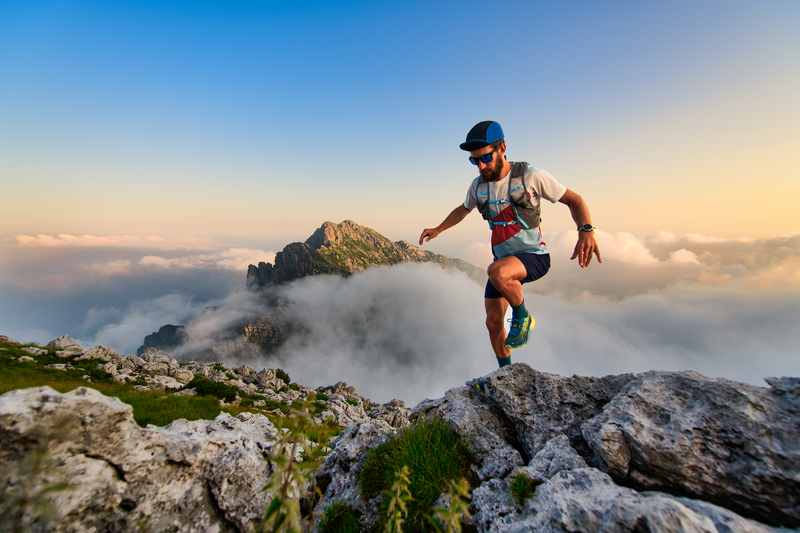
94% of researchers rate our articles as excellent or good
Learn more about the work of our research integrity team to safeguard the quality of each article we publish.
Find out more
ORIGINAL RESEARCH article
Front. Mar. Sci. , 22 October 2020
Sec. Marine Fisheries, Aquaculture and Living Resources
Volume 7 - 2020 | https://doi.org/10.3389/fmars.2020.568628
This article is part of the Research Topic Advances in the Biology, Aquaculture, and Conservation of Threatened Marine Species and their Application in Human Health and Nutrition View all 27 articles
This study evaluated two approaches to the aquaculture of Limulus polyphemus with the ultimate goal of harvesting Limulus amebocyte lysate (LAL) at an industrial scale. To monitor Horseshoe crabs (HSCs), a combination of physical, biochemical and cellular components were examined for HSC cohorts in an indoor recirculating aquaculture system (RAS) and an outdoor on-bottom pen culture system (PCS) over a 6-month period. The metrics included body weight, hemocyanin (Hc) concentration, amebocyte density, and LAL reactivity. In addition, a simulated biomedical bleeding event (extracting 30% of the total hemolymph volume) was performed to assess the impact on physiochemical properties of the hemolymph and amebocytes. Overall, the HSCs fared better in the RAS compared to the PCS, with higher rebound kinetics with respect to Hc, amebocyte density, LAL reactivity, and with 100% survival in the RAS cohort. Further, hemolymph reinfusion (after amebocyte removal) was shown to improve HSC recovery time. In summary, outcomes of this research show that a RAS, coupled with adequate nutrition and monitoring can provide HSCs with a suitable environment for sustainable hemolymph extraction and year-round LAL production.
The horseshoe crab (HSC) belongs to the order of arthropods, xiphosurans, dating back to the Ordovician period and are believed to be morphologically unchanged for some 148 million years (Rudkin and Young, 2009; Blazejowski, 2015; Manca et al., 2016; Akbar-John et al., 2018; Bicknell and Pates, 2020). Annually, several hundred thousand Atlantic HSCs (Limulus polyphemus) are collected from the wild along the Eastern shore of the United States for production of biomedical industry quality control tests. Upon harvesting amebocyte cells from HSC hemolymph or “blue blood,” a vital extract called Limulus amebocyte lysate (LAL) is used to ensure sterility of pharmaceutical and medical devices, worldwide. When combined with demand for HSCs as whelk and eel fishing bait, as well as climate change and consequent habitat erosion also threatening numerous migrating shorebird species, compounded by corresponding threats to Asian HSCs, alternative strategies to ensure sustainable supplies of LAL are increasingly vital.
Notwithstanding their distinction as “living fossils,” HSCs are of interest to the medical device and pharmaceutical industries given the importance of their blood (hemolymph), which contains a specialized cell, or amebocyte (Mürer et al., 1975; Van Holde and Miller, 1995; Rudkin et al., 2008). LAL extracted from the amebocytes contain clotting factors which catalyze a highly defined and immediate coagulation cascade when exposed to lipopolysaccharides (LPS) in the outer membranes of gram-negative bacterial endotoxins and/or fungal beta-glucans (Tai et al., 1977; Liu et al., 1979; Krisfalusi-Gannon et al., 2018). HSCs evolved with a form of “innate immunity” characterized by these granular amebocytes that can trigger extracellular clot formation (a polymer of protein coagulin) to act as an anti-microbial and anti-fungal barrier for the neutralization and immobilization of infectious pathogens (Shuster, 1990; Iwanaga et al., 1998; Medzhitov and Janeway, 2000; Iwanaga, 2002; Isakova and Armstrong, 2003). This immediate response to LPS and subsequent gel-clot formation has been employed in an assay using LAL, which has been considered the gold standard for quality assurance against pyrogens in the pharmaceutical and medical device industry since the 1970’s (Mikkelsen, 1988).
Currently, to obtain amebocytes for LAL extraction, HSCs are removed from their habitats, transported to secondary locations, bled, and then returned to the water. Given increasing international demand for LAL for the testing of biomedical products sold in markets requiring FDA compliance, these practices pose considerable uncertainties as to whether current harvesting levels are sustainable while meeting future requirements (Krisfalusi-Gannon et al., 2018). This demand has been fueled by rapid global growth in vaccines, pharmaceuticals and United States medical device markets (trending at 6–8% and 25% per annum, respectively; Novitsky, 2015; Smith et al., 2017). Bled for Asian and Pacific biomedical testing reagents, increasing threats to the Asian HSC species could also lead to increased international demand for American LAL or require industry use of other methods (Akbar-John et al., 2018). While testing alternatives such as recombinant factor C (rFC) have been introduced, the LAL assay has remained the most reproducible, sensitive, rapid, and accurate FDA-approved method for the detection and quantitation of LPS. Although the European health authorities have approved rFC as an alternative to the LAL assay, the United States Pharmacopeia has recommended more validation studies would be necessary before rFC can be considered equivalent to the LAL test. To date the FDA has denied approval of this alternative Bacterial Endotoxin Test (BET) as a standalone compendial standard. The LAL assay market has been entrenched by specifications in FDA guidance documents for nearly four decades, and quality standards for existing products have been based on the LAL test with rare exceptions (Hochstein, 1990). Accordingly, additional resources would be required for existing LAL users to adopt alternative methods. These hurdles represent a significant driver for industry to source LAL derived from an abundant, sustainable approach.
Beyond such demands, an immediate environmental consideration is the viability of various species of birds that depend on HSC eggs for fuel and nutrition during migration (Botton et al., 1994; Gillings et al., 2007; Krisfalusi-Gannon et al., 2018). Thus, the overall aim of this work was to develop a sustainable approach to address LAL supply by cultivating HSCs in captivity as an alternative to annual wild capture and the consequent ecological threats to HSCs and dependent species. Past captivity research was dissuasive and cited numerous reasons why the species would languish and die in captivity (Walls et al., 2002). However, such reports seemed counterintuitive, since HSCs have survived and adapted over millions of years in an almost unchanged form and appear readily adaptable (Kin and Blazejowski, 2014; Blazejowski, 2015; Bicknell et al., 2019). Nevertheless, the literature suggested that a natural habitat would satisfy the environmental needs of this species (Botton, 1984); therefore, a saltwater pond in Coastal Georgia was selected as an experimental location for an outdoor HSC habitat. A second cohort of animals were subsequently tested in an indoor aquaculture facility to compare the two cultivation models with respect to feasibility and practicability.
