- 1Department of Biological Sciences, Florida International University, Miami, FL, United States
- 2Department of Ecology, Evolution, and Marine Biology, University of California, Santa Barbara, Santa Barbara, CA, United States
- 3Department of Microbiology, Oregon State University, Corvallis, OR, United States
Reef-building corals face a multitude of threats not only from global climate change but also local stressors such as nutrient pollution. Nutrient enrichment can amplify the negative effects of predation on corals by facilitating dysbiosis in the coral microbiome that leads to coral mortality. These patterns suggest that nutrient pollution might suppress the capacity of the coral immune system to respond to microbial infections that follow mechanical injury from predation. Here, we measured the impact of nutrient enrichment and predation on tyrosinase-type and laccase-type phenoloxidase (PO) activities, which are key components of immune defense pathway involved in melanin synthesis and wound healing in corals. Corals were exposed in situ to either ambient or enriched nutrient levels in combination with either no damage, mechanical damage, or predation damage from parrotfishes. The activity of PO enzymes in response to both tyrosinase-type substrates significantly decreased under nutrient enrichment, suggesting that corals became immune-compromised. Predation damage also increased laccase activity, implicating it in tissue repair and potentially defense from pathogens. Our findings highlight the need to mitigate nutrient pollution on coral reefs, as higher nutrient levels suppress important coral immune pathways and likely contribute to patterns of increased coral disease and subsequent mortality on reefs plagued by nutrient enrichment.
Introduction
Chronic nutrient enrichment is endangering the stability and health of coastal ecosystems worldwide. Originating primarily from anthropogenic sources such as agricultural run-off (Nixon, 1995; Boesch, 2002), these sources of nutrient input often are major contributors to higher levels of nitrogen and phosphorus, which are common limiting nutrients in ecosystems (Howarth, 2008). The long-term effects of higher nutrient loads to coastal ecosystems are wide-ranging and complex such as decreased biodiversity (Howarth, 2008), increased growth of dead zones (Diaz and Rosenberg, 2008), and harmful algal blooms (Granéli et al., 2008).
In particular, the proposed connection between higher nutrient levels and coral disease and bleaching (Hayes et al., 2001; Vega Thurber et al., 2014) is especially troubling in light of the escalating severity of disease outbreaks over the past few decades (Harvell et al., 1999; Weil, 2004; Precht et al., 2016). Nutrient pollution not only causes coral diseases to progress more rapidly within coral colonies (Bruno et al., 2003; Voss and Richardson, 2006), but can also increase the overall prevalence of disease on reefs (Vega Thurber et al., 2014). Further, nutrient enrichment results in higher abundance of potential bacterial pathogens in the coral microbiome (Shaver et al., 2017) as well as increased fitness and virulence of pathogens (Vega Thurber et al., 2009), likely contributing to altered patterns in coral disease.
The repairing function of the coral immune system plays an important role during tissue recovery from injuries (Palmer et al., 2011b) such as wounds from predation, a common phenomenon for many coral species. Fishes such as scraping and excavating parrotfishes, which frequently prey on corals, can inflict significant damage to coral tissue and skeleton (Rotjan and Lewis, 2008; Burkepile, 2012). Consequently, corals with open wounds from parrotfish bites may be more susceptible to opportunistic pathogens in their environment compared to unbitten corals. While studies document increased disease incidence in corals when there is mechanical damage or open wounds (Aeby and Santavy, 2006; Page and Willis, 2008), it remains unknown if parrotfish vector or facilitate coral disease. Furthermore, corals under nutrient enrichment that are preyed on by parrotfish experience higher mortality rates and have more abundant opportunistic bacteria than unenriched corals (Zaneveld et al., 2016). Thus, to better understand the mechanisms that underlie the observed synergy between coral predation and nutrient enrichment, it is vital that we determine how elevated nutrient levels affect the immune competence of corals and subsequently corals’ capacity to heal wounds caused by predation.
In this study, we examined an evolutionarily important innate immune system pathway in invertebrates, the melanin synthesis pathway, which is central in healing physical wounds as well as in the defense against pathogens (Söderhall, 1982; Sugumaran, 2002; Cerenius et al., 2008). Integral components of the melanin synthesis pathway, phenoloxidase (PO) enzymes are stored in their inactive zymogen form as pro-phenoloxidases (proPO) until the PO pathway is activated in areas of damaged tissue, at which point proPO enzymes are converted into their active PO form via proteolysis (Cerenius et al., 2008, 2010). These active phenoloxidase enzymes will then defend the coral against invaders via the encapsulation and phagocytosis of pathogens (Nappi and Christensen, 2005) as well as the production of cytotoxic intermediates (Nappi and Ottaviani, 2000). Broadly classified as either tyrosinase-type or laccase-type POs based upon their substrate specificity, tyrosinase-type POs are believed to be responsible for the immune response of the phenoloxidase pathway in invertebrates via melanin synthesis (Nigam et al., 1997; Cerenius et al., 2003). In contrast, laccase-type POs exhibit a wide array of functions in the diverse organisms where they are found including cuticle sclerotization in arthropods (Andersen, 2012), lignification in plants (Barros et al., 2015), lignolysis in certain parasitic fungi (Dwivedi et al., 2011), and stress resistance in bacteria (Dwivedi et al., 2011).
While much remains to be understood, our knowledge of the diverse temporal and species-specific responses of phenoloxidase pathways in corals has grown in recent years to reflect the complexity of these pathways. Tyrosinase-type phenoloxidases have been shown to respond to heat stress (Mydlarz et al., 2009; Palmer et al., 2011a,b, Wall et al., 2018), disease (Mydlarz et al., 2008; Palmer et al., 2011a; Kelly et al., 2016), pathogen elicitors (Palmer et al., 2011b; Fuess et al., 2016), pathogens (van de Water, et al., 2018), tissue growth (D’Angelo et al., 2012), sedimentation (Sheridan et al., 2014), seasons (van de Water et al., 2015b, 2016), and damage (van de Water et al., 2015a,b). Tyrosinase-type POs have also been positively correlated with both disease and bleaching resistance variability between different coral species (Palmer et al., 2010, 2012a). This variability is believed to be partially due to different immune strategies for different life histories where some species (e.g., Porites spp.) heavily invest in high baseline immune levels making them more resistant to disease and heat stress at the expense of decreased growth while others exhibit the opposite pattern (e.g., Acropora spp.) (Palmer et al., 2008, 2011b; van de Water et al., 2016). On the other hand, the laccase-type POs are less understood in corals but have shown increased enzymatic activity upon contact with crustose coralline algae during larval settlement (Palmer et al., 2012b) and when exposed to damage (Palmer, 2018), suggesting that laccase-type POs might possess a wound healing and/or structural role in corals. Laccase-type POs are also correlated with disease resistance (Palmer et al., 2012a), indicating they likely play an important but complex role within the coral holobiont, the complex association between corals and the diverse symbiotic microorganisms including algae, fungi, archaea, bacteria, and viruses (Rohwer et al., 2002).
Here, we used a field experiment on a coral reef in the Upper Florida Keys, United States, to assess the synergistic effect of nutrients and predation on the activity of cresolase, catecholase, and laccase PO enzymes to investigate the variability of melanin synthesis pathways underlying the constituent immunity of corals in response to combined predation and nutrient enrichment. Understanding whether corals have the immunological capacity to simultaneously heal wounds from predators while combating stress from nutrient pollution is critical to understanding how their response to natural sources of wounding, such as predation, will be altered in areas of increasing anthropogenic influences.
