- 1Department of Environmental Science and Management, Portland State University, Portland, OR, United States
- 2Department of Fisheries, Quinault Indian Nation, Taholah, WA, United States
- 3Department of Fisheries and Wildlife, Coastal Oregon Marine Experiment Station, Oregon State University, Corvallis, OR, United States
Microplastics (MPs) are anthropogenic contaminants found in coastal and marine environments worldwide. Pacific razor clams (Siliqua patula), important for local indigenous culture, economy, gastronomy and food security along the United States West Coast, are subjected to myriad environmental stressors including predation, storm events, disease, toxins, and MPs. This study aimed to determine MP burdens in Olympic Coast, Washington Pacific razor clams and estimate annual MP exposure of recreational razor clam harvester-consumers from eating this species. We quantified suspected MP burdens in Pacific razor clams collected from eight tribal, recreational, and commercial harvest areas on the Olympic Coast in April 2018. We administered questionnaires to 107 recreational razor clam harvesters during the same timeframe to determine consumption patterns, preparation styles, knowledge and concerns about MPs, and demographics. Razor clams containing suspected MPs, primarily microfibers, were found at all eight sites. Average suspected MP burden differed by sample type (whole = 6.75 ± 0.60, gut-tissue = 7.88 ± 0.71, non-gut tissue = 4.96 ± 0.56, and cleaned samples = 3.44 ± 0.25). FTIR analyses of a random subset of microfiber-type MPs in whole and cleaned clams indicated material types of polyethylene terephthalate, cellulose acetate, cellophane, polyester, nylon, and cellulose. The average number of razor clams consumed per meal by Olympic Coast recreational razor clam harvesters was 4.27 ± 0.27, which varied by gender and ethnicity, but not income or age. Harvesters ate 0–209 meals/year of razor clams (16.2% harvested but did not eat razor clams), and most respondents (88.3%) fully cleaned razor clams before consuming them. Annual suspected MP exposure for razor clam harvester-consumers was 60–3,070 for cleaned and 120–6,020 for whole clams. Our findings suggest Olympic Coast recreational razor clam harvester-consumers are exposed to low levels of MPs from eating razor clams. MP exposure can be reduced by roughly 50% if clams are cleaned before consumption. Our work serves as an important reference in the growing portfolio of Pacific Northwest microplastic research, to inform future MP attenuation recommendations and development of human health standards for this type of pollution.
Introduction
Coastal organisms are subjected to a myriad of environmental stressors ranging from the predictable, such as changing tides, predation (e.g., by sea otters, fish, birds, and whales), and seasonal food availability, to the less predictable including storm events, disease, toxins, and environmental contaminants. Though numerous definitions exist, microplastics (MPs)–polymers between 1 μm and 5 mm in size (Frias and Nash, 2019)– represent a diverse contaminant suite (Rochman et al., 2019). These particles, either manufactured at this size or formed as breakdown products of larger plastic items, make their way to coastal environments via household gray water, wastewater treatment plant effluent, stormwater, rivers, atmospheric deposition and other means (e.g., Napper and Thompson, 2016; Lebreton et al., 2017; Mintenig et al., 2017; Zhang, 2017; Zhang Q. et al., 2020; Zhang Y. et al., 2020). Present in coastal and marine environments worldwide, plastics are incorporated into all environmental compartments (air, water, sediment, sand, soil, biota) and affect roughly 700 known species to date– a number that continues to grow with the addition of new research (Provencher et al., 2017). MPs have been found in a variety of edible seafood species globally (e.g., Van Cauwenberghe and Janssen, 2014; Li et al., 2015; Rochman et al., 2015; Davidson and Dudas, 2016; Lusher et al., 2017; Phuong et al., 2018), suggesting that marine predators and even human consumers of these species are exposed to MPs; however there are also myriad other non-seafood human consumables known to contain MPs including drinking water (e.g., Eerkes-Medrano et al., 2018; Tong et al., 2020), table salt (e.g., Kim et al., 2018; Cho et al., 2019; Lee et al., 2019), milk (Kutralam-Muniasamy et al., 2020), honey (Liebezeit and Liebezeit, 2013), beer (Liebezeit and Liebezeit, 2014), and packaged meats (Kedzierski et al., 2020). With trophic transfer of MPs previously demonstrated in laboratory settings (Farrell and Nelson, 2013; Nelms et al., 2018), there is little doubt that MPs are now inextricably incorporated into marine food webs and the human diet (Barboza et al., 2018; Carbery et al., 2018).
The Pacific Razor clam (Siliqua patula) is found on intertidal beaches along the Pacific coast of North America from Alaska to southern California (Link, 2000). On the Olympic Peninsula, Washington (WA), United States which supports the largest population of harvest-sized individuals within the species’ range, razor clams rely on the rich coastal waters to settle and grow (Huppert and Trainer, 2014). This historically important species is a key traditional food and cultural resource, and serves as a vital form of income for members of the Quinault Indian Nation and other Olympic Coast peoples (Crosman et al., 2019).
While there is a distinctive lack of data on the prevalence and effects of MPs in commercially important North American fishery species (Baechler et al., 2020b), recent research indicates that Pacific Northwest bivalves from less developed coastal areas are not immune to MP contamination (Davidson and Dudas, 2016; Covernton et al., 2019; Baechler et al., 2020a; Martinelli et al., 2020). Determining the prevalence of MPs in organisms from varied geographies provides context for environmental concentrations, bioavailability to marine species and potential risk to human consumers. Investigating MP prevalence in marine and seafood species from relatively undeveloped coastal areas contributes to our understanding of the range of environmental and human exposure to MPs through seafood consumption in areas of relatively low human impact. MPs become incorporated into bivalves through ingestion or adherence to soft tissues (e.g., de Witte et al., 2014; Mathalon and Hill, 2014; Kolandhasamy et al., 2018; Abidli et al., 2019; Teng et al., 2019), with potentially negative physiological impacts including increased respiration, altered reproduction and feeding, inflammation, neurotoxicity, and decreased energy reserves (e.g., von Moos et al., 2012; Avio et al., 2015; Sussarellu et al., 2016; Ribeiro et al., 2017; Xu et al., 2017; Bour et al., 2018).
We initiated this study on the sparsely populated Olympic Coast, WA, United States. to determine: (1) microplastic burdens in Pacific razor clams from the Olympic Coast; (2) annual human microplastic exposure ranges from Olympic Coast razor clam consumption based on clam preparation styles, consumption frequency and consumer demographics, and (3) level of knowledge and concerns Olympic coast razor clam harvesters have regarding microplastic exposure from seafood.
We expected MPs would be present in Olympic Coast razor clams, as has been found in other filter-feeding bivalves (clams, oysters) in the region (e.g., Davidson and Dudas, 2016; Covernton et al., 2019; Baechler et al., 2020b; Martinelli et al., 2020) and in a number of clam species globally (e.g., Li et al., 2015; Su et al., 2018; Abidli et al., 2019; Sparks, 2020), but that MP burdens would be highest at northernmost sites due to outflow from Puget Sound and the Salish Sea– waterbodies adjacent to the populous Seattle metropolitan area abutting the Olympic Peninsula. This hypothesis was founded upon the assumptions that densely populated areas can lead to high concentrations of MPs in the coastal marine environment, due to land-based inputs (Barnes et al., 2009; Hantoro et al., 2019), and that waterborne MP concentrations partially dictate bivalve MP burdens (Qu et al., 2018). We predicted Puget Sound and the Salish Sea would transport substantial waterborne MPs to the Olympic Coast, based on both existing hydrographic models of water movement from the highly populated Salish Sea region to the sparsely populated northern Olympic Coast (Pirhalla et al., 2009) and MP transport in coastal littoral drift cells- pockets of water (and associated particulates or contaminants) of varying energy levels that move alongshore (Black et al., 2018; Horn et al., 2019).
We hypothesized annual Olympic Coast razor clam harvester-consumer MP exposure would vary depending on clam preparation style, ethnicity, and frequency of consumption. This hypothesis was based on the knowledge that some ethnicities consume more seafood per capita than the general population, resulting in greater exposure to seafood-associated contaminants (Sechena et al., 1999; Mahaffey et al., 2009; EPA, 2014; Cisneros-Montemayor et al., 2016). Lastly, we hypothesized that razor clam harvesters would possess varying knowledge and concerns about MPs in seafood.
Materials and Methods
Study Area Context
“When the tide is out, the table is set” is a common saying on the Olympic Peninsula, the rugged coastal region which forms the western border of WA, United States (Charles et al., 2004). In this ecologically productive area, as in others across North America, harvest of the nearshore seafood bounty by Native Americans has occurred since time immemorial and is significantly integrated into local indigenous culture, economy, gastronomy, and food security (Olson, 1936; Charles et al., 2004; Anderson and Parker, 2009; Lepofsky et al., 2015).
The Olympic Coast is a region of low human presence relative to other coastal areas in North America. It is sparsely populated by about 195,000 people at a density of 12 people/km2 (30 people/mile2) in coastal Jefferson, Clallam, Grays Harbor, and Pacific counties (U.S. Census Bureau, 2012), with a very low proportion of developed lands compared to other land use types based on 2010 data (Supplementary Appendix 1; NOAA, 2020). For comparison, in the United States, average coastal population density (excluding Alaska) was 446 people/mile2 in 2010 (NOAA Office for Coastal Management, 2020). 2010 land cover data for the four coastal Olympic Peninsula counties (Clallam, Jefferson, Grays Harbor, Pacific) show forests are the most dominant land type, followed by scrublands (NOAA, 2020; Supplementary Appendix 1). While the west coast of the United States has the state with the highest coastal-dwelling population (California–26.5 million people), the east and gulf coasts have states with the second through fifth highest coastal populations (New York–16 million people; Florida–15.3 million people; New Jersey–7.1 million people; Texas–6.6 million people), providing evidence that coastal living is prized nationwide (Crossett et al., 2013; NOAA Office for Coastal Management, 2020). Unfortunately, land development, particularly near densely populated coastlines, can impact coastal ecology through environmental degradation such as loss of habitat for native species, erosion, and pollution in various forms (nutrient, chemical, noise, light, plastic).
The allure of the razor clam attracts thousands of visitors to the Olympic Coast’s sandy shores annually, as they try their luck at clamming during one or more recreational fishery openers. The recreational fishery injects what are usually sleepy coastal towns with visitors, filling hotels and restaurants to capacity, and bringing millions of dollars annually into coastal community economies. Roughly 250,000 clam digger trips are logged in this region each year (Huppert and Trainer, 2014).
Razor clams are co-managed in WA by both the Department of Fish and Wildlife (WDFW) and by tribal governments (Wyer, 2013). Tribal (ceremonial, subsistence and commercial) and state (recreational and commercial) fisheries have unique rules for who can participate and where harvest can occur. The Quinault Indian Nation is the sole manager and harvester of razor clams on beaches within their reservation boundaries (Figure 1). The Quinault Nation’s treaty rights extend to beaches north and south of their reservation, within their legally defined “usual and accustomed fishing grounds” (U&A), where they co-manage razor clams with WDFW and other tribes. Fifty percent of total allowable harvest is reserved per treaty between the United States and the Quinault people on U&A areas off-reservation (shared with the Hoh Tribe on Kalaloch Beach to the north), with the remainder available for recreational harvest in the state-managed fishery (Anderson, 1999). Commercial and subsistence razor clam harvest off-reservation is regulated by Quinault Indian Nation for tribal members at Copalis, Mocrocks, and Kalaloch beaches. Recreational harvest is permitted by WDFW on set harvest dates, and is open to anyone with a WA shellfish harvest permit (Crosman et al., 2019).
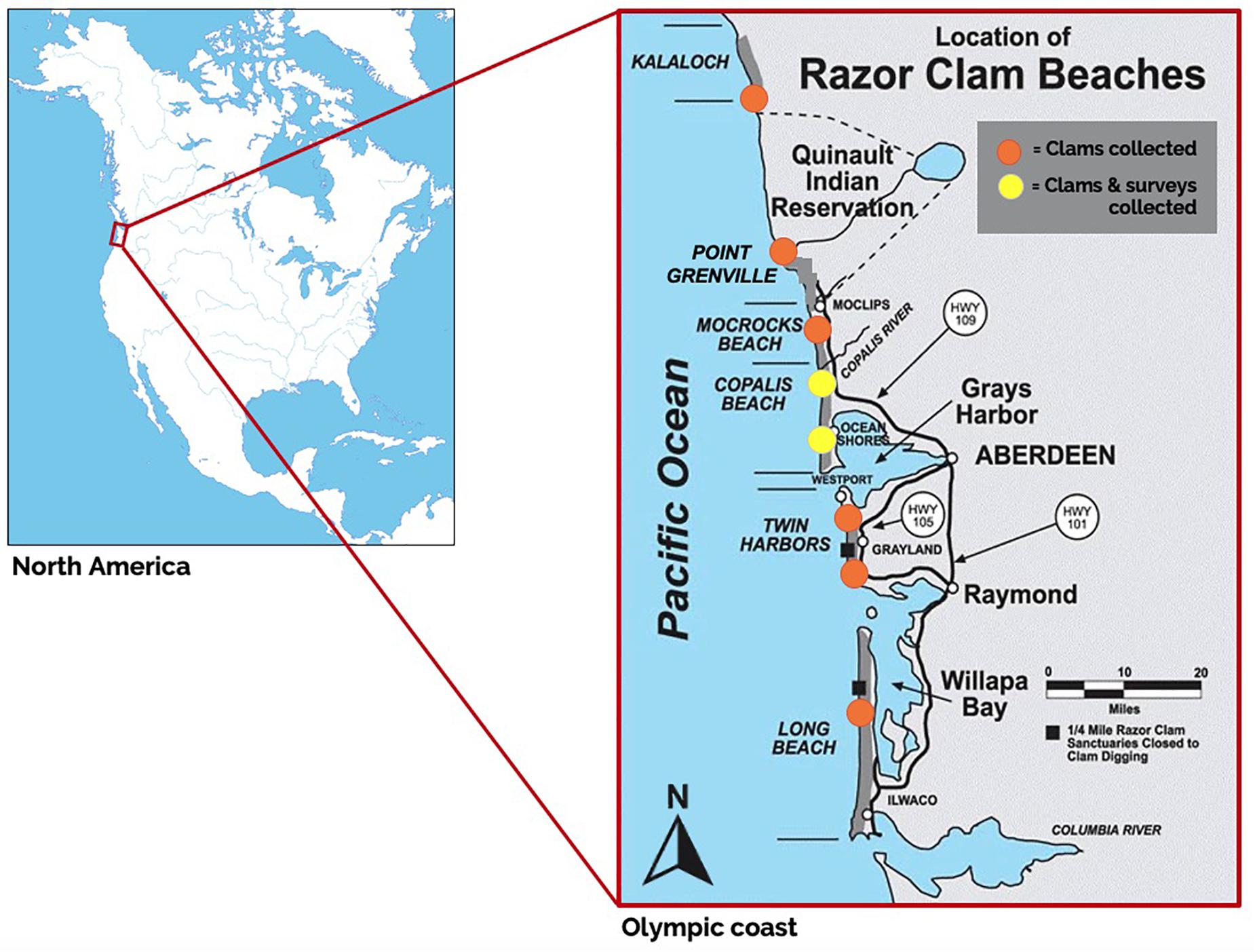
Figure 1. Modified publicly available map of recreational and commercial razor clam beaches on the Olympic Peninsula, Washington, with razor clam collection sites (orange circles) and sites where both razor clams were collected and clam harvester surveys were conducted (yellow circles) in April 2018. Sample beaches included (from North to South): Kalaloch beach, Point Grenville beach (within the Quinault Indian Reservation), Mocrocks Beach, Copalis Beach, Twin Harbors and Long Beach. Original map source: http://wdfw.wa.gov/fishing/shellfish/razorclams/.
