- 1CAS Key Laboratory of Experimental Marine Biology, Institute of Oceanology, Chinese Academy of Sciences, Qingdao, China
- 2Laboratory for Marine Biology and Biotechnology, Pilot National Laboratory for Marine Science and Technology, Qingdao, China
- 3Center for Ocean Mega-Science, Chinese Academy of Sciences, Qingdao, China
- 4National and Local Joint Engineering Laboratory of Ecological Mariculture, Institute of Oceanology, Chinese Academy of Sciences, Qingdao, China
- 5University of Chinese Academy of Sciences, Beijing, China
- 6Laboratory for Marine Fisheries Science and Food Production Processes, Pilot National Laboratory for Marine Science and Technology, Qingdao, China
- 7Ocean College, Beibu Gulf University, Qinzhou, China
Natural selection and isolation are both important for understanding the geographic distribution of marine species and environmental responses to changing climate. In this study, we revealed distinct genetic variation in Crassostrea ariakensis by comparing the COI gene segment sequence in northern and southern oysters partitioned by the Yangtze River estuary. Reciprocal hybridization and intrapopulation crosses clarified their taxonomic status as the same species. There was no heterosis in the survival and growth of the hybrids, while the maternal effect was observed in larvae from eggs in the native habitat that showed higher fitness. Both the northern and southern F1 progenies exhibited positive performance in fitness traits, including survivorship, respiration rate, and growth, in their native habitats compared to that in their non-native habitats, indicating a strong signature of local adaptation. The oysters dwelling in the warm/southern habitats evolved a higher thermotolerance of LT50, while the oysters inhabiting the high-salinity/northern habitats had a 2.43‰ higher LS50 than that of their southern counterparts. After strong natural selection in the northern environments, the higher survival of the F1 progenies from the southern oysters under heat shock indicates an evolved genetic basis for its higher thermal tolerance. Strong environmental gradients, especially for temperature and salinity, and geographic isolation by the interaction between coastal currents and the Yangtze River estuary potentially contribute to shaping the distribution pattern and adaptive divergence of C. ariakensis in China.
Introduction
Understanding geographic distribution and the causes that drive the spatiotemporal patterns of species or populations, including selection and isolation, can provide fundamental information on organismal responses and adaptive capacity in the face of rapid global change, as well as further direct implications of marine resources and fisheries management. A growing body of studies indicates that marine ecosystems are not open with high connectivity, but are accompanied by strong latitudinal, vertical, and mosaic environmental gradients, especially in coastal and estuarine areas, where the temperature, salinity, and other biotic and abiotic factors experience extreme variations at the fine-tuning scale (Bozinovic et al., 2011; Sanford and Kelly, 2011; Somero, 2012). Adaptive differentiation was subsequently reported in many marine species, even in high gene flow animals with high motility, such as fishes (Limborg et al., 2012; Dayan et al., 2015) or those with long-term planktonic stages in their life history, such as sessile mollusks occurring over tens of meters (De Wit and Palumbi, 2013; Burford et al., 2014; Li et al., 2017; Li A. et al., 2018; Li L. et al., 2018; Li et al., 2019; Ghaffari et al., 2019). Geographic or oceanographic isolation or homogenization can also contribute to shaping the spatial distribution and environmental responses of marine species. In China, the Yangtze (Chang Jiang) River has long been rendered as a marine biogeographic boundary causing distinct ecological gradients, especially for temperature and salinity, and shaping adaptive differentiations in warm-tolerant and cold-water populations or species of coastal animals under spatially varying selection. Ni et al. (2017) comprehensively summarized the effects of the Yangtze River as well as its interaction with coastal currents on the genetic diversity of many marine species, such as fishes, crabs, mollusks, etc., Most of the marine species exhibited divergent genetic structures between the northern and southern populations separated by the Yangtze River, while coastal currents facilitate internal gene flows within the northern or southern populations (Xu et al., 2009; Xiao et al., 2016; Li L. et al., 2018). These findings highlight the significant role of stochastic geographic isolation following environmental selection in ecological, evolutionary, and taxonomic studies.
Oysters are marine bivalve mollusks distributed worldwide in intertidal, estuarine, and shallow ocean areas where the environmental conditions are extremely variable, providing a model species for exploring adaptive evolution. Generally, different oyster species inhabit distinct latitudinal or vertical gradients because they have evolved species-specific adaptive capacities in different environments (Wang et al., 2008, 2010; Guo et al., 2018). For sympatric oyster species, however, we need to first classify them before conducting subsequent biological examinations. For example, the congeneric oysters Crassostrea hongkongensis sympatrically coexist with Crassostrea ariakensis in most estuarine habitats in southern China (Wang et al., 2006) and these two species can unidirectionally hybridize with each other based on molecular, morphological, and fitness assessments (Zhang et al., 2017; Qin et al., 2020). Oyster classification is always difficult because of their high plasticity in shell morphology, which is sensitive to environmental heterogeneity. Genetic markers such as mitochondrial cytochrome oxidase I (COI) or nuclear 28S ribosomal RNA genes have been developed to identify these oysters in previous studies (Wang et al., 2004; Wang and Guo, 2008; Guo et al., 2018). Moreover, a high-throughput, efficient, and easy to visualize method of high-resolution melting (HRM) curve analysis has been applied to taxonomic studies in the classification of Crassostrea oyster species (Wang et al., 2014, 2015), which can be extensively used to rapidly and reliably identify oysters collected from multiple locations in large sampling size.
Crassostrea ariakensis is one of the most common and well-known oysters in China, and is mainly found in rivers and estuaries with salinities of 10–25 ppt (Guo et al., 1999). It is one of the most important economic oyster species and is broadly distributed in China, ranging from the Lizijiang (Oyster River) in the north to the Beihai in the south (Guo et al., 1999; Zhou and Allen, 2003). However, natural resources have dramatically declined in the past decades, especially in the north due to overfishing, decreased runoff, and habitat destruction. Disconnected distribution and significant environmental gradients across inhabited estuaries imply differentiations in genomic components and plasticity among different populations, which requires investigations to assess their adaptive capacity in response to environmental challenges, particularly for elevated temperature and salinity. However, previous studies only revealed sequence variations between northern and southern populations of C. ariakensis separated by the Yangtze River estuary using neutral mitochondrial and nuclear gene markers, and speculated the occurrence of reproductive isolation between these two divergent populations (Wang et al., 2004; Zhang et al., 2005; Xiao et al., 2010; Kim et al., 2014). Hybridization experiments need to be carried out after knowing about genetic differentiation, which could provide the most informative findings to verify this hypothesis and further clarify their taxonomic status, e.g., whether they should be considered as two separate species or subspecies (Wang et al., 2004, 2010). Our previous study found divergent transcriptomic responses of eye-spot larvae to salinity gradients between different latitudinal C. ariakensis populations and identified several salinity-stress responsive genes (Liu et al., 2019). However, assessments of fitness-related traits, including survival, metabolic rate, and growth, via common garden and reciprocal transplant experiments in different environmental habitats (Sanford and Kelly, 2011) are needed to compare the environmental/stress responses between oysters from northern and southern sampling sites, which could provide direct evidence to reveal whether they are locally adapted to their native habitats as well as examine the primary environmental factors that drove their adaptive divergence if it occurred.
