- 1The Lyell Centre for Earth and Marine Science and Technology, Heriot-Watt University, Edinburgh, United Kingdom
- 2Royal Botanic Garden Edinburgh, Edinburgh, United Kingdom
- 3Institute of Life and Earth Sciences, Heriot-Watt University, Edinburgh, United Kingdom
- 4Scottish Natural Heritage, Great Glen House, Inverness, United Kingdom
- 5School of Biology, Faculty of Science, University of St Andrews, St Andrews, United Kingdom
Anthropogenic climate change presents a major challenge to coastal ecosystems. Mass population declines or geographic shifts in species ranges are expected to occur, potentially leading to wide-scale ecosystem disruption or collapse. This is particularly important for habitat-forming species such as free-living non-geniculate coralline algae that aggregate to form large, structurally complex reef-life ecosystems with high associated biodiversity and carbon sequestration capability. Coralline algal beds have a worldwide distribution, but have recently experienced global declines due to anthropogenic pressures and changing environmental conditions. However, the environmental factors controlling coralline algal bed distribution remain poorly understood, limiting our ability to make adequate assessments of how populations may change in the future. We constructed the first species distribution model for non-geniculate coralline algae (focusing on maerl-forming species but including crustose coralline algae associated with coralline algal beds) and showed that bathymetry, temperature at the seabed and light availability are the primary environmental drivers of present-day non-geniculate coralline algae distribution. Our model also identifies suitable areas for species presence that currently lack records of occurrence. Large-scale spatial declines in coralline algal distribution were observed under all IPCC Representative Concentration Pathways (ranging from 38% decline under RCP 2.6 up to 84% decline under RCP 8.5), with the most rapid rate of decline up to 2050. Refuge populations that may persist under projected climate change were also identified – informing priority areas for future conservation efforts to maximize the long-term survival of this globally important ecosystem.
Introduction
Rising anthropogenic carbon dioxide (CO2) emissions are causing rapid environmental change and under some scenarios, the mean ocean surface temperature is projected to rise by up to 4.3°C by 2100 (IPCC, 2014). Geographic shifts or declines in the distribution of species and ecosystems in response to this rapid environmental change have already been observed (e.g., Bellard et al., 2012; Gallon et al., 2014; Martínez et al., 2018). This can result in significant ecosystem disruption (Hoegh-Guldberg et al., 2007; Noisette et al., 2013a; Teagle and Smale, 2018), threatening the long-term survival of key ecosystem components and the associated biodiversity and ecosystem services they provide.
Free-living non-geniculate red coralline algae are globally distributed ecosystem engineers (Foster, 2001). They give rise to three-dimensional marine habitats, and these so-called “maerl” or “rhodolith” beds have high associated biodiversity (Barbera et al., 2003; Peña et al., 2014) – supporting many rare, endemic or commercially important species (Grall and Hall-Spencer, 2003; Wilson et al., 2004; Nelson, 2009; Riosmena-Rodriguez et al., 2016) – and globally significant roles in biogeochemical cycling, particularly long-term carbon burial (Burdett et al., 2015; van der Heijden and Kamenos, 2015; Mao et al., 2020). Aggregations of free-living, non-geniculate coralline algae (maerl-beds), although found globally, are particularly abundant in the North-East Atlantic (from the Arctic Ocean to the wider North Atlantic and the Canary Islands) (Pardo et al., 2014a), with Galicia, Brittany, Norway, Ireland, and Scotland being particular strongholds (Hall-Spencer et al., 2010). In this North-East Atlantic region, beds most abundant up to a depth of ∼50 m (Pardo et al., 2014a). There are thought to be around 24 maerl-forming species found throughout the North-East Atlantic but the most common are Phymatolithon calcareum, Lithothamnion coralloides, Lithothamnion glaciale, and Lithothamnion tophiforme (Pardo et al., 2014a). These species appear to follow a thermal cline, with L. tophiforme being limited to Arctic waters, L. glaciale occurring in Arctic and sub-Arctic areas, P. calcareum stretching from the sub-Arctic to tropical waters and L. coralloides occurring from the Southern British Isles to the Canary Islands and throughout the Mediterranean (Hall-Spencer et al., 2010; Pardo et al., 2014a). However, there are uncertainties regarding species identification and hence species’ ranges, as morphological identification is difficult and few widespread molecular studies have been carried out to-date. Poor species identification additionally makes identifying species-specific environmental requirements challenging. Current evidence suggests that L. glaciale mostly occurs between 6–50 m (Perry et al., 2017a; Schoenrock et al., 2018) while L. coralloides appears to be more restricted to shallower depths, rarely being recorded below 30 m (Perry et al., 2017a). P. calcareum is also more frequently recorded in shallower waters (above 30 m) (Perry et al., 2017a), but sporadic deep-water observations are known [e.g., 80 m in the Pontian Archipelago, Mediterranean (Basso, 1998)].
Currently, all coralline algae beds are listed as “Vulnerable” or “Endangered” on the IUCN Red List (Gubbay et al., 2016). In Europe they are protected under the EU Habitats Directive and the OSPAR Commission in the North Atlantic (under “OSPAR list of Threatened and/or Declining habitats”) (Hall-Spencer et al., 2008; European Commission, 2018), supported by local implementation such as designation as Priority Marine Features in Scotland (Scottish Government, 2018). Seven marine features were included in the EU Species and Habitats Directive some of which encompassed habitats where coralline algal beds occur (e.g., “Large shallow inlets and bays” and “Sandbanks which are slightly covered by seawater at all times”) which are listed under Annex I. Two maerl-forming species (L. coralloides and P. calcareum) are additionally listed under Annex V (The Council of the European Communities, 1992; European Commission, 2016; Riosmena-Rodriguez et al., 2016; Perry et al., 2017b). Although the Habitats Directive has since been superseded into national law, it is the starting point from which most non-geniculate coralline algal bed conservation and later European legislation [e.g., the United Kingdom Biodiversity Action Plan (JNCC, 2016)] has evolved.
Habitat-forming ecosystem engineers, such as free-living non-geniculate coralline algae, modify their environment in such a way that benefits other species, for example, through the provision of shelter and food, or by the reduction of environmental stress (Hastings et al., 2007; Peña et al., 2014; Sheehan et al., 2015; Martínez et al., 2018). These ecosystems, therefore, are of high conservation concern and understanding how they may react to changing environmental conditions is important as their loss or geographic shift could pose threats to whole systems (Martínez et al., 2018; Teagle and Smale, 2018; Cornwall et al., 2019). Projected changes in marine environmental conditions, particularly ocean warming (Martin and Gattuso, 2009; Noisette et al., 2013a; Qui-Minet et al., 2019) and acidification (Porzio et al., 2011; Noisette et al., 2013b; Legrand et al., 2017; Martin and Hall-Spencer, 2017), are expected to have significant impacts on non-geniculate coralline algal distribution (Brodie et al., 2014). This is due to the consequential negative impacts on species-specific thermal niches, reduced calcification (Burdett et al., 2012a, 2018; Kamenos et al., 2013; Qui-Minet et al., 2019) and potential overgrowth by opportunistic non-calcifying algae, as has been observed during coral reef degradation (Hughes et al., 2007).
Non-geniculate coralline algal beds form biogenic reefs, which provide a myriad of ecosystem services globally that are often overlooked and their potential underestimated (Bindoff et al., 2019). These bed systems are biodiversity hotspots forming a living layer over sediments that provides habitat and supports high numbers of species including many invertebrate phyla (crustaceans, echinoderms, polychaetes, and mollusks), fleshy macroalgae, and various species which burrow either into individual free-living algae or the sediments below the bed (Jackson et al., 2004; Hall-Spencer et al., 2008, 2010; Foster et al., 2013). When compared to adjacent habitat types it was found that coralline algal beds had nearly twice the species richness (Steller et al., 2003). Similarly, non-geniculate coralline algal beds support numerous commercial fish species, often forming nursery habitats for economically important species such as cod (Gadus morhua) and hake (Melanogrammus aeglefinus) (Kamenos et al., 2004; Hall-Spencer et al., 2006; Riosmena-Rodriguez et al., 2016; Elliott et al., 2017). However, despite their importance there is still a paucity of knowledge surrounding these ecosystems and how a rapidly changing climate will affect them (Bindoff et al., 2019).
Species distribution models (SDMs) or environmental niche models (ENMs) are commonly used tools to estimate the relationships between species and environmental or spatial factors (Elith et al., 2011; Merow et al., 2013). SDMs represent an important addition to direct environmental change experiments on non-geniculate coralline algae and their associated habitats which are difficult to extrapolate to range-wide predictions (Wernberg et al., 2012), and do not take into account the spatial variability of projected changes in environmental conditions. SDMs are capable of modeling both small-scale local responses and range-wide changes, provided that datasets are available at a suitable scale.
Here, we constructed the first SDM for coralline algal beds, focusing on Scottish regional waters – a European stronghold for this habitat (Hall-Spencer et al., 2008) and of conservation priority at national and international scales (Hall-Spencer et al., 2008; JNCC, 2016; European Commission, 2018; Scottish Government, 2018). This enabled us to identify: (1) the primary environmental drivers of present-day coralline algal bed distribution, (2) previously unknown areas of occurrence, (3) regional-scale impacts of projected climate change on coralline algal bed distribution, and (4) refuge populations tolerant to projected climate change. Synthesizing this information allowed us to identify areas of priority conservation effort to maximize the likelihood of long-term survival of this globally important marine habitat.
Materials and Methods
Study Area
Scotland is a European stronghold for non-geniculate coralline algae beds. Consequently, it was selected as the focus area for distribution modeling in this study. The study area runs from the Solway Firth in the southeast, to the northern coasts of the Shetland Isles, including the Outer Hebrides in the west (Supplementary Figure S1). Within this region “maerl beds” (i.e., aggregations of non-geniculate coralline algae) are Priority Marine Features and protected under Scottish legislation (Scottish Government, 2018), ten marine protected areas around the Scottish coastline are designated for the protection of coralline algal beds (referred to in legislation as “maerl beds”). Despite this, the true extent of these systems in Scottish waters remains unknown, and even less is known about the factors that govern their local distribution.