Materials and Methods
Coral Collection and Experimental Set-Up
We evaluated the effects of nutrient enrichment and predation on phenoloxidase pathways in the coral Porites porites using a fully factorial experiment conducted over the course of 70 days from June 2015 through August 2015 at Pickles Reef (24.989∘N, 80.376∘W), a shallow (5–7 m) fore reef in the Upper Florida Keys. Seventeen colonies of P. porites were collected from a nearby patch reef in the Florida Keys National Marine Sanctuary (permit no: FKNMS-2014-073). The colonies were fragmented into individual coral nubbins for a total of 108 fragments that were divided among 18 experimental blocks separated by ≥2 m. Each experimental block contained six fragments that were arranged into three groups of two fragments. All fragments were individually secured via a cable tie to a masonry nail hammered into the substrate. The three pairs of coral fragments in a block were separated by ∼30 cm while the two fragments within each pair were separated by ∼10 cm. All three pairs of fragments in each block were covered by separate mesh enclosures constructed of PVC-coated wire (2.5 cm diameter hole size) secured to the substrate that excluded corallivorous fishes. The three fragment pairs within each block were then randomly assigned one of three treatments: (1) parrotfish predation, (2) mechanical damage, or (3) undamaged control (Figure 1A).
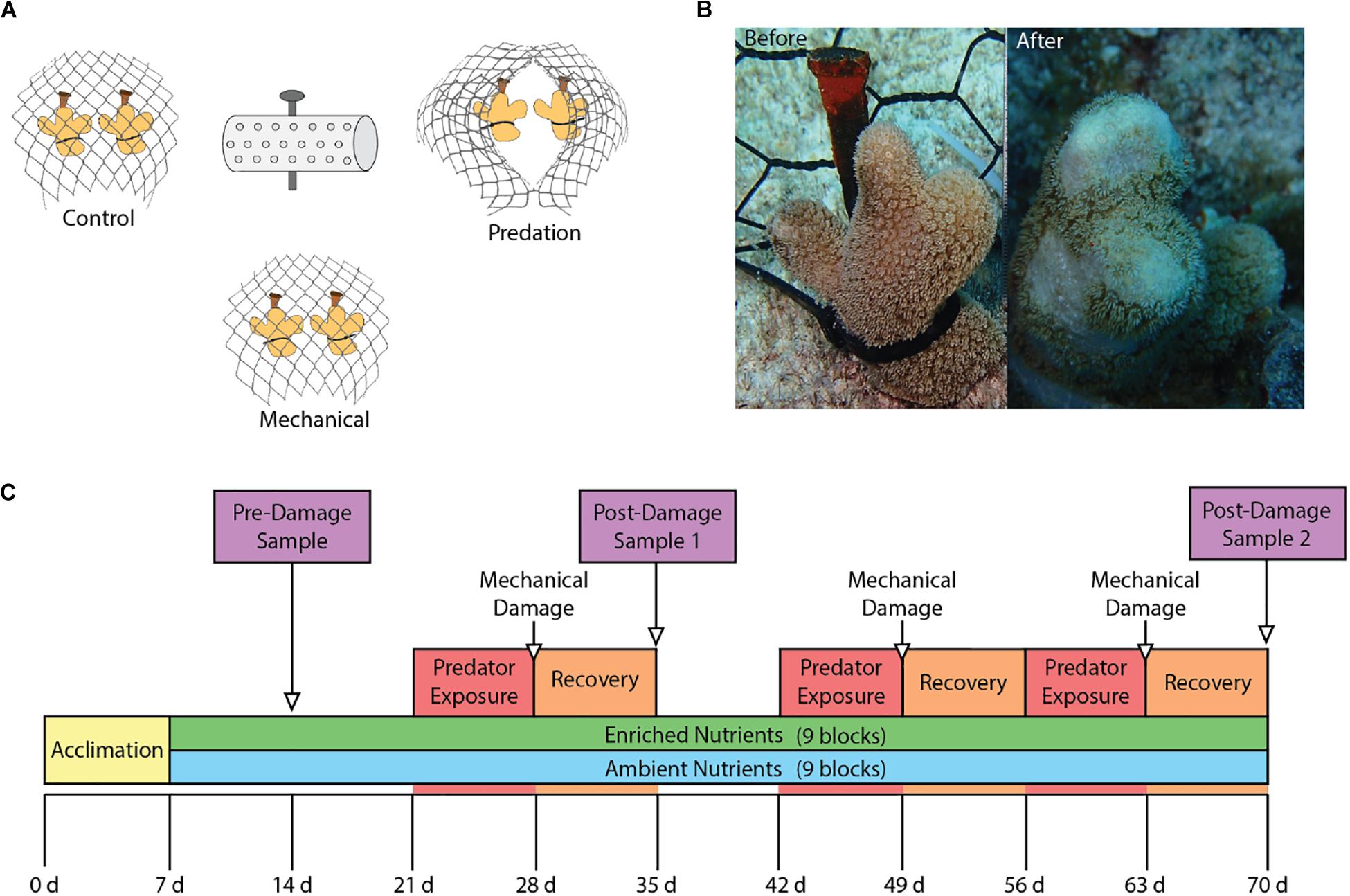
Figure 1. (A) Experimental group layout showing the three damage groups surrounding the nutrient diffuser that contained Osmocote fertilizer for enriched nutrient blocks. (B) Before and after photos showing results of parrotfish corallivory. (C) Experimental timeline showing the start of nutrient enrichment at week 1 as well as the times of damage exposures and sampling.
Exposure to Nutrient Enrichment and Predation Damage
After 1 week of recovery from fragmentation and experimental set-up, nine experimental blocks were exposed to enriched nutrient levels on day 7 following Shaver et al. (2017). Nutrient enrichment was achieved using nutrient diffusers constructed from a 15 cm long (5 cm diameter) PVC pipe with holes drilled in it and covered in window screen. Nutrient diffusers contained 150 g of Osmocote® 19-6-12 (N-P-K) Smart-Release plant fertilizer pellets that were replaced every 2 weeks. This enrichment protocol was previously validated to triplicate the ambient levels of DIN for a period of 30–45 days in similar conditions (Vega Thurber et al., 2014; Shaver et al., 2017). Measurement of DIN in the enrichment plots have been of 3.91 ± 1.34 μM compared to control plots of 1.15 ± 0.05 μM (Vega Thurber et al., 2014). Similarly, SRP were approximately nine times higher in the enrichment plots (0.27 ± 0.07 μM) as opposed to the controls (0.035 ± 0.006 μM) (Vega Thurber et al., 2014). No nutrients were added to the other nine blocks, which were maintained under local ambient nutrient conditions of ∼1.0 μM dissolved inorganic nitrogen and ∼0.4 μM soluble reactive phosphorus (the long-term average background level of nutrients on this reef) for the duration of the experiment (sensu Zaneveld et al., 2016).
The mesh enclosures were opened on days 21, 42, and 56 to allow access by corallivorous parrotfishes for pairs of coral fragments assigned to the parrotfish predation treatment. These corals were monitored every 1–2 days and the enclosures were closed when ∼50% of a coral was preyed upon. After 7 days of exposure to parrotfishes, regardless of the level of predation, all cages were closed to allow corals to recover from predation. At this time, corals in the mechanical damage treatments were artificially wounded using stainless steel concave cutters. Artificial wounds were inflicted to replicate levels of predation that occurred on the parrotfish exposure corals within the same block (Figure 1B).
