- 1Department of Life Science, National Taiwan Normal University, Taipei, Taiwan
- 2Biodiversity Program, Taiwan International Graduate Program, Academia Sinica and National Taiwan Normal University, Taipei, Taiwan
- 3Biodiversity Research Center, Academia Sinica, Taipei, Taiwan
- 4Institute of Marine Environment and Resources, Vietnam Academy of Science and Technology, Haiphong, Vietnam
- 5Graduate University of Sciences and Technology, Vietnam Academy of Science and Technology, Hanoi, Vietnam
Herbivores are an important functional group that control algae, create new space, and promote recruitment for coral recovery. However, on many coral reefs, overfishing has greatly decreased the density of herbivores, especially fishes and gastropods, impairing coral resilience. On such overfished reefs, remnant herbivores that are not target species of local fisheries, e.g., sea urchins, are expected to play an increasingly important role, yet few studies, except for those in the Caribbean and Kenya have examined non-fish herbivores in relation to coral resilience. Here, we conducted field surveys at 30 sites along three coral reefs in Taiwan between 2016 and 2017, to examine the relative importance of six key factors for coral resilience: herbivore abundance (fishes, gastropods, sea urchins), coral cover, macroalgal cover, habitat complexity, water depth, and wave exposure. The density of juvenile coral was used as a proxy of coral resilience. Diadematid sea urchins (Echinothrix spp. and Diadema spp.) dominated most sites (19 of 30 sites) and multivariable regression models showed sea urchin density as the best positive predictor of coral juvenile density. The results elucidated the increasing role diadematid sea urchins play as remnant herbivores on overfished coral reefs in Taiwan. Given that overfishing is a widespread issue, this phenomenon may be occurring globally. More studies are needed to examine the role of remnant, but often ignored, sea urchin herbivory on coral resilience. Reef managers should consider monitoring locally remnant herbivores and incorporating them into management strategies.
Introduction
Coral reefs are in global decline due to chronic anthropogenic disturbances (Pandolfi et al., 2003; Bellwood et al., 2004; Hoegh-Guldberg et al., 2007; Hughes et al., 2018). To reverse these trends, resilience-based management has been proposed to enhance the recovery potential of coral reefs (Bellwood et al., 2004; Hughes et al., 2017). Resilience-based management requires the control of current anthropogenic stressors and to encourage the recovery process (Mcleod et al., 2019). However, information on factors and conditions that promote recovery of coral reef is still scarce (Hughes et al., 2010).
Resilience studies are commonly based on long-term monitoring of recovery process seen in target populations/communities (Graham et al., 2015). This time-consuming process becomes a bottleneck in the advancement of resilience studies, particularly in slower dynamic communities such as coral reefs. The density of juvenile corals has been suggested as a good indicator to assess the resilience of coral populations (hereafter coral resilience) (Gilmour et al., 2013; Graham et al., 2015; Dajka et al., 2019; Nozawa et al., 2020). This is because coral recovery is typically initiated by recruits (Hughes et al., 2007b; Gilmour et al., 2013; Graham et al., 2015), and a positive correlation between juvenile coral density and recovery of coral populations has often been observed (Gilmour et al., 2013; Dajka et al., 2019; Nozawa et al., 2020). This new approach to evaluate coral resilience based on juvenile coral density significantly shortens study time and allows scientists to explore factors influencing coral resilience more efficiently.
Coral resilience has been explained by a hypothesis concerning the quantitative interactions between three key functional groups: herbivores, algae, and corals (Bellwood et al., 2004; Hughes et al., 2007a; Mumby et al., 2007; Graham et al., 2013). In a healthy coral reef ecosystem, herbivores and oligotrophic water control algal abundance, the main competitor of corals for space. However, along many contemporary coral reefs, overfishing and eutrophication weakens this balance, resulting in algal dominance on reef space that hinders coral recovery, via the coral recruitment process (Birrell et al., 2008; Mumby and Steneck, 2008). Therefore, the abundance of herbivores and algae has been considered as the main biotic factor determining coral resilience (Bellwood et al., 2004; Mumby et al., 2006).
Although less attention is paid to it, the abundance of coral itself could also influence coral resilience through stock-recruitment dynamics (Hughes et al., 2019) and competition for space between adults and recruits (Ledlie et al., 2007). Coral abundance determines the amount of locally produced larvae, which contributes to self-recruitment, especially for coral species with larvae of short-distance dispersal, such as Acropora spp. (Nozawa and Harrison, 2008) and Pocillopora spp. (Tioho et al., 2001). Coral abundance also determines the degree of competition for space between adult corals and recruits, thereby influencing coral recruit density (Baird and Hughes, 2000).
Besides the biotic factors, three abiotic factors have been proposed to influence coral resilience via the herbivore-alga-coral interaction: habitat complexity, water depth, and wave exposure (Mumby and Steneck, 2008; McClanahan and Muthiga, 2016). Habitat complexity influences the abundance of herbivorous fish with more complex habitats harboring increased herbivore fish abundance, while water depth covaries with light intensity, directly affecting algal abundance (Ledlie et al., 2007; Graham et al., 2015). Graham et al. (2015) highlighted habitat complexity and water depth as two key factors of coral resilience, among others, over a 17-year coral recovery documented in the Seychelles. Wave exposure is less examined in coral resilience studies, but known to influence the abundance of herbivores (Harborne et al., 2006; Bronstein and Loya, 2014) as well as the abundance and taxonomic composition of corals (Robinson et al., 2019).
To date, only a few comprehensive studies have been conducted to examine the relative importance of key factors proposed for coral resilience (Graham et al., 2015; Dajka et al., 2019; Mcleod et al., 2019). Furthermore, previous studies of coral resilience have mainly been conducted in the Caribbean (Carpenter and Edmunds, 2006; Furman and Heck, 2009; Idjadi et al., 2010), Great Barrier Reef (Darling et al., 2017), and the Seychelles (O’Leary et al., 2013; Graham et al., 2015; Dajka et al., 2019), with few studies from Southeast Asia (Muallil et al., 2020; Nozawa et al., 2020). In the present study, we examined the relative importance of herbivore and five other key factors for coral resilience: algae, corals, habitat complexity, water depth, and wave exposure at a total of 30 sites along three Taiwanese coral reefs, using juvenile coral density as an indicator of coral resilience. We focused on herbivores by covering both herbivorous fishes and benthic herbivores (sea urchins and gastropods).
Materials and Methods
Field surveys were conducted along three coral reefs in southern Taiwan between 2016 and 2017 (Figure 1). At each coral reef, 9–11 sites were haphazardly selected at 4–13 m depth (Figure 1). A total of 30 sites were investigated, including 10 sites at Green Island, 11 sites at Orchid Island, and 9 sites in Kenting. At each site, we collected data within an area approximately equal to 50 m × 50 m.
