- 1Department of Fisheries and Bioeconomics, Arctic University of Norway, Tromsø, Norway
- 2Department of Marine Ecosystem Acoustics, Institute of Marine Research, Bergen, Norway
- 3Institute of Marine Research, Fram Centre – High North Research Centre for Climate and Environment, Tromsø, Norway
- 4Department of Arctic and Marine Biology, Arctic University of Norway, Tromsø, Norway
- 5Akvaplan-niva, Fram Centre – High North Research Centre for Climate and the Environment, Tromsø, Norway
To achieve effective management and understanding of risks associated with increasing anthropogenic pressures in the ocean, it is essential to successfully and efficiently collect data with high spatio–temporal resolution and coverage. Autonomous Underwater Vehicles (AUVs) are an example of technological advances with potential to provide improved information on ocean processes. We demonstrate the capabilities of a low-power AUV buoyancy glider for performing long endurance biological and environmental data acquisition in Northern Norway. We deployed a passive acoustic sensor system onboard a SeagliderTM to investigate presence and distribution of cetaceans while concurrently using additional onboard sensors for recording environmental features (temperature, salinity, pressure, dissolved oxygen, and chlorophyll a). The hydrophone recorded over 108.6 h of acoustic data during the spring months of March and April across the continental shelf break and detected both baleen and odontocete species. We observed a change in cetacean detections throughout the survey period, with humpback whale calls dominating the soundscape in the first weeks of deployment, coinciding with the migration toward their breeding grounds. From mid-April, sperm whales and delphinids were the predominant species, which coincided with increasing chlorophyll a fluorescence values associated with the spring phytoplankton blooms. Finally, we report daily variations in background noise associated with fishing activities and traffic in the nearby East Atlantic shipping route. Our results show that gliders provide excellent platforms for collecting information about ecosystems with minimal disturbance to animals, allowing systematic observations of our ocean biodiversity and ecosystem dynamics in response to natural variations and industrial activities.
Introduction
In future decades, the highly productive Norwegian Sea is expected to experience an increase in anthropogenic activity (Gjøseter et al., 2010; Olafsen et al., 2012), accompanied by increased water temperature (ICES, 2010a,b), a shift in timing of the phytoplankton bloom (Rey, 2004), a decrease in the general zooplankton biomass (Huse et al., 2012), and changes in some of the most important commercial pelagic fish species’ stock density and biomass distribution (Skjoldal, 2004; ICES, 2010b, 2011; Utne et al., 2012). As concerns for the effects of sea exploitation and climate change continue to increase, the potentially conflicting stressors require integrated management (Suberg et al., 2014). Species distribution and behavior provide indicators of ecosystem health, revealing patterns of change in biological communities, associated with alterations in physical conditions and processes. Changes in the quality, phenology, and abundance of key prey species may constrain efficient transfer of marine primary and secondary production to higher trophic levels, such as cetaceans, which will result in cascading implications for entire food webs (Lauria et al., 2012; Sydeman et al., 2013; Silber et al., 2017).
Several cetacean species occur along the coast of Northern Norway, including resident and seasonally migrating species. North Atlantic humpback whales (Megaptera novaeangliae) have been reportedly present from late October to early February in northern Norway, coinciding with overwintering Norwegian Spring Spawning (NSS) herring (Jourdain and Vongraven, 2017). Male sperm whales (Physeter macrocephalus), which inhabit continental slope areas from northern Norway to Svalbard (Øien, 2009) year around, can act as an indicator species for significant alterations in the inaccessible deep-sea environment (Steiner et al., 2012). Sperm whales are more predominantly present in deep-water canyons north of 65° N during the summer months. However, only few surveys performed in the summer have reported sperm whales south of the Arctic Circle. Smaller cetaceans also occur frequently along the Norwegian coast, including orcas (Orcinus orca), long-finned pilot whales (Globicephala melas), minke whales (Balaenoptera acutorostrata), white-beaked dolphins (Lagenorhynchus albirostris), and harbor porpoises (Phocoena phocoena). The distribution of these species appears to be linked to prey distribution along the Norwegian coast, though there is little knowledge on other drivers for their seasonal presence and regional migrations (e.g., NAMMCO, 2019). Fin whales have also been recorded in the vicinity of the survey area (Øygard, 2018), perhaps representing a small subset of the population that remains outside the coast of Norway during winter and spring (Haug, 1998). However, it is believed that the whales mostly visit this region during migrations to and from feeding areas.
In marine mammal research, the deployment of Passive Acoustic Monitoring (PAM) sensors has been highlighted as one useful approach to overcoming the limitations of visual observations (Cauchy et al., 2020). Marine mammals, and particularly cetaceans, are challenging to survey, as they spend a large portion of their time vocalizing under water. Thus, sensors capable of recording such vocalizations to document animal presence and behavior provide additional information that could not be otherwise obtained from the surface. Also, PAM systems can be deployed for extended periods and operate continuously irrespective of weather and light conditions, which can severely limit visual observations. Passive Acoustic Monitoring tools also provide measures of background sounds of biotic and abiotic origins.