Coral Sampling
Six of the eighteen blocks were randomly selected for sampling at each of the three time points. The sampling timeline (Figure 1C) consisted of an initial baseline sampling on day 14 (Pre-damage) after 1 week of nutrient exposure, followed by two post-damage samplings on day 35 (Post-damage 1) and day 70 (Post-damage 2). We sampled corals after 1 week of recovery from damage from predation or mechanical wounds to allow phenoloxidase activity to return to baseline levels (van de Water et al., 2015a), and determine the effect of predation damage on constitutive immune levels. This time frame was chosen as Porites spp. have higher baseline phenoloxidase activity than most coral species (Palmer et al., 2010, 2012a; Mydlarz and Palmer, 2011; van de Water et al., 2016), hypothesized to be due to a unique immune strategy that relies on a continuous, energetically expensive investment in constituent immunity parameters for increased resistance to disease and thermal-induced bleaching at the expense of slower growth rates (Palmer et al., 2008, 2010, 2011b, 2012a; van de Water et al., 2016). One fragment from each of the three pairs in the six selected experimental replicates was designated for sampling for the phenoloxidase enzymatic assays. These fragments were photographed before being collected, placed into an individual zip lock bag, immediately frozen in liquid nitrogen, and then transported on dry ice to the lab where they were stored at −80°C until processing. The other fragment in each pair was used to quantify coral predation throughout the duration of the experiment. For each of these fragments we photographed, measured the size of each predation wound in situ to the nearest mm, visually estimated for percent of damage, and recorded data on the presence of bleaching and disease.
Extract Preparation
Tissue was scraped from each fragment using bone cutters for protein extract preparation following Mydlarz and Palmer (2011). Briefly, tissue was placed in an extraction buffer (50 mM phosphate buffer, pH 7.8 with 1 mM mercaptoethanol) over ice. Tissue samples were then homogenized using an Omni Bead Ruptor 24 for 20 s, incubated on ice for 5 min, vortexed with a spatula of glass beads for another 20 s, and incubated for a second time on ice for 5 min. Cellular debris was pelleted and separated from protein-containing supernatant by centrifugation of samples at 2400 g at 4°C for 5 min. Protein extracts were then stored at -80°C. To normalize enzymatic activity per total (holobiont) protein content, the concentration of proteins was quantified using the Bradford Protein Assay (#23236, Thermo Fisher Scientific) with Coomassie G-250 dye and bovine serum albumin as a standard.
Phenoloxidase Activity Assays
Total potential phenoloxidase activity (tpPO), which is the sum of both proPO and PO activity was measured along with PO activity according to Mydlarz and Palmer (2011) by diluting 20 μL of protein extracts in 40 μL of phosphate buffer (50 mM, pH 7.5). To measure tpPO levels, 5.25 μL of 0.1 mg mL–1 trypsin was added followed by a 10 min incubation at room temperature to allow the conversion of tpPO enzymes to their active PO form. Stocks of the selected substrates of L-DOPA (tyrosinase-type catecholase activity), L-tyrosine (tyrosinase-type cresolase activity), and hydroquinone (laccase activity) (10 mM, Sigma-Aldrich) were created fresh each day. The creation of the colored oxidative product after the addition of 30 μL of each substrate stock was monitored on a SpectraMax 340 PC 384 microplate reader at 490 nm for 30 min at 30°C. Data from initial velocity conditions, when the enzyme reaction is linear, is presented as Δ absorbance mg protein–1 min–2. All samples were run in triplicate on three separate 96-well plates at the same time as the negative controls of phosphate buffer (50 mM, pH 7.5) and boiled protein extracts.
Statistical Analysis
Mean tpPO and PO values of the phenoloxidase assay technical replicates for each of the 54 coral samples were calculated and then tested for normality and homoscedasticity using the Shapiro-Wilk’s Test and Levene’s tests, respectively. The effect of nutrient enrichment on the proportion of each colony preyed upon by parrotfishes was analyzed using a four-factor analysis of variance (ANOVA) that considered time (Post-damage 1 or 2) and nutrient treatment (ambient or enriched) as interacting factors, and parent colony and block as non-interacting factors. Data on the proportion of coral colony preyed upon were log transformed to meet assumptions of ANOVA.
To assess the effects of nutrients and damage on phenoloxidase activity, we built a distance matrix of the square root-transformed phenoloxidase values and performed four different permutational multivariate analysis of variance (PERMANOVA) analyses each on both the tpPO and PO phenoloxidase values using the vegan package in R (Oksanen et al., 2018). For both tpPO and PO values, PERMANOVAS were first conducted on the three phenoloxidase enzyme types collectively to assess the overall effects on phenoloxidase activity. This initial PERMANOVA was then followed by three individual PERMANOVA analyses for each of the three phenoloxidase enzyme types to identify any effects that were specific to that particular enzyme. A priori planned post-hoc tests were then carried out according to the significant main and/or interaction effects identified in each PERMANOVA analysis using two-way t-tests. The α for each set of post-hoc tests was adjusted accordingly based on the number of planned comparisons conducted for the particular PERMANOVA. Finally, ratios of PO to tpPO activity were calculated for each of the three phenoloxidase enzymes followed by a PERMANOVA analysis and a priori post-hoc tests as described above. All statistical analyses were conducted in R (R Core Team, 2014).
Phenoloxidase activity may have been influenced by multiple variables in this study. Therefore, for each sampling point we used model selection to test the effects of plausible predictors on PO activity using a likelihood ratio test (LRT). The LRT compared generalized linear mixed effects models with each main factor to a null model that only included phenoloxidase activity modeled by random block effects as well as a full model with all explanatory variables included. Factors were tested via stepwise selection of best fit model where each potential explanatory variable was added to the model and then compared to the null model that only included the random effect of block. To determine if including a factor significantly improved the model, we compared the model with the factor(s) in it to the null model using an ANOVA with p <0.05 indicating significant improvement of the model fit. Plausible explanatory variables for PO activity were: nutrient enrichment (ambient, enriched), parent colony (unique for each parent colony), predation type (control, mechanical, parrotfish), damage (binary; no, yes), number of wounds (continuous), and proportion of coral preyed upon (range = 0–1).
Results
Colony Damage
Parrotfish preyed on ambient and nutrient-enriched corals similarly, although there was a trend for more, larger wounds on enriched corals (Treatment effect: F1,17 = 3.871, p = 0.066; Supplementary Material S1). For corals exposed to parrotfishes, the average proportional area of each colony affected by predation did not change through time (Time effect: F1,17 = 3.255, p = 0.089) and was not influenced by block (Block effect: F6,17 = 1.107, p = 0.399) or parent colony (Parent colony effect: F12,17 = 0.422, p = 0.133).
Phenoloxidase tpPO and PO Enzyme Activity
The overall level of tpPO was only affected by nutrients [F(1,48) = 74.9781, p < 0.001, Figure 2] and damage [F(2,48) = 4.4329, p < 0.01, Figure 3], with a priori post-hoc tests revealing that phenoloxidase tpPO levels were lowered in corals exposed to predation by parrotfish compared to both control (p < 0.001) and mechanically damaged corals (p < 0.001). Nutrients also lowered the individual tpPO activity levels for all three types of phenoloxidase enzymes including cresolase [F(1,48) = 98.4896, p < 0.001], catecholase [F(1,48) = 78.7658, p < 0.001], and laccase [F(1,52) = 30.451, p < 0.001]. While damage did not affect laccase tpPO activity, it elevated overall cresolase [F(2,48) = 9.2281, p < 0.001] and catecholase [F(2,48) = 5.2131, p < 0.01] tpPO activity.