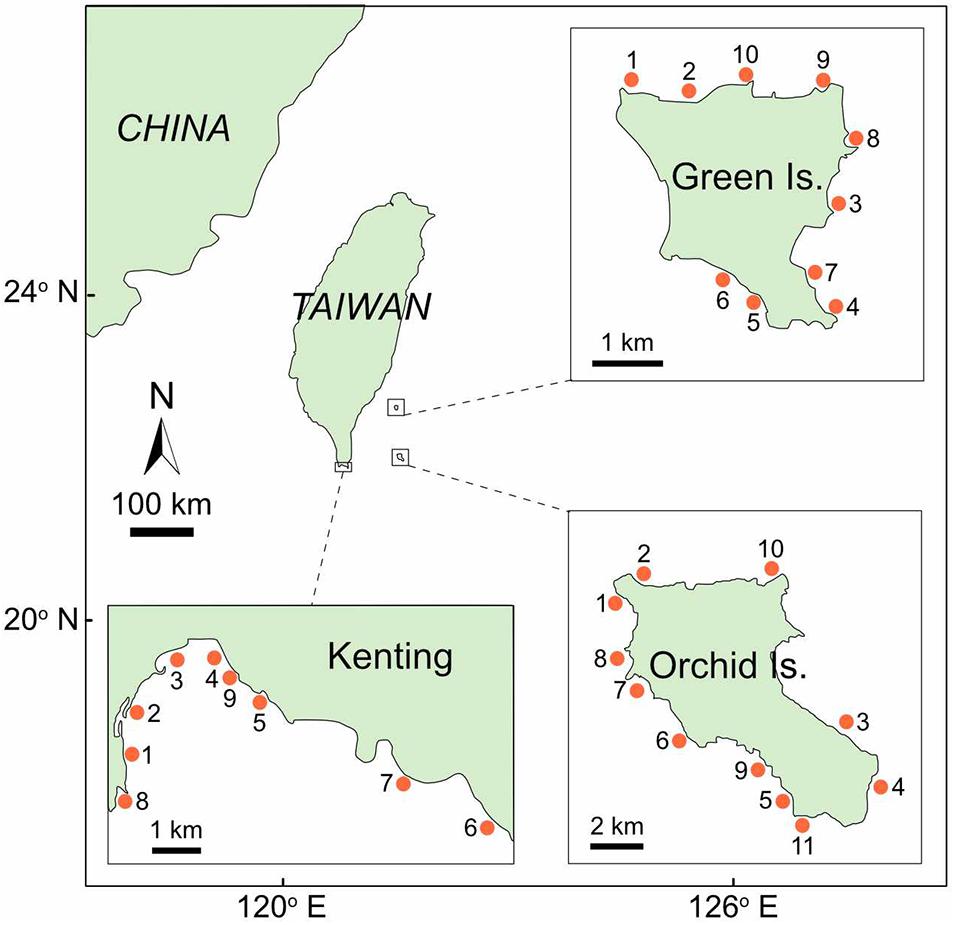
Figure 1. Survey sites (indicated by point) in three coral reef areas in southern Taiwan: Green Island, Orchid Island, and Kenting. The ID number of survey sites at each coral reef corresponds to Supplementary Table S1, where a summary of the results for each site is provided.
In this study, we investigated the density of juvenile corals (<5 cm in diameter) as a proxy for coral resilience and six factors that would influence juvenile coral densities: corals, macroalgae, herbivores, habitat complexity, water depth, and wave exposure. For herbivores, density of herbivorous fish (parrot and surgeonfish), sea urchins, and herbivorous gastropods were recorded. Habitat complexity was examined at two size-scales (decimeter and meter scale). Herbivorous fish, habitat complexity (m-scale), and wave exposure were measured at each site, while the other data were collected along a 10-m line transect (n = 5) at each site (Supplementary Figure S1). Haphazard sampling was frequently used when the spatial distribution of coral communities was unknown and random sampling raised implementational problems. Although haphazard sampling is common in field ecological studies, we acknowledge that statistical tests are not strictly valid and results could be more vulnerable to unmeasurable bias (Lewis, 2004).
Coral and macroalgal cover were estimated using the line intercept method. Five replicates of a 10-m line were placed haphazardly along hard substrate at each site (Supplementary Figure S1). Corals and macroalgae (>2 cm in trunk height) appearing below the line were photographed from above with the line. Close-up photographs were also taken for taxonomic identification to the genus level (Veron and Stafford-Smith, 2000; Wang et al., 2015). The sum of intercept distance of corals or macroalgae on each line transect was measured on photographs to determine coverage (%) as a proportion of tape length.
The density of benthic herbivores (sea urchins and herbivorous gastropods) were estimated within five replicate 10 × 2 m belt transects (Supplementary Figure S1). The number of sea urchins and macro-gastropods (>1 cm) appearing within a 1-m distance from the 10-m line-transect within both the left and right-hand sides were counted by a scuba diver using a 1-m scale as a reference. Observed individuals were photographed with a scale for taxonomic identification. At the study sites, sea urchins and gastropods were hiding in holes and crevices during the daytime survey. Therefore, care was taken to detect individuals by spending time (>10 min per survey area) looking closely into habitats. We focused on free-grazing sea urchins and ignored taxa that exclusively inhabit holes and burrows in the study locations, including Echinostrephus spp. and Echinometra spp.
The density of juvenile corals (<5 cm in diameter) was examined using 50 cm × 50 cm quadrats (n = 5) placed haphazardly on hard substrata along the 10-m line transect (Supplementary Figure S1). Quadrats were placed on relatively vacant space where >50% of the quadrat area was not occupied by sessile macro-benthos (e.g., hard corals, soft corals, macroalgae, sponges, and sea anemones). Juvenile corals in each quadrat were counted and photographed with a scale for taxonomic identification to the genus level (Veron and Stafford-Smith, 2000). The majority of juvenile corals that were larger than ∼1 cm in diameter could be taxonomically identified. The density of juvenile corals (m–2) was calculated by dividing the sum of juveniles by the amount of non-occupied area by the sessile macro-benthos in the five quadrants. The non-occupied area in each quadrat was estimated by using CPCe (Coral Point Count with Excel Extensions, ver. 4.0 software) with 100 random points (Kohler and Gill, 2006).
The abundance of herbivorous fish was estimated using the modified method of stationary point count (Colvocoresses and Acosta, 2007). Parrotfish and surgeonfish were surveyed as regionally dominant herbivorous fish groups (Muallil et al., 2020; Nozawa et al., 2020). Although we assumed both parrotfish and surgeonfish are herbivorous in this study, caution is advised when interpreting results as recent studies have indicated that many taxa in such groups are not entirely herbivores and instead feed mainly on cyanobacteria, microbes, and detritus (Clements et al., 2017; Dell et al., 2020). In the survey, three 360-degree video cameras (PIXPRO SP360, Kodak) were placed haphazardly (∼10 m away from each transect line) within the 50 m × 50 m area at each site. Each video camera was set at the time-lapse mode with an interval of 1 s with an image quality of 1440 × 1440 pixels. Videos were taken for approximately 40 min, while conducting other surveys at each site. Preliminary examination on the resolution of the video camera indicated that images of target fish taxa that are larger than 10 cm in total length could be identified within the radius of 5 m from the 360-degree video camera (ca. 78.5 m2). Therefore, we used these numbers for the abundance estimation. Due to the small survey area of each video camera, we estimated the density of herbivorous fishes at each site using the sum of fishes (>10 cm in total length) recorded by the three video cameras (total survey area of ca. 235 m2).