Microplastic Prevalence in Study Species
Sample Sites and Collection
Olympic Peninsula clams were collected from eight coastal beaches (Figure 1; Kalaloch, Point Grenville, Mocrocks, Copalis, South Copalis, North Twin Harbor, South Twin Harbor, and Long Beach) in April 2018 to quantify types and burdens of MPs in their tissues. Fifteen clams were collected at each of the eight sites, except at Mocrocks beach where only fourteen were collected due to challenging harvest conditions. Razor clams sampled averaged 69.90 ± 2.63 g wet body weight per individual (range = 4.66–167.02 g); therefore, on average, roughly 1.05 kg of razor clam tissue was collected per site (except at Mocrocks beach due to only fourteen clams being collected). Clams were not selected by size; rather, the first fifteen clams obtained per site were retained for analysis. Clams were transported in clean, covered 2L glass mason jars on ice to the Applied Coastal Ecology lab at Portland State University in Portland, Oregon (OR), where the exteriors of the organisms were rinsed with filtered deionized (DI) water filtered to 0.2 μm (Shelco MicroVantage WGPS02–20S7S213) and biological measurements (shell length in mm, body tissue weight in g) were collected. Individual organisms were processed as either whole (n = 7 individuals for each site), gut-tissue separated (n = 3 individuals for each site, divided into three gut-only and three tissue-only samples), or cleaned samples (n = 5 per site). “Whole” organisms were minimally processed except for shells being rinsed, to mimic MP burdens if the clam was consumed by an animal predator. “Gut-tissue” samples (stomach, intestine, and crystalline style) were separated from “non-gut tissue” samples (all remaining tissue; Table 1). “Cleaned” samples were prepared as if to be eaten by a human consumer; in order to remove gritty sand and other debris inside of or stuck to the clam, the tip of the siphon was removed, and both the incurrent and excurrent siphons were split using cleaned stainless steel scissors and rinsed thoroughly under a running filtered DI water tap. The clam foot was also split and thoroughly rinsed under a running filtered DI water tap. Digestive organs and gills were removed using cleaned stainless steel scissors (Table 1). Each sample was rinsed into individual cleaned and labeled mason jars and frozen at −20°C (Table 1). Of the 138 clam samples analyzed in this study, 52 were whole, with others separated into gut and tissue samples (“gut-tissue”; n = 24, and “non-gut tissue,” n = 23, respectively), or fully cleaned (“cleaned”; n = 39).
Sample Processing
Samples were thawed and digested individually using the protocol described in Baechler et al. (2020a). In brief, samples were individually chemically digested using a 10% potassium hydroxide (KOH) solution, then underwent a second 10% KOH digestion combined with hypersaline density separation (330 g/L Fisher Chemical Certified ACS Crystalline NaCl) and were visually analyzed for MPs under a Leica M165C stereomicroscope (10–120× magnification). Each suspected MP encountered was photographed with a Leica IC80 HD camera connected to a computer running Leica Application Suite X imaging software. Particle category (bead, fiber, fiber bundle, film, fragment, foam, unknown), color, and maximum length were recorded. All clam sample types (whole, gut-tissue, non-gut tissue, cleaned) underwent identical procedures during collection, transport, digestion and microscope analysis. In a randomly selected subset of approximately 10% of suspected MPs (53 total) extracted from whole and cleaned clams, material types were determined using a Thermo Nicolet is 20 Fourier-transform infrared spectrometer (FTIR) and iN5 microscope fitted with an Attenuated Total Reflectance accessory and germanium tip running at 128–512 scans in the Brander laboratory at Oregon State University. Spectral outputs were compared to FTIR spectral libraries (Omnic polymer database, Primpke et al., 2018, 2020), with a 70% minimum match threshold to determine a quality spectrum and material type. Material type was determined by the match with the highest percentage.
Quality Assurance/Quality Control
Microplastics and other anthropogenic fibers are airborne, waterborne, and present on surfaces, and are therefore inadvertently introduced to samples during laboratory processing or analysis. These anthropogenic fibers–MPs or otherwise–labeled “contamination,” are important to quantify, as they provide context for final particle counts. To minimize contamination, clams were immediately rinsed with filtered DI water and placed in cleaned, rinsed and covered 2L mason jars after field collection. One hundred percent cotton clothing and lab coats, as well as nitrile gloves were worn at all times in the laboratory during sample processing, chemical digestions, and microscope analyses. Nitrile gloves were changed after every sample, after handling tools or touching surfaces. Glassware and tools were all rinsed three times with filtered DI water between uses, and glassware was covered with cleaned watch glasses at all times. To quantify contamination, two control types (procedural and microscope blanks) were used to provide a snapshot of anthropogenic fibers inadvertently introduced into clam samples during laboratory processing and analysis (Brander et al., 2020). Procedural controls were samples of 50 ml filtered DI water run through the same chemical digestion and sieving steps as other sample types; microscope blanks were glass petri dishes filled with filtered DI water placed on the microscope base and left open to the air during visual inspection of samples for MPs (Baechler et al., 2020a; Supplementary Appendix 2). One procedural control was processed during each of the 4 weeks of laboratory chemical digestions (n = 4), and one microscope blank was processed per sample immediately following visual microscope analysis of that sample (n = 145).
Calculations and Statistical Analyses
Microplastics in this study refers to post-digestion visually identified fibers and few particles suspected to be plastics, a subset (10%) of which were validated using FTIR; while the validated subset was randomly selected, we cannot be certain of the material types or origins for all MPs in this study. To identify any differences in MP burden between sample sites or between sample types (whole, gut-only, tissue-only, and cleaned) analysis of variance (ANOVA) and post-hoc Tukey tests were conducted. Variables including the number of MPs per sample and per gram of tissue (MP g–1 tissue) were square root transformed to normalize data prior to conducting statistical analyses. Linear regression models were used to examine relationships between biological parameters (shell length, body tissue wet weight) and MP burdens (per whole individual and g–1 tissue) and were expressed as number of MPs per sample or g–1 tissue (wet weight; whole organisms only). The statistical cutoff (alpha) for all tests was 0.05 with standard error (SE) reported.
Razor Clam Harvester Questionnaire
Questionnaire Sites and Protocol
Written questionnaires were administered to recreational razor clam harvesters 18 years of age or older after concluding clamming activities at Mocrocks and Copalis beaches on April 21–22, 2018 (Figure 1). Frequencies of razor clam consumption, preparation type, number of individual razor clams consumed per meal, and demographics of respondents were determined using these questionnaires (Supplementary Appendix 3). Estimates of recreational razor clam harvester MP exposure were calculated based on self-reported razor clam consumption frequencies. While not central to research questions, data on consumption of other non-razor clam marine seafood species were also collected to provide further context to study results (Supplementary Appendix 4).
The questionnaire surveying effort occurred during state-managed recreational fishery openings on 2 days in April 2018 and not during tribal commercial or subsistence fishery opening dates. Thus, local tribes (Quinault, Quileute, Hoh, and others) were poorly represented in the survey. Questionnaires were administered within 2 h of low tide each day, with surveys beginning at 10:00am (low tide at 11:38am) and 11:00am (low tide at 12:30pm) on April 21 and 22, respectively. Three teams of two surveyors dispersed along the beach to cover as large an area as possible. Surveyors monitored the shoreline for every sixth clam harvester party that visually indicated they had completed clamming activities (i.e., turning their backs to the shore and walking toward the vehicle line carrying clam bag, shovel and any other equipment or personal effects). Surveyors intercepted a clam harvesting party, provided a brief description of the research project and asked if a representative of the party would be willing to complete a questionnaire. Upon agreement, they were provided a clipboard with the questionnaire and a pencil attached. Respondents wrote in answers themselves unless they requested for surveyors to write as they dictated. Responses were at-will (not required) except the first question indicating the respondent was 18 years of age or older (Supplementary Appendix 3); therefore, not all respondents answered every question.
MP Exposure Calculations and Statistical Analyses
Microplastic exposure ranges of Olympic Coast razor clam harvester-consumers from razor clam consumption were calculated by multiplying minimum and maximum positive consumptive responses (i.e., smallest non-zero consumption frequency) per season (winter, spring, summer, fall) by average suspected MP burden for whole or cleaned clams across all eight sample sites. These exposure ranges include a combination of MPs and fibers of unknown origins, based on FTIR results for randomly selected fibers in whole and cleaned clam samples. Seasonal totals were combined to generate MP exposure estimates. For our purposes, each season was represented evenly in 3-month (13.05 week) blocks.
Questionnaire data were plotted using Microsoft Excel and the R-studio statistical program (v1.2.1335). ANOVA, post-hoc Tukey tests, and Welch’s t-tests were run using the aov, tukey test and t-test functions in R-studio (R Core Team, 2020) to identify differences in MP exposure among demographic groups based on gender, age, income, ethnicity or education level. Standard error (SE; alpha = 0.05) was reported with averages. Razor clam consumption by ethnicity was reported but not analyzed for statistical differences due to small sample size for some ethnicities; instead, a Welch’s t-test was performed to elucidate differences in razor clam consumption between White/Caucasian and all other ethnic groups. For the single open-ended questionnaire item “Please expand on what concerns you have about MPs in your food,” responses were categorized into seven themes based on the short, written responses provided (Table 3).
Results
Biological Measurements
Razor clams averaged 115.71 ± 1.29 mm shell length (range = 63.91–156.76 mm) and 69.90 ± 2.63 grams (g) wet body weight (range = 4.66–167.02 g), as the first 15 clams harvested per site were retained regardless of size. Average clam size was similar for all sites except for the northernmost Kalaloch beach, where clams were significantly smaller than other sites for both shell length (avg = 102.85 ± 2.25 mm; range = 81.38–114.88 mm; F7,43 = 4.00, p = 0.002) and wet body weight (avg = 32.45 ± 2.12 g; range = 15.37–45.10 g; F7,44 = 7.60, p < 0.0001).
Microplastic Characteristics and Identification
Suspected MPs were found in razor clams at all eight sample sites. In total, 799 suspected MPs were found in the 138 clam samples collected during this study. Over 99% of suspected MPs encountered were microfibers (n = 793) with an average length of 1.33 ± 0.04 mm (range = 0.11–7.84 mm; Supplementary Appendix 5). Other categories represented were: unknown (n = 3), fiber bundles (n = 2), and fragments (n = 1). Colorless, blue, gray, and black were the most commonly observed colors at 55.19% (n = 441), 19.27% (n = 154), 12.27% (n = 98), and 4.63% (n = 37), respectively (Supplementary Appendix 6). FTIR analysis of 53 randomly selected suspected MPs (10.83% of suspected MPs encountered in whole clams and 10.45% of suspected MPs encountered in cleaned clams) yielded reliable matches for 52 fibers and verified that a total of 80.77% of the 52 fibers with matches were either synthetic (48.08%, n = 25), including polyethylene terephthalate (PET) (n = 21), polyester (n = 3), and nylon (n = 1), or semi-synthetic (32.69%; n = 17), including cellulose acetate (n = 11) and cellophane (n = 6; Supplementary Appendix 7,8). Cellulose, a naturally derived material, was also identified (n = 10; 19.23%). One additional suspected MP was analyzed but its spectral match did not meet the 70% minimum threshold; material type for that fiber was unconfirmed and reported as “low spectral match.” The average match used for identification was 86.6% ± 1.0%.
Microplastics Prevalence
Whole clams and gut-tissue samples contained more MPs per sample than cleaned clams (p = 0.0004 and p < 0.0001, respectively), and gut-tissue samples contained significantly more MP than non-gut tissue (p = 0.017; Figure 2). Across all sites, average whole clam suspected MP burden was 6.75 ± 0.60 (range = 0–20, n = 52; Figure 3A). For gut-tissue, non-gut tissue, and cleaned samples, average MP burdens across all sites were 7.88 ± 0.71 (n = 24; range = 3–16), 4.96 ± 0.56 (n = 23; range = 0–12), and 3.44 ± 0.25 (n = 39; range = 0–6), respectively. When comparing the number of MPs in all four sample types (Table 1) across all sites, significant differences were identified (F3,134 = 9.858, p < 0.0001). For whole clams, average MP g–1 burden was 0.15 ± 0.03 (range = 0–1.17, n = 52; Figure 3B). MP g–1 tissue differed by site, with higher MP g–1 tissue found at the northernmost Kalaloch site than all other sites (F7,44 = 7.171, p < 0.0001). Site-specific differences in MP g–1 tissue were identified between Kalaloch and: Copalis (p = 0.0002), Long Beach (p < 0.0001), Mocrocks (p = 0.002), North Twin Harbor (p = 0.003), South Copalis (p < 0.0001), South Twin Harbor (p = 0.0006), and Point Grenville (p = 0.01; Figures 3A,B). In contrast to differences in MP g–1 tissue, the number of MPs per clam differed minimally across sites (F7,44 = 1.892, p = 0.09). Number of MPs did not differ among sites for gut-tissue (F7,16 = 1.095, p = 0.41) or non-gut-tissue samples (F7,15 = 1.076, p = 0.42), but did for cleaned clams (F7,31 = 3.020, p = 0.02) for two site pairings: Copalis and South Copalis (p = 0.01) and South Copalis and North Twin Harbor (p = 0.04). Neither clam shell length (in mm; F1,49 = 0.941, p = 0.34) nor body weight (g wet weight; F1,50 = 0.010, p = 0.92) correlated with the number of MPs per whole organism.
