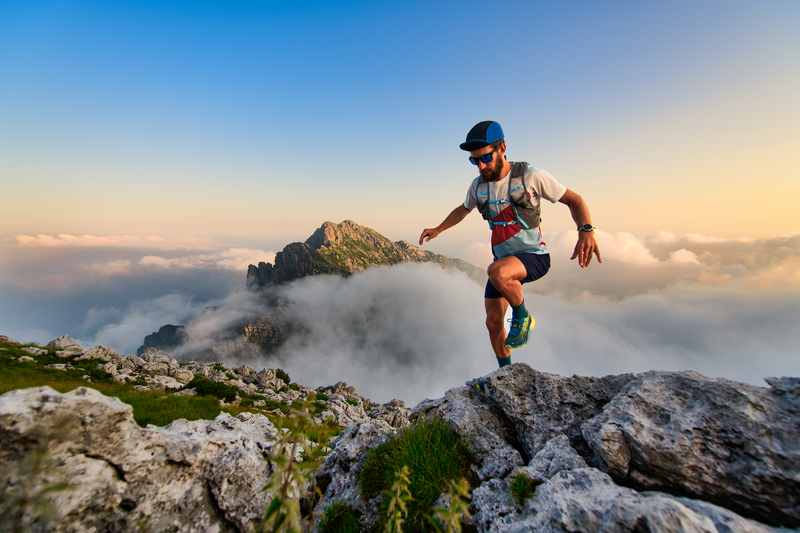
94% of researchers rate our articles as excellent or good
Learn more about the work of our research integrity team to safeguard the quality of each article we publish.
Find out more
REVIEW article
Front. Mar. Sci. , 25 November 2020
Sec. Marine Conservation and Sustainability
Volume 7 - 2020 | https://doi.org/10.3389/fmars.2020.584861
This article is part of the Research Topic Sustainable Development Goal 14 - Life Below Water: Towards a Sustainable Ocean View all 25 articles
The ocean plays a crucial role in the functioning of the Earth System and in the provision of vital goods and services. The United Nations (UN) declared 2021–2030 as the UN Decade of Ocean Science for Sustainable Development. The Roadmap for the Ocean Decade aims to achieve six critical societal outcomes (SOs) by 2030, through the pursuit of four objectives (Os). It specifically recognizes the scarcity of biological data for deep-sea biomes, and challenges the global scientific community to conduct research to advance understanding of deep-sea ecosystems to inform sustainable management. In this paper, we map four key scientific questions identified by the academic community to the Ocean Decade SOs: (i) What is the diversity of life in the deep ocean? (ii) How are populations and habitats connected? (iii) What is the role of living organisms in ecosystem function and service provision? and (iv) How do species, communities, and ecosystems respond to disturbance? We then consider the design of a global-scale program to address these questions by reviewing key drivers of ecological pattern and process. We recommend using the following criteria to stratify a global survey design: biogeographic region, depth, horizontal distance, substrate type, high and low climate hazard, fished/unfished, near/far from sources of pollution, licensed/protected from industry activities. We consider both spatial and temporal surveys, and emphasize new biological data collection that prioritizes southern and polar latitudes, deeper (> 2000 m) depths, and midwater environments. We provide guidance on observational, experimental, and monitoring needs for different benthic and pelagic ecosystems. We then review recent efforts to standardize biological data and specimen collection and archiving, making “sampling design to knowledge application” recommendations in the context of a new global program. We also review and comment on needs, and recommend actions, to develop capacity in deep-sea research; and the role of inclusivity - from accessing indigenous and local knowledge to the sharing of technologies - as part of such a global program. We discuss the concept of a new global deep-sea biological research program ‘Challenger 150,’ highlighting what it could deliver for the Ocean Decade and UN Sustainable Development Goal 14.
Researchers have long recognized the ecological, economic and social importance of the natural capital of the global ocean to humanity (Costanza, 1999; Baker et al., 2020). However, ample evidence shows that, over time, the ocean has suffered increased stress from resource extraction, pollution, and climate change (Díaz et al., 2019; IPCC, 2019; Rogers et al., 2020a), including in the deep sea (Glover and Smith, 2003; Ramirez-Llodra et al., 2011; Sweetman et al., 2017). In 2015, the United Nations (UN) General Assembly set out 17 Sustainable Development Goals (SDGs) as a universal call to action to end poverty, protect the planet and ensure that all people enjoy peace and prosperity by 2030. SDG 14 specifically relates to marine biodiversity and its sustainable use, whereas other SDGs, for example SDG 2 on food security, SDG 8 on economic growth, SDG 12 on sustainable consumption, and SDG 13 on climate, amongst others, also apply to ocean health. Sustainable use of the marine environment also features in other UN policy commitments, including the Convention on Biological Diversity’s Aichi Targets. Most recently the UN General Assembly proclaimed the UN Decade of Ocean Science for Sustainable Development (A/RES/72/73), hereinafter referred to as the Ocean Decade. The Ocean Decade will span a 10-year period commencing 1 January 2021, coordinated by UNESCO’s Intergovernmental Oceanographic Commission (IOC). In preparation, the IOC issued a Roadmap (revised June 2018), that emphasized the need to drastically improve the current conditions of the world’s ocean through science-based solutions and increased cooperation. To this end, the Revised Roadmap outlined six critical societal outcomes (SOs) that should be achieved through actions taken under the Decade (Figure 1) and identified the links between the strategic objectives of the Ocean Decade and the SDGs (United Nations, 2018). The Ocean Decade Draft Implementation Plan, published in March 2020, and revised in May 2020, presents the Objectives (Os) (Figure 1) for the Ocean Decade. It provides a framework within which to develop and deliver Ocean Decade Actions, defined in a scale hierarchy as programs, projects, activities, or contributions.
Figure 1. Decade of Ocean Science for Sustainable Development objectives identified in the recently published revised Draft Implementation Plan, and societal outcomes published in the revised Decade Roadmap.
The revised Roadmap for the Ocean Decade recognizes the deep sea as a frontier environment. In order to deliver the Ocean Decade SOs and Os, it specifically calls on the scientific community to conduct research that advances understanding of deep-sea ecosystems, and their functions and services to human society. The Roadmap identifies an aspiration of the Ocean Decade to expand sustained and systematic ocean observations to all ocean basins and depths, to enable characterization of Essential Ocean Variables (EOVs-physical, biogeochemical and biological) and detect natural and human-induced changes. The deep sea (> 200 m) encompasses the largest living space on Earth, and accounts for more than 95% of the habitable volume (Danovaro et al., 2017). It supports diverse species and habitats, with the continental slope supporting higher diversity than the continental shelf (Rex and Etter, 2010; Muthumbi et al., 2011). According to some estimates, bathyal and abyssal diversity are amongst the highest on the planet (Grassle and Maciolek, 1992; Mora et al., 2011). The combination of geological, physical, and geochemical attributes of the deep seafloor and water column creates a mosaic of complex habitats with unique characteristics (Ramirez-Llodra et al., 2010). While we have only sampled or visually investigated a very small proportion of the deep ocean to date (0.01% with remote instruments Ramirez-Llodra et al., 2010), our current state of knowledge links society’s well-being to the health of the deep sea through a wide range of ecosystem services (see Armstrong et al., 2012; Thurber et al., 2014; Baker et al., 2020 for a review). The remoteness of deep-sea ecosystems has historically led to a presumption that they are homogeneous and impervious to human activities; however, these diverse ecosystems increasingly face large-scale and cumulative impacts from multiple human activities with global influence (Ramirez-Llodra et al., 2011). Finding solutions to these risks challenges the scientific community, industry, national and international authorities and organizations to work collaboratively toward sustainable use and conservation of deep-sea ecosystems. Technological development, investment in research by both industry and philanthropic organizations, and an overall recognition of the significance of the deep-sea in broader Earth systems has driven rapid expansion in our investigation and understanding of deep-sea ecosystems over the last 20 years. However, fundamental questions remain in deep-sea biology and ecology that must be addressed in order to achieve the Ocean Decade SOs and Os. These questions have persisted despite almost 150 years of deep-sea research, and will continue to persist in the absence of a coordinated strategically targeted global effort to change the status quo.
In this paper, we review research needs of the Ocean Decade in the context of the design of a new 10 year deep-sea biology research program under the Ocean Decade. This paper aims to serve as the blueprint for global deep-sea research efforts for the next 10 years and likely beyond.
Various fora and groups have reviewed and documented critical outstanding research questions in deep-sea research, including the European Marine Board (Rogers et al., 2015) and Census of Marine Life deep-sea field projects [e.g., Chemosynthetic ecosystems (German et al., 2011); Seamounts (Clark et al., 2012)]. Most recently, the Deep-Ocean Stewardship Initiative (DOSI) specifically convened the Decade of Deep-Ocean Science working group (DOSI-DDOS WG) to promote global-scale research to understand the role of deep-sea ecosystems in ocean health and resilience. DOSI, a network of over 1400 experts from 77 different countries, seeks to integrate science, technology, policy, law and economics to advise on ecosystem-based management of resource use in the deep ocean. The DOSI-DDOS WG currently consists of 67 people from 21 countries and is open to new members at all times. This group worked with the wider DOSI community during 2 events: (1) DOSI Day 2018 (09/09/2018, Monterey, United States) and (2) a meeting of the working group (October 2018, Aveiro, Portugal), to summarize science priorities and knowledge gaps pertaining to the deep ocean, and place them in the context of the SOs identified in the Revised Roadmap. Here, we report the outcome of those discussions, and identify four broad questions and their links to the Ocean Decade SOs.
Although knowledge on deep-sea community composition and ecosystem functioning has advanced rapidly in recent decades, we still lack fundamental ecological data for much of the deep sea (Glover et al., 2018). Poor knowledge of what lives there, how it is distributed from global to local scales, over environmental gradients (depth, temperature, oxygen, pH, primary productivity, etc.), and over time (seasonality, event-based phenomena, hydrodynamic cycles), precludes establishing effective baselines; in some cases, we still do not know what species are common or rare. Science has described many species, but many more await discovery and description, with repeated examples where presumed “common” species mask the presence of cryptic species (Vrijenhoek et al., 1994; Etter et al., 1999; Havermans et al., 2013). Baseline ecological data form the input to all biological ecosystem models and maps. Our ability to forecast how marine biodiversity will respond to environmental changes and anthropogenic-related pressures (SO3), depends on good base knowledge such as species identities, distributions, physical and chemical drivers of distribution, abundance, biomass, growth rates, etc. Fundamental ecological knowledge severely limits efforts to model and map present-day species distributions to fill data gaps, and predict future distributions under climate change (SO2). Existing models and maps are simplistic, and of questionable accuracy due to limited, and/or poor quality input data (Davies and Guinotte, 2011; Howell et al., 2016; Morato et al., 2020).
Effective ocean management and sustainable use critically depend on identifying linkages among deep-sea ecosystems, communities, and populations. Collectively termed connectivity these linkages include: migration routes, ontogenetic or seasonal movement between habitats, spawning sites, larval dispersal pathways and genetic connectivity, or energy flow pathways in the form of trophic links and food webs. For example, maintaining well-connected populations, communities, and ecosystems underpins the design of effective networks of Marine Protected Areas (MPA) (Cowen and Sponaugle, 2009; Jenkins and Stevens, 2018) (SO2). Effective management of fish stocks (SO5) requires knowledge of how fish use their environment (essential fish habitats, spawning areas, migrations, larval and juvenile dispersal, food web interactions, etc.). Increasing evidence demonstrates that numerous commercially valuable fishes (e.g., tunas), marine mammals, and seabirds prey on deep-sea (mesopelagic) fishes, which form a significant component of their diet (Battaglia et al., 2013; Giménez et al., 2018; Watanuki and Thiebot, 2018). Strong connectivity promotes healthy and resilient populations (SO2), and disruptions to these connections, for example through changes in ocean circulation patterns or plumes from mining activities, can impact population persistence and recovery after disturbance, as well as the effectiveness of MPAs and other spatial protection measures. We must identify these connections to (1) help ensure that human activities do not alter them (SO2) and (2) enable us to predict the consequences of their disruption (SO3).
Sustainable development goal 14 widely recognizes the services provided by the ocean. Within the Decade Roadmap, SO5 focuses entirely on the provision of food supply and alternative livelihoods as key services provided by the ocean. We are at an early stage in understanding the role of the deep sea in provision of services (Armstrong et al., 2012; Thurber et al., 2014; Folkersen et al., 2018; Baker et al., 2020), with scant details on mechanisms of delivery. What are the key species/habitats involved in carbon sequestration? Are some groups more important than others? For example, we know sponges may play an important role in global Si cycling (Maldonado et al., 2019), as well as a sink for inorganic nitrogen, surpassing that of marine sediments at equivalent depths (Hoffmann et al., 2009). Does redundancy exist within the system, i.e., do more than one group of organisms perform the same functions associated with service delivery? The answer largely depends on the shape of the relationship between biodiversity and ecosystem functions (the so-called biodiversity-ecosystem functions curve; Danovaro et al., 2008). To ensure the ongoing provision of those services (SO2), and to understand better marine system processes such as biogeochemical cycling, we must identify the functional groups present, their role in ecosystem function, and how that function relates to delivery of services. Quantifying the variability, in space and time, of these processes on a global scale will enable us to predict changes to function and ecosystem service provision as a result of anthropogenic activities (SO3).
This question addresses both natural and anthropogenic disturbance (e.g., pollution, mining, fisheries, climate change, etc.) and gets to the heart of the knowledge required to manage marine ecosystem use effectively and deliver SO1 (a clean ocean), SO2 (a healthy and resilient ocean), SO3 (a predicted ocean), and SO5 (a sustainably harvested and productive ocean). Sustainable development requires knowledge of baseline environmental data and species tolerance thresholds to disturbance, as well as measurements and predictions of realistic natural disturbance regimes in order to place biological observations in context. Moreover, effective management of deep-ocean use in the future will hinge upon understanding impacts of multiple and cumulative stressors. Limited case studies from past and ongoing disturbances provide some information (e.g., Ashford et al., 2018; Vieira et al., 2020), but even fewer manipulative experiments address the mechanisms behind the responses at different temporal and / or spatial scales (Jones et al., 2017). This gap represents a key area for research development and one of the most important categories of information required for effective management efforts and stewardship of the global ocean. Related to this we must understand the potential for restoration of impacted deep-sea ecosystems with challenges largely associated with observation technologies (Van Dover et al., 2013; Levin et al., 2019), as well as with social, political, and economic interactions with science (Ehrlich and Pringle, 2008) and estimated costs (Van Dover et al., 2013).
These four broad questions lie at the heart of our ability to sustainably manage ocean use. The truly global challenge of addressing them requires a coordinated international and inclusive effort. As we approach the start of the Ocean Decade, the task before the scientific community is clear. We are charged with contributing knowledge to enable the Ocean Decade to achieve the stated SOs and Os. Our ability to plan for sustainable human use of the oceans, and adapt to environmental change lies in our ability to accurately predict possible outcomes and their socio-economic consequences. However, accurate forecasting requires at its base, ecological knowledge of species and habitats that, for the deep sea, is scant, highly spatially biased, with very few temporal data A new, globally coordinated program can address priority research questions that inform the development of a more holistic, non-sectoral, and equitable approach to sustainable use of deep-sea ecosystems. This program requires a coordinated, stepwise, and modular design. Next, we consider the design criteria in light of the identified research questions, and review current understanding of the role of key variables in shaping ecological pattern and process.
To address the SOs identified under the Ocean Decade we must quantify biodiversity and characterize species ecological niches, including their relationship to important climate-related variables. Until recently, the Inter-governmental Panel on Climate Change (IPCC) assessments had largely ignored climate change at the deep seafloor. However, the Special Report on Ocean and Cryosphere in a Changing Climate (SROCC) refers to clear regional and depth-related differences in projected temperature, POC flux, pH, and oxygen at the seafloor (Bindoff et al., 2019) under RCP 8.5 and 2.6 projections. To facilitate greater inclusion of the deep sea in IPCC assessment efforts we must stratify our sampling across these Essential Ocean Variables (EOVs) in order to quantify biological responses. Current and projected deep-ocean climate velocities exceed those at the surface, with consequences for the pelagic environment and consequently the benthos (Brito-Morales et al., 2020). Range mismatches among species across depths, could compromise vertical connectivity in the deep ocean (Brito-Morales et al., 2020).