Habitat complexity was assessed at 2 size-scales: decimeter-scale (relief of <1 m) and meter-scale (relief of >1 m). Habitat complexity at the dm-scale was measured based on the undulation of substrate surface along the 10-m transect line using a water level logger (HOBO Water Level Data Logger; Onset Computer Corporation, Bourne, MA, United States) following Dustan et al. (2013). The water level logger also recorded depth, and average depth was calculated for each line and used in the analysis. The rugosity index was calculated by dividing the measured length of surface undulation by the measured horizontal distance (10 m) (Graham and Nash, 2013). For m-scale complexity, the seascape complexity was determined by visual estimation using four categories defined based on the abundance of large relief (>1-m height) and deep crevices (>1-m deep) at the study site: category 1 = few (abundance < 3); category 2 = several (abundance of 3–10); category 3 = many and moderately complex (abundance of >10); category 4 = very complex (abundance of >10, the max size of relief and crevices is much larger than the category 3).
Wave exposure at each site was estimated by the fetch method (Chollett and Mumby, 2012). The fetch was calculated using the fetchR package in R (R Core Team, 2018) with 36 compass directions (angle intervals of 10°). Data on wind speed and direction (2007–2018) at each site were collected from the online archive, CODiS of Taiwan Central Weather Bureau1. Wave exposure at each site (J m–3) was calculated based on these values using the linear wave theory that includes equations of “fetch-limited” and “fully developed” seas (Ekebom et al., 2003; Harborne et al., 2006; Chollett and Mumby, 2012).
We examined factors that would influence the density of juvenile corals using generalized linear mixed models (GLMMs). A Gamma error distribution with a log link function was used for the response variable (juvenile coral density). In the model, eight predictor variables were considered: herbivorous fish density, sea urchin density, coral cover, macroalgal cover, dm-scale habitat complexity, m-scale habitat complexity, water depth, and wave exposure. Herbivorous gastropods were virtually absent at most sites and therefore ignored in the analysis (see “Results” section). Juvenile sea urchins (<2 cm in test size) are less influential in controlling algae and consequently were excluded from sea urchin density data. In the analysis, predictor variables were standardized by subtracting the mean and dividing by two standard deviations (Gelman, 2008). We first examined an overall model by combining all data from the three coral reef areas and then examining individual models for each coral reef area. Study sites that were nested within coral reef areas were treated as random factors. No multicollinearity was detected in the models at a criterion of variance inflation factors < 2.5. The Akaike information criterion corrected for small size (AICc) was used for model selection. Best models of <2 ΔAICc were selected, and model averaging was performed within them (Burnham and Anderson, 2002; Zuur et al., 2009). In the averaged model, the mean and 95% confidence intervals (CIs) of the estimated coefficients were calculated for each predictor variable. Predictors were inferred as significant when 95% CIs of coefficient excluded zero. The relative importance of each predictor in the averaged model was calculated by summing the Akaike weights for the predictor over the best models (Burnham and Anderson, 2002). This index ranges from 0 to 1 with a higher value for the higher relative importance. All analyses were performed in R ver. 3.6.1 (R Core Team, 2018) with the packages, lme4 ver. 1.1-23 and MuMin ver. 1.43.10.
Results
We described the state of the six biological variables: corals, juvenile corals, herbivorous fishes, sea urchins, herbivorous gastropods, and macroalgae recorded along the three Taiwanese coral reefs. A summary of results at each site for the biotic variables and three abiotic variables (habitat complexity, water depth, wave exposure) is provided in Supplementary Table S1.
Coral cover varied among the three reefs with the highest average cover recorded in Green Island [mean ± SE: 42.9 ± 1.8%], followed by Orchid Island [28.4 ± 1.9%], and Kenting [19.4 ± 1.7%] (Figure 2A). Of the total 50 coral genera identified, those dominant in total cover were Montipora (23.7%), Porites (16.9%), Pocillopora (8.4%), and Acropora (8%). In contrast, the density of juvenile coral was the highest in Orchid Island [mean ± SE: 34 ± 2.6 indiv./m2], followed by Kenting [22.7 ± 2.4 indiv./m2] and Green Island [20.8 ± 1.3 indiv./m2] (Figure 2B). The dominant genera of juvenile corals were similar to those of adult corals, including Porites (26%), Montipora (17%), and Pocillopora (14%).
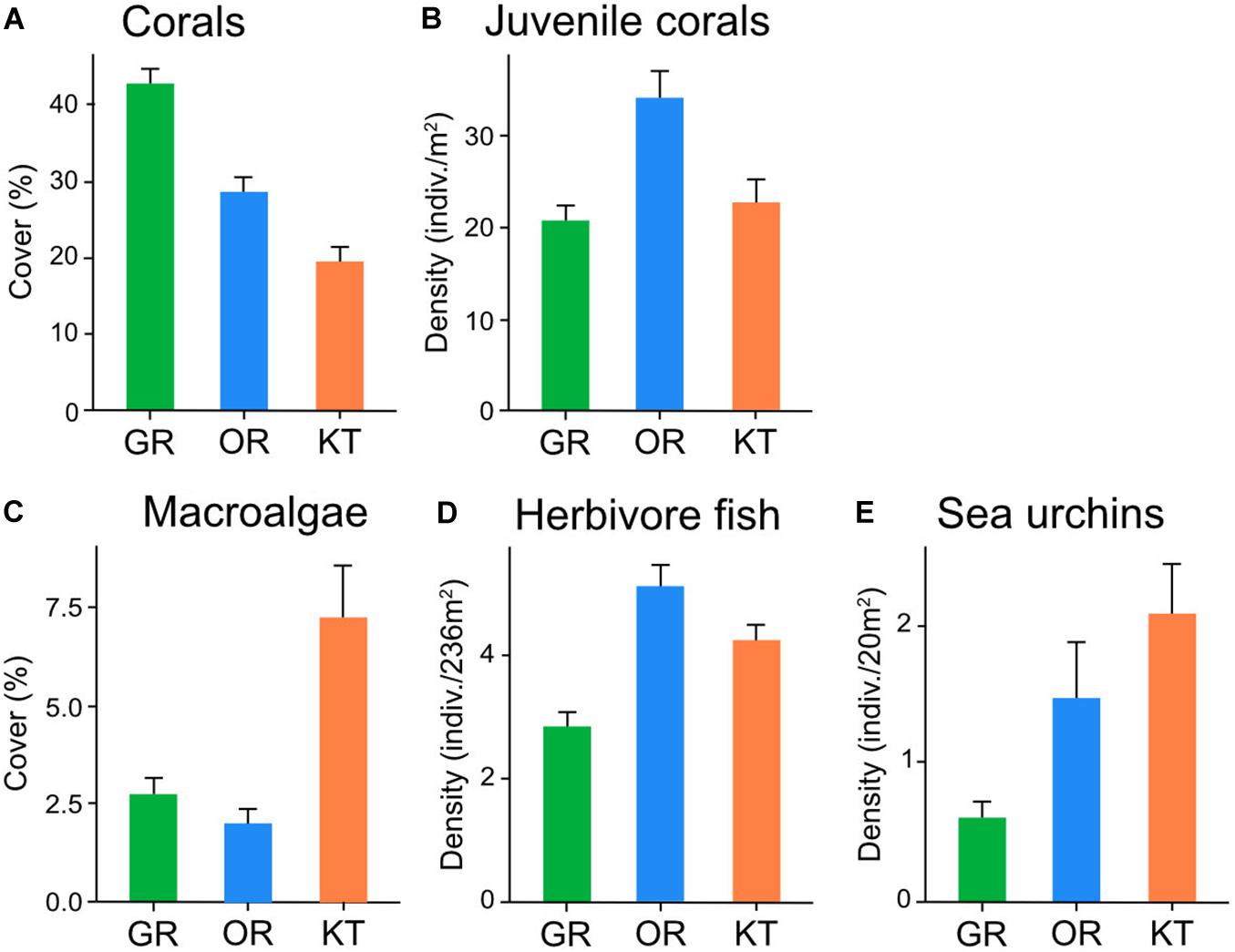
Figure 2. Abundance [mean ± SE] of (A) hard coral, (B) juvenile coral, (C) macroalgae, (D) herbivorous fish, (E) sea urchins (mostly diadematids) on three Taiwanese coral reefs in 2016–2017. The densities of juvenile coral, sea urchins, and herbivorous fish have different scales.