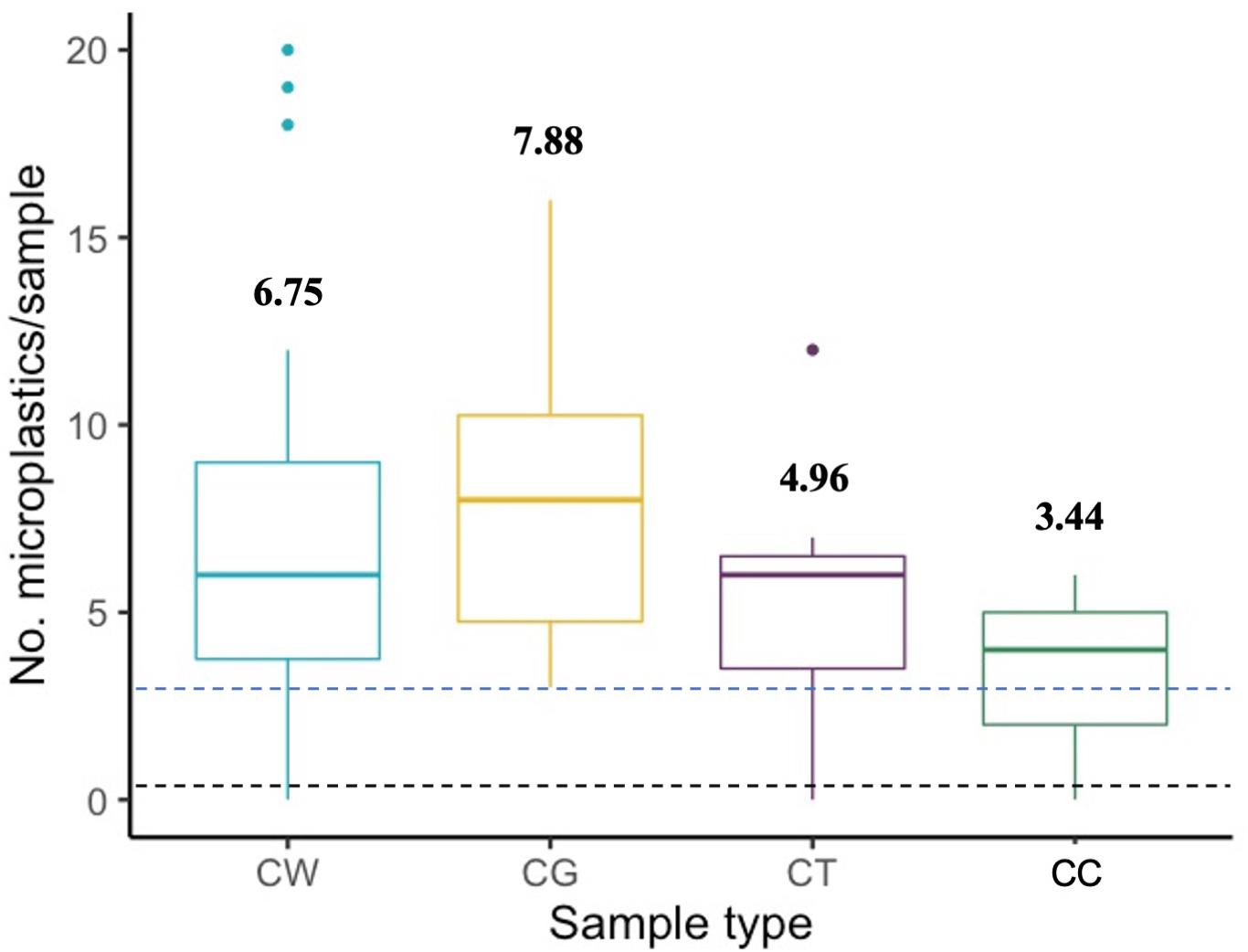
Figure 2. Number of microplastics (MPs) by sample type. CW = whole clam (n = 52); CG = gut-tissue only (n = 24); CT = tissue-only (n = 23); CC = cleaned clam (n = 39). No. = number. Black hashed line indicates average microscope blank contamination (Avg = 0.23 MPs/sample; n = 145). Blue hashed line indicates average procedural control contamination (Avg = 3.25 MPs/sample; n = 4). Bold numbers above boxes indicate average number of MPs per sample type.
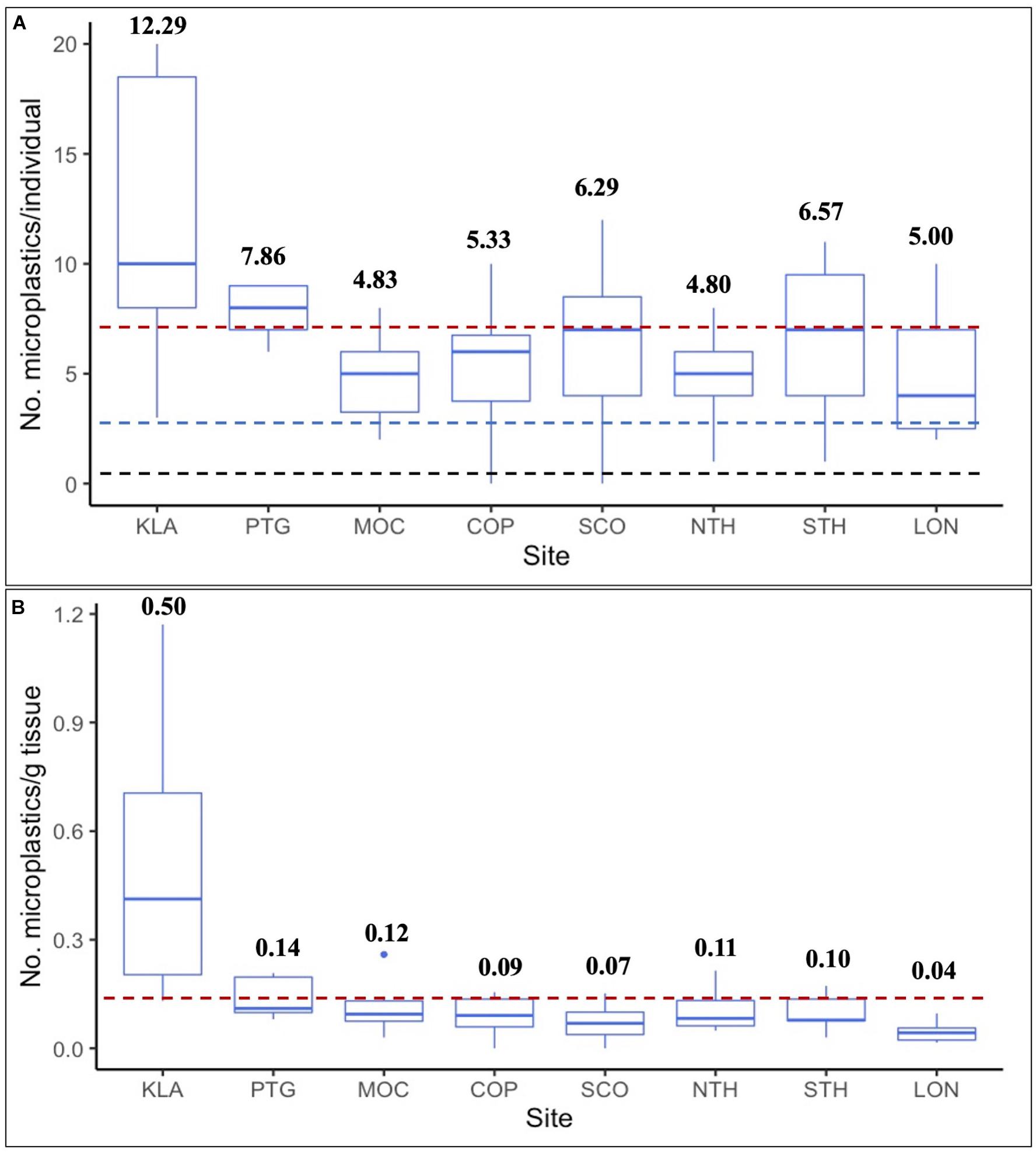
Figure 3. (A,B) Number of microplastics (MPs)- (A) Per individual whole razor clam, with red line indicating average number of MPs for all eight sites (Avg = 6.75 MPs/individual), black hashed line indicating average microscope blank contamination (Avg = 0.23 MPs/sample; n = 145), and blue hashed line indicating average procedural control contamination (Avg = 3.25 MPs/sample; n = 4); and (B) Per gram of tissue (wet weight), with red line indicating average number of MPs per gram of tissue (wet weight) for all eight sites (Avg = 0.15 MP g– 1 tissue). Bold numbers above boxes indicate average number of MPs per sample type. No. = number. Sites are arranged north to south by latitude. KLA = Kalaloch beach (n = 7), PTG = Point Grenville (within the Quinault Indian Reservation boundary; n = 7), MOC = Mocrocks Beach (n = 6), COP = Copalis Beach (n = 6), SCO = South Copalis Beach (n = 7), NTH = North Twin Harbor (n = 5), STH = South Twin Harbor (n = 7), LON = Long Beach (n = 7).
Quantifying Microplastic Contamination
In total, 46 suspected MPs were identified as contamination from microscope blanks (n = 145) and procedural controls (n = 4). On average, suspected MP contamination in microscope blanks was 0.23 ± 0.05 (range = 0–2), and in procedural controls was 3.25 ± 0.85 (range = 1–5; Figure 2). Average MP length detected as contamination was 1.60 ± 0.15 mm (range: 0.12–3.6 mm). One hundred percent (100%) of suspected MPs in microscope blanks and procedural controls were microfibers and were colorless (77.8%; n = 35), blue (15.6%; n = 7), black (4.4%; n = 2), or gray (2.2%; n = 1). Neither microscope blank nor procedural control MPs was subtracted from clam MP burdens because procedural controls were low in number and were intended to identify a range of contamination during laboratory processing, with microscope blanks showing a range of contamination during microscope analyses. Reported MP burdens in the four clam sample types may be lower than reported given ambient contamination found in microscope blank and procedural control samples; therefore MP burdens reported herein may be higher than would be expected in the environment.
Questionnaire Respondent Demographics
The 2-day survey effort at Mocrocks and Copalis beaches yielded 107 questionnaires from recreational razor clam harvesters representing an array of ethnicities, incomes, educational backgrounds, and ages. Questionnaire response rate was 94.7%, as 6 of the 113 individuals asked to participate declined. Respondents were 56.2% male, 43.8% female (Figure 4A), and lived in 14 Washington counties including Benton (n = 3), Chelan (n = 1), Clallam (n = 2), Clark (n = 4), Franklin (n = 1), Grays Harbor (n = 6), King (n = 24), Kitsap (n = 5), Lewis (n = 3), Mason (n = 6), Pierce (n = 17), Skagit (n = 3), Snohomish (n = 12), and Thurston (n = 15). None of the 107 respondents dug razor clams to sell commercially in April 2017–2018. Roughly 96% (n = 103) of questionnaire respondents reported their ethnicities. The majority of respondents (79.6%) were White/Caucasian, and the remaining (20.4%) were Asian, mixed race, Native American, Native Hawaiian or Pacific Islander, or Alaska Native (Figure 4B). Due to small sample size for most ethnic groups surveyed (White/Caucasian individuals: n = 82; Asian: n = 10; Mixed ethnicity: n = 6; Native American: n = 2; Native Hawaiian or Pacific islanders: n = 2; and Alaska Native: n = 1) differences in razor clam consumption were analyzed between White/Caucasian (79.6% of respondents) and all other ethnic groups (Non-White/Caucasian; 20.4% of respondents). Most respondents reported an annual income range of $50,001–$75,000 United States dollars (Figure 4C). Respondent ages were lumped into groups; the youngest respondents were 18–28 years and the oldest were 62+ years, but most (31.4%) were 51–61 years of age (Figure 4D).
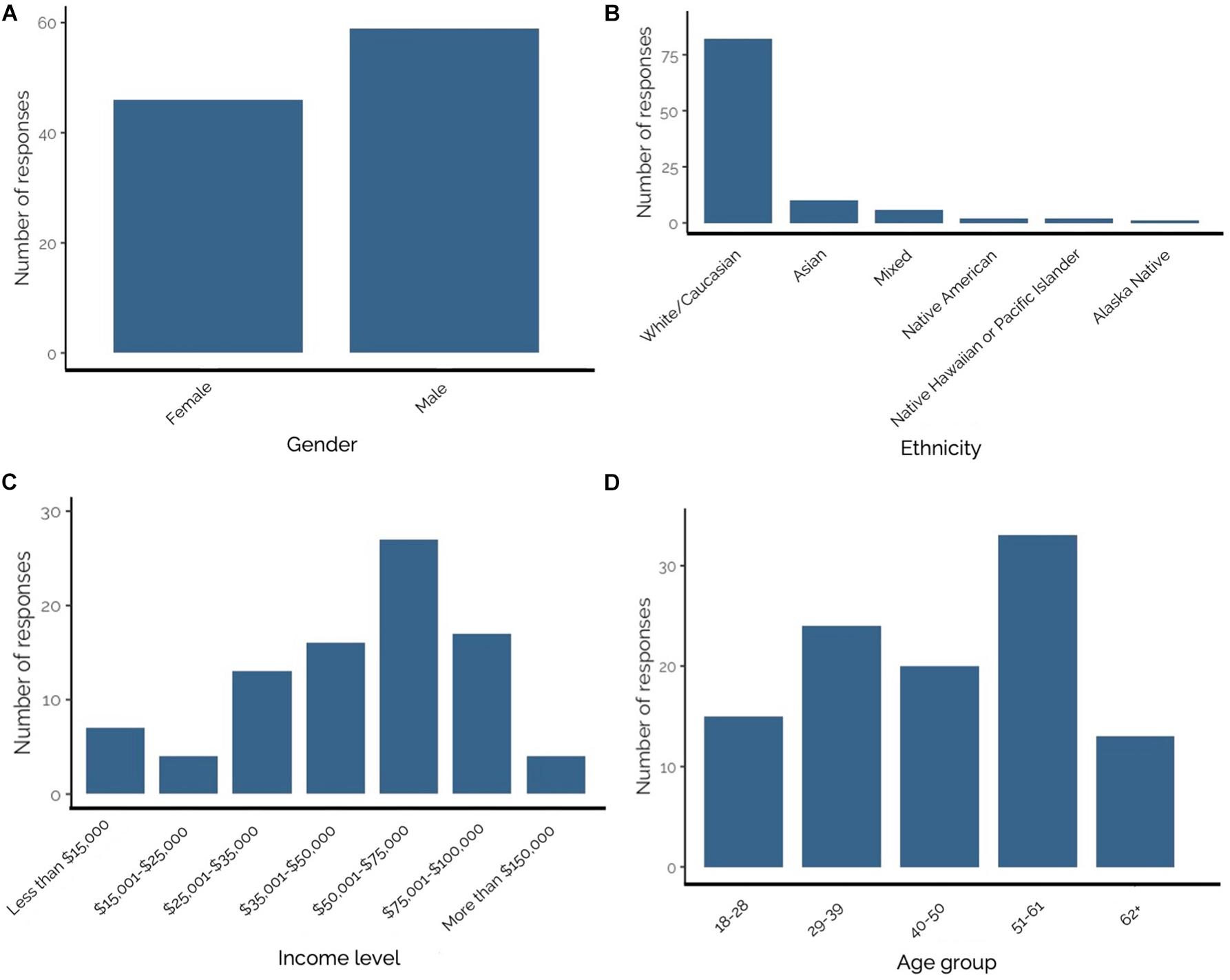
Figure 4. (A–D) From top left to bottom right–Number of Olympic Coast recreational razor clam harvester questionnaire responses by: (A) gender; (B) ethnicity; (C) income level; and (D) age group. Bold numbers above bars represent number of responses.