In this study, we collected 278 wild C. ariakensis oysters from 11 estuaries in China and found distinct genetic variation between the oysters from northern (seven locations) and southern (four locations) sampling sites using HRM analysis of the COI gene segment with C. hongkongensis as the outgroup. Reciprocal hybridization experiments were performed to clarify the taxonomic status of the northern and southern oysters. The viability of hybrids from bidirectional crosses classified them as the same species. Long-term common garden and reciprocal transplantation of the northern and southern F1 oysters between their native habitats were carried out, and fitness-related traits, including survival, metabolic rate, and growth, were measured to explore whether local adaptation occurred. To investigate the potential factors that shape adaptive divergence, we monitored the primary abiotic environmental factors, including temperature and salinity, of the northern and southern habitats, and assessed the stress tolerance of the northern and southern oysters in response to these two factors. We proposed that strong environmental gradients and the interaction between coastal currents and the Yangtze River estuary potentially contribute to shaping the geographic distribution and adaptive divergence of C. ariakensis in China. Identification of genetic diversity among wild C. ariakensis populations across most Chinese estuaries and documentation of strong local adaptation between northern and southern populations can provide biological significance for management strategies for the regional mariculture of C. ariakensis.
Materials and Methods
Sampling Locations and Monitoring of Seawater Temperature and Salinity in the Southern and Northern Habitats
We collected wild oysters of C. ariakensis from 11 sampling sites dwelling in northern (seven locations) and southern (four locations) estuary regions to explore the genetic variations in the COI gene segment (Figure 1A, Supplementary Table 1). We selected two locations in the northern (Binzhou, BZ, 38.18°N) and southern (Taishan, TS, 21.95°N) estuaries, which have tremendous environmental differences, especially for temperature and salinity, to monitor these two parameters of the field habitats during summer after larval attachment (July to October) using HOBO Conductivity U24 Data Loggers (ONSET, Adelaide, Australia) with a time interval of 1 h to record the data at the same depth as the cultured oysters.
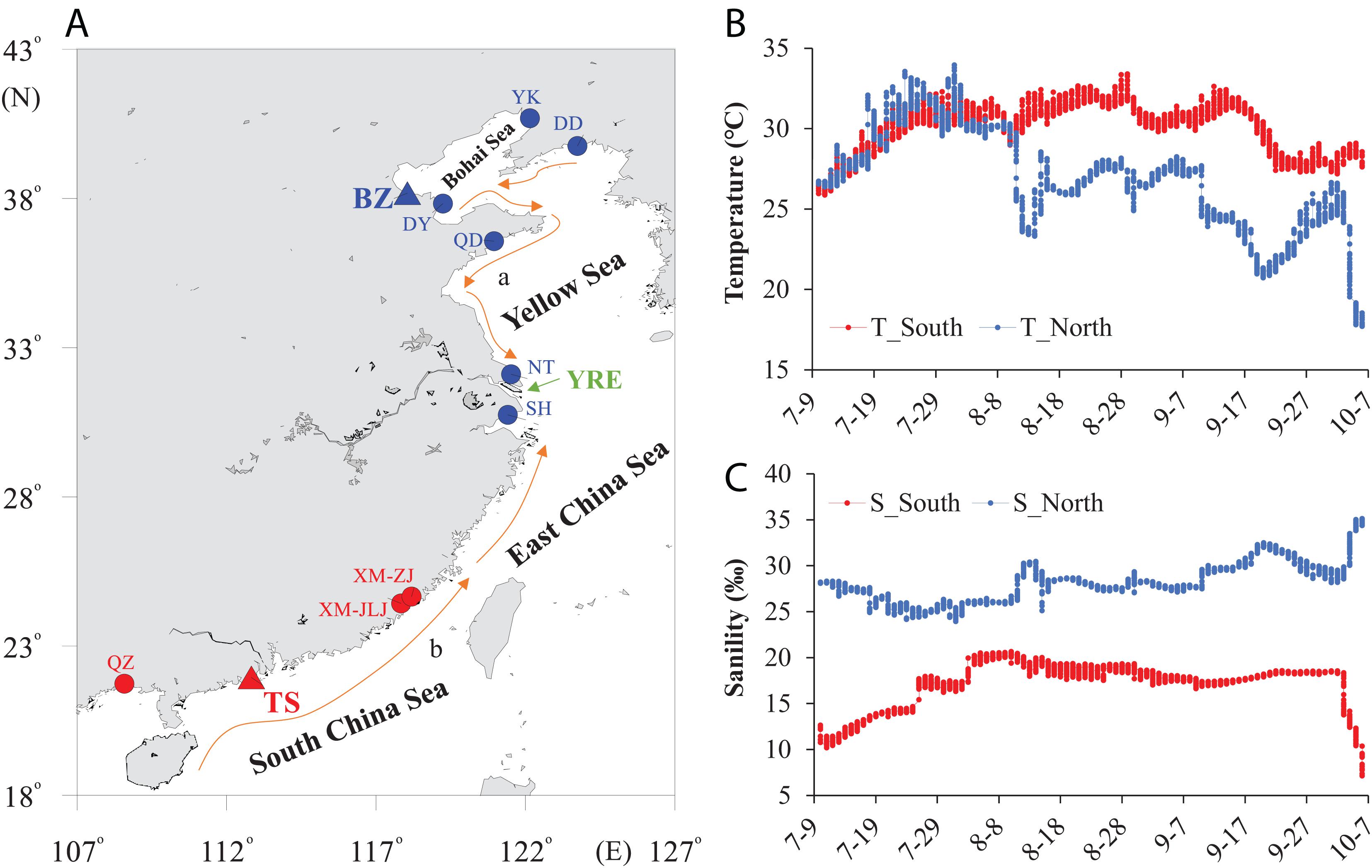
Figure 1. Sampling locations and environmental parameters. (A) Sampling map showing the locations of 11 C. ariakensis populations dwelling in estuaries along the coasts of China. Two populations derived from the southern (Taishan: TS) and northern (Binzhou: BZ) habitats were selected for the phenotype assessments (triangle). (A) China Coastal Current; (B) South China Sea Warm Current. (B) Seawater temperature and (C) salinity of the two selected locations recorded by a conductivity logger with a 1 h interval. T: temperature, S: salinity, YK: Yingkou, DD: Dandong, DY: Dongying, QD: Qingdao, NT: Nantong, SH: Shanghai, XM-ZJ: Xiamen-Zhangjiang, XM-JLJ: Xiamen-Jiulongjiang, QZ: Qinzhou, YRE: Yangtze river estuary. The arrowed curves indicate coastal currents in the summer in China.
Taxonomic Analyses of Sequence Variations of the COI Gene Segment in Wild Estuarine Oysters
DNA Isolation
Gills of the 278 collected wild oysters from 11 estuaries, as well as those from five C. hongkongensis oysters as the outgroup, were sampled for sequence variation detection. DNA of individuals from each oyster population was extracted using a TIANamp Marine Animals DNA Kit (Tiangen, Beijing, China). The quality and quantity of the DNA were determined by agarose gel (1%) electrophoresis and UV spectrometry on a NanoDrop 2000 (Thermo Fisher Scientific, Waltham, MA, United States), respectively.
High-Resolution Melting (HRM) Analysis
An improved small-amplicon HRM analysis (Wang et al., 2015) was adopted to characterize the COI gene segment of all sampled individuals. The forward and reverse primer sequences (5′-3′) were the same as those in a previous study (Wang et al., 2014): TACTTAATATTGGGTTTTTAGGGT and CGCGTATCAATATCCATTCC, respectively. The amplicon length was 76 bp, and the melting temperature (Tm) was set at 55°C. The PCR mixture contained 10 ng of template DNA, 5 μL of PCR mix, 0.5 μL (100 pmol/L) each of the forward and reverse primers, and water to 10 μL, which was covered with 15 μL of mineral oil. The COI gene fragment was amplified using the following protocol: an initial denaturing at 94°C for 5 min, followed by 55 cycles of denaturing at 95°C for 30 s, annealing at 55°C for 30 s, and extension at 72°C for 30 s, and a final extension at 72°C for 10 min.