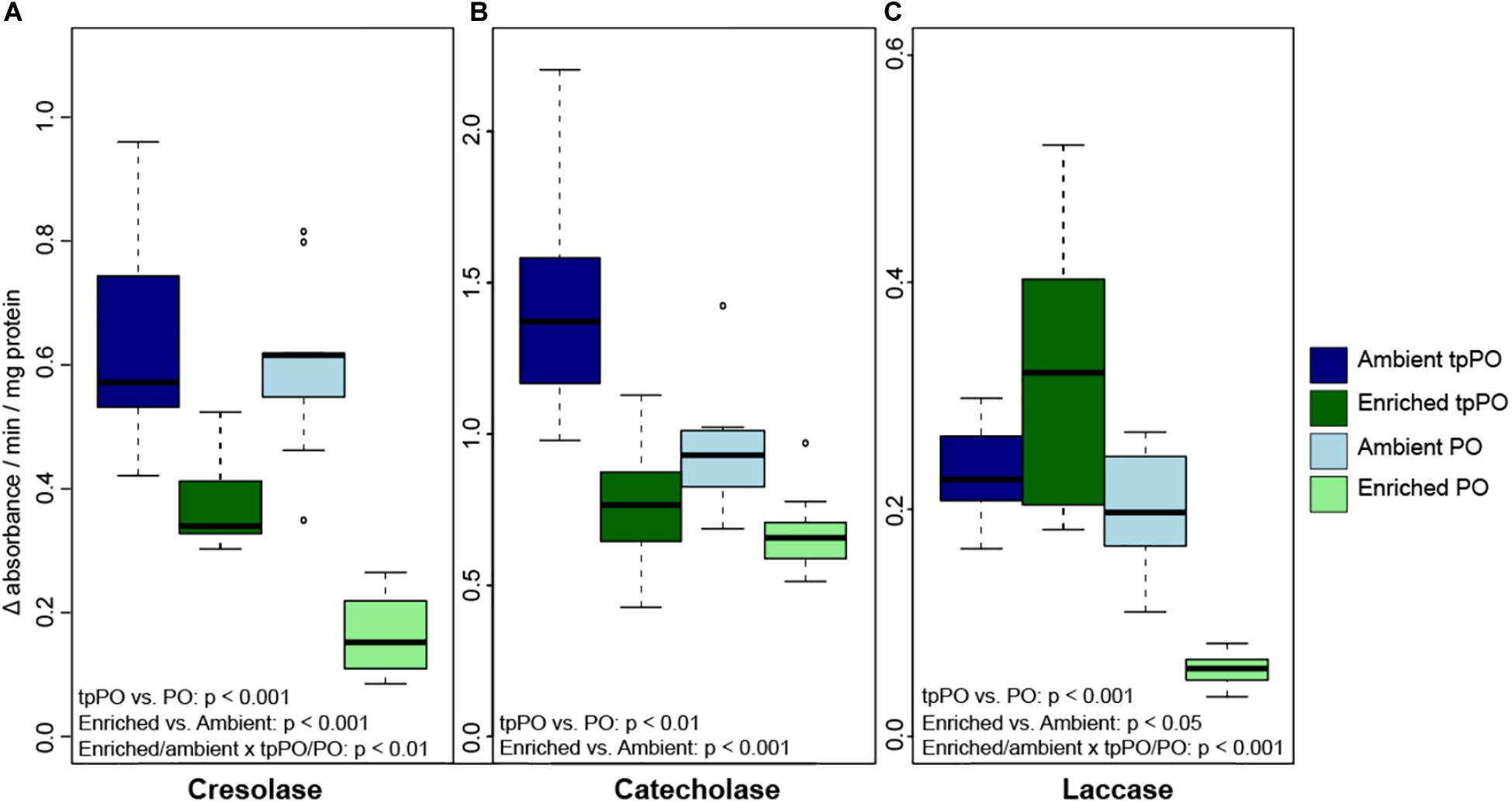
Figure 2. The level of tpPO and PO (±SD) in the coral P. porites for (A) tyrosinase-type catecholase, (B) tyrosinase-type cresolase, and (C) laccase phenoloxidase activity after 1 week of exposure to either ambient or enriched nutrient levels.
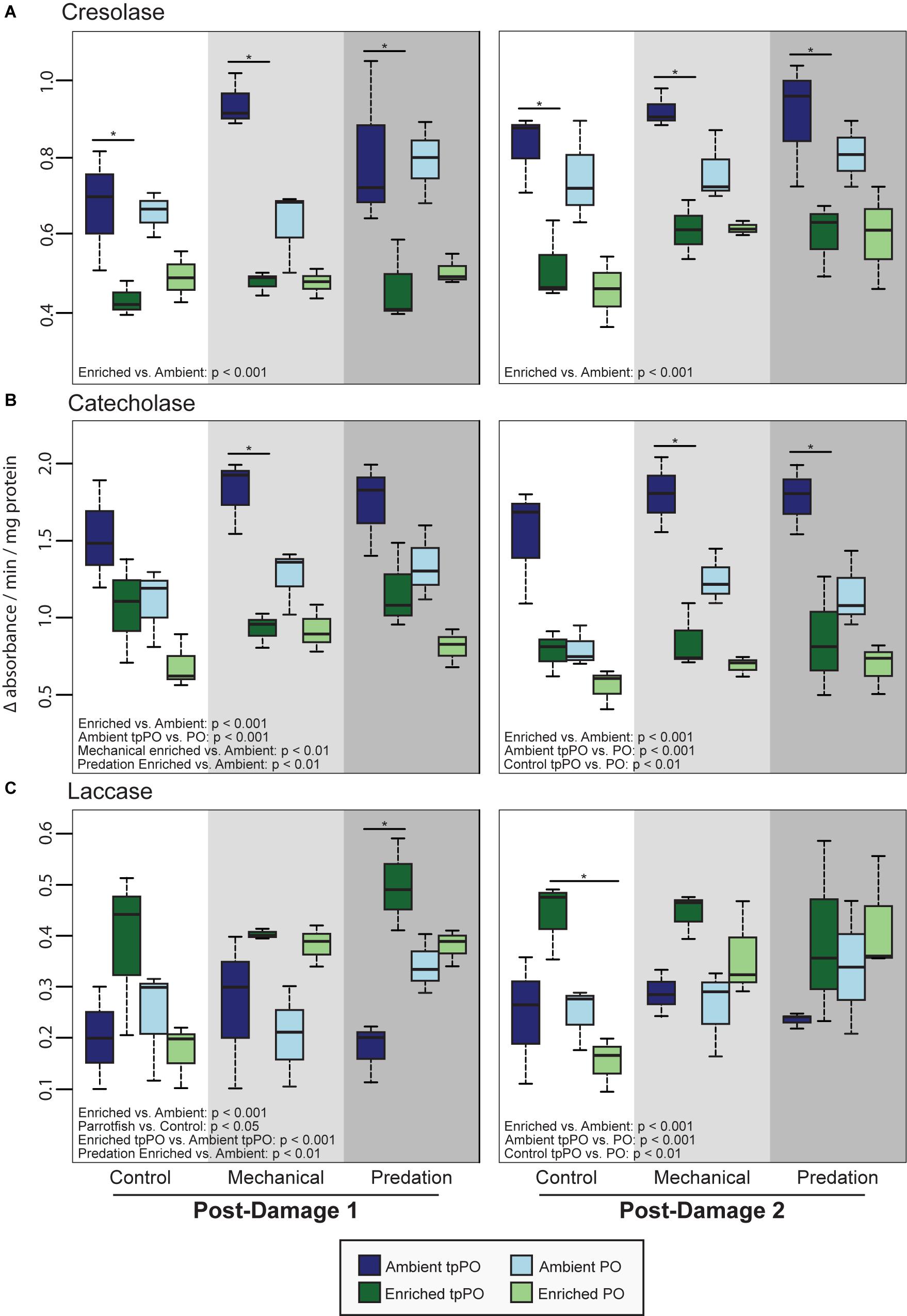
Figure 3. Mean tpPO and PO levels (±SD) in the coral Porites porites at the first post-damage and second post-damage sampling timepoints for (A) tyrosinase-type cresolase activity, (B) tyrosinase-type catecholase activity, and (C) laccase activity. Black bars within a time point represent a significant difference (p < 0.05) between ambient and enriched corals.
