- 1Department of Marine Science, University of Otago, Dunedin, New Zealand
- 2Fram Centre, Norwegian Polar Institute, Tromsø, Norway
- 3School of Biological Sciences, Victoria University of Wellington, Wellington, New Zealand
- 4School of Science, Waikato University, Hamilton, New Zealand
Chemical changes in the diffusive boundary layer (DBL) generated by photosynthesising macroalgae are expected to play an important role in modulating the effects of ocean acidification (OA), but little is known about the effects on early life stages of marine invertebrates in modified DBLs. Larvae that settle to macroalgal surfaces and remain within the DBL will experience pH conditions markedly different from the bulk seawater. We investigated the interactive effects of seawater pH and DBL thickness on settlement and early post-settlement growth of the sea urchin Pseudechinus huttoni, testing whether coralline-algal DBLs act as an environmental buffer to OA. DBL thickness and pH levels (estimated from well-established relationships with oxygen concentration) above the crustose coralline algal surfaces varied with light availability (with photosynthesis increasing pH to as high as pH 9.0 and respiration reducing pH to as low as pH 7.4 under light and dark conditions, respectively), independent of bulk seawater pH (7.5, 7.7, and 8.1). Settlement success of P. huttoni increased over time for all treatments, irrespective of estimated pH in the DBL. Juvenile test growth was similar in all DBL manipulations, showing resilience to variable and low seawater pH. Spine development, however, displayed greater variance with spine growth being negatively affected by reduced seawater pH in the DBL only in the dark treatments. Scanning electron microscopy revealed no observable differences in structural integrity or morphology of the sea urchin spines among pH treatments. Our results suggest that early juvenile stages of P. huttoni are well adapted to variable pH regimes in the DBL of macroalgae across a range of bulk seawater pH treatments.
Introduction
Ocean acidification (OA) occurs when seawater pH decreases due to the uptake of elevated atmospheric CO2 by the surface ocean water (Caldeira and Wickett, 2003). Reduced mainstream (or bulk) seawater pH due to OA poses a major threat to marine ecosystems including calcifying organisms (Dupont et al., 2010; Hendriks et al., 2010; Byrne, 2011; Dupont and Thorndyke, 2013; Kroeker et al., 2013). To date, most research on the effects of OA on marine invertebrates has utilized constant pH conditions representative of bulk seawater pH levels (Wahl et al., 2015; Boyd et al., 2016). However, newly-settled and metamorphosing organisms are likely to experience pH conditions different from the bulk seawater when settled on marine algae and biofilms, which may account for some of the variability found in settlement and post-settlement development of invertebrate responses to OA (Dupont et al., 2010; Albright et al., 2012; Wangensteen et al., 2013; Wolfe et al., 2013a; García et al., 2015). For example, Mos et al. (2019) observed greater survival and growth in newly settled Tripneustes gratilla raised on concrete substrates, attributed to the chemical buffering of reduced seawater pH in the boundary layers.
The diffusive boundary layer (DBL) is a micro-layer of seawater (μm to cm scale) around marine organisms where, due to biological activity, the chemical and physical environment is different from the surrounding bulk seawater (Denny and Wethey, 2000; Cornwall et al., 2013b). The potential thickness of the DBL near the surface of macroalgae and marine biofilms is inversely correlated to water velocity, with thicker DBLs resulting in greater gradients between the near-surface and bulk seawater in concentrations of metabolically used or excreted dissolved substances (Cornwall et al., 2015). Within DBLs around macroalgae, variations in light availability drive pH variability (Hurd et al., 2011), with pH generally increasing during the day due to photosynthetic uptake of dissolved inorganic carbon and decreasing at night due to respiration of CO2 (De Beer and Larkum, 2001; Cornwall et al., 2013b). The pH experienced by organisms within the DBL above photosynthesizing surfaces, therefore, likely differs greatly from surrounding seawater pH.
Thick DBLs that form at the surface of macroalgae may ameliorate some of the negative effects of OA due to the formation of a microenvironment where pH is increased during the day, resulting in exposure to higher mean pH over diel cycles (Hurd et al., 2011; Cornwall et al., 2013b, 2014). However, the potential impacts of seaweed DBLs are yet to be considered on early life stages of invertebrates under future OA conditions (Koehl and Hadfield, 2010; Espinel-Velasco et al., 2018), and a better understanding of how pH fluctuations within the DBL will amplify or alleviate the impacts of reduced bulk seawater pH on surrounding marine organisms is needed (Shaw et al., 2012; Cornwall et al., 2014; Wahl et al., 2015).
Sea urchins (Echinodermata: Echinoidea) are thought to be vulnerable to OA and their early life-history stages and adults have been widely used to investigate the effects of reduced seawater pH (Dupont and Thorndyke, 2013). Within indirect lifecycles, settlement, and early post-settlement stages are particularly vulnerable to OA and other environmental stressors, and therefore are a potential population bottleneck (Gosselin and Qian, 1997; Wolfe et al., 2013a). The effects of OA on the development of echinoderms in the settlement and early post-settlement period can be variable (Dupont et al., 2010; Byrne, 2011; Kroeker et al., 2013; Przeslawski et al., 2015; Byrne et al., 2017; Espinel-Velasco et al., 2018). Previous studies have found negative effects on settlement success in sea urchins due to reduced seawater pH, including delayed metamorphosis (Dupont et al., 2013; García et al., 2015), while other studies have found no effects (Byrne et al., 2010a; Wangensteen et al., 2013). Early post-settlement growth in response to reduced pH is similarly varied, with some juvenile sea urchin survival or test diameter growth being relatively robust to OA (Wolfe et al., 2013a), and even increasing (García et al., 2015). Spine development is similarly robust with reductions usually occurring under only the lowest pH levels (7.4) tested (Wolfe et al., 2013a). Byrne et al. (2010a) and Wangensteen et al. (2013) observed a reduction in size and a smaller proportion of juveniles with normal morphology in reduced bulk seawater pH.
Here, we investigated the ecological implications of pH modifications in the DBL, created by crustose coralline algae (CCA) under OA conditions, on the settlement and early post-settlement juvenile stage of sea urchin Pseudechinus huttoni Benham 1908 (Echinoidea, Temnopleuridae). P. huttoni is a species endemic to New Zealand found in slow-flow environments along the continental shelf (<60 m) and southern fjords (McClary and Sewell, 2003; Kirby et al., 2006). CCA are well-known settlement inducers of marine invertebrate larvae and also a food source for many (McCoy and Kamenos, 2015) including mollusks (Roberts, 2001; Roberts et al., 2010), corals (Foster and Gilmour, 2016), and echinoderms (Uthicke et al., 2013). Coralline algae were selected for this study, being the most abundant encrusting taxa on New Zealand’s shallow rocky reefs (Shears and Babock, 2007) and have been shown to be a key larval settlement substrate for sea urchins (Lamare and Barker, 2001). The pH and thickness of the DBLs were experimentally manipulated via light intensity in contemporary (pHT 8.1) or near-future bulk seawater pH levels (pHT 7.5 and 7.7) predicted for the end of century and beyond (IPCC 2019). We determined settlement and metamorphosis success of P. huttoni as well as early post-settlement growth (test diameter and spine length 4 day post-settlement) across these treatment combinations. Urchin skeletal elements and spines were also examined using scanning electron microscopy (SEM) to visualize effects on calcification and growth.
Materials and Methods
CCA Collection and Storage
Small CCA encrusted cobbles (0.5–2 cm diameter) were collected from the shallow subtidal (<2 m depth) adjacent to the Portobello Marine Laboratory (PML), Otago Harbor, New Zealand (45°52.51’S, 170°30.9’E) during July and August 2018. These cobbles consisted of a natural assemblage of CCA, likely containing multiple species, reflecting in situ conditions. While different cobbles were used during experiments, all cobbles were collected in the same location, were the same approximate size and had estimated coverage of about 80% CCA (Supplementary Figure 1). Prior to use in experiments, cobbles were visually examined for extraneous organisms, which were removed using a scalpel without damaging the CCA. Cobbles were kept at PML in 50 L flow-through tanks supplied with filtered seawater (10 μm). Tanks were covered with black mesh to limit excessive light. Typically during winter and spring, seawater from the Otago Harbor has a salinity of 33 and temperature 7–11°C (Nelson, 2016).
DBL Measurements
Oxygen profiles were measured above CCA substrates submerged in 3 L plastic experimental aquaria under six experimental treatment conditions: two irradiance levels (dark and light) and three bulk seawater pH levels (pHT 7.5, 7.7, and 8.1). Dark treatments received <1 μmol photons m–2 s–1 irradiance, while light treatments received a photosynthetically active radiation (PAR; 400 to 700 nm) of 10 μmol photons m–2 s–1. PAR was provided by overhead standard fluorescent tubes in an under-verandah housing, suspended approximately 1 m above the experimental aquaria. The tubes were cool white 845, producing approximately 80% of the natural spectrum at a color temperature of 4500K. Incident irradiance was measured using a flat terrestrial LI-250A quantum sensor (LI-COR, Lincoln, United Kingdom). These measurements were completed under static (no) flow conditions. Within macroalgal turf communities, flows can be extremely slow (<1 cm s–1; Pöhn et al., 2001; Cornwall et al., 2015). Measurements of the DBL were also completed above CCA covered acrylic disks in an annular flume to characterize and compare the DBL under quantifiable flow speeds (Methods, Supplementary Material).
Oxygen Profiles
Oxygen concentration profiles were measured using a microsensor from the Unisense MicroRespiration System (Unisense, Aarhus, Denmark) connected to a Unisense Microsensor Multimeter and controlled by a Unisense automatic micromanipulator. SensorTrace Profiling software was used to log the microsensor profiles. Prior to use, the oxygen microsensor was first pre-polarized with -0.80 V for a minimum 10 min period and then calibrated in a fully aerated (100% value) and anoxic (0% value) solution in the SensorTrace Logger software as per the Unisense Oxygen Sensor User Manual.
The sensor was manually lowered with the micromanipulator until it was touching the surface of the substrate, this was considered to be 0 μm above the substrate surface. In this position, a 40 min equilibration period was allowed for the DBL to re-establish before vertical profiles began. The micromanipulator was used to automatically raise the sensor and record 60 s of oxygen concentration (μmol O2 l–1) measurements at each height. The wait time between each measurement was 0.1 s and the measurement period was 0.9 s. Of the 60 measurements at each height, the first and last 5 measurements were discarded in case movement of the sensor affected readings, and the remaining 50 measurements were averaged. Measurements were taken every 100 μm from 0 to 4 mm above the substrate and then every 1 mm from 4–10 mm. A minimum of four replicate oxygen profiles was measured above CCA substrates for each experimental treatment.