Ocean gliders are autonomous underwater vehicles (AUVs) developed for the purpose of marine surveillance. They provide high resolution (sensor dependent) hydrographic profiles and perform long duration missions, unaffected by extreme weather events (Rudnick, 2016; Cauchy et al., 2018). The potential for glider deployments with PAM systems allows for data collection through varying water masses and for long periods of time. More recently, such sensors have been integrated onto gliders that can measure presence, abundance, and distribution, of higher trophic level organisms, e.g., fish and cetaceans (Baumgartner and Fratantoni, 2008; Ferguson et al., 2010; Klinck et al., 2012; Meyer-Gutbrod et al., 2012; Baumgartner et al., 2013; Marques et al., 2013; Send et al., 2013; Suberg et al., 2014; Cauchy et al., 2020). However, only a few studies have focused on deployments with simultaneous measurements of physical and biological components using gliders (e.g., Suberg et al., 2014; Benoit-Bird et al., 2018), particularly in terms of monitoring higher trophic organisms such as cetaceans. The purpose of this work is to show the capabilities of gliders in oceanic deployments and investigate cetacean ecology and background noise, while concurrently recording seawater characteristics and lower trophic-level features. By shedding light on a new aspect of glider surveys in integrating biotic and abiotic features, we provide an additional perspective to cetacean occurrence patterns in northern Norway.
Materials and Methods
We describe the timing of whale vocalizations collected by a SeagliderTM (Hydroid Inc.) AUV as it transitions through coastal shelf and shelf edge, and explore the ecological and physical context within which these vocalizations occur. This type of glider operates by moving through the water column in a saw-tooth motion induced by changes in buoyancy. The glider scanned the marine environment by diving up and down the water column, concurrently collecting data through its onboard sensors (see section “Seaglider Survey”). It is equipped with a suite of sensors that collect data while the glider is submerged and transmits these data during each surfacing period. The glider was equipped with multiple sensors, sampling at specific time resolutions (see section “Seaglider survey”).
Survey Area
The SeagliderTM operated in Northern Norway, between 68°N and 69°N (Figure 1) in the southern end of the Lofoten-Vesterålen (LoVe) region. This area is rich in fisheries resources and is a target for oil and gas exploration. The LoVe archipelago also has an important fishing and whale-watching tourism industry. The area therefore experiences intense maritime traffic as well as periodically occurring seismic explosions, which may affect local ecosystems.
Seaglider Survey
The SeagliderTM was deployed on March 15 and was recovered on May 1, 2018. A pre-programmed survey track was designed to cover both the continental shelf and the shelf break. The glider was equipped with a JASCO AMAR G4 hydrophone (JASCO Applied Sciences Ltd.) to detect and record cetacean vocalizations and other underwater noise, operating continuously during periods of 10 min during descent to 200 m depth. This was done in order to avoid the pump motor noise on ascent and preserve battery power. The hydrophone recording unit had a sampling frequency of 128 kHz and was mounted in the glider’s aft wet space close to the buoyancy bladder, with the hydrophone on top of the glider just in front of the rudder. When at the surface, the glider would report its position through Iridium satellite and relay systems operation data and sensor data. Glider position data are used to allocate individual hydrophone data files correctly to time, depth and geographic position. The glider was also equipped with a CTD (SeaBird GPCTD), along with oxygen (Contros Hydroflash Optode) and fluorometer (SeaBird SeaOWL UVA) sensors, to measure physical properties of the water column and chlorophyll a, respectively, for every minute of operation. Dive duration was dependent on the location surveyed, with shorter dives in shallow waters and longer in deeper waters. On-shelf and shelf break sampling were occasionally interrupted to ensure sufficient battery longevity to cover the entire survey period. All acoustic data were stored internally as WAV files and recovered at the end of the deployment period.
Analyses
Passive Acoustic Monitoring data files were processed for marine mammal presence and species identification, using RavenPro 1.5 (Cornell Lab of Ornithology, Ithaca, NY, United States), relying on a Hamming window, 4096 DFT size, 7.81 Hz grid spacing, with 60% overlap for humpback whales; Hamming window, 2048 DFT size, 125 Hz grid spacing, with 70% overlap for delphinids and sperm whales; and Hann window, 2048 DFT size, 0.98 Hz grid spacing, with 67% overlap for fin whales. Based on calibration data provided by Kongsberg Maritime (calibration performed by GTI prior to deployment), a system sensitivity correction factor for the frequency band of interest was estimated to −165.1 dB. The hydrophone sensitivity was found to vary less than 1 dB below 10 kHz (+0.6 to −0.2 dB). We created a time series for species presence/absence throughout the sampling period (March 15 to April 26) and area, thus investigating migratory patterns timing and detection location. Additionally, we describe variations in water temperature, salinity, and chlorophyll a levels along the entire depth coverage of the SeagliderTM track. We categorized whale detections into different classes for different species (see Table 1) and removed all missing values from the dataset. We then applied a generalized mixed model, using R package glmmTMB (Brooks et al., 2017), to explore possible effects of glider depth, time of day and time of year, and survey section (see Figure 1) on our observations of whale occurrence. Given that above the Arctic Circle at this time of the year, the maximum sun angle at noon increases rapidly each day, we account for this relationship between date and sun elevation by including both daily maximum sun elevation and the period of the day when the recordings took place (day, night, dawn, dusk). We thus tested the effect of glider depth, maximum sun elevation and time of day (fixed effects) against humpback and fin whale occurrence. We limited this analysis to humpback and fin whales due to their distinctively seasonal migratory pattern and likely long vocalization range. Odontocetes, on the other hand, may be more prone to the influence of prey distribution and most likely have a less distinct migratory pattern at this time of year, so we used survey area rather than maximum sun elevation in their respective models. By separating survey period and location we also account for collinearity between the two variables (space and time).
