- 1GEOMAR Helmholtz Centre for Ocean Research Kiel, Kiel, Germany
- 2Coral Reef Ecology and Optics Laboratory, Bermuda Institute of Ocean Sciences (BIOS), Saint George, Bermuda
- 3Bazaruto Center for Scientific Studies (BCSS), Benguerra Island, Mozambique
- 4State Key Laboratory of Tropical Oceanography, South China Sea Institute of Oceanology, Chinese Academy of Sciences, Guangzhou, China
Artificial upwelling (AU) is a novel geoengineering technology that brings seawater from the deep ocean to the surface. Within the context of global warming, AU techniques are proposed to reduce sea surface temperature at times of thermal stress around coral reefs. A computationally fast but coarse 3D Earth System model (3.6° longitude × 1.8° latitude) was used to investigate the environmental impacts of hypothetically implemented AU strategies in the Great Barrier Reef, South China Sea, and Hawaiian regions. While omitting the discussion on sub-grid hydrology, we simulated in our model a water translocation from either 130 or 550 m depth to sea surface at rates of 1 or 50 m3 s–1 as analogs to AU implementation. Under the Representative Concentration Pathway 8.5 emissions scenario from year 2020 on, the model predicted a prevention of coral bleaching until the year 2099 when AU was implemented, except under the least intense AU scenario (water from 130 m depth at 1 m3 s–1). Yet, intense AU implementation (water from 550 m depth at 50 m3 s–1) will likely have adverse effects on coral reefs by overcooling the surface water, altering salinity, decreasing calcium carbonate saturation, and considerably increasing nutrient levels. Our result suggests that if we utilize AU for mitigating coral bleaching during heat stress, AU implementation needs to be carefully designed with respect to AU’s location, depth, intensity and duration so that undesirable environmental effects are minimized. Following a proper installation and management procedure, however, AU has the potential to decelerate destructive bleaching events and buy corals more time to adjust to climate change.
Introduction
Shallow-water coral reefs sustain some of the most biodiverse ecosystems on the planet, providing numerous ecosystem services and values to both humans and the environment (Deloitte Access Economics, 2013). Thriving in the euphotic zone at tropical latitudes, corals face increasing temperature-induced bleaching events (Aronson et al., 2002; Hughes et al., 2018). During bleaching events the corals expel the zooxanthellae (symbiotic algae) living within their tissue as a response to the high temperature stress, leaving the white coral skeletons behind. Because zooxanthellae provide, through photosynthesis, most of the energy required by their hosts, the loss of zooxanthellae can lead to coral starvation and death, and ultimately to reef degradation. According to the current tendency of economy and population growth, Earth’s temperature is predicted to still increase across 1.5°C warming target by the end of 21st century, even if current climate mitigation efforts are adopted (Raftery et al., 2017). Sea surface temperature (SST) will rise in the next decades and increase the probability of extreme marine heat events (Frölicher and Laufkötter, 2018) and hence, the frequency and intensity of mass coral bleaching events contributing to reef degradation worldwide (Hughes et al., 2017). Consequently, climate change adaptation measures that could provide a longer time window for corals to cope with rising temperature, are needed along with the climate mitigation efforts.
Natural upwelling, which introduces cooler sub-thermocline water into shallow reef areas, can effectively cool surface waters, and thus provide temporal refugia for coral reefs when upwelling coincides with thermal events (Jiménez et al., 2001; Bayraktarov et al., 2013; Chollett and Mumby, 2013; Wall et al., 2015). Upwelling can also be achieved with a geoengineering technology called artificial upwelling (AU). Powered by either ocean waves, solar or ocean thermal energy, AU is designed to pump seawater adiabatically from depths below the thermocline to the sea surface, and discharges the upwelled water via a floating platform (Kirke, 2003). So far, AU has been considered primarily to increase surface ocean primary productivity by pumping up nutrient-rich deep water, to either enhance CO2 sequestration from the atmosphere and thereby mitigate global warming (Liu and Jin, 1995; Kirke, 2003) or to increase the yield of fisheries (Viúdez et al., 2016). AU, however, will also cool the ocean surface by up to several degrees Celsius (Oschlies et al., 2010) inferring that AU may be a viable tool to reduce heat stress for corals.