In summary, two approaches to HSC husbandry were evaluated by designing an indoor recirculating aquaculture system (RAS) and an outdoor on-bottom pen culture system (PCS). Water quality in both systems, as well as HSC health parameters were monitored [i.e., weight, hemocyanin (Hc) levels, amebocytes concentration, LAL reactivity, and survival]. Tests were performed to analyze the rebound kinetics of amebocytes and Hc levels after hemolymph extraction to help maximize LAL yield and maintain HSC vigor, allowing for more frequent, sustainable harvesting over the 6-month study.
Horseshoe crabs (n = 64) used in this study were procured manually, as bycatch from regional shrimpers, or purchased commercially from a sea life supplier (Dynasty Marine Associates, Marathon, FL, United States; NC Division of Marine Fisheries; and Georgia Department of Natural Resources) in accordance with an aquaculture operation permit (North Carolina Department of Environmental Quality, Division of Marine Fisheries #1946771 and #1947050; Georgia Department of Natural Resources LOP20180194). Mature HSCs used in this study on average weighed 1.77 ± 0.77 kg (3.91 ± 1.58 pounds). The salinity of both the indoor and outdoor culturing systems was consistent with the ambient salinities of where the HSCs were originally collected and/or maintained.
The HSC studies were conducted in a RAS (Clear Flow Aquaponic System, Nelson and Pade, Montello, WI, United States) as previously described (Tinker-Kulberg et al., 2020a, b; Figures 1A–C). The tanks were brought to a salinity of 20 to 22‰ using a commercial sea salt preparation (Crystal Sea®, Marine Enterprise International, Baltimore, MD, United States) and weekly saltwater exchanges were performed between 1 and 2%.
Figure 1. Indoor and Outdoor HSC culturing systems. (A) Recirculating aquaculture system (RAS; Greensboro, NC, United States). The temperature-controlled system is comprised of two flow-through saltwater aquaculture systems, each containing two raft tanks (one pair is outfitted with UV lights for sterility), and a hospital tank for extraction recovery. Open circles represent open valves, closed circles represent closed valves during normal operational procedures. Closed valves can be open for clean-out or for water temperature control. Photographs of (B) installed system and (C) HSCs in place. Tidelands pond pen culture system (PCS; Jekyll Island, GA, United States). Photographs of (D) enclosure location and (E) two of four pens positioned in Jekyll Island.
Target water-quality parameters were determined based on standard saltwater aquaculture methods, and comprehensive daily, weekly and monthly analyses were conducted as previously described (Timmons, 1994; Nolan and Smith, 2009; Schreibman and Zarnoch, 2009; Ebeling and Timmons, 2010; Shelley and Lovatelli, 2011; Tinker-Kulberg et al., 2020a, b). Briefly, daily water conditions were optimized as shown in Table 1 for: temperature (18.5–20°C); salinity (20–22‰); pH (7.5–8.2); dissolved oxygen (6–9 mgL–1); photoperiod (12-h of natural light); ammonia (0–1.5 ppm); nitrates (< 150 ppm); nitrites (2–5 ppm), and alkalinity (90–150 ppm). Water parameters were measured and adjusted as needed six times per week and did not vary significantly throughout the course of the study.
Table 1. HSC water quality and nutrient parameters comparisons between indoor (RAS) and outdoor (PCS) systems (value ranges throughout study).
In the indoor system, HSCs (n = 24) were provided a natural feed (e.g., glass minnows and krill; Aylesworth’s Fish and Bait, St. Petersburg, FL, United States) ad libitum and maintained as described previously (Tinker-Kulberg et al., 2020a). A health assessment was recorded for each HSC upon intake (i.e., sex, weight, carapace length/width, as well as an appendage, carapace, eye, and mouth evaluation). HSCs were stocked at a density of 0.454-kg (1.0-lb) per square foot, resulting in approximately one HSC per 4-ft3 of tank space. Each cohort (n = 6 per indoor tank) had the same number of males and female HSCs to avoid differences in feeding behavior or data bias.
An HSC outdoor PCS was designed using PVC pipe and crab trap netting and was located in a semi-tidal saltwater pond (Tidelands Pond, Jekyll Island, GA; 31° 2′ 19.2048″ N81° 25′ 12.8496″ W; ∼10.2 hectares). Designed to allow HSCs (n = 40) to naturally forage along the bottom of the salt marsh, four (4) outdoor PCS enclosures (15 ft long × 5 ft high × 5 ft wide) were erected to house HSCs at a density of 1 HSC per 6 ft2 (Figures 1D,E). Pens were positioned proximal to the shore, and they extended into the water to a minimum depth of 4 ft and staked to the bottom along the perimeter. The pens were separated by approximately 4 ft to support the availability of natural food and exchange of materials with the bottom, as well as adequate water flow through the cages to avoid formation of “dead zones” along the bottom. To decrease build-up of sediment pollution, one pen was left empty, and each week one HSC cohort was moved in a clockwise manner into the adjacent empty pen in succession, allowing the pen that previously held the HSCs to remain vacant over the ensuing week. The HSC cohorts in the outdoor PCS were fed ad libitum once per week on the same diet used in the indoor RAS study (e.g., glass minnows and krill; Aylesworth’s Fish and Bait, St. Petersburg, FL, United States). Due to the natural environmental limitations and in compliance with the governing ecological authorities, feeding was performed by hand outside of the PCS. These limitations represented a challenge with the experimental design, and the impact of such restrictions should be considered throughout the comparative analysis. Each cohort (n = 13–14 per outdoor enclosure) had the same number of males and female HSCs to avoid differences in feeding behavior or data bias.
The pH, ammonia, temperature, alkalinity, and carbon dioxide were recorded daily, while the remaining water parameters (i.e., nitrate, nitrite, calcium, hardness, and salinity) were conducted monthly at both aquaculture sites as shown in Table 1. Prior to hemolymph collection and periodically throughout the study, HSCs were weighed, measured (prosomal width and length from the tip to the tip of the telson) and inspected physically to determine whether the animals had sustained any injuries (i.e., to carapace, joints, eyes, and book gills), infections or other changes over time (Nolan and Smith, 2009).
The invertebrate benthic diversity of Tideland Pond was sampled from April-October 2019. Seven sites were sampled along the eastern shore of the pond near the HSC enclosures. Two of the sampling sites were culverts which connect the pond to the ocean. Kick sampling was conducted parallel to the eastern shore for 5 m in the direction of the current. One researcher held a D-net with 500 micron mesh against the bottom of the pond while another researcher dislodged benthic animals by shuffling their feet while moving toward the net. Specimens were preserved in 95% ethanol and identified to species or morphospecies if identification to species was not possible. Sampling was conducted every-other week or as often as weather conditions permitted. Alpha diversity was calculated using Simpson’s diversity index to give weight to more common species by using the formula Diversity = 1/Σ(pi)2 where pi is the relative abundance of the ith species.
Hemolymph was collected for amebocyte counting and for supernatant and amebocyte collection as described previously (Tinker-Kulberg et al., 2020a, b).
Hemolymph extraction for HSC “no bleed controls” (n = 4) and post-bleed health assessments to monitor recovery was carried out by removing 2.5 mL of hemolymph for analysis. To simulate a biomedical bleeding event, hemolymph volumes equivalent to a total of 30% of the total hemolymph were removed from HSCs (n = 4) and collected via syringe-free drip method directly into a pyrogen-free vial. Hemolymph volume of the HSCs was calculated as a percentage of wet body weight with 25 ± 2.2% for males and 25 ± 5.1% (mean ± SD) for females with the density of hemolymph estimated at 1.04 g/mL (0.0023-lbs per mL; Hurton et al., 2005).
For comparison, a subset of the HSCs (n = 4) were bled at 30%, amebocytes were pelleted and removed from the hemolymph by centrifugation (e.g., 1000-rcf for 5 min), and the amebocyte-free hemolymph (referred to as autologous supernatant, hereafter) was reinfused at different volumes back into the HSC. To do this, the autologous supernatant was transferred into an endotoxin-free 60 mL syringe (to maintain a closed system for reinfusion), and a standard evacuated tube/straight needle connected to tubing was then attached to the end of the syringe. The syringe was then placed in a calibrated pump, and the needle at the other end of the sterile tubing was inserted into the sterilized HSC pericardial membrane. The autologous supernatant was then reinfused into the HSC at a rate of 5,000 μL/min. Given the time and precision required for reinfusion, an apparatus was engineered that secured the HSC in place and gently pumped saltwater across its book gills throughout the procedure; within the inert polyethylene retention tub, an immobilizing block was affixed to prevent movement during reinfusion and to facilitate pericardial membrane access. A schematic and photographs of the reinfusion system are shown in Figure 2.