Razor Clam Harvest and Consumption
While only 83.8% of questionnaire respondents reportedly consumed razor clams in the past year (n = 88), all (100%; n = 107) participated in recreational razor clamming activities during the survey period (April 21–22, 2018). Razor clams consumed by respondents were obtained in a variety of ways; the majority (76.0%; n = 76) harvested the clams they ate, while some individuals obtained their clams in multiple ways (11.0%; n = 11), got clams from friends or family (4.0%; n = 4), purchased them (2.0%; n = 2), or didn’t know or obtained clams in other ways (3.0%; n = 3). The average number of razor clams consumed per meal was 4.27 ± 0.27 clams (range = 0–10); however, most respondents consumed either three or five clams per sitting (23.3 or 18.9%, respectively).
The majority of respondents (88.4%; n = 91) consumed razor clams without guts, while some did not know if they consumed the gut or not (9.7%; n = 10). One individual reported sometimes consuming them with, and sometimes without guts (1.0%; n = 1), and one individual reported consuming them with guts (1.0%; n = 1). More respondents reported consuming clams in the spring (81.1%; n = 77) than winter (72.8%; n = 67), fall (70.4%; n = 62), or summer (57.1%; n = 48) seasons.
To elucidate patterns in clam consumption, we considered demographic factors including gender, ethnicity, income and age group. The number of clams consumed per sitting varied by gender (Figure 5A), with males consuming significantly more than females (males: 4.81 ± 0.37, females: 3.78 ± 0.34; T = −2.051, p = 0.04). Clam consumption also varied by ethnicity, but these differences were not statistically analyzed due to low response numbers (as low as n = 1) for some categories (Figure 5B). On average, Alaska Native and Native American respondents consumed the highest number of clams per sitting at 7.0 (n = 1) and 7.0 ± 3.0 (n = 2), respectively. The next highest consumers of razor clams per sitting were Mixed ethnicity individuals (6.0 ± 0.40 clams; n = 4), followed by Native Hawaiian or Pacific Islanders (4.50 ± 4.50 clams; n = 2), White/Caucasians (4.46 ± 0.26 clams; n = 69), and Asians (1.56 ± 0.60; n = 9). No difference was found when comparing number of clams consumed per meal for White/Caucasian and Non-White/Caucasian (all ethnicities except White/Caucasian; T = −0.841, p = 0.41). The number of clams consumed per sitting did not vary by respondent income level (Figure 5C; F7,73 = 1.047, p = 0.41) or age group (Figure 5D; F4,83 = 0.924, p = 0.45).
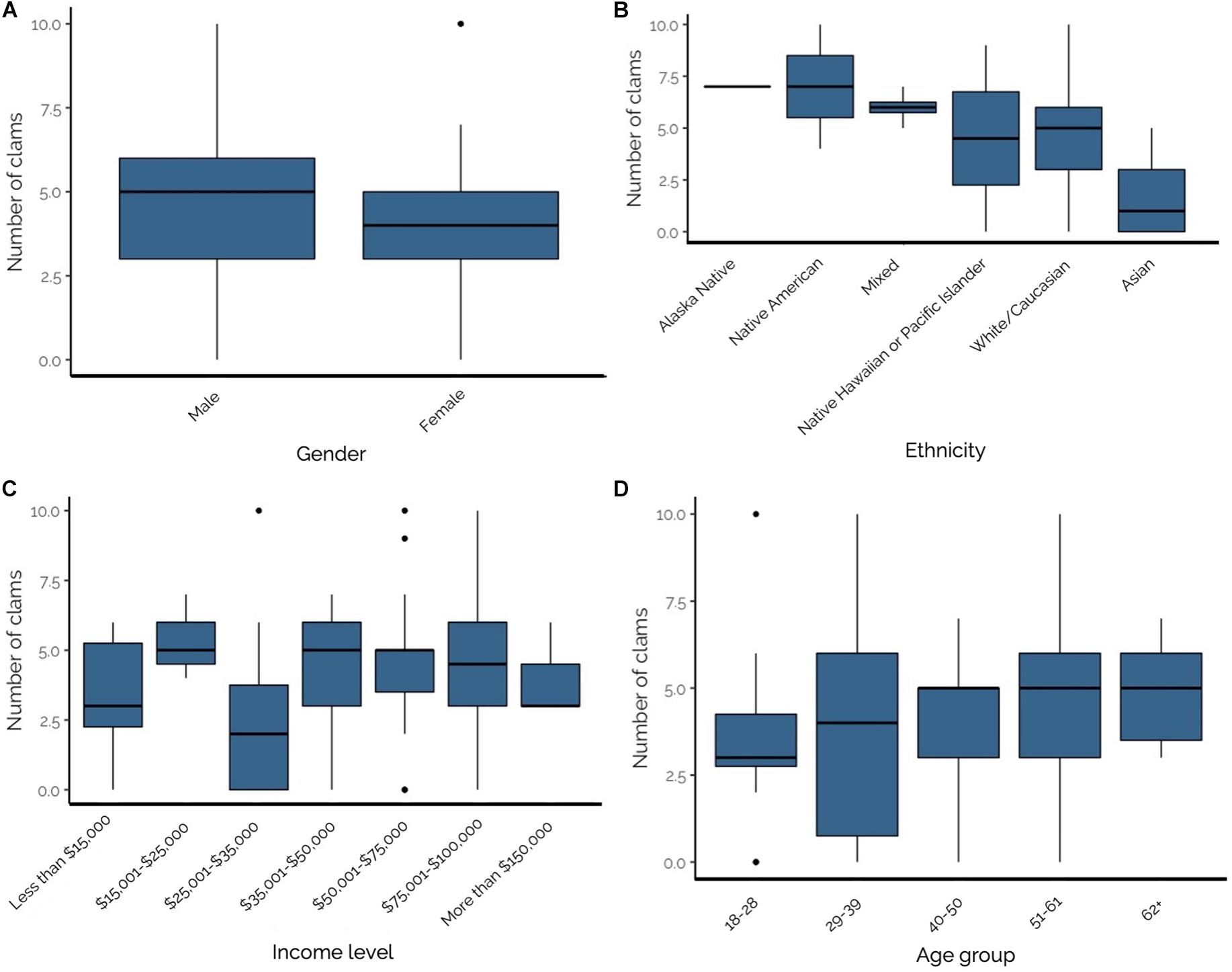
Figure 5. (A–D) From top left to bottom right–Number of razor clams consumed per sitting by Olympic Coast recreational razor clam harvester: (A) gender; (B) ethnicity; (C) income level; and (D) age group. Significant differences were identified between males and females (panel A; p = 0.04), but not by income level (p = 0.41) or age (p = 0.45).
In addition to razor clams, respondents reported consuming a variety of other common marine shellfish including bivalves (oysters, mussels, bay clams, hard shell clams, geoducks, scallops), crustaceans (Dungeness crab, other crab, lobster), gastropods (snails), and echinoderms (urchins; Supplementary Appendix 4).
Razor Clam Harvester-Consumer Microplastic Exposure
Using a combination of our razor clam MP burdens and questionnaire data, we estimated annual ranges of MP exposure through razor clam consumption by recreational harvester-consumers on the Olympic Coast (Table 2 and Figures 6, 7A,B). Of the questionnaire respondents, 16.2% (n = 17) harvested but did not consume razor clams in a given year, but those that did (83.8%; n = 88) consumed between 4 and 209 razor clam meals per year (Table 2 and Figure 6). Based on minimum and maximum number of razor clam meals per year and average suspected MP burdens in cleaned and whole clams, we estimated the range of MP exposure from razor clams by Olympic Coast harvester-consumers to be 60–3,070 MP/year for those that thoroughly clean their clams before consuming them, or 120–6,020 MP/year for those that consume clams whole without removing guts, gills or other organs (Table 2). Despite males consuming more clams per meal than females, female razor clam harvesters exhibited a broader range of exposure to MP due to higher maximum reported clam consumption than males in winter, summer and fall seasons (Table 2).
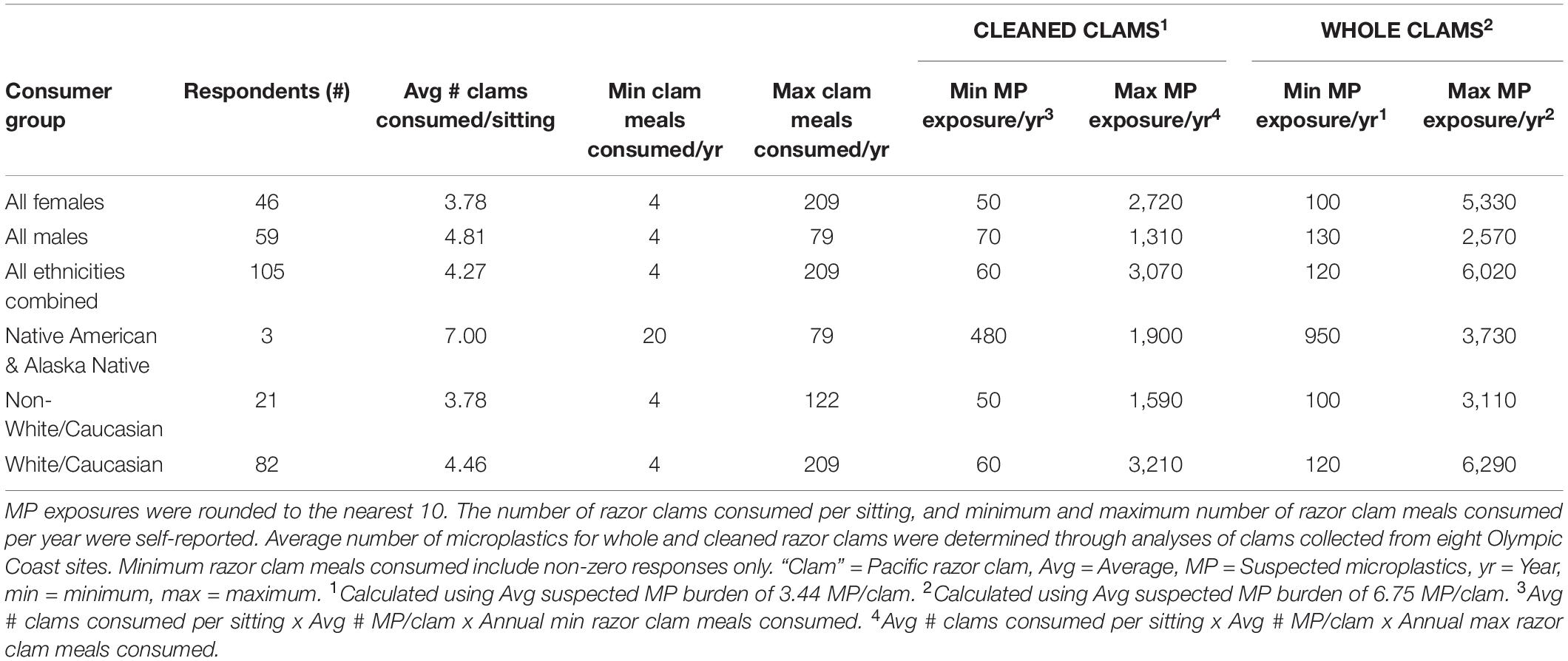
Table 2. The estimated annual microplastic exposure ranges by Olympic Coast, WA razor clam harvester-consumers.