DBL Calculations
Diffusive boundary layer thickness was defined as the distance above the surface of the CCA substrate at which the changes in concentrations of O2 (raw value) were <5% per 0.1 mm for four subsequent measurements. This 5% cutoff (Layton et al., 2019) balances the robustness of previous methods that used 10% (Hurd et al., 2011; Cornwall et al., 2013b; Lichtenberg et al., 2017) and the sensitivity of changes <1% (Cornwall et al., 2015; Noisette and Hurd, 2018). In order to account for small variation among replicates in bulk seawater oxygen concentration, oxygen profiles were standardized by dividing the concentration at any given height by the bulk seawater concentration (the oxygen concentration 10 mm above the CCA substrate).
H+ (pH) and O2 concentrations have a strong relationship within the DBL above CCA substrates (R2 = 0.82; Cornwall et al., 2014) due to the uptake and release of CO2 and O2 during photosynthesis and respiration (Cornwall, 2013; Chan et al., 2016; Noisette and Hurd, 2018). The magnitude and direction of pH changes within the DBL have been found to be almost identical to changes in O2 concentrations (Cornwall et al., 2014). Thus, O2 is commonly used as a robust proxy for H+ and pH (Cornwall et al., 2014; Layton et al., 2019). Standardized O2 concentrations were converted into H+ concentrations using separate relationships previously determined for CCA (Cornwall, 2013). These relationships were derived from measurements conducted in the DBL of coralline algae collected from the same region as those in this study (Cornwall et al., 2013b) and take into account potential modification of pH due to calcification and dissolution:
In the dark (R2 = 0.85):
and light (R2 = 0.73):
H+ concentrations were converted into pHT values using the equation pHT = -log [H+]. Seawater pH values were standardized for each profile by dividing the concentration at any given height by the bulk seawater pH concentration to get pH deviation in the DBL from the bulk (mainstream) pH. Estimated pH values were calculated by adding the pH deviation to the mean bulk seawater pH value for the respective treatment.
In order to estimate the pH conditions that metamorphosing and newly settled P. huttoni experienced during experiments (pHEXP), pH was averaged from 0 to 0.5 mm above the CCA surface. A height of 0 to 0.5 mm was chosen since the test diameter of newly settled P. huttoni ranged between 400 to 500 μm.
Sea Urchin Collection, Spawning and Rearing
Adult P. huttoni were collected from Doubtful Sound, Fiordland in June 2018 by SCUBA divers and transported to PML where they were kept in a flow through system prior to experiments. Four days after collection, adults were induced to spawn by an intra-coelomic injection of 0.5 M KCL per animal. The gametes obtained were visually inspected for viability under the microscope (swimming sperm and healthy eggs), and deemed suitable for fertilization. Sperm stock solution was added to the egg solution until reaching a final fertilization success of more than 95% (indicated by the appearance of the fertilization membrane). The resulting larvae were reared until competency (i.e., ready to settle) at 10–13°C and under ambient pHT = 8.1 in 20 glass jars (2.5 L) at densities ranging from 3 to 20 larvae per mL. Larvae were daily fed a combination of Rhodomonas sp. and Dunaliella primolecta at a total concentration of ∼8,000 cells ml–1. To keep larvae and food suspended, cultures were stirred continuously with large plastic paddles, at a rate of 10 cycles per minute. Water was changed every third day and jars were cleaned periodically. Most larvae showed signs of competency (e.g., well-developed rudiments) from 60 to 75 day post-fertilisation (dpf).
Settlement Experiment
Competent larvae were used to examine settlement and metamorphosis of P. huttoni in response to pH and light intensity (therefore DBL characteristics). CCA covered cobbles (0.5 to 2 cm diameter) were placed in custom-made inverted settlement chambers, as per methodology specifically developed for such experiments (Espinel-Velasco et al., 2020). The chambers were closed by a 100 μm mesh cap on the top end to allow water circulation while keeping the larvae inside. The chambers were then placed in 3 L airtight aquaria containing water at the desired target pH (bulk pH) in both light and dark conditions. Three pH treatments were used for this experiment (pHT 7.5, 7.7, and 8.1). Each treatment (3 L aquaria) contained four inverted chambers and was replicated 3 times. Competent larvae of all the glass cultures were pooled and homogenized before using for the settlement assay. Approximately 30 competent larvae (61 dpf) were added into each chamber and left to settle. After 6, 18, 30, and 51 h, the chambers were checked for settlement under a dissecting microscope. Larvae were counted and placed into two categories: (1) settled and metamorphosed: indicated by the presence of juveniles at the bottom of the chambers, and (2) not-settled: remaining larvae, still in the larval form, swimming in the water column or crawling on the bottom.
Post-settlement Growth Experiment
Post-settlement growth of P. huttoni was determined in response to pH and light intensity (therefore DBL characteristics). For this, approximately 4,700 competent larvae (69 dpf) were placed into a 3 L, airtight aquaria with ∼200 CCA covered cobbles, with a diameter between 0.5 and 2 cm. The larvae were left for 24 h to settle on the cobbles, based on results from the settlement experiment. After 24 h, cobbles were visually examined under the dissecting microscope. Cobbles with at least one juvenile attached to them were used for experiments. A total of six cobbles were placed into each experimental tank and left there for a total of 4 days (5 days after introduction to the cue), given that sea urchin juveniles undergo a perimetamorphic period that generally lasts at least 4 day, where they are unable to feed because they have yet to develop a digestive system and other internal structures (Gosselin and Jangoux, 1998; Rahman et al., 2012). Newly settled P. huttoni juveniles were grown under six experimental treatment conditions, comprised of three bulk seawater pH levels (pHT 7.5, 7.7, and 8.1) and two irradiance levels (light and dark), with each treatment replicated three times.
After 4 days in the treatment conditions, cobbles were examined under a dissecting microscope and juveniles were removed from the substrate by a strong water blast with a Pasteur pipette. After removal, juveniles were transferred into eppendorf tubes and fixed with 0.5 mL of paraformaldehyde solution (4% PFA in FSW, buffered). Juveniles were stored in a fridge and photographed with a camera (Olympus XC50) under a compound microscope (Olympus BX51) within the three following days, since preliminary tests had showed no signs of post-fixation change. Images were discarded if juveniles were (1) not lying flat on their aboral or oral surface, (2) covered by algae, another urchin or other unknown material, or (3) if the sea urchin showed signs of disintegration (i.e., did not successfully metamorphose and survive). Each juvenile was photographed multiple times in different focal planes. Average spine length and test diameter were measured for each juvenile with Image-J (NIH, Cary, NC, United States; Figure 1). Two test diameters were measured, approximately perpendicular to each other at the longest diameter of the test, and subsequently averaged. The three longest spines were measured from the outer edge of the test to the tip of the spine and subsequently averaged. The number of juveniles measured per replicate (3 replicates per treatment), ranged from 3 to 11, with an average of 7 juveniles measured per replicate.
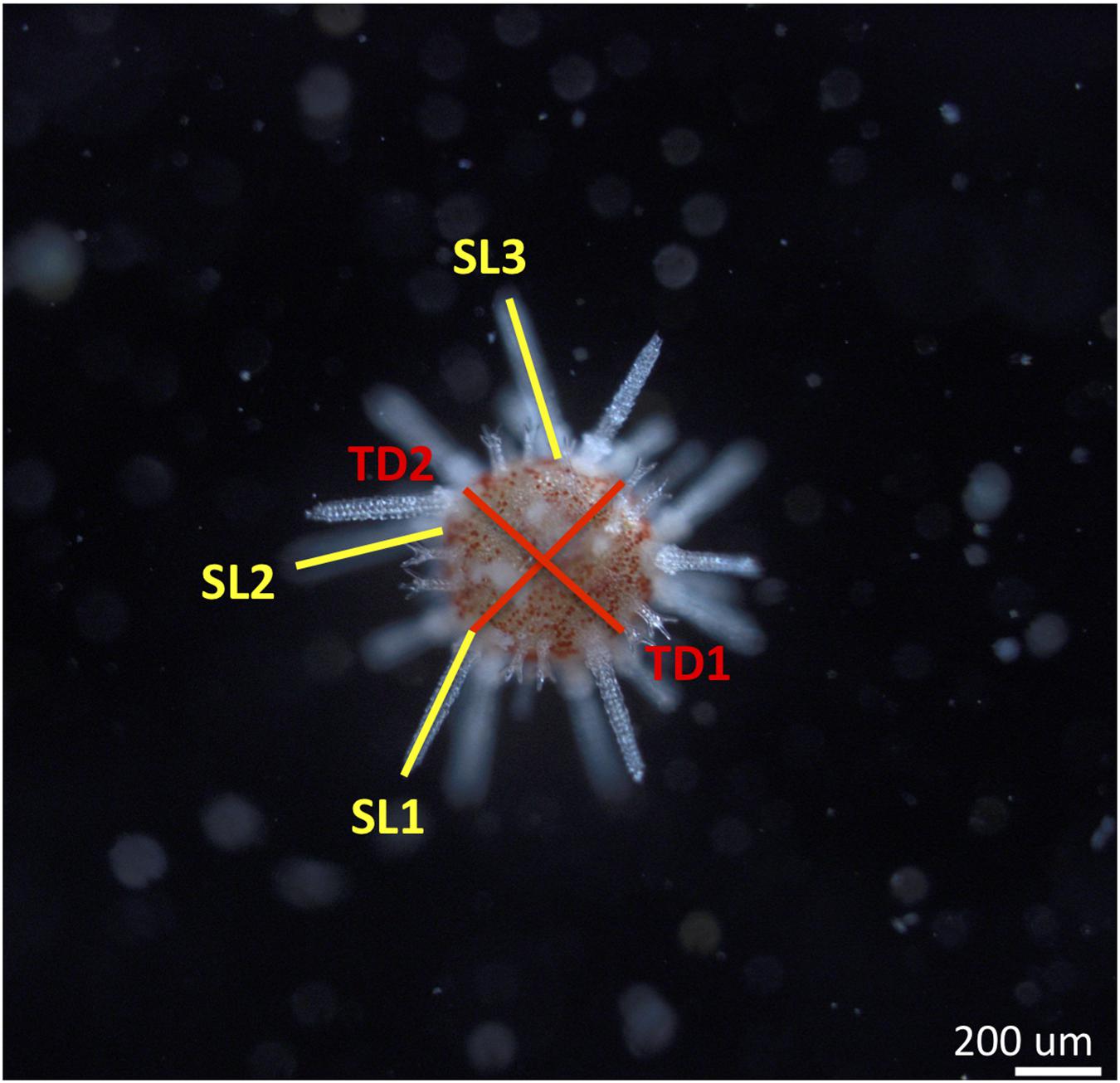
Figure 1. Image taken with a compound microscope of a juvenile sea urchin Pseudechinus huttoni 4 days post-settlement. Morphometric measurements are spine length (average of three longest spines or SL) and test diameter (average of two longest diameters or TD). Scale bar 200 μm.