Figure 2. HSC reinfusion and immobilizing station. (A) The immobilizing block is comprised of two ventilated chambers attached perpendicularly and fitted with foam padding in a 24-gallon reinforced PTFE (polytetrafluoroethylene) tub with pipe to allow saltwater flow outward. A separate tube is positioned beneath the foam padding to allow flow over the HSC book gills (maintaining respiration rates) during the biomedical bleeding procedure. (B) Side-view of system displaying internal saltwater flow tubing to the HSC. Two photographs (C, D) of the hemolymph reinfusion process.
Total serum protein concentration was determined as described previously (Tinker-Kulberg et al., 2020a, b) via UV-Vis Spectrometer (Cary 6000i, Agilent Technologies, Santa Clara, CA, United States) and measured at 280 nm. Dioxygen-bound Hc (Oxy-Hc) was likewise measured at 340 nm. The total protein concentration was calculated according to Nickerson and Van Holde (1971), using the absorption coefficient A280 = 1.39 mg–1 ml–1 cm–1 for Hc and A350 = 0.223 mg–1 ml–1 cm–1 for Oxy-Hc. Amebocyte density was calculated as described previously (Tinker-Kulberg et al., 2020a, b).
To determine LAL reactivity to endotoxin, LAL was purified as described previously (Tinker-Kulberg et al., 2020a, b) and fractions were then tested for LAL reactivity against a commercial endotoxin standard (Escherichia coli 055:B5 lipopolysaccharide, Sigma Aldrich, St. Louis, MO, United States). Aliquots that had been frozen and thawed only once were used for comparison assays. Four different batches of commercial LAL (E-ToxateTM, Sigma Aldrich, St. Louis, MO, United States) were evaluated, yielding reproducible results likewise from freshly thawed commercial lysates in all LAL experiments.
A gel clot assay was performed by incubating a standard endotoxin solution (50 EU/mL) with varying concentrations of the LAL extracts (ranging from 25 to 75 μg) in a 96-well plate. The gel-clot was allowed to form for 1 h at 37°C. Results were measured using a microplate reader (BioTek 800, BioTek Instruments, Winooski, VT, United States) at 340 nm, and the relative absorbance readings between experiments were reported.
Data from 24 HSCs (procured from Georgia) were included in this study with 12 each in the respective indoor and outdoor systems. Data from indoor HSCs (n = 12) procured from Florida were also collected to serve as a control. Three groups of 4 HSCs served as: “No Bleed” controls, as “30% Bleed” controls, and “30% Bleed + Reinfusion” controls in each system. Each data point reported represents the combined data from four of the HSCs in each system (indoor versus outdoor); thus, the unit is equivalent to the average data collected from each HSC cohort and not the individual crabs, unless otherwise noted. All data points represent the mean ± standard error, where n equals the number of HSCs in each data subset. Data were subjected to a one-way analysis of variance (ANOVA) to determine significant differences among groups. If a difference was determined, it was compared by a Student’s t-test, assuming equal variance, with a significant difference set at p ≤ 0.05.
As shown in Table 1, both the indoor and outdoor systems revealed similar water quality parameters, with only temperature and DO (dissolved oxygen) differing over the course of the study in the outdoor system (Figure 3). In the indoor RAS, water temperature varied between 17.1–21.7°C and DO between 6.3–11.1 mgL–1 across all tank systems using supplemental aeration, without discernable trends appearing over time (Figures 3A,C). The temperature in the outdoor system increased steadily (∼15°C) over the course of the study due to seasonal fluctuations and consequently, the DO varied between 3.7–7.6 mgL–1 (supplemental aeration was not utilized in the outdoor system), with a subtle, yet concurrent, decrease over time (Figures 3C,D).
Figure 3. Key metrics for RAS (Greensboro, NC) and PCS (Jekyll Island, GA). Areas within the green bars indicate safe ranges for temperature (°C) and dissolved oxygen (mgL–1), yellow or red bars and shading indicate caution or danger levels, respectively (Table 1). Graphs represent temperature and dissolved oxygen for RAS (A, B) and for PCS (C, D) installations. X: Indicates each horseseho crab (HSC) that expired during the study.
The indoor system yielded greater viability with survival of all HSCs over the course of the study. The outdoor system, however, recorded a loss of 15% of the HSCs over a 3-month period, which was concurrent with high water temperatures and lower levels of DO, with an initial loss of the outdoor HSCs occurring prior to any non-health bleeding events (Figures 3C,D).
All HSCs were physically inspected prior to introduction into the study and at various intervals over the course of this research. HSCs included in both the indoor and outdoor systems were deemed acceptable for inclusion in the study based on physical characteristics (free of crush fractures or open wounds); and any arthropod (e.g., barnacles) or mollusk growth was carefully removed using a hard bristle brush without damaging the shell. For all animals included in the RAS cohort, any cosmetic observations noted at the beginning of the study were monitored and remained unchanged over the course of the study. However, HSCs in the PCS accumulated a layer of biofilm and discoloration on their shells, without apparently affecting their health.
All HSCs were mature and no longer prone to molting, and thus their growth rate was consistent over the course of the study (data not shown). The weight of HSCs not subjected to a bleeding regimen was recorded and also remained constant in both the indoor and outdoor cohorts (Table 2).
Although HSC weight did not vary throughout the study in the RAS or PCS groups (Table 2), mortality occurred in the outdoor PCS cohort with a recorded loss of 15%. Behavior and health parameters of indoor HSCs (n = 12) procured from Florida were indistinguishable from that of HSCs procured from Georgia, and therefore data from the latter were only reported in this study.
The following invertebrate species identified as potential natural HSC feed sources in Tideland pond were indexed: Cancer irroratus (Atlantic rock crab); Dosinia discus clam; Geukensia demissa (ribbed mussel); Mnemiopsis leidyi (sea walnut comb jelly); Molgula manhattensis (sea grape tunicate); Neopetrolisthes maculatus (spotted porcelain crab); Palaemonetes pugio (grass shrimp); Penaeus setiferus (white shrimp); Petrolisthes armatus (porcelain crab); Phrontis vibex (Bruised nassa snail); Styela plicata (rough tunicate); Tritia obsolete (Eastern mud snail); Tritia trivittata (threeline mudsnail); and Tubeworms (species unknown). Invertebrate diversity decreased over the summer as shown in Table 3. The diversity index takes into account the number of species in a habitat but also the relative abundance of each species (i.e., how many of each species are sampled). The higher the index, the greater the diversity of species were collected.
Hemocyanin concentration was tracked in HSCs maintained in both the PCS and the RAS. Results shown in Figure 4 revealed that Hc concentrations remained stable in the HSC “No Bleed” RAS cohort, with a slight, but not statistically significant decrease over time (48.9 ± 11.13 mg/mL to 37.1 ± 9.85 mg/mL). The mean concentration observed in this group was within the range of values observed in wild caught HSCs from past studies (i.e., 32–97 mg/mL; Smith et al., 2002; James-Pirri et al., 2012). In contrast, a significant decrease was observed in HSCs in the “No Bleed” control group maintained in the outdoor environment, with an absolute decrease from 61.1 ± 9.45 mg/mL to 11.5 ± 9.45 mg/mL at the culmination of this study. Similarly, the proportion of dioxygen bound Hc (i.e., Oxy-Hc), an indicator of health status, ranged from 60 to 76% across all assays in both the indoor and outdoor systems. Statistically significant effects were not observed in either control group.
Figure 4. Hemocyanin concentrations in the HSC “No Bleed” control group cultured in RAS and PCS. (A) HSC Hemocyanin (Hc) Concentrations in RAS and PCS. Hc concentrations were unchanged in the RAS but decreased over time in PCS cohorts. (B) Percent Hc with Dioxygen Bound (Oxy-Hc) in RAS and PCS. Hc concentration and Oxy-Hc remained stable in HSCs in the RAS but decreased over time for those in the PCS. Results are represented as mean ± S.E. (n = 4); bars sharing different letters in each index are significantly different (P < 0.05). *, ** represent significance (p < 0.05) between groups.