The overall level of PO was more variable than tpPO levels, as it was affected by nutrients [F(1,40) = 73.6032, p < 0.001, Figure 2], damage [F(2,40) = 30.7031, p < 0.001, Figure 3], and timepoint [F(2,40) = 8.8214, p < 0.001] along with the combined influence of nutrients and damage [F(2,40) = 8.8236, p < 0.001] and nutrients and timepoint [F(2,40) = 4.3326, p < 0.05]. In contrast to the overall patterns in PO levels, catecholase PO activity was only affected by nutrients [F(1,48) = 65.1892, p < 0.001] and damage [F(2,48) = 16.1323, p < 0.001]. While mechanical damage had a particularly strong effect on increasing catecholase PO activity (p < 0.01) there was also an increase in response to predation although it was not significant after adjusting for the multiple a priori comparisons (p < 0.05).
On the other hand, cresolase and laccase PO activity were both affected by not only nutrients, damage, and timepoint but also the interactions between nutrients and damage as well as nutrients and timepoint. While a priori post-hoc tests revealed that predation (p < 0.001) and mechanical damage (p < 0.001) elevated laccase PO levels as a whole, particularly under enriched nutrient levels (p < 0.001), cresolase PO was only significantly elevated in combination with enriched nutrient levels and either predation (p < 0.001) or mechanical damage (p < 0.001).
When examining the ratio of PO to tpPO for the different phenoloxidase enzymes, cresolase ratios were affected by the interaction of nutrients and damage [F(2,48) = 5.7124, p < 0.01] while catecholase ratios were only affected by nutrients [F(1,52) = 9.1486, p < 0.01]. On the other hand laccase ratios were affected by nutrients [F(1,48) = 33.0044, p < 0.001], damage [F(2,48) = 13.8797, p < 0.001], and the interaction between nutrients and damage [F(2,48) = 8.6651, p < 0.001]. While nutrients decreased the ratios (p < 0.001), predation damage increased the ratios (p < 0.01) while mechanical damage had no significant effect on the ratios. This suggests that while nutrients decrease the level of PPO to PO conversion, predation damage in particular causes a higher level of conversion to the active PO enzyme.
Generalized Linear Mixed Effects Models of PO Activity in Relation to Experimental Conditions
First Post-damage Sampling
When we tested for the best predictors of PO activity, we found that nutrient enrichment improved the model fit over the null model for tpPO [LRT: χ2(4) = 13.92, p < 0.001] and PO [LRT: χ2(4) = 12.67, p < 0.001] catecholase activity as well as tpPO [LRT: χ2(4) = 14.49, p < 0.001] and PO cresolase activity [LRT: χ2(4) = 11.68, p < 0.001]. Predation type marginally improved fit for PO cresolase activity [LRT: χ2(4) = 5.86, p = 0.054]. Nutrient enrichment also significantly improved the model fit for tpPO laccase activity [LRT: χ2(4) = 9.50, p = 0.002] while none of the predictors were better than the null model for PO laccase activity.
Second Post-damage Sampling
Nutrient enrichment improved the model fit for both tpPO [LRT: χ2(4) = 17.65, p < 0.001] as well as PO [LRT: χ2(4) = 10.88, p < 0.001] catecholase activity. Both the percent of the colony wounded [LRT: χ2(4) = 6.67, p = 0.009] as well as the number of wounds on a colony [LRT: χ2(4) = 5.75, p = 0.016] improved model fit for PO catecholase activity, suggesting an increase in catecholase activity with increased predation. Nutrient enrichment improved the model for cresolase tpPO [LRT: χ2(4) = 14.93, p < 0.001] while cresolase PO was improved by both nutrient enrichment [LRT: χ2(4) = 10.48, p = 0.001] as well as parent colony identity [LRT: χ2(7) = 12.65, p = 0.013]. The model for laccase tpPO was improved by nutrient enrichment [LRT: χ2(4) = 9.79, p = 0.001] while the model for laccase PO was improved by the number of wounds on a colony [LRT: χ2(4) = 4.46, p = 0.035].
Discussion
This study is the first to examine both the combined and independent effects of nutrient enrichment and predation on essential immune pathways used to combat infectious agents in a coral. Our findings indicate that nutrients differentially affect tyrosinase-type (i.e., catecholase and cresolase) and laccase-type phenoloxidase immune pathways in the scleractinian coral Porites porites. This discovery is timely and important, as it may represent a mechanism explaining why coral disease increases with nutrient enrichment (Bruno et al., 2003; Voss and Richardson, 2006; Vega Thurber et al., 2014; Zaneveld et al., 2016). Nutrient pollution may trigger the loss of immune competence, contributing to the increased prevalence and severity of coral disease in areas of higher nutrient levels. This finding is particularly alarming, as anthropogenically derived nutrients on coral reefs continue increasing with the development of coastal areas (Fabricius, 2005; Todd et al., 2010; Nava and Ramírez-Herrera, 2012).
Differential Responses of Tyrosinase vs. Laccase Type PO Pathways
Specifically, nutrients elevated laccase activity and suppressed tyrosinase-type activity, which is more cytotoxic against invaders making it normally preferred under healthy conditions (Cerenius and Söderhall, 2004; Cerenius et al., 2008, 2010). Nutrient pollution has also been shown to destabilize coral microbiomes and increase the dominance of bacteria believed to be pathogenic (Zaneveld et al., 2016; Shaver et al., 2017), which would require an immune response from the coral to regulate or defend themselves from these potentially disease-causing species. As studies have already demonstrated that enzymatic activity of phenoloxidase enzymes in corals will increase in response to immune elicitors (Palmer et al., 2011b; Fuess et al., 2018) as well as pathogens (Zhou et al., 2019), this suppression of tyrosinase-type activity might be the result of the depletion of the reserve tyrosinase-type proPO enzyme levels.
The increase in laccase activity in response to nutrient enrichment might be more beneficial for corals that are already stressed from high nutrient levels and have lower tyrosinase-type activity, despite its lower cytotoxicity than tyrosinase-type activity. In fact, we observed an interaction between damage from predation and nutrients suggesting that the increased laccase activity might be fulfilling an immunological role within the coral and defending it from pathogens introduced by predation. Alternatively, this increased laccase activity might simply be due to the process of wound healing as suggested by a recent study (Palmer, 2018) rather than immune defense from pathogens. Future experiments that link increased laccase activity to specific functions will be important to improving our understanding of how this pathway contributes to coral immunity in response to predation.
Increased nutrient availability severely decreased the enzymatic activity, particularly tpPO, for both tyrosinase-type enzymes (i.e., catecholase, cresolase) examined in this study. The suppression of enzymatic activity in these pathways is significant as tyrosinase-type phenoloxidase activity has been positively correlated to immunocompetence in diverse coral species (Palmer et al., 2008, 2010, 2012a). Previous studies on corals have shown that the level of catecholase tpPO is a strong predictor of disease susceptibility (Palmer et al., 2012a), possibly due to an increased capability of colonies with higher baseline tpPO levels to mount an immediate antimicrobial response against pathogens. In this study, we found that nutrient enrichment lowered overall catecholase and cresolase activity, particularly tpPO, across all three sampling time points. It is essential the coral host have sufficient tpPO reserves that can be quickly converted into their active form to carry out an immune response via the encapsulation and phagocytosis of pathogens along with the production of cytotoxic intermediates. If nutrient enrichment decreases baseline tpPO, then the capacity of a coral to mount a quick and effective immune response via the phenoloxidase pathway is likely hindered as well.
Interplay and Potential Cumulative Effects of Stressors on Immune Responses Over Time
Compounding the detrimental effect of nutrients on phenoloxidase activity, nutrient enriched corals at the second post-damage sampling had ∼2× more of the colony impacted by predation than control corals (Supplementary Material S2). It is possible that the nutrient-induced decrease in phenoloxidase activity, which is known to facilitate wound healing (D’Angelo et al., 2012; van de Water et al., 2015a), could have contributed to this trend by limiting the capacity of nutrient enriched corals to heal their wounds similarly to previous studies (Zaneveld et al., 2016). Thus, the increased damage in enriched corals as time progressed might be due to the effects of either decreased wound healing via suppression of phenoloxidase activity or increased predation or, more likely, the combination of the two. Nevertheless, this increased accumulation of damage from predation on enriched corals over time has important implications for the interplay of predation, nutrient enrichment, and coral disease dynamics. Not only has predation by itself been implicated as a vector for coral disease (Williams and Miller, 2005; Gignoux-Wolfsohn et al., 2012), but predation has also been associated with higher mortality rates in corals under enriched nutrients and destabilization of coral microbiomes (Zaneveld et al., 2016, 2017). If predators can serve as vectors for disease in corals, then the accumulation of increased damage over time from predation on enriched corals also increases the likelihood of the introduction of pathogens that could coincide with suppressed immune responses in corals also exposed to higher nutrient levels.