Species Distribution Model
Maximum entropy (MaxEnt) modeling was chosen to construct a species distribution model (SDM) for maerl-forming species (i.e., non-geniculate coralline algae) as it is effective with presence-only data, is well regarded in the literature and can be highly modified so all modeling decisions are based on biological justifications (Pearson, 2010; Elith et al., 2011; Merow et al., 2013). MaxEnt v3.4.1 (Phillips et al., 2006) was run through the R v 3.6.1 (R Core Team, 2019) package DISMO v1.4 (Hijmans, 2017) to model the species niche and construct a probabilistic distribution map of non-geniculate coralline algal beds around Scotland.
Non-geniculate Coralline Algae
Occurrence Records
Occurrence records for the study region were obtained from the Geodatabase of Marine Features in Scotland (GeMS) (Scottish Natural Heritage, 2019a) under the Open Government and Creative Commons License. Geographic locations and study area are provided in Supplementary Figure S1. The annually updated database includes observed, georeferenced occurrence records of Priority Marine Features in Scotland from 1970 to 2017 curated by Scottish Natural Heritage (SNH). “Maerl beds” are one of these features. For this study, cut-offs were imposed on occurrence data by removing entries from before 1990. This was done to increase reliability, as older records may have been subject to different environmental conditions than recent records – for example due to construction of causeways changing tidal patterns [known to have happened in several locations, for example the Vatersay causeway (1989) (Comhairle nan Eilean Siar, 2020b) or the Benbecula causeway (1983) (Comhairle nan Eilean Siar, 2020a)]. Similarly, where there was evidence of a bed no longer supporting live individuals the occurrence point was removed prior to analysis.
Keyword searches in the GeMS database for “maerl” yielded species-specific records of free-living coralline algae species (i.e., “L. glaciale” or “P. calcareum”) and records with no species identification (“maerl beds”). All records pertaining to aggregations of free-living non-geniculate coralline algae are labeled as “maerl bed” in GeMS and therefore no other search terms were needed. Within the study region species ranges overlap and species identification in coralline algae without molecular analyses can be difficult and unreliable (Pardo et al., 2014a,b). In order to maintain the maximum number of results for the model, no attempt was therefore made to split these records up into species-specific groups, however; it is assumed that the model will target the two main species in this region P. calcareum and L. glaciale. The database searching yielded 1430 occurrence records.
Although all records in the GeMS database are of free-living, non-geniculate coralline algae, not enough is known about the differentiation between this and crustose coralline algae (CCA) to conclusively say the model will only predict the distribution of free-living non-geniculate coralline algae, especially when considering free-living specimens that are grown around a non-algal core.
Observed occurrence records within the database which were not the result of a targeted survey, e.g., SeaSearch data1, have inherent amounts of observer and spatial bias (Veloz, 2009; Fithian et al., 2015). Similarly, some beds have multiple (but geographically close) location points, creating further spatial bias (Veloz, 2009). To eliminate the subsequent spatial-autocorrelation a 2-dimensional kernel density estimation of the data was created in R (R Core Team, 2019) using the package “spatstat” v1.61-0 (Baddeley et al., 2019). This was then used in the Java application OccurenceThinner (Verbruggen et al., 2013) to reduce geographical sampling bias leaving 314 occurrence points in the model.
Environmental Variables
Environmental layers were accessed from bio-ORACLE v2 (Tyberghein et al., 2012). The datasets have a spatial resolution of five arc minutes (Tyberghein et al., 2012; Assis et al., 2018). Bathymetry data were accessed through Bio-ORACLE from MARSPEC (Assis et al., 2018) and masked to the same resolution as the Bio-ORACLE datasets (Assis et al., 2018).
Environmental variables were selected for their potential links to coralline algae distribution. Initially 21 datasets were considered (Supplementary Table S1). Variables were examined for collinearity which may interfere with the performance of the model (Júnior and Nóbrega, 2018). This was done by calculating the Pearson correlation coefficient (r) between the datasets; datasets were considered collinear when −0.6 ≤ r ≥ 0.6 (Assis et al., 2018; Júnior and Nóbrega, 2018). A high degree of collinearity was observed between the 16 available variables. Where inclusion of maximum and minimum datasets created collinearity with another dataset, the mean datasets were used instead. Following analysis for collinearity, the most ecologically significant datasets were selected, according to literature evidence (e.g., Dutertre et al., 2014). These were: bathymetry (m), pH, mean diffuse attenuation coefficient (DA) (m–1), mean dissolved nitrate concentration (μmol L–1), minimum benthic temperature (°C) (i.e., average minimum temperature recorded at average seabed depth across the coastal shelf ranging from 0 to 200 m depth), mean photo-active radiation (PAR) at seabed (E m–2 year–1), mean seabed salinity (PSS), maximum current velocity at seabed (m s–1) and minimum current velocity at seabed (m s–1).
The modeled benthic datasets used here contain some inherent collinearity, however, this is negligible in the Bio-ORACLE datasets as in situ quality control has been carried out by the Global Ocean Data Analyses Project (Assis et al., 2018) and high agreement found between the interpolated benthic datasets and in situ data gathered (Assis et al., 2018). For pH and DA, benthic datasets were not available, so sea surface datasets were used. Since these may not accurately represent benthic conditions (where non-geniculate coralline algal beds are found), test models were run with and without these datasets and the probabilistic distribution maps produced compared against known occurrence data. The results of these test models showed that the overall models were more statistically robust with the inclusion of surface datasets. pH was consistently found to be the variable that contributed fourth highest to the model (10.8% of the observed distribution) and is known to be environmentally relevant to non-geniculate coralline algal survival (McCoy and Kamenos, 2015), and so warranted inclusion in the model. Additionally, when run without pH and DA, overfitting (Pearson et al., 2007; Muscarella et al., 2014) was observed [Area Under Curve (AUC) with pH and DA = 0.0757; without pH and DA = 0.108] (Supplementary Table S3). Average omission rates for the minimum training presence were higher for models without pH and DA compared to those with them (0.310 and 0.263, respectively) thus also suggesting models were more over fit without pH and DA (Pearson et al., 2007; Muscarella et al., 2014) (Supplementary Table S3). Model evaluation was conducted in the R package ENMeval v0.3.0 (Muscarella et al., 2018). Model performance was assessed based on standard statistical assessments including AUC, receiver operating characteristics (ROC) and Akaike Information Criterion (AIC) (Muscarella et al., 2014; Steen, 2019).
Spatial Distribution Model Settings
ENMeval was further used to select model parameters that enabled the most efficient model performance (Supplementary Material). The optimal settings were found to be a regularization multiplier of one, and all feature classes. Models were clamped to training conditions to avoid extrapolation uncertainty. Once these settings were established the model was run in the R package Dismo v1.4 (Hijmans, 2017). The contribution of each environmental variable was assessed by excluding it from the model and assessing the performance of the model without it (jack-knifing). Forty per cent of the occurrence data points were randomly held back for model calibration and the model was trained on the remaining 60% of the occurrence data. The resultant model performed well under cross validation and evaluation statistics (Supplementary Table S3). In the final model, the AUC value was 0.959 ± 0.008 (mean ± SD), indicating high predictive ability. Some areas of predicted distribution were not covered by presence records. Field validation of two positive prediction sites were found to be true (Daliburgh, South Uist [57.160913, −7.408005] and Kildonan [57.218810, −7.427824]) and one modeled “absence” site was confirmed to not have any free-living coralline algae or CCA present (Loch Eyenort, South Uist [around 57.230275, −7.3514]), providing further evidence of model reliability.
Threshold Selection
Implementation of thresholds was carried out in R to transform logarithmic model predictions of probability of presence of non-geniculate coralline algae to binary presences and absences (Supplementary Material).
Climate Projections
Climate projection datasets were sourced from Bio-ORACLE for Representative Concentration Pathway (RCP) scenarios 2.6, 4.5, 6.0, and 8.5 for the years 2050 and 2100 (Assis et al., 2018). These are all based on the IPCC Coupled Model Intercomparison Project (CMIP) 5 model for climate scenarios, ranging from a significant reduction in greenhouse gas emissions (RCP 2.6) to a continuation at the current level (RCP 8.5) (Assis et al., 2018). Where available, the environmental variables used to train the MaxEnt SDM were replaced with the projected future levels – this was possible for benthic temperature, salinity and current velocity (Assis et al., 2018) (Supplementary Table S2). Projection datasets for other environmental variables (pH, diffuse attenuation, nitrate concentration, photoactive radiation at seabed and bathymetry), were not available, so present-day datasets were used.
Results
Present Day Coralline Algal Distribution
The top three primary environmental drivers (total explanation = 85%) of non-geniculate coralline algal bed distribution were bathymetry (51.8%), minimum benthic temperature (17.4%) and PAR at the seabed (15.8%). Sea surface pH accounted for an additional 10.8% of the association between environmental variables and non-geniculate coralline algal distribution. Coralline algal beds were predicted from the intertidal, down to 40 m depth, within a minimum temperature range of 2–9°C, a PAR range of 0–25 (E m–2 yr–1) and a pH range of 8.16–8.21. Predicted non-geniculate coralline algae habitat was spatially heterogeneous, characterized by a general east/west divide. The most suitable areas for non-geniculate coralline algal habitats were predominantly located in the coastal waters and sea lochs of the west coast of mainland Scotland, around Orkney, and Shetland (north of mainland Scotland) and around the southern islands of the Outer Hebrides (Figure 1). The east coast of Scotland is predominantly devoid of suitable areas for non-geniculate coralline algal habitats, except for the Moray Firth on the northeast mainland (Figure 1). The total area suitable for non-geniculate coralline algal bed presence during present-day conditions was predicted to be 7130 km2.