Ocean temperature above the permanent thermocline decreases with increasing latitude with sea-ice present in polar regions. POC export has a more complex relationship with latitude, but peaks at mid-latitudes (40–60 degrees) north and south of the Equator, with minima at ∼20 degrees (Lutz et al., 2007). POC export relates to POC flux to the seafloor, which in turn shapes benthic community composition (Billett et al., 2010). Both temperature and POC flux are implicated in driving global patterns of species diversity, but relationships between drivers and responses are still uncertain (Worm and Tittensor, 2018). The latitudinal diversity gradient (LDG) in species richness is one of the most well-established ecological paradigms for many terrestrial, freshwater, and coastal systems (Hillebrand, 2004), but with equivocal support for LDGs in the deep sea. Originally described by Humboldt and Bonpland (1807), the pattern predicts highest species diversity at the equator, with decreasing diversity toward the poles. The diverse proposed processes underlying LDGs include wide spatial variation in biological interactions, evolutionary processes, energy availability, climatic variability, physical heterogeneity and patchiness, and neutral processes (Pianka, 1966; Rohde, 1992).
Evidence suggests latitudinal gradients of diversity in benthic deep-sea fauna, although this conclusion rests on much lower sampling effort compared to other ecosystems. Rex et al. (1993, 2000) reported LDGs in the North Atlantic for gastropods, bivalves, and isopods. Patterns in the South Atlantic were weak and only present in some taxa (Brandt et al., 2005). This weaker South Atlantic pattern could relate to either lower sampling effort or strong regional effects on diversity, e.g., terrigenous carbon inputs in the Amazon Basin (Rex et al., 1997). Elevated Antarctic deep-sea biodiversity could also weaken the South Atlantic LDG if deep-sea biodiversity mirrors the high diversity of the Antarctic continental shelf (Clarke, 2008). Gage et al. (2004) found poleward declines in the diversity of deep-sea cumaceans for the entire Atlantic but only on the eastern corridor, supporting earlier observations of regional and basin effects. However, polychaetes in the Arctic Ocean illustrate that LDGs may occur even with a basin (Bodil et al., 2011). Among the benthic meiofauna, deep-sea foraminiferans show latitudinal gradients in the North and South Atlantic (Culver and Buzas, 2000) related to seasonality in pelagic production (Corliss et al., 2009). Nematodes peak in diversity at mid-latitudes in the North Atlantic (Mokievsky and Azovsky, 2002). More recent research demonstrates the complexity and variability of LDGs in the deep sea. Woolley et al. (2016) reported that both the patterns and underlying drivers of ophiuroid diversity clines can vary with depth, transitioning from diversity patterns driven by temperature at shallower depths to productivity at deeper depths. This pattern, along with an earlier body of evidence (Gooday et al., 1990; Lambshead et al., 2002; Rosa et al., 2008; Smith et al., 2008; Corliss et al., 2009; Tittensor et al., 2011; McClain et al., 2012a), points to the importance of organic matter availability in driving large scale patterns of diversity in the deep oceans.
In polar latitudes, where the effects of climate change are being expressed more rapidly, changes in surface primary production as a result of decreasing sea-ice cover will likely influence carbon supply to the deep sea (Rogers et al., 2020b). In the Arctic, declining summer sea-ice cover with additional strong and complex multiyear sea ice effects (CAFF, 2017), has resulted in a 30 percent increase in surface primary production (Arrigo et al., 2008; Arrigo and van Dijken, 2015). Regional patterns of change in the duration of sea ice as well as the collapse of ice shelves characterize Antarctica. In the Atlantic sector, including the Antarctic Peninsula, the present rapid decrease in sea ice coverage and duration follows a 40-year increasing trend (Parkinson, 2019; Vernet et al., 2019). Collapse of ice shelves in the Weddell Sea has exposed new areas of the ocean for primary production. Changes in surface primary production, including the size (AMAP, 2017; CAFF, 2017) and functional types (Orkney et al., 2020) of phytoplankton cells, will alter pelagic food webs as well as POC export and flux to the seabed, subsequently affecting benthic communities (Gutt et al., 2011).
Although surface pH tends to increase with latitude, at the deep seafloor, Highest pH values occur in the North Atlantic, and lowest values in the North Pacific (Sweetman et al., 2017). Intense research in shallow water marine ecosystems over the last decade has examined biological responses to ocean pH stemming from the predicted decrease in pH under climate change scenarios termed ‘ocean acidification.’ Ocean acidification results from the absorption of atmospheric CO2 by the ocean. This facet of global climate change significantly affects calcifying organisms by requiring them to utilize larger proportions of their energy budget to offset calcium carbonate dissolution (Muller and Nisbet, 2014). In surface waters, this change can alter phytoplankton community composition, potentially altering primary productivity (Dutkiewicz et al., 2015). These surface level changes will impact pelagic food webs and POC flux to deep-sea communities. In addition, the saturation state of carbonate decreases with depth, and therefore calcifying organisms in much of the deep ocean already face energetic challenges. Although deep-sea corals show remarkable capacity to maintain calcification in waters undersaturated in carbonate (Thresher et al., 2011; Gómez et al., 2018), further declines in pH will further challenge their calcification and growth, potentially leading to dissolution of existing deep-water coral reefs.
Highest sea surface oxygen concentrations occur in polar regions and lowest concentrations in equatorial regions. At the seafloor, oxygen patterns resemble those for pH, with highest dissolved oxygen concentrations in the North Atlantic, and lowest concentrations in the North Pacific (Sweetman et al., 2017). Oxygen strongly influences benthic fauna density, biodiversity, species distributions, taxonomic composition, food web structure, biogeochemical cycling, body size and species-level population and physiological rates (Levin and Gooday, 2003; Muthumbi et al., 2004; Laffoley and Baxter, 2019; Wishner et al., 2020). In the deep sea, the strongest influence of oxygen occurs at bathyal depths (200–1200 m) within oxygen minimum zones, but particularly in the prevalent extreme OMZs in the North and Eastern Pacific Ocean, Northern Indian Ocean, and off west Africa (Helly and Levin, 2004) as well as the Western Indian Ocean (Muthumbi et al., 2004). Many of these low oxygen areas are expanding under climate change (Stramma et al., 2008; Breitburg et al., 2018; Levin, 2018). The North and East Pacific and Southern Ocean have experienced the greatest oxygen losses over the last half century (Levin, 2018; Bindoff et al., 2019).
Although stratification in relation to latitude captures a range of current environmental variability, it does not capture evolutionary scale processes that have shaped faunal patterns of diversity and distribution. Many studies have considered regionalization of the marine environment into biogeographic zones. Watling et al. (2013) provides a detailed review of the history of deep-sea benthic biogeography as part of their development and refinement of a Global Open Ocean and Deep Sea (GOODS) classification (UNESCO, 2009; Watling et al., 2013). The GOODS classification was initially developed in 2009 in an expert consultation workshop for use in high seas management, initially basing proposed units on regions and provinces recommended by Menzies et al. (1973); Zezina (1973)Zezina (1997), and Vinogradova (1979) for bathyal and abyssal regions. However, boundaries were modified with recent data, published and unpublished observations, or re-analyses of existing data. Watling et al. (2013) further developed the classification, using physical and chemical proxies considered good predictors of benthic population distribution, to delineate 14 lower bathyal and 14 abyssal provinces. The fully classification also incorporated hadal provinces defined by Beliaev (1989).
Although the GOODS classification provides a convenient system by which to stratify benthic survey and monitoring, it does not consider the pelagic environment. Surface ocean properties from the basis of most pelagic biogeographic schemes (e.g., Longhurst, 1998; Spalding et al., 2012). However, in order to characterize the mesopelagic realm (200 — 1000 m), Sutton et al. (2017) collated expert opinion on physical and chemical oceanographic conditions, and biological expertise to define 33 mesopelagic ecoregions within four biomes (polar, westerly winds, trade wind, distant neritic). Their ecoregions reflected broad-scale patterns in the daytime distributions of mesopelagic fauna, with water mass structure, surface productivity, oxygen minimum zones and temperature extremes included as variables of particular importance. These ecoregions closely parallel those identified by Watling et al. (2013) in their classification of deep bathyal and abyssal biogeography. However, Sutton et al. (2017) note that their classification omits temporal variability in conditions, which plays a central role in the fluid and dynamic pelagic realm, and, like much of the deep pelagic biome, contains extensive data gaps (Webb et al., 2010). Using back scatter data, Proud et al. (2018) examined the global distribution of biomass (as backscatter intensity) within the deep scattering layer and identified 22 provinces, that correlated with overlying primary productivity and temperature at the depth of the deep scattering layer. No study to date has attempted to classify the bathypelagic realm, likely reflecting the lack of available data.
We propose a global program uses the revised GOODS classification (Watling et al., 2013) and mesopelagic ecoregions from Sutton et al. (2017), to stratify respectively benthic and pelagic deep-sea survey and monitoring, ensuring overall stratification by latitude.
While latitude/biogeography can serve as a proxy for many key environmental variables, vertical gradients with depth arguably represent the strongest gradient of environmental change in the global ocean. Physical and chemical oceanographic drivers, and biogeochemical and biological responses all vary with depth. Key variables that correlate with depth (not always monotonically), and play a significant role in determining species distributions and community composition, include temperature (Haedrich et al., 1975), pressure (Somero et al., 1983), oxygen (Gallo and Levin, 2016), sediment type (Day and Pearcy, 1968), water mass structure (Howell et al., 2002), pH and aragonite saturation (Guinotte et al., 2006), and food supply (Rowe and Menzies, 1969), amongst others. Fauna undergo a non-repeating sequential change in composition with depth, with the combination of environmental variables that correlate with depth defining species depth ranges, as well as the fundamental ecological niche occupied by the species. Changes in environmental variables over depth also influence many ecological measures including diversity, abundance, and biomass. Stratifying sampling by each of these key environmental variables would be challenging. As with latitude/biogeography, depth itself serves as an easily measurable, widely accepted proxy for environmental variation. We therefore outline guidance on stratification of sampling by depth.
Although experts generally accept 200 m as the start depth for the deep sea, little evidence supports the existence of a benthic faunal boundary at this depth. Geomorphological rather than biological criteria define the transition between the deep circalittoral and deep sea, which occurs at the shelf edge break, typically taken as 200 m. Coral reef biologists recently described a new rariphotic zone extending from 150 to 300 m with community members predominantly related to shallow-water families (Baldwin et al., 2018). Carney (2005) summarized patterns of deep-sea benthic faunal zonation with depth and concluded that transition zones typically occur at 300 - 500 m (the shelf-slope zone of transition), ∼1000 m (upper slope zone of transition), and 2000 —3000 m (lower slope zone of transition). Researchers typically classify the pelagic ocean by downwelling solar light levels where: the epipelagic zone (0 — 200 m), receives sufficient solar light for photosynthesis; the mesopelagic zone (200 — 1000 m), receives sufficient solar light for vision; the bathypelagic zone (> 1000 m) receives light coming only from biological sources (Sutton, 2013).
We propose a global program uses the following indicative depth horizons as a general guide for a target range for all biogeographic regions in order to achieve an unbiased global dataset: 150 — 300, 300 — 500 then every 500 m to the deepest point of the oceans at ∼10 km. We chose these horizons to capture the scales of known faunal transitions over the depth gradient. These depth-delineated horizons should serve both pelagic and benthic known faunal zonation patterns. We do not dictate the density of sampling (fine or coarse) at any individual site.
The degree of faunal turnover (β-diversity) or its converse (similarity) between pairs of communities links closely to the spatial or environmental differences between them. The presence or absence of a species at one location, and similarity to another location, can reflect the geographic distance between them (i.e., the distance-decay relationship), acknowledging interplay with dispersal ability of the species, ocean currents, and availability of suitable habitat. Animal migrations can occur on scales of 1000s of kilometers. Larval dispersal of brooding invertebrates span scales on the order of meters to 10s of meters, whereas broadcast spawners disperse 10s to 1000s of kilometers depending on ocean currents and planktonic larval duration (McClain and Hardy, 2010; Hilário et al., 2015). Dispersal of adult stages can vary from centimeters to 1000s of kilometers, depending on size, swimming ability (e.g., crawling isopods versus migrating fish) and current patterns. Alternatively, these biogeographic and community patterns can reflect habitat heterogeneity (Cordes et al., 2010), niche-based processes such as environmental filtering, i.e., how species specific niche requirements map out on the environmental landscape, and the long-term consequences of interspecies interactions (e.g., Quattrini et al., 2017; Ashford et al., 2018).
At large geographical scales, the deep sea varies considerably in species diversity over latitude and depth (Rex and Etter, 2010). Few studies address regional to oceanic patterns of deep-sea β-diversity for megafauna. In general, specialist assume that even at larger scales, matching environment and species’ niche requirements (i.e., environmental filtering) primarily drives patterns in biogeography and biodiversity (reviewed in McClain and Hardy, 2010). Some studies downplay the importance of dispersal limitation because the planktonic larval phases of many deep-sea invertebrates theoretically allow long-distance dispersal and potentially large biogeographic ranges (reviewed in McClain and Hardy, 2010), although empirical evidence suggests limited realized connectivity of 100s rather than 1000s of kms (Baco et al., 2016). Yet few studies have evaluated these concepts.
Most research on deep-sea β-diversity has focused on benthic macrofauna or meiofauna. McClain et al. (2012b) explored patterns of β-diversity in taxonomic, phylogenetic, and functional diversity across the Atlantic Ocean, using the bivalve data set of Allen (2008). Strong environmental filtering and dispersal limitation both drove turnover in taxonomic, functional, and phylogenetic composition. Blake and Grassle (1994) detected faunal changes both across and along isobaths in the southern region of the ACSAR program, NW Atlantic off North and South Carolina (United States). Depth explained most faunal variation, but with pronounced horizontal variation in the bathyal region. For meiofauna, Danovaro et al. (2009) found significant differences in assemblages across a longitudinal gradient for the entire ocean for abyssal but not bathyal sites in the Mediterranean Sea. Bianchelli et al. (2013) subsequently found evidence that energy availability was an important driver of the structure of deep-sea nematode assemblages. Food quantity drove patterns at larger scales, and the quality and bioavailability of food determined small-to-local scale patterns. However, other studies of nematodes found that productivity played a subordinate role to sedimentary characteristics (Vanreusel et al., 2010). In contrast Leduc et al. (2012) found distances between sites, both horizontally and vertically, explained the greatest proportion of variance in β-diversity of nematodes on the upper New Zealand continental slope.
The high level of coexistence of species in the deep sea represents one of the most intriguing paradoxes in ecology. Species richness in some deep-sea localities can exceed 300 species of macrofauna within a square meter (Grassle, 1989; Etter and Mullineaux, 2001). Sampling often yields species-accumulation curves that rarely reach an asymptote (reviewed in Etter and Mullineaux, 2001; Snelgrove and Smith, 2002), a pattern frequently interpreted as evidence of high turnover of local species. Empirical studies on the role of environmental patchiness in explaining these findings is mixed (Jumars, 1975, 1976; Thistle, 1979; Jumars and Eckman, 1983; Lamont et al., 1995; reviewed in Rex and Etter, 2010; McClain et al., 2011).
Although latitude/biogeography and depth provide useful frameworks for understanding ecological processes at a global scale, comprehensive understanding requires studies that describe and quantify ecological patterns at finer spatial scales. To enable us to begin to identify the scales of faunal turnover and connectivity, we propose that regional modules of a global sampling program stratify by horizontal distance at resolutions of 1 m, 10 m, 100 m, 1 km, 10 km, 100 km. Not all spatial scales will be appropriate for all size fractions or faunal components and this stratification should serve as a guide to interpret as appropriate to the specific study system.