In contrast to the suppressive effect of nutrients on tyrosinase-type tpPO activity, the accumulation of damage in corals over time correlated with increased catecholase and laccase PO activity and marginally increased cresolase PO activity similarly to previous studies (van de Water et al., 2015a; Palmer, 2018). This increased phenoloxidase activity might represent an increased cytotoxic defense response toward pathogens or disease. Interestingly, the increase in catecholase PO activity after damage was only present in ambient corals despite the higher level of damage from predation on nutrient-enriched corals. Differences in catecholase PO activity could be due to immunosuppression of nutrient-enriched corals, preventing them from mounting an immune response through the classical tyrosinase-type phenoloxidase immune pathways. However, increased laccase PO activity after sustained damage was present under both enriched and ambient nutrient conditions. One potential explanation for this is that the increased laccase PO activity might be carrying out wound healing or structural reinforcement functions in damaged tissues as has been previously proposed (van de Water et al., 2015a; Palmer, 2018). Overall, the contrasting responses between laccase-type and tyrosinase-type PO activity in response to damage and nutrient enrichment suggest they possess distinct functional roles.
Physiological/Ecological Implications
Out of the three types of phenoloxidase enzymes examined in this study, only laccase-type phenoloxidase enzymes exhibited a specific response to predation, suggesting they play a role in responding to tissue damage. Laccase PO activity was elevated in corals after predation at both post-damage timepoints but was only marginally elevated in response to mechanical damage when in the presence of elevated nutrient levels. As laccase PO activity has been correlated to disease susceptibility in corals (Palmer et al., 2012a), increases in laccase PO activity might signal a long-term attempt by the coral’s immune system to eliminate a pathogen. In the context of this study, this would suggest that predation by corallivores, in contrast to mechanical damage, may have transmitted pathogens directly into the wound and increased the coral’s susceptibility to downstream disease processes regardless of nutrient enrichment.
Overall, the suppression of an important immunity pathway in corals due to enriched nutrient levels is an important step for understanding the effect of nutrients on coral health and disease dynamics. While nutrients have already been shown to influence coral disease dynamics (Bruno et al., 2003; Voss and Richardson, 2006; Vega Thurber et al., 2014) through the change in the abundance (Shaver et al., 2017) and virulence of pathogens (Vega Thurber et al., 2009), we now demonstrate that a suppressed host immune system could be a contributor to altered disease dynamics under nutrient enrichment. The decreased tpPO catecholase activity levels suggest that under nutrient enrichment P. porites has a limited capability to launch a quick, immune response when damaged. Furthermore, we identified predation-specific elevation in the laccase PO activity that requires future exploration to determine if this relates to previous hypotheses that parrotfishes can serve as vectors of coral disease. Notwithstanding, it is critical that we mitigate rising levels of nutrient pollution to coral reefs, as our findings indicate that this stressor likely hinders the immunological capacity of corals to respond to pathogens and contributes to documented patterns of increased disease and mortality on coral reefs.
Potential Future Studies
One limitation of enzymatic assays is the inability to discriminate between different sources of a particular enzyme. Due to the widespread prevalence of laccase-type PO activity in organisms ranging from bacteria to invertebrates, an important next step is to confirm the component of the coral holobiont responsible for increased laccase PO activity in response to predation. The coral holobiont also contains symbiotic bacteria, archaea, and fungi, all of which have been documented to produce laccase enzymes in other systems (Thurston, 1994; Mayer and Staples, 2002; Claus, 2004), therefore the generation and incorporation of omics data are needed. Interestingly, laccase enzymes are a common virulence factor employed by many species of fungi (Thurston, 1994), a member of the coral holobiont that is still not very well-understood (Bonthond et al., 2018). However, it has been suggested that some fungi might be potential parasites/pathoges in coral holobionts (Le Campion-Alsumard et al., 1995). If the observed increased laccase activity in the present study originated from fungal symbionts within the coral holobiont, instead of representing an increased immune defense, increased laccase activity could actually represent increased pathogenicity of a microbiome member toward the coral animal.
Data Availability Statement
The raw data supporting the conclusions of this article will be made available by the authors, without undue reservation.
Author Contributions
MR-L, RV, and DB designed and provided funds to carry out the experiments and wrote parts of the manuscript. KD executed all the immunological assays, analyzed the data, and wrote the major part of the manuscript. ML and CF carried out the experiments in the field and did some partial analyses. All authors contributed to the article and approved the submitted version.
Funding
This work was conducted with permission from the Florida Keys National Marine Sanctuary (permit no: FKNMS-2014-073) and supported by a grant from the National Science Foundation, Integrative Organismal System – CAREER (IOS-1453519) to ML, and Biological Oceanography Program (OCE-1130786) to DB and RV.
Conflict of Interest
The authors declare that the research was conducted in the absence of any commercial or financial relationships that could be construed as a potential conflict of interest.
Acknowledgments
We thank A. Durán for assistance in the field. This is contribution #214 from the Coastlines and Oceans Division of the Institute of Environment at Florida International University.
Supplementary Material
The Supplementary Material for this article can be found online at: https://www.frontiersin.org/articles/10.3389/fmars.2020.563865/full#supplementary-material
MATERIAL S1 | Proportional area of colony preyed upon by parrotfishes for ambient and enriched corals at each post-damage sampling time point.
MATERIAL S2 | Phenoloxidase assay ANOVA and Tukey post-hoc test results.
References
Aeby, G., and Santavy, D. (2006). Factors affecting susceptibility of the coral Montastraea faveolata to black-band disease. Mar. Ecol. Prog. Ser. 318, 103–110. doi: 10.3354/meps318103
Andersen, S. O. (2012). “Cuticular sclerotization and tanning,” in Insect Molecular Biology and Biochemistry, ed. L. I. Gilbert (Cambridge, MA: Academic Press), 167–192. doi: 10.1016/b978-0-12-384747-8.10006-6
Barros, J., Serk, H., Granlund, I., and Pesquet, E. (2015). The cell biology of lignification in higher plants. Ann. Bot. 115, 1053–1074. doi: 10.1093/aob/mcv046
Boesch, D. F. (2002). Challenges and opportunities for science in reducing nutrient over-enrichment of coastal ecosystems. Estuaries 25, 886–900. doi: 10.1007/bf02804914
Bonthond, G., Merselis, D. G., Dougan, K. E., Graff, T., Todd, W., Fourqurean, J. W., et al. (2018). Inter-domain microbial diversity within the coral holobiont Siderastrea siderea from two depth habitats. PeerJ 6:e4323. doi: 10.7717/peerj.4323
Bruno, J. F., Petes, L. E., Harvell, C. D., and Hettinger, A. (2003). Nutrient enrichment can increase the severity of coral diseases. Ecol. Lett. 6, 1056–1061. doi: 10.1046/j.1461-0248.2003.00544.x
Burkepile, D. E. (2012). Context-dependent corallivory by parrotfishes in a Caribbean reef ecosystem. Coral Reefs 31, 111–120. doi: 10.1007/s00338-011-0824-5
Cerenius, L., Bangyeekhun, E., Keyser, P., Söderhall, I., and Söderhall, K. (2003). Host prophenoloxidase expression in freshwater crayfish is linked to increased resistance to the crayfish plague fungus, Aphanomyces astaci. Cell. Microbiol. 5, 353–357. doi: 10.1046/j.1462-5822.2003.00282.x
Cerenius, L., Kawabata, S., Lee, B. L., Nonaka, M., and Söderhäll, K. (2010). Proteolytic cascades and their involvement in invertebrate immunity. Trends Biochem. Sci. 35, 575–583. doi: 10.1016/j.tibs.2010.04.006
Cerenius, L., Lee, B. L., and Söderhäll, K. (2008). The proPO-system: pros and cons for its role in invertebrate immunity. Trends Immunol. 29, 263–271. doi: 10.1016/j.it.2008.02.009
Cerenius, L., and Söderhall, K. (2004). The prophenoloxidase-activating system in invertebrates. Immunol. Rev. 198, 116–126. doi: 10.1111/j.0105-2896.2004.00116.x
Claus, H. (2004). Laccases: structure, reactions, distribution. Micron 35, 93–96. doi: 10.1016/j.micron.2003.10.029
D’Angelo, C., Smith, E. G., Oswald, F., Burt, J., Tchernov, D., and Wiedenmann, J. (2012). Locally accelerated growth is part of the innate immune response and repair mechanisms in reef-building corals as detected by green fluorescent protein (GFP)-like pigments. Coral Reefs 31, 1045–1056. doi: 10.1007/s00338-012-0926-8
Diaz, R. J., and Rosenberg, R. (2008). Spreading dead zones and consequences for marine ecosystems. Science 321, 926–929.