In order to gain a first understanding on the effect of AU on reef waters and the surrounding system, we simulated the implementation of vertical tracer translocation as an analog to AU (hereafter for descriptive convenience, we do not distinguish between AU and water translocation unless specifically noted), in three major coral reef systems in the Indo-Pacific (Great Barrier Reef, South China Sea, Hawaiian islands) from year 2020 to 2100, using the University of Victoria Earth System Model (UVic). The model uses a spatial and temporal resolution that allows a cost-effective representation of the complex evolution of three-dimensional (3D) ocean hydrology and biogeochemistry under future climate change. Although this rather coarse model does not provide definitive answers regarding AU implementation on small local scales, its main advantage lays in the simultaneous exploration of multiple parameters that may be altered by AU in the context of global warming, which is the main goal of this study. Therefore, we investigated the potential efficiency of AU in mitigating coral bleaching by analyzing its effects on SST and accumulated heat stress in the three test regions. We also assessed the potential side effects of AU practices in terms of changes in ocean circulation and marine biogeochemistry (i.e., ocean temperature, salinity, nutrients and calcium carbonate saturation states et al.). We hypothesized that AU will reduce SST and coral bleaching effectively; however, depending on the implementation strategy of AU, some undesirable side effects might occur. Possible side effects include severe reduction in temperature (overcooling), salinity changes, acidification and nutrient-enrichment, which may affect the biogeochemistry and hydrology of reef waters as well as of neighboring water masses. Depending on the severity of these changes and the susceptibility of ecosystems, this may entail substantial pressure on coral health and ecosystem functioning (Kleypas et al., 1999; Guan et al., 2015). For example, excessively cooled water can have similarly negative effects on the corals’ metabolism as thermal stress (Saxby et al., 2003; Lirman and Manzello, 2009; Kemp et al., 2011; Roth et al., 2012). Seemingly, the enrichment of dissolved inorganic carbon (DIC), and subsequent reduction of ocean pH, can impair coral growth (e.g., calcification) (Schneider and Erez, 2006). Increased nitrogen and phosphorus input may promote macroalgae growth, which could outcompete corals (McCook, 1999; Szmant, 2002) and may also affect coral health (Wiedenmann et al., 2012). Generally speaking, any drastic change in the physical and chemical properties of water masses has the potential to affect exposed organisms, which can ultimately alter ecosystem composition and functioning. Therefore, before AU can be implemented, we need to gain a holistic understanding of the benefits and risks of AU. The first step towards that goal is to numerical model effect of AU on the water properties, which will provide some initial insight into answers for the following questions:
(i) Can AU reduce the duration and intensity of heat stress in coral reef systems?
(ii) What are the possible adverse environmental effects of AU?
(iii) What are the optimal AU operational strategies that would lead to successful bleaching mitigation, with minimum harmful impacts on environment and acceptable costs?
Materials and Methods
Model Description
Model simulations were performed with the coarse-resolution Earth system model University of Victoria Earth System Climate Model (UVic) version 2.9 (Weaver et al., 2001). The ocean component of UVic is formed by a general ocean circulation model (Pacanowski, 1996) coupled to a nutrient–phytoplankton–zooplankton–detritus (NPZD) module and a marine carbon cycle, so that 3D marine biogeochemical processes can be represented. The terrestrial module of UVic is modified from the Top-down Representation of Interactive Foliage and Flora Including Dynamics (TRIFFID) vegetation model (Meissner et al., 2003). Within UVic, the atmosphere is described by an energy–moisture balance model (Fanning and Weaver, 1996), and processes related to sea ice are characterized with a sea ice module (Bitz and Liscomb, 1999). The oceanic component has a horizontal resolution of 3.6° longitude × 1.8° latitude. A full seawater column has 19 layers, with a gradually coarser resolution starting from 50 m thickness near the surface, up to 500 m thickness at the deepest layer near the ocean floor. The UVic’s isopycnal mixing follows Gent-McWilliams parameterization (800 m2 s–1 for diffusivities) for mesoscale eddies and Bryan-Lewis scheme for the vertical tracer diffusivity (0.3 – 1.3 cm2 s–1)(Weaver et al., 2001). Ocean wave states are not considered for air-sea heat, momentum, gas, aerosol and moisture fluxes, hence no wave excitation energy accounted. The simulated SST and oceanic pCO2 were evaluated against data from the World Ocean Atlas (Locarnini et al., 2013), and the Surface Ocean CO2 Atlas (Landschützer et al., 2014; Feng et al., 2016). This evaluation showed that UVic simulates sea surface pCO2 within the range of observations, and the resulting SST was also well within range of date-error bands from the Coupled Model Intercomparison Project (CMIP) models (Wang et al., 2014; Feng et al., 2016). UVic has been previously used to investigate the climate mitigation potential of regionally implemented ocean alkalinization under a business-as-usual emission scenario, in the Great Barrier Reef, Caribbean Sea, and South China Sea (Feng et al., 2016). The robustness of its representation of the equatorial current system in the upper Pacific Ocean has also been demonstrated (Supplementary Text S1 and Figure S1). Despite the relatively coarse spatial resolution of UVic, the results of previous modeling studies (Oschlies et al., 2010; Keller et al., 2014) indicate it is an effective tool to simulate AU for providing a first understanding on how AU may effect SST, biogeochemistry and hydrology of ocean waters and presenting referential information to assist future assessment on this topic.