SO’s 1, 2, 3 and 5 all require an enhanced understanding of human impacts on deep-sea ecosystems, in order to guide marine spatial planning and the sustainable exploitation of resources while safeguarding deep-sea life (Manea et al., 2020). Therefore, a comprehensive global study should stratify sampling by single and multiple anthropogenic pressures.
A design approach that stratifies by latitude will enable us to understand how species might respond to climate change. However, the degree of climate hazard (change relative to natural variability), and the time of emergence of climate change in the deep sea vary spatially (FAO, 2018; Bindoff et al., 2019; Levin et al., 2020). Some sites will experience climate change sooner than others. We propose targeting some early and some late emergence (e.g., potential climate refugia) sites. To effectively distinguish climate impacts requires selection of sites otherwise un-impacted, or minimally impacted by other anthropogenic pressures (e.g., not fished, low levels of pollution). This strategy implies inference from some prior knowledge on the intensity of human impacts at a given site from proxy data.
Global overfishing of coastal fish stocks and the need to feed a growing human population have led capture fisheries to target stocks inhabiting progressively deeper waters (e.g., Watson and Morato, 2013) and Areas Beyond National Jurisdiction (ABNJ) (e.g., Merrie et al., 2014) since the 1950s. In general, life-history traits such as slow growth, late maturation, and low fecundity (e.g., Drazen and Haedrich, 2012) increase the vulnerability of deep-sea fishes to fishing pressures. Long-lived species that form dense, local aggregations, such as orange roughy and oreos, are particularly vulnerable to rapid overexploitation, but approximately equal population reductions occur in all species whose ranges fall within the fished depth range (Bailey et al., 2009). The economic returns from demersal fishing decrease, and ecological costs increase with depth below c. 600 m in the NE Atlantic (Clark et al., 2016a). Restrictive measures to support sustainability of deep-water fisheries and to protect benthic ecosystems are now in place in EU Atlantic waters, including a depth ban on bottom trawl fishing below 800 m (Regulation (EU) 2016/2336). Recent papers suggesting exceptionally high mesopelagic fish biomass (Kaartvedt et al., 2012; Irigoien et al., 2014) have helped drive a resurgence of interest in targeting mesopelagic fishes as a source of protein for fishmeal. Acknowledging that deep-living pelagic fauna represent a largely unexploited marine resource, any future use must carefully balance benefits against both the considerable lack of knowledge about the ecology and ecosystem function of the deep pelagic realm (Webb et al., 2010), the high value of mesopelagic fauna in carbon transport and sequestration and their role in oceanic food webs (Colaço et al., 2013; Trueman et al., 2014). In addition to impacts on fish stocks, fishing, and specifically use of bottom contact gear, can cause significant adverse change or serious harm to benthic habitats and species (Rogers and Gianni, 2010), reducing structural and functional diversity and altering biogeochemical cycles (Puig et al., 2012; Ramalho et al., 2018; Vieira et al., 2019). Little information exists on resistance to, and rates of recovery from, the physical damage associated with bottom contact gear for many species. However, available data indicate limited resilience and thus high vulnerability in some species. At regional levels, Regional Fisheries Management Organizations (RFMOs) and national monitoring programs can provide satellite-derived data on marine vessel movements. These data enable a reasonably accurate assessment of the spatial distribution of fishing pressure (e.g., NAFO data input to benthic studies in the NW Atlantic; Ashford et al., 2018, 2019).
Litter and contaminants from different sources now infiltrate the deep sea, and have been identified in sediments and in biota (e.g., Ramirez-Llodra et al., 2010; Pham et al., 2014; Woodall et al., 2015; Courtene-Jones et al., 2020). These contaminants include debris of different types and sizes, such as plastics (ranging in size from nano- to macro-) or fishing gear, and other particulate or dissolved chemicals such as hydrocarbons, metals, and legacy and emergent persistent organic pollutants (POPs) (e.g., Pham et al., 2014; Woodall et al., 2014; Taylor et al., 2016; Jamieson et al., 2017). Nevertheless, we lack information on how these materials spread, whether a gradient of contamination decreases away from terrestrial sources, or how, and for how long, they move into the deep sea. High resolution ocean circulation models (van Gennip et al., 2019), indicate a ∼2 year transit time of the litter produced in the western coast of South America, including debris produced by the industrial fishery operating in the high seas off Chile and Peru, to the center of the South Pacific Gyre. However, we lack any reliable estimate of the portion of this debris reaching the deep sea and the time it takes. Importantly, how do pollutants accumulate in sediments and biota, and how do they impact fauna? Some initial studies reported microplastics in megafauna from the Rockall Trough dating back to the mid-1970s (Courtene-Jones et al., 2017) and we now know these contaminants spread to the deepest ocean trenches (Jamieson et al., 2019). Environmental risk assessment, for instance, requires knowledge of baseline levels for concentrations of contaminants, in order to develop sediment quality guidelines and to assess baseline levels of biomarkers of stress in organisms, against which to measure effects of disturbance. Stratification of sampling over a gradient of contamination adds significant challenges; ocean circulation and topography can concentrate litter (e.g., canyons, gyres), whereas bioaccumulation can concentrate pollutants. Distance from pollution sources, such as land, river mouths, or major shipping lanes offers one potential proxy.
Other forms of anthropogenic activities are more spatially constrained. Licensing requirements limit deep-seabed mining and oil and gas activities to specific locations best studied through dedicated regional monitoring programs. A global program could provide a baseline against which to monitor, and therefore should ensure inclusion of sampling locations within areas licensed for oil and gas extraction, or contracted for seabed mining exploration, as well as comparable areas (e.g., potential reference sites) that are protected from various forms of anthropogenic impact where possible.
We propose a global program replicates the following treatments in regional designs where possible: high and low climate hazard under early/late time of climate change emergence, fished/un-fished, near/far from pollution sources, licensed/protected from industry activities.
Substrate type, an essential ocean variable, shapes benthic biological community composition. Historically, we largely based our knowledge of deep-sea benthic ecosystems on data obtained using trawls and sledges from soft-sediment seafloors. However, over the last 40 years, advances in technology coupled with decreases in cost, have supported growth in the use of video and still image-based tools for semi-quantitative sampling of previously inaccessible hard substrate habitats. The resulting new findings challenge the prevailing view of deep-sea ecosystems (Danovaro et al., 2014). The global design must factor in substrate type. However, lack of knowledge on seafloor composition constrains a priori stratification by substrate type. Collecting acoustic survey data (multibeam/sidescan sonar) prior to any biological work, undertaking topographic and acoustic backscatter classification, and stratifying biological surveys by remotely sensed bottom type (Brown et al., 2011; Riehl et al., 2020) provides a useful approximation. Alternatively, published models of seafloor lithology may be useful (Diesing, 2020). However, these models likely lack the resolution required for realizing stratification by substrate type in survey design.
Global bathymetry data, such as the General Bathymetric Chart of the Oceans, offers another approach to stratification, based on topography. This simplified form of geomorphological classification analyses terrain derived variables such as slope, rugosity, bathymetric position index, to differentiate terrain types. Bottom slope provides a particularly useful proxy for multiple ecologically relevant variables (McArthur et al., 2010). Existing geomorphological classifications (e.g., Harris et al., 2014) may provide a useful standardized means by which to consider the global stratification of sampling. However, such classification may not produce ecologically meaningful geomorphological classes, and thus should not form the basis of stratification efforts. Nevertheless, a global program should strive to include different ecosystem types, an issue addressed further in section 6.
We propose that a global program should stratify sampling by substrate type and / or topography, including bottom slope, within regional designs.
Effective assessment of human impacts requires long-term monitoring (time series) of both impacted and control sites. In addition, to determine the functional significance of organisms and their role in the delivery of goods and services to humankind critically requires temporal sampling and experimentation. Although our blueprint focuses on setting spatial design criteria for a global survey program, the design must include sites prioritized for monitoring and temporal surveys.
Within each biogeographic region experts should identify and include potential monitoring sites in the design. Levin et al. (2019) and the DOOS initiative provide an inventory of current sustained deep-ocean observing activities, and propose a series of potential region-specific, interdisciplinary projects to demonstrate the feasibility of sustained deep-ocean observing, relevant technologies, and the impact and utilization of deep-ocean observations. These proposed locations include the Clarion-Clipperton Zone, Azores Archipelago, Northeast Pacific: Cascadia Margin to the Juan de Fuca Ridge, Western Pacific, and Ocean Trenches: Izu-Ogasawara Trench and Mariana Trench. Researchers selected these sites on the basis of strategic advantages and existing infrastructure, and they represent excellent choices for demonstration projects, but we must now identify further sites for all biogeographic regions (e.g., Indian Ocean) and take the first steps toward establishment of a globally comprehensive network of sites for sustained observations. Following Levin et al. (2019), we propose a global program use the following criteria in site selection: access to different strata outlined in the global design, availability of existing observing infrastructure, opportunity and ease of installing and maintaining new infrastructure.
The strong spatial bias in our knowledge of the marine environment largely drives the need for a globally coordinated and inclusive program. Latitudinally, the most undersampled regions include equatorial and polar areas as well as southerly latitudes (Menegotto and Rangel, 2018). Researchers have prioritized the data poor south Atlantic, south and central Pacific and Indian Oceans for research (Clark et al., 2012; Saeedi et al., 2019). Globally, sampling effort decreases with depth. For example as of 2019 10.4% of the Ocean Biodiversity Information System (OBIS) records were from > 200 m, with only 1.5% of time series data (> 5 years) falling below 200 m. Only 158,000 records fell between 500 and 10,900 m. At the conclusion of the Census of Marine Life, Clark et al. (2010) highlighted the lack of data available on deeper sections of seamounts (> 2000 m depth). Recently, Taylor and Roterman (2017) identified only nine published papers that dealt with population genetics below 3500 m depth. The bathypelagic environment is the least studied, and largest component of the deep oceans by volume (Webb et al., 2010). The BioTIME initiative reports similar spatial bias in time series data with most studies occurring in Europe, North America and Australia (Dornelas et al., 2018), with large data gaps in the Pacific, South Atlantic and Indian Oceans. As ice cover in the Arctic continues to decline, this ocean basin will experience increasing anthropogenic influence and deep-sea research efforts should also prioritize this key region. There is a clear and well documented need to prioritize research effort in southern and polar latitudes, deeper depths, and midwater environments.
The global design detailed above sets out a strategy that is independent of any perceived ecosystem type, and thus moves away from the traditional silos in which many deep-sea researchers find themselves. However, the global design should preferentially ensure inclusion of different ecosystem types. Here, Harris et al.’s (2014) global geomorphological classification scheme may provide a useful standard against which to classify a given study site. However, from an ecological perspective some of Harris et al. (2014) classes easily group into single ecosystem types that correspond to established research areas with the deep-sea biological research community. Some of these ecosystem types require additional design considerations to facilitate a more complete representation of these specific systems with respect to addressing the Ocean Decade SOs. We identify different ecosystem types, the equivalent Harris et al. (2014) class(es) and further variables by which to stratify individual or regional project level designs (Table 1).
Increasing evidence in recent years illustrate that inconsistencies in sampling of different habitats and regions have challenged efforts to bring datasets together and provide a global picture. Several deep-sea field projects under the Census of Marine Life (2001–2010) noted this issue when collating and collectively analyzing their data. The highly variable array of sampling equipment and survey approaches constrained analyses. Effective broad-scale analyses of ecological patterns and processes, and human impacts requires standardized comparable data (Clark et al., 2016b). The high diversity of life forms, from microscopic bacteria to large cetaceans, require different sampling approaches and methods depending on the composition and abundance of the biological communities and environmental characteristics of their habitat. Although national goals often drive scientific objectives and specific survey design, we identified consistent sampling across national and international programs as a priority to advance our knowledge of deep-sea ecosystems. The guidance on “best-practice” sampling of deep-sea environments (Clark et al., 2016b) complemented other deep-sea texts (e.g., Danovaro et al., 2010, 2020; Eleftheriou, 2013) and initiatives such as the Global Ocean Observing System (GOOS) and the Deep Ocean Observation Strategy (DOOS) in trying to improve global-scale science.
Building on the efforts of GOOS (Miloslavich et al., 2018a), DOOS has proposed essential ocean variables (EOVs) for the deep ocean (Levin et al., 2019). Many of the GOOS variables identified by the Bio/Eco panel occur only in shallow water (e.g., mangrove, seagrass, algal cover, turtles). DOOS has identified a suite of physical, biogeochemical, and biological/ecological variables that are sufficiently mature (technologically ready), suitable for sustained, and, in some cases automated, observing; but this prioritization omits many critical kinds of information. Danovaro et al. (2020) identified, through expert elicitation, a set of essential ecological variables necessary to address (1) biodiversity; (2) ecosystem functions; (3) impacts and risk assessment; (4) climate change, adaptation and evolution; and (5) ecosystem conservation at the deep seafloor.
The need for a consistent approach to data collection and close collaboration between marine scientists from different countries and disciplines to advance knowledge of the ocean also catalyzed the development of the General Ocean Survey and Sampling Iterative Protocol (GOSSIP) (Woodall et al., 2018). Focusing on 20 biological, chemical, physical, and socioeconomic parameters the detailed GOSSIP framework supports consistency in future marine data collections. It highlights standardized collection methods and discusses their relevant limitations and caveats to help researchers apply (or at least understand) best practice techniques for generating globally comparable marine data. Although concerned with mesophotic, deep-pelagic, and bathyal biological communities, application of this protocol more widely offers a good starting point for research efforts under the Ocean Decade. In addition, the International Oceanographic Data and Information Exchange of the IOC has developed the Ocean Best Practices System (OBPS) including an open access, permanent, digital repository of community best practices in ocean-related sciences and applications (Pearlman et al., 2019). This repository contains highly detailed standard operating procedures and operational field manuals for a range of survey and sampling equipment and techniques, and may provide additional detail and points of reference for specific gear types and procedures, including quality assurance and archiving of data.
Although standardized observations and methodologies require further work, we recommend that a global program adapt Woodall et al. (2018); Levin et al. (2019), and Danovaro et al. (2020), relevant archived best practice documents in the OBPS (Pearlman et al., 2019) as a basis for further development of standardized approaches to deep-sea biological survey and monitoring. Danovaro et al.’s (2020) and Table 1 provides a summary of actions required for deep-sea monitoring of the most important essential ecological variables. Woodall et al.’s (2018) and Table 1 summarizes key measurements and methods to obtain such measurements in a robust, standardized, and affordable approach. Levin et al.’s (2019) and Table 2 provides a list of biological and ecosystems Essential Ocean Variables, including new EOVs proposed by the Deep Ocean Observing Strategy (DOOS). Here, we align these three study recommendations on what to measure and how (Table 2) to provide advance understanding of deep-sea ecosystems and form a basis for further discussion on this topic under a global program.
Table 2. Woodall et al. (2018); Levin et al. (2019), and Danovaro et al. (2020) recommendations for what to measure and how, aligned for equivalency, and supplemented by additional considerations.
Importantly many of the measures identified in Table 2 require the collection of physical specimens. Biodiversity measures (KSQi) require physical specimens for biomass measurements, and for morphological and genetic analysis (DNA barcoding) to confirm organism identification and resolve taxonomy and phylogeny unambiguously. Connectivity studies (KSQii) specifically population connectivity require physical specimens for microsatellite, AFLP, or NGS studies, as well as reproductive studies such as fecundity, reproductive mode and timing of spawning. Trophic studies require samples for stomach content, fatty acid, pigment, stable isotopes, and potentially eDNA analyses. Further questions around ecosystem function (KSQiii) require physical specimens to quantify physiological processes and ecosystem services such as carbon sequestration, and to determine biological traits such as growth rates, longevity, age and size at first maturity, population size structure, and length/weight relationships. Earlier efforts to develop a functional traits database for vent species highlighted how few life-history traits could be assembled for all species (Chapman et al., 2019). Impact and risk assessment measures (KSQiv) require physical specimens for analyses of contamination such as microplastics, particulate or dissolved metals, hydrocarbon exposure, and legacy and emergent persistent organic pollutants. We propose that targeted physical specimen sampling form an important part of a global program. Coordinated and targeted physical specimen sampling efforts, and development of processing pipelines that include access to experts and effective archiving require further consideration.