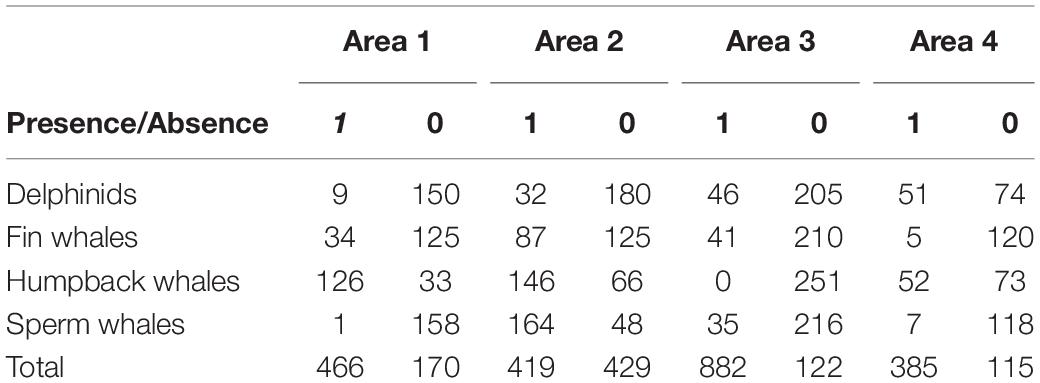
Table 1. Number of files with species presence/absence (Presence: 1, Absence: 0) recorded between March 15 and April 26, 2018 per area surveyed on the shelf (Areas 1, 3, and 4) and shelf edge (Area 2).
The relationship between species’ occurrences and depth was found to be occasionally curvilinear, and we therefore included glider depth in the model with a quadratic term for humpback and sperm whales (hence, linear term for the remaining species). Additionally, to correct for the possible influence of the thermocline on sound propagation at different depths, we included the glider location in relation to the depth of the thermocline for each glider dive (above, crossing, below) as a random effect in each model. The thermocline was calculated by first identifying a dive using the R package diveMove (Luque, 2007), and then using the clined function of the castr package (Irisson, 2020) to estimate the respective depth value for each dive. We dealt with temporal autocorrelation resulting from the transitory nature of glider surveys by implementing an autoregressive correlation error structure (AR 1) into each model. The autocorrelation in the models was designated by the dive id and date (day of the year, grouping parameter) to account for variability within dives occurring within the same day.
Seagliders are acoustically quiet platforms, however there are periods of intermittent platform noise due to the altimeter, buoyancy pump, and pitch/roll regulation, which needs to be identified and removed prior to analysis. All analyses were performed in Matlab (MATLABTM version 2019b, Mathworks, Inc.). Spectrogram correlation was used to identify different types of self-noise throughout the data set and remove them from original wave files. In addition, the first minutes of each dive was removed, where the SeagliderTM was at the surface prior to descent. A new data set was created without SeagliderTM self-noise and concatenated to files of minimum 60 s duration. The new data set was manually scrutinized to ensure that all significant self-noise events were removed. The data set was resampled to 32 kHz and noise was analysed from 10 Hz to 10 kHz. The hydrophone is not expected to have a flat response below 20 Hz, and significant hydrodynamic noise is likely present in the dataset below 20 Hz. Acoustic power spectral density (PSD) was calculated using a 1-second Hann window, no padding or overlap, and spectral levels and corresponding probability distribution from 10 Hz to 10 kHz were calculated.
We investigated the potential interference of background noise on the species groups detected by incorporating band Sound Pressure Level (SPL) values into a separate model in order to avoid overparameterization of the original model for each species. The bands used reflected noise derived from ship traffic, turbulence, and other biological activity, occurring within the frequency range of vocalizations for the species groups in this study (50–1500 Hz, 1500–10,000 Hz, and 50–10,000 Hz for baleen whales, sperm whales, delphinids, respectively). Removal of periods of substantial glider self-noise meant that SPL levels could not be calculated for some sound files, which therefore had to be removed from statistical analyses. Though fin whales vocalize below 50 Hz (e.g., 20-Hz call) where there is also often ocean traffic noise, we set this as the lower limit for pressure level calculations due to the likely effects of flow noise and unknown hydrophone response at lower frequencies. The model was thus specified with whale occurrence as a function of the SPL band levels with the same covariance structure as above, though without the random effects for thermocline.
All statistical analyses were conducted using R software (version 4.0.1). Model selection was based on Akaike Information Criterion (AIC), and using the dredge function of the MuMIn package for R (Bartoń, 2019).
Results
Over the course of the two months of deployment, the glider performed a total of 284 dives (each dive comprising of one up and downcast), covering a total of 800 km (horizontal distance derived from GPS fixes). This resulted in over 108.6 h of hydrophone data, and 58496 fluorescence, CTD, and oxygen measurements. A total of 529 acoustic files contained whale vocalizations. We placed the detections in a time context to assess possible whale migration in the region and possible habitat selection, while estimating background noise levels for the same time period (Figure 2).
PAM Detections
The number of files with different species at the different survey areas is described in Table 1. We observed humpback whale detections throughout the entire spring, until late April both on the shelf and shelf edge. Sperm whales, on the other hand, were mostly detected at the opening and within a 400 m underwater canyon (Figure 1, bottom left, Area 2), coinciding with their preferred foraging habitat. The presence of delphinids was more sporadic, though with a more pronounced occurrence on shelf during the last week of survey. Fin whales were also present in the recordings, with a considerable presence in late March, early April, which gradually decreased as the glider moved from the shelf edge, toward the end of the sampling period.
Results from the best fit model showed that daily maximum sun elevation has a negative effect on the probability of occurrence of humpback and fin whales, indicating that the whales were more likely to be detected at the beginning of the survey. Humpback whales were the only species for which glider depth was found to have a considerable effect, suggesting that this species was more likely to be detected at shallower depths. No species were found to be affected by period of the day. See Tables 2, 3 for model output summary (further detail for model output including random effects in Supplementary Tables 1, 2).
For the various survey areas, we found that sperm whales are more likely to be found at the shelf edge. The same was not evident for delphinids, However, there was a considerable positive effect of the last survey section (area 4). See Tables 4, 5 for model output summary (details for model estimates with random effects in Supplementary Tables 3, 4).