In this study, the fast and inexpensive global UVic model was chosen over slow and expensive regional models, since it is an efficient tool to gain some initial insights into how water translocation can affect the physical characteristics and the biogeochemistry of surface waters and the water column. To the best of our knowledge, even for very fine-resolution biogeochemical models, simulating detailed ocean dynamics and biogeochemistry near shallow coral-inhabiting waters remains a challenge because of the complex nature of shallow-water environments (Zhang et al., 2012; Lessin et al., 2018) and the difficulties in coupling ocean currents, waves, and biogeochemistry within numerical models (Mongin and Baird, 2014). Coupling AU hydrological modules with a regional biogeochemical model imposes another technical challenge. Such sophisticated numerical coupler has only been seen from environmental assessments provided by dedicated enterpriser teams (Pat Grandelli et al., 2012). The UVic model instead, allows simulations of complex 3D physical and biogeochemical properties on large spatial scales, from which key information is transformable and valuable to understanding smaller scale (localized) impacts where knowledge on 3D dynamics are limited. Therefore, with carefully designed simulations to minimize possible caveats, UVic can be employed as an useful tool to studying ocean interventions at both global and regional scales (Bonan and Doney, 2018), especially to gain a first understanding in a timely and efficient fashion (Keller et al., 2014; Feng et al., 2016).
Regions of Interest
We chose experimental areas larger than 100,000 km2 for testing AU to fully utilize the current advantage of UVic that is capable of simulating marine biogeochemistry across large ocean basins efficiently and robustly in three dimensions. With this large-scaled implementation of AU and the global coverage of UVic, mesoscale ocean dynamics, such as eddies, are presumed insignificant in modulating AU plumes, hence not necessarily less accurate than high-resolution models (Petoukhov et al., 2005). Accordingly, the trajectories of upwelled water over long distances are obtainable for investigating the impacts of AU beyond our regions of interest. Those large-scaled simulations could also be viewed as ambitious AU implementation scenarios with extremely large amount of water being manually relocated, which is scientifically meaningful to investigate the possible environmental constraints. Those important features usually cannot be offered by regional models in a cost-effective manner.
The Great Barrier Reef (GBR; 140.4°E–154.8°E and 9.0°S–27.0°S, 1.7 × 106 km2), South China Sea (SCS; 104.4°E–129.6°E and 0°N–23.4°N, 5.2 × 106 km2), and Hawaii (HWI; 176.4°E–151.2°W and 16.2°N–27°N, 4.0 × 106 km2) were chosen as the test regions (Figure 1 and Supplementary Figure S2). Corals from these regions have experienced bleaching in the past (Marshall and Baird, 2000; Jokiel and Brown, 2004; Li et al., 2011; Waheed et al., 2015; Hughes et al., 2017). GBR, SCS and HWI together contain more than 50% of the coral reef locations in the world, while GBR and SCS have both been used as test regions in previous modeling experiments to investigate the implementation of other geoengineering strategies (Feng et al., 2016). GBR and SCS represented continental marginal seas, whereas HWI was selected as an open ocean setting. Our aim was to investigate the possible environmental changes caused by AU implemented in regions with distinct hydrographic and topographic patterns.
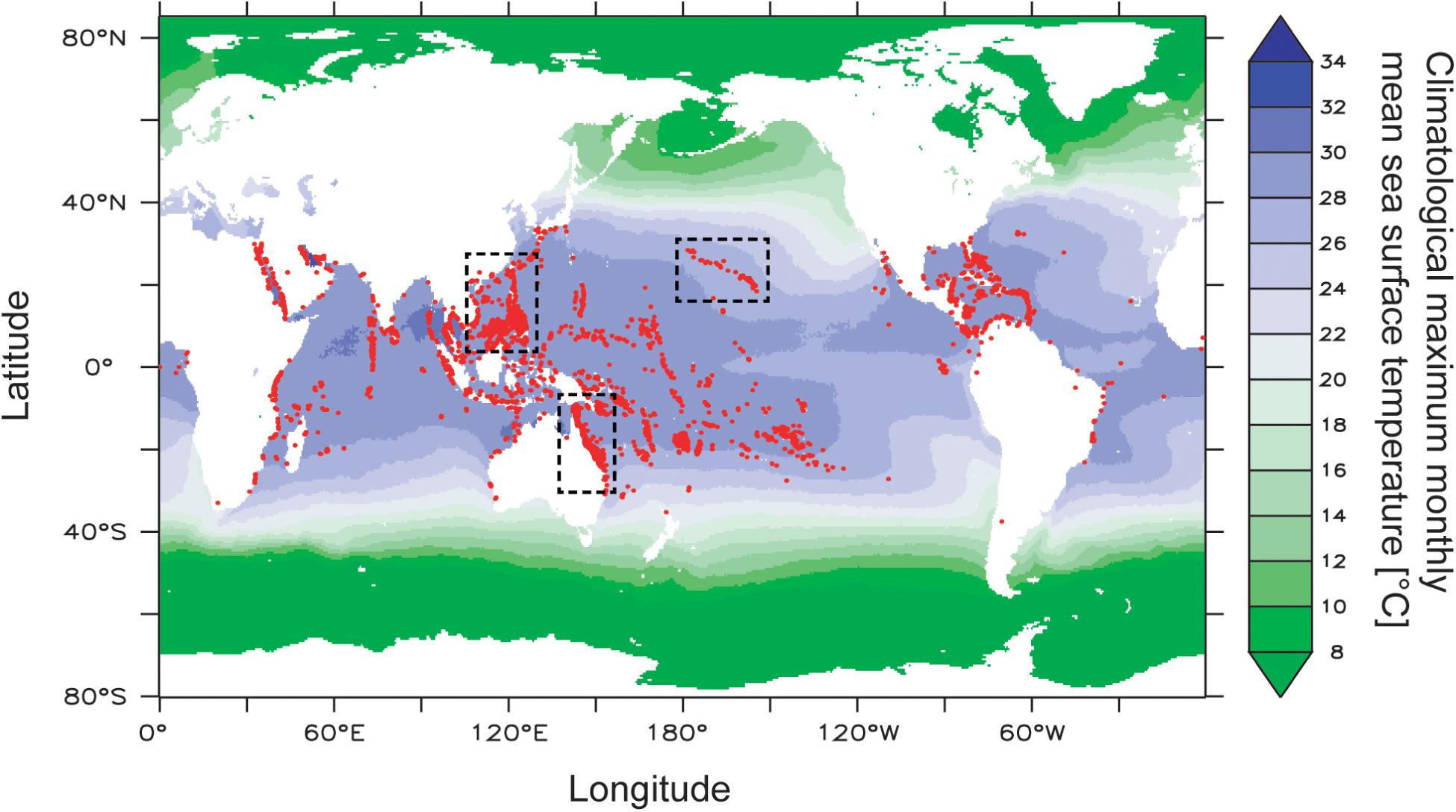
Figure 1. Map containing grid cells in which artificial upwelling (AU) has been implemented (marked with black boxes), overlaid onto the maximum monthly mean (Max-MM) sea surface temperature (SST) based on 1985–1993 climatology data. Max-MM SST data was downloaded from Coral Reef Watch (https://coralreefwatch.noaa.gov/satellite/hdf/index.php), and the coral reef locations, marked as red spots, were obtained from ReefBase (http://www.reefbase.org/main.aspx).
Simulating AU and Model Run Setup