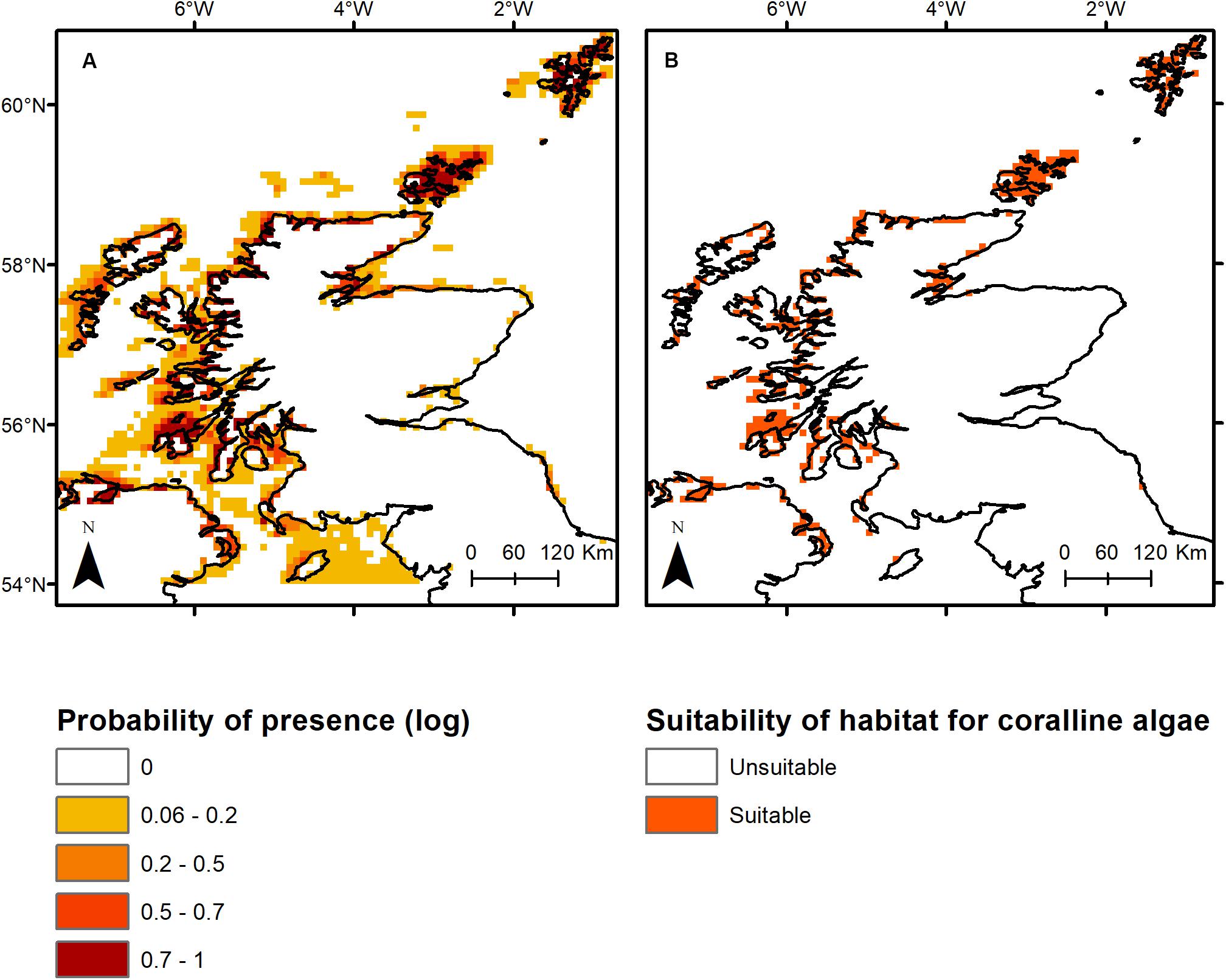
Figure 1. Present-day modeled occurrence of coralline algae around Scotland as (A) probability of presence on a log likelihood scale ranging from 1 (dark red) to 0 (white), and (B) the probabilistic distribution with threshold of occurrence applied, where orange indicates presence while white (background) indicates absence.
Regional-Scale Distribution Under Projected Environmental Change
Under all RCPs, a large decrease in suitable habitat is predicted with at least a 38% decline by 2050 (Table 1). The most immediate losses in suitable habitat are in southern areas of mainland Scotland under all RCPs (Figures 2, 3). At the most extreme, under RCP 8.5 a predicted 83.7% reduction in suitable habitat for non-geniculate coralline algae was found in 2100 compared to the present-day (Figure 3E and Table 1). The north-west mainland coast of Scotland, patches around the Northern Islands and the area around the inner Moray Firth remained strongholds for non-geniculate coralline algae under all future climate projections (Figures 2, 3). No habitat that was previously unsuitable for non-geniculate coralline algal beds (present day) became suitable under any of the RCP scenarios.
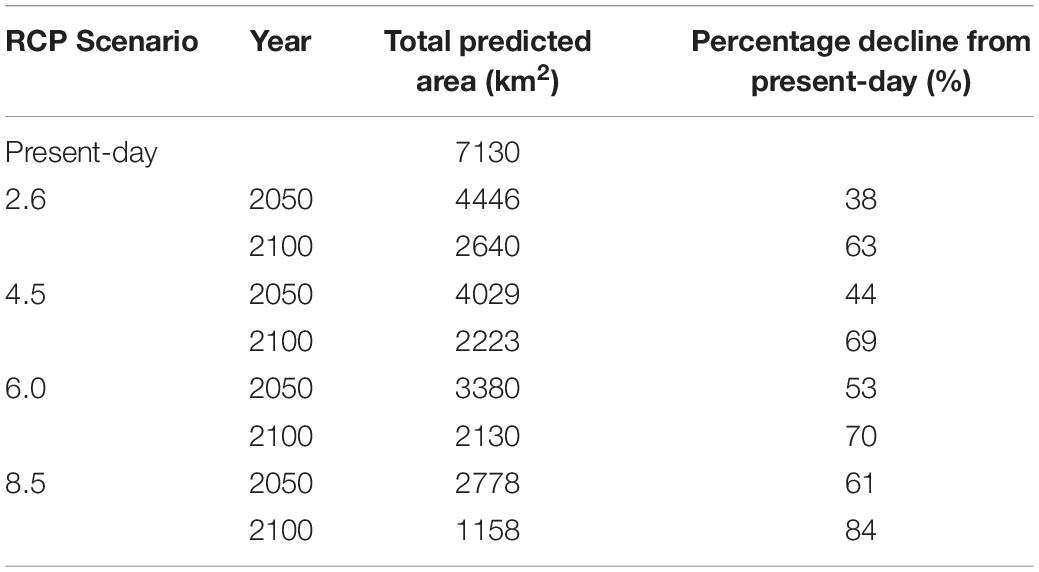
Table 1. Predicted coralline algal bed extent around Scotland in the present-day and under Representative Concentration Pathway (RCP) emission scenarios in the years 2050 and 2100.
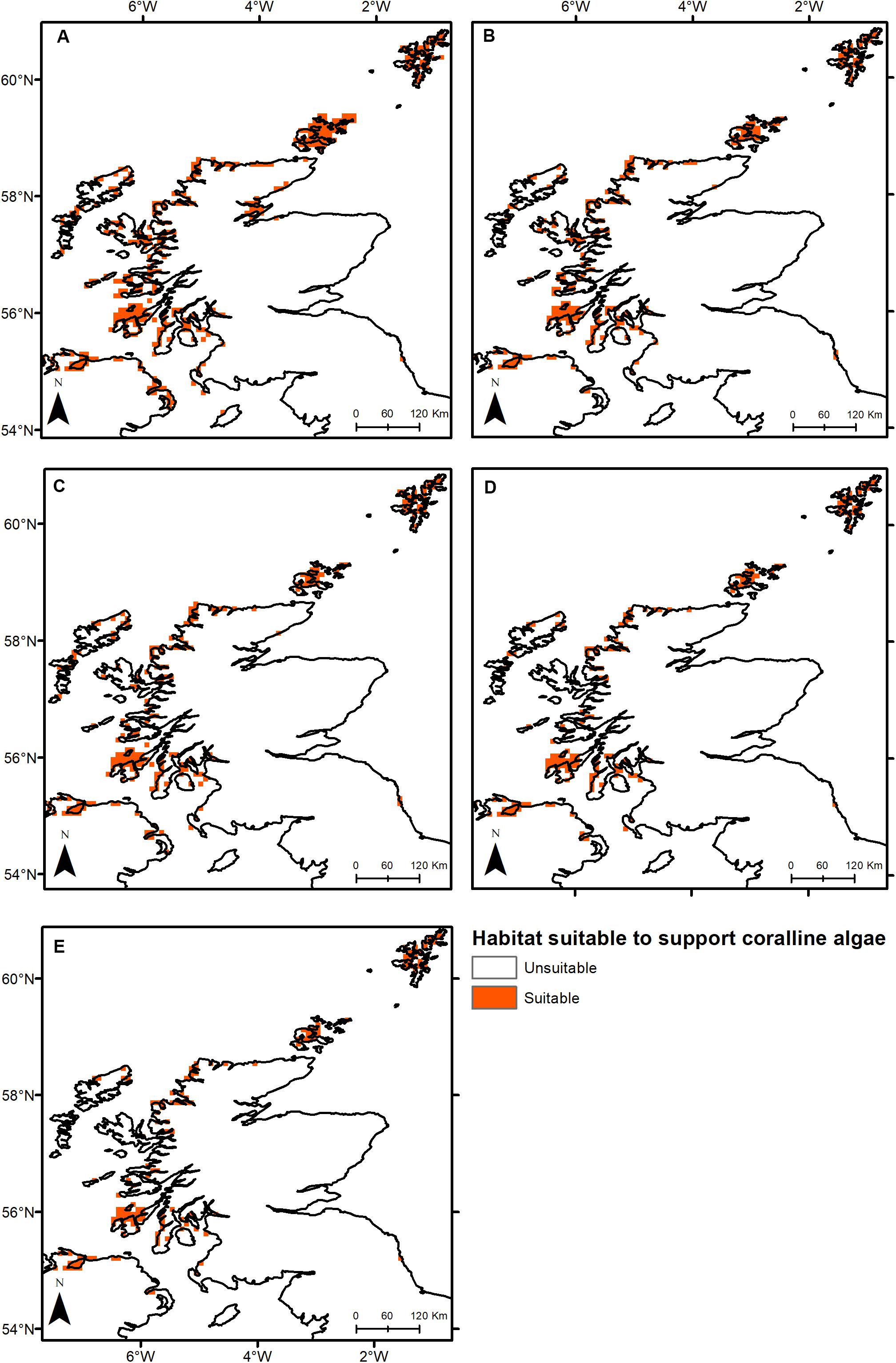
Figure 2. Threshold maps of coralline algal bed distribution around Scotland in (A) the present-day and by 2050 under (B) RCP 2.6, (C) RCP 4.5, (D) RCP 6.0, and (E) RCP 8.5. Orange indicates predicted presence of coralline algal beds.
