S.-J., Walsh, J. E., Wang, M., et al. (2019). “Surface air temperature,” in Arctic Report Card 2019, eds J. Richter-Menge, M. L. Druckenmiller, and M. Jeffries (Washington, D.C: NOAA). Available online at: https://arctic.noaa.gov/Report-Card/Report-Card-2019
Palmisano, A. C., and Sullivan, C. W. (1982). Physiology of sea ice diatoms. I. response of three polar diatoms to a simulated summer-winter transition. J. Phycol. 18, 489–498. doi: 10.1111/j.1529-8817.1982.tb03215.x
Patro, R., Duggal, G., Love, M. I., Irizarry, R. A., and Kingsford, C. (2017). Salmon provides fast and bias-aware quantification of transcript expresion. Nat. Methods 14, 417–419. doi: 10.1038/nmeth.4197
Pavlova, O., Gerland, S., and Hop, H. (2019). “Changes in sea-ice extent and thickness in Kongsfjorden, Svalbard (2003-2016),” in The Ecosystem of Kongsfjorden, Svalbard. Advances in Polar Ecology, eds H. Hop and C. Wiencke (Cham: Springer), 105–136. doi: 10.1007/978-3-319-46425-1_4
Percival, E., and Ross, A. (1951). The constitution of laminarin. Part II. the soluble laminarin of laminaria digitata. J. Chem. Soc. 720–726. doi: 10.1039/JR9510000720
Peters, E., and Thomas, D. N. (1996). Prolonged darkness and diatom mortality I: marine Antarctic species. J. Exp. Mar. Biol. Ecol. 207, 25–41. doi: 10.1016/S0022-0981(96)02520-8
Poirier, Y., Antonenkov, V. D., Glumoff, T., and Hiltunen, J. K. (2006). Peroxisomal β-oxidation - a metabolic pathway with multiple functions. Biochim. Biophys. Acta Mol. Cell Res. 1763, 1413–1426. doi: 10.1016/j.bbamcr.2006.08.034
Reeves, S., McMinn, A., and Martin, A. (2011). The effect of prolonged darkness on the growth, recovery and survival of Antarctic sea ice diatoms. Polar Biol. 34, 1019–1032. doi: 10.1007/s00300-011-0961-x
Rosa-Téllez, S., Anoman, A. D., Flores-Tornero, M., Toujani, W., Alseek, S., Fernie, A. R., et al. (2018). Phosphoglycerate kinases are co-regulated to adjust metabolism and to optimize growth. Plant Physiol. 176, 1182–1198. doi: 10.1104/pp.17.01227
Rouhier, N., Gelhaye, E., Gualberto, J. M., Jordy, M.-N., De Fay, E., Hirasawa, M., et al. (2004). Poplar peroxiredoxin Q. A thioredoxin-linked chloroplast antioxidant functional in pathogen defense. Plant Physiol. 134, 1027–1038. doi: 10.1104/pp.103.035865
Schaub, I., Wagner, H., Graeve, M., and Karsten, U. (2017). Effects of prolonged darkness and temperature on the lipid metabolism in the benthic diatom Navicula perminuta from the Arctic Adventfjorden. Svalbard. Polar Biol. 40, 1425–1439. doi: 10.1007/s00300-016-2067-y
Scheschonk, L., Becker, S., Hehemann, J., Diehl, N., Karsten, U., and Bischof, K. (2019). Arctic kelp eco-physiology during the polar night in the face of global warming: a crucial role for laminarin. Mar. Ecol. Prog. Ser. 611, 59–74. doi: 10.3354/meps12860
Sheath, R. G., Hellebust, J. A., and Sawa, T. (1977). Changes in plastid structure, pigmentation and photosynthesis of the conchocelis stage of Porphyra leucosticta (Rhodophyta, Bangiophyceae) in response to low light and darkness. Phycologia 16, 265–275. doi: 10.2216/i0031-8884-16-3-265.1
Shi, H., Quintero, F. J., Pardo, J. M., and Zhu, J.-K. (2002). The putative plasma membrane Na+/ H+antiporter SOS1 controls long-distance Na+ transport in plants. Plant Cell 14, 465–477. doi: 10.1105/tpc.010371
Smayda, T. J., and Mitchell-Innes, B. (1974). Dark survival of autotrophic, planktonic marine diatoms. Mar. Biol. 25, 195–202. doi: 10.1007/BF00394965
Smith, R. A., and Gunsalus, I. C. (1954). Isocitritase: a new tricarboxylic acid cleavage system. J. Am. Chem. Soc. 76, 5002–5003. doi: 10.1021/ja01648a084