Fluorescent melting curves of PCR amplicon duplexes were analyzed using a Light Scanner 96 (Idaho Technology Inc., Salt Lake City, UT, United States). Two unblocked, double-stranded oligonucleotides were used as the high- and low-temperature internal controls in the experiment to calibrate the temperature variation between reactions (Gundry et al., 2008). The duplex controls consisted of the following sequences and their complements: GCGGTCAGTC GGCCTAGCGGTAGCCAGCTGCGGCACTGCGTGACGCTC AG. (high-temperature sequence) and ATCGTGATTTCTATA GTTATCTAAGTAGTTGGCATTAATAATTTCATTTT (low-temperature sequence). Specifically, 1 μL (100 pmol) of the internal controls and 1 μL of LC-green were added to the amplification products, and denaturation was performed at 95°C for 10 min using a thermal cycler prior to HRM analysis. Melting curve data were collected using continuous fluorescence acquisition at 55–98°C at a thermal transition rate of 0.1°C/s. Genotypes were identified by the melting temperatures indicated by peaks on the derived plots using the Light Scanner 96 software.
Sequence Variation Determination
We randomly selected 10 individuals from each of the northern (BZ) and southern (TS) wild oyster populations to sequence the COI gene segment using Sanger sequencing based on the observation of their different melting curves (Figure 2A).
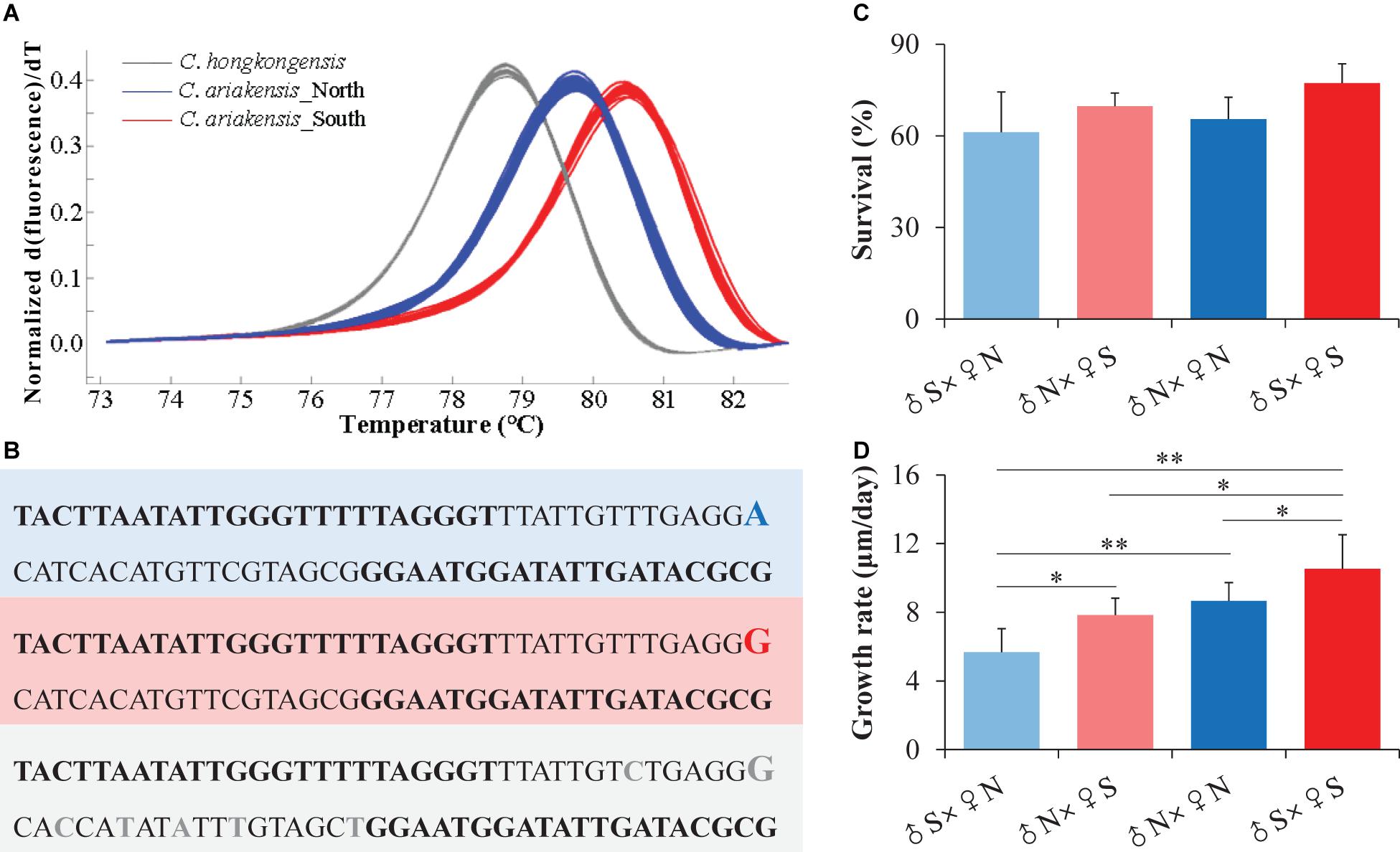
Figure 2. Taxonomic analyses of the northern and southern estuarine Crassostrea ariakensis oysters. (A) Normalized melting curves of the amplicons from the COI gene segment for the northern (blue) and southern (red) C. ariakensis, and Crassostrea hongkongensis (gray) oysters. (B) Sequence variations of the COI gene segment among the northern (blue) and southern (red) C. ariakensis, and C. hongkongensis (gray) oysters. (C) Survival and (D) growth rates during larvae development from the D-shaped to eye-spot stages of the four full-sib families. ♂S: sperm of the southern oysters (TS), ♀S: eggs of the southern oysters (TS), ♀N: sperm of the northern oysters (BZ), ♀N: eggs of the northern oysters (BZ). Asterisks indicate significant differences (*p < 0.05 and **p < 0.01), and error bars represent sd values.
Cross-Breeding Experiments
We further explored the classification of the southern and northern estuarine oysters by constructing an intrapopulation and their hybrid families of reciprocal crosses in the southern habitat using sperm (♂) and eggs (♀) from the wild northern (N,BZ) and southern (S,TS) parental oysters. The gamete was microscopically examined to filter out hermaphrodites before breeding. Each of the four families was incubated with three replicates in 70 L plastic containers. Larvae and spats were reared using standard practices with temperature and salinity ranges of 22–26°C and 20 ± 1.5‰, respectively. The shell length of the larvae was measured at the D-shaped stage and the density was controlled at 10 ind./mL for each family. At the eye-spot stage (occurrence time of the first family), the shell length and density of the larvae of the four families were further determined to compare their survival and growth rates.
Reciprocal Transplantation Experiments for Fitness Trait Measurements
Two populations derived from the southern (TS) and northern (BZ) environments were selected to reveal the responses of fitness-related traits to each of the natural habitats. To alleviate environmental and maternal effects (Sanford and Kelly, 2011; Somero, 2012; Li L. et al., 2018). Wild oysters from two populations were collected from the estuary and then translocated to identical conditions for a one-month acclimation before breeding. For each population, hermaphrodites were excluded by microscopic examination, and 40 mature male and female oysters were selected to maintain an effective population size. Eggs from the female oysters were mixed and equally divided into 40 beakers. Sperm from the 40 male oysters were individually crossed with each piece of mixed eggs. This breeding protocol warranted each sperm to cross with eggs from different female oysters. The zygotes were individually cultured in 70 L plastic containers until the D-shaped stage, and then larvae were combined into one nursery pond. The culturing conditions for the larvae and spat was the same as those for the cross-breeding experiments described in section “Cross-breeding Experiments.”