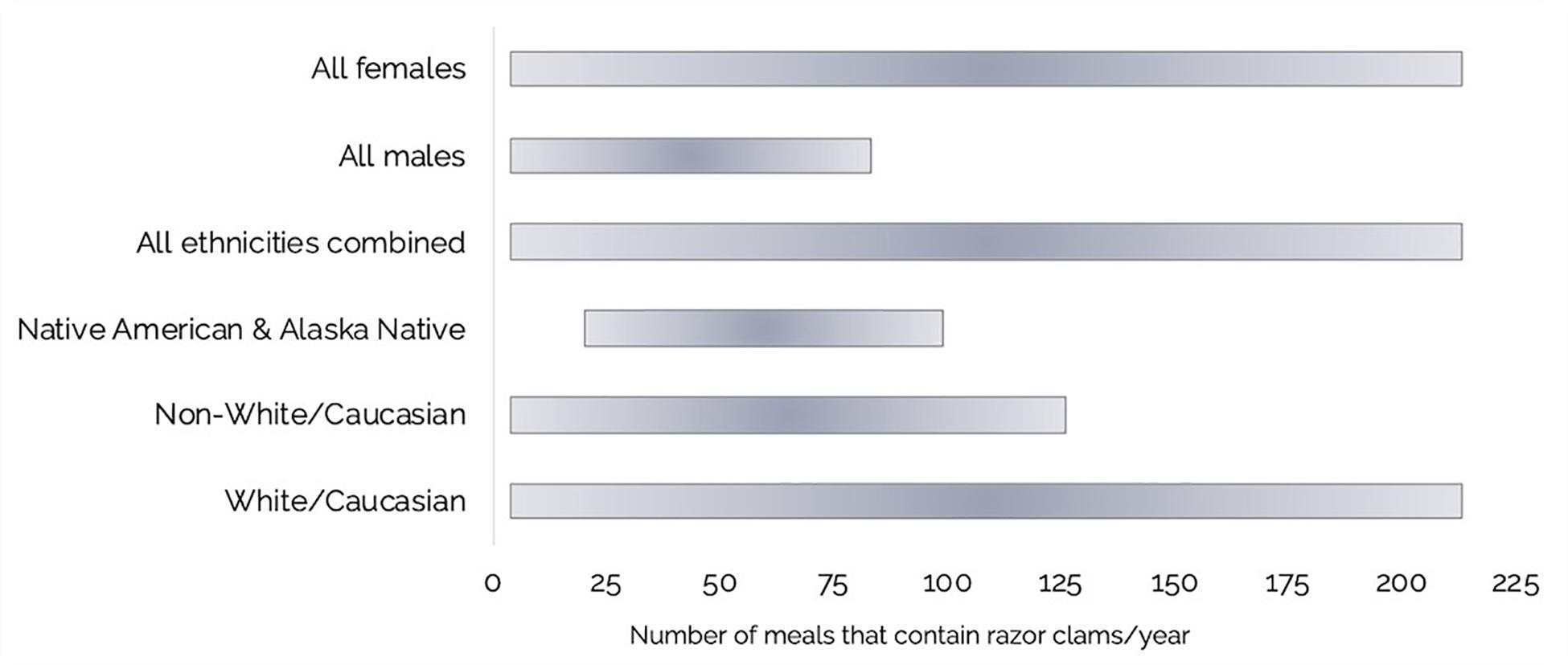
Figure 6. Ranges for the number of razor clam meals consumed per year by Olympic Coast harvester-consumers: Females (n = 46), Males (n = 59), All ethnicities combined (n = 87), Native American and Alaska Native (n = 3), Non-White/Caucasian (includes all ethnicities except White/Caucasian; n = 18), and White/Caucasian (n = 69).
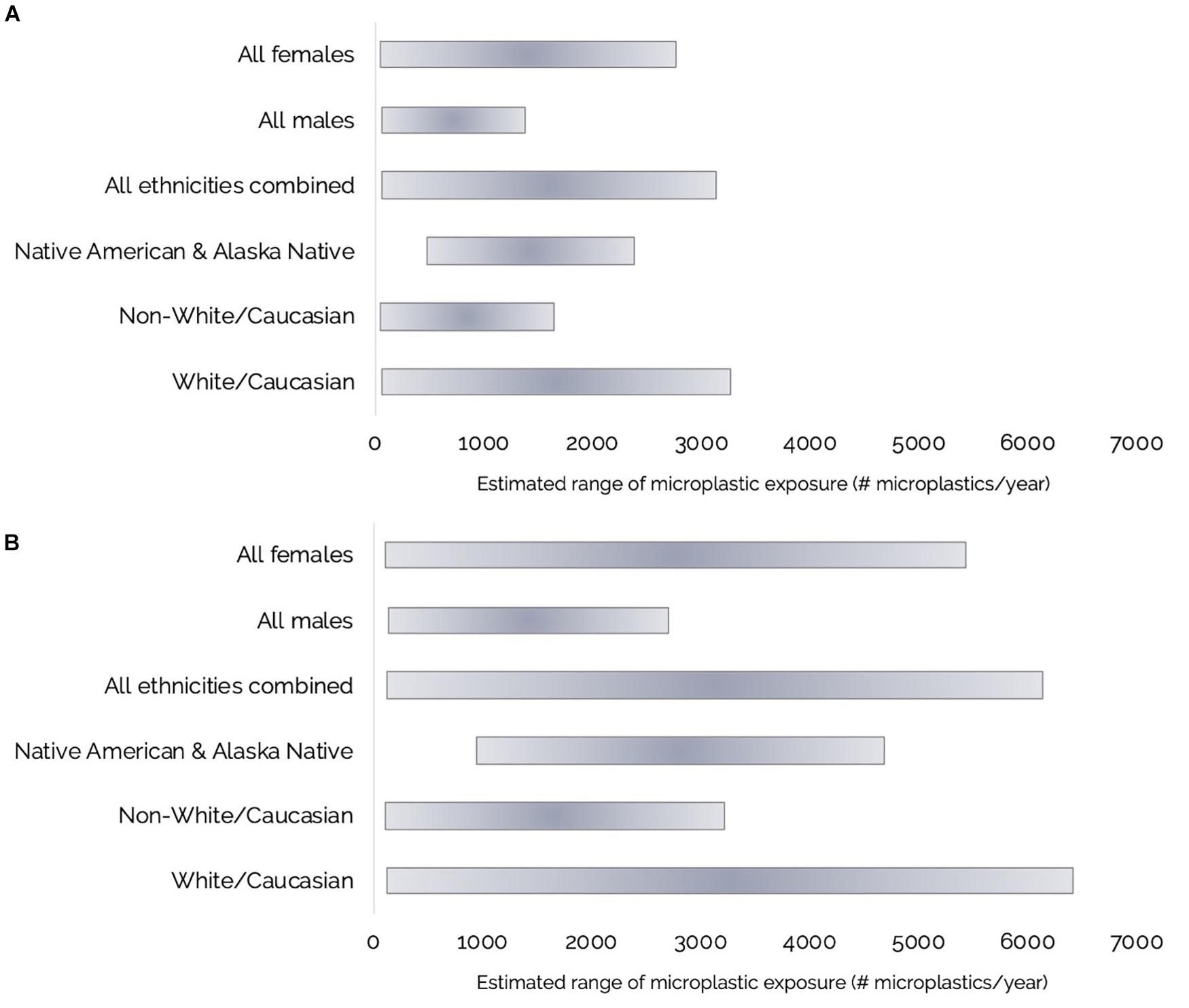
Figure 7. (A,B) Ranges of estimated annual Olympic Coast razor clam harvester-consumer microplastic (MP) exposure for: Females (n = 46), males (n = 59), all ethnicities combined (n = 87), Native American and Alaska Native (n = 3), Non-White/Caucasian (n = 18), or White/Caucasian (n = 69) respondents from consumption of: (A) Cleaned razor clams with the foot and siphons cleaned and rinsed and the siphon tips, digestive organs and gills removed; and (B) Whole razor clams.
Microplastic exposure from razor clam consumption was also broken down by ethnicity into: All (all ethnicities), Native American and Alaska Native, Non-White/Caucasian (all other ethnicities excluding White/Caucasian), and White/Caucasian groups (Table 2 and Figures 7A,B). The three Native American and Alaska Native respondents in our sample consumed more clams per sitting than other respondents; therefore the minimum exposure to MP through razor clam consumption for this group is higher than for the other ethnicities analyzed (Table 2 and Figures 7A,B).
Questionnaire Respondent Knowledge and Concerns About Microplastics in Food
When asked “how familiar are you with the concept of MPs in food?,” 64.8% (n = 68) of respondents said they were not familiar, 28.6% (n = 30) said they were moderately familiar, and 6.7% (n = 7) said they were very familiar. When asked “do you have concerns about MPs in your food,” 41.9% (n = 44) indicated yes, 39.0% (n = 41) indicated no, and 19.0% (n = 20) did not know. Twenty-five respondents (39.0% of those that indicated “Yes” or “I don’t know” for the question “do you have concerns about MPs in your food”) answered the open-ended question “Please expand on what concerns you have about MPs in your food.” Concerns recorded were categorized into seven themes: Impacts on humans (n = 6), General concern (n = 5), Health, chemicals and toxins (n = 4), Impacts on humans and the environment (n = 4), Uncertainty or unknown (n = 3), Impacts on the environment (n = 2), and Management (n = 1; Table 3).
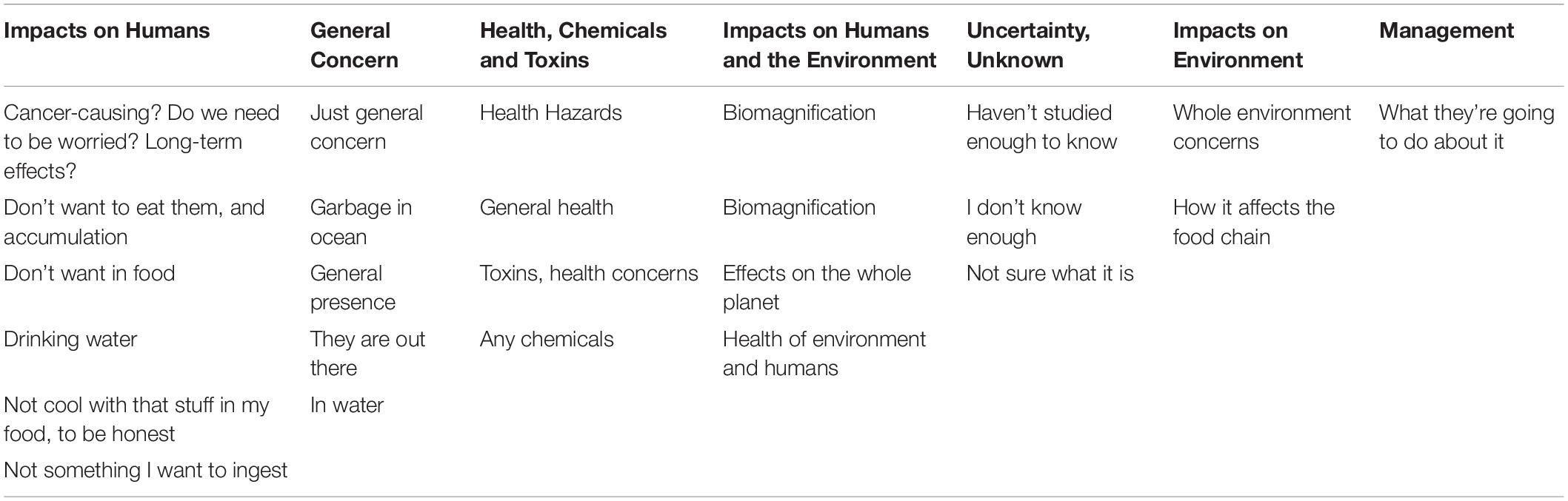
Table 3. Themes elucidated from Olympic Coast razor clam harvester questionnaire survey responses (n = 25) for the question “Please expand on what concerns you have about microplastics in your food.”
Discussion
This study confirms MP presence in Olympic Coast Pacific razor clams, a species important to the PNW’s coastal ecology, culture, food security, and economy. Razor clams represent not only a traditional but also a critical present-day food source for members of the Quinault Indian Nation and other Olympic Coast peoples. As the Olympic Peninsula is sparsely populated relative to other (non-Alaskan) coastal areas in the United States, this study provides a snapshot of MP prevalence in an area of relatively low human influence. A number of existing studies have found that elevated environmental MP concentrations can be attributed to proximity to dense human population (Barnes et al., 2009; Hantoro et al., 2019); therefore, this study may represent the lower end of human MP exposure from Pacific razor clam consumption in the continental United States.
The highest MP g–1 tissue site average was from our northernmost study site, Kalaloch beach (Figure 4B). With development and agriculture representing a small proportion of land use in these counties (Supplementary Appendix 1), we expect factors other than land use/land cover are responsible for Kalaloch clams containing significantly more MPs than clams at other sites. There are no major rivers near the Kalaloch sample site to deliver large loads of MPs here relative to the other seven sites; however, Kalaloch the northernmost of our eight sites, is closest to the heavily populated Salish Sea region. We predicted substantial waterborne MPs would be transported from Puget Sound and the Salish Sea to the Olympic Coast, based on existing hydrographic models (Pirhalla et al., 2009). Proximity of these large water bodies to the Kalaloch site may contribute to its higher-than-average MP concentrations in Olympic Coast razor clams. Kalaloch clams were the smallest, on average (both shell length and body weight), of all eight study sites. While we did not find a correlation between MPs and body size (shell length or body weight) across study sites, clam size may have contributed to high MP g–1 tissue at Kalaloch, as mass-specific filtration can be faster in smaller bivalves relative to their larger counterparts (e.g., Powell et al., 1992; Ehrich and Harris, 2015).
Average MP burdens per whole razor clam and g–1 tissue in this study fell roughly mid-range compared to MP burdens reported in other bivalves (manila clams, Pacific razor clams, Pacific oysters) throughout the region: the Salish Sea, WA (Martinelli et al., 2020), British Columbia, Canada (Davidson and Dudas, 2016; Covernton et al., 2019), and the Oregon coast (Baechler et al., 2020a; Figure 8). It should be noted that these studies employed varying methodologies for MP extraction, identification, and reporting, suggesting the need for caution in interpreting direct comparisons. Methodological variations included differences in digestion chemicals (KOH, H2O2), microplastic isolation methods (density separation, sieving, vacuum filtration), MP g–1 tissue calculations (use of wet weight vs. dry weight), and validation techniques (none, Raman, or FTIR spectroscopy; Figure 8).