Starr, R. C., and Zeikus, J. A. (1993). UTEX - the culture collection of algae at the University of Texas at Austin 1993 list of cultures. J. Phycol. 29, 1–106. doi: 10.1111/j.0022-3646.1993.00001.x
Supek, F., Bošnjak, M., Škunca, N., and Šmuc, T. (2011). REVIGO summarizes and visualizes long lists of gene ontology terms. PLoS One 6:e21800. doi: 10.1371/journal.pone.0021800
Svendsen, H., Beszczynska-Møller, A., Hagen, J. O., Lefauconnier, B., Tverberg, V., Gerland, S., et al. (2002). The physical environment of Kongsfjorden-Krossfjorden, an Arctic fjord system in Svalbard. Polar Res. 21, 133–166. doi: 10.3402/polar.v21i1.6479
Teo, S.-S., Ho, C.-L., Teoh, S., Rahim, R. A., and Phang, S.-M. (2009). Transcriptomic analysis of Gracilaria changii (Rhodophyta) in response to hyper- and hypoosmotic stresses. J. Phycol. 45, 1093–1099. doi: 10.1111/j.1529-8817.2009.00724.x
Wang, T., Lu, Y., Cao, D., Gao, S., and Zhang, Y. (2014). “Research development on vanadium-dependent haloperoxidases in marine algae,” in Proceedings of the 2012 International Conference on Applied Biotechnology (ICAB 2012). Lecture Notes in Electrical Engineering, eds T.-C. Zhang, P. Ouyang, S. Kaplan, and B. Skarnes (Berlin: Springer), 1741–1748. doi: 10.1007/978-3-642-37925-3_187
Weykam, G., Thomas, D. N., and Wiencke, C. (1997). Growth and photosynthesis of the Antarctic red algae Palmaria decipiens (Palmariales) and Iridaea cordata (Gigartinales) during and following extended periods of darkness. Phycologia 36, 395–405. doi: 10.2216/i0031-8884-36-5-395.1
Wulff, A., Roleda, M. Y., Zacher, K., and Wiencke, C. (2008). Exposure to sudden light burst after prolonged darkness-a case study on benthic diatoms in Antarctica. Diatom Res. 23, 519–532. doi: 10.1080/0269249X.2008.9705774
Xiong, L., Schumaker, K. S., and Zhu, J.-K. (2002). Cell signaling during cold, drought and salt stress. Plant Cell 14, S165–S183. doi: 10.1105/tpc.000596
Yamaguchi, T., Ikawa, T., and Nisizawa, K. (1966). Incorporation of radioactive carbon from H14CO3– into sugar constituents by a brown alga, Eisenia bicyclis, during photosynthesis and its fate in the dark. Plant Cell Physiol. 7, 217–229. doi: 10.1093/oxfordjournals.pcp.a079175
Keywords: kelp, Saccharina latissima, growth rates, transcriptomic responses, dark exposure, Arctic
Citation: Li H, Scheschonk L, Heinrich S, Valentin K, Harms L, Glöckner G, Corre E and Bischof K (2020) Transcriptomic Responses to Darkness and the Survival Strategy of the Kelp Saccharina latissima in the Early Polar Night. Front. Mar. Sci. 7:592033. doi: 10.3389/fmars.2020.592033
Received: 06 August 2020; Accepted: 02 November 2020;
Published: 23 December 2020.
Edited by:
Qinglu Zeng, Hong Kong University of Science and Technology, Hong KongReviewed by:
Fraser Kennedy, University of Tasmania, AustraliaAdam Michael Reitzel, University of North Carolina at Charlotte, United States
Concepcion Iñiguez, University of the Balearic Islands, Spain
Copyright © 2020 Li, Scheschonk, Heinrich, Valentin, Harms, Glöckner, Corre and Bischof. This is an open-access article distributed under the terms of the Creative Commons Attribution License (CC BY). The use, distribution or reproduction in other forums is permitted, provided the original author(s) and the copyright owner(s) are credited and that the original publication in this journal is cited, in accordance with accepted academic practice. No use, distribution or reproduction is permitted which does not comply with these terms.
*Correspondence: Kai Bischof, a2Jpc2Nob2ZAdW5pLWJyZW1lbi5kZQ==