Reciprocal transplantation was conducted as follows: two-month-old juvenile individuals from each of two populations were outplanted to two source habitats to compare the responses of their fitness-related traits, including survival, respiration rate, and growth parameters (wet weight and shell height, length, and width), after acclimation for three months from July to October. Oysters of each population were cultured with three replicates in cages with the same density at both locations. Live oysters were counted to determine the survival. After cleaning off the attached barnacles, mussels, and other aufwuchs, approximately 100 oysters were used to measure growth parameters, and six oysters were used to measure respiration rate.
Oysters used for the respiration rate measurements were further cleaned by wiping with 50% alcohol on the shell to avoid the influence of epiphytes. Individual oysters were placed in 1.2 L acrylic chambers filled with air-saturated, sand-filtered seawater at ambient temperature controlled by a water bath (20°C). A rotating magnetic stir bar was used to slowly circulate the seawater beneath the experimental chamber. A needle-type fiber-optic oxygen microsensor (oxygen optode) and a temperature probe (PreSens, Regensburg, Germany) were glued into two small holes in the lid. An oxygen transmitter (Microx 4; PreSens) was connected to these two probes and the temperature and oxygen concentration were recorded every 3 s for 1 h. The oxygen microsensors were calibrated to the corresponding temperature and salinity conditions prior to each trial according to the manufacturer’s instructions. The slope of the decrease in oxygen concentration was calculated as the respiration rate after correcting the wet weight (% as g–1 L–1 h–1).
Semi-Lethal Temperature (LT50) and Salinity (LS50) Determination and Stress Responses of the Northern and Southern Oysters
Ten-month-old F1 progeny of the southern (TS) and northern (BZ) oysters cultured in their native habitat were used to determine LT50 after heat shock and LS50 after high salinity in the laboratory. Acute short-term exposure to thermal conditions (1 h) and a one-week recovery time were applied in the LT50 determination, while long-term exposure under high salinity gradients was applied in the LS50 determination. Twenty individuals were used in each treatment gradient. Based on previous determinations of LT50 for northern Crassostrea gigas and southern Crassostrea angulata (Ghaffari et al., 2019), and a preliminary experiment was performed to narrow the range of treatment gradients; the survival of oysters at 30, 35, 40, and 45°C and 50, 55, 60, and 65‰ were explored. The second part of the experiment with a narrower treatment range was deployed to precisely assess the LT50 and LS50 of each population. An increase of 1°C from 34°C to 44°C and 2‰ from 50‰ to 64‰ were performed. A trail of oysters that were not stressed was set as a control (temperature [average seawater temperature in the northern habitats during experiment]: 26 ± 1°C; salinity [average seawater salinity in the southern habitats during experiment]: 17 ± 2‰). Stressed oysters were fed with commercial spirulina powder each day, and the seawater was changed every two days. The dead oysters, who could not close the shell after touch, were removed from the tanks.
Ten-month-old southern and northern F1 progenies cultured in the northern habitat were used to compare stress responses under the LT50 of the southern population and the LS50 of the northern population in the laboratory, considering that the northern oysters cannot survive in the southern habitat (Figure 3). The same stress methods that caused acute heat shock followed recovery and long-term high salinity exposure were applied. Each treatment had three replicates and experimental management was the same as that for LT50 and LS50 determination.
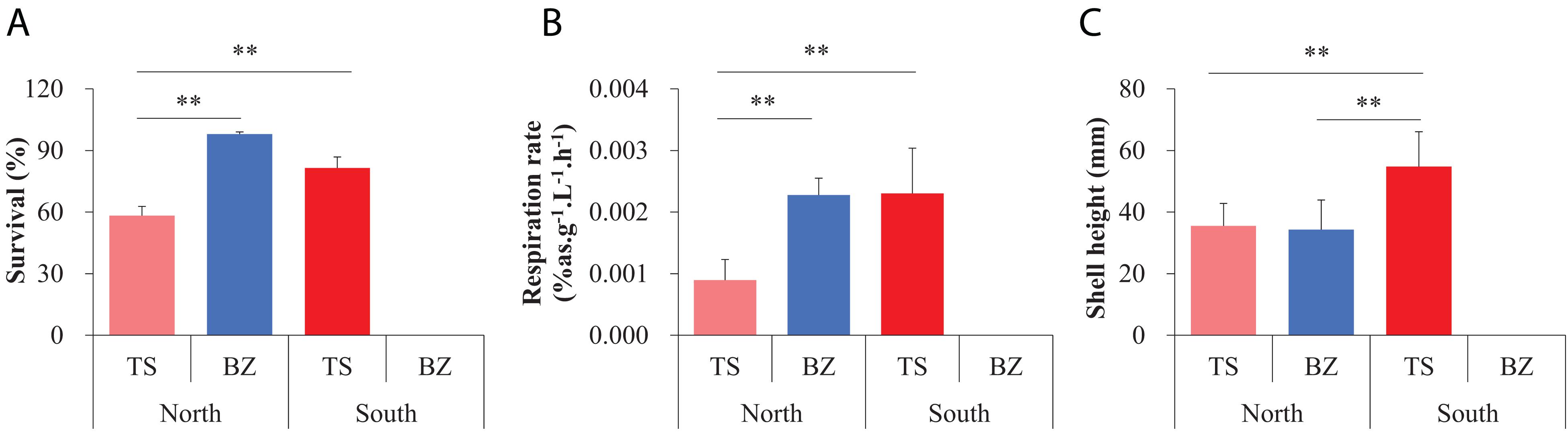
Figure 3. Measurements for fitness-related traits, including (A) survival, (B) respiration rate, and (C) shell height, of the northern and southern estuarine oyster populations in the northern and southern habitats using reciprocal transplant experiments for three months post attachment. Asterisks indicate significant differences (**p < 0.01), and error bars represent sd values.
Statistical Analysis
Shapiro–Wilk and Bartlett tests were used to check the normality and homogeneity of the variances, respectively. Comparisons of the temperature and salinity between two sampling sites were tested with the function aov in R software if the data followed a normal distribution and homoscedasticity of variances. Otherwise, a non-parametric Kruskal–Wallis test was performed. Two-way ANOVA was carried out followed by Bonferroni post hoc test to determine the differences in survival and growth rates of the larvae from four crosses, growth parameters and metabolic rates of the F1 progenies among populations cultured in the southern and northern natural habitats, while significant differences in survival at the field for these populations were determined by Pearson’s chi-square test. Polynomial (three order) regressions were used to model LT50 and LS50. The Kaplan-Meier analysis with log-rank test in the Survival module using SPSS version 19.0 software (IBM SPSS Statistics) was carried out to determine the difference in mortality between the two populations when exposed to LT50 and LS50. All data are presented as mean ± standard error of mean (SEM), and a significance level of α = 0.05 was applied.
Results
Monitoring Seawater Temperature and Salinity in the Southern and Northern Habitats
We observed tremendous environmental differences in the seawater temperature and salinity between the southern and northern field habitats during summer after larval attachment (p < 0.01) (July to October; Figures 1B,C). The southern estuary showed a higher seawater temperature, while the northern estuary showed a higher salinity, and the differences in the average seawater temperature (northern: 26.83°C; southern: 30.12°C) and salinity (northern: 28.17%; southern: 17.19%) between the northern and southern habitats were 3.29°C and 10.98%, respectively. In addition, the amplitude of the temperature fluctuation in the northern habitat was 16.25°C, while it was 7.52°C in the southern habitat (Figure 1B). Furthermore, the amplitude of the salinity fluctuation in the southern habitat was 2.33 times higher than that in the northern habitat (Figure 1C).