Juveniles from the post-settlement growth experiment were prepared for analysis with SEM in order to determine calcification patterns of skeletal elements. Juveniles were placed in a 1% NaClO treatment for 30 min in order to remove organic material. The juveniles were consequently rinsed three times with distilled water and placed in a drying oven at 45°C for 48 h (Wolfe et al., 2013a). The juvenile skeletons lost their structure after the treatment, leaving disarticulated pieces of the spine and skeleton. These pieces were mounted on stubs with conductive double-sided tape and coated with gold using a Quorum gold coater. Observations and photographs were taken using a Hitachi TM3000 tabletop SEM.
Water Chemistry
Target experimental seawater pH was obtained in a 90 L tank for each treatment that was constantly maintained at each target pH level by controlled bubbling of 100% CO2 gas into the system as required via TUNZE pH/CO2 controllers (TUNZE AQUARIENTECHNIK GMBH, Penzberg, Germany; Comeau et al., 2018). Seawater pH and temperature were recorded at the beginning and end of every profile during measurements of the DBL in the 3 L aquaria (Table 2). Temperature was measured using a mercury thermometer, while pH was measured spectrophotometrically using Cresol purple dye (Dickson et al., 2007). Seawater pH is reported on the total scale in this manuscript, unless specifically mentioned otherwise. Target bulk pH levels were maintained in the settlement experiment by adding new water from the 90 L header tanks at the target pH every 6, 18, 30, and 51 h (Supplementary Table 1) and every 24 h in the post-settlement growth experiment (Supplementary Table 2). One liter seawater samples for AT and DIC were fixed with saturated HgCl. Seawater samples for AT and DIC for each treatment were taken at the beginning of the settlement experiment.
Dissolved inorganic carbon and total alkalinity were later determined by a Single Operator Multi-parameter Metabolic Analyzer (SOMMA) and closed-cell potentiometric titration, respectively (Dickson et al., 2007). CRM batch number 168 was used for this analysis in September and October 2018. During which, the precision of the DIC analyses was 0.72 μmol kg–1. The analyzed DIC values were corrected by a factor CRMcertified/CRManalyzed, determined daily. The analysis accuracy is 0.7 μmol kg–1. Other carbonate system parameters, including the partial pressure of CO2 (pCO2) and the saturation states for calcite (Ωcalcite) and aragonite (Ωaragonite), were calculated using SWCO2 (Mosley et al., 2010). Seawater properties were determined using CO2 equilibrium constants from Mehrbach et al. (1973) refitted by Dickson and Millero (1987).
Statistical Analysis
Statistical analyses were performed using RStudio, v1.1.453 (RStudio Team, 2016) unless otherwise mentioned. Data was checked for homogeneity of variance using Levene’s tests and checked for normality using QQplots and Shapiro–Wilk Tests.
DBL Measurements
We tested for significant differences in DBL thickness and O2 concentration at the surface of CCA among treatments using non-parametric Kruskal–Wallis tests (Table 3), since assumptions of normality and heteroscedasticity were not met. Kruskal–Wallis tests were supported by results of a Welch’s analysis of variance (ANOVA) with unequal variances. Post hoc Dunn tests (Supplementary Table 3) were used to show pairwise differences in all of the independent treatment conditions in DBL thickness and O2 concentration at the surface of CCA.
Settlement Data
The settlement data was analyzed by means of fitting a general linear mixed regression model (linear model with binomial distribution: function glmer, family: binomial, R v.2.13) to describe the effect of the pH treatment (bulk seawater pH) and irradiance (light vs. dark) on the percentage settlement of the larvae over time. This model, using a binomial distribution and settlement chambers as random factor, allowed us to include the information of the repeated measures of settlement in the chambers over time. Model selection included all variables initially (bulk seawater pH, irradiance, and time) and was based on selecting the best model based on the Akaike information criterion (AIC) value. Significance and model fit (AIC, residual plots) can be found in Supplementary Material (Supplementary Table 4).
Post-settlement Data
The post-settlement data was analyzed using a linear mixed model (function: lmer, R v.3.5.2) to describe the effect of the bulk seawater pH treatment (treated as a continuous variable) and irradiance (light vs. dark) on spine length and test diameter of 4-day-old juvenile sea urchins. Replicate containers were used as a random factor in the model (3 replicate containers per treatment). Significance and model (AIC, residual plots) can be found in Supplementary Material (Supplementary Table 5).
Results
Water Chemistry
Seawater carbonate chemistry was taken at the beginning of the settlement experiment from the 90 L header tanks (Table 1, and for additional experiments reported in Supplementary Table 6). During the course of the settlement and post-settlement experiments, pH stayed relatively stable (±0.1 units) between water changes (Supplementary Tables 1, 2). While completing DBL measurements in the 3 L aquaria, bulk seawater pH also remained similar between oxygen profiles, with clear differences among the three bulk pH treatments and no effect of light on bulk pH (Table 2).
DBL Measurements
DBL Measurements in Static Conditions
The chemical environment immediately above the coralline algal surface differed from that of the bulk seawater. At all levels of bulk seawater pH, pH increased in the light treatment above CCA and decreased in the dark treatment (Figure 2 and Supplementary Figure 2), with irradiance having a significant effect on substrate surface oxygen concentration (Chi Square = 17.28, p < 0.001, df = 1, and Table 3). In the light, estimated pH deviated by up to ∼0.8 units from bulk pH (Figure 2), with the pH at the surface of the CCA in bulk seawater pH 7.5 being estimated as above that of today’s average seawater pH levels of 8.1 (Figure 2). In the dark, oxygen concentration at the surface of CCA had much smaller deviations, with pH varying less than 0.1 units below median values in all bulk seawater conditions (Figure 2). In the dark, pH values at the surface of CCA were similar to those of the bulk seawater pH and bulk seawater pH had no significant effect on oxygen concentration at the surface of CCA (Chi Square = 0.215, p-value > 0.1, df = 2, and Table 3).
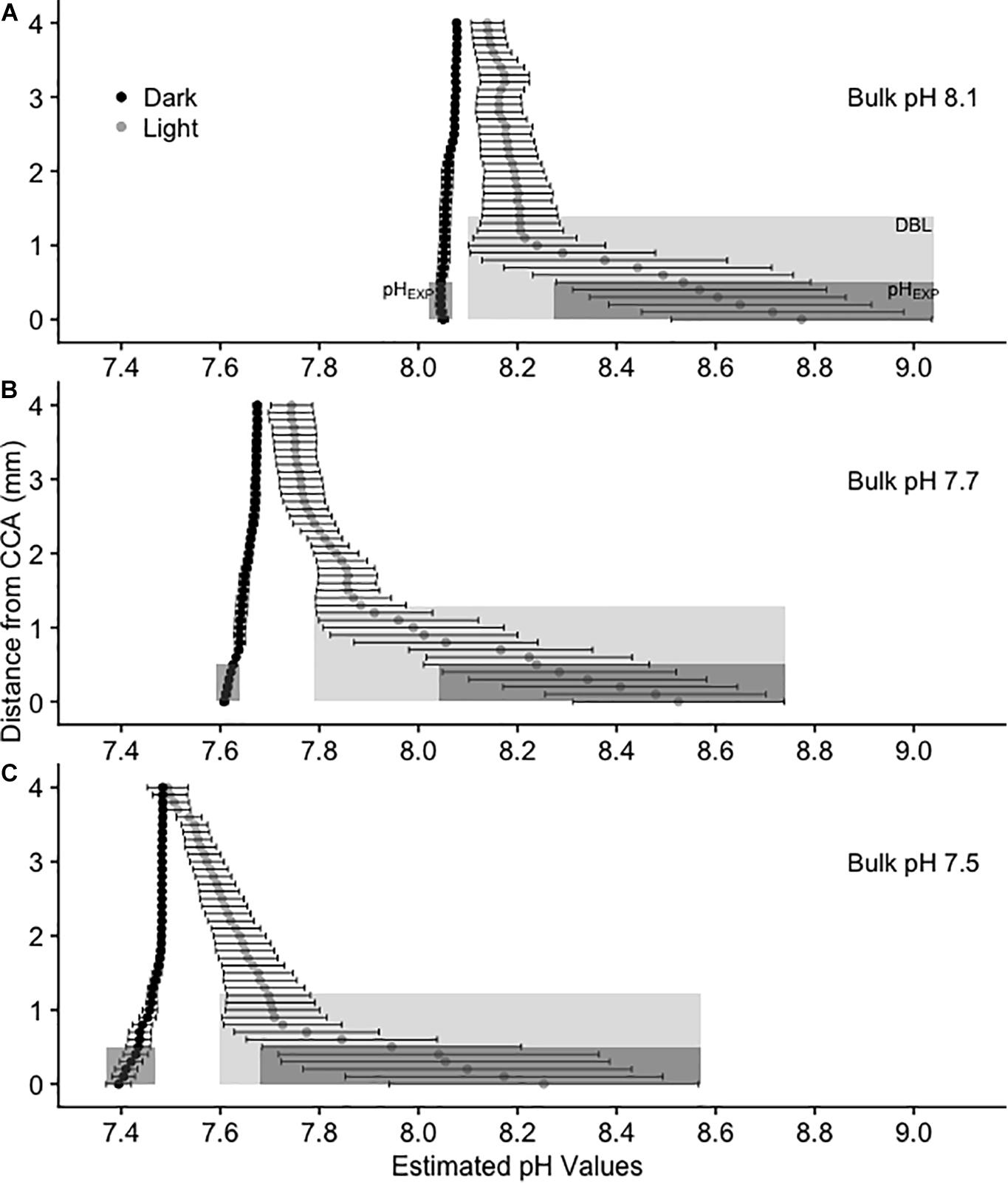
Figure 2. Calculated average pH(T) values above CCA in 3 L experimental aquaria at three bulk pH levels: (A) 8.1, (B) 7.7, and (C) 7.5. Values are mean pH ± SE, n = 4. pH values were estimated using oxygen as a proxy. Black circles represent dark treatments and gray circles represent light treatments. The light gray shaded rectangle represents the diffusion boundary layer and the dark gray shaded rectangle represents the pH from 0 to 0.5 mm above the CCA surface (pHEXP), or the average pH that metamorphosing and newly settled P. huttoni on CCA substrates experience.