Since the UVic model can simulate large scale ocean warming under climate change, we analyzed the environmental changes under the long-termed future warming trajectory for cases without and with the implementation of AU. We focused on the variance between different AU scenarios and the control run (no AU) instead of analyzing the absolute values calculated from the AU simulations. By investigating the differences in the model outputs of control versus AU runs, we were able to examine the environmental changes caused by AU while minimizing the model-oriented biases. The model was spun-up for 10,000 years from the preindustrial period with a prescribed atmospheric CO2 concentration and then ran for another 200 years to year 1765 without it to avoid climatic drifts. It was run further from year 1765 to 2005 under historical CO2 emissions forcing, followed by another period of simulation until the end of 2099 under the Representative Concentration Pathway 8.5 (RCP 8.5) high-CO2-emission scenario (Meinshausen et al., 2011) as extensive forcing. We chose the model simulation from 2020 to the end of 2099 for the control run (no AU) and for the AU simulations.
As UVic cannot resolve the sub-grid hydrodynamics rigorously, the simulation of AU cannot comply to parameterizations in respect to water lifting, discharging and remixing from in situ experiments (White et al., 2010). Therefore, in this study we described AU process as a special oceanic tracer (temperature, salinity, et al.) translocation from one depth to the surface with no detailed characterization of the upwelling hydrology, and used flow units (Sv) to constrain the assigned AU rates. This approach could impose uncertainties given the simplification of crucial hydrological processes, thus future studies are required to test the validity of such simplification as well as suggesting improvements to overcome such common caveat. With currently no related studies available to validate or invalidate such a method, we hereby use this method to investigate AU’s environmental impacts, focusing on crucial parameters like temperature, nutrients and aragonite saturation state while omitting the hydrodynamic component. Currently proposed AU prototypes pump seawater at rates ranging from 1 to 50 m3 s–1 (Liu and Jin, 1995; Kirke, 2003; Trench et al., 2004) with pipes that can extend down to several thousand meters below the sea surface (Matsuda et al., 1999; Kirke, 2003). Since the UVic model does not resolve the sub-grid hydrology below its resolution, simulating AU by 1 m3 s–1 at a geographic density of 1 device per km2 is equivalent to simulating it by 0.01 m3 s–1 at a density of 100 devices per km2. We chose the upper and lower ends of potential AU upwelling rates (1 and 50 m3 s–1), and used the suffix “low” (1 m3 s–1) or “high” (50 m3 s–1) in the names of the model runs to indicate the upwelling rate applied. It is noted that in practice, such upwelling rates might not lead to full surface mixing as the AU plumes can be diluted and sink rapidly (Pan et al., 2019). In the model, we set that AU operating at rate 1 or 50 m3 s–1 were virtually deployed uniformly at density of one device per square kilometer, and such scenario also refers to more realistic implementation strategies under which upwelling rates and density at localized scales could be adjusted as long as the area-integrated flow rate match the values given in Sv (1.7, 5.2 and 4.0 Sv for “low” runs, and 85, 260, 200 Sv for “high” runs). We set the local seawater to be upwelled vertically from a depth of 130 or 550 m below the sea surface, therefore the suffix “shallow” for 130 m or “deep” for 550 m was added to the names of these AU runs. If the seawater column was shallower than these two depths, the water was upwelled from the deepest ocean layer possible. We also assumed that the upwelled water was adiabatically transported, with no changes in its original physical or chemical properties during the translocation process (Oschlies et al., 2010). The four AU scenarios, namely “shallow_low,” “shallow_high,” “deep_low,” and “deep_high,” as analogs to AU implementations, and a control run without AU, were simulated to examine the effect of AU on temperature, hydrodynamics and biogeochemical properties of ocean water. This is expected to provide some insight into how different AU scenarios may affect coral reefs during thermal stress.