Taxonomic Analyses of the Sequence Variation in the COI Gene Segments and Cross-Breeding Experiments
The PCR amplicon melting curves could distinguish all of the C. ariakensis individuals as well as the C. hongkongensis oysters, which showed three independent homozygote peaks, and the peaks of the southern and northern C. ariakensis oyster groups were separated according to their different melting temperatures (Figure 2A). Two populations inhabiting the northern (NT) and southern (SH) estuaries of the Yangtze River showed the same melting curves that were consistent with the other five northern oyster populations (DD, YK, BZ, DY, and QD), while four southern oyster populations (XM-ZJ, XM-JLJ, TS, and QZ) were clustered together (Figure 1A and Supplementary Figure 1). We only identified an SNP as A to G between the northern and southern individuals of C. ariakensis in this COI gene segment using Sanger sequencing. In accordance with the southern C. ariakensis oysters, the same G in this locus was detected in C. hongkongensis, but was accompanied by six other SNPs in comparison with only one in C. ariakensis (Figure 2B).
There were no differences in survival during larval development from the D-shaped to eye-spot stages among the four full-sib families derived from BZ (N: northern), TS (S: southern), and their reciprocal cross oysters (p > 0.05, two-way ANOVA, Figure 2C, Supplementary Table 2a). However, the growth rates exhibited significant difference among these four families and interaction between sex and the origin of oysters (p < 0.05, Figure 2D, Supplementary Table 2b), where larvae reproduced from the sperm of the southern oysters and the eggs of the northern oysters (♂S × ♀N) showed the lowest growth rate (5.67 μm/day) compared to those of the larvae reproduced from the sperm of the northern oysters and the eggs of the southern oysters (♂N × ♀S) (7.83 μm/day, p = 0.034, t-test), the northern family (♂N × ♀N) (8.67 μm/day, p = 0.012), and the southern family (♂S × ♀S) (10.54 μm/day, p = 0.010).
Comparison of Fitness-Related Traits Between the Northern and Southern F1 Oyster Populations Using Reciprocal Transplant Experiments
Both the northern (BZ) and southern (TS) F1 oyster populations exhibited significantly greater performance in fitness-related traits in their native environments, including survival, growth, and metabolic rate, and statistically significant interaction between sampling sites and the origin of oysters using reciprocal transplant experiments for three months post-attachment (Figure 3, Supplementary Table 2c,d). The survival and respiration rates of the northern oysters (97.96%, 0.0024% as g–1 L–1 h–1) were significantly higher than those of the southern oysters (58.27%, 0.00090% as g–1 L–1 h–1, p < 0.01) in the northern habitats, while the northern oysters could not survive in the southern habitat. Southern oysters showed higher survival and respiration rates in their native environment (81.51%, 0.0023% as g–1 L–1 h–1) than in the northern habitat (p < 0.01) (Figures 3A,B). The shell height of the southern oysters in the southern habitats (54.77 mm) was significantly greater than that of their counterparts (35.52 mm, p < 0.01) and the northern oysters (34.42 mm, p < 0.01) in the northern habitats, and no difference was detected between the two populations in the northern habitat (p = 0.32) (Figure 3C). In addition, other growth parameters, including shell length, shell width, and wet weight, exhibited consistent differentiation patterns (Supplementary Figure 2).
Responses to High Salinity and Heat Stress in the Northern and Southern F1 Oyster Populations
Preliminary experiments to evaluate the thermal tolerance limits indicated that 100% survival and mortality occurred at temperatures of 35°C and 45°C, respectively, in both oyster populations, and the tolerance limits of high salinity for 100% survival and mortality occurred at salinities of 50% and 65%, respectively (Supplementary Figure 3). Narrower stress ranges were used to determine LT50 and LS50 values. The survival data were significantly fit to third-order regressions for both oyster populations (p < 0.01, Table 1). Both oyster populations died at 43°C. There was no mortality at 35°C in the northern population or at 37°C in the southern population. Regression models predicted the LT50 to be 40.32°C for the northern oysters and 41.11°C for the southern oysters (Figure 4A). Southern oysters showed 100% survival and mortality at a salinity of 52% and 66%, respectively, while no mortality was observed at 54% for northern oysters. Regression models predicted the LS50 to be 62.09% for northern oysters and 59.66% for southern oysters (Figure 4B). For F1 progenies of both oyster populations acclimated in the northern habitats, the southern oysters (45.0%) exhibited a significantly higher survival than their northern counterparts (29.2%) when exposed to the temperature of LT50 (p < 0.05), while the northern oysters (49.3%) exhibited a significantly higher survival than their southern counterparts (23.9%) when exposed to the salinity of LT50 (p < 0.01) (Figure 4C).

Table 1. LT50, LS50, and third order regression equations for the northern (BZ) and southern (TS) oysters cultured in their native habitat when exposed to higher temperature and salinity.
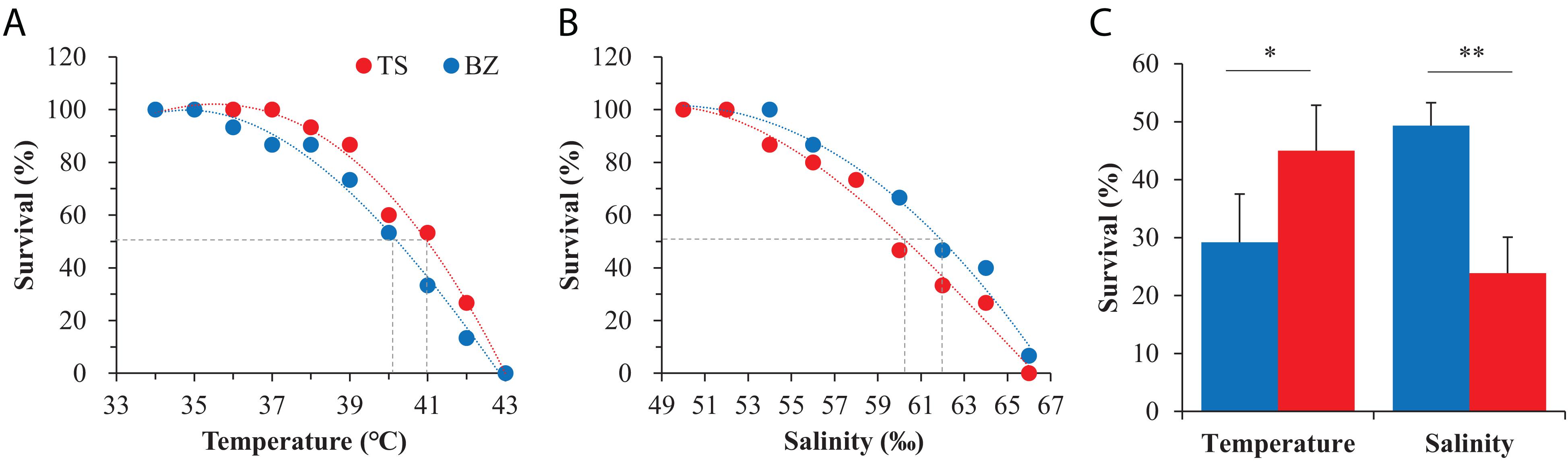
Figure 4. Responses to heat shock and high salinity of the northern (BZ, blue) and southern (TS, red) oysters. (A) LT50 and (B) LS50 determinations for the northern and southern F1 oysters in their native habitats when exposed to high temperature and salinity gradients, respectively. (C) Survival of the northern and southern F1 progeny after exposure to the LT50 of the southern population and the LS50 of the northern population acclimated to the northern environment.