Amebocyte concentrations in hemolymph from HSCs in both systems were monitored to assess immune function, as decreases had been observed among stressed HSCs (Coates et al., 2012). Using a standard hemocytometer and cell counting technique, results showed that amebocyte density remained consistent in “No Bleed” HSCs maintained in the RAS; whereas, amebocytes decreased slightly over time in HSCs maintained in the outdoor PCS (Figure 5).
Figure 5. Amebocyte density in the HSCs “No Bleed” control group in RAS and PCS. Amebocyte concentrations were stable for the RAS cohort, but decreased over time in the PCS HSCs. Results are represented as mean ± S.E. (n = 4); bars sharing different letters in each index are significantly different (P < 0.05). *, ** represent significance (p < 0.05) between groups.
Changes in LAL reactivity to endotoxins from the respective aquaculture settings were directly analyzed. Pooled LAL extracts from 4 individual HSCs in the “No Bleed” control groups reared in either the PCS or RAS were sampled for LAL reactivity (Figure 6). Results showed that gel-clot formation increased as LAL concentrations increased in both the indoor and outdoor cohorts, which was consistent with the commercial control (E-ToxateTM, Sigma Aldrich, St. Louis, MO, United States). Additionally, LAL extracts from both the indoor and outdoor cohorts, exposed to higher endotoxin concentrations (e.g., 25 and 75 μg), had higher levels of reactivity when compared with that of the commercial control (E-ToxateTM, Sigma Aldrich, St. Louis, MO, United States).
Figure 6. LAL reactivity of HSCs in the “No Bleed” control group in RAS and PCS. LAL reactivity of the RAS and PCS HSC cohorts compared to control (commercial kit LAL; E-ToxateTM, Sigma Aldrich, St. Louis, MO, United States) at increasing LAL concentrations (25–75 μg protein) exposed to 50 EU/mL of LPS. Reactivity of LAL derived from RAS and PCS HSCs were consistent with commercial LAL (E-ToxateTM, Sigma Aldrich, St. Louis, MO, United States). Results represented as mean ± S.E. (n = 4).
To determine if hemolymph rebound rates after simulated biomedical bleeding differed in indoor and outdoor systems, HSCs were separated into a control (No Bleed) group; those from which 30% of the hemolymph was extracted (30% bleed); and those with 30% extracted, followed by reinfusion of autologous supernatant (i.e., amebocyte-free hemolymph; 30% bleed + reinfusion). Hc concentration and amebocyte densities were assessed just prior to bleeding, 72 h after, and 7 days following the procedure.
Notably, the weight of all HSCs decreased after a 30% hemolymph extraction (without reinfusion) and recovered to baseline within 72 h. As expected, serum protein concentrations and amebocyte density decreased initially during this period, presumably due to a dilution effect caused by the animal replacing its lost hemolymph with interstitial fluid. As expected, Hc concentrations were more stable after 30% of the hemolymph was removed when followed by reinfusion of autologous supernatant (i.e., Hc restored); a decrease in amebocyte density was also seen due to the dilution effect during this 72-h window (data not shown). Results, presented as a function of the percent change before and after bleeding, showed that HSCs reared in the indoor environment showed improved rebounding based on Hc (Figures 7A,C; 30% Bleed) and amebocyte concentrations (Figures 7B,D; 30% Bleed) 7 days after the 30% bleed compared to the outdoor cohort.
Figure 7. Effect of 30% hemolymph extraction and autologous supernatant reinfusion on hemocyanin (Hc) and amebocyte density in HSCs in RAS and PCS. Note: black bars = with reinfusion; blue bars = without reinfusion. RAS: (A) The percent change in serum protein concentration, measured by Hc and (B) in amebocyte density, 7 days after 30% bleed (n = 4) with and without hemolymph reinfusion. PCS: (C) Percent change in Hc and (D) amebocyte density, 7 days after 30% bleed (n = 4) with and without reinfusion.
However, 7 days after HSCs were bled at 30% and immediately reinfused with a portion of autologous supernatant, Hc concentration rose (∼10%) above pre-bleed baselines (Figure 7A). After this 7-day recovery period, amebocyte density increased ∼31% after reinfusion of the RAS cohort (Figure 7B). Results from the outdoor study were similar; with reinfusion resulting in a higher rebound of Hc (∼4.5%; Figure 7C) and circulating amebocytes surging almost 25% following reinfusion at 7 days post-recovery (Figure 7D).
With respect to LAL reactivity before and after bleeding, reinfusion also appeared to have enhanced LAL reactivity when compared to that of HSCs that were not reinfused (Figure 8). However, after hemolymph removal with or without reinfusion, the average LAL reactivity across the indoor HSC groups decreased below baselines, despite increasing amebocyte counts. All HSCs tolerated bleeding in both the RAS and PCS groups.
Figure 8. Effect of 30% hemolymph extraction and autologous supernatant reinfusion on LAL reactivity. Average LAL reactivity from amebocytes extracted at Day 0 and a week after 30% bleed (Day 7). Reactivity of a commercial LAL control (E-ToxateTM, Sigma Aldrich, St. Louis, MO, United States) is represented by a dashed line. Results are represented as mean ± S.E. (n = 4).
In the wild, HSCs consume a diverse array of prey in their natural habitat (Botton, 1984; Botton and Ropes, 1989; Hu et al., 2013; Kin and Blazejowski, 2014). Although information concerning the natural diet of HSCs has been reported (Botton, 1984), dietary requirements under natural pond-farming systems and how natural food supplies impact overall health have not been previously established. While macronutrient and caloric densities of feed for aquatic species can be matched in captivity, compositional disparities exist, which was illustrated in bottlenose dolphin research showing a lack of diversity in the diet contributes to poor nutrition and health (Barros and Wells, 1998; Cunningham-Smith et al., 2006; Berens-McCabe et al., 2010; Powell and Wells, 2011). In the case of HSCs, the most abundant food source in the wild may not be the most suitable feed source for aquaculture (Botton, 1984; Hu et al., 2013). Likewise, the nutritional requirements of HSCs in captivity based on controlled feeding trials have not yet been defined (Botton, 1984; Carmichael and Brush, 2012). Inadequate nutrition and poor water quality (due to high stocking density) can also contribute to HSC stress, increasing susceptibility to pathogens and vulnerability to captivity-induced disease (Kautsky et al., 2000; Carmichael et al., 2003; Smith and Berkson, 2005; Defoirdt et al., 2007; Nolan and Smith, 2009; Schreibman and Zarnoch, 2009; Kwan et al., 2014).
The objective of this research was aimed at comparing an indoor RAS and an outdoor PCS for sustainable LAL production. The initial hypothesis was that HSCs raised in an outdoor PCS with access to natural sources of feed, as well as their exposure to tidal rhythms and circadian light cycles approximating their wild habitat, would fare better than HSCs reared in an indoor RAS (Shuster, 1982; Barlow et al., 1986; Botton and Ropes, 1989). However, the results of the studies suggest that indoor RAS cohorts demonstrated more vigor across all health parameters, with significant monitoring, access and management advantages over the PCS approach.