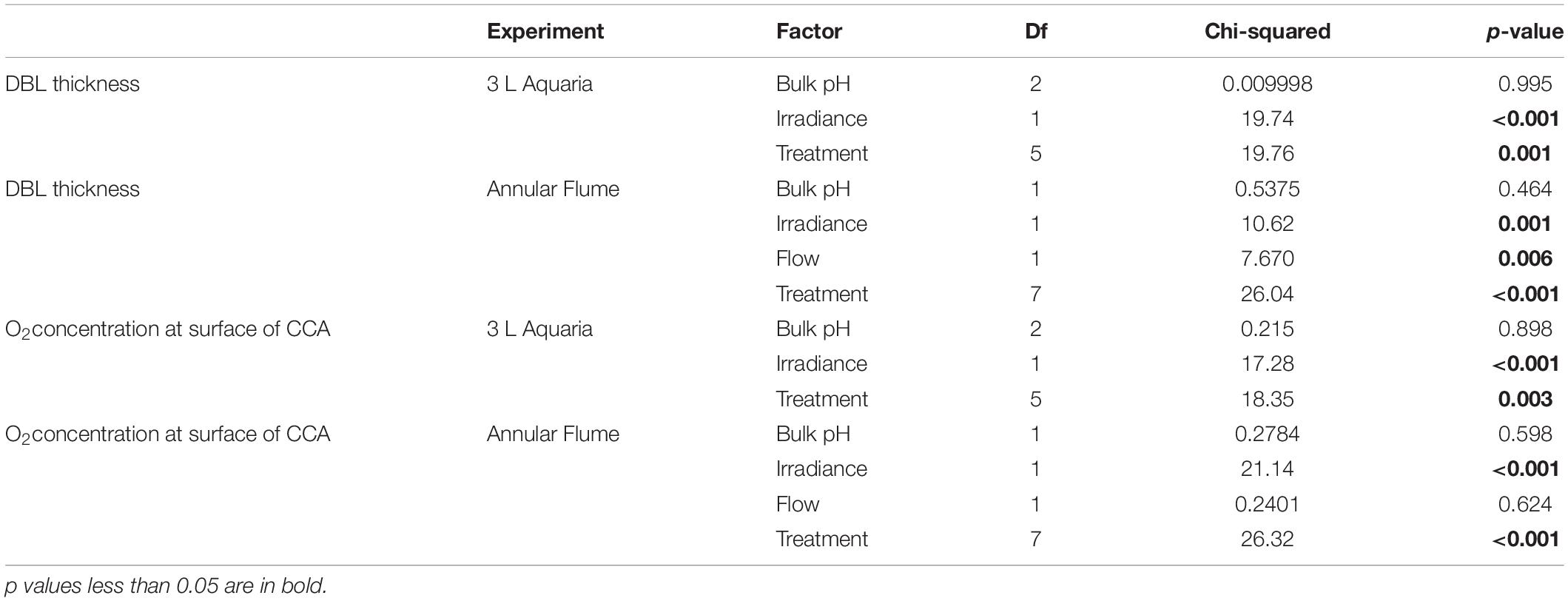
Table 3. Non-parametric Kruskal–Wallis examining the effects of treatments, bulk pH, flow, and irradiance on DBL thickness and O2 Concentration at surface of CCA in 3 L aquaria and the annular flume.
The release of CO2 due to the respiration of CCA, was evidently too low to generate a measurable DBL in the dark treatments. DBLs were significantly different (thicker) in the light than in the dark (Chi Square = 19.74, p < 0.001, df = 1, and Table 3). In the light, mean (±SE) DBL thickness was 1.4 mm ± 0.53 at bulk pH 8.1, 1.3 mm ± 0.40 at bulk pH 7.7 and 1.2 mm ± 0.4 at bulk pH 7.5 but these differences were not significant (Chi Square = 0.01, p > 0.1, df = 2, and Table 3).
In the dark treatments, P. huttoni likely experienced pH levels similar to the bulk seawater (pHEXP was reduced by less than 0.1 units below mainstream values), whereas in the light, P. huttoni experienced pH levels substantially higher (pHEXP increased by 0.5 to 0.7 units above mainstream values). Values are reported in Supplementary Table 7. Due to the DBL, the pHEXP that P. huttoni experienced in light treatments with bulk seawater pH 7.5, was estimated to be 8.05, comparable to contemporary seawater pH levels of 8.1.
DBL Measurements in Flow
Oxygen profiles were also completed above CCA covered acrylic disks in an annular flume to characterize oxygen and pH conditions above CCA under quantifiable flow speeds (Methods, Supplementary Material). This allowed us to compare the DBL under more ecologically relevant flow speeds to the static flow conditions used in this experiment that enabled us to settle and grow P. huttoni juveniles. The DBL profiles and DBL thicknesses in static flow in the 3 L experimental aquaria displayed similar trends to those at slow flow speeds of 1 cm s–1 in the annular flume (Supplementary Figures 3, 4). In the light, pH varied up to ∼0.8 units above the bulk seawater pH in static (no) flow, and up to ∼0.6 units above the bulk seawater pH in slow (1 cm s–1) flow. In the dark, pH varied by <0.1 units under both static and slow flow. As under static flow, irradiance had a significant effect on substrate surface oxygen concentration in slow flow (p < 0.001, df = 1, and Table 3), while bulk seawater pH did not (p > 0.1, df = 1, and Table 3). In the light under slow flow, mean (±SE) DBL thickness was 0.78 mm ± 0.28 in the pH 8.1 treatment and 0.50 mm ± 0.14 in the pH 7.4 treatment. In the dark under slow flow, mean (±SE) DBL thickness was 0.1 mm ± 0.04 in the pH 8.1 treatment and 0.08 mm ± 0.03 in the pH 7.4 treatment. The static flow conditions used in these sea urchin experiments are similar to slow flow speeds (1 to 2 cm s–1, Supplementary Figure 5) found in situ in the fiord environment where the P. huttoni used in this study were collected.
Increased speeds of flow altered the shape of oxygen (and accordingly the pH) profiles and DBL thickness. Oxygen profiles at fast flow speeds of 5.5 cm s–1 displayed a thinner DBL and a smaller range of O2 and pH values (Supplementary Figures 3, 4). At 5.5 cm s–1, mean pH values varied by only up to ∼0.2 units in the light. Additionally, flow had a significant effect on DBL thickness (p = 0.006, df = 1, and Table 3). In the light, mean (±SE) DBL thickness under slow flow was 0.78 mm ± 0.28 in the pH 8.1 treatment and 0.50 mm ± 0.14 in the pH 7.4 treatment compared to 0.05 mm ± 0.05 and 0.10 ± 0.00 in fast flow, respectively.
Settlement
After 6 h, 14% to 33% of larvae had settled on the CCA. Cumulatively, percentage settlement on CCA increased with time for all treatments, reaching a final settlement of 58% to 77% after 51 h (Figure 3 and Supplementary Table 7). Bulk seawater pH showed no significant effect on its own, but light conditions and their interaction with the bulk seawater pH of the water were significant factors influencing settlement success in P. huttoni over the course of the experiment (Supplementary Table 4). After the initial 6 h, percentage settlement was significantly lower in bulk pH 8.1:dark conditions (pHEXP 8.01) compared to the other treatments (14%). After 18 h, percentage settlement in bulk pH 7.7:light conditions (pHEXP 8.38) did not increase as greatly compared to the other treatments (37%). However, toward the end of the experiment (after 30 and 51 h), only the settlement in bulk pH 8.1:light conditions (pHEXP 8.64) was significantly greater than in the remaining treatments (68% and 77%, respectively). The pH that the sea urchins likely experienced while settling on CCA substrate (pHEXP) showed no consistent or strong effect on settlement success.
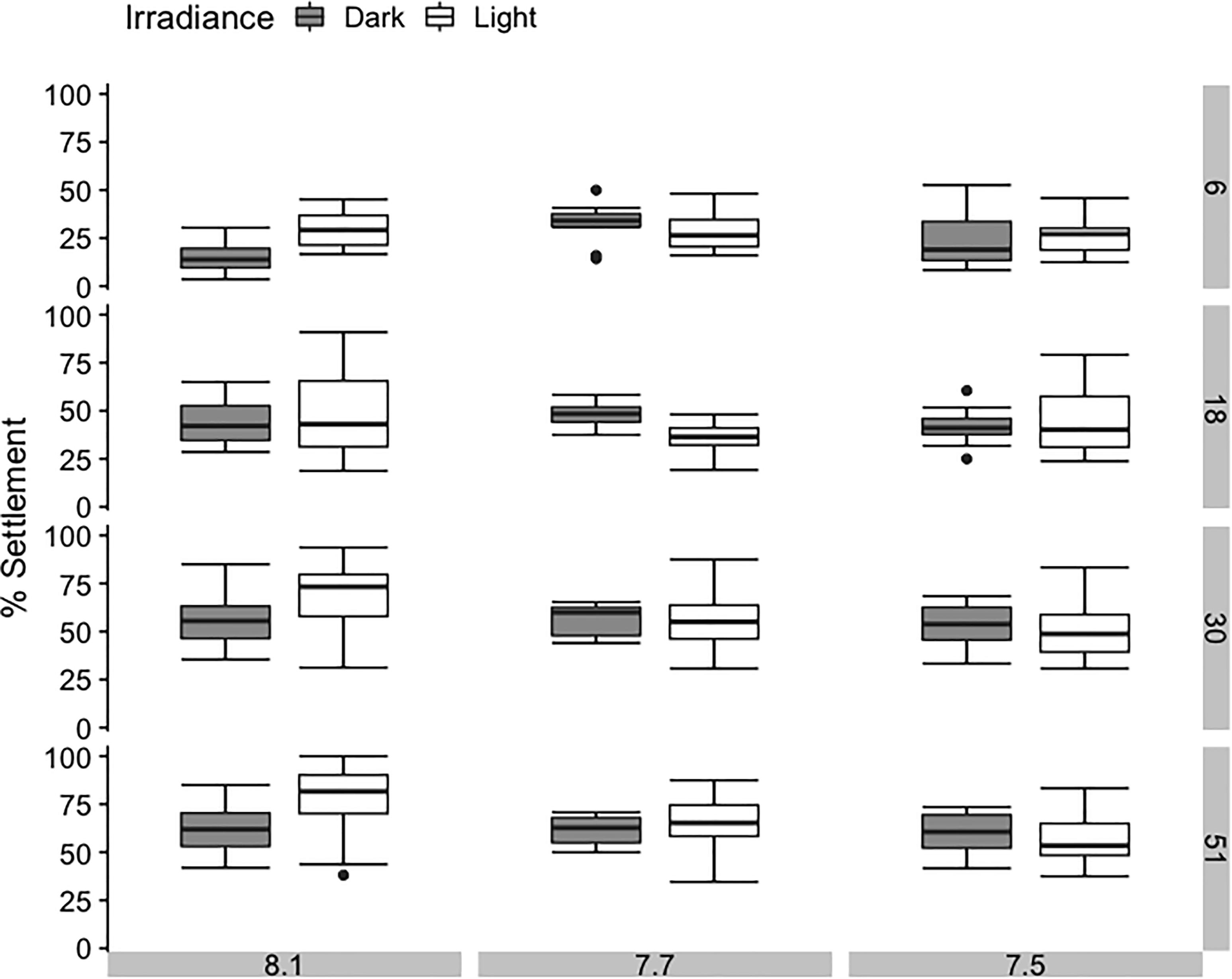
Figure 3. Average percentage settlement of sea urchins Pseudechinus huttoni during a 51-h period under three bulk seawater pH and two irradiance treatments. Each treatment (3 L aquaria) contained four inverted chambers and was replicated three times. Error bars are ±1 SE of the mean.