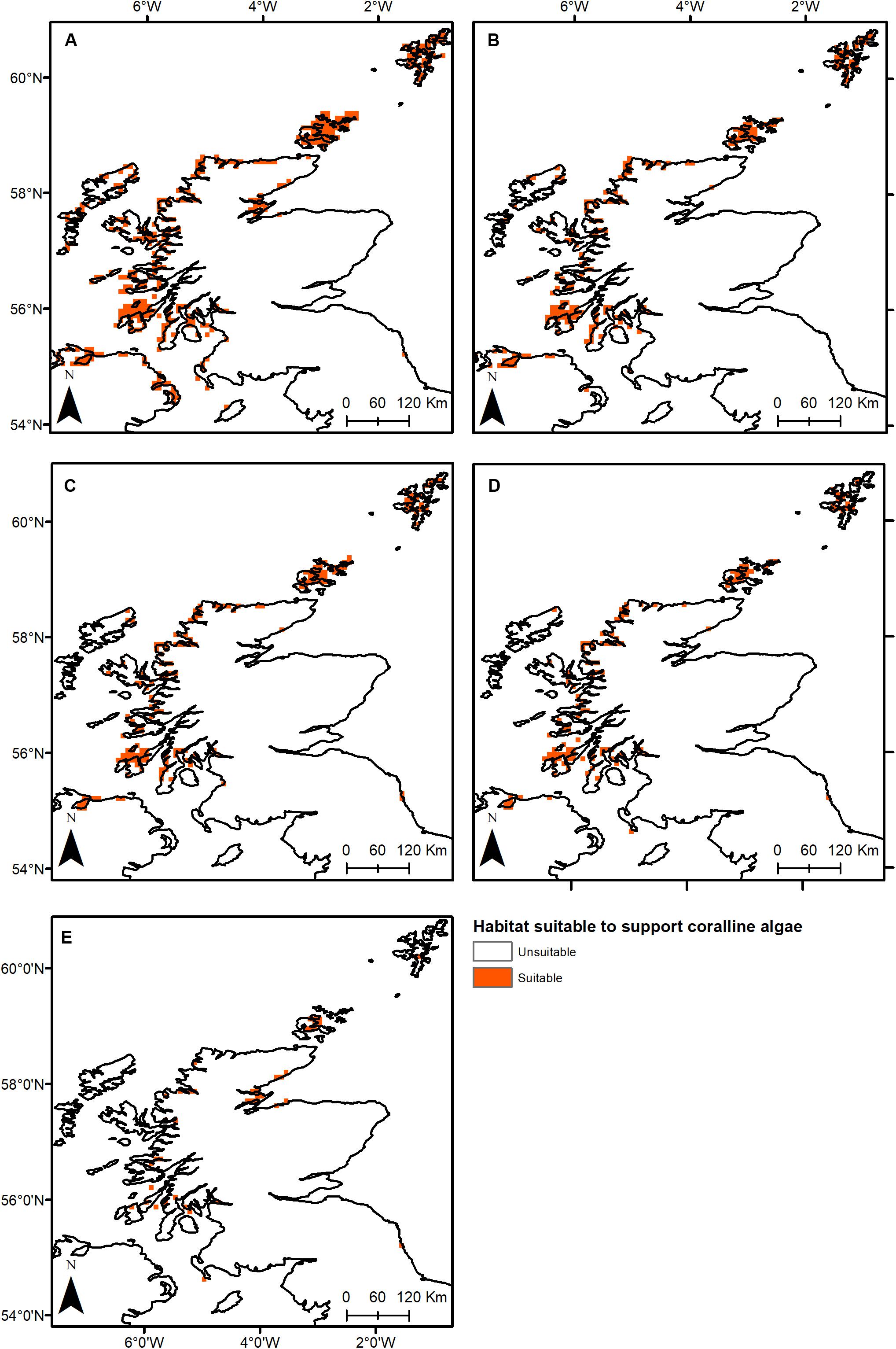
Figure 3. Threshold maps of coralline algal bed distribution around Scotland in (A) the present-day and by 2100 under (B) RCP 2.6, (C) RCP 4.5, (D) RCP 6.0, and (E) RCP 8.5. Orange indicates predicted presence of coralline algal beds.
Regional Variation in Distribution Under Projected Climate Change
Some areas were predicted to be more negatively affected, in terms of coralline algal distribution, by projected environmental change. This spatial variability in persistence was exacerbated under higher-emission scenarios. The Outer Hebrides – currently one of the most important regions for non-geniculate coralline algae – were predicted to experience a 29% decline in coralline algal distribution by 2050 under RCP 2.6, leaving only small patches remaining in the Sound of Barra, west of North Uist and the north of Lewis (Figure 2A). Apart from under RCP 2.6, suitable habitat in the Outer Hebrides was predicted to be restricted to small areas in the north of Lewis by 2100 and was completely lost under RCP 8.5 (Figure 3). By 2050, the Isle of Skye (Inner Hebrides) – was predicted to no longer support non-geniculate coralline algae under RCPs 6.0 and 8.5 (Figures 2D,E). Interestingly, the area east of Skye, which was deemed unsuitable for non-geniculate coralline algal beds under RCP 6.0 in 2050 was retained under RCP 6.0 in 2100, but then lost under RCP 8.5 (2100) (Figures 3D,E).
Some areas were predicted to maintain non-geniculate coralline algal populations throughout the coming decades. In the north of mainland Scotland (Loch Laxford and Ullapool/Loch Broom) and around Orkney and Shetland, high probabilities of coralline algae occurrence (>0.7) were maintained by 2100, even under RCP 8.5 (Figure 3 and Supplementary Figures S2, S3). A relatively high probability (>0.7) of persistence in 2100 under all RCPs was also predicted for Loch Sunart (mainland Scotland) and north Bute (Inner Hebrides) (Figure 3 and Supplementary Figure S3). Under RCP 2.6, 6.0, a refuge population remained on the west of Islay by 2100 (Figures 3A–C) but there was lower probability of it being maintained under RCP 8.5 (0.5–0.7) (Figure 3 and Supplementary Figure S3). There is also a small patch of habitat near Carnigaan (south-west mainland) that retains a higher probability of occurrence even under RCP 8.5 in 2100 (>0.7) (Figure 3E and Supplementary Figure S3).
Similarly, under all RCPs the inner Moray Firth retains several areas of suitable habitat by 2100 (>0.7) (Figure 3E and Supplementary Figure S3).
Discussion
Free-living non-geniculate coralline algal beds are a globally distributed, ecologically important ecosystem (Pardo et al., 2014a; Riosmena-Rodriguez, 2016). Using regional-scale modeling, bathymetry, water temperature and light availability were found to be the primary environmental drivers of non-geniculate coralline algal bed distribution. Projected environmental change is predicted to have a severe negative impact on overall regional-scale coralline algal distribution in Scotland, although spatial heterogeneity in the magnitude of environmental change with the current distributional range has allowed potential future refugia to be identified – areas we propose for priority conservation status.
This regional-scale model highlights the need to understand how small-scale environmental changes affect non-geniculate coralline algae and how beds may be adapted to very localized conditions. Further investigation and understanding of both are key to fully understanding the implications of climate change for non-geniculate coralline algae populations globally.
Spatial Distributions Driven by Environmental Heterogeneity
We have identified a regional-scale east/west division in coralline algal habitat. Coralline algae have not been extensively observed on the east coast, despite extensive surveys (both grab and SCUBA) in the area (e.g., Dargie, 2001), and as the model also identifies this area as predominantly unsuitable, with a low probability of presence, this is unlikely to purely be due to a lack of colonization opportunity in this area, but more to environmental conditions. Higher temperatures on the east coast, coupled with lower current velocities, are likely to be the reason the region is predominantly devoid of non-geniculate coralline algal bed populations. It is noteworthy that overall, the differences in environmental conditions between the east and west coasts of Scotland are relatively minor, indicating how sensitive these non-geniculate coralline algal populations are to their local conditions.
Fine sediments are known to be detrimental to coralline algal survival due to smothering (Fabricius and De’ath, 2001). This may explain why the only predicted east-coast population (inner Moral Firth) is not backed up by records of non-geniculate coralline algal presence – this area is comprised of patchy gravel and sand (British Geological Survey, 2020). Where this mixed sediment is present on the west coast, coralline algae are commonly only found in areas of gravel (e.g., Sound of Barra, Outer Hebrides). This may be further compounded by dredging and shipping activities – extensive in Scotland (Fox et al., 2015) – leading to a resuspension and subsequent smothering of suitable bottom-types for coralline algal colonization. Unfortunately, substrate-type could not be included in the model due to incompatibilities in the dataset resolution.
Looking forward, the ability to identify local areas within a regional space which have the potential to support non-geniculate coralline algae but where it is currently not present highlights a potential opportunity for the translocation of populations as climate change progresses (National Species Reintroduction Forum, 2014). This is particularly pertinent given that no new areas of suitable habitat were identified under climate change scenarios, in line with other previous distributional studies on other calcified marine organisms such as corals (Freeman et al., 2013). Reef restoration is of growing conservation interest, particularly on tropical coral reefs (Suggett et al., 2019), but this is likely to be even more challenging for maerl-forming species due to their slow growth rates (Adey and McKibbin, 1970; Blake and Maggs, 2003; Brodie et al., 2014; Pardo et al., 2019), even if environmentally suitable areas can be identified.