Background Noise
Spectral levels from 10 Hz to 10 kHz over the duration of the glider mission is shown in Figure 3 (top) along with the corresponding spectral probability distribution (Figure 3 (bottom), SPD, Merchant et al., 2013). The spectrogram shows long periods of wideband noise associated with wind and rain (Figure 3a), turbulent flow noise (Figure 3c), and acoustic emissions from nearby vessels (Figure 3d) occurring as wideband noise and discrete harmonic banding. Periods of signals from seismic sources, and marine mammals with the exception of fin whales (Figure 3b), are not clearly seen in the figure.
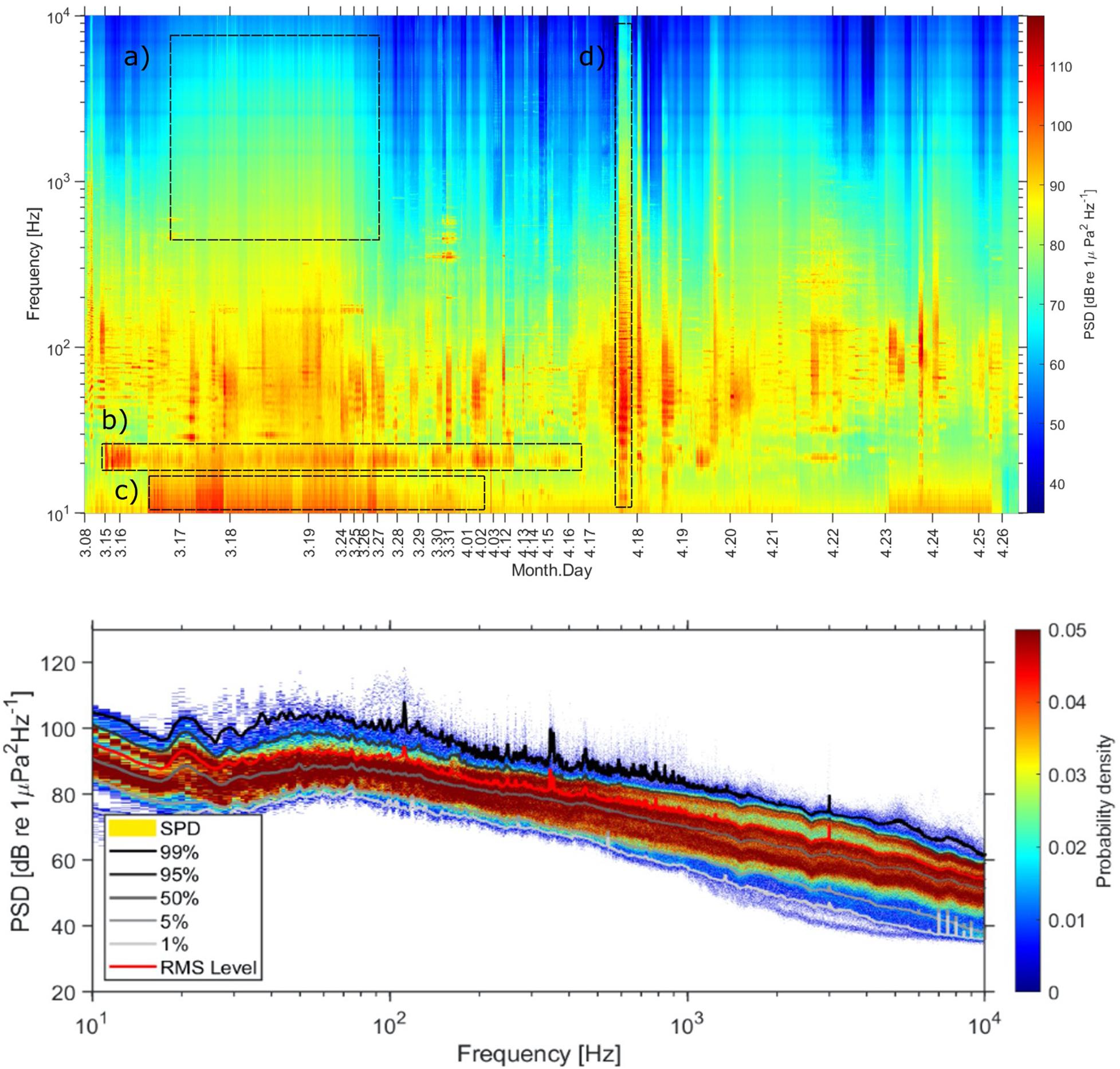
Figure 3. Glider spectrogram (PSD, 1s Hann window, 60 s average) (top) and spectral probability distributions (SPD) of acoustic energy (bottom). The different boxes indicate examples of the different sound sources; (a) wind and rain, (b) fin whale, (c) turbulent flow noise, (d) vessel in close proximity.
The analysis of underwater noise effects on our detections of marine mammals revealed that detections of sperm whales and delphinids are affected by noise levels in the 1500–10,000 Hz band (P = 0.016, P < 0.001, respectively. See Supplementary Tables 5, 6). For humpback and fin whales however, the model output showed no effect, which is likely due to the strong effect whale vocalizations have in the noise band between 50 to 1500 Hz (Humpback whales: P = 0.22; Fin whales: P = 0.740) and 1500–10,000 Hz (Humpback whales: P = 0.64; Fin whales: P = 0.359). See Supplementary Tables 7, 8 for further details on model output.