In addition to the fundamental need for standardization of measures, collection and processing methods, data accessibility following collection also remains a challenge. O1 and SO6 (Figure 1) consider a transformative increase in ocean knowledge that include data and specimen archiving as part of the desire to expand, innovate and integrate knowledge in global systems (O2). We expect that this information will enhance understanding and prediction of the global oceans (O3) as part of the interconnected system and the need to develop a decision support system (O4). Critical elements include data storage, and ensuring it follows both the Findable, Accessible, Interoperable, and Reusable (FAIR) principle and the principles of Collective benefit, Authority to control, Responsibility and Ethics (CARE). These principles apply not only to digital products, but also to physical specimens, which all form the foundation of repeatability in science.
The IOC, together with the Center for the Fourth Industrial Revolution: Ocean under the Ocean Data Platform1, are developing an Ocean Data and Information System to improve significantly the availability of ocean data and information, and to enable open source products and services catered to the needs of a broad community of users, including academia and ocean managers. This, together with existing UN supported initiatives such as OBIS, will provide the means to archive data collected under a global program. However, whether this initiative includes provision for open sharing of specimens as well as data remains unclear. Participants in a global program should commit to the open sharing of specimens as well as data, including deposition of specimens with an established museum, an institution with a recognized charter that supports both the permanent storage and care of archive specimens, and access to those specimens by the scientific community. Natural history museums facilitate loans to enable the global community to utilize specimens in their care. Nonetheless, any material collected within the territorial waters of a specific nation falls under the purview of the Convention on Biological Diversity and particularly the Nagoya Protocol and its access and benefit sharing rules. A framework governing access and benefit sharing for marine genetic resources in areas beyond national jurisdiction (ABNJ) is in discussion at the UN (The International Legally Binding Instrument for the Conservation and Sustainable Use of the Biological Diversity of ABNJ; Wright et al., 2018). Both of these legal frameworks require traceability of biological specimens and any digital sequence information from the origin of the samples through subsequent uses, especially if used commercially (Rabone et al., 2019). This traceability means that a unique identifier for samples taken at sea may become an important requirement both for tracing the use of samples, but also for linking specimens or digital sequence information to sample locations and their associated metadata.
Rapid technological change over the coming decade will parallel accelerating species loss. Consequently, a standardized repository approach, to archive or ‘bank’ frozen specimens, tissue samples, and specimens fixed for morphological visualization, will enable scientists to address future questions not yet envisaged or for which technology does not yet exist (e.g., regarding functions). Museum specimens represent the pinnacle of sustainable science: material collected now will be used by future generations another 150 years in the future, just as we use the original specimens collected on the Challenger Expedition, the birth place of deep-sea science, 150 years ago. Guiding principles in both data and specimen archiving should include rapid accession and minimal embargo, and a commitment to collect broadly. Collection and archiving of data and specimens should not only serve the goals of an individual project, but prepare for synergistic and unpredictable future uses.
Objective 1 of the Ocean Decade (Figure 1) focuses on increasing capacity to generate, understand, manage, and use ocean knowledge. This objective has particular relevance for deep-sea research. While more than 70% of countries have a deep-sea environment within their EEZ, economically developed nations (sensu UN Department of Economic and Social Affairs) conduct most deep-sea research. Availability of samples, bias in available data, and overall knowledge of deep-sea ecosystems all reflect this bias. Countries with developing economies face significant barriers to participating in deep-sea research, including access to technological capability and infrastructure, and specific expertise. Yet the least studied parts of the deep sea often occur within the EEZs of less economically developed nations. A global assessment of capacity development needs in ocean science was undertaken by the UN through a series of regional workshops between 2011 and 2013. This assessment highlighted particular needs for capacity building in deep-sea research (Ruwa et al., 2016). In 2015, the Intergovernmental Oceanographic Commission Assembly adopted its Capacity Development Strategy for 2015–2021 (IOC-UNESCO, 2016), identifying six high-level outputs to address on a long-term and sustained basis:
(1) Human resources developed
(2) Access to physical infrastructure established or improved
(3) Global, regional and sub-regional mechanisms strengthened
(4) Development of ocean research policies in support of sustainable development objectives promoted
(5) Visibility and awareness increased
(6) Sustained (long-term) resource mobilization reinforced.
A global program should aspire to contribute to the Ocean Decade O1 by committing to core principles of effective research capacity sharing and building (e.g., Hind et al., 2015):
(1) Co-development and co-creation of contributing regional research projects. To ensure a truly global and inclusive program, the community should consider their proposed research region, and actively seek early engagement with other region-based collaborators to facilitate co-design and development of research plans and funding applications. Particularly in the case of Small Island Developing States (SIDS), a.k.a. Large Ocean States, local/indigenous methodologies and epistemologies have great potential in ocean observations from under sampled locations as well as knowledge production. Many countries increasingly focus on applying traditional ecological knowledge to coastal and shallow marine research, but less so in deep-sea research. We propose that capacity sharing and building actions actively invest in and support diverse practitioners to pursue deep-sea research. A new generation of deep-sea scientists, from a more diverse geographic pool, would bring new perspectives and approaches to research in the open ocean. In addition, local/indigenous knowledge systems linked to the deep ocean should be given a voice and considered alongside natural science in evolving deep sea exploration targets and management.
(2) Investment in training for scientists from economically developing countries. Previous studies have identified the crucial need to develop human capacity for oceanographic research in economically developing countries, as well as examples of activities that can achieve this objective (Morrison et al., 2013; Miloslavich et al., 2018b). Research projects contributing to a global program should, where possible, include a budget for full participation of regional partners in ship-board training activities, as well as knowledge-exchange and networking activities, e.g., conference and meeting attendance (Stefanoudis et al., 2020). Projects should also consider small investments in local research infrastructure that may enable long-term data collection (see new technologies section below), allowing local researchers to continue producing data beyond specific projects (Hind et al., 2015).
(3) Sharing research products. Projects should assign time and resources for co-analysis of data and dissemination of research outcomes with regional partners. We also strongly recommend open access publication of research, a small but valuable step in international engagement under a global program.
Technological development forms the nexus in our ability to identify and generate ocean data, information and knowledge (Figure 1, O2). Access to technology is one of the barriers to broadening participation in deep-sea research (Figure 1, O1). The revised Roadmap for the Ocean Decade outlines the potential role for new technologies in helping researchers better measure biodiversity, functions of deep-sea ecosystems and cumulative impacts of ocean stressors, and define the carrying capacity of ocean ecosystems to sustain human impacts and economic development. Anticipated developments over the decade largely grouped into three areas: improved access to the oceans, in terms of exploration (spatial coverage), variability (temporal monitoring) and costs (low cost technology); improved extraction of information from observations including automated data processing; and democratization of the sharing of both data and knowledge obtained.
Historically, access to the deep ocean has been both expensive and sparse, reliant upon access to large ocean-going ships and deep submergence research assets (both human occupied and robotic) operated by relatively few nations and often in regions far away from the largest of Earth’s ocean basins. Moving beyond satellite-based remote sensing, other fields of ocean research have advanced the use of new technologies that enable larger-scale coverage for global-scale ocean investigations. For example, recently developed biogeochemical (BGC) ARGO floats extend the capabilities of the ARGO array to measure important parameters in the deep ocean including pH, oxygen, chlorophyll, nitrate, suspended particles and downwelling irradiance. Simultaneously, the development of autonomous surface vessels such as the sail drone have enabled completion of demonstration projects that couple persistent presence at the ocean surface with remote sensing satellite data to guide vehicles (equipped with suitable instrument payloads) to ground-truth observations from remote sensing data. Under the Ocean Decade, judicious use of deep gliders and long-range AUVs, reporting back to shore-based scientists via autonomous surface vessels, could begin to conduct first-pass exploration of remote portions of the deep ocean floor (German et al., 2012). Even this modest contribution could immediately begin to improve the efficiency and efficacy with which the science community can deploy the most expensive assets among the international research community (global-class research ships and deep diving submersible assets).
Although recent technological advances leave no part of the world’s deep ocean out of reach, the capacity to deploy those assets remains limited worldwide. The extreme expense associated with buying, operating and maintaining large-scale oceanographic infrastructure, including ships and deep - submergence facilities pushes them out of the reach of most developing countries. However, satellite-enabled telepresence has enabled many thousands of individuals across the planet to join in discoveries and investigations in the deep ocean in real time but only if they have internet access. Partnerships between research institutes, universities, museums, and aquaria that can offer free access to the video, annotation, and scientists themselves during an expedition could augment this access. However, broader participation in this discovery field clearly requires the development of low-cost and smaller technologies to apply to deep-sea research (Phillips et al., 2019).
Extraction of information from acquired data also offers ripe opportunity for technological advancement over the Decade. Miniaturization and increasingly lower power requirements for in situ sensors for multiple environmental parameters enable installation on an increasing number of platforms obviating the need for laborious analyses and increasing coverage in the oceans, both in terms of spatial coverage and temporal monitoring. In parallel, non-invasive identification of species through genetic samples via environmental DNA (eDNA) sequencing provides an important, portable, and non-invasive technique enabled by emerging sequencing technology (Mariani et al., 2019). The approach offers an exciting opportunity to quickly analyze the diversity of fauna present within any given environment, although lack of an effective reference library to identify sequences by comparisons constrains application of the approach to the deep-sea biota (Howell et al., 2019). Many deep-sea eukaryotic species have never been sequenced before, or may be species new to science, and eDNA therefore cannot yet offer definitive identification. An emphasis on well-curated physical specimens entrusted to museums and the principles of open data sharing will overcome this bottleneck to identifying many deep-sea species.
In parallel, the increasing volumes of image and video data we anticipate scientists will acquire over the decade, not just from conventional methods but from expanded use of ship-free deep-ocean robotic assets will require a comparable ramp-up in the throughput of image analysis, and highlight a need for software solutions to improve pipeline processing and automatic analysis. Howell et al. (2019) identify the requirement for manual image analysis as a significant bottleneck in image-based marine ecological survey and monitoring, and that artificial intelligence (AI) and computer vision (CV) offer a potential means by which to both accelerate and standardize the interpretation of ecological image-based data (Piechaud et al., 2019). However, significant barriers to further development of these methods remain, including the lack of a standard morphospecies reference image catalog against which to base identifications and annotations. Such a catalog is in development for deep-sea fauna (Howell et al., 2019), and other potentially useful classification schemes already exist (Althaus et al., 2015). The deep-sea research community should prioritize agreement on a standard approach in order to expedite the use of AI and CV.
Technological advance will form a significant aspect of the Ocean Decade, and a global program should seek to contribute to, and benefit from, these developments in progressing toward the achieving the SOs.
In this paper, we have reviewed research needs of the Ocean Decade in the context of the design of a new 10 year deep-sea biology research program. This paper offers a blueprint for the further development of a global program as an official ‘Action’ of the Ocean Decade that we name here Challenger 150. Scientists and the public alike associate the name “Challenger” with exploration of new frontiers. One hundred and fifty years ago ‘HMS Challenger’ spent 4 years circumnavigating the globe, mapping the seafloor, recording the global ocean temperature, and providing us with a first panoramic view of life in the deep sea. Rightly scientists now attribute the birth of deep-sea biology and oceanography to HMS Challenger. The NASA space program later used the same name, first for the Apollo 17 Lunar Module that landed on the Moon in 1972, and later forthe space shuttle Challenger that flew the first American woman, African-American, Dutchman and Canadian into space. Today the name describes the deepest point of the ocean, the bottom of the Mariana Trench where, in January 1960 Jacques Piccard and Don Walsh made the first human descent to the Challenger Deep in the bathyscaphe Trieste. More recently, in March 2012 film director James Cameron made the first solo descent in the deep-submergence vehicle Deepsea Challenger. We use the name Challenger here to invoke the same spirit of exploration embodied by the previous Challengers, and to recognize the importance of that first global deep-sea biological dataset that, for some parts of the ocean, remain the only data available. However, we fully acknowledge that past exploration involved colonialism and exclusion. We advocate that in keeping with the Ocean Decade objectives Challenger 150 should forge a new inclusive, representative and equitable face for an historic name.
We present Challenger 150 as a concept for an Ocean Decade ‘Program-level Action’ as defined in the draft Implementation Plan. It would serve as a community-led collaborative endeavor in the stepwise development of a coherent, well-designed, deep-sea global survey and monitoring program. The concept would be realized through individual research projects committing to align with the blueprint presented here, and in so doing, becoming a piece in a larger global jigsaw puzzle. Such a program would require an unprecedented level of communication and coordination between research projects over the course of the Ocean Decade, and thus the program would require an effective management structure established to coordinate with, and support the community to follow the outlined design criteria regionally, annually reviewing progress toward the overall global design and Ocean Decade SOs and Os (Figure 2). We envision a process where-by individual projects, supported by a diverse range of funders, will formally align with the Challenger 150 program and the recommendations within this text. Lead PIs of projects from the same region, together with other relevant regional researchers will form a regional field committee (Figure 2A) to coordinate and monitor fieldwork efforts at that scale, and to support regional teams to develop new projects to fill survey gaps over the course of the Ocean Decade (Figure 2B). They will also interact with a regionally relevant stakeholder pool to ensure field projects complement research occurring within other disciplines, and remain aligned with end-user needs. Development of these regionally relevant stakeholder forums will draw upon existing regionally relevant bodies, for example the Second International Indian Ocean Expedition in the Indian Ocean, or the Benguela Current Commission in the South East Atlantic. Representative membership of each regional field committee will sit on the Challenger 150 steering committee to ensure coordination of field projects at a global scale, and monitor progress against the program aims and global survey design. The steering committee will report on progress to the IOC. They will also interact with a global stakeholder pool specific to the program, or possibly at the level of the IOC, where a global stakeholder pool may interact with multiple Ocean Decade programs. A communications and education team will coordinate activities between projects, and liaise with the IOC’s communications and education team, to ensure joined up efforts between different Ocean Decade programs. Participating research projects would be required to commit to use of new knowledge, as it accumulates, to address the Ocean Decade SOs and Os. By the end of the Ocean Decade the data from all projects could be combined to provide ‘one giant decadal leap’ for human knowledge of the deep ocean. Through this approach, contributing projects could potentially make a major contribution to both the Ocean Decade (Table 3) and SDG14, that simply could not be achieved working in isolation.
Figure 2. A framework for realizing the Challenger 150 Ocean Decade ‘Program-level Action’ concept, using the South Atlantic as an example region. (A) A proposed management structure for the program. (B) An example of how different projects operating within a region will be monitored against the global design and gaps in survey coverage identified.
Table 3. What an inclusive global program could deliver against each of the relevant Ocean Decade societal outcomes and by inference SDG14.
The revised Roadmap for the Ocean Decade calls upon the scientific community to think beyond business as usual and aspire for real change in the level of knowledge of the ocean in support of sustainable development. The implementation plan foresees a funding resource base that is multi-actor in nature, broad and flexible, taking a variety of forms. As we stand on the brink of the Ocean Decade, the Challenger 150 concept seeks to coordinate global research efforts in deep-sea ecology and oceanography for the express purpose of contributing to the delivery of the Ocean Decade SOs. However, success depends upon the nations and their research communities mobilizing and obtaining funding to support such efforts. Ship time represents a significant cost, and the only realistic option for some nations will be to seek public-private or philanthropic partnerships such as the REV Ocean, Schmidt Ocean Institute, Ocean Exploration Trust, Nekton Foundation, OceanX, The International Seakeepers Society, and industry to provide access to appropriate platforms. Industry could play a particularly important role to play in less economically developed countries. The IOC and REV Ocean have already agreed on several areas of collaboration under the Ocean Decade, including use of the REV Ocean vessel, offering a real opportunity to advance the Challenger 150 concept in the identified priority areas for new biological data collection. For other nations who can access large infrastructure, national, regional and bilateral funding mechanisms may be more appropriate or accessible as a means to fund contributing projects.