Bathymetry and Light Availability Limit Depth Distribution
Despite low-light adaption (Burdett et al., 2012b) and known worldwide deep-water occurrences (Peña and Barbara, 2009; Hall-Spencer et al., 2010; Friedlander et al., 2014; Pardo et al., 2014a), non-geniculate coralline algal survival was limited to <40 m depth in this region of the NE Atlantic, with the majority found between 5 and 20 m depth. Mitigation of some effects of climate change may be possible for marine organisms that can shift their habitats along a vertical gradient, i.e., moving into deeper cooler waters (Jorda et al., 2020). However, our results do not predict this kind of range shift to be available for non-geniculate coralline algae because of light limitation. Increased Arctic melt-water introduction (Pan et al., 2019), may enable a downward expansion or movement of non-geniculate coralline algae populations due to clearing of the water column. If this was to occur, there would also be knock-on implications for the biodiversity associated with this habitat, which may be depth limited or increased light penetration may lead to macroalgae overgrowth. This could result in major shifts in community structure and subsequent changes in ecosystems services, as have been seen and modeled in coastal fish and invertebrate communities of Australia (Cheung et al., 2012).
Temperature Exerts Control on Latitudinal Extent
The second most important environmental driver of distribution was minimum benthic temperature – known to be a key factor in determining non-geniculate coralline algal species extent globally (McCoy and Kamenos, 2015). Where regional-scale non-geniculate coralline algal communities consist of species with differing thermal envelopes, future distributional changes may become more complex. This is the case here: the two dominant free-living species – P. calcareum and L. glaciale – have different distributional ranges that overlap but show different thermal tolerances; this may affect their survival under projected climate change (especially warming). P. calcareum, one of the dominant free-living non-geniculate coralline algal species throughout Europe (Pardo et al., 2014a; Qui-Minet et al., 2019) is at its northerly limit in Scotland, suggesting a potential capacity to tolerate warming [although this has not been supported by laboratory experimentation (e.g., Qui-Minet et al., 2019)]. Conversely, L. glaciale is at its southerly limit in Scotland (Pardo et al., 2014a) and is known to be prevalent across the Atlantic Arctic (Schoenrock et al., 2018). Calcification and temperature in L. glaciale are known to be negatively related (Kamenos and Law, 2010), suggesting projected warming over the coming century will negatively impact L. glaciale populations, supporting the habitat declines seen here. Species-specific responses to projected environmental change will thus determine the magnitude of coralline algal ecosystem change but input data at the species level was not available for this study. However, it is clear from the results presented here that coralline algal beds at the edges of their range (be that geographically, or by depth) face severe threats over the coming century and should be the focus of immediate conservation management.
Future Drivers of Distributional Change
Under all RCPs, there was a significant decrease in the amount of suitable areas for non-geniculate coralline algal habitat, but this was accompanied by high spatial variability. Warming projections for our study region were characterized by latitudinal trends in the magnitude of projected warming (higher in the south, lower in the north; Supplementary Figure S4) combined with localized areas of little warming compared to the overall trend – this local-regional scale heterogeneity in warming has also been projected globally (IPCC, 2014). Importantly, variability in warming led to the persistence of refuge populations throughout the study region. However, this may increase habitat fragmentation, which can lead to inbreeding depression and decreasing population health (Pavlova et al., 2017; Pardo et al., 2019), ultimately weakening the likelihood of long-term survival throughout the coming century and beyond.
Clamping of the SDM to conditions only encountered in the training data mean that the results presented here perhaps represent a “worst-case scenario” for the effects of environmental change – it is assumed that environmental conditions beyond present-day variability will not be suitable for non-geniculate coralline algal habitat in the future. Present-day latitudinal distributions, especially for P. calcareum, suggest this may not be representative, but the capacity for coralline algae to shift poleward is expected to be minimal because of their slow growth rates and limited dispersal abilities (Brodie et al., 2014). This contrasts with other ecosystem engineers such as kelp, for which poleward shifts have already been recorded around the world (Smale, 2020). Likewise, there may be some opportunity for in situ adaptation to changing conditions, although there is little supporting evidence to suggest this is likely to occur within the short-time frame involved, and this is confounded by a general lack of knowledge of population dynamics and the extent of sexual reproduction in non-geniculate coralline algal populations (Pardo et al., 2019).
Future non-geniculate coralline algal bed distribution may also be affected by projected declines in ocean pH (McCoy and Kamenos, 2015) – red coralline algae and their associated communities are generally considered to be highly sensitive to low pH (Martin and Gattuso, 2009; Burdett et al., 2012a, 2018; Kroeker et al., 2012; Kamenos et al., 2013, 2016; Qui-Minet et al., 2019). Thus, it would be expected that projected declines in ocean pH would further limit the distributional extent of non-geniculate coralline algae, perhaps counter-balancing the effects of clamping in the model. Unfortunately, a pH projection dataset at a suitable spatial resolution and extent was not available for inclusion in the projection modeling and so could not be tested here.
Wider Ecosystem Implications
Reduced coralline algal habitat as a result of environmental change will also have significant effects on associated species and biogeochemical cycling. This may result in large community shifts (Brodie et al., 2014), as has been previously observed e.g., during the Little Ice Age/Medieval Warm Period transition (Mao et al., 2020). Coralline algal beds are a globally important store of blue carbon (van der Heijden and Kamenos, 2015; Porter et al., 2020), with the potential to store the most carbon per unit area of all coastal ecosystems in the study area (Burrows et al., 2014, 2017). The magnitude of stored carbon in coralline algal beds is known to be positively related to water temperature (Mao et al., 2020), suggesting areas of future coralline algal persistence may also experience a proportional increase in carbon storage. However, in areas of coralline algal bed loss, disturbance to the system may release carbon that had been previously locked away for centuries if not millennia (Macreadie et al., 2019). Conversely, without disturbance, the persistence of a dead skeletal framework may enable the continued accumulation of incoming organic carbon and maintain sediment carbon stability, albeit without photosynthetic carbon sequestration.
The value of these non-geniculate coralline algal habitats, along with other biogenic reef habitats, has been broadly overlooked to-date (Sunday et al., 2017; Bindoff et al., 2019). Globally, the value of these habitats as ecosystem engineers, biodiversity hotspots and nursey habitats is still underestimated and under-protected despite the growing threat they face due to increasingly changing climates (Bindoff et al., 2019). Understanding, the environmental conditions needed to maintain these habitats and the degree to which they are locally adapted, emphasizes the need for better protection and further study of these fragile but globally important systems (Kamenos et al., 2008b; Sheehan et al., 2015; Sunday et al., 2017; Bindoff et al., 2019).
Policy Implications
Few marine protected areas (MPAs) or special areas of conservation (SACs) around the world are specifically designated for the protection of non-geniculate coralline algal beds (Barbera et al., 2003), but the results from our study suggest their long-term large-scale distribution is at risk. Although conservation management is not able to directly protect against changing environmental conditions, it does lessen the impact of other pressures, enhancing the potential for climate change-related mitigation and/or adaptation (Roberts et al., 2017).
In our study region, although the majority of known non-geniculate coralline algal beds are located within the Scottish MPA and SAC network (Supplementary Figure S6), only ten of 217 are designated for free-living coralline algae bed protection (as “maerl beds”) (Fetlar to Haroldswick, Wyre and Rousay Sounds, Loch Laxford, Wester Ross, Loch Carron, Loch nam Madadh, Sound of Barra, Loch Sween, South Arran and Luce Bay, and Sands) (Supplementary Figure S6). Even in these areas some permitted activities (e.g., licensed fishing) may detrimentally affect this ecosystem, Scottish Natural Heritage (2019b). The placement of non-geniculate coralline algae-specific MPAs does not cover full present-day extent, and only 20% of the potential future refuge populations are currently covered (Supplementary Figures S7, S8).
Connectivity between refugia and neighboring habitats may strengthen the future persistence of non-geniculate coralline algal beds, similar to the “spill over” of ecosystem services seen in other MPA networks (Lynham et al., 2020). We therefore propose that the refuge areas (Loch Laxford, inner Moray Firth, north of Arran, north of Islay, Mainland Orkney and Mainland Shetland) should be of high conservation priority since they are expected to offer persistently suitable habitat for the coming decades – expected to be the period of most rapid distributional decline. In support of this, a current consultation by the Scottish Government and Marine Scotland, supported by Scottish Natural Heritage, aims to develop fisheries management measures to protect particularly sensitive benthic habitats and PMFs (including coralline algal beds) outside of MPAs (Marine Scotland, 2018; Scottish Government, 2019).
Summary and Conclusion
Non-geniculate coralline algal beds are a globally important ecosystem for biodiversity, socio-economic benefit and carbon storage. Scotland is a European regional stronghold for these coralline algal beds, but here we demonstrate their high sensitivity to projected climate change, and demonstrate the value of SDMs for understanding regional-scale environmental drivers of their distribution. Projected climate change over the coming century is predicted to leave fragmented refugia that may be more vulnerable to long-term survival and therefore should be a priority for conservation management for favorable conditions. Applications of this SDM approach to free-living, non-geniculate coralline algae in other regions around the world will enable a global network of distributional change to be determined, facilitating international-scale conservation decisions and an assessment of the knock-on implications for the future of coralline algal ecosystem service provision.
This paper highlights the need for a greater understanding of non-geniculate coralline algal bed habitats in order to prepare for various climate futures. Increased survey activity in order to better establish true presences and absences of beds, and high resolution data on environmental conditions would be appropriate places to start and allow for more detailed and in depth modeling to be carried out.
Data Availability Statement
Publicly available datasets were analyzed in this study. This data can be found here: Geodatabase of Marine Features in Scotland (GeMS) (https://data.gov.uk/dataset/0e78afea-ac1e-4080-8758-980f2d5cff6d/gems-scottish-priority-marine-features-pmf). Bio-ORACLE (https://www.bio-oracle.org/).
Author Contributions
HB, TF, JB, PH, and CS-N designed the experiment. LK and CS-N identified and accessed the data. CS-N carried out modeling and analysis, with help from HB, and wrote the manuscript. LK and JB provided information on conservation management in Scotland and provided links to policy organizations. All authors have confirmed and approved the submitted version of the manuscript, provided input on methods and provided detailed edits, and feedback on the manuscript.