Physical Environment
The SeagliderTM predominantly sampled thermally stratified waters during its mission. In CTD measurements, a strong thermocline at 20–40 m depth was recorded (Figure 4). This was particularly evident in mid-March, when the SeagliderTM remained on the continental shelf. The deeper and longer dives performed off the continental shelf from the end of March did not allow for a clear distinction of water layers above 250 m during this period. However, we did observe changes in the salinity content at the end of the deployment (Figure 4), with lower concentrations at the continental shelf within a small 400-m deep underwater canyon. Levels of chlorophyll a fluorescence were more pronounced during April (Figure 4), nearly a month after the equinox (March 20, 2018), which is usually taken as the beginning of the primary production period in more southern latitudes.
Discussion and Conclusion
Ocean gliders are efficient and quiet platforms for measuring changes in the marine environment through PAM of marine mammals, with the additional capability of profiling the water column and providing sound speed profiles valuable for underwater sound propagation modeling. The use of technological advances equipped with multiple sensors provides a unique view of the marine environment, and allows for improved understanding of marine animal ecology.
Temporal Variation of PAM Detections
In this study, we recorded humpback whales on the shelf and shelf edge, indicating that they tend to remain close to the shelf slope on their way to the breeding grounds. Though our analyses show that this species was more likely to be encountered on the continental shelf, prior to reaching the slope, these whales were detected until late April. This indicates that humpback whales remain in Norwegian waters longer than previously assumed (Broms et al., 2015; Ryan et al., 2015), likely foraging on herring. The assumption that the Norwegian Sea is a migratory corridor for this species (Jourdain and Vongraven, 2017) is therefore not valid, as such late departure from foraging grounds ultimately indicates either a delay in arrival at breeding grounds or that these animals may remain in foraging areas for periods when higher energetic reserves are required. Though our study represents a few months of a single year of whale acoustic behavior, sporadic observations reported on social media channels validate this finding (e.g., Bergersen et al., n.d.) across multiple years.
The recordings of sperm whale click at the opening of a small canyon and shelf edge represents foraging activity outside known locations above the Arctic Circle. Sperm whales in Norway appear to remain in foraging hotspots from May to September, though may also be present at those same areas during the winter (Rødland and Bjørge, 2015). The social structure of sperm whales is, however, poorly understood in the region and it is likely that bachelor groups disperse to other less-productive areas to avoid competition by larger males, or the survey region is a pit-stop on their way to better grounds.
Though we describe the surrounding environment through CTD and fluorometer data, we were unable at this stage to estimate detection ranges to the whales from the glider and assess spatial overlap. This was due to the fact that such estimates would require either triangulation for animals’ geographical position or assumptions in species’ source levels and calculations of sound propagation, which were beyond the scope of this work. Nevertheless, our analyses reveal variations in marine mammal detections throughout the sampling season, which may reflect changes in behavior and detectability. Levels of chlorophyll a fluorescence was more pronounced during April (Figure 4) and coincided with a higher rate of detections of delphinids and sperm whales though the opposite was observed for humpback and fin whales. Increases in daily sun elevation had a negative significant effect on the probability of obtaining either a humpback of fin whale presence, indicating that at the time of our survey, these species were likely migrating away from the survey region.
Spatial Variation of PAM Detections
The different sections of survey locations (survey area) were chosen based on the performed task of the glider (in transect or not) and on the actual location in relation to the coast to reflect different bathymetric features. Sperm whales and delphinids were found to be associated to different areas; Sperm whales were more likely to be close to the shelf edge, while delphinids were mainly associated with regions closer to the coast. Glider depth, i.e., recording depth, was only found to be significant for humpback whales, which may indicate a higher rate of surface vocalizations even though we corrected the effect of thermocline depth in our model as a random effect. There was no statistical evidence to suggest time of day (day, night, dawn and dusk) had any effect on the detection of any species. This contradicts previous studies on fin whale vocal activity (e.g., Víkingsson, 1997; Simon et al., 2010). We believe that this is mainly a result of different model structure, as we accounted for variability in both random effects and therefore temporal autocorrelation structure (by including glider dive and Julian day as random effects), which is necessary and relevant when investigating animal presence with temporal relationships.
The combined effect of spatio-temporal changes occurring as the glider progresses along the track can raise several questions as to the relevance of some of the variations observed in whale detections. This study shows a nearly two-month snapshot of whale detections in different areas, though the sampled areas were not revisited. Though some of the species identified are notoriously known for their migratory behavior, for which the space-time issue may be considerable, others (sperm whales and delphinids) seem to confirm known habitat preferences (Whitehead, 2018; Jourdain et al., 2019; NAMMCO, 2019). However, future glider endeavors are advised to sample the same study regions multiple times to allow clarity in measured variations across different habitats.
Background Noise
The noise spectrum is dominated by noise due to weather and near and distant vessels, with periods of seismic surveying also observed. Storms are common in this area during spring, and apparent in the dataset as broadband wind generated noise persisting for days. It has been shown that in shallow regions on-shelf Northern Norway the noise levels due to wind at lower frequencies (<400 Hz) are higher than expected from traditional Wenz curves (Ødegaard et al., 2019). The SeagliderTM operated in the area around the continental shelf break with the major north-south shipping lane for large commercial vessels, which is apparent in the data set as broadband and tonal noise. The SeagliderTM performed well for underwater noise measurements, however, self-noise (buoyancy pump, pitch/roll adjustment, altimeter) needs to be removed from the data set prior to analysis. In shallow water areas, and areas with high and variable current, such as on the continental shelf, the rate of self-noise is higher than off-shelf as the vehicle needs to use the buoyancy pump, altimeter and perform pitch/roll compensation more frequently. In addition, turbulent flow around the hydrophone results in flow noise proportional to the speed of the glider, particularly visible bellow 20 Hz, but likely present in the 5–50 Hz frequency band (dos Santos et al., 2016; Cauchy et al., 2018). This noise increases significantly above approximately 30 cm/s absolute velocity, indicating that for (a) reliable noise measurements at low frequencies (<50 Hz) and (b) high signal-to-noise ratio (SNR) for marine mammal detection at low frequencies, the glider should be operated with a low glide velocity when possible. The analysis of underwater noise revealed that detections of sperm whales and delphinids are affected by noise levels in the 1500–10,000 Hz band. Such results can originate from multiple factors, such as masking (i.e., the noise levels overlap with the whales’ calls and these are no longer available for detection), animal behavior, and/or the spatio-temporal distribution of the glider in relation to these species. Naturally, such effects would require more detailed and controlled studies, dedicated to estimating the effects of background noise on marine mammal species.