Nutrition and farm maintenance strategies will undoubtedly play a critical role in HSC immunocompetence and disease resistance. For outdoor farming strategies, HSCs need to forage along the bottom of the pond; therefore, PCS enclosures could not float and instead needed to be fixed in place. This presented two major health problems with respect to depletion of natural feed sources in confined bottom surface areas and waste build-up, as well as difficulty in animal collection. The labor involved in HSC collection was complicated by the depth and murkiness of the water which made it difficult to find and retrieve the animals, especially considering their ability to burrow into the sediment that created a suction-like force or vacuum around the shell and the bottom of the sea floor. The unexpected difficulty in retrieving HSCs from such outdoor PCS traps for frequent bleeding and routine health maintenance checks made this an unsuitable aquaculture approach for LAL production. In addition, outdoor (PCS) approaches between different farming operations could vary depending on where and how HSC are maintained in the outdoor facility in relation to water quality, culture density, feed availability, natural waste build-up and removal strategies, and other abiotic environmental variables such as seasonal temperatures. In fact, earlier research showed batch-to-batch seasonal variations in LAL prepared from wild harvested populations in their sensitivity to LPS, which may be related to HSC health and diet at varying times of the year (i.e., after, during, or before spawning), as well as conditions in the surrounding ecosystem (Lindberg et al., 1972). Thus, HSCs cultured in an indoor RAS controlled diets and environmental conditions should reduce these batch-to-batch variations that might be expected in HSCs cultured in outdoor PCS or retrieved from the wild. HSCs maintained in RAS after the bleeding process may also provide more protection during this recovery period; whereas, bled HSCs returned to the wild may be more susceptible to disease from external influences related to poor water quality and diet (Rudloe, 1983; Hurton et al., 2009; Anderson et al., 2013).
Due to existing nature conservation, water policy/legislation and environmental constraints on the selected outdoor study site, the ability to match the feeding regimen of the indoor system was limited. Alterations to the habitat were also restricted; thus, additional aeration was not incorporated. The mortality in the outdoor system was attributed to diurnal and seasonal fluctuations in water temperatures over the hotter summer months (June-July). This was consistent with past results showing that higher temperatures result in poorer HSC hemolymph quality and lower survival rates (Coates et al., 2012). A lack of feed diversity in the diet during the summer months (June-Aug; Table 3) may have also contributed to poor nutrition and consequent mortality. Although the HSCs in the PCS were given supplemental feed weekly, the loss of 6 HSCs was not attributed to starvation because the HSCs in that cohort rejected more frequent feeding events (>1X per week when fed ad libitum). However, the actual available feed intake, caloric density, and nutrient complexity of the natural feed was not examined at the pond foraging HSC site and should be considered further when evaluating differences between the two systems.
Evidence from this study suggest that differences in environmental conditions between outdoor PCS and indoor RAS bring about major changes in terms of HSC health performance and vitality. Further studies and research would be required to determine whether supplemental feed and presentation strategies could help address the nutritional needs and overall health of pond-cultured HSCs without deleterious effects on water quality (i.e., waste build-up). However, other uncontrollable environmental factors such as fluctuating seasonal temperatures can also directly influence oxygen and food consumption, as well as natural feed (invertebrates) populations, affecting overall HSC health. Appropriate site selection for pond-based PCS farms would need to be evaluated to ensure that optimal seasonal water and feed conditions could offer economic advantages over environmentally controlled indoor RAS practices (e.g., reduced feed, infrastructure, and operational costs). Further, hemolymph quality, as assessed by Hc concentration and amebocyte density, was shown to be consistent over the course of the 6-month study in HSCs maintained in the RAS. In contrast, the HSC mortality, coupled with declining Hc and amebocyte concentrations in the outdoor study, suggested that this approach was suboptimal for husbandry and therefore, LAL production.
LAL reactivity to an endotoxin standard (measured by the opacity of gel-clot) was similar in the indoor and outdoor HSC “No Bleed” test cohorts. LAL reactivity was also higher in LAL prepared from husbanded HSCs compared to commercially available LAL preparations (E-ToxateTM, Sigma Aldrich, St. Louis, MO, United States); although additional studies would be needed to identify and assess possible variables in reagent preparation versus the harvest, freezing and single-thaw methods herein (Figure 6). These results also indicate that husbandry systems and nutrition influence the ratio and/or biochemical properties of LAL protein factors (Tinker-Kulberg et al., 2020a, b).
The impact and long-lasting effects of biomedical bleeding and handling stress on hemolymph properties of HSCs has not been fully characterized. The traditional LAL extraction procedure involves capturing HSCs during the spawning season, ambient exposure during transport and containment of the animal up to 72 h at the biomedical facility, and substantial blood loss before HSCs are returned to the point of capture, resulting in 15–30% mortality or long-term health sequalae (Atlantic States Marine Fisheries Commission (ASMFC), 1998; Leschen and Correia, 2010; Anderson et al., 2013). Past studies addressing rebound kinetics of blood components after extensive bleeding have shown that HSCs regain their blood volume within 1–3 days after a 30% bleeding extraction, but hemolymph protein concentration (e.g., 90% being Hc) takes 2–6 weeks to return to normal; whereas, restoration of amebocytes takes up to 4 months (Rudloe, 1983; Novitsky, 1984; James-Pirri et al., 2012). In comparison, Hufgard (2012) showed that amebocyte density can be recovered after 2 weeks within ten percent of the baseline value following significant hemolymph removal, and that the new amebocytes are smaller than fully mature and degranulating amebocytes.
The effect of biomedical bleeding of 30% of total HSC hemolymph volume was studied in both RAS and PCS to determine the rebound kinetics of Hc and amebocyte concentration after a 7-day recovery period (Figure 7). In contrast to prior studies, HSCs immediately regained their weight within hours after a 30% bleed extraction presumably through water sequestration from the recovery tank, a normal phenomenon used by aquatic animals to regulate their volume and ion concentrations (Rudloe, 1983; Novitsky, 1984; James-Pirri et al., 2012). As expected, Hc levels were not fully recovered within 7 days after being bled in the absence of supernatant reinfusion, however, in comparison to prior studies the amebocyte counts were fully restored within this 1-week period. HSCs that received reinfusion of the autologous supernatant (amebocyte-free hemolymph) showed increased serum protein levels and amebocyte counts above baseline levels (Figure 7). Further studies have shown similar results when two successive 10% bleeds, separated by a 1-week recovery period, were performed on indoor cultured HSCs (Tinker-Kulberg et al., 2020b). This rapid upregulation of Hc and circulating amebocytes above baseline levels may be a physiologic response to compensate for natural blood loss and injury (Hufgard, 2012).
Notably, although amebocyte levels rebounded 1 week after the 30% bleeding, LAL reactivity did not, with or without reinfusion. Thus, during amebocyte biosynthesis and regeneration, immature amebocytes may contain lower concentrations of LAL components (i.e., lower reactivity per μg of LAL); and as ameboctyes mature, maximum LAL reactivity would presumably be reached. Although HSCs maintained in the RAS and PCS demonstrated similar Hc and amebocyte rebound kinetics, a slight improvement was observed in RAS HSCs. In summary, reinfusion appears to enhance post-bleed recovery, which would allow captive HSCs to be bled more often if routinely reinfused, and high LAL yields may be obtained if newly formed amebocytes are allowed to mature.
Reinfusion of supernatant after hemolymph collection and amebocyte extraction appeared to be not only salutary for HSC recovery, but it also establishes an entirely new method in HSC bleeding and management. The commercially vital component of HSC hemolymph comprises 5–10% of overall hemolymph volume, leaving some 90% as waste in current industry practices, and its loss may contribute to subsequent mortality and deleterious effects after being returned to the water (Anderson et al., 2013). That is, reinfusion to restore hemolymph volume and oxygen transfer could prove beneficial to the HSCs by reducing the risks of hypoxia while accelerating recovery of metabolic homeostasis and amebocyte rebound; and therefore, allow for more frequent hemolymph collection. Further validation of HSC aquaculture could yield significant industry disruption of current practices, given that a small, captive cohort of HSCs could be sustainably bled up 12 times per year with 100% survival in an industry with 30% mortality resulting from random wild capture and bleeding just once per year. Further studies to determine the precise relationship between maximum threshold levels of bleeding and recovery time required for optimal amebocyte and Hc rebound kinetics will be particular useful to safeguard against such post-bleeding mortality in HSCs.