Funding
This work was funded by a NERC iCASE studentship award to HB, PH, JB, and TF (NE/R007233/1). The Royal Botanic Garden Edinburgh acknowledges funding support from the Scottish Government Rural and Environment Sciences and Analytical Services Division (RESAS). This is a contribution to the Scottish Blue Carbon Forum.
Conflict of Interest
The authors declare that the research was conducted in the absence of any commercial or financial relationships that could be construed as a potential conflict of interest.
Supplementary Material
The Supplementary Material for this article can be found online at: https://www.frontiersin.org/articles/10.3389/fmars.2020.575825/full#supplementary-material
Footnotes
References
Adey, W. H., and McKibbin, D. L. (1970). Studies on the maerl species Phymatolithon calcareum (Pallas) nov. comb. and Lithothamnium coralloides Crouan in the Ria de Vigo. Bot. Mar. 13, 100–106.
Assis, J., Tyberghein, L., Bosch, S., Verbruggen, H., Serrão, E. A., and Clerck, O. D. (2018). Bio-ORACLE v2.0: extending marine data layers for bioclimatic modelling. Glob. Ecol. Biogeogr. 27, 277–284. doi: 10.1111/geb.12693
Baddeley, A., Turner, R., and Rubak, E. (2019). CRAN - Package Spatstat. Available online at: https://cran.r-project.org/web/packages/spatstat/index.html (accessed November 8, 2019).
Barbera, C., Bordehore, C., Borg, J. A., Glémarec, M., Grall, J., Hall-Spencer, J. M., et al. (2003). Conservation and management of northeast Atlantic and Mediterranean maerl beds. Aquat. Conserv. Mar. Freshw. Ecosyst. 13, S65–S76. doi: 10.1002/aqc.569
Basso, D. (1998). Deep rhodolith distribution in the Pontian Islands, Italy: a model for the paleoecology of a temperate sea. Palaeogeogr. Palaeoclimatol. Palaeoecol. 137, 173–187. doi: 10.1016/s0031-0182(97)00099-0
Bellard, C., Bertelsmeier, C., Leadley, P., Thuiller, W., and Courchamp, F. (2012). Impacts of climate change on the future of biodiversity. Ecol. Lett. 15, 365–377. doi: 10.1111/j.1461-0248.2011.01736.x
Bindoff, N., Cheung, W. W. L., Kairo, J. G., Arístegui, J., Guinder, V. A., Hallberg, R., et al. (2019). Changing Ocean, Marine Ecosystems, and Dependent Communities.. Available online at: https://www.ipcc.ch/srocc/chapter/chapter-5/ (accessed August 3, 2020).
Blake, C., and Maggs, C. (2003). Comparative growth rates and internal banding periodicity of maerl species (Corallinales, Rhodophyta) from northern Europe. Phycologia 42, 606–612. doi: 10.2216/i0031-8884-42-6-606.1
British Geological Survey (2020). British Geological Survey (BGS) Offshore Marine Products. Available online at: https://www.bgs.ac.uk/data/services/offprodwms.html (accessed January 23, 2020).
Brodie, J., Williamson, C. J., Smale, D. A., Kamenos, N. A., Mieszkowska, N., Santos, R., et al. (2014). The future of the northeast Atlantic benthic flora in a high CO2 world. Ecol. Evol. 4, 2787–2798. doi: 10.1002/ece3.1105
Burdett, H., Perna, G., McKay, L., Broomhead, G., and Kamenos, N. (2018). Community-level sensitivity of a calcifying ecosystem to acute in situ CO2 enrichment. Mar. Ecol. Prog. Ser. 587, 73–80. doi: 10.3354/meps12421
Burdett, H. L., Aloisio, E., Calosi, P., Findlay, H. S., Widdicombe, S., Hatton, A. D., et al. (2012a). The effect of chronic and acute low pH on the intracellular DMSP production and epithelial cell morphology of red coralline algae. Mar. Biol. Res. 8, 756–763. doi: 10.1080/17451000.2012.676189
Burdett, H. L., Hatton, A. D., and Kamenos, N. A. (2015). Coralline algae as a globally significant pool of marine dimethylated sulfur. Glob. Biogeochem. Cycles 29, 1845–1853. doi: 10.1002/2015GB005274
Burdett, H. L., Hennige, S. J., Francis, F. T.-Y., and Kamenos, N. A. (2012b). The photosynthetic characteristics of red coralline algae, determined using pulse amplitude modulation (PAM) fluorometry. Bot. Mar. 55, 499–509. doi: 10.1515/bot-2012-0135
Burrows, M. T., Hughes, D. J., Austin, W. E. N., Smeaton, C., Hicks, N., Howe, J. A., et al. (2017). Assessment of blue carbon resources in Scotland’s inshore marine protected area network. Scott. Nat. Herit. Comm. Rep. 957:283.
Burrows, M. T., Kamenos, N. A., Hughes, D. J., Stahl, H., Howe, J. A., and Tett, P. (2014). Assessment of carbon budgets and potential blue carbon stores in Scotland’s coastal and marine environment. Scott. Nat. Herit. Comm. Rep. 761:90.
Cheung, W. W. L., Meeuwig, J. J., Feng, M., Harvey, E., Lam, V. W. Y., Langlois, T., et al. (2012). Climate-change induced tropicalisation of marine communities in Western Australia. Mar. Freshw. Res. 63, 415. doi: 10.1071/MF11205
Comhairle nan Eilean Siar (2020a). South Ford Causeway. Available online at: https://www.cne-siar.gov.uk/roads-travel-and-parking/bridges-causeways-and-ferries/south-ford-causeway/ (accessed May 14, 2020).
Comhairle nan Eilean Siar (2020b). Vatersay Causeway. Vatersay Causew. Available online at: https://www.cne-siar.gov.uk/roads-travel-and-parking/bridges-causeways-and-ferries/vatersay-causeway/ (accessed May 14, 2020).
Cornwall, C. E., Diaz-Pulido, G., and Comeau, S. (2019). Impacts of ocean warming on coralline Algal calcification: meta-analysis, knowledge gaps, and key recommendations for future research. Front. Mar. Sci. 6:186. doi: 10.3389/fmars.2019.00186
Dargie, T. (2001). Sand Dune Vegetation Survey of Scotland: East Coast. Volume 1: Main report. Available online at: https://www.nature.scot/sites/default/files/2017-07/Publication%202001%20-%20SNH%20Research%2C%20Survey%20and%20Monitoring%20Report%20179%20-%20Sand%20dune%20vegetation%20survey%20of%20Scotland%20-%20East%20Coast%20-%20Volume%201%20-%20Main%20report.pdf (accessed August 9, 2019).
Dutertre, M., Grall, J., Ehrhold, A., and Hamon, D. (2014). Environmental factors affecting maerl bed structure in Brittany (France). Eur. J. Phycol 50, 371–383. doi: 10.1080/09670262.2015.1063698
Elith, J., Phillips, S. J., Hastie, T., Dudík, M., Chee, Y. E., and Yates, C. J. (2011). A statistical explanation of MaxEnt for ecologists: statistical explanation of MaxEnt. Divers. Distrib. 17, 43–57. doi: 10.1111/j.1472-4642.2010.00725.x
Elliott, S., Turrell, W., Heath, M., and Bailey, D. (2017). Juvenile gadoid habitat and ontogenetic shift observations using stereo-video baited cameras. Mar. Ecol. Prog. Ser. 568, 123–135. doi: 10.3354/meps12068
European Commission (2016). The Habitats Directive - Environment - European Commission. EU Nat. Law. Available online at: http://ec.europa.eu/environment/nature/legislation/habitatsdirective/index_en.htm (accessed November 10, 2018).
European Commission (2018). Natura 2000. Available online at: http://ec.europa.eu/environment/nature/natura2000/index_en.htm (accessed November 10, 2018).
Fabricius, K., and De’ath, G. (2001). Environmental factors associated with the spatial distribution of crustose coralline algae on the great barrier reef. Coral Reefs 19, 303–309. doi: 10.1007/s003380000120
Fithian, W., Elith, J., Hastie, T., and Keith, D. A. (2015). Bias correction in species distribution models: pooling survey and collection data for multiple species. Methods Ecol. Evol. 6, 424–438. doi: 10.1111/2041-210X.12242
Foster, M. S. (2001). Rhodoliths: between rocks and soft places. J. Phycol. 37, 659–667. doi: 10.1046/j.1529-8817.2001.00195.x
Foster, M. S., Amado Filho, G. M., Kamenos, N. A., Riosmena-Rodríguez, R., Steller, D. L., Lang, M. A., et al. (2013). “Rhodoliths and Rhodolith Beds,” in Research and Discoveries: The Revolution of Science through Scuba, (Washington: Smithsonian Contributions to the Marine Sciences), 143–155.
Fox, C. J., Valcic, L., and Veszelovsxki, A. (2015). Evidence Gathering in Support of Sustainable Scottish Inshore Fisheries: Work Package 4 Final Report A Pilot Study to Define the Footprint and Activities of Scottish Inshore Fisheries by Identifying Target Fisheries, Habitats and Associated Fish Stocks. Scotland: Marine Alliance for Science and Technology Scotland.
Freeman, L., Kleypas, J. A., and Miller, A. J. (2013). PLoS ONE 8:e82404. doi: 10.1371/journal.pone.0082404
Friedlander, A. M., Caselle, J. E., Ballesteros, E., Brown, E. K., Turchik, A., and Sala, E. (2014). The real bounty: marine biodiversity in the pitcairn Islands. PLoS One 9:e100142. doi: 10.1371/journal.pone.0100142
Gallon, R. K., Robuchon, M., Leroy, B., Le Gall, L., Valero, M., and Feunteun, E. (2014). Twenty years of observed and predicted changes in subtidal red seaweed assemblages along a biogeographical transition zone: inferring potential causes from environmental data. J. Biogeogr. 41, 2293–2306. doi: 10.1111/jbi.12380
Grall, J., and Hall-Spencer, J. M. (2003). Problems facing maerl conservation in Brittany. Aquat. Conserv. Mar. Freshw. Ecosyst. 13, S55–S64. doi: 10.1002/aqc.568
Gubbay, S., Sanders, N., Haynes, T., Janssen, J. A. M., Airoldi, L., Battelli, C., et al. (2016). European Red list of habitats. Luxembourg: Publications Office of the European Union.