Although data collection represents a challenge, data processing, interpretation, archiving and storage represent a significant and on-going cost that at present can only be met by multiple applications for funding from national research budgets or philanthropic mechanisms. Regardless of where scientists apply for funding, alignment of projects with the blueprint outlined here as part of a community led, globally coordinated 10 year program brings greater opportunity for both efficiency and impact. Therefore projects aligned with the Challenger 150 concept may appear more attractive to funders thus offering benefits to the wider community.
The Ocean Decade begins on the 1st January 2021 and already the deep-sea research community has begun to sow the first seeds of coordination between projects to contribute to a global program via the Deep Ocean Stewardship Initiative’s (DOSI) Decade of Ocean Science working group and the Scientific Committee on Oceanic Research (SCOR) working group 159. We hope that the blueprint provided in this paper helps the wider deep-sea community to engage with the Challenger 150 concept as a shared endeavor; to forge regional and inclusive consortia, co-develop research plans and funding bids aligned with the blueprint, and help achieve the SOs and Os of the Ocean Decade.
(1) uses the revised GOODS classification (Watling et al., 2013) and Sutton et al. (2017) mesopelagic ecoregions to stratify respectively benthic and pelagic deep-sea survey and monitoring, ensuring an overall stratification by latitude.
(2) uses the following indicative depth horizons as a general guide for a target range for all biogeographic regions to achieve an unbiased dataset: 150 — 300, 300 — 500 then every 500 m to the deepest point of the oceans at 10 km.
(3) uses the following indicative horizontal distances as a general guide for component projects to stratify sampling by: 1 m, 10 m, 100 m, 1 km, 10 km, 100 km.
(4) uses the following replicated treatments in regional designs where possible: high and low climate hazard under early/late time of climate change emergence, fished/unfished, near/far from sources of pollution, licensed/protected from industry activities.
(5) stratifies sampling by substrate type and / or topography, including slope, within regional designs.
(6) uses the following criteria in selection of sites for potential monitoring: access to different strata outlined in the global design, availability of existing observing infrastructure, opportunity for and ease of installing and maintaining new infrastructure.
(7) prioritizes research effort in southern and polar latitudes, deeper depths, and midwater environments.
(8) considers additional ecosystem-specific stratification in addition to those of the main design (Table 1).
(9) uses Table 2, and the papers cited within, to provide guidance on what to measure and how in order that the data can be used to help deliver the SOs; and visit the OBPS digital repository at oceanbestpractices.org for more specific guidance.
(10) ensures that targeted physical specimen sampling form an important part of the program.
(11) follows the following guiding principles in both data and specimen archiving: rapid accession and minimal embargo, a commitment to collect broadly, FAIR, CARE.
(12) commits to and provide for the deposition of specimens with an established regionally relevant museum.
(13) commits to core principles of effective research capacity sharing and building, including engagement with local and indigenous communities.
(14) seeks to contribute to, and benefit from, technological developments in progressing toward the achieving the SOs.
KH and AH conceived the idea and convened the working group. KH, AH, AM, LL, ER-L, MB, PVRS, JX, HW, MC, EE, CG, SR, PE, and LM helped to conceive the manuscript and outline structure. All authors contributed ideas, text, and edits.
Development of this paper was supported by funding from the Scientific Committee on Oceanic Research (SCOR) awarded to KH and AH as working group 159 co-chairs. KH, BN, and KS are supported by the UKRI funded One Ocean Hub NE/S008950/1. AH work is supported by the CESAM (UIDP/50017/2020 + 1432 UIDB/50017/2020) that is funded by Fundação para a Ciência e a Tecnologia (FCT)/MCTES through national funds. AA is supported by Science Foundation Ireland and the Marine Institute under the Investigators Program Grant Number SFI/15/IA/3100 co-funded under the European Regional Development Fund 2014–2020. AC is supported through the FunAzores -ACORES 01-0145-FEDER-000123 grant and by FCT through strategic project UID/05634/2020 and FCT and Direção-Geral de Politica do Mar (DGPM) through the project Mining2/2017/005. PE is funded by national funds (OE), through FCT in the scope of the framework contract foreseen in the numbers 4, 5 and 6 of the article 23, of the Decree-Law 57/2016, of August 29, changed by Law 57/2017, of July 19. SG research is supported by CNRS funds. CG is supported by an Independent Study Award and the Investment in Science Fund at WHOI. KG gratefully acknowledges support from Synchronicity Earth. LL is funded by the NOAA Office of Ocean Exploration and Research (NA19OAR0110305) and the US National Science Foundation (OCE 1634172). NM is supported by FCT and DGPM, through the project Mining2/2017/001 and the FCT grants CEECIND/00526/2017, UIDB/00350/2020 + UIDP/00350/2020. SR is funded by the FCTgrant CEECIND/00758/2017. JS is supported by ANID FONDECYT #1181153 and ANID Millennium Science Initiative Program #NC120030. JX research is funded by the European Union’s Horizon 2020 research and innovation program through the SponGES project (grant agreement no. 679849) and further supported by national funds through FCT within the scope of UIDB/04423/2020 and UIDP/04423/2020. The Natural Sciences and Engineering Council of Canada supports AM and PVRS. MB and the Deep-Ocean Stewardship Initiative are supported by Arcadia - A charitable fund of Lisbet Rausing and Peter Baldwin. BN work is supported by the NERC funded Arctic PRIZE NE/P006302/1.
The authors declare that the research was conducted in the absence of any commercial or financial relationships that could be construed as a potential conflict of interest.
Allen, J. A. (2008). Bivalvia of the deep Atlantic. Malacologia 50, 57–173. doi: 10.4002/0076-2997-50.1.57
Althaus, F., Hill, N., Ferrari, R., Edwards, L., Przeslawski, R., Schönberg, C. H., et al. (2015). A standardised vocabulary for identifying benthic biota and substrata from underwater imagery: the CATAMI classification scheme. PLoS One 10:e0141039. doi: 10.1371/journal.pone.0141039
AMAP (2017). Snow, Water, Ice and Permafrost in the Arctic (SWIPA) 2017. Oslo: Arctic Monitoring and Assessment Programme.
Armstrong, C. W., Foley, N. S., Tinch, R., and van den Hove, S. (2012). Services from the deep: steps towards valuation of deep sea goods and services. Ecosyst. Serv. 2, 2–13. doi: 10.1016/j.ecoser.2012.07.001
Arrigo, K. R., van Dijken, G., and Pabi, S. (2008). Impact of a shrinking Arctic ice cover on marine primary production. Geophys. Res. Lett. 35, 1–6. doi: 10.1029/2008GL035028
Arrigo, K. R., and van Dijken, G. L. (2015). Continued increases in Arctic Ocean primary production. Prog. Oceanogr. 136, 60–70. doi: 10.1016/j.pocean.2015.05.002
Ashford, O. S., Kenny, A. J., Barrio Frojan, C. R. S., Bonsall, M. B., Horton, T., Brandt, A., et al. (2018). Phylogenetic and functional evidence suggests that deep-ocean ecosystems are highly sensitive to environmental change and direct human disturbance. Proc. R. Soc. B Biol. Sci. 285:20180923. doi: 10.1098/rspb.2018.0923
Ashford, O. S., Kenny, A. J., Barrio Froján, C. R. S., Horton, T., and Rogers, A. D. (2019). Investigating the environmental drivers of deep-seafloor biodiversity: a case study of peracarid crustacean assemblages in the Northwest Atlantic Ocean. Ecol. Evol. 9, 14167–14204. doi: 10.1002/ece3.5852
Baco, A. R., Etter, R. J., Ribeiro, P. A., von, der Heyden, S., Beerli, P., et al. (2016). A synthesis of genetic connectivity in deep-sea fauna and implications for marine reserve design. Mol. Ecol. 25, 3276–3298. doi: 10.1111/mec.13689
Bailey, D. M., Collins, M. A., Gordon, J. D. M., Zuur, A. F., and Preide, I. G. (2009). Long-term changes in deep-water fish populations in the North East Atlantic: a deeper reaching effect of fisheries? Proc. R. Soc. B 276, 1965–1969. doi: 10.1098/rspb.2009.0098
Baker, M., Ramirez-Llodra, E., and Tyler, P. A. (2020). Natural Capital and Exploitation of the Deep Sea. Oxford: Oxford University Press.
Baldwin, C., Tornabene, L., and Robertson, D. (2018). Below the mesophotic. Sci. Rep. 8:4920. doi: 10.1038/s41598-018-23067-1
Battaglia, P., Andaloro, F., Consoli, P., Esposito, V., Malara, D., Musolino, S., et al. (2013). Feeding habits of the Atlantic Bluefin Tuna, Thunnus Thynnus (L. 1758), in the Central Mediterranean Sea (Strait of Messina). Helgol. Mar. Res. 67, 97–107. doi: 10.1007/s10152-012-0307-2
Beaulieu, S. E., Baker, E. T., and German, C. R. (2015). Where are the undiscovered hydrothermal vents on oceanic spreading ridges? Deep-Sea Res. 121, 202–212. doi: 10.1016/j.dsr2.2015.05.00
Bernhardt, A., Melnick, D., Jara-Muñoz, J., Argandoña, B., González, J., and Strecker, M. R. (2015). Controls on submarine canyon activity during sea-level highstands: the Biobío canyon system offshore Chile. Geosphere 11, 1226–1255. doi: 10.1130/GES01063.1
Bianchelli, S., Gambi, C., Mea, M., Pusceddu, A., and Danovaro, R. (2013). Nematode diversity patterns at different spatial scales in bathyal sediments of the Mediterranean Sea. Biogeosciences 10, 5465–5479. doi: 10.5194/bg-10-5465-2013
Billett, D. S. M., Bett, B. J., Reid, W. D. K., Boorman, B., and Priede, M. (2010). Long-term change in the abyssal NE Atlantic: the ‘Amperima event’ revisited. Deep Sea Res. Part II 57, 1406–1417. doi: 10.1016/j.dsr2.2009.02.001
Bindoff, N. L., Cheung, W. W. L., Kairo, J. G., Arístegui, J., Guinder, V. A., Hallberg, R., et al. (2019). “Changing ocean, marine ecosystems, and dependent communities,” in IPCC Special Report on the Ocean and Cryosphere in a Changing Climate, eds H.-O. Pörtner, D. C. Roberts, V. Masson-Delmotte, P. Zhai, M. Tignor, E. Poloczanska, et al. (Geneva: IPCC).
Blake, J. A., and Grassle, J. F. (1994). Benthic community structure on the US South Atlantic slope off the Carolinas: spatial heterogeneity in a current-dominated system. Deep Sea Res. Part II 41, 835–874. doi: 10.1016/0967-0645(94)90051-5
Bodil, B. A., Ambrose, W. G. Jr., Bergmann, M., Clough, L. M., Gebruk, A. V., Hasemann, C., et al. (2011). Diversity of the Arctic deep-sea benthos. Mar. Biodivers. 41, 87–107. doi: 10.1007/s12526-010-0078-4
Boetius, A., Bach, W., Borowski, C., Diehl, A., German, C., Kaul, N., et al. (2015). Exploring the Habitability of Ice-Covered Waterworlds: the Deep-Sea hydrothermal system of the Aurora Mount at Gakkel Ridge, Arctic Ocean (82°54’ N, 6°15W, 4000 m). Proceedings of the Fall Meeting. San Francisco, CA: American Geophysical Union.
Brandt, A., Ellingsen, K. E., Brix, S., Brökeland, W., and Malyutina, M. (2005). Southern Ocean deep-sea isopod species richness (Crustacea, Malacostraca): influences of depth, latitude and longitude. Polar Biol. 28, 284–289. doi: 10.1007/s00300-004-0688-z
Breitburg, D., Levin, L. A., Oschlies, A., Grégoire, M., Chavez, F. P., Conley, D. J., et al. (2018). Declining oxygen in the global ocean and coastal waters. Science 359:6371. doi: 10.1126/science.aam7240
Brito-Morales, I., Schoeman, D. S., Molinos, J. G., Burrows, M. T., Klein, C. J., Arafeh-Dalmau, N., et al. (2020). Climate velocity reveals increasing exposure of deep-ocean biodiversity to future warming. Nat. Clim. Chang. 10, 576–581. doi: 10.1038/s41558-020-0773-5
Brown, C. J., Smith, S. J., Lawton, P., and Anderson, J. T. (2011). Benthic habitat mapping: a review of progress towards improved understanding of the spatial ecology of the seafloor using acoustic techniques. Estuar. Coast. Shelf Sci. 92, 502–520. doi: 10.1016/j.ecss.2011.02.007
CAFF (2017). State of the Arctic Marine Biodiversity Report. Akureyri: Conservation of Arctic Flora and Fauna International Secretariat.
Carney, R. S. (2005). Zonation of deep biota on continental margins. Oceanog. Mar. Biol. 43, 211–278. doi: 10.1201/9781420037449.ch6
Chapman, A. S., Beaulieu, S. E., Colaço, A., Gebruk, A. V., Hilario, A., Kihara, T. C., et al. (2019). sFDvent: a global trait database for deep−sea hydrothermal−vent fauna. Glob. Ecol. Biogeogr. 28, 1538–1551. doi: 10.1111/geb.12975
Clark, M. R., Althaus, F., Schlacher, T. A., Williams, A., Bowden, D. A., and Rowden, A. A. (2016a). The impacts of deep-sea fisheries on benthic communities: a review. ICES J. Mar. Sci. 73, 151–169. doi: 10.1093/icesjms/fsv123
Clark, M. R., Consalvey, M., and Rowden, A. A. (2016b). Biological Sampling in the Deep Sea. Chichester: John Wiley and Sons.