Discussion
We collected 11 oyster populations of C. ariakensis throughout estuaries in China, ranging from the Bohai Sea and Yellow Sea in the north to the East and South China Seas in the south, which represent their natural distribution in China (Zhou and Allen, 2003; Wang et al., 2004). Phylogenetic analysis using HRM with an SNP of the neutral COI marker can clearly reveal genetic variation between northern and southern oyster populations whose melting curves are genetically distinctive. HRM analysis of the specific SNP in the mitochondrial COI gene segment can not only identify five common Crassostrea oysters in China (Wang et al., 2014), but can also distinguish between northern and southern populations of C. ariakensis. Moreover, compared to electrophoresis, this simple, fast, and high-throughput method could be applied to reliably and efficiently identify the existent natural hybrids from the unidirectional cross between C. hongkongensis eggs and C. ariakensis sperm (Zhang et al., 2017; Qin et al., 2020). This method is easy to visualize and can be extensively applied to studies of genetic diversity in C. ariakensis. Our findings were consistent with those of previous molecular taxonomic studies which found that C. ariakensis from northern China, Korea, and Japan were more closely related, but did not share a common haplotype with the southern populations using polymorphic microsatellite markers, mitochondrial COI and 16S rRNA sequence variation, and nuclear ITS-1 locus (Wang et al., 2004; Zhang et al., 2005; Xiao et al., 2010; Kim et al., 2014). The molecular data indicate that biogeographic barriers, such as the Yangtze River estuary, exist and isolate the northern and southern C. ariakensis populations. The Yangtze River estuary is known to be a notable barrier for the distribution of many marine invertebrates with lower gene flow, such as two oyster congeners of C. gigas and C. angulata (Xu, 1997; Li et al., 2017), an interpopulation of limpet (Cellana toreuma) (Dong et al., 2012) and the bivalve Cyclina sinensis (Ni et al., 2012, 2017). Even marine species exhibiting high gene flow with high mobility or a long-term larval stage showed a distinctive genetic structure between the northern and southern coasts of the Yangtze River (Xu et al., 2009; Xiao et al., 2016). In addition, in line with a previous study (Xiao et al., 2010), oysters inhabiting the Yangtze River estuary were clustered with their northern counterparts. We propose that the interaction between coastal currents (Li L. et al., 2018) and the Yangtze River estuary potentially contributes in shaping the distribution of C. ariakensis in China.
Hybridization experiments between the northern and southern populations of C. ariakensis provide initial evidence to reject the hypothesis of reproductive isolation, and they should not be considered as two species (Wang et al., 2004; Zhang et al., 2005). Whether they should be considered two subspecies requires genomic variations in future studies to classify their taxonomic status by comparing the sequence variation between intra- and inter-specific oysters (Wang et al., 2010). The lower survival and growth rates of larvae bred from reciprocal hybrid crosses between the northern and southern populations than in those from intrapopulation crosses indicate that there is no intraspecific heterosis, which is concordant with interspecific reciprocal crosses between high-salt habit C. gigas and low-salt habit C. ariakensis in that their hybrids had lower fitness (Yao et al., 2015). For species distributed along similar environmental (salinity) gradients, however, significant interspecific heterosis was detected between C. ariakensis and C. hongkongensis (Qin et al., 2020). We propose that severe environmental variations (especially for temperature and salinity) and distant geographic distance strongly contribute to genetic incompatibility and subsequently influence the potential heterosis. In addition, environmental and maternal effects (Sanford and Kelly, 2011; Somero, 2012) showed that larvae bred from the eggs of southern oysters had higher fitness of survival and growth, which was also revealed in the hybrids crossed from C. angulata eggs and C. gigas sperms cultured in southern environments (Tan et al., 2020).
We found a strong signature of local adaptation based on the observations of adaptive differentiations of fitness-related phenotypes between the northern and southern populations. Oysters from the northern estuaries cannot survive in southern environments, and the survival, metabolic rate, and growth for both populations showed greater performance in their native habitats than in their translocated non-native habitats. These findings provide critical evidence for adaptive divergence between the northern and southern C. ariakensis in China, which supports previous observations of their differentiation using limited neutral markers (Wang et al., 2004; Zhang et al., 2005; Xiao et al., 2010; Kim et al., 2014). Adaptive divergence in these fitness-related traits as well as in physiological and molecular parameters was also found in two congeneric oyster species, C. gigas and C. angulata, which are naturally distributed along the northern and southern coasts of the Yangtze River, respectively (Wang et al., 2010; Beck et al., 2011), using the same experimental approaches by combining common garden and reciprocal transplantation (Li et al., 2017, 2018, 2019).
We detected greater climate gradients in both temperature and salinity between the monitored northern and southern locations; the northern locations had lower temperatures but higher salinity. We propose that the tremendous environmental variations resulting from these two fundamental factors have profound effects on adaptive capacity and shape the distribution of northern and southern estuarine oysters (Bozinovic et al., 2011; Sanford and Kelly, 2011; Somero, 2012; Nadeau et al., 2017). Correspondingly, estuarine oysters dwelling in the northern habitat evolved higher tolerance to high salinity (2.43‰), while their southern counterparts evolved higher thermotolerance (0.79°C). The results were in accordance with the general cognition that stress tolerance of organisms is highly related to the conditions of their microhabitats (Sanford and Kelly, 2011; Somero, 2012). Higher thermal tolerance for species/populations inhabiting southern or warmer environments has been pervasively observed in mussels, oysters, corals, and other marine species (Tomanek and Zuzow, 2010; Somero, 2012; Kenkel et al., 2013; Ghaffari et al., 2019). Therefore, we address whether the developmental stage and ambient environmental conditions can severely influence stress tolerance of marine species. The LT50 of immature animals of northern C. gigas and southern C. angulata was 2°C higher than that of mature oysters of northern and southern populations of C. ariakensis, respectively (Ghaffari et al., 2019), although the paired latitude and geographic distance and oceanographic conditions were similar. In addition, oysters derived from high-salinity environments evolved higher LS50, which was consistent with our previous findings in eye-spot larvae using transcriptomic analysis (Liu et al., 2019). The higher survival rate of the F1 progenies from southern oysters under heat shock and from northern oysters under high salinity after strong natural selection in northern environments indicated an evolved genetic basis for their higher stress tolerance. Further research focusing on comparative genomic analysis is needed to reveal the underlying adaptive mechanisms.
Regulation of energy metabolism is one of the critical responses of marine species for survival in challenging environments (Sokolova et al., 2012; Schulte, 2015). We noticed that both the northern and southern oyster populations had lower respiration rates in the translocated habitats than those in their native habitats. This finding indicates the protective role of metabolic repression of estuarine oysters as a physiological mechanism to increase their survivorship in response to non-native challenging environments, which is in agreement with the reduced metabolic index of other marine species suffering from global warming (Deutsch et al., 2015) as well as the calorie restriction found in other stressed animals (Hebert et al., 2013; Vermeij et al., 2016). However, in contrast with the present results, our previous study found that the northern populations of Pacific oysters increase their metabolic rate to reach optimum fitness levels when translocated to the southern part of the environment (Li L. et al., 2018). We speculate that strong natural selection and intense biogeographic gradients compelled the estuarine oysters to decrease their metabolism in non-native habitats. Furthermore, the estuarine oysters grew slower in non-native habitats, suggesting energy allocation from growth to environmental stress tolerance, which is a pervasive adaptive strategy for adaptation to various changing climates in many marine species by trade-offs among different fitness-related traits (Sokolova et al., 2012; Han et al., 2013; Sussarellu et al., 2016; Li et al., 2017, Li L. et al., 2018). Integrative works focusing on physiological, molecular, and genomic regulations of energy allocation need to be investigated in future studies.
Data Availability Statement
The original contributions presented in the study are included in the article/Supplementary Materials, further inquiries can be directed to the corresponding author.
Author Contributions
LL and GZ conceived the study. AL carried out the field and laboratory work, participated in the data analysis, and drafted the manuscript. CW contributed to the field work and taxonomic analyses. AL, WW, ZS, ZJ, and LL collected the oyster samples and produced the F1 progeny. AL, CW, WW, ZZ, ML, ZJ, and ZS contributed to experimental management at the North and South sites. AL, LL, and GZ revised the manuscript. All authors approved the manuscript for publication.