Post-settlement Growth
Test diameter was similar across all treatments, with no significant effect of bulk seawater pH or irradiance (Figure 4, p = 0.290 and p = 0.755, Supplementary Table 5). Spine length displayed greater variance across all treatments, but also showed no significant effect of bulk seawater pH on its own (p = 0.090, Supplementary Table 5). However, the results of the linear mixed modeling indicated that irradiance and the interaction of pH and irradiance had a significant effect on spine length of 4-day-old juveniles (p = 0.039 and p = 0.035, respectively, Supplementary Table 5). In the dark, mean spine length increased with increasing bulk seawater pH, while in the light, mean spine length decreased with increasing bulk seawater pH. The shortest mean spine length was found in the treatment with the highest pHEXP, bulk pH 8.1:light. The pH that juvenile sea urchins likely experienced while settling on CCA substrate (pHEXP) showed no consistent or strong effect on test diameter or spine length.
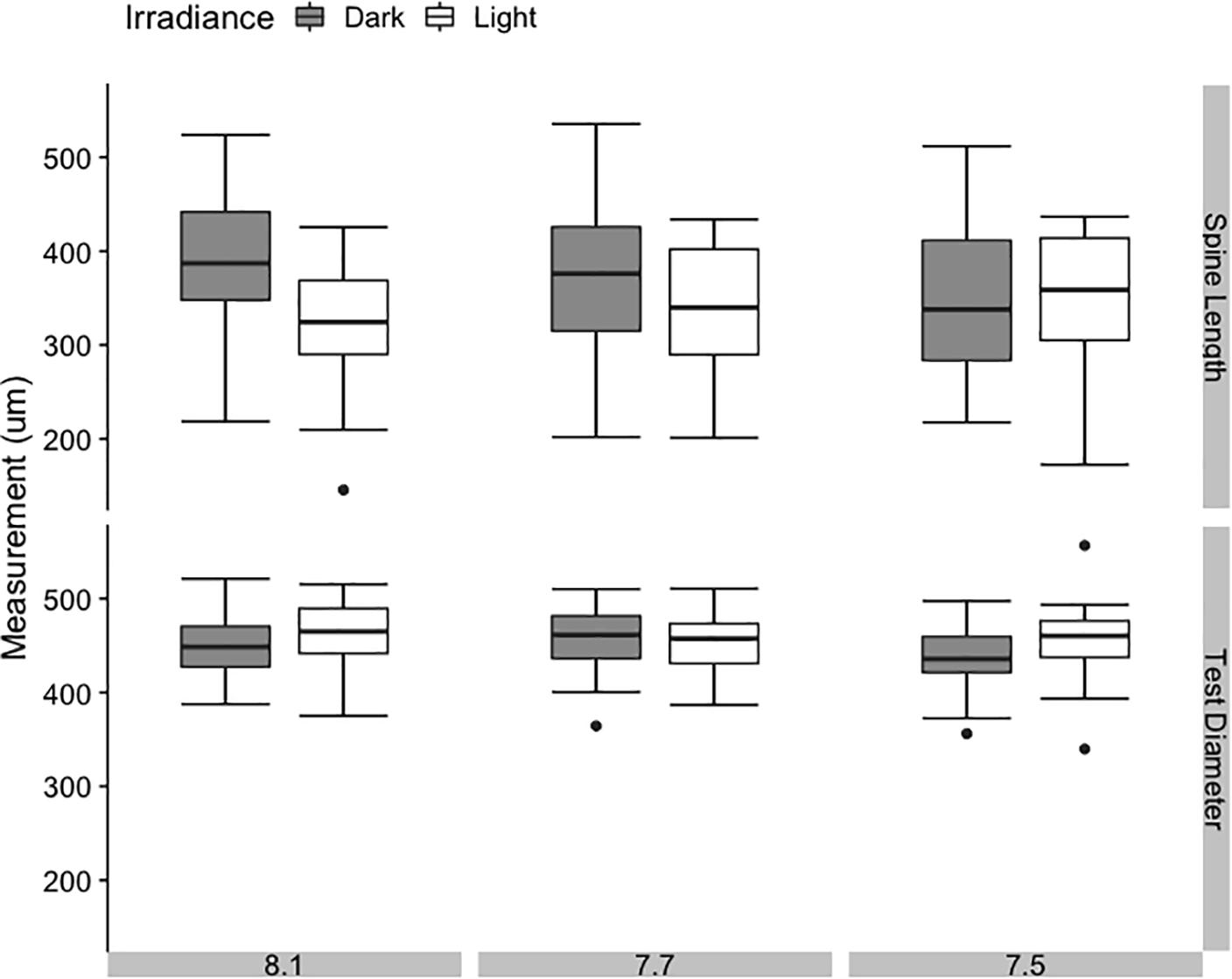
Figure 4. Average spine length and test diameter in juvenile sea urchins Pseudechinus huttoni at 4 days post-settlement under three bulk seawater pH and two irradiance treatments. Each treatment (3 L aquaria) was replicated three times. Error bars are ±1 SE of the mean.
Additionally, SEM revealed no observable differences in structural integrity or morphology of the sea urchin spines in any of the treatments (and thus at any levels of pHEXP; Figure 5, Supplementary Figure 6). This suggests, visually, that calcification was not impaired, nor was there any indication of degradation of calcified surfaces. Juvenile skeletal element morphology appeared similar in all treatments, showing no loss of structural integrity, while bearing smooth surfaces and no evidence of pitting or erosion on the primary and secondary spines. Evidence of fine calcification (i.e., secondary spination) was present along the edges of the juvenile spine. Additionally, stereom lattice structure was well developed in the spines and test.
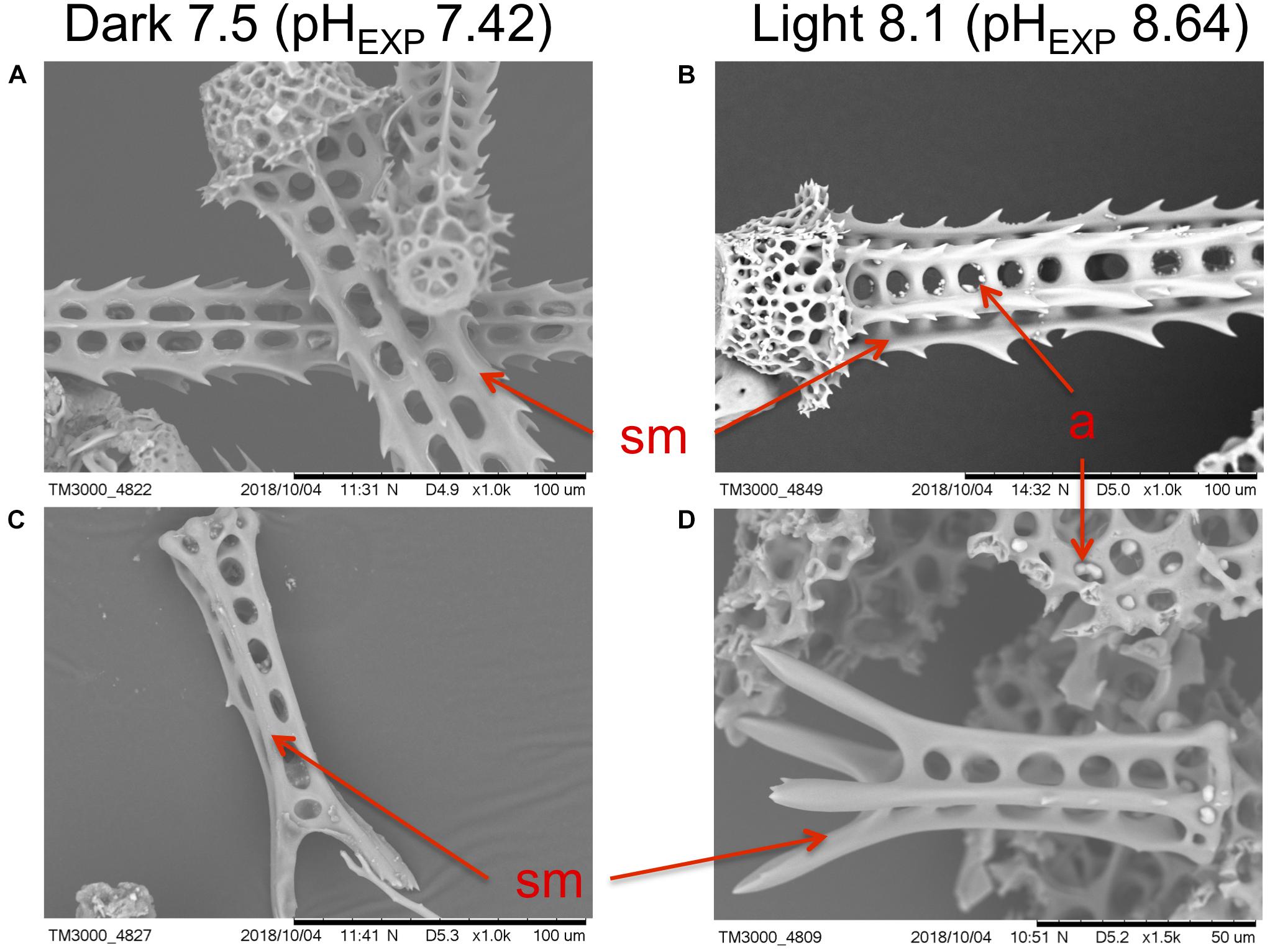
Figure 5. Examples of scanning electron micrographs of Pseudechinus huttoni juveniles reared in the dark at bulk seawater pH 7.5 (pHEXP 7.42) and in the light at bulk seawater pH 8.1 (pHEXP 8.64), for 4 days post-settlement. Scale bars are (A–C) 100 μm and (D) 50 μm, as indicated on SEM micrographs. Micrographs depict (A,B) base and attachment site of adult spines, and (C,D) juvenile spines. sm smooth surface. a artifact (a product of sample preparation for the SEM).