Macroalgal cover was relatively low at all sites, ranging from 0 to 28.1% on average. Kenting had the highest macroalgal cover [mean ± SE: 7.4 ± 1.3%], followed by Green Island [2.8 ± 0.4%], and Orchid Island [2.1 ± 0.3%] (Figure 2C). Of a total of 19 genera identified, dominant genera in total cover were Codium (29.3%), Galaxaura (21.6%), and Laurencia (16.1%).
The overall density of herbivorous fish (parrotfish and surgeonfish) was low [mean ± SE: 4.2 ± 0.2 indiv./235 m2] on these Taiwanese coral reefs. Orchid Island had the highest average density [5.1 ± 0.3 indiv./235 m2], followed by Kenting [4.3 ± 0.3 indiv./235 m2] and Green Island [2.93 ± 0.2 indiv./235 m2] (Figure 2D). Surgeonfish dominated herbivore fish assemblages (91.6%). Although taxonomic identification to genus level was difficult using the method in the present study, Nozawa et al. (2020) reported four surgeonfish genera Acanthurus, Ctenochaetus, Naso, and Zebrasoma within the coral reef areas in 2012–2014. Herbivorous gastropods were virtually absent at all sites, with only nine individuals recorded (five in Green Island, two in Orchid Island, and two in Kenting): These were Turbo chrysostomus (seven individuals) and Monetaria sp. (two individuals). Compared with herbivorous fishes and gastropods, sea urchins were the most abundant herbivores (in density) on 19 of 30 coral reef sites (Figure 2E and Supplementary Table S1). However, their density varied greatly from 0 to 14 indiv./20 m2 among sites. The average density of sea urchins [mean ± SE] was 2 ± 0.4 indiv./20 m2 in Kenting, 1.4 ± 0.4 indiv./20 m2 in Orchid Island, and 0.6 ± 0.2 indiv./20 m2 in Green Island (Figure 2E). Most sea urchin species belonged to the family Diadematidae, consisting of the genera Diadema (66.1%) and Echinothrix (33.9%). Except for diadematids, only three individuals of Tripneustes gratilla were recorded (note that Echinostrephus spp. and Echinometra spp. were not considered in this study).
Sea urchin density was the only significant positive predictor of juvenile coral density in the overall GLMM for the three Taiwanese coral reefs (coefficient = 0.415; 95% CI lower = 0.227, upper = 0.604) (Figures 3A, 4A) and was also the only significant positive predictor in individual GLMMs for each reef (coefficient = 0.339–0.551) (Figures 3B–D, 4B–D). The relative importance of sea urchin density was the maximum value (1.00) in all the GLMMs (Supplementary Table S2). Besides sea urchin density, water depth (coefficient = −0.269; 95% CI lower = −0.501, upper = −0.037) and habitat complexity at m-scale (coefficient = −0.405; 95% CI lower = −0.648, upper = −0.161) were selected as significant negative predictors in the overall GLMM for all reefs (Figure 3A). However, these were not significant predictors in the GLMMs for the individual reefs, except for habitat complexity at m-scale in Orchid Island (Figure 3C). In addition to these predictors, habitat complexity at dm-scale was selected as a significant positive predictor only in the GLMM for Green Island (Figure 3B).
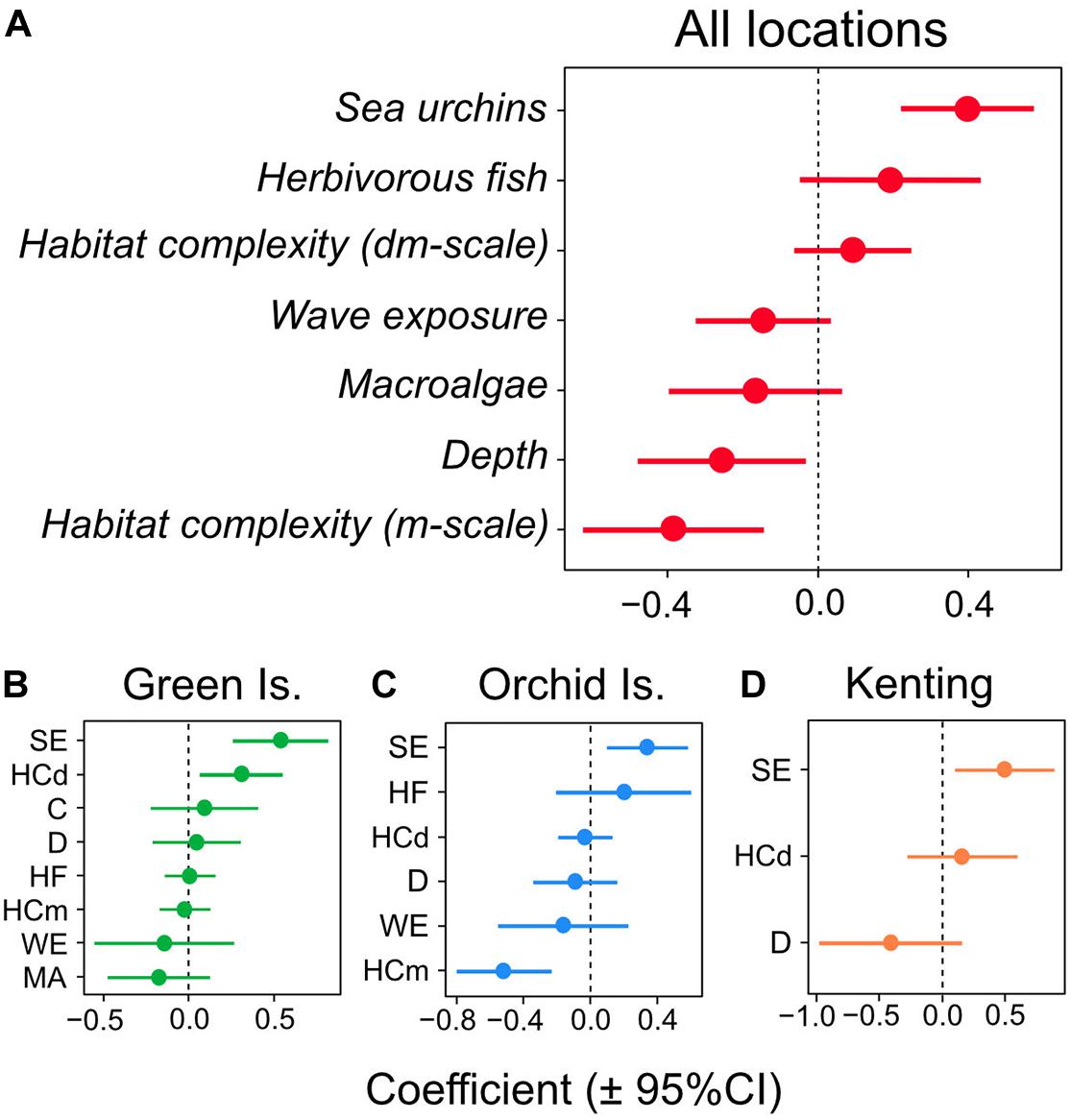
Figure 3. Multi-model-averaged parameter estimates for the predictors of juvenile coral density in the multivariable regression analysis. Mean coefficient estimates (±95% confidence intervals) are plotted for (A) all locations, and for individual coral reefs for (B) Green Island, (C) Orchid Island, and (D) Kenting. Abbreviations of predictors in figures (B–D) denote, SE, sea urchin density; HF, herbivorous fish density; C, coral cover; MA, macroalgal cover; HCdm, habitat complexity at decimeter-scale; HCm, habitat complexity at meter-scale; D, water depth; WE, wave exposure.