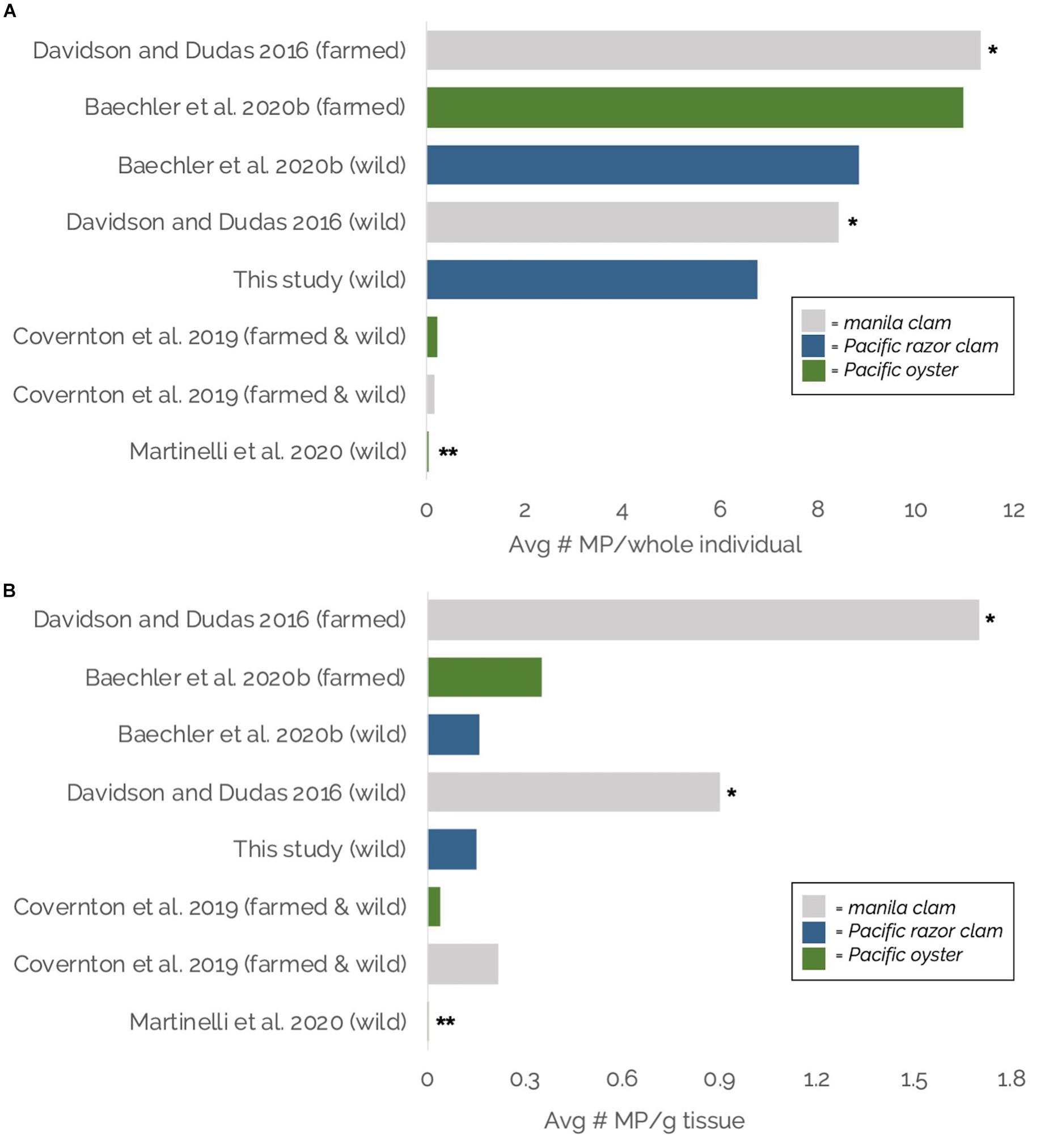
Figure 8. (A,B) Comparisons of average microplastic burdens in Pacific Northwest bivalves (Washington and Oregon, United States and British Columbia, Canada), in– (A) Average number of microplastics per individual; and (B) Average number of microplastics per gram of tissue (wet weight; except dry weight for Covernton et al., 2019). Gray bars indicate manila clams, blue bars indicate Pacific razor clams, green bars indicate Pacific oysters. Avg = Average, MP = microplastic, g = gram. * = All studies except Davidson and Dudas included suspected microplastic material validation using Fourier Transform Infrared (FTIR) or Raman spectroscopy. ** = For general context only; calculated from Table 2 of Martinelli et al. (2020) as average of site-specific visually identified number of particles per oyster (panel A) or per gram of tissue (panel B) for 10 sites × average site-specific percentage of suspected microplastics confirmed to be plastic through Raman spectroscopy for 10 sites.
Using FTIR we determined that the randomly selected suspected MPs analyzed (n = 53) were plastic, semi-synthetic, and natural, with 80.77% confirmed to be either synthetic or semi-synthetic (Kroon et al., 2018; Supplementary Appendix 7). PET, a member of the polyester family and a widely used thermoplastic polymer resin in clothing and drink containers, was the most commonly identified synthetic material (n = 21; Supplementary Appendix 7,8). PET fibers are ubiquitous in coastal areas globally and are often detected in bivalves (Mishra et al., 2019; Hope et al., 2020). The most commonly identified semi-synthetic fiber was cellulose acetate (n = 11; Supplementary Appendix 7,8), a material found in cigarette filters and clothing, that is commonly detected on beaches and in waterways (Miller et al., 2017; Mishra et al., 2019). Cellulose was the lone natural material present in our subsample (n = 10; Supplementary Appendix 7).
Due to our methodology, the lower size limit of detectable MPs was 63 μm, though smaller MPs and nanoplastics (NPs) (<1 μm) are known to migrate into the tissues and organs of marine organisms (Lusher et al., 2017). While methodologically, any suspected MPs greater than 63 μm in size were included in our samples, the smallest MP we identified was 110 μm. Therefore, MPs and NPs smaller than 110 μm may have been present but undetected in our samples. Additionally, it is unclear if the MPs identified in our whole, cleaned or non-gut-tissue samples were incorporated into clams through ingestion or adherence to soft tissues, as both avenues of incorporation have been reported in bivalves (e.g., Xu et al., 2017; Kolandhasamy et al., 2018).
Combining ecological data on razor clam MP burdens with structured questionnaires allowed us to calculate MP exposure ranges for the Olympic Coast razor clam harvester-consumers surveyed. MP exposure calculations included natural cellulose particles representing 19.23% of suspected MPs based on FTIR material confirmation results; therefore exposure ranges to MPs may be overestimates. Regardless, our findings suggest the harvester-consumers in this study are likely exposed to low levels of confirmed MPs (relative to other known sources of ingested MPs). Razor clams are unique in that, unlike many other clam and oyster species which are generally consumed whole, they are typically cleaned with parts removed prior to consumption. The Quinault Indian Nation Department of Fisheries suggests the Quinault and other coastal tribal peoples clean razor clams prior to consumption, and the Quinault commercial razor clam processing plant in Taholah similarly cleans clams prior to sale to eliminate guts and gills (Ervin “Joe” Schumacker, Quinault Indian Nation Department of Fisheries, pers. comm. May 1, 2020). While most questionnaire respondents fully cleaned razor clams before consuming them, 11.7% either consumed the gut or weren’t sure if they did. Based on our findings, removal of the gut prior to consumption for this particular species reduces MP burden by nearly 50% and may be a prudent measure to reduce consumer MP exposure from this particular seafood source. While cleaned clams may substantially reduce MP exposure for human consumers, this approach is not an option for marine razor clam predators.
There are currently no established safety thresholds for human MP intake; however, established thresholds in Olympic Coast razor clams exist for domoic acid, a neurotoxin that can cause direct and severe harm to human consumers, including affecting memory when consumed in low doses over long periods of time (Chadsey et al., 2012; Grattan et al., 2016; Ferriss et al., 2017; Lefebvre et al., 2017). Recently, fishery openings and closures have been affected by the presence of domoic acid which is regularly tested in clams by the Washington Department of Health. Fisheries are closed when the domoic acid level meets or exceeds 20 parts per million (ppm) (Chadsey et al., 2012; WDFW, 2020). In 2016, the state of WA issued a public health advisory on razor clam consumption, recommending no more than 15 clams be consumed per month, due to the propensity of razor clams to contain low levels of domoic acid below the Department of Health action level of 20 ppm (Washington Department of Health, 2016).
Human ingestion of MPs via seafood can be significant in some geographic areas (e.g., Li et al., 2015); however, MP exposure of our study group from razor clam consumption is low relative to other expected exposure routes such as inhalation (estimated maximum of 30,000,000 MP/year; Zhang Q. et al., 2020), deposition of microfibers during meals (estimated 13,731–68,415 MPs/year; Catarino et al., 2018), consumption of table salt (estimated maximum of 73,000 MPs/year; Zhang Q. et al., 2020) or drinking bottled water (estimated 90,000 MPs/year; Cox et al., 2019). Our estimates of Olympic Coast razor clam harvester-consumer MP exposure are lower than existing estimates of dietary MPs through bivalve consumption in Europe (∼11,000 MP/year; Van Cauwenberghe and Janssen, 2014) and China (∼110,000 MP/year; Li et al., 2015). This result is expected considering razor clams are only one of many bivalves consumed in the region, this species is frequently cleaned prior to consumption, and the Olympic Coast is sparsely populated (roughly 12 people/km2 or 30 people/mile2; U.S. Census Bureau, 2012) whereas Southeast Asia and Europe support some of the most densely populated coastal areas in the world (>10,000 people/km2; Nicholls and Small, 2002).
Although the vast majority of MPs orally ingested by humans are thought to be excreted (Efsa Panel on Contaminants in the Food Chain Contam, 2016), some proportion of particles may remain in the body. While effects of MPs on human health are not well known, MPs may cause harm due to blockage, release of associated additives, or liberation of sorbed chemicals (Wright and Kelly, 2017). Inhaled MPs may become trapped in the lung, purged from the body (i.e., from coughing or sneezing) or, depending on size, enter the digestive tract (Wright and Kelly, 2017; Cox et al., 2019). Differential effects from consuming versus inhaling MPs may be present, though this area is understudied and warrants additional research.
Ferriss et al. (2017) estimated the frequency of razor clam consumption by WA coast recreational razor clam harvesters in 2015–2016 at 6.0 clams per day, on days that clams were eaten. Males consumed, on average, more clams/day (6.5 ± 0.8) than females (5.4 ± 0.6), with higher overall consumption in the spring than in other seasons (Ferriss et al., 2017). While not directly comparable to our results because we report consumption in number of clams per sitting (4.27 ± 0.27) instead of per day, the pattern of males consuming more than females, and seasonal clam consumption being highest in spring relative to other seasons are similar. An Olympic Coast razor clam domoic acid study by Grattan et al. (2016), placed those that consume 15 or more razor clams per month in a “high” consumer group, and those that consume fewer than 15 per month into a “low” consumer group based on the 2016 WA-recommended consumption limit of 15 clams per month (Washington Department of Health, 2016). According to our findings, “high” consumers (2.67%, n = 7 responses in our study) would then be exposed to a minimum of roughly 600 MP/year if thoroughly cleaning 15 clams per month before consuming them, or a minimum of 1,200 MP/year if consuming 15 clams per month without cleaning them. Exposure values would increase proportionally with the number of clams consumed per month. Due to the low sample size of Alaska Native and Native American respondents (n = 3) in this study, it is not possible to broadly generalize about clam consumption frequencies in either group; however, responses from these few individuals were striking considering the much higher minimum exposure level of MPs through razor clam consumption than other ethnicities, due to a higher minimum number of meals containing razor clams eaten annually and more clams consumed per sitting than other groups. Due to this finding and the knowledge that seafood consumption tendencies vary by ethnicity (e.g., Sechena et al., 1999; Mahaffey et al., 2009; EPA, 2014; Cisneros-Montemayor et al., 2016), additional research regarding microplastic exposure from seafood consumption for these and other high-seafood consumer demographics is both warranted and necessary.
To conclude, MP presence in the PNW coastal environment and in food webs, raises concern about potential for ecological harm to Pacific razor clams, their predators, and innumerable other marine species. There is an immense need to reduce future transmission of MPs, and in particular microfibers, to the marine environment, as these are commonly found in biotic and environmental samples (Barnes et al., 2009; Barboza et al., 2018). A focus on land-based solutions to microplastic pollution is imperative, as more than 80% of primary and secondary MPs originate on land (Miranda et al., 2020). Apparel washing is a significant vector of synthetic microfibers to the environment; since the 1950’s, over 5.9 million metric tons of synthetic MPs have been generated through laundering (Gavigan et al., 2020). Our findings of primarily microfibers in the guts and tissues of Olympic Coast razor clams highlight the need to develop effective upstream pollution control solutions that capture this type of pollution at the source. One solution to significantly reduce transmission of microfibers into the environment would be the integration of microplastic capture technologies into washing machines. These technologies prevent synthetic microfibers from flowing through household graywater to wastewater treatment plants, where they settle and are frequently extracted along with biosolids and applied to agricultural soils as fertilizer (Mahon et al., 2017; Gavigan et al., 2020). Fiber catchment-integrated washing machines have already been deployed in Japan and, per legislation, will be required in France beginning in 2025 (European Parliament, 2020). Broader adoption of similar technologies in the United States and around the world could drastically reduce global laundry microfiber emissions in the future.
In summary, we identified MPs in razor clams from all of our coastal study sites, highlighting the ubiquity of MPs along the Olympic Coast. The Olympic Coast recreational razor clam harvester-consumers represented in this study were from 14 WA counties and encompassed an array of ethnicities, incomes, education levels, and concerns about the concept of MPs in food. This work improves understanding of MP prevalence in an important and desirable edible shellfish species. It highlights that MPs are present in seafood even in relatively low-impact areas; however, when put into context, this specific seafood item represents a minimal vector of MPs for the recreational razor clam harvester-consumer group studied. Our work serves as an important reference in the growing portfolio of MP research in the PNW to inform future MP attenuation. This work contributes to estimations of overall human MP exposure and could aide in the development of human health standards for this pollution type.
Data Availability Statement
The raw data supporting the conclusions of this article will be made available by the authors, without undue reservation.
Ethics Statement
The studies involving human participants were reviewed and approved by Portland State University Human Research Protection Program Research Integrity/Institutional Review Board. The ethics committee waived the requirement of written informed consent for participation.
Author Contributions
BRB and EFG identified the research question and study approach, designed laboratory methods, and are responsible for manuscript data. EFG, BRB, MN-P, and SJM designed the razor clam sampling and questionnaire formats. SJM and BRB collected the razor clams. BRB conducted the laboratory analyses and led the manuscript effort. SMB oversaw FTIR analysis and interpretation. EFG, SJM, SMB, and MN-P contributed ideas and edits to the manuscript. All authors contributed to the article and approved the submitted version.
Funding
Funding for this project was provided by the Edward D. and Olive C. Bushby Scholarship, the Oregon Chapter of the American Fisheries Society, the Sustainable Fisheries Foundation, the Puget Sound Anglers: Fidalgo–San Juan Chapter, and the Oregon State University Hatfield Marine Science Center. Publication of this article in an open access journal was funded by the Portland State University Library’s Open Access Fund.
Conflict of Interest
The authors declare that the research was conducted in the absence of any commercial or financial relationships that could be construed as a potential conflict of interest.