Though marine mammals are often challenging to survey, this study shows that the use of autonomous PAM systems provides new insights on animal distribution that would otherwise be difficult to obtain in the Norwegian Sea. Though the pump and pitch/roll adjustment created noise during certain operations, the gliders were mainly silent throughout the entire survey. This allowed for very clear detections of marine mammal vocalizations. Detailed analysis of the background noise and potential consequences for marine mammal detection showed that odontocetes seem to be negatively affected by increasing noise levels in the 1500–10,000 Hz frequency band. However, it is worth noting that the same animal groups vocalize within this range and the results could also reveal effects of co-occurrence rather than background noise. Due to the fact that it was not possible at this stage to discard all animal vocalizations from the analyses, we only included in the analyses bands that represented a wide range of frequencies so that the assessment of potential effect would be more likely due to background noise rather than whale vocalizations.
Gliders are robust in poor sea conditions and their low power requirements allow them to remain at sea for several months. The use of oceanic autonomous platforms to investigate changes in both time and space represents therefore a great technological advance that can improve ecosystem-based management and conservation decisions. Advances in glider-based technology and its applications ultimately lead to improved long-term ecological monitoring over finer spatial scales and longer temporal scales. By conducting reproducible and multidisciplinary (combining physics, ecology, and engineering) sampling, glider-based studies provide systematic observations that can address questions concerning whale behavior and ecology, which are of high importance given the current pressures to natural marine environments.
Data Availability Statement
The raw data supporting the conclusions of this article will be made available upon request to the authors, without undue reservation.
Ethics Statement
Ethical review and approval was not required for the animal study because the subjects were not under a protocol of experimentation. The purpose of this study was to survey animals in their natural habitat, with minimal disturbance to their natural behavior.
Author Contributions
AA, GP, and LC conceptualized and designed this study with input of RP, UL, and MB. LC procured funding and conducted the field experiment with the support of GP. This study took place within the frame of the projects “Spatial and temporal analyses of marine mammal vocalizations using unmanned systems” led by ASA and the GLIDER project led by LC. AA performed the statistical analysis of the data, while GP performed analysis on background noise. UL provided estimates of sun elevation. ASA and GP led the writing of the manuscript with input of RP, MB, UL, and LC. All authors approved the submitted version.
Funding
Funding for this work was provided by a postdoc scholarship from VISTA – a research program in collaboration between the Norwegian Academy of Sciences and Letters and Equinor. Instrumentation, field deployment, data acquisition, and background noise analyses were supported by the GLIDER project, which was funded by the DEMO2000 research program (Norwegian Research Council and ConocoPhillips, project 269188, “Unmanned ocean vehicles, a flexible and cost-efficient offshore monitoring and data management approach”).
Conflict of Interest
The authors declare that this study received partial funding from ConocoPhillips. This funder was not involved in the study design, collection, analysis, interpretation of data, the writing of this article or the decision to submit it for publication.
Acknowledgments
The authors thank the field crew of the SeagliderTM and Dan Hayes (from Cyprus Subsea Consulting & Services) for operating the glider. Many thanks to Heidi Ahonen and Claudia Vieira for assisting in deciphering the identification of challenging whale vocalizations. Finally, we thank the ARCTOS Network for providing an environment of constructive discussions.
Supplementary Material
The Supplementary Material for this article can be found online at: https://www.frontiersin.org/articles/10.3389/fmars.2020.585754/full#supplementary-material
Supplementary Table 1 | Conditional glmmTMB model random effects (html with results per dive) Humpback whales.
Supplementary Table 2 | Conditional glmmTMB model random effects (html with results per dive) Fin whales.
Supplementary Table 3 | Conditional glmmTMB model random effects (html with results per dive) Sperm whales.
Supplementary Table 4 | Conditional glmmTMB model random effects (html with results per dive) Delphinids.
Supplementary Table 5 | SPL glmmTMB model Humpback Whales.
Supplementary Table 6 | SPL glmmTMB model Fin whales.
Supplementary Table 7 | SPL glmmTMB model Sperm whales.
Supplementary Table 8 | SPL glmmTMB model Delphinids.
References
Bartoń, K. (2019). MuMIn: Multi-Model Inference. R package version 1.43.15. Available online at: https://cran.r-project.org/package=MuMIn (accessed on April, 15 2020).
Baumgartner, M. F., and Fratantoni, D. M. (2008). Diel periodicity in both sei whale vocalization rates and the vertical migration of their copepod prey observed from ocean gliders. Limnol. Oceanogr. 53, 2197–2209. doi: 10.4319/lo.2008.53.5_part_2.2197
Baumgartner, M. F., Fratantoni, D. M., Hurst, T. P., Brown, M. W., Cole, T. V. N., Van Parijs, S. M., et al. (2013). Real-time reporting of baleen whale passive acoustic detections from ocean gliders. J. Acoust. Soc. Am. 134:1814. doi: 10.1121/1.4816406
Benoit-Bird, K. J., Patrick Welch, T., Waluk, C. M., Barth, J. A., Wangen, I., McGill, P., et al. (2018). Equipping an underwater glider with a new echosounder to explore ocean ecosystems. Limnol. Oceanogr. Methods 16, 734–749. doi: 10.1002/lom3.10278
Bergersen, E., Aniceto, A. S., Berg, E., Beuchel, F., Karlsen, T. I., and Eide, B. (n.d.). hvaler i nord. Available online at: https://www.facebook.com/groups/hvalerinord/
Broms, F., Wezel, F., López Suárez, P., Stevick, P., Biuw, M., Jann, B., et al. (2015). “Recent research on the migratory destinations of humpback whales (Megaptera novaeangliae) from a mid-winter feeding stop-over area in Northern Norway,” in Recent research on the migratory destinations of humpback whales, ed. F. W. Wenzel (St. Juliens, Malta: European Cetacean Society Special Publication).