Clark, M. R., Rowden, A. A., Schlacher, T., Williams, A., Consalvey, M., Stocks, K. I., et al. (2010). The ecology of seamounts: structure, function, and human impacts. Annu. Rev. Mar. Sci. 2, 253–278. doi: 10.1146/annurev-marine-120308-081109
Clark, M. R., Schlacher, T. A., Rowden, A. A., Stocks, K. I., and Consalvey, M. (2012). Science priorities for seamounts: research links to conservation and management. PLoS One 7:e29232. doi: 10.1371/journal.pone.0029232
Clarke, A. (2008). Antarctic marine benthic diversity: patterns and processes. J. Exp. Mar. Biol. Ecol. 366, 48–55. doi: 10.1016/j.jembe.2008.07.008
Colaço, A., Giacomello, E., Porteiro, F., and Menezes, G. M. (2013). Trophodynamic studies on the condor seamount (Azores, Portugal, North Atlantic). Deep-Sea Res. Pt II. 98, 178–189. doi: 10.1016/j.dsr2.2013.01.010
Cordes, E. E., Cunha, M. R., Galéron, J., Mora, C., Olu-Le Roy, K., Sibuet, M., et al. (2010). The influence of geological, geochemical, and biogenic habitat heterogeneity on seep biodiversity. Mar. Ecol. 31, 51–65. doi: 10.1111/j.1439-0485.2009.00334.x
Corliss, B. H., Brown, C. W., Sun, X., and Showers, W. J. (2009). Deep-sea benthic diversity linked to seasonality of pelagic productivity. Deep Sea Res. Part I 56, 835–841. doi: 10.1016/j.dsr.2008.12.009
Costanza, R. (1999). The ecological, economic, and social importance of the oceans. Ecol. Econ. 31, 199–213. doi: 10.1016/S0921-8009(99)00079-8
Courtene-Jones, W., Quinn, B., Ewins, C., Gary, S. F., and Narayanaswamy, B. E. (2020). Microplastic accumulation in deep-sea sediments from the Rockall Trough. Mar. Pollut. Bull. 154, 111092. doi: 10.1016/j.marpolbul.2020.111092
Courtene-Jones, W., Quinn, B., Gary, S. F., Mogg, A. O. M., and Narayanaswamy, B. E. (2017). Microplastic pollution identified in deep-sea water and ingested by benthic invertebrates in the Rockall Trough, North Atlantic Ocean. Environ. Pollut. 231(Pt 1), 271–280. doi: 10.1016/j.envpol.2017.08.026
Cowen, R. K., and Sponaugle, S. (2009). Larval dispersal and marine population connectivity. Ann. Rev. Mar. Sci. 1, 443–466. doi: 10.1146/annurev.marine.010908.163757
Culver, S. J., and Buzas, M. A. (2000). Global latitudinal species diversity gradient in deep-sea benthic foraminifera. Deep Sea Res. Part I 47, 259–275. doi: 10.1016/S0967-0637(99)00055-2
Danovaro, R., Bianchelli, S., Gambi, C., Mea, M., and Zeppilli, D. (2009). α-, β-, γ-, δ-and ε-diversity of deep-sea nematodes in canyons and open slopes of Northeast Atlantic and Mediterranean margins. Mar. Ecol. Prog. Ser. 396, 197–209. doi: 10.3354/meps08269
Danovaro, R., Company, J. B., Corinaldesi, C., D’Onghia, G., Galil, B., Gambi, C., et al. (2010). Deep-Sea Biodiversity in the Mediterranean Sea: the known, the unknown, and the unknowable. PLoS One 5:e11832. doi: 10.1371/journal.pone.0011832
Danovaro, R., Corinaldesi, C., Dell’Anno, A., and Snelgrove, P. V. R. (2017). The deep-sea under global change. Curr. Biol. 27, R461–R465. doi: 10.1016/j.cub.2017.02.046
Danovaro, R., Fanelli, E., Aguzzi, J., Billett, D., Carugati, L., Corinaldesi, C., et al. (2020). Ecological variables for developing a global deep-ocean monitoring and conservation strategy. Nat. Ecol. Evol. 4, 181–192. doi: 10.1038/s41559-019-1091-z
Danovaro, R., Gambi, C., Dell’Anno, A., Corinaldesi, C., Fraschetti, S., Vanreusel, A., et al. (2008). Exponential decline of deep-sea ecosystem functioning linked to benthic biodiversity loss. Curr. Biol. 18, 1–8. doi: 10.1016/j.cub.2007.11.056
Danovaro, R., Snelgrove, P. V., and Tyler, P. (2014). Challenging the paradigms of deep-sea ecology. Trends Ecol. Evol. 29, 465–475. doi: 10.1016/j.tree.2014.06.002
Davies, A. J., and Guinotte, J. M. (2011). Global habitat suitability for framework-forming cold-water corals. PLoS One 6:e18483. doi: 10.1371/journal.pone.0018483
Day, D. S., and Pearcy, W. G. (1968). Species associations of benthic fishes on the continental shelf and slope off Oregon. J. Fish. Res. Board Can. 25, 2665–2675. doi: 10.1139/f68-236
Díaz, S., Settele, J., Brondízio, E., Ngo, H., and Guèze, M. (2019). Summary for Policymakers of the Global Assessment Report on Biodiversity and Ecosystem Services of the Intergovernmental Science-Policy Platform on Biodiversity and Ecosystem Services. Bonn: IPBES Secretariat.
Diesing, M. (2020). Deep-sea sediments of the global ocean. Earth Syst. Sci. Data Discuss. doi: 10.5194/essd-2020-22 [Epub ahead of print],
Dornelas, M., Antão, L. H., Moyes, F., Bates, A. E., Magurran, A. E., Adam, D., et al. (2018). BioTIME: a database of biodiversity time series for the Anthropocene. Glob. Ecol. Biogeogr. 27, 760–786. doi: 10.1111/geb.12729
Drazen, J. C., and Haedrich, R. L. (2012). A continuum of life histories in deep-sea demersal fishes. Deep. Res. Part I 61, 34–42. doi: 10.1016/j.dsr.2011.11.002
Dutkiewicz, S., Morris, J. J., Follows, M. J., Scott, J., Levitan, O., Dyhrman, S. T., et al. (2015). Impact of ocean acidification on the structure of future phytoplankton communities. Nat. Clim. Chang. 5, 1002–1006. doi: 10.1038/nclimate2722
Ehrlich, P. R., and Pringle, R. M. (2008). Where does biodiversity go from here? A grim business-as-usual forecast and a hopeful portfolio of partial solutions. Proc. Natl. Acad. Sci. U.S.A. 105, 11579–11586. doi: 10.1073/pnas.0801911105
Eleftheriou, A. (2013). Methods for the Study of Marine Benthos, 4th Edn. Oxford: John Wiley and Sons, Ltd.
Etter, R. J., and Mullineaux, L. S. (2001). Deep−sea Communities. Marine Community Ecology (ed. by M.D. Bertness, S.D. Gaines and M.E. Hay). Sunderland, MA: Sinauer Associates, Inc, 367–394.
Etter, R. J., Rex, M. A., Chase, M. C., and Quattro, J. M. (1999). A genetic dimension to deep-sea biodiversity. Deep Sea Res. Part I 46, 1095–1099. doi: 10.1016/S0967-0637(98)00100-9
FAO (2018). Deep-Ocean Climate Change Impacts on Habitat, Fish and Fisheries: FAO Fisheries and Aquaculture, (eds) L. A. Levin, M. Baker, and A. Thompson. Rome: FAO.
Fernandez-Arcaya, U., Ramirez-Llodra, E., Aguzzi, J., Allcock, A. L., Davies, J. S., Dissanayake, A., et al. (2017). Ecological role of submarine canyons and need for canyon conservation: a review. Front. Mar. Sci. 4:5. doi: 10.3389/fmars.2017.00005
Folkersen, M. V., Fleming, C. M., and Hasan, S. (2018). The economic value of the deep sea: a systematic review and meta-analysis. Mar. Policy 94, 71–80. doi: 10.1016/j.marpol.2018.05.003
Gage, J. D., Lambshead, P. J. D., Bishop, J. D. D., Stuart, C. T., and Jones, N. S. (2004). Large-scale biodiversity pattern of Cumacea (Peracarida: Crustacea) in the deep Atlantic. Mar. Ecol. Prog. Ser. 277, 181–196. doi: 10.3354/meps277181
Gallo, N. D., and Levin, L. A. (2016). Fish ecology and evolution in the world’s oxygen minimum zones and implications of ocean deoxygenation. Adv. Mar. Biol. 74, 117–198. doi: 10.1016/bs.amb.2016.04.001
Gennip, S. J. V., Dewitte, B., Garçon, V., Thiel, M., Popova, E., Drillet, Y., et al. (2019). In search for the sources of plastic marine litter that contaminates the Easter Island Ecoregion. Sci. Rep. 9:19662. doi: 10.1038/s41598-019-56012-x
German, C. R., Jakuba, M. V., Kinsey, J. C., Partan, J., Suman, S., Belani, A., et al. (2012). “A long term vision for long-range ship-free deep ocean operations: persistent presence through coordination of Autonomous Surface Vehicles and Autonomous Underwater Vehicles,” in Proceedings of the IEEE-Oceans (Autonomous Underwater Vehicles) Conference, (Southampton: National Oceanography Centre), 1–7.
German, C. R., Ramirez-Llodra, E., Baker, M. C., Tyler, P. A., and ChEss Scientific Steering Committee (2011). Deep- water chemosynthetic ecosystem research during the census of marine life decade and beyond: a proposed deep-ocean road map. PLoS One 6:e23529. doi: 10.1371/journal.pone.0023259
Giménez, J., Marçalo, A., García−Polo, M., García−Barón, I., Castillo, J. J., Fernández−Maldonado, C., et al. (2018). Feeding ecology of Mediterranean common dolphins: the importance of mesopelagic fish in the diet of an endangered subpopulation. Mar. Mammal Sci. 34, 136–154. doi: 10.1111/mms.12442
Glover, A., and Smith, C. (2003). The deep-sea floor ecosystem: current status and prospects of anthropogenic change by the year 2025. Environ. Conserv. 30, 219–241. doi: 10.1017/S0376892903000225
Glover, A. G., Wiklund, H., Chen, S., and Dahlgren, T. G. (2018). Managing a sustainable deep-sea “blue economy” requires knowledge of what actually lives there. eLife 7:e41319. doi: 10.7554/eLife.41319
Gómez, C. E., Wickes, L., Deegan, D., Etnoyer, P. J., and Cordes, E. E. (2018). Growth and feeding of deep-sea coral Lophelia pertusa from the California margin under simulated ocean acidification conditions. PeerJ 6:e5671. doi: 10.7717/peerj.5671
Gooday, A. J., Turley, C. M., and Allen, J. (1990). Responses by benthic organisms to inputs of organic material to the ocean floor: a review. Philos. Trans. R. Soc. Lond. Ser. A Math. Phys. Sci. 331, 119–138. doi: 10.1098/rsta.1990.0060
Grassle, J. F. (1989). Species diversity in deep-sea communities. Trends Ecol. Evol. 4, 12–15. doi: 10.1016/0169-5347(89)90007-4
Grassle, J. F., and Maciolek, N. J. (1992). Deep-sea species richness: regional and local diversity estimates from quantitative bottom samples. Am. Nat. 139, 313–341. doi: 10.1086/285329
Guinotte, J. M., Orr, J., Cairns, S., Freiwald, A., Morgan, L., and George, R. (2006). Will human induced changes in seatwater chemistry alter the distribution of deep-sea scleractinian corals? Front. Ecol. Environ. 4:141–146. doi: 10.1890/1540-92952006004[0141:WHCISC]2.0.CO;2
Gutt, J., Barratt, I., Domack, E., D’acoz, C. D. U., Dimmler, W., Grémare, A., et al. (2011). Biodiversity change after climate-induced ice-shelf collapse in the Antarctic. Deep Sea Res. 58, 74–83. doi: 10.1016/j.dsr2.2010.05.024
Haedrich, R. L., Rowe, G. T., and Polloni, P. T. (1975). Zonation and faunal composition of epibenthic populations on the continental slope south of New England. J. Mar. Res. 33, 191–212.
Harris, P. T., Macmillan-Lawler, M., Rupp, J., and Baker, E. K. (2014). Geomorphology of the oceans. Mar. Geol. 352, 4–24. doi: 10.1016/j.margeo.2014.01.011
Havermans, C., Sonet, G., d’Acoz, C. D. U., Nagy, Z. T., Martin, P., Brix, S., et al. (2013). Genetic and morphological divergences in the cosmopolitan deep-sea amphipod Eurythenes gryllus reveal a diverse abyss and a bipolar species. PLoS One 8:e74218. doi: 10.1371/journal.pone.0074218
Helly, J. J., and Levin, L. A. (2004). Global distribution of naturally occurring marine hypoxia on continental margins. Deep Sea Res. Pt. I. 51, 1159–1168. doi: 10.1016/j.dsr.2004.03.009
Heymans, J. J., Howell, K. L., Ayers, M., Burrows, M. T., Gordon, J. D., Jones, E. G., et al. (2011). Do we have enough information to apply the ecosystem approach to management of deep-sea fisheries? An example from the West of Scotland. ICES J. Mar. Sci. 68, 265–280. doi: 10.1093/icesjms/fsq065
Hilário, A., Metaxas, A., Gaudron, S., Howell, K., Mercier, A., Mestre, N., et al. (2015). Estimating dispersal distance in the deep sea: challenges and applications to marine reserves. Front. Mar. Sci. 2:6. doi: 10.3389/fmars.2015.00006
Hillebrand, H. (2004). Strength, slope and variability of marine latitudinal gradients. Mar. Ecol. Prog. Ser. 273, 251–267. doi: 10.1371/journal.pone.0067596
Hind, E. J., Alexander, S. M., Green, S. J., Kritzer, J. P., Sweet, M. J., Johnson, A. E., et al. (2015). Fostering effective international collaboration for marine science in small island states. Front. Mar. Sci. 2:86. doi: 10.3389/fmars.2015.00086
Hoffmann, F., Radax, R., Woebken, D., Holtappels, M., Lavik, G., Rapp, H. T., et al. (2009). Complex nitrogen cycling in the sponge Geodia barretti. Environ. Microbiol. 11, 2228–2243. doi: 10.1111/j.1462-2920.2009.01944.x
Howell, K. L., Billett, D. S., and Tyler, P. A. (2002). Depth-related distribution and abundance of seastars (Echinodermata: Asteroidea) in the porcupine seabight and porcupine abyssal plain. N.E. Atlantic. Deep Sea Res. I. 49, 1901–1920. doi: 10.1016/S0967-0637(02)00090-0
Howell, K. L., Davies, J. S., Allcock, A. L., Braga-Henriques, A., Buhl-Mortensen, P., Carreiro-Silva, M., et al. (2019). A framework for the development of a global standardised marine taxon reference image database (SMarTaR-ID) to support image-based analyses. PLoS One 14:e0218904. doi: 10.1371/journal.pone.0218904
Howell, K. L., Heymans, J. J., Gordon, J. D. M., Duncan, J., Ayers, M., and Jones, E. G. (2009). “DEEPFISH Project: Applying an Ecosystem Approach to the Sustainable Management of Deep-Water Fisheries. Part 1 and 2: Development of the Ecopath with Ecosim model,” in Scottish Association for Marine Science Report (Oban: SAMS).
Howell, K. L., Piechaud, N., Downie, A. L., and Kenny, A. (2016). The distribution of deep-sea sponge aggregations in the North Atlantic and implications for their effective spatial management. Deep Sea Res. I 115, 309–320. doi: 10.1016/j.dsr.2016.07.005
Huang, Z., Nichol, S. L., Harris, P. T., and Caley, M. J. (2014). Classification of submarine canyons of the Australian continental margin. Mar. Geol. 357, 362–383. doi: 10.1016/j.margeo.2014.07.007
Humboldt, A., and Bonpland, A. (1807). Essai sur la Geographie des Plantes Accompagne d’une Tableau Physique des Regions Equinoxiales. New York, NY: Arno Press.
IPCC (2019). in IPCC Special Report on the Ocean and Cryosphere in a Changing Climate, eds H. O. Pörtner, D. C. Roberts, V. Masson-Delmotte, P. Zhai, M. Tignor, E. Poloczanska, et al. (Geneva: IPCC).
Irigoien, X., Klevjer, T. A., Røstad, A., Martinez, U., Boyra, G., Acuña, J. L., et al. (2014). Large mesopelagic fishes biomass and trophic efficiency in the open ocean. Nat. Commun. 5:3271. doi: 10.1038/ncomms4271
Jamieson, A. J., Brooks, L. S. R., Reid, W. D., Piertney, S. B., Narayanaswamy, B. E., and Linley, T. D. (2019). Microplastics and synthetic particles ingested by deep-sea amphipods in six of the deepest marine ecosystems on Earth. R. Soc. Open Sci. 6:180667. doi: 10.1098/rsos.180667
Jamieson, A. J., Fujii, T., Mayor, D. J., Solan, M., and Priede, I. G. (2010). Hadal trenches: the ecology of the deepest places on Earth. Trends Ecol. Evol. 25, 190–197. doi: 10.1016/j.tree.2009.09.009
Jamieson, A. J., Malkocs, T., Piertney, S. B., Fujii, T., and Zhang, Z. (2017). Bioaccumulation of persistent organic contaminants in the deepest ocean fauna. Nat. Ecol. Evol. 1, 1–4. doi: 10.1038/s41559-016-0051
Jenkins, T. L., and Stevens, J. R. (2018). Assessing connectivity between MPAs: selecting taxa and translating genetic data to inform policy. Mar. Pol. 94, 165–173. doi: 10.1016/j.marpol.2018.04.022
Jones, D. O. B., Kaiser, S., Sweetman, A. K., Smith, C. R., Menot, L., Vink, A., et al. (2017). Biological responses to disturbance from simulated deep-sea polymetallic nodule mining. PLoS One 12:e0171750. doi: 10.1371/journal.pone.0171750
Jumars, P. A. (1975). Environmental grain and polychaete species’ diversity in a bathyal benthic community. Mar. Biol. 30, 253–266. doi: 10.1007/BF00390748
Jumars, P. A. (1976). Deep-sea species diversity: does it have a characteristic scale. J. Mar. Res. 34, 217–246.