Discussion
Marine invertebrates that settle and live on algal surfaces experience pH levels that differ from the bulk seawater pH due to biologically driven changes within the DBL associated with irradiance and water flow. Our observations align with previous investigations showing that macroalgae modify the chemical environment immediately above their surface in the DBL (De Beer and Larkum, 2001; Hurd et al., 2011; Cornwall et al., 2013b; Hurd, 2015; Noisette and Hurd, 2018; Wahl et al., 2018; Comeau et al., 2019; Layton et al., 2019). In this respect, we focused on how the DBL could influence settlement success and early post-settlement growth in the sea urchin P. huttoni under contemporary and future OA conditions. It was hypothesized that reduced bulk seawater pH would negatively affect settlement success and post-settlement growth of sea urchins, but that thick macroalgal DBLs in the daytime (light), where pH is raised, would protect against these effects. If this occurred, we would observe higher settlement success under thick DBLs in the light, compared to the dark in our OA treatments. However, the observed settlement success and growth of newly settled sea urchins was relatively similar across all treatments, suggesting that P. huttoni juveniles are able to grow in a range of pH levels, even when pH is reduced in the DBL.
Settlement rates showed high variability among treatments, but settlement success was not significantly reduced at low pH levels in the DBL. Juveniles in our study were examined only 4 day post-settlement, so it is possible that the growth period was too short to reveal potential greater differences in skeletal morphology. Preliminary experiments yielded similar results of no differences among treatments, and our observations are also consistent with previous studies on sea urchins, where no direct effects of OA on larval settlement have been reported (Dupont et al., 2013; Wangensteen et al., 2013; Espinel-Velasco et al., 2020). The few significant differences on settlement in this study were primarily observed in the bulk pH 8.1 treatments, suggesting that high pH can reduce settlement success, as seen in Mos et al. (2020). While not tested, other environmental factors, that covaried with pH in our experiment, such as light, may also have significant effects on sea urchin settlement success (Rodriguez et al., 1993; Scheibling and Robinson, 2008). Additionally, in situ juveniles may also be able to undergo post-settlement transport or movement to improve the environmental conditions under which they first settled (Pilditch et al., 2015).
Early post-settlement growth also showed high variability among treatments, however, P. huttoni juveniles successfully grew in a range of pH levels within the DBL (pH 7.4 to 9.0). Thus, low pH levels in the DBL do not appear to impair growth. Our observations are consistent with those of previous studies on sea urchins, which have found that test diameter is robust to low pH, although spine length can be altered under extremely low pH values (7.4; Wolfe et al., 2013a). Our SEM observations revealed no apparent differences in structural details of the juvenile P. huttoni skeletons in any of the treatments. SEM micrographs have highlighted some of the greatest differences in calcification and supported other growth measurements in other sea urchin species, such as quantitative reductions in weight (Albright et al., 2012). Thus, our visual observations qualitatively suggest that growth was not substantially reduced at lower pH levels in the DBL.
This is one of the first studies to measure sea urchin settlement and early post-settlement development under DBLs of measured thicknesses. Previous work on the sea urchin Centrostephanus rodgersii accounting for the DBL, found that both low pH and high pH conditions have effects on settlement and post-settlement growth (Mos et al., 2020). Additionally, chemical buffering of reduced seawater pH has been found in the boundary layers above concrete surfaces (Mos et al., 2019). Our study examines a similar effect above macroalgal surfaces. Our results suggest that juvenile sea urchins may, to a certain extent, already be adapted to lower pH, with effects of low pH potentially only seen at extreme levels in the bulk seawater (pHT 7.4 and below; Byrne et al., 2009, 2017; Dupont et al., 2010; Byrne, 2011; Wolfe et al., 2013a; Espinel-Velasco et al., 2018). This has previously been seen in other marine taxa; for example, some oyster species have the potential to acclimatize to OA over multiple generations, where carryover effects mediate impacts of reduced seawater pH on later generations (Parker et al., 2011, 2015). Similarly, newly-settled sea urchins may be more tolerant to pH variability over temporal scales of hours/days because previous generations have already experienced significant natural pH variability, as has been suggested for other taxa in such environments (Melzner et al., 2009; Hurd et al., 2011; Wahl et al., 2015, 2018; Boyd et al., 2016; Noisette and Hurd, 2018). Indeed, organisms experiencing greater environmental variability are thought to possess higher phenotypic plasticity, and generally have greater resistance to mean changes in the environment due to global change (Boyd et al., 2016). Fluctuations in seawater carbonate chemistry in situ may mean that many taxa are already acclimatized or adapted to calcify in these extreme pH levels (Wolfe et al., 2013a; Eriander et al., 2015). Presently, there are no published in situ measurements of pH within the DBL of nearshore marine organisms, and the role of the DBL pH variability has largely gone underappreciated.
In addition to environmental history, fluctuations in pH during exposure to stressors will also likely modify responses to OA conditions (Rivest et al., 2017). Fluctuating environments may ameliorate negative effects of OA because either mean pH is higher (i.e., reductions at night being less than the increases during the day) or the fluctuations may yield periods of favorable pH conditions for organisms where they are able, for example, to calcify (Melzner et al., 2009; Cornwall et al., 2014; Wahl et al., 2015, 2018; Noisette and Hurd, 2018). Previous research has found that fluctuating pH treatments can elicit different effects on organisms than stable acidified conditions. For example, diurnal pH fluctuations increased the variance in growth of the barnacle Balanus improvisus, but not its mean growth (Eriander et al., 2015). The timing of exposure to different pH levels impacted larval development of the mussel Mytilus galloprovincialis, while larval shell growth was correlated with mean exposure regardless of variability (Kapsenberg et al., 2018). This may be another explanation for the lack of detectable results on effects of pH in the DBL on settlement and post-settlement growth in this study; our irradiance treatments were constant rather than on a diel cycle. Further research is needed to understand how natural small-scale temporal pH fluctuations will interact with bulk seawater pH decreases due to OA.
While juvenile sea urchins appeared robust to reduced pH in this study, it is also possible that a physiological limit may be surpassed when natural fluctuations are superimposed onto mean predictions of global change (Boyd et al., 2016), particularly in kelp beds with large diurnal pH changes (Cornwall et al., 2013a). Calcifying organisms living in these fluctuating environments already likely experience a degree of stress, and the combined effects of environmental fluctuations and global change related stressors (e.g., reduced seawater pH due to OA) may expose organisms to conditions beyond their thresholds of tolerance (Hofmann et al., 2011; Boyd et al., 2016). Effects of reduced pH may only become discernible at very extreme pH values below those used in this experiment (bulk seawater pH 7.5). Indeed, negative effects on skeleton growth (test diameter, spine number, and stereom pore size) and spine growth of juvenile H. erythrogramma were largely restricted to the lowest pH treatments used (pH 7.4 and below; Wolfe et al., 2013a, b).
The effects of OA on sea urchins are almost certainly life history specific, with effects on juveniles being variable and in some cases undetectable (Byrne et al., 2009, 2010b, 2017; Dupont et al., 2010; Byrne, 2011; Dupont and Thorndyke, 2013; Wolfe et al., 2013a; Chan et al., 2015; Espinel-Velasco et al., 2018). The negative effects of reduced seawater pH previously seen in larval P. huttoni (Clark et al., 2009) are not reflected in the responses of juveniles in this study. However, the larval urchins used in this study were reared in present-day conditions. Carry-over effects from the larval stage, such as energy reserves, may have allowed juveniles to grow successfully in varying pH conditions. There is a possibility for negative carryover effects from the juvenile stage to later life stages or future generations that this study did not explore (Karelitz et al., 2019). Thus, the larval rather than the juvenile stage may be a potential life-history bottleneck under OA conditions, as previously suggested (Byrne et al., 2013; Lamare et al., 2016). Life in the DBL may have pre-adapted juvenile P. huttoni to living and calcifying in a range of pH levels, thus conferring potential resilience to OA conditions at this life-history stage.
Data Availability Statement
The raw data supporting the conclusions of this article will be made available by the authors, without undue reservation.
Author Contributions
EH and ML conceived and designed the research, with input from NE-V for the settlement assay and CC for the boundary layer measurements. EH and NE-V carried out the experimentation. CP provided experimental resources for the boundary layer measurements in the annular flume. EH and NE-V analyzed the data. EH drafted the manuscript, with editorial input from all co-authors. All authors contributed to the article and approved the submitted version.
Funding
EH received funding from a Fulbright US Graduate Award. ML contributions were supported by CARIM (Coastal Acidification: Rate, Impacts and Management), funded by the New Zealand Ministry of Business, Innovation and Employment. CC was supported by a Rutherford Discovery Fellowship by the Royal Society of New Zealand Te Apârangi (RDF-VUW1701).
Conflict of Interest
The authors declare that the research was conducted in the absence of any commercial or financial relationships that could be construed as a potential conflict of interest.
Acknowledgments
Thanks to Dr. Kim Currie and Judith Murdoch at the National Institute of Water and Atmospheric Research (NIWA), New Zealand, for the dissolved inorganic carbon and total alkalinity measurements. Thank you to Anna Kluibenschedl at the University of Otago, New Zealand for the use of the CCA disks.