Funding
This work was supported by the Strategic Priority Research Program of the Chinese Academy of Sciences (No. XDA23050402 to LL) and National Key R&D Program of China (No. 2018YFD0900304 to LL), China Postdoctoral Science Foundation (No. 2019TQ0324 to AL), Key Deployment Project of Centre for Ocean Mega-Research of Science, Chinese Academy of Sciences (No. COMS2019Q06 to AL and LL), Distinguished Young Scientists Research Fund of the Key Laboratory of Experimental Marine Biology, Chinese Academy of Sciences (No. KLEMB-DYS04 to AL), Technology and Modern Agro-Industry Technology Research System (No. CARS-49 to LL), and Guangxi Key Laboratory of Beibu Gulf Marine Biodiversity Conservation (No. 2020ZB04 to ZJ).
Conflict of Interest
The authors declare that the research was conducted in the absence of any commercial or financial relationships that could be construed as a potential conflict of interest.
Acknowledgments
The authors would like to thank Xuegang Wang and Zemin Zhao for their support and assistance in oyster breeding, and Zhen Jia and Yeshao Peng for cultural management and sample collection at the southern site.
Supplementary Material
The Supplementary Material for this article can be found online at: https://www.frontiersin.org/articles/10.3389/fmars.2020.589099/full#supplementary-material
References
Beck, M. W., Brumbaugh, R. D., Airoldi, L., Carranza, A., Coen, L. D., Crawford, C., et al. (2011). Oyster reefs at risk and recommendations for conservation, restoration, and management. BioScience 61, 107–116. doi: 10.1525/bio.2011.61.2.5
Bozinovic, F., Calosi, P., and Spicer, J. I. (2011). Physiological correlates of geographic range in animals. Annu. Rev. Ecol. Evol. Syst. 42, 155–179. doi: 10.1146/annurev-ecolsys-102710-145055
Burford, M. O., Scarpa, J., Cook, B. J., and Hare, M. P. (2014). Local adaptation of a marine invertebrate with a high dispersal potential: evidence from a reciprocal transplant experiment of the eastern oyster Crassostrea virginica. Mar. Ecol. Prog. Ser. 505, 161–175. doi: 10.3354/meps10796
Dayan, D. I., Crawford, D. L., and Oleksiak, M. F. (2015). Phenotypic plasticity in gene expression contributes to divergence of locally adapted populations of Fundulus heteroclitus. Mol. Ecol. 24, 3345–3359. doi: 10.1111/mec.13188
De Wit, P., and Palumbi, S. R. (2013). Transcriptome-wide polymorphisms of red abalone (Haliotis rufescens) reveal patterns of gene flow and local adaptation. Mol. Ecol. 22, 2884–2897. doi: 10.1111/mec.12081
Deutsch, C., Ferrel, A., Seibel, B., Pörtner, H.-O., and Huey, R. B. (2015). Climate change tightens a metabolic constraint on marine habitats. Science 348, 1132–1135. doi: 10.1126/science.aaa1605
Dong, Y., Wang, H., Han, G., Ke, C., Zhan, X., Nakano, T., et al. (2012). the impact of yangtze river discharge, ocean currents and historical events on the biogeographic pattern of Cellana toreuma along the China Coast. PLoS One 7:e36178. doi: 10.1371/journal.pone.0036178.g001
Ghaffari, H., Wang, W., Li, A., Zhang, G., and Li, L. (2019). Thermotolerance divergence revealed by the physiological and molecular responses in two oyster subspecies of Crassostrea gigas in China. Front. Physiol. 10:1137. doi: 10.3389/fphys.2019.01137
Gundry, C. N., Dobrowolski, S. F., Martin, Y. R., Robbins, T. C., Nay, L. M., Boyd, N., et al. (2008). Base-pair neutral homozygotes can be discriminated by calibrated high-resolution melting of small amplicons. Nucleic Acids Res. 36, 3401–3408. doi: 10.1093/nar/gkn204
Guo, X., Li, C., Wang, H., and Xu, Z. (2018). Diversity and evolution of living oysters. J. Shellfish Res. 37, 755–771. doi: 10.2983/035.037.0407
Guo, X. M., Ford, S. E., and Zhang, F. S. (1999). Molluscan aquaculture in China. J. Shellfish Res. 18, 19–31.
Han, G. D., Zhang, S., Marshall, D. J., Ke, C. H., and Dong, Y. W. (2013). Metabolic energy sensors (AMPK and SIRT1), protein carbonylation and cardiac failure as biomarkers of thermal stress in an intertidal limpet: linking energetic allocation with environmental temperature during aerial emersion. J. Exp. Biol. 216(Pt 17), 3273–3282. doi: 10.1242/jeb.084269
Hebert, A. S., Dittenhafer-Reed, K. E., Yu, W., Bailey, D. J., Selen, E. S., Boersma, M. D., et al. (2013). Calorie restriction and SIRT3 trigger global reprogramming of the mitochondrial protein acetylome. Mol. Cell 49, 186–199. doi: 10.1016/j.molcel.2012.10.024
Kenkel, C. D., Meyer, E., and Matz, M. V. (2013). Gene expression under chronic heat stress in populations of the mustard hill coral (Porites astreoides) from different thermal environments. Mol. Ecol. 22, 4322–4334. doi: 10.1111/mec.12390
Kim, W.-J., Dammannagoda, S. T., Jung, H., Baek, I. S., Yoon, H. S., and Choi, S. D. (2014). Mitochondrial DNA sequence analysis from multiple gene fragments reveals genetic heterogeneity of Crassostrea ariakensis in East Asia. Genes Genomics 36, 611–624. doi: 10.1007/s13258-014-0198-5
Li, A., Li, L., Song, K., Wang, W., and Zhang, G. (2017). Temperature, energy metabolism, and adaptive divergence in two oyster subspecies. Ecol. Evol. 7, 6151–6162. doi: 10.1002/ece3.3085
Li, A., Li, L., Wang, W., Song, K., and Zhang, G. (2018). Transcriptomics and fitness data reveal adaptive plasticity of thermal tolerance in oysters inhabiting different tidal zones. Front. Physiol. 9:825. doi: 10.3389/fphys.2018.00825
Li, L., Li, A., Song, K., Meng, J., Guo, X., Li, S., et al. (2018). Divergence and plasticity shape adaptive potential of the Pacific oyster. Nat. Ecol. Evol. 2, 1751–1760. doi: 10.1038/s41559-018-0668-2
Li, A., Li, L., Wang, W., and Zhang, G. (2019). Evolutionary trade-offs between baseline and plastic gene expression in two congeneric oyster species. Biol. Lett. 15:20190202. doi: 10.1098/rsbl.2019.0202
Limborg, M. T., Helyar, S. J., Bruyn, M. D., Martin, I. T., Einar, E. N., Rob, O., et al. (2012). Environmental selection on transcriptome-derived SNPs in a high gene flow marine fish, the Atlantic herring (Clupea harengus). Mol. Ecol. 21, 3686–3703.
Liu, X., Li, L., Li, A., Li, Y., Wang, W., and Zhang, G. (2019). Transcriptome and gene coexpression network analyses of two wild populations provides insight into the high-salinity adaptation mechanisms of Crassostrea ariakensis. Mar. Biotechnol. 21, 596–612. doi: 10.1007/s10126-019-09896-9
Nadeau, C. P., Urban, M. C., and Bridle, J. R. (2017). Climates past, present, and yet-to-come shape climate change vulnerabilities. Trends Ecol. Evol. 32, 786–800. doi: 10.1016/j.tree.2017.07.012
Ni, G., Kern, E., Dong, Y. W., Li, Q., and Park, J. K. (2017). More than meets the eye: the barrier effect of the Yangtze River outflow. Mol. Ecol. 26, 4591–4602. doi: 10.1111/mec.14235
Ni, G., Li, Q., Kong, L., and Zheng, X. D. (2012). Phylogeography of bivalve Cyclina sinensis: testing the historical glaciations and Changjiang River outflow hypotheses in northwestern Pacific. PLoS One 7:e49487. doi: 10.1371/journal.pone.0049487.g001
Qin, Y. P., Li, X. Y., Noor, Z., Li, J., Zhou, Z. H., Ma, H. T., et al. (2020). A comparative analysis of the growth, survival and reproduction of Crassostrea hongkongensis. Crassostrea ariakensis, and their diploid and triploid hybrids. Aquaculture 520:734946.