Characterization of the Occurrence and Severity of Coral Bleaching
Coral bleaching is predominantly induced by accumulated heat stress over a given period of time. Corals are reported to be stressed under abnormally elevated sea temperatures (defined as the bleaching threshold), which is commonly found to be 1°C higher than the maximum mean temperature during the climatological summer months (Max-MM) (Ncrw, 2000). To quantify heat stress, the metric “degree heating weeks” (DHW; unit:°C-week) is used, which is calculated by adding the positive weekly mean temperature anomaly referenced to bleaching threshold over a period of 84 days (12 weeks). Coral bleaching events are most likely to appear when DHW starts to exceed 4°C-weeks, while areas with DHW higher than 8°C-weeks have a high probability of bleaching and consequent coral mortality (Liu et al., 2006). Hence, bleaching threat level 1 (BL-LV1) is defined by DHW of 4°C-weeks to 8°C-weeks, and bleaching threat level 2 (BL-LV2) by DHW > 8°C-weeks. In this study, we used a modified version of DHW, namely “degree heating 5 days” (DH-5d; unit:°C-5 days), to characterize the occurrence, duration, and intensity of coral bleaching threat. DH-5d instead of DHW was used, because the UVic model worked with a 5-day mean temperature anomaly instead of a weekly mean. Accordingly, the corresponding bleaching alert levels were 5.7°C-5 days and 11.33°C-5 days for BL-LV1 and BL-LV2, respectively.
Max-MM SST of each study regions was derived from satellite-based datasets from year 1985 to 1993 (excluding 1991 and 1992. Liu et al., 2006) downloaded from CoralWatch (Ncrw, 2000), and the following bleaching thresholds were calculated for each region: 29.57°C for GBR, 30.50°C for SCS, and 28.30°C for HWI. The model results were presented as time series of area-averaged 5-day SST (5 day-SST) values for each region from 2020 to 2099. In the next step, SST positive anomalies were calculated by subtracting the bleaching threshold of each region from the respective 5 day-SST values. Time points with no positive anomaly were assigned to the value zero. Finally, the sum of the calculated SST anomalies over a period of 85 days were calculated (i.e., 85 days adjusting for the temporal interval of UVic, approximately 12 weeks) from the beginning of 2020 to the end of 2099, providing a time series of DH-5d for each region. These procedures were applied to each of the five model runs (one control and four AU runs).
Coral bleaching and subsequent coral death do not only occur during high temperature anomalies, but can also during abnormally cold periods (Hoegh-Guldberg and Fine, 2004; Lirman et al., 2011). Cold water stress event are, however, much less studied than heat stress events, therefore no comparable bleaching thresholds exist for cold stress events. To estimate potential stressful conditions due to overcooling, we simply compared the area-averaged SST time series for the three test regions (GBR, SCS, and HWI) with the coldest monthly mean SST among all 12 months for the UVic climatological period 2000–2020 (referred as Min-MM SST; area-mean values: 24.74°C for GBR, 26.2°C for SCS, and 23.01°C for HWI). We assumed that corals experiencing temperatures below Min-MM SST could be stressed and calculated the number of days that would undergo < Min-MM SST conditions for all model runs. This provides an estimate of potential AU-induced cold water stress.
Characterization of the Environmental Changes Driven by AU
Besides SST, we also examined the AU-induced changes in sea water salinity, aragonite saturation state (Ω-Arag), and nutrient concentration (e.g., nitrate), at the implementation sites and in surrounding areas. These properties are known as important environmental drivers for the functioning of various coral reef organisms as well as for ocean dynamics (e.g., currents). As for temperature, thresholds of these parameters are strongly dependent on the history of local conditions to which organisms and communities are adjusted. However, since these thresholds are much less well defined than temperature thresholds, we used conditions that have been describe as the boundaries of shallow water coral reef habitat distribution worldwide (Kleypas, 1997). This includes a lower and upper salinity threshold of 28.7 and 40.4 PSU, a lower Ω-Arag threshold of 2.82, and an upper nitrate concentration threshold of 4.51 μmol L–1 (Guan et al., 2015). Those thresholds roughly frame the conditions in which tropical shallow water corals exist, and we therefore used them as a reference to discuss AU-induced environmental perturbations and their potential effect on corals and coral reefs.
Results and Discussion
The model results provide insights on the effects of simulated AU on a very large spatial scale (106 km2-scale). While, in practice AU would be implemented on much smaller scales (likely < km2-scale), the model results can still provide a much required baseline of understanding about how AU may change temperature regimes as well as other parameters that may or may not cause unwanted side effects. Therefore, in the following, we will discuss the knowledge gained from the large-scale model in the context of small-scale/localized AU in coral reefs, assuming that similar dynamics of flow patterns and water mixing occur on large and small spatial scales, while keeping in mind the existing uncertainties from other high-resolution models in simulating coral reef ecosystems.