Supplementary Material
The Supplementary Material for this article can be found online at: https://www.frontiersin.org/articles/10.3389/fmars.2020.577562/full#supplementary-material
References
Albright, R., Bland, C., Gillette, P., Serafy, J. E., Langdon, C., and Capo, T. R. (2012). Juvenile growth of the tropical sea urchin Lytechinus variegatus exposed to near-future ocean acidification scenarios. J. Exp. Mar. Biol. Ecol. 426–427, 12–17. doi: 10.1016/j.jembe.2012.05.017
Boyd, P. W., Cornwall, C. E., Davison, A., Doney, S. C., Fourquez, M., Hurd, C. L., et al. (2016). Biological responses to environmental heterogeneity under future ocean conditions. Glob. Change Biol. 22, 2633–2650. doi: 10.1111/gcb.13287
Byrne, M. (2011). “Impact of ocean warming and ocean acidification on marine invertebrate life history stages: vulnerabilities and potential for persistence in a changing ocean,” in Oceanography and Marine Biology: An Annual Review, eds R. N. Gibson, R. Atkinson, J. Gordon, I. Smith, and D. Hughes (Boca Raton, FL: CRC Press), 1–42. doi: 10.1155/2011/473615
Byrne, M., Ho, M., Selvakumaraswamy, P., Nguyen, H. D., Dworjanyn, S. A., and Davis, A. R. (2009). Temperature, but not pH, compromises sea urchin fertilization and early development under near-future climate change scenarios. Proc. R. Soc. B Biol. Sci. 276, 1883–1888. doi: 10.1098/rspb.2008.1935
Byrne, M., Ho, M., Wong, E., Soars, N. A., Selvakumaraswamy, P., Shepard-Brennand, H., et al. (2010a). Unshelled abalone and corrupted urchins: development of marine calcifiers in a changing ocean. Proc. R. Soc. B Biol. Sci. 278, 2376–2383. doi: 10.1098/rspb.2010.2404
Byrne, M., Soars, N., Selvakumaraswamy, P., Dworjanyn, S. A., and Davis, A. R. (2010b). Sea urchin fertilization in a warm, acidified and high pCO2 ocean across a range of sperm densities. Mar. Environ. Res. 69, 234–239. doi: 10.1016/j.marenvres.2009.10.014
Byrne, M., Ho, M. A., Koleits, L., Price, C., King, C. K., Virtue, P., et al. (2013). Vulnerability of the calcifying larval stage of the Antarctic sea urchin Sterechinus neumayeri to near-future ocean acidification and warming. Glob. Change Biol. 19, 2264–2275. doi: 10.1111/gcb.12190
Byrne, M., Ross, P. M., Dworjanyn, S. A., and Parker, L. (2017). “Larval ecology in the face of changing climate–impacts of ocean warming and ocean acidification,” in Evolutionary Ecology of Marine Invertebrate Larvae, eds T. Carrier, A. Reitzel, and A. Heyland (Oxford: Oxford University Press), 251–272.
Caldeira, K., and Wickett, M. E. (2003). Anthropogenic carbon and ocean pH. Nature 425:365. doi: 10.1038/425365a
Chan, K. Y. K., García, E., and Dupont, S. (2015). Acidification reduced growth rate but not swimming speed of larval sea urchins. Sci. Rep. 5:9764. doi: 10.1038/srep09764
Chan, N. C. S., Wangpraseurt, D., Kühl, M., and Connolly, S. R. (2016). Flow and coral morphology control coral surface pH: implications for the effects of ocean acidification. Front. Mar. Sci. 3:10. doi: 10.3389/fmars.2016.00010
Clark, D., Lamare, M., and Barker, M. (2009). Response of sea urchin pluteus larvae (Echinodermata: Echinoidea) to reduced seawater pH: a comparison among a tropical, temperate, and a polar species. Mar. Biol. 156, 1125–1137. doi: 10.1007/s00227-009-1155-8
Comeau, S., Cornwall, C., Pupier, C., DeCarlo, T., Alessi, C., Trehern, R., et al. (2019). Flow-driven micro-scale pH variability affects the physiology of corals and coralline algae under ocean acidification. Sci. Rep. 9:12829.
Comeau, S., Cornwall, C. E., DeCarlo, T. M., Krieger, E., and McCulloch, M. T. (2018). Similar controls on calcification under ocean acidification across unrelated coral reef taxa. Glob. Change Biol. 24, 4857–4868. doi: 10.1111/gcb.14379
Cornwall, C. E. (2013). Macroalgae as Ecosystem Engineers and the Implications for Ocean Acidification. Ph.D. thesis, University of Otago, Dunedin.
Cornwall, C. E., Boyd, P. W., McGraw, C. M., Hepburn, C. D., Pilditch, C. A., Morris, J. N., et al. (2014). Diffusion boundary layers ameliorate the negative effects of ocean acidification on the temperate coralline macroalga Arthrocardia corymbosa. PLoS One 9:e97235. doi: 10.1371/journal.pone.0097235
Cornwall, C. E., Hepburn, C. D., McGraw, C. M., Currie, K. I., Pilditch, C. A., Hunter, K. A., et al. (2013a). Diurnal fluctuations in seawater pH influence the response of a calcifying macroalga to ocean acidification. Proc. R. Soc. B Biol. Sci. 280:20132201. doi: 10.1098/rspb.2013.2201
Cornwall, C. E., Hepburn, C. D., Pilditch, C. A., and Hurd, C. L. (2013b). Concentration boundary layers around complex assemblages of macroalgae: implications for the effects of ocean acidification on understory coralline algae. Limnol. Oceanogr. 58, 121–130. doi: 10.4319/lo.2013.58.1.0121
Cornwall, C. E., Pilditch, C. A., Hepburn, C. D., and Hurd, C. L. (2015). Canopy macroalgae influence understorey corallines’ metabolic control of near-surface pH and oxygen concentration. Mar. Ecol. Prog. Ser. 525, 81–95. doi: 10.3354/meps11190
De Beer, D., and Larkum, A. (2001). Photosynthesis and calcification in the calcifying algae Halimeda discoidea studied with microsensors. Plant Cell Environ. 24, 1209–1217. doi: 10.1046/j.1365-3040.2001.00772.x
Denny, M. W., and Wethey, D. S. (2000). “Physical processes that generate patterns in marine communities,” in Marine Community Ecology, eds M. Bertness, M. Hay, and S. Gaines (Sunderland, MA: Sinauer Associates), 3–37.
Dickson, A., and Millero, F. (1987). A comparison of the equilibrium constants for the dissociation of carbonic acid in seawater media. Deep Sea Res. A Oceanogr. Res. Pap. 34, 1733–1743. doi: 10.1016/0198-0149(87)90021-5
Dickson, A. G., Sabine, C. L., and Christian, J. R. (2007). Guide to Best Practices for Ocean CO2 Measurements. Sidney: North Pacific Marine Science Organization.
Dupont, S., Dorey, N., Stumpp, M., Melzner, F., and Thorndyke, M. (2013). Long-term and trans-life-cycle effects of exposure to ocean acidification in the green sea urchin Strongylocentrotus droebachiensis. Mar. Biol. 160, 1835–1843. doi: 10.1007/s00227-012-1921-x
Dupont, S., Ortega-Martínez, O., and Thorndyke, M. (2010). Impact of near-future ocean acidification on echinoderms. Ecotoxicology 19, 449–462. doi: 10.1007/s10646-010-0463-6
Dupont, S., and Thorndyke, M. (2013). “Direct impacts of near-future ocean acidification on sea urchins,” in Climate Change Perspective from the Atlantic: Past, Present and Future, eds J. M. Fernández-Palacios, L. de Nascimento, J. C. Hernández, S. Clemente, A. González, and J. P. Díaz-González (La Laguna: Universidad de La Laguna), 461–485.
Eriander, L., Wrange, A.-L., and Havenhand, J. (2015). Simulated diurnal pH fluctuations radically increase variance in—but not the mean of—growth in the barnacle Balanus improvisus. ICES J. Mar. Sci. 73, 596–603. doi: 10.1093/icesjms/fsv214
Espinel-Velasco, N., Agüera, A., and Lamare, M. (2020). Sea urchin larvae show resilience to ocean acidification at the time of settlement and metamorphosis. Mar. Environ. Res. 159:104977. doi: 10.1016/j.marenvres.2020.104977
Espinel-Velasco, N., Hoffmann, L., Agüera, A., Byrne, M., Dupont, S., Uthicke, S., et al. (2018). Effects of ocean acidification on the settlement and metamorphosis of marine invertebrate and fish larvae: a review. Mar. Ecol. Prog. Ser. 606, 237–257. doi: 10.3354/meps12754
Foster, T., and Gilmour, J. (2016). Seeing red: coral larvae are attracted to healthy-looking reefs. Mar. Ecol. Prog. Ser. 559, 65–71. doi: 10.3354/meps11902
García, E., Hernández, J. C., Clemente, S., Cohen-Rengifo, M., Hernández, C. A., and Dupont, S. (2015). Robustness of Paracentrotus lividus larval and post-larval development to pH levels projected for the turn of the century. Mar. Biol. 162, 2047–2055. doi: 10.1007/s00227-015-2731-8
Gosselin, P., and Jangoux, M. (1998). From competent larva to exotrophic juvenile: a morphofunctional study of the perimetamorphic period of Paracentrotus lividus (Echinodermata, Echinoida). Zoomorphology 118, 31–43. doi: 10.1007/s004350050054
Gosselin, L. A., and Qian, P. Y. (1997). Juvenile mortality in benthic marine invertebrates. Mar. Ecol. Prog. Ser. 146, 265–282. doi: 10.3354/meps146265
Hendriks, I. E., Duarte, C. M., and Álvarez, M. (2010). Vulnerability of marine biodiversity to ocean acidification: a meta-analysis. Estuar. Coast. Shelf Sci. 86, 157–164. doi: 10.1016/j.ecss.2009.11.022
Hofmann, G. E., Smith, J. E., Johnson, K. S., Send, U., Levin, L. A., Micheli, F., et al. (2011). High-frequency dynamics of ocean pH: a multi-ecosystem comparison. PLoS One 6:e28983. doi: 10.1371/journal.pone.0028983
Hurd, C. L. (2015). Slow-flow habitats as refugia for coastal calcifiers from ocean acidification. J. Phycol. 51, 599–605. doi: 10.1111/jpy.12307
Hurd, C. L., Cornwall, C. E., Currie, K., Hepburn, C. D., McGraw, C. M., Hunter, K. A., et al. (2011). Metabolically induced pH fluctuations by some coastal calcifiers exceed projected 22nd century ocean acidification: a mechanism for differential susceptibility? Glob. Change Biol. 17, 3254–3262. doi: 10.1111/j.1365-2486.2011.02473.x
Kapsenberg, L., Miglioli, A., Bitter, M. C., Tambutté, E., Dumollard, R., and Gattuso, J.-P. (2018). Ocean pH fluctuations affect mussel larvae at key developmental transitions. Proc. R. Soc. B Biol. Sci. 285:20182381. doi: 10.1098/rspb.2018.2381
Karelitz, S., Lamare, M. D., Mos, B., De Bari, H., Dworjanyn, S. A., and Byrne, M. (2019). Impact of growing up in a warmer, lower pH future on offspring performance: transgenerational plasticity in a pan-tropical sea urchin. Coral Reefs 38, 1085–1095. doi: 10.1007/s00338-019-01855-z
Kirby, S., Lamare, M. D., and Barker, M. F. (2006). Growth and morphometrics in the New Zealand sea urchin Pseudechinus huttoni (Echinoidea: Temnopleuridae). N. Z. J. Mar. Freshwater Res. 40, 413–428. doi: 10.1080/00288330.2006.9517432
Koehl, M. A. R., and Hadfield, M. G. (2010). Hydrodynamics of larval settlement from a larva’s point of view. Integr. Comp. Biol. 50, 539–551. doi: 10.1093/icb/icq101
Kroeker, K. J., Kordas, R. L., Crim, R., Hendriks, I. E., Ramajo, L., Singh, G. S., et al. (2013). Impacts of ocean acidification on marine organisms: quantifying sensitivities and interaction with warming. Glob. Change Biol. 19, 1884–1896. doi: 10.1111/gcb.12179
Lamare, M., and Barker, M. (2001). Settlement and recruitment of the New Zealand sea urchin Evechinus chloroticus. Mar. Ecol. Prog. Ser. 218, 153–166. doi: 10.3354/meps218153
Lamare, M. D., Liddy, M., and Uthicke, S. (2016). In situ developmental responses of tropical sea urchin larvae to ocean acidification conditions at naturally elevated pCO2 vent sites. Proc. R. Soc. B Biol. Sci. 283:20161506. doi: 10.1098/rspb.2016.1506
Layton, C., Cameron, M. J., Shelamoff, V., Fernández, P. A., Britton, D., Hurd, C. L., et al. (2019). Chemical microenvironments within macroalgal assemblages: implications for the inhibition of kelp recruitment by turf algae. Limnol. Oceanogr. 64, 1600–1613. doi: 10.1002/lno.11138
Lichtenberg, M., Nørregaard, R. D., and Kühl, M. (2017). Diffusion or advection? Mass transfer and complex boundary layer landscapes of the brown alga Fucus vesiculosus. J. R. Soc. Interface 14:20161015. doi: 10.1098/rsif.2016.1015
McClary, D. J., and Sewell, M. A. (2003). Hybridization in the sea: gametic and developmental constraints on fertilization in sympatric species of Pseudechinus (Echinodermata: Echinoidea). J. Exp. Mar. Biol. Ecol. 284, 51–70. doi: 10.1016/s0022-0981(02)00487-2
McCoy, S. J., and Kamenos, N. A. (2015). Coralline algae (Rhodophyta) in a changing world: integrating ecological, physiological, and geochemical responses to global change. J. Phycol. 51, 6–24. doi: 10.1111/jpy.12262
Mehrbach, C., Culberson, C. H., Hawley, J. E., and Pytkowicz, R. M. (1973). Constants of Carbonic Acid in Seawater at Atmospheric Pressure. Corvallis, OR: Oregon State University.