Dwivedi, U. N., Singh, P., Pandey, V. P., and Kumar, A. (2011). Structure–function relationship among bacterial, fungal and plant laccases. J. Mol. Catal. BEnzym. 68, 117–128. doi: 10.1016/j.molcatb.2010.11.002
Fabricius, K. E. (2005). Effects of terrestrial runoff on the ecology of corals and coral reefs: review and synthesis. Mar. Pollut. Bull. 50, 125–146. doi: 10.1016/j.marpolbul.2004.11.028
Fuess, L. E., Mann, W. T., Jinks, L. R., Brinkhuis, V., and Mydlarz, L. D. (2018). Transcriptional analyses provide new insight into the late-stage immune response of a diseased caribbean coral. R. Soc. Open Sci. 5:172062. doi: 10.1098/rsos.172062
Fuess, L. E., Pinzün,, J. H., Weil, E., and Mydlarz, L. D. (2016). Associations between transcriptional changes and protein phenotypes provide insights into immune regulation in corals. Dev. Comp. Immunol. 62, 17–28. doi: 10.1016/j.dci.2016.04.017
Gignoux-Wolfsohn, S. A., Marks, C. J., and Vollmer, S. V. (2012). White Band Disease transmission in the threatened coral, Acropora cervicornis. Sci. Rep. 2:804.
Granéli, E., Weberg, M., and Salomon, P. S. (2008). Harmful algal blooms of allelopathic microalgal species: the role of eutrophication. Harmful Algae 8, 94–102. doi: 10.1016/j.hal.2008.08.011
Harvell, C. D., Kim, K., Burkholder, J. M., Colwell, R. R., Epstein, P. R., Grimes, D. J., et al. (1999). Emerging marine diseases–climate links and anthropogenic factors. Science 285, 1505–1510. doi: 10.1126/science.285.5433.1505
Hayes, M. L., Bonaventura, J., Mitchell, T. P., Prospero, J. M., Shinn, E. A., Van Dolah, F., et al. (2001). How are climate and marine biological outbreaks functionally linked? Hydrobiologia 460, 213–220. doi: 10.1007/978-94-017-3284-0_19
Howarth, R. W. (2008). Coastal nitrogen pollution: a review of sources and trends globally and regionally. Harmful Algae 8, 14–20. doi: 10.1016/j.hal.2008.08.015
Kelly, L. A., Heintz, T., Lamb, J. B., Ainsworth, T. D., and Willis, B. (2016). Ecology and pathology of novel plaque-like growth anomalies affecting a reef-building coral on the great barrier reef. Front. Mar. Sci 3:151. doi: 10.3389/fmars.2016.00151
Le Campion-Alsumard, T., Golubic, S., and Priess, K. (1995). Fungi in corals: symbiosis or disease? Interaction between polyps and fungi causes pearl-like skeleton biomineralization. Mar. Ecol. Prog. Ser. 117, 137–147. doi: 10.3354/meps117137
Mayer, A. M., and Staples, R. C. (2002). Laccase: new functions for an old enzyme. Phytochemistry 60, 551–565. doi: 10.1016/s0031-9422(02)00171-1
Mydlarz, L., Couch, C., Weil, E., Smith, G., and Harvell, C. (2009). Immune defenses of healthy, bleached and diseased Montastraea faveolata during a natural bleaching event. Dis. Aquat. Org. 87, 67–78. doi: 10.3354/dao02088
Mydlarz, L. D., Holthouse, S. F., Peters, E. C., and Harvell, C. D. (2008). Cellular responses in sea fan corals: granular amoebocytes react to pathogen and climate stressors. PLoS One 3:e1811. doi: 10.1371/journal.pone.0001811
Mydlarz, L. D., and Palmer, C. V. (2011). The presence of multiple phenoloxidases in Caribbean reef-building corals. Comp. Biochem. Physiol. A Mol. Integr. Physiol. 159, 372–378. doi: 10.1016/j.cbpa.2011.03.029
Nappi, A. J., and Christensen, B. M. (2005). Melanogenesis and associated cytotoxic reactions: applications to insect innate immunity. Insect Biochem. Mol. Biol. 35, 443–459. doi: 10.1016/j.ibmb.2005.01.014
Nappi, A. J., and Ottaviani, E. (2000). Cytotoxicity and cytotoxic molecules in invertebrates. Bioassays 22, 469–480. doi: 10.1002/(sici)1521-1878(200005)22:5<469::aid-bies9>3.0.co;2-4
Nava, H., and Ramírez-Herrera, M. T. (2012). Land use changes and impact on coral communities along the central Pacific coast of Mexico. Environ. Earth Sci. 65, 1095–1104. doi: 10.1007/s12665-011-1359-3
Nigam, Y., Maudlin, I., Welburn, S., and Ratcliffe, N. A. (1997). Detection of phenoloxidase activity in the Hemolymph of tsetse flies, refractory and susceptible to infection with Trypanosoma brucei rhodesiense. J. Invertebr. Pathol. 69, 279–281. doi: 10.1006/jipa.1996.4652
Nixon, S. W. (1995). Coastal marine eutrophication: a definition, social causes, and future concerns. Ophelia 41, 199–219. doi: 10.1080/00785236.1995.10422044
Oksanen, J., Blanchet, G. F., Friendly, M., Kindt, R., Legendre, P., McGlinn, D., et al. (2018). vegan: Community Ecology Package. Available online at: https://cran.r-project.org/package=vegan
Page, C. A., and Willis, B. L. (2008). Epidemiology of skeletal eroding band on the great barrier reef and the role of injury in the initiation of this widespread coral disease. Coral Reefs 27, 257–272. doi: 10.1007/s00338-007-0317-8
Palmer, C. V. (2018). Warmer water affects immunity of a tolerant reef coral. Front. Mar. Sci. 5:253. doi: 10.3389/fmars.2018.00253
Palmer, C. V., Bythell, J. C., and Willis, B. L. (2010). Levels of immunity parameters underpin bleaching and disease susceptibility of reef corals. FASEB J. 24, 1935–1946. doi: 10.1096/fj.09-152447
Palmer, C. V., Bythell, J. C., and Willis, B. L. (2011a). A comparative study of phenoloxidase activity in diseased and bleached colonies of the coral Acropora millepora. Dev. Comp.Immunol. 35, 1098–1101. doi: 10.1016/j.dci.2011.04.001
Palmer, C. V., Bythell, J. C., and Willis, B. L. (2012a). Enzyme activity demonstrates multiple pathways of innate immunity in Indo-Pacific anthozoans. Proc. Biol. Sci. R. Soc 279, 3879–3887. doi: 10.1098/rspb.2011.2487
Palmer, C. V., Graham, E. M., and Baird, A. H. (2012b). Immunity through early development of coral larvae. Dev. Comp. Immunol. 38, 395–399. doi: 10.1016/j.dci.2012.07.008
Palmer, C. V., McGinty, E. S., Cummings, D. J., Smith, S. M., Bartels, E., and Mydlarz, L. D. (2011b). Patterns of coral ecological immunology: variation in the responses of Caribbean corals to elevated temperature and a pathogen elicitor. J. Exp. Biol. 214, 4240–4249. doi: 10.1242/jeb.061267
Palmer, C. V., Mydlarz, L. D., and Willis, B. L. (2008). Evidence of an inflammatory-like response in non-normally pigmented tissues of two scleractinian corals. Proc. Biol. Sci. R. Soc. 275, 2687–2693. doi: 10.1098/rspb.2008.0335
Precht, W. F., Gintert, B. E., Robbart, M. L., Fura, R., and van Woesik, R. (2016). Unprecedented disease-related coral mortality in southeastern Florida. Sci. Rep. 6:31374.