Impact of AU on SST and Coral Bleaching Threat
Overall, the model results showed that AU reduced SST markedly and hence the number of days of thermal stress. Furthermore, on the 80-year scale, AU caused significant reduction in the occurrence of bleaching events, assuming that bleaching thresholds are not changing over time. More specifically, under control conditions, the model predicted an increase in the area-averaged annual mean SST (aa-SST) over the next 80 years of 1.9–2.4°C for the three test regions. Under simulated year-round upwelling, the model predicted the following reductions in aa-SST compared with that in the control run by the end of this century (year 2099): shallow_low by 0.06 – 0.2°C, shallow_high by 0.2 – 1.0°C, deep_low by 1.5 – 2.3°C, and deep_high by 4.6 – 8.9°C (Supplementary Figure S3). AU induced reductions in SST resulted in a reduction of number of days under BL-LV1 and BL-LV2 conditions (Figures 2A–C). Under control conditions and across the entire 80-year period from 2020 to 2099, predicted climate change resulted in approximately 12,145 (GBR), 10,440 (SCS), and 430 (HWI) days (Supplementary Figure S4) under bleaching threatening (BL-LV1&2, including BL-LV1 and BL-LV2) conditions, implying that bleaching was likely to occur. AU from “shallow_low” reduced the duration of BL-LV1&2 conditions to 11,750 (GBR), 7,115 (SCS), and 1,25 (HWI) days. The period of BL-LV1&2 conditions for the “shallow_high” model run were 290 (GBR), 775 (SCS), and 0 (HWI) days. The values of DH-5d with “deep_low” remained zero in SCS and HWI once AU was firstly started after year 2020 hence no BL-LV1&2 days at all for those two regions, whereas duration of BL-LV1&2 for GBR was 1,325 days. Intense AU implementation within “deep_high” produced very strong cooling effects and values of DH-5d remained zero for all regions after 2021, therefore no coral bleaching events due to heat stress were expected. The model showed, that depending on the AU settings, bleaching threat is reduced as well as delayed. While the “shallow_low” scenario delayed the occurrence of BL-LV1 condition by < 1 (GBR), 12 (SCI), and 4 years (HWI) after the year 2020, the “shallow_high” scenario delayed it by 74 years (GBR), 52 years (SCS), and 4 years (HWI) (Supplementary Figure S4). Both, “deep” scenarios completely eliminated BL-LV1 condition over the modeled 80-year period, with the exception of “deep_low” at GBR (delay of 62 years) (Supplementary Figure S4). These results imply that increasing upwelling rate and depth increase the effectiveness of AU in mitigating coral bleaching caused by heat stress.
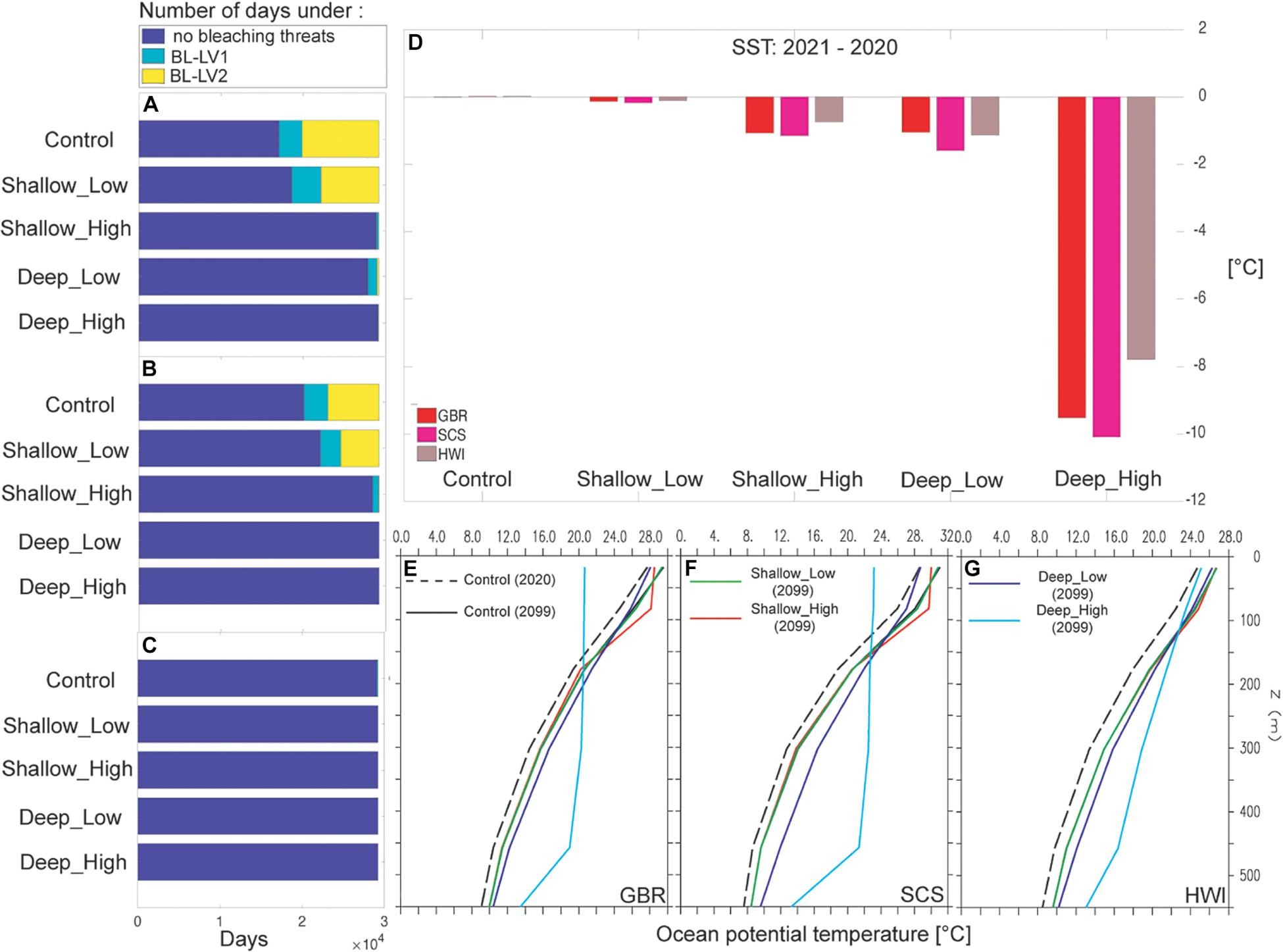
Figure 2. Results of model runs for the Great Barrier Reef (GBR), South China Sea (SCS), and Hawaii (HWI) regions: The panels show, for the end of 2099, the accumulated number of days under different coral bleaching threat levels for region GBR (A), SCS (B), and HWI (C); area-averaged difference in annual mean sea surface temperature (SST) between 2021 and 2020 (D); and area-averaged annual mean sea potential temperature for depths to 550 m (E–G).