Hall-Spencer, J., White, N., Gillespie, E., Gillham, K., and Foggo, A. (2006). Impact of fish farms on maerl beds in strongly tidal areas. Mar. Ecol. Prog. Ser. 326, 1–9. doi: 10.3354/meps326001
Hall-Spencer, J. M., Kelly, J., and Maggs, C. A. (2008). Assessment of Maerl Beds in the OSPAR Area and the Development of A Monitoring Program. Ireland: Department of the Environment HaLG, Ireland.
Hall-Spencer, J. M., Kelly, J., and Maggs, C. A. (2010). OSPAR Commission Background Document for Maerl beds. London: OSPAR Commission.
Hastings, A., Byers, J. E., Crooks, J. A., Cuddington, K., Jones, C. G., Lambrinos, J. G., et al. (2007). Ecosystem engineering in space and time. Ecol. Lett. 10, 153–164. doi: 10.1111/j.1461-0248.2006.00997.x
Hijmans, R. (2017). Package “Dismo.”. Available online at: https://www.rdocumentation.org/packages/dismo/versions/1.1-4 (accessed August 28, 2019).
Hoegh-Guldberg, O., Mumby, P. J., Hooten, A. J., Steneck, R. S., Greenfield, P., Gomez, E., et al. (2007). Coral reefs under rapid climate change and ocean acidification. Science 318, 1737–1742. doi: 10.1126/science.1152509
Hughes, T. P., Rodrigues, M. J., Bellwood, D. R., Ceccarelli, D., Hoegh-Guldberg, O., McCook, L., et al. (2007). Phase shifts. herbivory, and the resilience of coral reefs to climate change. Curr. Biol. 17, 360–365. doi: 10.1016/j.cub.2006.12.049
IPCC (2014). Climate Change 2014: Synthesis Report. Contribution of Working Groups I, II and III to the Fifth Assessment Report of the Intergovernmental Panel on Climate Change. Available online at: https://www.ipcc.ch/report/ar5/syr/ (accessed February 3, 2020).
Jackson, C. M., Kamenos, N. A., Moore, P. G., and Young, M. (2004). Meiofaunal bivalves in maerl and other substrata; their diversity and community structure. Ophelia 58, 49–60.
JNCC (2016). UK BAP Priority Habitats. Available online at: http://jncc.defra.gov.uk/page-5718 (accessed November 10, 2018).
Jorda, G., Marbà, N., Bennett, S., Santana-Garcon, J., Agusti, S., and Duarte, C. M. (2020). Ocean warming compresses the three-dimensional habitat of marine life | Nature Ecology & Evolution. Nat. Ecol. Evol. 4, 109–114. doi: 10.1038/s41559-019-1058-0
Júnior, P. D. M., and Nóbrega, C. C. (2018). Evaluating collinearity effects on species distribution models: An approach based on virtual species simulation. PLoS One 13:e0202403. doi: 10.1371/journal.pone.0202403
Kamenos, N., and Law, A. (2010). Temperature Controls on Coralline Algal Skeletal Growth. J. Phycol. 46, 331–335. doi: 10.1111/j.1529-8817.2009.00780.x
Kamenos, N., Moore, G., and Hall-Spencer, J. M. (2004). Small-scale distribution of juvenile gadoids in shallow inshore waters; what role does maerl play? ICES J. Mar. Sci. 61, 422–429. doi: 10.1016/j.icesjms.2004.02.004
Kamenos, N. A., Burdett, H. L., Aloisio, E., Findlay, H. S., Martin, S., Longbone, C., et al. (2013). Coralline algal structure is more sensitive to rate, rather than the magnitude, of ocean acidification. Glob. Change Biol. 19, 3621–3628. doi: 10.1111/gcb.12351
Kamenos, N. A., Perna, G., Gambi, M. C., Micheli, F., and Kroeker, K. J. (2016). Coralline algae in a naturally acidified ecosystem persist by maintaining control of skeletal mineralogy and size. Proc. R. Soc. B Biol. Sci. 283:20161159. doi: 10.1098/rspb.2016.1159
Kamenos, N. A., Strong, S., Shenoy, D., Wilson, S., Hatton, A., and Moore, P. (2008b). Red coralline algae as a source of marine biogenic dimethylsulphoniopropionate. Mar. Ecol. Prog. Ser. 372, 61–66. doi: 10.3354/meps07687
Kroeker, K. J., Micheli, F., and Gambi, M. C. (2012). Ocean acidification causes ecosystem shifts via altered competitive interactions. Nat. Clim. Change 3, 156–159. doi: 10.1038/nclimate1680
Legrand, E., Riera, P., Lutier, M., Coudret, J., Grall, J., and Martin, S. (2017). Species interactions can shift the response of a maerl bed community to ocean acidification and warming. Biogeosciences 14, 5359–5376. doi: 10.5194/bg-14-5359-2017
Lynham, J., Nikolaev, A., Raynor, J., Vilela, T., and Villaseñor-Derbez, J. C. (2020). Impact of two of the world’s largest protected areas on longline fishery catch rates. Nat. Commun 11, 1–9.
Macreadie, P. I., Anton, A., Raven, J. A., Beaumont, N., Connolly, R. M., Friess, D. A., et al. (2019). The future of blue carbon science. Nat. Commun. 10, 1–13. doi: 10.1038/s41467-019-11693-w
Mao, J., Burdett, H. L., McGill, R. A. R., Newton, J., Gulliver, P., and Kamenos, N. A. (2020). Carbon burial over the last four millennia is regulated by both climatic and land use change. Glob. Change Biol. 26, 2496–2504. doi: 10.1111/gcb.15021
Marine Scotland. (2018). Improving Protection Given to Priority Marine Features Outside the Marine Protected Area Network. Available online at: https://www.gov.scot/publications/improving-protection-given-pmfs-outside-mpa-network/pages/8/ (accessed January 30, 2020).
Martin, S., and Gattuso, J.-P. (2009). Response of Mediterranean coralline algae to ocean acidification and elevated temperature. Glob. Change Biol. 15, 2089–2100. doi: 10.1111/j.1365-2486.2009.01874.x
Martin, S., and Hall-Spencer, J. M. (2017). “Effects of Ocean Warming and Acidification on Rhodolith/Maërl Beds,” in Rhodolith/Maërl Beds: A Global Perspective Coastal Research Library, eds R. Riosmena-Rodriguez, W. Nelson, and J. Aguirre (Berlin: Springer).
Martínez, B., Radford, B., Thomsen, M. S., Connell, S. D., Carreño, F., Bradshaw, C. J. A., et al. (2018). Distribution models predict large contractions of habitat-forming seaweeds in response to ocean warming. Divers. Distrib. 24, 1350–1366. doi: 10.1111/ddi.12767
McCoy, S. J., and Kamenos, N. A. (2015). Coralline algae (Rhodophyta) in a changing world: integrating ecological, physiological, and geochemical responses to global change. J. Phycol. 51, 6–24. doi: 10.1111/jpy.12262
Merow, C., Smith, M. J., and Silander, J. A. (2013). A practical guide to MaxEnt for modeling species’ distributions: what it does, and why inputs and settings matter. Ecography 36, 1058–1069. doi: 10.1111/j.1600-0587.2013.07872.x
Muscarella, R., Galante, P. J., Soley-Guardia, M., Boria, R. A., Kass, J. M., Uriarte, M., et al. (2018). ENMeval: Automated Runs and Evaluations of Ecological Niche Models. Available online at: https://cran.r-project.org/web/packages/ENMeval/index.html (accessed November 22, 2018).
Muscarella, R., Galante, P. J., Soley-Guardia, M., Boria, R. A., Kass, J. M., Uriarte, M., et al. (2014). ENMeval: an R package for conducting spatially independent evaluations and estimating optimal model complexity for Maxent ecological niche models. Methods Ecol. Evol. 5, 1198–1205. doi: 10.1111/2041-210X.12261
National Species Reintroduction Forum (2014). The Scottish Code for Conservation Translocations. Available online at: https://www.nature.scot/sites/default/files/Publication%202014%20-%20The%20Scottish%20Code%20for%20Conservation%20Translocations.pdf (accessed March 23, 2020).
Nelson, W. A. (2009). Calcified macroalgae - critical to coastal ecosystems and vulnerable to change: a review. Mar. Freshw. Res. 60:787. doi: 10.1071/MF08335
Noisette, F., Duong, G., Six, C., Davoult, D., and Martin, S. (2013a). Effects of elevated pCO2 on the metabolism of a temperate rhodolith Lithothamnion corallioides grown under different temperatures. J. Phycol. 49, 746–757. doi: 10.1111/jpy.12085
Noisette, F., Egilsdottir, H., Davoult, D., and Martin, S. (2013b). Physiological responses of three temperate coralline algae from contrasting habitats to near-future ocean acidification. J. Exp. Mar. Biol. Ecol. 448, 179–187. doi: 10.1016/j.jembe.2013.07.006
Pan, B. J., Vernet, M., Reynolds, R. A., and Mitchell, B. G. (2019). The optical and biological properties of glacial meltwater in an Antarctic fjord. PLoS One 14:e0211107. doi: 10.1371/journal.pone.0211107
Pardo, C., Guillemin, M.-L., Peña, V., Bárbara, I., Valero, M., and Barreiro, R. (2019). Local coastal configuration rather than latitudinal gradient shape clonal diversity and genetic structure of phymatolithon calcareum maerl beds in North European Atlantic. Front. Mar. Sci. 6:149. doi: 10.3389/fmars.2019.00149
Pardo, C., Lopez, L., Peña, V., Hernández-Kantún, J., Gall, L. L., Bárbara, I., et al. (2014a). A Multilocus species delimitation reveals a striking number of species of coralline algae forming maerl in the OSPAR maritime area. PLoS One 9:e104073. doi: 10.1371/journal.pone.0104073
Pardo, C., Peña, V., Bárbara, I., Valero, M., and Barreiro, R. (2014b). Development and multiplexing of the first microsatellite markers in a coralline red alga (Phymatolithon calcareum, Rhodophyta). Phycologia 53, 474–479. doi: 10.2216/14-031.1
Pavlova, A., Beheregaray, L. B., Coleman, R., Gilligan, D., Harrisson, K. A., Ingram, B. A., et al. (2017). Severe consequences of habitat fragmentation on genetic diversity of an endangered Australian freshwater fish: a call for assisted gene flow. Evol. Appl. 10, 531–550. doi: 10.1111/eva.12484
Pearson, R. G. (2010). Species’ Distribution Modeling for Conservation Educators and Practitioners. Lessons Conserv. 3, 54–89.