Brooks, M. E., Kristensen, K., van Benthem, K. J., Magnusson, A., Berg, C. W., Nielsen, A., et al. (2017). glmmTMB balances speed and flexibility among packages for zero-inflated generalized linear mixed modeling. R J. 9:378. doi: 10.32614/rj-2017-066
Cauchy, P., Heywood, K. J., Merchant, N. D., Queste, B. Y., and Testor, P. (2018). Wind speed measured from underwater gliders using passive acoustics. J. Atmos. Ocean. Technol. 35, 2305–2321. doi: 10.1175/JTECH-D-17-0209.1
Cauchy, P., Heywood, K., Risch, D., Merchant, N., Queste, B., and Testor, P. (2020). Sperm whale presence observed using passive acoustic monitoring from gliders of opportunity. Endanger. Species Res. 42, 133–149. doi: 10.3354/esr01044
dos Santos, F. A., São Thiago, P. M., de Oliveira, A. L. S., Barmak, R., Lima, J. A. M., de Almeida, F. G., et al. (2016). Investigating flow noise on underwater gliders acoustic data. J. Acoust. Soc. Am. 140, 3409–3409. doi: 10.1121/1.4970954
Ferguson, B. G., Lo, K. W., and Rodgers, J. D. (2010). “Sensing the underwater acoustic environment with a single hydrophone onboard an undersea glider,” in OCEANS’10 IEEE Sydney, OCEANSSYD 2010, (Sydney, NSW: IEEE), doi: 10.1109/OCEANSSYD.2010.5603889
Gjøseter, H., Haug, T., Hauge, M., Karlsen, Ø., Knutsen, J. A., Røttingen, I., et al. (2010). Fisken og Havet. Bergen: Fiskeridirektoratets havforskningsinstitutt.
Huse, G., Holst, J. C., Utne, K., Nøttestad, L., Melle, W., Slotte, A., et al. (2012). Effects of interactions between fish populations on ecosystem dynamics in the Norwegian Sea – results of the INFERNO project. Mar. Biol. Res. 8, 415–419. doi: 10.1080/17451000.2011.653372
ICES (2011). Report of the Working Group on Widely Distributed Stocks (WGWIDE), 23−29 August 2011. Copenhagen: ICES.
Irisson, J.-O. (2020). castr: Process CTD casts. Available online at: https://rdrr.io/github/jiho/castr/man/d.html.
Jourdain, E., and Vongraven, D. (2017). Humpback whale (Megaptera novaeangliae) and killer whale (Orcinus orca) feeding aggregations for foraging on herring (Clupea harengus) in Northern Norway. Mamm. Biol. 86, 27–32. doi: 10.1016/j.mambio.2017.03.006
Jourdain, E., Ugarte, F., Víkingsson, G. A., Samarra, F. I. P., Ferguson, S. H., Lawson, J., et al. (2019). North Atlantic killer whale Orcinus orca populations: a review of current knowledge and threats to conservation. Mamm. Rev. 49, 384–400. doi: 10.1111/mam.12168
Klinck, H., Mellinger, D. K., Klinck, K., Bogue, N. M., Luby, J. C., Jump, W. A., et al. (2012). Near-real-time acoustic monitoring of beaked whales and other cetaceans using a SeagliderTM. PLoS One 7:e36128. doi: 10.1371/journal.pone.0036128
Lauria, V., Attrill, M. J., Pinnegar, J. K., Brown, A., Edwards, M., and Votier, S. C. (2012). Influence of Climate Change and Trophic Coupling across Four Trophic Levels in the Celtic Sea. PLoS One 7:e47408. doi: 10.1371/journal.pone.0047408
Marques, T. A., Thomas, L., Martin, S. W., Mellinger, D. K. D. K., Ward, J. A., Moretti, D. J., et al. (2013). Estimating animal population density using passive acoustics. Biol. Rev. Camb. Philos. Soc. 88, 287–309. doi: 10.1111/brv.12001
Merchant, N. D., Barton, T. R., Thompson, P. M., Pirotta, E., Dakin, D. T., and Dorocicz, J. (2013). Spectral probability density as a tool for ambient noise analysis. J. Acoust. Soc. Am. 133, EL262–EL267. doi: 10.1121/1.4794934
Meyer-Gutbrod, E., Greene, C. H., Packer, A., Dorn, H., and Griffith, J. (2012). Long term autonomous fisheries survey utilizing active acoustics. in OCEANS 2012 MTS/IEEE: Harnessing the Power of the Ocean. Hampton Roads, VA: IEEE, doi: 10.1109/OCEANS.2012.6405100
NAMMCO (2019). Report of the Abundance Estimates Working Group. Tromsø, Norway. Tromsø: North Atlantic Marine Mammal Commission. Available online at: https://nammco.no/topics/abundance_estimates_reports/.
Ødegaard, L., Pedersen, G., and Johnsen, E. (2019). “Underwater Noise From Wind At the High North Love Ocean Observatory,” in Proceeding of the UACE 2019 Conf, (Canberra: Universities Australia Conference), 359–366.