R Core Team (2014). R: A Language and Environment for Statistical Computing. Vienna, Austria. Available online at: https://www.R-project.org/
Rohwer, F., Seguritan, V., Azam, F., and Knowlton, N. (2002). Diversity and distribution of coral-associated bacteria. Mar. Ecol. Prog. Ser. 243, 1–10. doi: 10.3354/meps243001
Rotjan, R., and Lewis, S. (2008). Impact of coral predators on tropical reefs. Mar. Ecol. Prog. Ser. 367, 73–91. doi: 10.3354/meps07531
Shaver, E. C., Shantz, A. A., McMinds, R., Burkepile, D. E., Vega Thurber, R. L., and Silliman, B. R. (2017). Effects of predation and nutrient enrichment on the success and microbiome of a foundational coral. Ecology 98, 830–839. doi: 10.1002/ecy.1709
Sheridan, C., Grosjean, P., Leblud, J., Palmer, C. V., Kushmaro, A., and Eeckhautm, I. (2014). Sedimentation rapidly induces an immune response and depletes energy stores in a hard coral. Coral Reefs 33, 1067–1076. doi: 10.1007/s00338-014-1202-x
Söderhall, K. (1982). Prophenoloxidase activating system and melanization -a recognition mechanism of arthropods? A review. Dev. Comp. Immunol. 6, 601–611.
Sugumaran, M. (2002). Comparative biochemistry of eumelanogenesis and the protective roles of phenoloxidase and melanin in insects. Pigment Cell Res. 15, 2–9. doi: 10.1034/j.1600-0749.2002.00056.x
Thurston, C. F. (1994). The structure and function of fungal laccases. Microbiology 1743, 19–26. doi: 10.1099/13500872-140-1-19
Todd, P. A., Ong, X., and Chou, L. M. (2010). Impacts of pollution on marine life in Southeast Asia. Biodivers. Conserv. 19, 1063–1082. doi: 10.1007/s10531-010-9778-0
van de Water, J. A. J. M., Chaib De Mares, M., Dixon, G. B., Raina, B. J., Willis, B. L., Bourne, D. G., et al. (2018). Antimicrobial and stress responses to increased temperature and bacterial pathogen challenge in the holobiont of a reef-building coral. Mol. Ecol. 27, 1065–1080. doi: 10.1111/mec.14489
van de Water, J. A. J. M., Ainsworth, T. D., Leggat, W., Bourne, D. G., Willis, B. L., and van Oppen, M. J. H. (2015a). The coral immune response facilitates protection against microbes during tissue regeneration. Mol. Ecol. 24, 3390–3404. doi: 10.1111/mec.13257
van de Water, J. A. J. M., Lamb, J. B., Heron, S. F., Van Oppen, M. J. H., and Willis, B. L. (2016). Temporal patterns in innate immunity parameters in reef-building corals and linkages with local climatic conditions. Ecosphere 7:e01505.
van de Water, J. A. J. M., Lamb, J. B., van Oppen, M. J. H., Willis, B. L., and Bourne, D. G. (2015b). Comparative immune responses of corals to stressors associated with offshore reef-based tourist platforms. Conserv. Physiol. 3:cov032. doi: 10.1093/conphys/cov032
Vega Thurber, R. L., Burkepile, D. E., and Fuchs, C. (2014). Chronic nutrient enrichment increases prevalence and severity of coral disease and bleaching. Glob. Change Biol. 20, 544–554. doi: 10.1111/gcb.12450
Vega Thurber, R. L., Willner-Hall, D., Rodriguez-Mueller, B., Desnues, C., Edwards, R. A., Angly, F., et al. (2009). Metagenomic analysis of stressed coral holobionts. Environ. Microbiol. 11, 2148–2163. doi: 10.1111/j.1462-2920.2009.01935.x
Voss, J. D., and Richardson, L. L. (2006). Nutrient enrichment enhances black band disease progression in corals. Coral Reefs 25, 569–576. doi: 10.1007/s00338-006-0131-8
Wall, C. B., Ricci, C. A., Foulds, G. E., Mydlarz, L. D., Gates, R. D., and Putnam, H. M. (2018). The effects of environmental history and thermal stress on coral physiology and immunity. Mar. Biol. 165:56.
Weil, E. (2004). “Coral Reef Diseases in the Wider Caribbean,” in Coral Health and Disease Rosenberg, E., and Loya, Y. (Berlin: Springer).
Williams, D. E., and Miller, M. W. (2005). Coral disease outbreak?: pattern, prevalence and transmission in Acropora cervicornis. Mar. Ecol. Prog. Ser. 301, 119–128. doi: 10.3354/meps301119
Zaneveld, J. R., Burkepile, D. E., Shantz, A. A., Pritchard, C. E., McMinds, R., Payet, J. P., et al. (2016). Overfishing and nutrient pollution interact with temperature to disrupt coral reefs down to microbial scales. Nat. Commun. 7:11833.
Zaneveld, J. R., McMinds, R., and Vega Thurber, R. (2017). Stress and stability: applying the Anna Karenina principle to animal microbiomes. Nat. Microbiol. 2:17121.
Keywords: phenoloxidase, Porites porites, nutrients, parrotfish, coral, predation, disease, corallivory
Citation: Dougan KE, Ladd MC, Fuchs C, Vega Thurber R, Burkepile DE and Rodriguez-Lanetty M (2020) Nutrient Pollution and Predation Differentially Affect Innate Immune Pathways in the Coral Porites porites. Front. Mar. Sci. 7:563865. doi: 10.3389/fmars.2020.563865
Received: 19 May 2020; Accepted: 13 August 2020;
Published: 03 September 2020.
Edited by:
Linda Wegley Kelly, San Diego State University, United StatesReviewed by:
Robert H. Richmond, University of Hawai‘i at Mānoa, United StatesClaudia Pogoreutz, University of Konstanz, Germany
Copyright © 2020 Dougan, Ladd, Fuchs, Vega Thurber, Burkepile and Rodriguez-Lanetty. This is an open-access article distributed under the terms of the Creative Commons Attribution License (CC BY). The use, distribution or reproduction in other forums is permitted, provided the original author(s) and the copyright owner(s) are credited and that the original publication in this journal is cited, in accordance with accepted academic practice. No use, distribution or reproduction is permitted which does not comply with these terms.
*Correspondence: Mauricio Rodriguez-Lanetty, cm9kbWF1cmlAZml1LmVkdQ==; rodriguezlanetty@gmail.com