Sanford, E., and Kelly, M. W. (2011). Local adaptation in marine invertebrates. Ann. Rev. Mar. Sci. 3, 509–535. doi: 10.1146/annurev-marine-120709-142756
Schulte, P. M. (2015). The effects of temperature on aerobic metabolism: towards a mechanistic understanding of the responses of ectotherms to a changing environment. J. Exp. Biol. 218(Pt 12), 1856–1866. doi: 10.1242/jeb.118851
Sokolova, I. M., Frederich, M., Bagwe, R., Lannig, G., and Sukhotin, A. A. (2012). Energy homeostasis as an integrative tool for assessing limits of environmental stress tolerance in aquatic invertebrates. Mar. Environ. Res. 79, 1–15. doi: 10.1016/j.marenvres.2012.04.003
Somero, G. N. (2012). The physiology of global change: linking patterns to mechanisms. Ann. Rev. Mar. Sci. 4, 39–61. doi: 10.1146/annurev-marine-120710-100935
Sussarellu, R., Suquet, M., Thomas, Y., Lambert, C., Fabioux, C., Pernet, M. E., et al. (2016). Oyster reproduction is affected by exposure to polystyrene microplastics. Proc. Natl. Acad. Sci. U.S.A. 113, 2430–2435. doi: 10.1073/pnas.1519019113
Tan, K., Liu, H., Ye, T., Ma, H., Li, S., and Zheng, H. (2020). Growth, survival and lipid composition of Crassostrea gigas, C. angulata and their reciprocal hybrids cultured in southern China. Aquaculture 516:734524. doi: 10.1016/j.aquaculture.2019.734524
Tomanek, L., and Zuzow, M. J. (2010). The proteomic response of the mussel congeners Mytilus galloprovincialis and M. trossulus to acute heat stress: implications for thermal tolerance limits and metabolic costs of thermal stress. J. Exp. Biol. 213(Pt 20), 3559–3574. doi: 10.1242/jeb.041228
Vermeij, W. P., Dolle, M. E., Reiling, E., Jaarsma, D., Payan-Gomez, C., Bombardieri, C. R., et al. (2016). Restricted diet delays accelerated ageing and genomic stress in DNA-repair-deficient mice. Nature 537, 427–431. doi: 10.1038/nature19329
Wang, H., and Guo, X. (2008). Identification of Crassostrea ariakensis and related oysters by multiplex species-specific PCR. J. Shellfish Res. 27, 481–487.
Wang, H., Guo, X., Zhang, G., and Zhang, F. (2004). Classification of jinjiang oysters Crassostrea rivularis (Gould, 1861) from China, based on morphology and phylogenetic analysis. Aquaculture 242, 137–155. doi: 10.1016/j.aquaculture.2004.09.014
Wang, H., Qian, L., Liu, X., Zhang, G., and Guo, X. (2010). Classification of a common cupped oyster from southern China. J. Shellfish Res. 29, 857–866. doi: 10.2983/035.029.0420
Wang, H., Qian, L., Zhang, G., Liu, X., Wang, A., Shi, Y., et al. (2006). Distribution of Crassostrea ariakensis in China. J. Shellfish Res. 25, 789–790.
Wang, H., Zhang, G., Liu, X., and Guo, X. (2008). Classification of Common Oysters from North China. J. Shellfish Res. 27, 495–503.
Wang, J., Qi, H., Li, L., Que, H., Wang, D., and Zhang, G. (2015). Discovery and validation of genic single nucleotide polymorphisms in the Pacific oyster Crassostrea gigas. Mol. Ecol. Resour. 15, 123–135. doi: 10.1111/1755-0998.12278
Wang, J., Xu, F., Li, L., and Zhang, G. (2014). A new identification method for five species of oysters in genus Crassostrea from China based on high-resolution melting analysis. Chin. J. Oceanol. Limnol. 32, 419–425. doi: 10.1007/s00343-014-3124-4
Xiao, J., Cordes, J. F., Wang, H., Guo, X., and Reece, K. S. (2010). Population genetics of Crassostrea ariakensis in Asia inferred from microsatellite markers. Mar. Biol. 157, 1767–1781. doi: 10.1007/s00227-010-1449-x
Xiao, Y., Ma, D., Dai, M., Liu, Q., Xiao, Z., Li, J., et al. (2016). The impact of Yangtze River discharge on the genetic structure of a population of the rock bream, Oplegnathus fasciatus. Mar. Biol. Res. 12, 426–434. doi: 10.1080/17451000.2016.1154576
Xu, J., Chan, T. Y., Tsang, L. M., and Chu, K. H. (2009). Phylogeography of the mitten crab Eriocheir sensu stricto in East Asia: pleistocene isolation, population expansion and secondary contact. Mol. Phylogenet. Evol. 52, 45–56. doi: 10.1016/j.ympev.2009.02.007
Yao, T., Zhang, Y., Yan, X., Wang, Z., Li, D., Su, J., et al. (2015). Interspecific hybridization between Crassostrea angulata and C. ariakensis. J. Ocean Univ. China 14, 710–716. doi: 10.1007/s11802-015-2546-8
Zhang, Q., Allen, S. K. Jr., and Reece, K. S. (2005). Genetic variation in wild and hatchery stocks of Suminoe Oyster (Crassostrea ariakensis) assessed by PCR-RFLP and microsatellite markers. Mar. Biotechnol. 7, 588–599. doi: 10.1007/s10126-004-5105-7
Zhang, Y., Zhang, Y., Li, J., and Yu, Z. (2017). Morphological and molecular evidence of hybridization events between two congeneric oysters, Crassostrea hongkongensis and C. ariakensis, in southern China. J. Molluscan Stud. 83, 129–131. doi: 10.1093/mollus/eyw035
Keywords: environmental selection, genetic drift, genetic variation, local adaptation, taxonomic analyses, reciprocal hybridization, Crassostrea ariakensis
Citation: Li A, Wang C, Wang W, Zhang Z, Liu M, She Z, Jia Z, Zhang G and Li L (2020) Molecular and Fitness Data Reveal Local Adaptation of Southern and Northern Estuarine Oysters (Crassostrea ariakensis). Front. Mar. Sci. 7:589099. doi: 10.3389/fmars.2020.589099
Received: 30 July 2020; Accepted: 15 October 2020;
Published: 03 November 2020.
Edited by:
Marcos Rubal, University of Porto, PortugalReviewed by:
Zhang Yuehuan, South China Sea Institute of Oceanology (CAS), ChinaCristián E. Hernández, University of Concepcion, Chile
Yang Zhang, South China Sea Institute of Oceanology (CAS), China
Copyright © 2020 Li, Wang, Wang, Zhang, Liu, She, Jia, Zhang and Li. This is an open-access article distributed under the terms of the Creative Commons Attribution License (CC BY). The use, distribution or reproduction in other forums is permitted, provided the original author(s) and the copyright owner(s) are credited and that the original publication in this journal is cited, in accordance with accepted academic practice. No use, distribution or reproduction is permitted which does not comply with these terms.
*Correspondence: Li Li, bGlsaUBxZGlvLmFjLmNu
†These authors have contributed equally to this work