Melzner, F., Gutowska, M., Langenbuch, M., Dupont, S., Lucassen, M., Thorndyke, M., et al. (2009). Physiological basis for high CO2 tolerance in marine ectothermic animals: pre-adaptation through lifestyle and ontogeny? Biogeosciences 6, 2313–2331. doi: 10.5194/bg-6-2313-2009
Mos, B., Byrne, M., and Dworjanyn, S. A. (2020). Effects of low and high pH on settlement and post-settlement growth of a sea urchin in culture. Aquaculture 528:735618. doi: 10.1016/j.aquaculture.2020.735618
Mos, B., Dworjanyn, S. A., Mamo, L. T., and Kelaher, B. P. (2019). Building global change resilience: concrete has the potential to ameliorate the negative effects of climate-driven ocean change on a newly-settled calcifying invertebrate. Sci. Total Environ. 646, 1349–1358. doi: 10.1016/j.scitotenv.2018.07.379
Mosley, L., Peake, B., and Hunter, K. (2010). Modelling of pH and inorganic carbon speciation in estuaries using the composition of the river and seawater end members. Environ. Model. Softw. 25, 1658–1663. doi: 10.1016/j.envsoft.2010.06.014
Nelson, K. S. (2016). Biofilm Response to Ocean Acidification and the Effects on Serpulid Polychaete Settlement. Masters of Science thesis, University of Otago, Dunedin.
Noisette, F., and Hurd, C. (2018). Abiotic and biotic interactions in the diffusive boundary layer of kelp blades create a potential refuge from ocean acidification. Funct. Ecol. 32, 1329–1342. doi: 10.1111/1365-2435.13067
Parker, L. M., O’Connor, W. A., Raftos, D. A., Pörtner, H.-O., and Ross, P. M. (2015). Persistence of positive carryover effects in the oyster, Saccostrea glomerata, following transgenerational exposure to ocean acidification. PLoS One 10:e0132276. doi: 10.1371/journal.pone.0132276
Parker, L. M., Ross, P. M., and O’Connor, W. A. (2011). Populations of the Sydney rock oyster, Saccostrea glomerata, vary in response to ocean acidification. Mar. Biol. 158, 689–697. doi: 10.1007/s00227-010-1592-4
Pilditch, C. A., Valanko, S., Norkko, J., and Norkko, A. (2015). Post-settlement dispersal: the neglected link in maintenance of soft-sediment biodiversity. Biol. Lett. 11:20140795. doi: 10.1098/rsbl.2014.0795
Pöhn, M., Vopel, K., Grünberger, E., and Ott, J. (2001). Microclimate of the brown alga Feldmannia caespitula interstitium under zero-flow conditions. Mar. Ecol. Prog. Ser. 210, 285–290. doi: 10.3354/meps210285
Przeslawski, R., Byrne, M., and Mellin, C. (2015). A review and meta-analysis of the effects of multiple abiotic stressors on marine embryos and larvae. Glob. Change Biol. 21, 2122–2140. doi: 10.1111/gcb.12833
Rahman, M. A., Yusoff, F. M., Arshad, A., Shamsudin, M. N., and Amin, S. (2012). Embryonic, larval, and early juvenile development of the tropical sea urchin, Salmacis sphaeroides (Echinodermata: Echinoidea). Sci. World J. 2012:938482.
Rivest, E. B., Comeau, S., and Cornwall, C. E. (2017). The role of natural variability in shaping the response of coral reef organisms to climate change. Curr. Clim. Change Rep. 3, 271–281. doi: 10.1007/s40641-017-0082-x
Roberts, R. (2001). A review of settlement cues for larval abalone (Haliotis spp.). J. Shellfish Res. 20, 571–586.
Roberts, R. D., Barker, M. F., and Mladenov, P. (2010). Is settlement of Haliotis iris larvae on coralline algae triggered by the alga or its surface biofilm? J. Shellfish Res. 29, 671–678. doi: 10.2983/035.029.0317
Rodriguez, S. R., Ojeda, F. P., and Inestrosa, N. C. (1993). Settlement of benthic marine invertebrates. Mar. Ecol. Prog. Ser. 97, 193–207. doi: 10.3354/meps097193
Scheibling, R. E., and Robinson, M. C. (2008). Settlement behaviour and early post-settlement predation of the sea urchin Strongylocentrotus droebachiensis. J. Exp. Mar. Biol. Ecol. 365, 59–66. doi: 10.1016/j.jembe.2008.07.041
Shaw, E. C., McNeil, B. I., and Tilbrook, B. (2012). Impacts of ocean acidification in naturally variable coral reef flat ecosystems. J. Geophys. Res. Oceans 117:C03038.
Shears, N. T., and Babock, R. C. (2007). Quantitative description of mainland New Zealand’s shallow subtidal reef communities. Sci. Conserv. 280, 5–14.
Uthicke, S., Pecorino, D., Albright, R., Negri, A. P., Cantin, N., Liddy, M., et al. (2013). Impacts of ocean acidification on early life-history stages and settlement of the coral-eating sea star Acanthaster planci. PLoS One 8:e82938. doi: 10.1371/journal.pone.0082938
Wahl, M., Covachã, S. S., Saderne, V., Hiebenthal, C., Müller, J. D., Pansch, C., et al. (2018). Macroalgae may mitigate ocean acidification effects on mussel calcification by increasing pH and its fluctuations. Limnol. Oceanogr. 63, 3–21. doi: 10.1002/lno.10608
Wahl, M., Saderne, V., and Sawall, Y. (2015). How good are we at assessing the impact of ocean acidification in coastal systems? Limitations, omissions and strengths of commonly used experimental approaches with special emphasis on the neglected role of fluctuations. Mar. Freshw. Res. 67, 25–36. doi: 10.1071/MF14154
Wangensteen, O. S., Dupont, S., Casties, I., Turon, X., and Palacín, C. (2013). Some like it hot: temperature and pH modulate larval development and settlement of the sea urchin Arbacia lixula. J. Exp. Mar. Biol. Ecol. 449, 304–311. doi: 10.1016/j.jembe.2013.10.007
Wolfe, K., Dworjanyn, S. A., and Byrne, M. (2013a). Effects of ocean warming and acidification on survival, growth and skeletal development in the early benthic juvenile sea urchin (Heliocidaris erythrogramma). Glob. Change Biol. 19, 2698–2707. doi: 10.1111/gcb.12249
Keywords: Pseudechinus huttoni, macroalgae, seawater pH, settlement substrates, early post-settlement, juveniles
Citation: Houlihan EP, Espinel-Velasco N, Cornwall CE, Pilditch CA and Lamare MD (2020) Diffusive Boundary Layers and Ocean Acidification: Implications for Sea Urchin Settlement and Growth. Front. Mar. Sci. 7:577562. doi: 10.3389/fmars.2020.577562
Received: 29 June 2020; Accepted: 22 October 2020;
Published: 13 November 2020.
Edited by:
Iris Eline Hendriks, University of the Balearic Islands, SpainReviewed by:
Benjamin Mos, Southern Cross University, AustraliaNarimane Dorey, UMR 8539 Laboratoire de météorologie dynamique (LMD), France
Copyright © 2020 Houlihan, Espinel-Velasco, Cornwall, Pilditch and Lamare. This is an open-access article distributed under the terms of the Creative Commons Attribution License (CC BY). The use, distribution or reproduction in other forums is permitted, provided the original author(s) and the copyright owner(s) are credited and that the original publication in this journal is cited, in accordance with accepted academic practice. No use, distribution or reproduction is permitted which does not comply with these terms.
*Correspondence: Erin P. Houlihan, erinphoulihan@gmail.com