Finally, with respect to outdoor aquaculture as a sustainable means of producing LAL, and notwithstanding these findings, the labor and logistics associated with accessing the outdoor cultivated HSCs for bleeds and health assessments proved a significant challenge throughout the course of this work. Despite the custom design of an inshore pen system, the labor and time associated with swimming/wading to retrieve the HSCs (via tags affixed to the HSC shell), were especially problematic aspects of the outdoor system. The indoor system, on the other hand, proved to be conducive to regular handling of the HSCs, with the potential for more frequent, low-impact hemolymph harvesting. Outdoor pond-culturing systems would also be highly variable in abiotic and biotic factors. While seasonal changes in salinity and temperature in an outdoor system are inevitable, there is a greater degree of control in the indoor system and therefore, reproducibility. Thus, while there were indeed slight differences in specific water quality parameters between our indoor and outdoor system (Table 1), this study was aiming to assess how those differences might affect HSC health and hemolymph quality. As such, irrespective of the outdoor limitations, indoor RAS husbandry provided greater environmental control (e.g., DO, temperature, and water chemistry) and ease of operation that would be more practicable for year-round LAL production.
The effects and optimization of diet and different bleeding volumes and regimens, coupled with optimal recovery times, on HSC health and LAL production from HSCs raised in an indoor RAS have been recently reported (Tinker-Kulberg et al., 2020a). The use of intravascular catheters surgically implanted in the respective pericardial membranes of the HSCs, which allowed for routine hemolymph collection without repetitive membrane punctures, has also been recently investigated (Tinker-Kulberg et al., 2020b). Notably, these additional strategies would help to ensure wellbeing of HSC in captivity for optimal LAL production in indoor culturing systems.
During this study, HSC culture conditions, health parameters, collection and processing SOPs for both indoor and outdoor aquaculture systems were established. The process was designed to establish an alternative, sustainable LAL source to address current and projected issues that face wild HSC populations, the global biomedical industry demands, and the patients that depend on safe products. Indoor HSC aquaculture was proven practicable by demonstrating that indicators of well-being, including weight, Hc concentrations, amebocyte levels, and LAL reactivity remained constant; however, the outdoor PCS in this study posed overarching regulatory, environmental and accessibility challenges and yielded inferior results across all parameters, including HSC survival. Additional application of the indoor RAS method and HSC catheterization, combined with nutritional optimization could therefore serve to enhance HSC wellbeing and allow for sustainable, year-round harvesting of LAL, a wholly unique and globally vital resource. Once thoughtful innovation and stewardship are amalgamated into LAL production, the discussion should move toward integrated aquaculture of the species to ensure sustainability for both HSC and human medicine.
The raw data supporting the conclusions of this article will be made available by the authors, without undue reservation.
The research on the HSCs (Limulus polyphemus) involved in this study did not require approval, as HSCs are classified as an exempt invertebrate species. For RAS experiments (Greensboro, NC), all HSC collection and aquaculture techniques and methods were reviewed and approved by the North Carolina Department of Environmental Quality, Division of Marine Fisheries (Collection permit #1946771 and Aquaculture operation permit #1947050). For PCS experiments (Brunswick and Jekyll Island, GA), all HSC collection and aquaculture techniques were performed by University of Georgia Marine Extension and Georgia Sea Grant with approval from the Jekyll Island Authority Committee Members, Director of Conservation, and Georgia Department of Natural Resources (Georgia Department of Natural Resources LOP20180194).
RT-K, KD, TB, MG, CK, LT, and AD conceived the research hypothesis. RT-K, KD, TB, MG, CK, LT, and AD developed the research objectives and experimental plans. RT-K, KD, and TB verified the materials, methods and design of the RAS. RT-K, KD, LR, and AD conducted the RAS research experiments. RT-K, KD, BF, and LG designed and deployed the PCS system. BF, LG, IS, and CW collected, maintained, monitored HSCs, and performed the outdoor studies on Jekyll Island, with oversight from RT-K and KD. DS collected the data on the group composition of invertebrate samples from Tideland Pond. KD supervised all of findings of this work. MG and AD performed the final editing of the manuscript for publication. All authors prepared analysis of the primary research and contributed to the final writing of the manuscript.
The authors would like to acknowledge the following funding sources: National Science Foundation Small Business Innovation Research Grant Program (Grant # 1819562), North Carolina Board of Science & Technology, One North Carolina SBIR Matching Funds Program, and North Carolina Sea Grant (Grant # 2017-R/MG-1712).
RT-K, AD, LR, TB, and LT are employed by Kepley BioSystems, Inc. CK is a founding member of Kepley BioSystems. KD is a former employee, who was working for Kepley BioSystems during the experimental planning, data acquisition, and analysis portions of the project. MG was employed by the company ClienTell Consulting, LLC.
The remaining authors declare that the research was conducted in the absence of any commercial or financial relationships that could be construed as a potential conflict of interest.
The authors would like to thank Ben Carswell and Joseph Colbert from the Jekyll Island Authority, as well as Brianna Marquez, Johan Rigdon from the College of Coastal Georgia, and Dawn Zenkert and Corinne Zelie from the Georgia 4-H Tidelands Nature Center for their assistance throughout this research. Special acknowledgment to LT for his key role in establishing the collaboration between the Georgia Department of Natural Resources, Jekyll Island, GA, United States, and Kepley BioSystems, Inc., for the purpose of this study. They thank their peers and collaborators at Gateway Research Park for designing a facility to aquaculture HSCs in a RAS environment. They would like to express appreciation to Dr. Ben Moorman for his assistance in helping engineer the RAS system for HSC aquaculture. They are grateful for the guidance and support from Dr. Terry Norton of the Georgia Sea Turtle Center and to the Ecological Research & Development Group (Mayor Glenn Gauvry) for assistance and introduction to the broader, international HSC aquaculture research cohorts. They also thank the Joint School of Nanoscience and Nanoengineering for providing access to their facilities to conduct the research (Nanomanufacturing Innovation Consortium). They also appreciate the hard work of all of the members and collaborators at the University of Georgia Marine Extension and Georgia Sea Grant at Brunswick, GA, United States, including Captain Patrick Griffin and crew of the R/V Georgia Bulldog. They also thank Lauren Perdue, a dedicated senior at Northeast Guilford High School (McLeansville, NC, United States), for her daily help in measuring aquaculture system water parameters and HSC feeding.
‰, Parts per thousand; BET, Bacterial endotoxin test; ° C, Celsius; ANOVA, Analysis of variance; DO, Dissolved oxygen; HSC, Horseshoe crab; EC, Electric conductivity; EU, Endotoxin unit; FDA, Food and Drug Administration; ft, Feet; Hc, Hemocyanin; LAL, Limulus amebocyte lysate; lb, pound; LPS, Lipopolysaccharide; NaCl, Sodium Chloride; NEM, N-ethylmalemide; nm, Nanometer; OD, Optical density; Oxy-Hc, Oxyhemocyanin; PCS, Pen culture system; ppm, Parts per million; PTFE, polytetrafluoroethylene; RAS, Recirculating aquaculture system; rcf, Relative centrifugal force; rFC, Recombinat Factor C; SD, Standard deviation; SE, Standard Error; SOP, Standard operating procedure; Tris–HCl, Tris(hydroxymethyl)aminomethane hydrochloride; μ, Micro; UV-Vis, Ultraviolet-visible; v/v, Volume/volume.
Akbar-John, B., Nelson, B. R., Sheikh, H. I., Cheung, S. G., Wardiatno, Y., Dash, B. P., et al. (2018). A review on fisheries and conservation status of Asian horseshoe crabs. Biodiver. Conserv. 27, 3573–3598. doi: 10.1007/s10531-018-1633-8
Anderson, R. L., Watson, W. H. III, and Chabot, C. C. (2013). Sublethal behavioral and physiological effects of the biomedical bleeding process on the American horseshoe crab, Limulus polyphemus. Biol. Bull. 225, 137–151. doi: 10.1086/bblv225n3p137
Atlantic States Marine Fisheries Commission (ASMFC) (1998). Interstate Fishery Management Plan for Horseshoe Crab. Fishery Management Report No. 32 of the Atlantic States Marine Fisheries Commission. Available online at: https://www.asmfc.org/uploads/file/hscFMP.pdf (accessed March 12, 2019).