Jumars, P. A., and Eckman, S. (1983). “Spatial structure within deep-sea benthic communities,” in The sea, Deep-sea biology, Vol. 8, ed. G. T. Rowe (New York, NY: Wiley), 399–452.
Kaartvedt, S., Staby, A., and Aksnes, D. L. (2012). Efficient trawl avoidance by mesopelagic fishes causes large underestimation of their biomass. Mar. Ecol. Prog. Ser. 456, 1–5. doi: 10.3354/meps09785
Laffoley, D., and Baxter, J. M. (2019). Ocean Deoxygenation: Everyone’s problem – Causes, Impacts, Consequences and Solutions. Full Report. Gland: IUCN, 580.
Lambshead, P. J. D., Brown, C. J., Ferrero, T. J., Mitchell, N. J., Smith, C. R., Hawkins, L. E., et al. (2002). Latitudinal diversity patterns of deep-sea marine nematodes and organic fluxes: a test from the central equatorial Pacific. Mar. Ecol. Prog. Ser. 236, 129–135. doi: 10.3354/meps236129
Lamont, P. A., Gage, J. D., and Tyler, P. A. (1995). Deep-sea macrobenthic communities at contrasting sites off Portugal, preliminary results: II Spatial dispersion. Int. Rev. Gesamten Hydrobiol. Hydrogr. 80, 251–265. doi: 10.1002/iroh.19950800212
Leduc, D., Rowden, A. A., Bowden, D. A., Nodder, S. D., Probert, P. K., Pilditch, C. A., et al. (2012). Nematode beta diversity on the continental slope of New Zealand: spatial patterns and environmental drivers. Mar. Ecol. Prog. Ser 454, 37–52. doi: 10.3354/meps09690
Levin, L., Betts, B. J., Gates, A. R., Heimbach, P., Howe, B. M., Janssen, F., et al. (2019). Global observational needs in the deep ocean. Front. Mar. Sci. 6:241. doi: 10.3389/fmars.2019.00241
Levin, L., and Gooday, A. J. (2003). “The deep atlantic ocean,” in Ecosystems of the Deep Oceans. Ecosystems of the World, Vol. 28, ed. P. A. Tyler, (Amsterdam: Elsevier), 111–178.
Levin, L. A. (2018). Manifestation, drivers, and emergence of open ocean deoxygenation. Annu. Rev. Mar. Sci. 10, 229–260. doi: 10.1146/annurev-marine-121916-163359
Levin, L. A., Baco, A. R., Bowden, D. A., Colaco, A., Cordes, E. E., Cunha, M. R., et al. (2016a). Hydrothermal vents and methane seeps: rethinking the sphere of influence. Front. Mar. Sci. 3:580. doi: 10.3389/fmars.2016.00072
Levin, L. A., Etter, R. J., Rex, M. A., Gooday, A. J., Smith, C. R., Pineda, J., et al. (2001). Environmental influences on regional deep-sea species diversity. Annu. Rev. Ecol. Evol. Syst. 32, 51–93.
Levin, L. A., Mendoza, G. F., and Grupe, B. M. (2016b). Methane seepage effects on biodiversity and biological traits of macrofauna inhabiting authigenic carbonates. Deep Sea Res. Pt II 137, 1–16. doi: 10.1016/j.dsr2.2016.05.021
Levin, L. A., Wei, C. L., Dunn, D. C., Amon, D. J., Ashford, O. S., Cheung, W. W. L., et al. (2020). Climate change considerations are fundamental to management of deep-sea resource extraction. Glob. Change Biol. 26, 4664–4678. doi: 10.1111/gcb.15223
Lutz, M. J., Caldeira, K., Dunbar, R. B., and Behrenfeld, M. J. (2007). Seasonal rhythms of net primary production and particulate organic carbon flux to depth describe the efficiency of biological pump in the global ocean. J. Geophys. Res. Oceans 112:C10011. doi: 10.1029/2006jc003706
Maldonado, M., López-Acosta, M., Sitjà, C., García-Puig, M., Galobart, C., Ercilla, G., et al. (2019). Sponge skeletons as an important sink of silicon in the global oceans. Nat. Geosci. 12, 815–822. doi: 10.1038/s41561-019-0430-7
Manea, E., Bianchelli, S., Fanelli, E., Danovaro, R., and Gissi, E. (2020). Towards an ecosystem-based marine spatial planning in the deep Mediterranean Sea. Sci. Total Environ. 715:136884. doi: 10.1016/j.scitotenv.2020.136884
Mariani, S., Baillie, C., Colosimo, G., and Riesgo, A. (2019). Sponges as natural environmental DNA samplers. Curr. Biol. 29, R401–R402. doi: 10.1016/j.cub.2019.04.031
McArthur, M. A., Brooke, B. P., Przeslawski, R., Ryan, D. A., Lucieer, V. L., Nichol, S., et al. (2010). On the use of abiotic surrogates to describe marine benthic biodiversity. Estuar. Coast. Shelf Sci. 88, 21–32. doi: 10.1016/j.ecss.2010.03.003
McClain, C. R., Allen, A. P., Tittensor, D. P., and Rex, M. A. (2012a). Energetics of life on the deep seafloor. Proc. Natl. Acad. Sci. U.S.A. 109, 15366–15371. doi: 10.1073/pnas.1208976109
McClain, C. R., and Hardy, S. M. (2010). The dynamics of biogeographic ranges in the deep sea. Proc. R. Soc. B. 277, 3533–3546. doi: 10.1098/rspb.2010.1057
McClain, C. R., Nekola, J. C., Kuhnz, L., and Barry, J. P. (2011). Local-scale faunal turnover on the deep Pacific seafloor. Mar. Ecol. Prog. Ser. 422, 193–200. doi: 10.3354/meps08924
McClain, C. R., Stegen, J. C., and Hurlbert, A. H. (2012b). Dispersal, niche dynamics, and oceanic patterns in beta-diversity in deep-sea bivalves. Proc. R. Soc. B Biol. Sci. 279, 1933–2002. doi: 10.1098/rspb.2011.2166
Menegotto, A., and Rangel, T. F. (2018). Mapping knowledge gaps in marine diversity reveals a latitudinal gradient of missing species richness. Nat. Commun. 9:4713. doi: 10.1038/s41467-018-07217-7
Menzies, R. J., George, R., and Rowe, G. T. (1973). Abyssal Environment and Ecology of the World Oceans. New York, NY: John Wiley and Sons.
Merrie, A., Dunn, D. C., Metian, M., Boustany, A. M., Takei, Y., Oude, A., et al. (2014). An ocean of surprises – trends in human use, unexpected dynamics and governance challenges in areas beyond national jurisdiction. Glob. Environ. Chang. 27, 19–31. doi: 10.1016/j.gloenvcha.2014.04.012
Miloslavich, P., Bax, N., Simmons, S. E., Klein, E., Appeltans, W., Aburto-Oropeza, O., et al. (2018a). Essential Ocean Variables for sustained observations of marine biodiversity and ecosystems. Glob. Change Biol. 24, 2416–2433. doi: 10.1111/gcb.14108
Miloslavich, P., Seeyave, S., Muller-Karger, F., Bax, N., Ali, E., Delgado, C., et al. (2018b). Challenges for global ocean observation: the need for increased human capacity. J. Oper. Oceanogr. 12, S137–S156. doi: 10.1080/1755876X.2018.1526463
Mokievsky, V., and Azovsky, A. (2002). Re-evaluation of species diversity patterns of free-living marine nematodes. Mar. Ecol. Prog. Ser. 238, 101–108. doi: 10.3354/meps238101
Mora, C., Tittensor, D. P., Adl, S., Simpson, A. G. B., and Worm, B. (2011). How many species are there on Earth and in the Ocean? PLoS Biol. 9:e1001127. doi: 10.1371/journal.pbio.1001127
Morato, T., Gonzaìlez-Irusta, J. M., Dominguez-Carrioì, C., Wei, C.-L., Davies, A. A., Sweetman, A. K., et al. (2020). Climate-induced changes in the habitat suitability of cold-water corals and commercially important deep-sea fish in the North Atlantic. Glob. Chang. Biol. 26, 2181–2202. doi: 10.1111/gcb.14996
Morrison, R. J., Zhang, J., Urban, E. R., Hall, J., Ittekkot, V., Avril, B., et al. (2013). Developing human capital for successful implementation of international marine scientific research projects. Mar. Pollut. Bull. 77, 11–22. doi: 10.1016/j.marpolbul.2013.09.001
Muller, E. B., and Nisbet, R. M. (2014). Dynamic energy budget modeling reveals the potential of future growth and calcification for the coccolithophore Emiliania huxleyi in an acidified ocean. Glob. Change Biol. 20, 2031–2038. doi: 10.1111/gcb.12547
Muthumbi, A., Vanreusel, A., and Vincx, M. (2011). Taxon-related diversity patterns from the continental shelf to the slope: a case study on nematodes from the Western Indian Ocean. Mar. Ecol. 32, 453–467. doi: 10.1111/j.1439-0485.2011.00449.x
Muthumbi, A. W., Vanreusel, A., Duineveld, G., Soetaert, K., and Vincx, M. (2004). Nematode community structure along the continental slope off the Kenyan Coast. West. Indian Ocean. Int. Rev. Hydrobiol. 89, 188–205. doi: 10.1002/iroh.200310689
Orkney, A., Platt, T., Narayanaswamy, B. E., Kostakis, I., and Bouman, H. A. (2020). Bio-optical evidence for increasing Phaeocystis dominance in the Barents Sea. Philos. Trans. A Math. Phys. Eng. Sci. 378:20190357.
Parkinson, C. L. (2019). A 40-y record reveals gradual Antarctic sea ice increases followed by decreases at rates far exceeding the rates seen in the Arctic. Proc. Natl. Acad. Sci. U.S.A. 116, 14414–14423. doi: 10.1073/pnas.1906556116
Pearlman, J., Bushnell, M., Coppola, L., Karstensen, J., Buttigieg, P. L., and Pearlman, F. (2019). Evolving and sustaining ocean best practices and standards for the next decade. Front. Mar. Sci. 6:277. doi: 10.3389/fmars.2019.00277
Pham, C. K., Ramirez-Llodra, E., Alt, C. H., Amaro, T., Bergmann, M., Canals, M., et al. (2014). Marine litter distribution and density in European seas, from the shelves to deep basins. PLoS One 9:e95839. doi: 10.1371/journal.pone.0095839
Phillips, B. T., Licht, S., Haiat, K. S., Bonney, J., Allder, J., Chaloux, N., et al. (2019). DEEPi: a miniaturized, robust, and economical camera and computer system for deep-sea exploration. Deep Sea Res. Part I 153, 103136. doi: 10.1016/j.dsr.2019.103136
Pianka, E. R. (1966). Latitudinal gradients in species diversity: a review of concepts. Am. Nat. 100, 33–46. doi: 10.1086/282398
Piechaud, N., Hunt, C., Culverhouse, P. F., Foster, N. L., and Howell, K. L. (2019). Automated identification of benthic epifauna with computer vision. Mar. Ecol. Prog. Ser. 615, 15–30. doi: 10.3354/meps12925
Proud, R., Cox, M. J., Le Guen, C., and Brierley, A. S. (2018). Fine-scale depth structure of pelagic communities throughout the global ocean based on acoustic sound scattering layers. Mar. Ecol. Prog. Ser. 598, 35–48. doi: 10.3354/meps12612
Puig, P., Canals, M., Company, J. B., Martin, J., Amblas, D., Lastras, G., et al. (2012). Ploughing the deep sea floor. Nature 489, 286–289. doi: 10.1038/nature11410
Quattrini, A. M., Gómez, C. E., and Cordes, E. E. (2017). Environmental filtering and neutral processes shape octocoral community assembly in the deep sea. Oecologia 183, 221–236. doi: 10.1007/s00442-016-3765-4
Rabone, M., Harden-Davies, H., Collins, J. E., Zajderman, S., Appeltans, W., Droege, G., et al. (2019). Access to marine genetic resources (MGR): raising awareness of best-practice through a new agreement for biodiversity beyond national jurisdiction (BBNJ). Front. Mar. Sci. 6:520. doi: 10.3389/fmars.2019.00520
Ramalho, S. P., Almeida, M., Esquete, P., Geìnio, L., Ravara, A., Rodrigues, C., et al. (2018). Bottom-trawling fisheries influence on standing stocks, composition, diversity and trophic redundancy of macrofaunal assemblages from the West Iberian Margin. Deep Sea Res. Part I 138, 131–145. doi: 10.1016/j.dsr.2018.06.004
Ramirez-Llodra, E., Brandt, A., Danovaro, R., De Mol, B., Escobar, E., German, C. R., et al. (2010). Deep, diverse and definitely different: unique attributes of the world’s largest ecosystem. Biogeosciences 7, 2851–2899. doi: 10.5194/bg-7-2851-2010
Ramirez-Llodra, E., Shank, T. M., and German, C. R. (2007). Biodiversity and biogeography of hydrothermal vent species thirty years of discovery and investigations. Oceanography 20, 30–41. doi: 10.5670/oceanog.2007.78
Ramirez-Llodra, E., Tyler, P. A., Baker, M. C., Bergstad, O. A., Clark, M. R., Escobar, E., et al. (2011). Man and the last great wilderness: human impact on the deep sea. PLoS One 6:e22588. doi: 10.1371/journal.pone.0022588
Rex, M., Etter, R., and Stuart, C. (1997). “Large scale patterns of species diversity in the deep-sea benthos,” in Marine Biodiversity: Patterns and Processes, eds R. F. G. Ormond, J. D. Gage, and M. V. Angel (Cambridge: Cambridge University Press), 94–121.
Rex, M. A., and Etter, R. J. (2010). Deep-Sea Biodiversity: Pattern and Scale. Harvard: Harvard University Press.
Rex, M. A., Stuart, C. T., and Coyne, G. (2000). Latitudinal gradients of species richness in the deep-sea benthos of the North Atlantic. Proc. Natl. Acad. Sci. U.S.A. 97, 4082–4085. doi: 10.1073/pnas.050589497
Rex, M. A., Stuart, C. T., Hessler, R. R., Allen, J. A., Sanders, H. L., and Wilson, G. D. F. (1993). Global-scale latitudinal patterns of species diversity in the deep sea benthos. Nature 365, 636–638. doi: 10.1038/365636a0
Riehl, T., Wölfl, A. C., Augustin, N., Devey, C. W., and Brandt, A. (2020). Discovery of widely available abyssal rock patches reveals overlooked habitat type and prompts rethinking deep-sea biodiversity. Proc. Natl. Acad. Sci. U.S.A. 117, 15450–15459. doi: 10.1073/pnas.1920706117
Rodrigues, C. F., Hilario, A., and Cunha, M. R. (2013). Chemosymbiotic species from the Gulf of Cadiz (NE Atlantic): distribution, life styles and nutritional patterns. Biogeosciences 10, 2569–2581. doi: 10.5194/bg-10-2569-2013
Rogers, A. D., Aburto-Oropeza, O., Appeltans, W., Assis, J., Balance, L. T., Cury, P., et al. (2020a). Blue Paper 10: Critical Habitats and Biodiversity: Inventory, Thresholds, and Governance. Report prepared for the (Norwegian) Prime Minister’s High Level Panel on a Sustainable Ocean Economy. Washington DC: World Resources Institute.