Acknowledgments
We thank Drs. Kathleen Conn, Eugene Foster, and Erin Shortlidge for their extensive and thoughtful input and guidance. Many thanks to Emily Pedersen and Katherine Lasdin of Oregon State University for performing and advising on FTIR analyses, respectively. Thanks to Dr. Angela Strecker and Rich Miller for allowing us the use of their stereomicroscope. Much appreciation to Ervin “Joe” Schumacker, Staci Bruce (Quinault Indian Nation) and John Deibert, Clayton Parson, Zach Forster and Dan Ayres (WDFW), for assistance with sample collection, coordination, and providing input on the manuscript. Thanks to the Applied Coastal Ecology lab for assistance and support throughout the study. Assistance with questionnaire administration was provided by Alex Bienko, Amy Hemingway, Anna Bolm, Scott Holland, and Kaegan Scully-Engelmeyer; sample processing assistance was provided by Scott Holland, Alexandra Tissot, and Rosemary Wood.
Supplementary Material
The Supplementary Material for this article can be found online at: https://www.frontiersin.org/articles/10.3389/fmars.2020.588481/full#supplementary-material
Supplementary Appendix 1 | 2010 Land use cover (km2) for the four coastal counties located on the Olympic Peninsula, WA. Counties are ordered north to south. Razor clam sample site names are listed below their respective county names. No razor clam sites were located in Clallam county. Data were obtained from NOAA’s Digital Coast at: https://coast.noaa.gov/digitalcoast/tools/lca.html.
Supplementary Appendix 2 | Schematic of the two microplastic (MP) contamination quantification techniques employed in this study. Procedural controls were deionized water filtered to 50 μm and run through the same process as all other samples. Microscope blanks were glass petri dishes filled with filtered DI water, placed on the microscope base, and left open to the air during visual inspection of samples for MPs.
Supplementary Appendix 3 | Five-page structured questionnaire administered to Olympic Coast razor clam harvesters in April 2018 at Mocrocks and Copalis Beaches, Olympic Peninsula, Washington, United States.
Supplementary Appendix 4 | Number and percentage of Olympic Coast questionnaire respondents that consumed marine shellfish species or species groups. Consumption frequency is ordered from most to least frequent. 1Calculated as number of respondents that reported consuming a particular species or species group (i.e., all consumption frequencies except “I don’t know” and “Never”), divided by total responses for that species or species group, multiplied by 100.
Supplementary Appendix 5 | Example photos of suspected microplastics (MPs) extracted from Olympic Coast razor clams (top) and total counts of each MP category encountered (bottom) in the four razor clam sample types included in this study (whole, cleaned, gut-tissue only, tissue-only). Black bars represent a length of 0.5 mm in each of the images shown.
Supplementary Appendix 6 | Numbers and percentages of suspected microplastics identified in 138 Olympic Coast razor clam samples (n = 799) by color. Colors in figure correspond to actual colors encountered: colorless (n = 441; 55.19%), blue (n = 154; 19.27%), gray (n = 98; 12.27%), black (n = 37; 4.63%), red (n = 26; 3.25%), purple (n = 19; 2.38%), brown (n = 15; 1.88%), green (n = 5; 0.63%), and pink (n = 4; 0.50%).
Supplementary Appendix 7 | Characteristics, material types and FTIR spectral match percentages for 53 randomly selected suspected microplastics isolated from Olympic Coast razor clams. Results are sorted by FTIR % top match from highest to lowest.
Supplementary Appendix 8 | Example FTIR-ATR spectra of microplastics isolated from Olympic Coast razor clams, stacked along with the two highest percentage matches from Omnic, Primpke et al. (2018) and Primpke et al. (2020) FTIR polymer databases for a: (A) Polyethylene Terephthalate fiber (1.9 mm total length); and (B) Cellulose acetate fiber (3.2 mm total length).
References
Abidli, S., Lahbib, Y., and Trigui El Menif, N. (2019). Microplastics in commercial molluscs from the lagoon of Bizerte (Northern Tunisia). Mar. Pollut. Bull. 142, 243–252. doi: 10.1016/j.marpolbul.2019.03.048
Anderson, J. W. (1999). World is Their Oyster - Interpreting the Scope of Native American Off-Reservation Shellfish Rights in Washington State. Vol. 23, Seattle, WA: Seattle University Law Reviwe, 145–174.
Anderson, T. L., and Parker, D. P. (2009). Economic development lessons from and for North American Indian economies∗. Aust. J. Agric. Resour. Econ. 53, 105–127. doi: 10.1111/j.1467-8489.2007.00426.x
Avio, C. G., Gorbi, S., Milan, M., Benedetti, M., Fattorini, D., d’Errico, G., et al. (2015). Pollutants bioavailability and toxicological risk from microplastics to marine mussels. Environ. Pollut. 198, 211–222. doi: 10.1016/j.envpol.2014.12.021
Baechler, B. R., Granek, E. F., Hunter, M. V., and Conn, K. E. (2020a). Microplastic concentrations in two Oregon bivalve species: spatial, temporal, and species variability. Limnol. Oceanogr. Lett. 5, 54–65. doi: 10.1002/lol2.10124
Baechler, B. R., Stienbarger, C. D., Horn, D. A., Joseph, J., Taylor, A. R., Granek, E. F., et al. (2020b). Microplastic occurrence and effects in commercially harvested North American finfish and shellfish: current knowledge and future directions. Limnol. Oceanogr. Lett. 5, 113–136. doi: 10.1002/lol2.10122
Barboza, L. G. A., Dick Vethaak, A., Lavorante, B. R. B. O., Lundebye, A.-K., and Guilhermino, L. (2018). Marine microplastic debris: an emerging issue for food security, food safety and human health. Mar. Pollut. Bull. 133, 336–348. doi: 10.1016/j.marpolbul.2018.05.047
Barnes, D. K., Galgani, F., Thompson, R. C., and Morton, B. (2009). Accumulation and fragmentation of plastic debris in global environments. Philos. Trans. R. Soc. B Biol. Sci. 364, 1985–1998. doi: 10.1098/rstb.2008.0205
Black, R. W., Barnes, A., Elliot, C., and Lanksbury, J. (2018). Nearshore Sediment Monitoring for the STORMWATER Action Monitoring (SAM) Program, Puget Sound, Western Washington. Reston, VA: U.S. Geological Survey, doi: 10.3133/sir20185076
Bour, A., Haarr, A., Keiter, S., and Hylland, K. (2018). Environmentally relevant microplastic exposure affects sediment-dwelling bivalves. Environ. Pollut. 236, 652–660. doi: 10.1016/j.envpol.2018.02.006
Brander, S. M., Renick, V. C., Foley, M. M., Steele, C., Woo, M., Lusher, A., et al. (2020). EXPRESS: sampling and QA/QC: a guide for scientists investigating the occurrence of microplastics across matrices. Appl. Spectrosc. 74, 1099–1125. doi: 10.1177/0003702820945713
Carbery, M., O’Connor, W., and Palanisami, T. (2018). Trophic transfer of microplastics and mixed contaminants in the marine food web and implications for human health. Environ. Int. 115, 400–409. doi: 10.1016/j.envint.2018.03.007
Catarino, A. I., Macchia, V., Sanderson, W. G., Thompson, R. C., and Henry, T. B. (2018). Low levels of microplastics (MP) in wild mussels indicate that MP ingestion by humans is minimal compared to exposure via household fibres fallout during a meal. Environ. Pollut. 237, 675–684. doi: 10.1016/j.envpol.2018.02.069
Chadsey, M., Trainer, V. L., and Leschine, T. M. (2012). Cooperation of science and management for harmful algal blooms: domoic acid and the washington coast razor clam fishery. Coas. Manag. 40, 33–54. doi: 10.1080/08920753.2011.639865
Charles, B., Cooke, V., Grinnell, E., Morganroth, C. III, Morganroth, L. M., Peterson, M., et al. (2004). When the Tide is Out: An Ethnographic Study of Nearshore Use on the Northern Olympic Peninsula. Native American Traditional and Contemporary Knowledge of the Northern Olympic Peninsula. Port Angeles: Coastal Watershed Institute and Olympic National Park.
Cho, S.-A., Cho, W.-B., Kim, S.-B., Chung, J.-H., and Kim, H.-J. (2019). Identification of microplastics in sea salts by raman microscopy and FT-IR microscopy. Anal. Sci. Technol. 32, 243–251. doi: 10.5806/AST.2019.32.6.243
Cisneros-Montemayor, A. M., Pauly, D., Weatherdon, L. V., and Ota, Y. (2016). A global estimate of seafood consumption by coastal indigenous peoples. PLoS One 11:e0166681. doi: 10.1371/journal.pone.0166681
Covernton, G. A., Collicutt, B., Gurney-Smith, H. J., Pearce, C. M., Dower, J. F., Ross, P. S., et al. (2019). Microplastics in bivalves and their habitat in relation to shellfish aquaculture proximity in coastal British Columbia. Canada. Aquacult. Environ. Interact. 11, 357–374. doi: 10.3354/aei00316
Cox, K. D., Covernton, G. A., Davies, H. L., Dower, J. F., Juanes, F., and Dudas, S. E. (2019). Human consumption of microplastics. Environ. Sci. Technol. 53, 7068–7074. doi: 10.1021/acs.est.9b01517
Crosman, K., Petrou, E., Rudd, M., and Tillotson, M. (2019). Clam hunger and the changing ocean: characterizing social and ecological risks to the Quinault razor clam fishery using participatory modeling. Ecol. Soc. 24:16. doi: 10.5751/ES-10928-240216
Crossett, K., Ache, B., Pacheco, P., and Haber, K. (2013). National Coastal Population Report, Population Trends From 1970 to 2020. NOAA State of the Coast Report Series. Washington: US Department of Commerce.
Davidson, K., and Dudas, S. E. (2016). Microplastic ingestion by wild and cultured manila Clams (Venerupis philippinarum) from baynes sound. Br. Columbia. Arch. Environ. Contam. Toxicol. 71, 147–156. doi: 10.1007/s00244-016-0286-4
de Witte, B., Devriese, L., Bekaert, K., Hoffman, S., Vandermeersch, G., Cooreman, K., et al. (2014). Quality assessment of the blue mussel (Mytilus edulis): comparison between commercial and wild types. Mar. Pollut. Bull. 85, 146–155. doi: 10.1016/j.marpolbul.2014.06.006
Eerkes-Medrano, D., Leslie, H. A., and Quinn, B. (2018). Microplastics in drinking water: a review and assessment of an emerging concern. Curr. Opin. Environ. Sci. Health 7, 69–75. doi: 10.1016/j.coesh.2018.12.001
Efsa Panel on Contaminants in the Food Chain Contam (2016). Statement on the presence of microplastics and nanoplastics in food, with particular focus on seafood. EFSA J. 14, 1–30. doi: 10.2903/j.efsa.2016.4501
Ehrich, M. K., and Harris, L. A. (2015). A review of existing eastern oyster filtration rate models. Ecol. Modelling 297, 201–212. doi: 10.1016/j.ecolmodel.2014.11.023
EPA (2014). Estimated Fish Consumption Rates for the U.S. Population and Selected Subpopulations (NHANES 2003 – 2010). EPA-820-R-14-002. Washington, DC: United States Environmental Protection Agency.
European Parliament (2020). Parliamentary Questions: Plastic Microfibre Filters for New Washing Machines by 2025. Avaliable at: https://www.europarl.europa.eu/doceo/document/E-9-2020-001371_EN.html#:$nsim$:text=France%20has%20just%20adopted%20a,away%20from%20clothing%20during-%20washing (accessed September 20, 2020).
Farrell, P., and Nelson, K. (2013). Trophic level transfer of microplastic: Mytilus edulis (L.) to Carcinus maenas (L.). Environ. Pollut. 177, 1–3. doi: 10.1016/j.envpol.2013.01.046
Ferriss, B. E., Marcinek, D. J., Ayres, D., Borchert, J., and Lefebvre, K. A. (2017). Acute and chronic dietary exposure to domoic acid in recreational harvesters: a survey of shellfish consumption behavior. Environ. Int. 101, 70–79. doi: 10.1016/j.envint.2017.01.006
Frias, J. P. G. L., and Nash, R. (2019). Microplastics: finding a consensus on the definition. Mar. Pollut. Bull. 138, 145–147. doi: 10.1016/j.marpolbul.2018.11.022
Gavigan, J., Kefela, T., Macadam-Somer, I., Suh, S., and Geyer, R. (2020). Synthetic microfiber emissions to land rival those to waterbodies and are growing. PLoS One 15:e0237839. doi: 10.1371/journal.pone.0237839
Grattan, L. M., Boushey, C., Tracy, K., Trainer, V. L., Roberts, S. M., Schluterman, N., et al. (2016). The association between razor clam consumption and memory in the CoASTAL cohort. Harmful Algae 57, 20–25. doi: 10.1016/j.hal.2016.03.011
Hantoro, I., Löhr, A. J., Belleghem, F. G. A. J. V., Widianarko, B., and Ragas, A. M. J. (2019). Microplastics in coastal areas and seafood: implications for food safety. Food Addit. Contam. Part A 36, 674–711. doi: 10.1080/19440049.2019.1585581
Hope, J. A., Coco, G., and Thrush, S. F. (2020). Effects of polyester microfibers on microphytobenthos and sediment-dwelling infauna. Environ. Sci. Technol. 54, 7970–7982. doi: 10.1021/acs.est.0c00514
Horn, D., Miller, M., Anderson, S., and Steele, C. (2019). Microplastics are ubiquitous on California beaches and enter the coastal food web through consumption by Pacific mole crabs. Mar. Pollut. Bull. 139, 231–237. doi: 10.1016/j.marpolbul.2018.12.039
Huppert, D. D., and Trainer, V. L. (2014). Economics of razor clam fishery closures due to harmful algal blooms in Washington State. PICES Sci. Rep. 47, 59–71.