Barlow, R. B. Jr., Powers, M. K., Howard, H., and Kass, L. (1986). Migration of Limulus for mating: relation to lunar phase, tide height, and sunlight. Biol. Bull. 171, 310–329. doi: 10.2307/1541674
Barros, N. B., and Wells, R. S. (1998). Prey and feeding patterns of resident bottlenose dolphins (Tursiops truncatus) in Sarasota Bay, Florida. J. Mammal. 79, 1045–1059. doi: 10.2307/1383114
Berens-McCabe, E. J., Gannon, D. P., Barros, N. B., and Wells, R. S. (2010). Prey selection in a resident common bottlenose dolphin (Tursiops truncatus) community in Sarasota Bay, Florida. Mar. Biol. 157, 931–942. doi: 10.1007/s00227-009-1371-2
Bicknell, R. D. C., Lustri, L., and Brougham, T. (2019). Revision of “Bellinurus” carteri (Chelicerata: Xiphosura) from the Late Devonian of Pennsylvania, USA. Comptes Rendus Palevol. 18, 967–976. doi: 10.1016/j.crpv.2019.08.002
Bicknell, R. D. C., and Pates, S. (2020). Pictorial Atlas of fossil and extant horseshoe crabs, with focus on xiphosurida. Front. Earth Sci. 8:98. doi: 10.3389/feart.2020.00098
Blazejowski, B. (2015). The Oldest Species of the Genus Limulus from the LATE Jurassic of Poland: Changing Global Perspectives on Horseshoe Crab Biology, Conservation and Management. Cham: Springer, 3–14. doi: 10.1007/978-3-319-19542-1_28
Botton, M. L. (1984). Diet and food preferences of the adult horseshoe crab Limulus polyphemus in Delaware Bay, New Jersey, USA. Mar. Biol. 81, 199–207. doi: 10.1007/bf00393118
Botton, M. L., Loveland, R. E., and Jacobsen, T. R. (1994). Site selection by migratory shorebirds in Delaware Bay, and its relationship to beach characteristics and abundance of horseshoe crab (Limulus polyphemus) eggs. Auk 111, 605–616. doi: 10.1093/auk/111.3.605
Botton, M. L., and Ropes, J. W. (1989). Feeding ecology of horseshoe crabs on the continental shelf, New Jersey to North Carolina. Bull. Mar. Sci. 45, 637–647.
Carmichael, R. H., and Brush, E. (2012). Three decades of horseshoe crab rearing: a review of conditions for captive growth and survival. Rev. Aquacult. 4, 32–43. doi: 10.1111/j.1753-5131.2012.01059.x
Carmichael, R. H., Rutecki, D., and Valiela, I. (2003). Abundance and population structure of the Atlantic horseshoe crab Limulus polyphemus in Pleasant Bay, Cape Cod. Mar. Ecol. Prog. Ser. 246, 225–239. doi: 10.3354/meps246225
Coates, C. J., Bradford, E. L., Krome, C. A., and Nairn, J. (2012). Effect of temperature on biochemical and cellular properties of captive Limulus polyphemus. Aquaculture 334, 30–38. doi: 10.1016/j.aquaculture.2011.12.029
Cunningham-Smith, P., Colbert, D. E., Wells, R. S., and Speakman, T. (2006). Evaluation of human interactions with a provisioned wild bottlenose dolphin near Sarasota Bay, Fl, and efforts to curtail the interactions. Aquatic Mammals 32, 346–356. doi: 10.1578/AM.32.3.2006.346
Defoirdt, T., Boon, N., Sorgeloos, P., Verstraete, W., and Bossier, P. (2007). Alternatives to antibiotics to control bacterial infections: luminescent vibriosis in aquaculture as an example. Trends Biotechnol. 25, 472–479. doi: 10.1016/j.tibtech.2007.08.001
Ebeling, J. M., and Timmons, M. B. (2010). Recirculating Aquaculture. Ithaca, NY: Cayuga Aqua Ventures.
Gillings, S., Atkinson, P. W., Bardsley, S. L., Clark, N. A., Love, S. E., Robinson, R. A., et al. (2007). Shorebird predation of horseshoe crab eggs in Delaware Bay: species contrasts and availability constraints. J. Anim. Ecol. 76, 503–514. doi: 10.1111/j.1365-2656.2007.01229.x
Hochstein, H. D. (1990). “Role of the FDA in regulating the Limulus amoebocyte lysate test,” in Clinical Applications of the Limulus Amoebocyte Lysate Test, ed. R. B. Prior (Boca Raton, CA: CRC Press), 37–50.
Hu, M., Wang, Y., Cheung, S. G., and Shin, P. K. (2013). Comparison of different frozen natural foods on survival and growth of juvenile Chinese horseshoe crab Tachypleus tridentatus (Leach, 1819): Implications on laboratory culture. Aquacult. Res. 44, 567–573. doi: 10.1111/j.1365-2109.2011.03059.x
Hufgard, J. R. (2012). Amebocyte Diameter and Density After Partial Exsanguinations in Limulus Polyhemus Hemolymph. Terre Haute, IN: Rose-Hulman Institute of Technology Research Publications.
Hurton, L., Berkson, J., and Smith, S. (2005). Estimation of total hemolymph volume in the horseshoe crab Limulus polyphemus. Mar. Freshw. Behav. Physiol. 38, 139–147. doi: 10.1080/10236240500064354
Hurton, L., Berkson, J., and Smith, S. (2009). The Effect of Hemolymph Extraction Volume and Handling Stress on Horseshoe Crab Mortality: Biology and Conservation of Horseshoe Crabs. Boston, MA: Springer, 331–346. doi: 10.1007/978-0-387-89959-6_21
Isakova, V., and Armstrong, P. B. (2003). Imprisonment in a death-row cell: the fates of microbes entrapped in the Limulus blood clot. Biol. Bull. 205, 203–204. doi: 10.2307/1543253
Iwanaga, S. (2002). The molecular basis of innate immunity in the horseshoe crab. Curr. Opin. Immunol. 14, 87–95. doi: S0952-7915(01)00302-8
Iwanaga, S., Kawabata, S. I., and Muta, T. (1998). New types of clotting factors and defense molecules found in horseshoe crab hemolymph: their structures and functions. J. Biochem. 123, 1–15. doi: 10.1016/S0140-6736(82)91016-9
James-Pirri, M. J., Veillette, P. A., and Leschen, A. S. (2012). Selected hemolymph constituents of captive, biomedically bled, and wild caught adult female American horseshoe crabs (Limulus polyphemus). Mar. Freshw. Behav. Physiol. 45, 281–289. doi: 10.1080/10236244.2012.730216
Kautsky, N., Rönnbäck, P., Tedengren, M., and Troell, M. (2000). Ecosystem perspectives on management of disease in shrimp pond farming. Aquaculture 191, 145–161. doi: 10.1016/s0044-8486(00)00424-5
Kin, A., and Blazejowski, B. (2014). The horseshoe crab of the genus Limulus: living fossil or stabilomorph? PLoS One 9:e108036. doi: 10.1371/journal.pone.0108036
Krisfalusi-Gannon, J., Ali, W., Dellinger, K., Robertson, L., Brady, T. E., Goddard, M. K., et al. (2018). The role of horseshoe crabs in the biomedical industry and recent trends impacting species sustainability. Front. Mar. Sci. 5:185. doi: 10.3389/fmars.2018.00185
Kwan, B. K., Chan, A. K., Cheung, S. G., and Shin, P. K. (2014). Hemolymph quality as indicator of health status in juvenile Chinese horseshoe crab Tachypleus tridentatus (Xiphosura) under laboratory culture. J. Exp. Mar. Biol. Ecol. 457, 135–142. doi: 10.1016/j.jembe.2014.04.011
Leschen, A. S., and Correia, S. J. (2010). Mortality in female horseshoe crabs (Limulus polyphemus) from biomedical bleeding and handling: implications for fisheries management. Mar. Freshw. Behav. Physiol. 43, 135–147. doi: 10.1080/10236241003786873
Lindberg, R. B., Inge, W., Mason, A., and Pruitt, B. (1972). Natural variations in sensitivity of Limulus amoebocyte lysate to endotoxin. Federat. Proc. 31:791.
Liu, T. Y., Seid, R. C., Tai, J. Y., Liang, S. M., Sakmar, T. P., and Robbins, J. B. (1979). Studies on Limulus lysate coagulating system. Biomed. Appl. Horseshoe Crab (Limulidae) 79, 147–158.