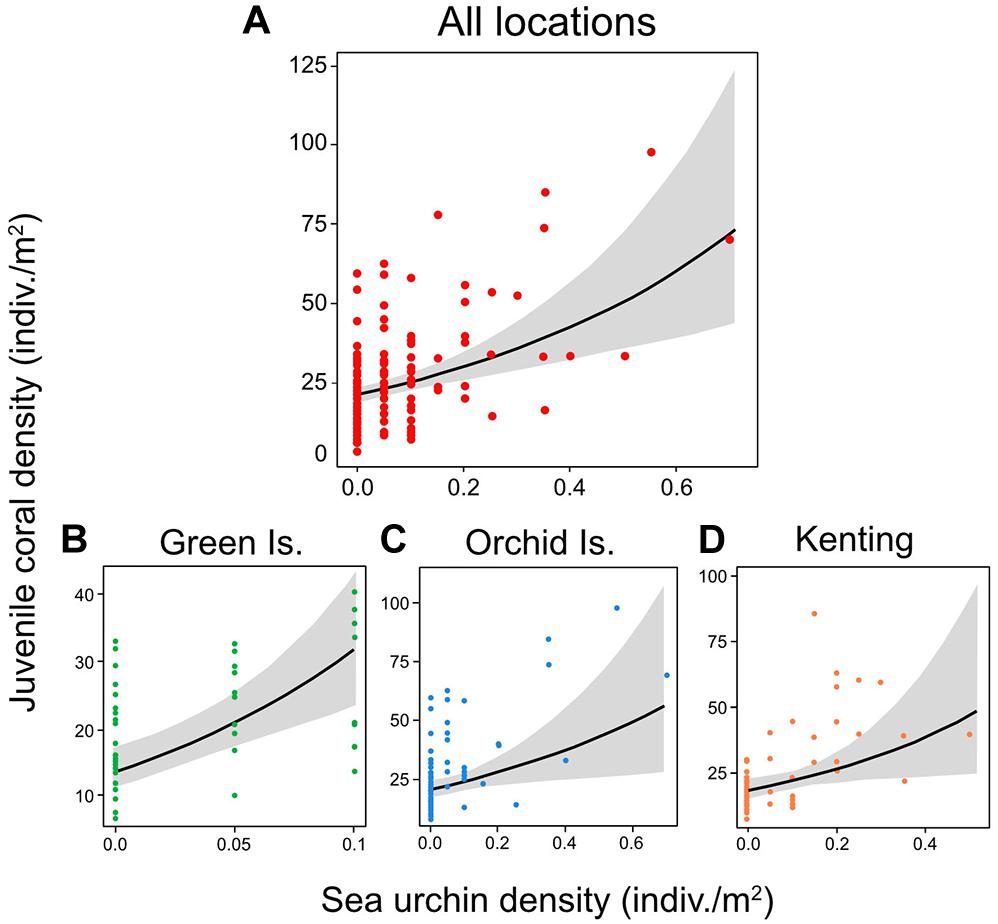
Figure 4. The marginal effect of sea urchin (mostly diadematids) density (±95% confidence intervals) on juvenile coral density, estimated at the mean of other predictors in the GLMM for (A) all locations, and for individual coral reefs for (B) Green Island, (C) Orchid Island, and (D) Kenting.
Discussion
The present study demonstrated that diadematid density was the primary positive driver of juvenile coral density, suggesting the important role of diadematids for coral resilience on the Taiwanese coral reefs. The dominance of diadematids was most likely owing to the population decline of other macro-herbivores, fishes and gastropods that are target species of local fisheries on coral reefs, whereas diadematids are not. In the present study, non-diadematid sea urchins and herbivorous gastropods were rarely seen, and many herbivorous fishes were small in size (personal observation). This reflects the severe overfishing problems occurring along Taiwanese coral reefs (Dai et al., 2002) and elucidates the increasing role of diadematids as remnant herbivores on the overfished coral reefs.
A shift in dominant herbivore taxa from herbivorous fishes to sea urchins has been documented on overfished coral reefs in other regions of the world, including the Caribbean and eastern Africa (McClanahan and Kurtis, 1991; Jackson et al., 2001). Of these, the best example is Diadema antillarum, that became the dominant herbivore on coral reefs in the Caribbean due to historical overfishing of other herbivorous animals (Jackson et al., 2001). However, the sudden regional die-off of D. antillarum by an epidemic in 1983 was followed by the significant decline of coral due to overgrowth by blooms of macroalgae across the Caribbean (Lessios, 1988; Hughes, 1994). This catastrophic event revealed the important role D. antillarum plays as the remaining dominant herbivores pre-1983, facilitating a coral-dominated state by controlling macroalgae on the overfished Caribbean reefs (Lessios, 1988; Hughes et al., 1999; Edmunds and Carpenter, 2001; Carpenter and Edmunds, 2006). Although the recovery of D. antillarum populations has been prolonged and patchy (Lessios, 2016), studies have reported the strong association between their recovery, lower macroalgal abundance, and higher coral recruit abundance (Edmunds and Carpenter, 2001; Carpenter and Edmunds, 2006; Idjadi et al., 2010).
The multivariable regression analyses performed in this study indicated that only a few of the examined factors, that have been proposed as key factors for coral resilience elsewhere were significant drivers of juvenile coral abundance along Taiwanese coral reefs. The results suggest a high variation in primary factors of coral resilience among locations (Roff and Mumby, 2012) and indicates the importance of examining factors at each location. Herbivorous fishes are often dominant, regarded as the key functional group of coral resilience on pristine coral reefs (Gilmour et al., 2013) or in marine reserves (Mumby et al., 2007; Graham et al., 2015). However, on Taiwanese reefs, herbivorous fishes were not abundant, and their function was weakened (i.e., no significant correlation with juvenile coral density). Furthermore, recent studies have indicated that many nominal herbivorous fish taxa such as parrotfish and surgeonfish actually feed on non-algal foods including cyanobacteria, microbes, and detritus and are not main contributors of algal removal (Clements et al., 2017; Dell et al., 2020).