Pearson, R. G., Raxworthy, C. J., Nakamura, M., and Peterson, A. T. (2007). Predicting species distributions from small numbers of occurrence records: a test case using cryptic geckos in Madagascar. J. Biogeogr. 34, 102–117. doi: 10.1111/j.1365-2699.2006.01594.x
Peña, V., and Barbara, I. (2009). Distribution of the Galician maerl beds and their shape classes (Atlantic Iberian Peninsula): proposal of areas in future conservation actions. Cah. Biol. Mar. 50, 335–368.
Peña, V., Barbara Criado, I., Grall, J., Maggs, C. A., and Hall-Spencer, J. (2014). The diversity of seaweeds on maerl in the NE Atlantic. Mar. Biodivers. 44, 533–551. doi: 10.1007/s12526-014-0214-7
Perry, F., Jackson, A., and Garrard, S. L. (2017a). “Lithothamnion glaciale Maerl,” in Marine Life Information Network: Biology and Sensitivity Key Information Reviews, eds H. Tyler-Walters and K. Hiscock (Plymouth: Marine Biological Association of the United Kingdom).
Perry, F., Jackson, A., Garrard, S. L., and Hiscock, K. (2017b). “Phymatolithon calcareum Maerl,” in Marine Life Information Network: Biology and Sensitivity Key Information Reviews, ed. H. Tyler-Walters (Plymouth: Marine Biological Association of the United Kingdom).
Phillips, S. J., Dudík, M., and Schapire, R. E. (2006). Maxent Software for Modeling Species Niches and Distributions. Available online at: http://biodiversityinformatics.amnh.org/open_source/maxent (accessed January 20, 2019).
Porter, J. S., Austin, W. E. N., Burrows, M. T., Davies, G., Kamenos, N., Riegel, S., et al. (2020). Blue Carbon Audit of Scottish Waters. Available online at: https://www.researchgate.net/publication/338585272_Blue_Carbon_Audit_of_Orkney_Waters (accessed March 12, 2020).
Porzio, L., Buia, M. C., and Hall-Spencer, J. M. (2011). Effects of ocean acidification on macroalgal communities. J. Exp. Mar. Biol. Ecol. 400, 278–287. doi: 10.1016/j.jembe.2011.02.011
Qui-Minet, Z. N., Coudret, J., Davoult, D., Grall, J., Mendez-Sandin, M., Cariou, T., et al. (2019). Combined effects of global climate change and nutrient enrichment on the physiology of three temperate maerl species. Ecol. Evol. 9, 13787–13807. doi: 10.1002/ece3.5802
R Core Team (2019). R: A Language and Environment for Statistical Computing. Vienna: R Foundation for Statistical Computing.
Riosmena-Rodriguez, R. (ed.) (2016). “The Role of Rhodolith/Maërl Beds in Modern Oceans,” in Rhodolith/maerl Beds: A Global Perspective. New York, NY: Springer, 3–26.
Riosmena-Rodriguez, R., Nelson, W. A., and Aguirre, J. (eds) (2016). Rhodolith/maerl Beds: A Global Perspective. New York, NY: Springer.
Roberts, C. M., O’Leary, B. C., McCauley, D. J., Cury, P. M., Duarte, C. M., Lubchenco, J., et al. (2017). Marine reserves can mitigate and promote adaptation to climate change. Proc. Natl. Acad. Sci. U.S.A. 114, 6167–6175. doi: 10.1073/pnas.1701262114
Schoenrock, K. M., Bacquet, M., Pearce, D., Rea, B. R., Schofield, J. E., Lea, J., et al. (2018). Influences of salinity on the physiology and distribution of the Arctic coralline algae, Lithothamnion glaciale (Corallinales, Rhodophyta). J. Phycol. 54, 690–702. doi: 10.1111/jpy.12774
Scottish Government (2018). Priority Marine Features. Available online at: http://www2.gov.scot/Topics/marine/marine-environment/mpanetwork/PMF (accessed January 11, 2019).
Scottish Government (2019). Improving protection given to Priority Marine Features Outside the Marine Protected Area Network - Scottish Government - Citizen Space. Available online at: https://consult.gov.scot/marine-scotland/priority-marine-features/ (accessed January 30, 2020).
Scottish Natural Heritage (2019a). GeMS - Scottish Priority Marine Features (PMF). Available online at: https://gateway.snh.gov.uk/natural-spaces/dataset.jsp?dsid=GEMS-PMF (accessed August 28, 2019).
Scottish Natural Heritage (2019b). Loch nam Madadh SAC. Available online at: https://sitelink.nature.scot/site/8301 (accessed December 18, 2018).
Sheehan, E. V., Bridger, D., and Attrill, M. J. (2015). The ecosystem service value of living versus dead biogenic reef. Estuar. Coast. Shelf Sci. 154, 248–254. doi: 10.1016/j.ecss.2014.12.042
Smale, D. A. (2020). Impacts of ocean warming on kelp forest ecosystems. New Phytol. 225, 1447–1454. doi: 10.1111/nph.16107
Steen, B. (2019). Modelling hot spot areas for the invasive alien plant Elodea nuttallii in the EU. Manag. Biol. Invasions 10, 151–170. doi: 10.3391/mbi.2019.10.1.10
Steller, D. L., Riosmena-Rodríguez, R., Foster, M. S., and Roberts, C. A. (2003). Rhodolith bed diversity in the Gulf of California: the importance of rhodolith structure and consequences of disturbance. Aquat. Conserv. Mar. Freshw. Ecosyst. 13, S5–S20. doi: 10.1002/aqc.564
Suggett, D. J., Camp, E. F., Edmondson, J., Boström-Einarsson, L., Ramler, V., Lohr, K., et al. (2019). Optimizing return-on-effort for coral nursery and outplanting practices to aid restoration of the great barrier reef. Restor. Ecol. 27, 683–693. doi: 10.1111/rec.12916
Sunday, J. M., Fabricius, K. E., Kroeker, K. J., Anderson, K. M., Brown, N. E., Barry, J. P., et al. (2017). Ocean acidification can mediate biodiversity shifts by changing biogenic habitat. Nat. Clim. Change 7, 81–85. doi: 10.1038/nclimate3161
Teagle, H., and Smale, D. A. (2018). Climate-driven substitution of habitat-forming species leads to reduced biodiversity within a temperate marine community. Divers. Distrib. 24, 1367–1380. doi: 10.1111/ddi.12775
The Council of the European Communities (1992). Council Directive 92/43/EEC of 21 May 1992 on the Conservation of Natural Habitats and of Wild Fauna and Flora. Available online at: https://eur-lex.europa.eu/legal-content/EN/TXT/?uri=CELEX:01992L0043-20070101 (accessed January 10, 2019).
Tyberghein, L., Verbruggen, H., Pauly, K., Troupin, C., Mineur, F., and Clerck, O. D. (2012). Bio-ORACLE: a global environmental dataset for marine species distribution modelling. Glob. Ecol. Biogeogr. 21, 272–281. doi: 10.1111/j.1466-8238.2011.00656.x
van der Heijden, L. H., and Kamenos, N. A. (2015). Reviews and syntheses: calculating the global contribution of coralline algae to total carbon burial. Biogeosciences 12, 6429–6441. doi: 10.5194/bg-12-6429-2015
Veloz, S. D. (2009). Spatially autocorrelated sampling falsely inflates measures of accuracy for presence-only niche models. J. Biogeogr. 36, 2290–2299. doi: 10.1111/j.1365-2699.2009.02174.x
Verbruggen, H., Tyberghein, L., Belton, G. S., Mineur, F., Jueterbock, A., Hoarau, G., et al. (2013). Improving transferability of introduced species’ distribution models: new tools to forecast the spread of a highly invasive seaweed. PLoS One 8:e68337. doi: 10.1371/journal.pone.0068337
Wernberg, T., Smale, D. A., and Thomsen, M. S. (2012). A decade of climate change experiments on marine organisms: procedures, patterns and problems. Glob. Change Biol. 18, 1491–1498. doi: 10.1111/j.1365-2486.2012.02656.x
Keywords: biodiversity, biogeography, climate change, Scotland, ecology, marine conservation, maerl, rhodolith
Citation: Simon-Nutbrown C, Hollingsworth PM, Fernandes TF, Kamphausen L, Baxter JM and Burdett HL (2020) Species Distribution Modeling Predicts Significant Declines in Coralline Algae Populations Under Projected Climate Change With Implications for Conservation Policy. Front. Mar. Sci. 7:575825. doi: 10.3389/fmars.2020.575825
Received: 24 June 2020; Accepted: 21 August 2020;
Published: 14 September 2020.
Edited by:
Jonathan Y. S. Leung, University of Adelaide, AustraliaReviewed by:
Cristina Pardo Carabias, University of A Coruña, SpainChiara Lombardi, Italian National Agency for New Technologies, Energy and Sustainable Economic Development (ENEA), Italy
Copyright © 2020 Simon-Nutbrown, Hollingsworth, Fernandes, Kamphausen, Baxter and Burdett. This is an open-access article distributed under the terms of the Creative Commons Attribution License (CC BY). The use, distribution or reproduction in other forums is permitted, provided the original author(s) and the copyright owner(s) are credited and that the original publication in this journal is cited, in accordance with accepted academic practice. No use, distribution or reproduction is permitted which does not comply with these terms.
*Correspondence: Cornelia Simon-Nutbrown, Y2NzM0Body5hYy51aw==