Manca, A., Mohamad, F., Nelson, B. R., Mohd Sofa, M. F. A., Alia’m, A. A., and Ismail, N. (2016). Trailing the spawning horseshoe crab Tachypleus Gigas (Müller, 1785) at designated natal beaches on the east coast of Peninsular Malaysia. Cell Dev. Biol. 5, 1–6. doi: 10.4172/2168-9296.1000171
Medzhitov, R., and Janeway, C. Jr. (2000). Innate immunity. New Engl. J. Med. 343, 338–344. doi: 10.1056/nejm200008033430506
Mürer, E. H., Levin, J., and Holme, R. (1975). Isolation and studies of the granules of the amebocytes of Limulus polyphemus, the horseshoe crab. J. Cell. Physiol. 86, 533–542. doi: 10.1002/jcp.1040860310
Nickerson, K. W., and Van Holde, K. E. (1971). A comparison of molluscan and arthropod hemocyanin-I. Circular dichroism and absorption spectra. Compar. Biochem. Physiol. Part B Biochem. 39, 855–872. doi: 10.1016/0305-0491(71)90109-X
Nolan, M. W., and Smith, S. A. (2009). Clinical Evaluation, Common Diseases, and Veterinary Care of the Horseshoe Crab, Limulus Polyphemus: Biology and Conservation of Horseshoe Crabs. Boston, MA: Springer, 479–499. doi: 10.1007/978-0-387-89959-6_30
Novitsky, T. J. (1984). Discovery to commercialization-the blood of the horseshoe-crab. Oceanus 27, 13–18.
Novitsky, T. J. (2015). Biomedical Implications for Managing the Limulus Polyphemus Harvest Along the Northeast Coast of the United States: Changing Global Perspectives on Horseshoe Crab Biology, Conservation and Management. Cham: Springer, 483–500. doi: 10.1007/978-3-319-19542-1_28
Powell, J. R., and Wells, R. S. (2011). Recreational fishing depredation and associated behaviors involving common bottlenose dolphins (Tursiops truncatus) in Sarasota Bay, Florida. Mar. Mam. Sci. 27, 111–129. doi: 10.1111/j.1748-7692.2010.00401.x
Rudkin, D. M., Graham, A. Y., and Nowlan, G. S. (2008). The oldest horseshoe crab: a new Xiphosurid from Late ordovician konservat−lagerstätten deposits, Manitoba, Canada. Palaeontology 51, 1–9. doi: 10.1111/j.1475-4983.2007.00746.x
Rudkin, D. M., and Young, G. A. (2009). Horseshoe Crabs–An Ancient Ancestry Revealed: Biology and Conservation of Horseshoe Crabs. Boston, MA: Springer, 25–44. doi: 10.1007/978-0-387-89959-6_31
Rudloe, A. (1983). The effect of heavy bleeding on mortality of the horseshoe crab, Limulus polyphemus, in the natural environment. J. Invert. Pathol. 42, 167–176. doi: 10.1016/0022-2011(83)90059-9
Schreibman, M. P., and Zarnoch, C. B. (2009). Aquaculture Methods and Early Growth of Juvenile Horseshoe Crabs (Limulus Polyphemus): Biology and Conservation of Horseshoe Crabs. Boston, MA: Springer, 501–511. doi: 10.1007/978-0-387-89959-6_31
Shelley, C., and Lovatelli, A. (2011). Mud Crab Aquaculture: A Practical Manual. FAO Fisheries and Aquaculture Technical Paper, 567. Rome: FAO.
Shuster, C. N. Jr. (1982). A pictorial review of the natural history and ecology of the horseshoe crab Limulus polyphemus, with reference to other Limulidae. Prog. Clin. Biol. Res. 81:1.
Shuster, C. N. Jr. (1990). “The American horseshoe crab, Limulus polyphemus,” in Clinical Applications of the Limulus Amoebocyte Lysate Test, ed. R. B. Prior (Boston, CA: CRC Press).
Smith, D. R., Brockmann, J. H., Beekey, M., King, T. L., and Millard, M. J. (2017). Conservation status of the American horseshoe crab, (Limulus Polyphemus): a regional assessment. Rev. Fish Biol. Fish. 27, 135–175. doi: 10.1007/s11160-016-9461-y
Smith, S. A., and Berkson, J. (2005). Laboratory culture and maintenance of the horseshoe crab (Limulus polyphemus). Lab. Anim. 34, 27–34. doi: 10.1038/laban0705-27
Smith, S. A., Berkson, J. M., and Barratt, R. A. (2002). “Horseshoe crab (Limulus polyphemus) hemolymph, biochemical and immunological parameters,” in Proceedings of the 33rd Annual Conference of the International Association for Aquatic Animal Medicine, Albufeira.
Tai, J. Y., Seid, R. C., Huhn, R. D., and Liu, T. Y. (1977). Studies on Limulus amoebocyte lysate II. Purification of the coagulogen and the mechanism of clotting. J. Biol. Chem. 252, 4773–4776.
Timmons, M. B. (1994). Aquaculture reuse systems: engineering design and management. Dev. Aquacult. Fisher. Sci. 27:346.
Tinker-Kulberg, R., Dellinger, A. L., Brady, T., Robertson, L., Goddard, M. K. M., Bowzer, J. A. S., et al. (2020a). Effects of diet on the biochemical properties of amebocytelysates from Limulus polyphemus in an aquaculture setting. Front. Mar. Sci. (in press). doi: 10.3389/fmars.2020.541604
Tinker-Kulberg, R., Dellinger, K., Brady, T., Robertson, L., Levy, J., Abood, S., et al. (2020b). Horseshoe crab aquaculture as a sustainable endotoxin testing resource. Front. Mar. Sci. 7:153. doi: 10.3389/fmars.2020.00153
Van Holde, K. E., and Miller, K. I. (1995). Hemocyanins. Adv. Protein Chem. 47, 1–81. doi: 10.1016/S0065-3233(08)60545-8
Keywords: horseshoe crab (Limulus polyphemus), hemocyanin, pen culture system, recirculating aquaculture system, Limulus amebocyte lysate
Citation: Tinker-Kulberg R, Dellinger AL, Gentit LC, Fluech BA, Wilder CA, Spratling IL, Stasek DJ, Kepley CL, Robertson L, Goddard MK, Brady TE, Tōland L and Dellinger K (2020) Evaluation of Indoor and Outdoor Aquaculture Systems as Alternatives to Harvesting Hemolymph From Random Wild Capture of Horseshoe Crabs. Front. Mar. Sci. 7:568628. doi: 10.3389/fmars.2020.568628
Received: 01 June 2020; Accepted: 04 September 2020;
Published: 22 October 2020.
Edited by:
Morten Omholt Alver, Norwegian University of Science and Technology, NorwayReviewed by:
Md Tawheed Hasan, Sylhet Agricultural University, BangladeshCopyright © 2020 Tinker-Kulberg, Dellinger, Gentit, Fluech, Wilder, Spratling, Stasek, Kepley, Robertson, Goddard, Brady, Tōland and Dellinger. This is an open-access article distributed under the terms of the Creative Commons Attribution License (CC BY). The use, distribution or reproduction in other forums is permitted, provided the original author(s) and the copyright owner(s) are credited and that the original publication in this journal is cited, in accordance with accepted academic practice. No use, distribution or reproduction is permitted which does not comply with these terms.
*Correspondence: Kristen Dellinger, a2RlbGxpbmdlckBuY2F0LmVkdQ==; Anthony L. Dellinger, YWRlbGxpbmdlckBrZXBsZXliaW9zeXN0ZW1zLmNvbQ==; YWRlbGxpbmdlckBnbWFpbC5jb20=
Disclaimer: All claims expressed in this article are solely those of the authors and do not necessarily represent those of their affiliated organizations, or those of the publisher, the editors and the reviewers. Any product that may be evaluated in this article or claim that may be made by its manufacturer is not guaranteed or endorsed by the publisher.
Research integrity at Frontiers
Learn more about the work of our research integrity team to safeguard the quality of each article we publish.