Although AU reduced SST in all tested areas, the distinct features of these three regions, especially in their local topographies and thermoclines, led to noticeable differences in the AU-induced temperature perturbations and the changes in vertical stratification over time (Supplementary Text S2). In the three regions from 2020 to 2021, aa-SST dropped by (i) 0.13 (GBR), 0.17 (SCS), and 0.11°C (HWI) for “shallow_low” (ii) 1.07, 1.15, and 0.74°C, respectively, for “shallow_high”, (iii) 1.04, 1.59, and 1.13°C, respectively, for “deep_low”, (iv) and 9.52, 10.09, and 7.97°C, respectively, for “deep_high” (Figure 2D). SCS experienced the strongest reduction in temperature under all four AU scenarios. This is because within the UVic model, SCS was treated as a semi-enclosed region, where the water exchange with other ocean basins was lower compared to GBR and HWI (Supplementary Figure S2; Feng et al., 2016). The comparatively strong temperature gradient between the sea surface and the deep water (Δ temperature between surface and 550 m depth: 20.02°C in SCS, 17.29°C in GBR, and 15.49°C in HWI) also contributed to the strong cooling effect of AU at SCS. Furthermore, we observed that due to AU, the original warm sea surface water downwelling at and near the sites of AU implementation. Subsequently, the water temperature near the AU water source warmed leading to a gradually weakening of the vertical temperature stratification and hence reduced AU efficiency (Figures 2E–G).
The AU-induced reductions in SST within the three test regions were remarkable, and periods with 5 day-SST lower than Min-MM were seen in the model runs “deep_low” and “deep_high” (Figure 3). A particularly strong reduction in SST occurred in the “deep_high” scenario, where within the first 2 weeks after AU initiation 5 day-SST decreased to levels as low as 18°C or slightly lower (Figures 3A–C), while similar decline was much less severe (< 0.5°C) in the “shallow_low” and “shallow_high” scenarios (Figures 3A–C). The extent to which such overcooling will harm corals cannot be provided by our model. However, it has been shown that corals are susceptible to cold-water stress (Saxby et al., 2003; Hoegh-Guldberg and Fine, 2004; Howells et al., 2013), and tropical shallow water corals do not typically exist in waters below 18°C (Kleypas et al., 1999). For example in the GBR, corals which were transplanted to a region whose temperature minimum was 1.1°C cooler than the original region experienced a mortality rate of 40% (Howells et al., 2013). Therefore, we conclude that pronounced water cooling, with reduction of up to 7.97°C (Figures 3A–C) in “deep_high”, is very likely stressful for corals that are not used to such temperature drops. Therefore, such extreme AU scenarios need to be avoided. This result demonstrates that choosing an appropriate upwelling rate and depth of water source with respect to temperature, are critical for AU implementations in order to minimize the impact of overcooling.