Rogers, A. D. (1994). The biology of seamounts. Adv. Mar. Biol. 30, 305–350. doi: 10.1016/S0065-2881(08)60065-6
Rogers, A. D. (2000). The role of the oceanic oxygen minima in generating biodiversity in the deep sea. Deep Sea Res. Pt II 47, 119–148. doi: 10.1016/S0967-0645(99)00107-1
Rogers, A. D., Brierley, A., Croot, P., Cunha, M. R., Danovaro, R., Devey, C., et al. (2015). “Delving deeper: critical challenges for 21st century deep-sea research,” in Position Paper 22 of the European Marine Board, eds K. E. Larkin, K. Donaldson, and N. McDonough (Ostend: European Marine Board), 224.
Rogers, A. D., Frinault, B. A. V., Barnes, D. K. A., Bindoff, N. L., Downie, R., Ducklow, H. W., et al. (2020b). Antarctic futures: an assessment of climate-driven changes in ecosystem structure, function, and service provisioning in the Southern Ocean. Ann. Rev. Mar. Sci. 12, 7.1–7.34. doi: 10.1146/annurev-marine-010419-011028
Rogers, A. D., and Gianni, M. (2010). The Implementation of UNGA Resolutions 61/105 and 64/72 in the Management of Deep-Sea Fisheries on the High Seas. Washington, DC: DSCC, 97.
Rogers, A. D., Tyler, P. A., Connelly, D. P., Copley, J. T., James, R., Larter, R. D., et al. (2012). The discovery of new Deep-Sea Hydrothermal Vent communities in the Southern Ocean and implications for biogeography. PLoS Biol. 10:e1001234. doi: 10.1371/journal.pbio.1001234
Rohde, K. (1992). Latitudinal gradients in species-diversity—the search for the primary cause. Oikos 65, 514–527. doi: 10.2307/3545569
Rosa, R., Dierssen, H. M., Gonzalez, L., and Seibel, B. A. (2008). Large-scale diversity patterns of cephalopods in the Atlantic open ocean and deep-sea. Ecology 89, 3449–3461. doi: 10.1890/08-0638.1
Ross, R. E., and Howell, K. L. (2013). Use of predictive habitat modelling to assess the distribution and extent of the current protection of listed’ deep-sea habitats. Divers Distrib. 19, 433–445. doi: 10.1111/ddi.12010
Rowe, G. T., and Menzies, R. J. (1969). Zonation of large benthic invertebrates in the deep-sea off the Carolinas. Deep Sea Res. Part I 16, 531–537. doi: 10.1016/0011-7471(69)90041-2
Ruwa, R., Ajawin, A., Green, S., Kamara, O. K., Rice, J., Inniss, L., et al. (2016). Capacity-Building Needs in Relation to the Status of Species and Habitats. Chapter 53. The First Global Integrated Marine Assessment: World Ocean Assessment I (New York, NY: United Nations), 1–16.
Saeedi, H., Costello, M. J., Warren, D., and Brandt, A. (2019). Latitudinal and bathymetrical species richness patterns in the NW Pacific and adjacent Arctic Ocean. Sci. Rep. 9, 1–10. doi: 10.1038/s41598-019-45813-9
Smith, C. R., De Leo, F. C., Bernardino, A. F., Sweetman, A. K., and Arbizu, P. M. (2008). Abyssal food limitation, ecosystem structure and climate change. Trends Ecol. Evol. 23, 518–528. doi: 10.1016/j.tree.2008.05.002
Snelgrove, P. V., and Smith, C. R. (2002). A riot of species in an environmental calm: the paradox of the species-rich deep-sea floor. Oceanogr. Mar. Biol. Annu. Rev. 40, 311–342. doi: 10.1201/9780203180594.ch6
Somero, G. N., Siebenaller, J. F., and Hochachka, P. W. (1983). “Biochemical and physiological adaptations of deep-sea animals,” in The Sea, Vol. 8, Deep-Sea Biology, ed. G. T. Rowe (New York, NY: Wiley).
Spalding, M. D., Agostini, V. N., Rice, J., and Grant, S. M. (2012). Pelagic provinces of the world: a biogeographic classification of the world’s surface pelagic waters. Ocean Coas. Manag. 60, 19–30. doi: 10.1016/j.ocecoaman.2011.12.016
Stefanoudis, P., Talma, S., Samimi-Namin, K., and Woodall, L. (2020). Deep reef ecosystems of the Western Indian Ocean: addressing the great unknown. Res. Ideas Outcomes 6:e53913. doi: 10.3897/rio.6.e53913
Stramma, L., Johnson, G. C., Sprintall, J., and Mohrholz, V. (2008). Expanding oxygen-minimum zones in the tropical oceans. Science 320, 655–658. doi: 10.1126/science.1153847
Sutton, T. (2013). Vertical ecology of the pelagic ocean: classical patterns and new perspectives. J. Fish Biol. 83, 1508–1527. doi: 10.1111/jfb.12263
Sutton, T. T., Clark, M. R., Dunn, D., Halpin, P. N., Rogers, A. D., Guinotte, J., et al. (2017). A global biogeographic classification of the mesopelagic zone. Deep Sea Res. Part I Oceanogr. Res. Papers 126, 85–102. doi: 10.1016/j.dsr.2017.05.006
Sweetman, A. K., Thurber, A. R., Smith, C. R., Levin, L. A., Mora, C., Wei, C.-L., et al. (2017). Major impacts of climate change on deep-sea benthic ecosystems. Elem. Sci. Anth. 5:4. doi: 10.1525/elementa.203
Taylor, M. L., Gwinnett, C., Robinson, L. F., and Woodall, L. C. (2016). Plastic microfibre ingestion by deep-sea organisms. Sci. Rep. 6:33997. doi: 10.1038/srep33997
Taylor, M. L., and Roterman, C. N. (2017). Invertebrate population genetics across Earth’s largest habitat: the deep-sea floor. Mol. Ecol. 26, 4872–4896. doi: 10.1111/mec.14237
Thistle, D. (1979). “Harpacticoid copepods and biogenic structures: implications for deep-sea diversity maintenance,” in Ecological Processes in Coastal and Marine Systems. Marine Science, Vol. 10, ed. R. J. Livingston (Boston, MA: Springer). doi: 10.1007/978-1-4615-9146-7_11
Thresher, R. E., Tilbrook, B., Fallon, S., Wilson, N. C., and Adkins, J. (2011). Effects of chronic low carbonate saturation levels on the distribution, growth and skeletal chemistry of deep-sea corals and other seamount megabenthos. Mar. Ecol. Prog. Ser. 442, 87–99. doi: 10.3354/meps09400
Thurber, A. R., Sweetman, A. K., Narayanaswamy, B. E., Jones, D. O. B., Ingels, J., and Hansman, R. L. (2014). Ecosystem function and services provided by the deep sea. Biogeosciences 11, 3941–3963. doi: 10.5194/bg-11-3941-2014
Tittensor, D. P., Rex, M. A., Stuart, C. T., McClain, C. R., and Smith, C. R. (2011). Species–energy relationships in deep-sea molluscs. Biol. Lett. 7, 718–722. doi: 10.1098/rsbl.2010.1174
Trueman, C. N., Johnston, G., O’hea, B., and Mackenzie, K. M. (2014). Trophic interactions of fish communities at midwater depths enhance long-term carbon storage and benthic production on continental slopes. Proc. R. Soc. B Biol. Sci. 281:20140669. doi: 10.1098/rspb.2014.0669
UNESCO (2009). Global Open Oceans and Deep Seabed (GOODS)—Biogeographic Classification. Paris: UNESCO.
United Nations (2018). Revised Roadmap for the UN Decade of Ocean Science for Sustainable Development. Available online at: http://www.fao.org/3/CA0463EN/ca0463en.pdf
Van Dover, C. L. (2019). Inactive sulfide ecosystems in the deep sea: a review. Front. Mar. Sci. 6:2305. doi: 10.3389/fmars.2019.00461
Van Dover, C. L., Arnaud-Haond, S., Gianni, M., Helmreich, S., Huber, J. A., Jaeckel, A. L., et al. (2018). Scientific rationale and international obligations for protection of active hydrothermal vent ecosystems from deep-sea mining. Mar. Pol. 90, 20–28. doi: 10.1016/j.marpol.2018.01.020
Van Dover, C. L., Aronson, J., Pendleton, L., Smith, S., Arnaud-Haond, S., Moreno-Mateos, M., et al. (2013). Ecological restoration in the deep sea: Desiderata. Mar. Policy 44, 98–106.
Vanreusel, A., Fonseca, G., Danovaro, R., Da Silva, M. C., Esteves, A. M., Ferrero, T., et al. (2010). The contribution of deep-sea macrohabitat heterogeneity to global nematode diversity. Mar. Ecol. 31, 6–20. doi: 10.1111/j.1439-0485.2009.00352.x
Vernet, M., Geibert, W., Hoppema, M., Brown, P. J., Haas, C., Hellmer, H. H., et al. (2019). The Weddell Gyre, Southern Ocean: present knowledge and future challenges. Rev. Geophys. 57, 623–708. doi: 10.1029/2018RG000604
Vieira, R. P., Bett, B. J., Jones, D. O., Durden, J. M., Morris, K. J., Cunha, M. R., et al. (2020). Deep-sea sponge aggregations (Pheronema carpenteri) in the Porcupine Seabight (NE Atlantic) potentially degraded by demersal fishing. Prog. Oceanogr. 183:102189. doi: 10.1016/j.pocean.2019.102189
Vieira, R. P., Trueman, C. N., Readdy, L., Kenny, A., and Pinnegar, J. K. (2019). Deep-water fisheries along the British Isles continental slopes: status, ecosystem effects and future perspectives. J. Fish Biol. 94, 981–992. doi: 10.1111/jfb.13927
Vinogradova, N. G. (1979). The geographical distribution of the abyssal and hadal (ultra-abyssal) fauna in relation to the vertical zonation of the ocean. Sarsia 64, 41–49. doi: 10.1080/00364827.1979.10411361
Vrijenhoek, R. C., Schutz, S. J., Gustafson, R. G., and Lutz, R. A. (1994). Cryptic species of deep-sea clams (Mollusca: Bivalvia: Vesicomyidae) from hydrothermal vent and cold-water seep environments. Deep Sea Res. Part I 41, 1171–1189. doi: 10.1016/0967-0637(94)90039-90036
Watanuki, Y., and Thiebot, J. B. (2018). Factors affecting the importance of myctophids in the diet of the world’s seabirds. Mar. Biol. 165:79. doi: 10.1007/s00227-018-3334-y
Watling, L., Guinotte, J., Clark, M. R., and Smith, C. R. (2013). A proposed biogeography of the deep ocean floor. Prog. Oceanogr. 111, 91–112. doi: 10.1016/j.pocean.2012.11.003
Watson, R. A., and Morato, T. (2013). Fishing down the deep: accounting for within-species changes in depth of fishing. Fish. Res. 140, 63–65. doi: 10.1016/j.fishres.2012.12.004
Webb, T. J., Vanden Berghe, E., and O’Dor, R. K. (2010). Biodiversity’s big wet secret: The global distribution of marine biological records reveals chronic under-exploration of the deep pelagic ocean. PLoS One 5:e10223. doi: 10.1371/journal.pone.0010223
Wishner, K. F., Seibel, B., and Outram, D. (2020). Ocean deoxygenation and copepods: coping with oxygen minimum zone variability. Biogeosciences 17, 2315–2339. doi: 10.5194/bg-17-2315-2020
Woodall, L., Andradi-brown, D., Brierley, A., Clark, M., Connelly, D., Hall, R., et al. (2018). A multidisciplinary approach for generating globally consistent data on mesophotic, deep-pelagic, and bathyal biological communities. Oceanography 31, 76–89. doi: 10.5670/oceanog.2018.301
Woodall, L. C., Robinson, L. F., Rogers, A. D., Narayanaswamy, B. E., and Paterson, G. L. J. (2015). Deep-sea litter: a comparison of seamounts, banks and a ridge in the Atlantic and Indian Oceans reveals both environmental and anthropogenic factors impact accumulation and composition. Front. Mar. Sci. 2:3. doi: 10.3389/fmars.2015.00003
Woodall, L. C., Sanchez-Vidal, A., Canals, M., Paterson, G. L. J., Coppock, R., Sleight, V., et al. (2014). The deep sea is a major sink for microplastic debris. R. Soc. Open Sci. 1:140317. doi: 10.1098/rsos.140317
Woolley, S. N. C., Tittensor, D. P., Dunstan, P. K., Guillera-Arroita, G., Lahoz-Monfort, J. J., Wintle, B. A., et al. (2016). Deep-sea diversity patterns are shaped by energy availability. Nature 533, 393–396. doi: 10.1038/nature17937
Worm, B., and Tittensor, D. P. (2018). A Theory of Global Biodiversity. Monographs in Population Biology 60. Princeton, NJ: Princeton University Press, 214.
Wright, G., Rochette, J., Gjerde, K. M., and Seeger, I. (2018). The Long and Winding Road: Negotiating a Treaty for the Conservation and Sustainable Use of Marine Biodiversity in Areas Beyond National Jurisdiction. Paris: Institut du développement durable et des relations internationales, 82.
Zezina, O. N. (1973). “Biogeographical division of the benthic area of the ocean by brachiopods,” in Proceedings of the All-Union Research Institute of Marine Fisheries and Oceanography (Trudy VNIRO), Vol. 84, (Jerusalem: Israel Program for Scientifiic Translations), 166–180.
Keywords: deep sea, blue economy, Ocean Decade, Biodivercity, essential ocean variables
Citation: Howell KL, Hilário A, Allcock AL, Bailey DM, Baker M, Clark MR, Colaço A, Copley J, Cordes EE, Danovaro R, Dissanayake A, Escobar E, Esquete P, Gallagher AJ, Gates AR, Gaudron SM, German CR, Gjerde KM, Higgs ND, Le Bris N, Levin LA, Manea E, McClain C, Menot L, Mestre NC, Metaxas A, Milligan RJ, Muthumbi AWN, Narayanaswamy BE, Ramalho SP, Ramirez-Llodra E, Robson LM, Rogers AD, Sellanes J, Sigwart JD, Sink K, Snelgrove PVR, Stefanoudis PV, Sumida PY, Taylor ML, Thurber AR, Vieira RP, Watanabe HK, Woodall LC and Xavier JR (2020) A Blueprint for an Inclusive, Global Deep-Sea Ocean Decade Field Program. Front. Mar. Sci. 7:584861. doi: 10.3389/fmars.2020.584861
Received: 18 July 2020; Accepted: 28 October 2020;
Published: 25 November 2020.
Edited by:
Rowan Trebilco, Centre for Marine Socioecology, AustraliaReviewed by:
Rachel Przeslawski, Geoscience Australia, AustraliaCopyright © 2020 Howell, Hilário, Allcock, Bailey, Baker, Clark, Colaço, Copley, Cordes, Danovaro, Dissanayake, Escobar, Esquete, Gallagher, Gates, Gaudron, German, Gjerde, Higgs, Le Bris, Levin, Manea, McClain, Menot, Mestre, Metaxas, Milligan, Muthumbi, Narayanaswamy, Ramalho, Ramirez-Llodra, Robson, Rogers, Sellanes, Sigwart, Sink, Snelgrove, Stefanoudis, Sumida, Taylor, Thurber, Vieira, Watanabe, Woodall and Xavier. This is an open-access article distributed under the terms of the Creative Commons Attribution License (CC BY). The use, distribution or reproduction in other forums is permitted, provided the original author(s) and the copyright owner(s) are credited and that the original publication in this journal is cited, in accordance with accepted academic practice. No use, distribution or reproduction is permitted which does not comply with these terms.
*Correspondence: Kerry L. Howell, a2VycnkuaG93ZWxsQHBseW1vdXRoLmFjLnVr
Disclaimer: All claims expressed in this article are solely those of the authors and do not necessarily represent those of their affiliated organizations, or those of the publisher, the editors and the reviewers. Any product that may be evaluated in this article or claim that may be made by its manufacturer is not guaranteed or endorsed by the publisher.
Research integrity at Frontiers
Learn more about the work of our research integrity team to safeguard the quality of each article we publish.