Macroalgae are also the well-known primary negative driver of coral recruits and resilience on Caribbean coral reefs (Hughes, 1994; Edmunds and Carpenter, 2001; Carpenter and Edmunds, 2006). However, outside the region, macroalgae are often not abundant and hence not a major competitor for space, as seen in the present study (Bruno et al., 2009; Roff and Mumby, 2012). Water depth was negatively associated with juvenile coral abundance in the present study, possibly owing to larval settlement patterns of the dominant coral taxa, Porites and Pocillopora spp., with more recruits at shallower sites observed locally (Nozawa et al., 2013). In contrast, it was the positive driver of coral resilience in the Seychelles (Graham et al., 2015).
Habitat complexity is a well-studied habitat characteristic that often indicates positive effects on coral recruits and resilience, owing to its positive association with herbivorous fish abundance (Graham and Nash, 2013; Graham et al., 2015). In our multivariable regression analyses, opposite effects of habitat complexity emerged depending on the habitat scale; the decimeter-scale habitat complexity showed a tendency of a positive effect on juvenile coral abundance, whereas the meter-scale habitat complexity indicated negative effects. This contrasting pattern suggests the importance of scale in habitat complexity (Tokeshi and Arakaki, 2012). It might be partially explained by the possible scale-dependent impact of habitat on grazing activity of the locally dominant herbivore, diadematids. The decimeter-scale habitat complexity could enhance diadematid abundance by providing shelter and hence, increasing gross grazing rate (Lee, 2006), whereas the meter-scale complexity would create a dynamic water environment that often reduces diadematid grazing (Foster, 1987; Rogers and Lorenzen, 2016).
A key strategy, for resilience-based management of coral reefs, is to restore functional herbivory via recovering herbivorous fish populations (Graham et al., 2013; MacNeil et al., 2015; Topor et al., 2019), while the use of benthic herbivores, including sea urchins has rarely been discussed outside the Caribbean. The use of benthic herbivores has two clear advantages over herbivorous fishes, especially for small-sized marine reserves: (1) fewer protection efforts for species like diadematids as they are often not fishery species and (2) reduced management effort because of their limited mobility. Given the time-consuming and challenging process in recovering and maintaining the sufficient density of herbivorous fish populations, including strict fishery regulations and large-sized marine reserves (MacNeil et al., 2015), it would be wise to consider benthic herbivores, ahead of, or alongside herbivorous fishes, as they may be easier to recover and manage than fish (Maciá et al., 2007; Neilson et al., 2018). However, more information is needed to realize the full potential of benthic herbivores in coral reef management. For example, grazing activity of Diadema sea urchins could cause damage to corals and coral recruits under high-density conditions (Sammarco, 1980, 1982; Bronstein and Loya, 2014). Research areas to fully understand the value of benthic herbivores are a priority and may include the selection of local candidate species, their effective population densities, and their effects on the alga-coral interaction.
Data Availability Statement
The raw data supporting the conclusions of this article will be made available by the authors, without undue reservation.
Author Contributions
VD and YN conceived and designed the study, collected and analyzed the data, and drafted the manuscript. P-YC collected and analyzed the data. C-LF, J-HS, and C-HL collected the data. AM collected the data and edited the manuscript. All authors contributed to the article and approved the submitted version.
Funding
The study was supported by the Ministry of Science and Technology, R.O.C (106-2611-M-001-004 and 107-2611-M-001-003) and an internal research grant of Biodiversity Research Center, Academia Sinica to YN.
Conflict of Interest
The authors declare that the research was conducted in the absence of any commercial or financial relationships that could be construed as a potential conflict of interest.
Acknowledgments
We thank H.-S. Hsieh and C.-H. Liu for data analyses and assistance.
Supplementary Material
The Supplementary Material for this article can be found online at: https://www.frontiersin.org/articles/10.3389/fmars.2020.581945/full#supplementary-material
Footnotes
References
Baird, A., and Hughes, T. (2000). Competitive dominance by tabular corals: an experimental analysis of recruitment and survival of understorey assemblages. J. Exp. Mar. Biol. Ecol. 251, 117–132. doi: 10.1016/S0022-0981(00)00209-4
Bellwood, D. R., Hughes, T. P., Folke, C., and Nystrom, M. (2004). Confronting the coral reef crisis. Nature 429, 827–833. doi: 10.1038/nature02691
Birrell, C. L., McCook, L. J., Willis, B. L., and Diaz-Pulido, G. A. (2008). Effects of benthic algae on the replenishment of corals and the implications for the resilience of coral reefs. Oceanogr. Mar. Biol. Annu. Rev. 46, 25–63. doi: 10.1201/9781420065756.ch2
Bronstein, O., and Loya, Y. (2014). Echinoid community structure and rates of herbivory and bioerosion on exposed and sheltered reefs. J. Exp. Mar. Biol. Ecol. 456, 8–17. doi: 10.1016/j.jembe.2014.03.003
Bruno, J. F., Sweatman, H., Precht, W. F., Selig, E. R., and Schutte, V. G. (2009). Assessing evidence of phase shifts from coral to macroalgal dominance on coral reefs. Ecology 90, 1478–1484. doi: 10.1890/08-1781.1
Burnham, K. P., and Anderson, D. R. (2002). Model Selection and Multimodel Inference: A Practical Information-Theoretic Approach, 2nd Edn. New York: Springer Science and Business Media.
Carpenter, R. C., and Edmunds, P. J. (2006). Local and regional scale recovery of Diadema promotes recruitment of scleractinian corals. Ecol. Lett. 9, 271–280. doi: 10.1111/j.1461-0248.2005.00866.x
Chollett, I., and Mumby, P. (2012). Predicting the distribution of Montastraea reefs using wave exposure. Coral Reefs 31, 493–503. doi: 10.1007/s00338-011-0867-7
Clements, K. D., German, D. P., Piché, J., Tribollet, A., and Choat, J. H. (2017). Integrating ecological roles and trophic diversification on coral reefs: multiple lines of evidence identify parrotfishes as microphages. Biol. J. Linn. Soc. 120, 729–751. doi: 10.1111/bij.12914
Colvocoresses, J., and Acosta, A. (2007). A large-scale field comparison of strip transect and stationary point count methods for conducting length-based underwater visual surveys of reef fish populations. Fish. Res. 85, 130–141. doi: 10.1016/j.fishres.2007.01.012
Dai, C., Soong, K., Chen, C. A., Hwang, J., Fan, T., Hsieh, H., et al. (2002). “The status of coral reefs in Taiwan and the conservation problems,” in The Proceedings of the IUCN/WCPA EA4 Taipei Conference, Taipei, 265–276.