Øien, N. (2009). Distribution and abundance of large whales in Norwegian and adjacent waters based on ship surveys 1995–2001. NAMMCO Scientific Publications 7, 31–47. doi: 10.7557/3.2704
Olafsen, T., Winther, U., Olsen, Y., and Skjermo, J. (2012). Verdiskaping basert på produktive hav i 2050. Available at online at: https://www.sintef.no/globalassets/upload/fiskeri_og_havbruk/publikasjoner/verdiskaping-basert-pa-produktive-hav-i-2050.pdf. 1–76.
Øygard, S. H. (2018). “Simulations of acoustic transmission loss of Fin whale calls reaching the LoVe Ocean Observatory,” in 42th Scandinavian Symposium on Physical Acoustics, Geilo, Norway, Jan. 27–30, 2019, (Bergen: The University of Bergen).
Rey, F. (2004). “Phytoplankton: the grass of the sea,” in The Norwegian Sea Ecosystem, ed. H. R. Skjoldal (Trondheim: Academic Press), 97–136.
Rødland, E. S., and Bjørge, A. (2015). Residency and abundance of sperm whales (Physeter macrocephalus) in the Bleik Canyon. Norway. Mar. Biol. Res. 11, 974–982. doi: 10.1080/17451000.2015.1031800
Rudnick, D. L. (2016). Ocean Research Enabled by Underwater Gliders. Ann. Rev. Mar. Sci. 8, 519–541. doi: 10.1146/annurev-marine-122414-033913
Ryan, C. G., Whooley, P., Berrow, S. D., Barnes, C., Massett, N., Strietman, W. J., et al. (2015). A longitudinal study of humpback whales in Irish waters. J. Mar. Biol. Assoc. U K 96, 877–883. doi: 10.1017/S0025315414002033
Send, U., Regier, L., and Jones, B. (2013). Use of underwater gliders for acoustic data retrieval from subsurface oceanographic instrumentation and bidirectional communication in the deep ocean. J. Atmos. Ocean. Technol. 30, 984–998. doi: 10.1175/JTECH-D-11-00169.1
Silber, G. K., Lettrich, M. D., Thomas, P. O., Baker, J. D., Baumgartner, M., Becker, E. A., et al. (2017). Projecting Marine Mammal Distribution in a Changing Climate. Front. Mar. Sci. 4:413. doi: 10.3389/fmars.2017.00413
Simon, M., Stafford, K. M., Beedholm, K., Lee, C. M., and Madsen, P. T. (2010). Singing behavior of fin whales in the Davis Strait with implications for mating, migration and foraging. J. Acoust. Soc. Am. 128, 3200–3210. doi: 10.1121/1.3495946
Skjoldal, H. R. (2004). in The Norwegian Sea Ecosystem, eds R. Sætre and A. Fernö Trondheim (Cambridge: Academic Press).
Steiner, L., Lamoni, L., Plata, M. A., Jensen, S., Lettevall, E., and Gordon, J. (2012). A link between male sperm whales, Physeter macrocephalus, of the Azores and Norway. J. Mar. Biol. Assoc. U K. 92, 1751–1756. doi: 10.1017/S0025315412000793
Suberg, L., Wynn, R. B., Kooij, J., Van Der, Fernand, L., Fielding, S., et al. (2014). Assessing the potential of autonomous submarine gliders for ecosystem monitoring across multiple trophic levels (plankton to cetaceans) and pollutants in shallow shelf seas. Methods Oceanogr. 10, 70–89. doi: 10.1016/j.mio.2014.06.002
Sydeman, W. J., Poloczanska, E., Reed, T. E., and Thompson, S. A. (2013). Marine vertebrates. Radioecol. North. Eur. Seas 350, 224–265. doi: 10.1007/978-3-662-09658-1_6
Utne, K. R., Huse, G., Ottersen, G., Holst, J. C., Zabavnikov, V., Jacobsen, J. A., et al. (2012). Horizontal distribution and overlap of planktivorous fish stocks in the Norwegian Sea during summers 1995–2006. Mar. Biol. Res. 8, 420–441. doi: 10.1080/17451000.2011.640937
Víkingsson, G. A. (1997). Feeding of fin whales (Balaenoptera physalus) off Iceland – diurnal and seasonal variation and possible rates. J. Northwest Atl. Fish. Sci. 22, 77–89. doi: 10.2960/J.v22.a7
Keywords: underwater vehicles, glider, marine mammals, passive acoustics, ecology, seasonality
Citation: Aniceto AS, Pedersen G, Primicerio R, Biuw M, Lindstrøm U and Camus L (2020) Arctic Marine Data Collection Using Oceanic Gliders: Providing Ecological Context to Cetacean Vocalizations. Front. Mar. Sci. 7:585754. doi: 10.3389/fmars.2020.585754
Received: 21 July 2020; Accepted: 23 October 2020;
Published: 16 November 2020.
Edited by:
Sabine Mathesius, Simon Fraser University, CanadaReviewed by:
Heather D. Penney, Memorial University of Newfoundland, CanadaAndrew M. Fischer, University of Tasmania, Australia
Copyright © 2020 Aniceto, Pedersen, Primicerio, Biuw, Lindstrøm and Camus. This is an open-access article distributed under the terms of the Creative Commons Attribution License (CC BY). The use, distribution or reproduction in other forums is permitted, provided the original author(s) and the copyright owner(s) are credited and that the original publication in this journal is cited, in accordance with accepted academic practice. No use, distribution or reproduction is permitted which does not comply with these terms.
*Correspondence: Ana Sofia Aniceto, c29maWEuYW5pY2V0b0BvdXRsb29rLmNvbQ==