Kedzierski, M., Lechat, B., Sire, O., Le Maguer, G., Le Tilly, V., and Bruzaud, S. (2020). Microplastic contamination of packaged meat: occurrence and associated risks. Food Packag. Shelf Life 24:100489. doi: 10.1016/j.fpsl.2020.100489
Kim, J.-S., Lee, H.-J., Kim, S.-K., and Kim, H.-J. (2018). Global pattern of microplastics (MPs) in commercial food-grade salts: sea salt as an indicator of seawater MP pollution. Environ. Sci. Technol. 52, 12819–12828. doi: 10.1021/acs.est.8b04180
Kolandhasamy, P., Su, L., Li, J., Qu, X., Jabeen, K., and Shi, H. (2018). Adherence of microplastics to soft tissue of mussels: a novel way to uptake microplastics beyond ingestion. Sci. Total Environ. 610–611, 635–640. doi: 10.1016/j.scitotenv.2017.08.053
Kroon, F. J., Motti, C. E., Jensen, L. H., and Berry, K. L. E. (2018). Classification of marine microdebris: a review and case study on fish from the Great Barrier Reef, Australia. Sci. Rep. 8:16422. doi: 10.1038/s41598-018-34590-6
Kutralam-Muniasamy, G., Pérez-Guevara, F., Elizalde-Martínez, I., and Shruti, V. C. (2020). Branded milks – Are they immune from microplastics contamination? Scie. Total Environ. 714:136823. doi: 10.1016/j.scitotenv.2020.136823
Lebreton, L. C. M., van der Zwet, J., Damsteeg, J.-W., Slat, B., Andrady, A., and Reisser, J. (2017). River plastic emissions to the world’s oceans. Nat. Commun. 8:15611. doi: 10.1038/ncomms15611
Lee, H., Kunz, A., Shim, W. J., and Walther, B. A. (2019). Microplastic contamination of table salts from Taiwan, including a global review. Sci. Rep. 9:10145. doi: 10.1038/s41598-019-46417-z
Lefebvre, K. A., Kendrick, P. S., Ladiges, W., Hiolski, E. M., Ferriss, B. E., Smith, D. R., et al. (2017). Chronic low-level exposure to the common seafood toxin domoic acid causes cognitive deficits in mice. Harmful Algae 64, 20–29. doi: 10.1016/j.hal.2017.03.003
Lepofsky, D., Smith, N. F., Cardinal, N., Harper, J., Morris, M., Elroy White, G., et al. (2015). Ancient shellfish mariculture on the Northwest Coast of North America. Am. Antiq. 80, 236–259. doi: 10.7183/0002-7316.80.2.236
Li, J., Yang, D., Li, L., Jabeen, K., and Shi, H. (2015). Microplastics in commercial bivalves from China. Environ. Pollut. 207, 190–195. doi: 10.1016/j.envpol.2015.09.018
Liebezeit, G., and Liebezeit, E. (2013). Non-pollen particulates in honey and sugar. Food Addit. Contam. Part A Chem. Anal. Control Expo Risk Assess. 30, 2136–2140. doi: 10.1080/19440049.2013.843025
Liebezeit, G., and Liebezeit, E. (2014). Synthetic particles as contaminants in German beers. Food Addit. Contam. Part A Chem. Anal. Control Expo Risk Assess. 31, 1574–1578. doi: 10.1080/19440049.2014.945099
Link, T. (2000). History and Status of Oregon’s Pacific Razor Clam Resources. Information Reports 2000-06. Astoria, OR: Oregon Department of Fish and Wildlife, 1–25.
Lusher, A., Hollman, P., and Mendoza-Hill, J. (2017). Microplastics in Fisheries and Aquaculture: Status of Knowledge on Their Occurrence and Implications for Aquatic organisms and Food Safety. FAO Fisheries and Aquaculture Technical Paper. Rome: FAO.
Mahaffey, K. R., Clickner Robert, P., and Jeffries Rebecca, A. (2009). Adult Women’s blood mercury concentrations vary regionally in the United States: association with patterns of fish consumption (NHANES 1999–2004). Environ. Health Perspect. 117, 47–53. doi: 10.1289/ehp.11674
Mahon, A. M., O’Connell, B., Healy, M. G., O’Connor, I., Officer, R., Nash, R., et al. (2017). Microplastics in sewage sludge: effects of treatment. Environ. Sci. Technol. 51, 810–818. doi: 10.1021/acs.est.6b04048
Martinelli, J. C., Phan, S., Luscombe, C. K., and Padilla-Gamiño, J. L. (2020). Low incidence of microplastic contaminants in Pacific oysters (Crassostrea gigas Thunberg) from the Salish Sea. USA. Sci. Total Environ. 715:136826. doi: 10.1016/j.scitotenv.2020.136826
Mathalon, A., and Hill, P. (2014). Microplastic fibers in the intertidal ecosystem surrounding Halifax Harbor. Nova Scotia. Mar. Pollut. Bull. 81, 69–79. doi: 10.1016/j.marpolbul.2014.02.018
Miller, R. Z., Watts, A. J. R., Winslow, B. O., Galloway, T. S., and Barrows, A. P. W. (2017). Mountains to the sea: river study of plastic and non-plastic microfiber pollution in the northeast USA. Mar. Pollut. Bull. 124, 245–251. doi: 10.1016/j.marpolbul.2017.07.028
Mintenig, S. M., Int-Veen, I., Löder, M. G. J., Primpke, S., and Gerdts, G. (2017). Identification of microplastic in effluents of waste water treatment plants using focal plane array-based micro-Fourier-transform infrared imaging. Water Res. 108, 365–372. doi: 10.1016/j.watres.2016.11.015
Miranda, M. N., Silva, A. M. T., and Pereira, M. F. R. (2020). Microplastics in the environment: a DPSIR analysis with focus on the responses. Sci. Total Environ. 718:134968. doi: 10.1016/j.scitotenv.2019.134968
Mishra, S., Rath, C. C., and Das, A. P. (2019). Marine microfiber pollution: a review on present status and future challenges. Mar. Pollut. Bull. 140, 188–197. doi: 10.1016/j.marpolbul.2019.01.039
Napper, I. E., and Thompson, R. C. (2016). Release of synthetic microplastic plastic fibres from domestic washing machines: effects of fabric type and washing conditions. Mar. Pollut. Bull. 112, 39–45. doi: 10.1016/j.marpolbul.2016.09.025
Nelms, S. E., Galloway, T. S., Godley, B. J., Jarvis, D. S., and Lindeque, P. K. (2018). Investigating microplastic trophic transfer in marine top predators. Environ. Pollut. 238, 999–1007. doi: 10.1016/j.envpol.2018.02.016
Nicholls, R. J., and Small, C. (2002). Improved estimates of coastal population and exposure to hazards released. EOS Trans. Am. Geophys. Union 83, 301–305. doi: 10.1029/2002EO000216
NOAA (2020). C-CAP Land Cover Atlas. Avaliable at: https://coast.noaa.gov/digitalcoast/tools/lca.html (accessed September 20, 2020).
NOAA Office for Coastal Management (2020). Fast Facts: Economics and Demographics. Avaliable at: https://coast.noaa.gov/states/fast-facts/economics-and-demographics.html (accessed April 4, 2020).
Phuong, N. N., Poirier, L., Pham, Q. T., Lagarde, F., and Zalouk-Vergnoux, A. (2018). Factors influencing the microplastic contamination of bivalves from the French Atlantic coast: Location, season and/or mode of life? Mar. Pollut. Bull. 129, 664–674. doi: 10.1016/j.marpolbul.2017.10.054
Pirhalla, D. E., Ransibrahmanakul, V., Clark, R., Desch, A., Wynne, T., and Edwards, M. (2009). An Oceanographic Characterization of the Olympic Coast National Marine Sanctuary and Pacific Northwest: Interpretive Summary of Ocean Climate and Regional Processes Through Satellite Remote Sensing. NOAA Technical Memorandum NOS NCCOS 90. Silver Spring, MD: NCCOS’s Coastal Oceanographic Assessments, 1–53.
Powell, E. N., Hofmann, E. E., Klinck, J. M., and Ray, S. M. (1992). Modeling oyster populations—A commentary on filtration rate—Is faster always better? J. Shellfish Res. 11, 387–398.
Primpke, S., Christiansen, S., Cowger, W., De Frond, H., Deshpande, A., Fischer, M., et al. (2020). Critical assessment of analytical methods for the harmonized and cost-efficient analysis of microplastics. Appl. Spectrosc. 74, 1012–1047. doi: 10.1177/0003702820921465
Primpke, S., Wirth, M., Lorenz, C., and Gerdts, G. (2018). Reference database design for the automated analysis of microplastic samples based on Fourier transform infrared (FTIR) spectroscopy. Anal. Bioanal. Chem. 410, 5131–5141. doi: 10.1007/s00216-018-1156-x
Provencher, J., Bond, A. L., Avery-Gomm, S., Borrelle, S. B., Rebolledo, E. L. B., Hammer, S., et al. (2017). Quantifying ingested debris in marine megafauna: a review and recommendations for standardization. Anal. Methods 9, 1454–1469. doi: 10.1039/C6AY02419J
Qu, X., Su, L., Li, H., Liang, M., and Shi, H. (2018). Assessing the relationship between the abundance and properties of microplastics in water and in mussels. Sci. Total Environ. 621, 679–686. doi: 10.1016/j.scitotenv.2017.11.284
R Core Team (2020). R: A Language and Environment for Statistical Computing. Vienna: R Foundation for Statistical Computing.
Ribeiro, F., Garcia, A. R., Pereira, B. P., Fonseca, M., Mestre, N. C., Fonseca, T. G., et al. (2017). Microplastics effects in Scrobicularia plana. Mar. Pollut. Bull. 122, 379–391. doi: 10.1016/j.marpolbul.2017.06.078
Rochman, C. M., Brookson, C., Bikker, J., Djuric, N., Earn, A., Bucci, K., et al. (2019). Rethinking microplastics as a diverse contaminant suite. Environ. Toxicol. Chem. 38, 703–711. doi: 10.1002/etc.4371
Rochman, C. M., Tahir, A., Williams, S. L., Baxa, D. V., Lam, R., Miller, J. T., et al. (2015). Anthropogenic debris in seafood: plastic debris and fibers from textiles in fish and bivalves sold for human consumption. Sci. Rep. 5:14340. doi: 10.1038/srep14340
Sechena, R., Nakano, C., Liao, S., Polissar, N., Lorenzana, R., Truong, S., et al. (1999). Asian and Pacific Islander Seafood Consumption Study, EPA 910/R-99-003. WA: EPA Office of Environmental Assessment, 1–169.
Sparks, C. (2020). Microplastics in mussels along the coast of cape town. South Africa. Bull. Environ. Contam. Toxicol. 104, 423–431. doi: 10.1007/s00128-020-02809
Su, L., Cai, H., Kolandhasamy, P., Wu, C., Rochman, C. M., and Shi, H. (2018). Using the Asian clam as an indicator of microplastic pollution in freshwater ecosystems. Environ. Pollut. 234, 347–355. doi: 10.1016/j.envpol.2017.11.075
Sussarellu, R., Suquet, M., Thomas, Y., Lambert, C., Fabioux, C., Pernet, M. E. J., et al. (2016). Oyster reproduction is affected by exposure to polystyrene microplastics. Proc. Natl. Acad. Sci. U.S.A. 113, 2430–2435. doi: 10.1073/pnas.1519019113
Teng, J., Wang, Q., Ran, W., Wu, D., Liu, Y., Sun, S., et al. (2019). Microplastic in cultured oysters from different coastal areas of China. Sci. Total Environ. 653, 1282–1292. doi: 10.1016/j.scitotenv.2018.11.057
Tong, H., Jiang, Q., Hu, X., and Zhong, X. (2020). Occurrence and identification of microplastics in tap water from China. Chemosphere 252:126493. doi: 10.1016/j.chemosphere.2020.126493
U.S. Census Bureau (2012). Washington: 2010 Population and Housing Unit Counts. 2010 Census of Population and Housing. Avaliable t: https://www2.census.gov/library/publications/decennial/2010/cph-2/cph-2-49.pdf (accessed April 8, 2020).
Van Cauwenberghe, L., and Janssen, C. R. (2014). Microplastics in bivalves cultured for human consumption. Environ. Pollut. 193, 65–70. doi: 10.1016/j.envpol.2014.06.010
von Moos, N., Burkhardt-Holm, P., and Köhler, A. (2012). Uptake and effects of microplastics on cells and tissue of the blue mussel Mytilus edulis L. after an experimental exposure. Environ. Sci. Technol. 46, 11327–11335. doi: 10.1021/es302332w
Washington Department of Health (2016). Domoic Acid in Razor Clams: Interim Health Advisory on Eating Razor Clams. Avaliable at: https://www.doh.wa.gov/CommunityandEnvironment/Shellfish/RecreationalShellfish/Illnesses/Biotoxins/DomoicAcidinRazorClams (accessed April 6, 2020).
WDFW (2020). Latest Domoic Acid Levels. Avaliable at: https://wdfw.wa.gov/fishing/basics/domoic-acid/levels (accessed April 15, 2020).
Wright, S. L., and Kelly, F. J. (2017). Plastic and human health: a micro issue? Environ. Sci. Technol. 51, 6634–6647. doi: 10.1021/acs.est.7b00423
Wyer, H. (2013). Pacific Razor clam Siliqua patula. Monterey, CA: Monterey Bay Aquarium Seafood Watch.
Xu, X.-Y., Lee, W. T., Chan, A. K. Y., Lo, H. S., Shin, P. K. S., and Cheung, S. G. (2017). Microplastic ingestion reduces energy intake in the clam Atactodea striata. Mar. Pollut. Bull. 124, 798–802. doi: 10.1016/j.marpolbul.2016.12.027
Zhang, H. (2017). Transport of microplastics in coastal seas. Estuar. Coast. Shelf Sci. 199, 74–86. doi: 10.1016/j.ecss.2017.09.032
Zhang, Q., Xu, E. G., Li, J., Chen, Q., Ma, L., Zeng, E. Y., et al. (2020). A review of microplastics in table salt, drinking water, and air: direct human exposure. Environ. Sci. Technol. 54, 3740–3751. doi: 10.1021/acs.est.9b04535
Keywords: microplastic, microfiber, Olympic Coast, Washington, bivalve, razor clam, Siliqua patula
Citation: Baechler BR, Granek EF, Mazzone SJ, Nielsen-Pincus M and Brander SM (2020) Microplastic Exposure by Razor Clam Recreational Harvester-Consumers Along a Sparsely Populated Coastline. Front. Mar. Sci. 7:588481. doi: 10.3389/fmars.2020.588481
Received: 29 July 2020; Accepted: 23 October 2020;
Published: 30 November 2020.
Edited by:
Juan José Alava, The University of British Columbia, CanadaReviewed by:
Gabriela Verónica Aguirre-Martínez, Universidad Arturo Prat, ChileOlgaç Güven, Akdeniz University, Turkey
Copyright © 2020 Baechler, Granek, Mazzone, Nielsen-Pincus and Brander. This is an open-access article distributed under the terms of the Creative Commons Attribution License (CC BY). The use, distribution or reproduction in other forums is permitted, provided the original author(s) and the copyright owner(s) are credited and that the original publication in this journal is cited, in accordance with accepted academic practice. No use, distribution or reproduction is permitted which does not comply with these terms.
*Correspondence: Britta R. Baechler, YmFlY2hsZXJAcGR4LmVkdQ==