Dajka, J.-C., Wilson, S. K., Robinson, J. P. W., Chong-Seng, K. M., Harris, A., and Graham, N. A. J. (2019). Uncovering drivers of juvenile coral density following mass bleaching. Coral Reefs 38, 637–649. doi: 10.1007/s00338-019-01785-w
Darling, E. S., Graham, N. A., Januchowski-Hartley, F. A., Nash, K. L., Pratchett, M. S., and Wilson, S. K. (2017). Relationships between structural complexity, coral traits, and reef fish assemblages. Coral Reefs 36, 561–575. doi: 10.1007/s00338-017-1539-z
Dell, C. L. A., Longo, G. O., Burkepile, D. E., and Manfrino, C. (2020). Few herbivore species consume dominant macroalgae on a Caribbean coral reef. Front. Mar. Sci. 7:676. doi: 10.3389/fmars.2020.00676
Dustan, P., Doherty, O., and Pardede, S. (2013). Digital reef rugosity estimates coral reef habitat complexity. PLoS One 8:e57386. doi: 10.1371/journal.pone.0057386
Edmunds, P. J., and Carpenter, R. C. (2001). Recovery of Diadema antillarum reduces macroalgal cover and increases abundance of juvenile corals on a Caribbean reef. PNAS 98, 5067–5071. doi: 10.1073/pnas.071524598
Ekebom, J., Laihonen, P., and Suominen, T. (2003). A GIS-based step-wise procedure for assessing physical exposure in fragmented archipelagos. Estuar. Coast. Shelf Sci. 57, 887–898. doi: 10.1016/S0272-7714(02)00419-5
Foster, S. A. (1987). The relative impacts of grazing by Caribbean coral reef fishes and Diadema: effects of habitat and surge. J. Exp. Mar. Biol. Ecol. 105, 1–20. doi: 10.1016/S0022-0981(87)80026-6
Furman, B., and Heck, K. L. (2009). Differential impacts of echinoid grazers on coral recruitment. Bull. Mar. Sci. 85, 121–132.
Gelman, A. (2008). Scaling regression inputs by dividing by two standard deviations. Stat. Med. 27, 2865–2873. doi: 10.1002/sim.3107
Gilmour, J. P., Smith, L. D., Heyward, A. J., Baird, A. H., and Pratchett, M. S. (2013). Recovery of an isolated coral reef system following severe disturbance. Science 340, 69–71. doi: 10.1126/science.1232310
Graham, N., and Nash, K. (2013). The importance of structural complexity in coral reef ecosystems. Coral Reefs 32, 315–326. doi: 10.1007/s00338-012-0984-y
Graham, N. A., Bellwood, D. R., Cinner, J. E., Hughes, T. P., Norström, A. V., and Nyström, M. (2013). Managing resilience to reverse phase shifts in coral reefs. Front. Ecol. Environ. 11:541–548. doi: 10.1890/120305
Graham, N. A., Jennings, S., MacNeil, M. A., Mouillot, D., and Wilson, S. K. (2015). Predicting climate-driven regime shifts versus rebound potential in coral reefs. Nature 518, 94–97. doi: 10.1038/nature14140
Harborne, A. R., Mumby, P. J., Zychaluk, K., Hedley, J. D., and Blackwell, P. G. (2006). Modelling the beta diversity of coral reefs. Ecology 87, 2871–2881. doi: 10.1890/0012-9658(2006)87[2871:MTBDOC]2.0.CO;2
Hoegh-Guldberg, O., Mumby, P. J., Hooten, A. J., Steneck, R. S., Greenfield, P., Gomez, E., et al. (2007). Coral reefs under rapid climate change and ocean acidification. Science 318, 1737–1742. doi: 10.1126/science.1152509
Hughes, T., Szmant, A. M., Steneck, R., Carpenter, R., and Miller, S. (1999). Algal blooms on coral reefs: what are the causes? Limnol. Oceanogr. 44, 1583–1586. doi: 10.4319/lo.1999.44.6.1583
Hughes, T. P. (1994). Catastrophes, phase shifts, and large-scale degradation of a Caribbean coral reef. Science 265, 1547–1551. doi: 10.1126/science.265.5178.1547
Hughes, T. P., Anderson, K. D., Connolly, S. R., Heron, S. F., Kerry, J. T., Lough, J. M., et al. (2018). Spatial and temporal patterns of mass bleaching of corals in the Anthropocene. Science 359, 80–83. doi: 10.1126/science.aan8048
Hughes, T. P., Barnes, M. L., Bellwood, D. R., Cinner, J. E., Cumming, G. S., Jackson, J. B. C., et al. (2017). Coral reefs in the Anthropocene. Nature 546, 82–90. doi: 10.1038/nature22901
Hughes, T. P., Bellwood, D. R., Folke, C. S., McCook, L. J., and Pandolfi, J. M. (2007a). No-take areas, herbivory and coral reef resilience. Trends Ecol. Evol. 22, 1–3. doi: 10.1016/j.tree.2006.10.009
Hughes, T. P., Graham, N. A., Jackson, J. B., Mumby, P. J., and Steneck, R. S. (2010). Rising to the challenge of sustaining coral reef resilience. Trends Ecol. Evol. 25, 633–642. doi: 10.1016/j.tree.2010.07.011
Hughes, T. P., Kerry, J. T., Baird, A. H., Connolly, S. R., Chase, T. J., Dietzel, A., et al. (2019). Global warming impairs stock-recruitment dynamics of corals. Nature 568, 387–390. doi: 10.1038/s41586-019-1081-y
Hughes, T. P., Rodrigues, M. J., Bellwood, D. R., Ceccarelli, D., Hoegh-Guldberg, O., McCook, L., et al. (2007b). Phase shifts, herbivory, and the resilience of coral reefs to climate change. Curr. Biol. 17, 360–365. doi: 10.1016/j.cub.2006.12.049
Idjadi, J. A., Haring, R. N., and Precht, W. F. (2010). Recovery of the sea urchin Diadema antillarum promotes scleractinian coral growth and survivorship on shallow Jamaican reefs. Mar. Ecol. Prog. Ser. 403, 91–100. doi: 10.3354/meps08463
Jackson, J. B., Kirby, M. X., Berger, W. H., Bjorndal, K. A., Botsford, L. W., Bourque, B. J., et al. (2001). Historical overfishing and the recent collapse of coastal ecosystems. Science 293, 629–637. doi: 10.1126/science.1059199
Kohler, K. E., and Gill, S. M. (2006). Coral point count with excel extensions (CPCe): a Visual Basic program for the determination of coral and substrate coverage using random point count methodology. Comput. Geosci. 32, 1259–1269. doi: 10.1016/j.cageo.2005.11.009
Ledlie, M., Graham, N. A., Bythell, J., Wilson, S., Jennings, S., Polunin, N. V., et al. (2007). Phase shifts and the role of herbivory in the resilience of coral reefs. Coral Reefs 26, 641–653. doi: 10.1007/s00338-007-0230-1
Lee, S. C. (2006). Habitat complexity and consumer-mediated positive feedbacks on a Caribbean coral reef. Oikos 112, 442–447. doi: 10.1111/j.0030-1299.2006.14247.x
Lessios, H. (1988). Mass mortality of Diadema antillarum in the Caribbean: what have we learned? Annu. Rev. Ecol. Syst. 19, 371–393. doi: 10.1146/annurev.es.19.110188.002103
Lessios, H. A. (2016). The great Diadema antillarum die-off: 30 years later. Annu. Rev. Mar. Sci. 8, 267–283. doi: 10.1146/annurev-marine-122414-033857
Lewis, J. B. (2004). Has random sampling been neglected in coral reef faunal surveys? Coral Reefs 23, 192–194. doi: 10.1007/s00338-004-0377-y
Maciá, S., Robinson, M. P., and Nalevanko, A. (2007). Experimental dispersal of recovering Diadema antillarum increases grazing intensity and reduces macroalgal abundance on a coral reef. Mar. Ecol. Prog. Ser. 348, 173–182. doi: 10.3354/meps06962
MacNeil, M. A., Graham, N. A., Cinner, J. E., Wilson, S. K., Williams, I. D., Maina, J., et al. (2015). Recovery potential of the world’s coral reef fishes. Nature 520, 341–344. doi: 10.1038/nature14358
McClanahan, T., and Kurtis, J. (1991). Population regulation of the rock-boring sea urchin Echinometra mathaei (de Blainville). J. Exp. Mar. Biol. Ecol. 147, 121–146. doi: 10.1016/0022-0981(91)90041-T
McClanahan, T., and Muthiga, N. (2016). Geographic extent and variation of a coral reef trophic cascade. Ecology 97, 1862–1872. doi: 10.1890/15-1492.1
Mcleod, E., Anthony, K. R., Mumby, P. J., Maynard, J., Beeden, R., Graham, N. A., et al. (2019). The future of resilience-based management in coral reef ecosystems. J. Environ. Manage. 233, 291–301. doi: 10.1016/j.jenvman.2018.11.034
Muallil, R. N., Deocadez, M. R., Martinez, R. J. S., Panga, F. M., Atrigenio, M. P., and Aliño, P. M. (2020). Negative trophic relationship between parrotfish biomass and algal cover on Philippine coral reefs. Reg. Stud. Mar. Sci. 39:101471. doi: 10.1016/j.rsma.2020.101471
Mumby, P. J., Dahlgren, C. P., Harborne, A. R., Kappel, C. V., Micheli, F., Brumbaugh, D. R., et al. (2006). Fishing, trophic cascades, and the process of grazing on coral reefs. Science 311, 98–101. doi: 10.1126/science.1121129
Mumby, P. J., Harborne, A. R., Williams, J., Kappel, C. V., Brumbaugh, D. R., Micheli, F., et al. (2007). Trophic cascade facilitates coral recruitment in a marine reserve. PNAS 104, 8362–8367. doi: 10.1073/pnas.0702602104
Mumby, P. J., and Steneck, R. S. (2008). Coral reef management and conservation in light of rapidly evolving ecological paradigms. Trends Ecol. Evol. 23, 555–563. doi: 10.1016/j.tree.2008.06.011
Neilson, B. J., Wall, C. B., Mancini, F. T., and Gewecke, C. A. (2018). Herbivore biocontrol and manual removal successfully reduce invasive macroalgae on coral reefs. PeerJ 6:e5332. doi: 10.7717/peerj.5332
Nozawa, Y., and Harrison, P. L. (2008). Temporal patterns of larval settlement and survivorship of two broadcast-spawning acroporid corals. Mar. Biol. 155, 347–351. doi: 10.1007/s00227-008-1034-8
Nozawa, Y., Lin, C.-H., and Chung, A.-C. (2013). Bathymetric Variation in Recruitment and Relative Importance of Pre-and Post-Settlement Processes in Coral Assemblages at Lyudao (Green Island), Taiwan. PLoS One 8:e81474. doi: 10.1371/journal.pone.0081474
Nozawa, Y., Lin, C.-H., and Meng, P.-J. (2020). Sea urchins (diadematids) promote coral recovery via recruitment on Taiwanese reefs. Coral Reefs 39, 1199–1207. doi: 10.1007/s00338-020-01955-1
O’Leary, J., Potts, D., Schoenrock, K., and McClahanan, T. (2013). Fish and sea urchin grazing opens settlement space equally but urchins reduce survival of coral recruits. Mar. Ecol. Prog. Ser. 493, 165–177. doi: 10.3354/meps10510
Pandolfi, J. M., Bradbury, R. H., Sala, E., Hughes, T. P., Bjorndal, K. A., Cooke, R. G., et al. (2003). Global trajectories of the long-term decline of coral reef ecosystems. Science 301, 955–958. doi: 10.1126/science.1085706
R Core Team (2018). R: A Language and Environment for Statistical Computing. Vienna: R Foundation for Statistical Computing.
Robinson, J. P., Wilson, S. K., and Graham, N. A. (2019). Abiotic and biotic controls on coral recovery 16 years after mass bleaching. Coral Reefs 38, 1255–1265. doi: 10.1007/s00338-019-01831-7
Roff, G., and Mumby, P. J. (2012). Global disparity in the resilience of coral reefs. Trends Ecol. Evol. 27, 404–413. doi: 10.1016/j.tree.2012.04.007
Rogers, A., and Lorenzen, K. (2016). Does slow and variable recovery of Diadema antillarum on Caribbean fore-reefs reflect density-dependent habitat selection? Front. Mar. Sci. 3:63. doi: 10.3389/fmars.2016.00063
Sammarco, P. W. (1980). Diadema and its relationship to coral spat mortality: grazing, competition, and biological disturbance. J. Exp. Mar. Biol. Ecol. 45, 245–272. doi: 10.1016/0022-0981(80)90061-1
Sammarco, P. W. (1982). Echinoid grazing as a structuring force in coral communities: whole reef manipulations. J. Exp. Mar. Biol. Ecol. 61, 31–55. doi: 10.1016/0022-0981(82)90020-X
Tioho, H., Tokeshi, M., and Nojima, S. (2001). Experimental analysis of recruitment in a scleractinian coral at high latitude. Mar. Ecol. Prog. Ser. 213, 79–86. doi: 10.3354/meps213079
Tokeshi, M., and Arakaki, S. (2012). Habitat complexity in aquatic systems: fractals and beyond. Hydrobiologia 685, 27–47. doi: 10.1007/s10750-011-0832-z
Topor, Z. M., Rasher, D. B., Duffy, J. E., and Brandl, S. J. (2019). Marine protected areas enhance coral reef functioning by promoting fish biodiversity. Conserv. Lett. 12:e12638. doi: 10.1111/conl.12638
Veron, J. E. N., and Stafford-Smith, M. (2000). Corals of the World. Townsville: Australian Institute of Marine Science.
Wang, W.-L., Liu, S.-L., and Li, T.-H. (2015). Seaweeds of Dongsha Atoll in the South China Sea. Kaohsiung: Marine National Park Headquarters.
Keywords: overfishing, herbivore, sea urchin, coral recruitment, Taiwan, Southeast Asia
Citation: Dang VDH, Cheung P-Y, Fong C-L, Mulla AJ, Shiu J-H, Lin C-H and Nozawa Y (2020) Sea Urchins Play an Increasingly Important Role for Coral Resilience Across Reefs in Taiwan. Front. Mar. Sci. 7:581945. doi: 10.3389/fmars.2020.581945
Received: 10 July 2020; Accepted: 04 November 2020;
Published: 15 December 2020.
Edited by:
Anastazia T. Banaszak, National Autonomous University of Mexico, MexicoReviewed by:
Douglas Fenner, NOAA NMFS Contractor, United StatesAldo Cróquer, Simón Bolívar University, Venezuela
Copyright © 2020 Dang, Cheung, Fong, Mulla, Shiu, Lin and Nozawa. This is an open-access article distributed under the terms of the Creative Commons Attribution License (CC BY). The use, distribution or reproduction in other forums is permitted, provided the original author(s) and the copyright owner(s) are credited and that the original publication in this journal is cited, in accordance with accepted academic practice. No use, distribution or reproduction is permitted which does not comply with these terms.
*Correspondence: Yoko Nozawa, nozaway@gate.sinica.edu.tw