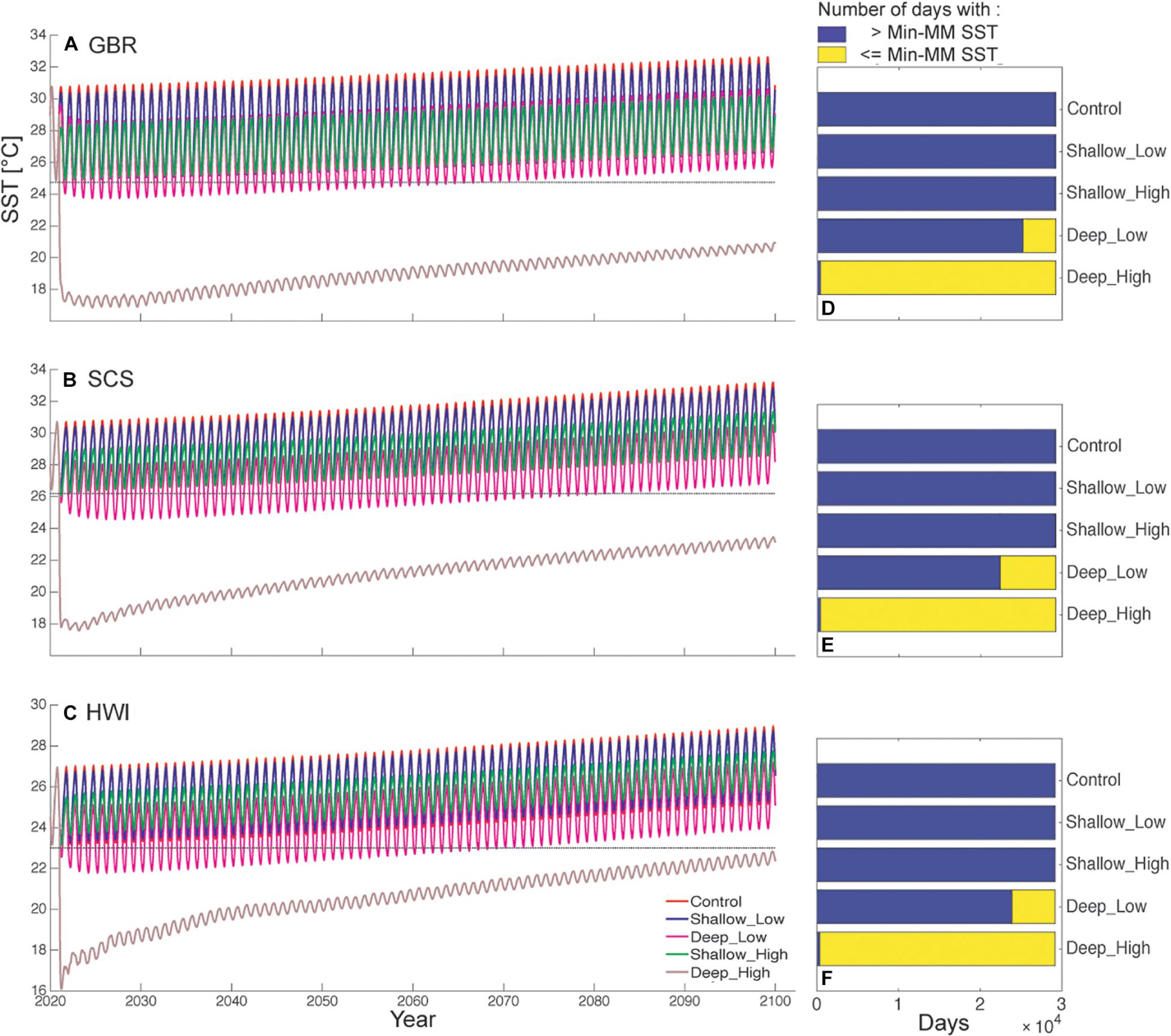
Figure 3. Results of model runs for the Great Barrier Reef (GBR), South China Sea (SCS), and Hawaii (HWI) regions: The panels show area-averaged 5-day mean time series of sea surface temperature (SST) since 2020 (A–C), and accumulated number of days with area-averaged SST below the coldest monthly mean climatology (Min-MM) by the end of 2099 (D–F). Coldest monthly mean climatology SST was highlighted with black lines for each region in panel (A–C).
There are some key limitations to the model, which need to be considered when interpreting the results of the model runs. The UVic model, like many CMIP models, is biased in representing ocean temperature. In the GBR and SCS regions, the model generated an SST offset of up to + 0.8°C in reference to in situ observations, while the offset was up to -0.5°C in HWI (Supplementary Figure S4 from Feng et al., 2016). Such representation was similar to most CMIP models and hence it was used in this study (Wang et al., 2014). However, because we used observed temperature datasets to calculate Max-MM SST as thermal thresholds, our test regions were warm (GBR and SCS) and cool-biased (HWI). Consequently, the DH-5ds heat stress levels generated by the model were slightly overestimated (GBR and SCS) or underestimated (HWI). Furthermore, in reality, massive bleaching events are often connected to large scale climate phenomena such as ENSO (El Niño-Southern Oscillation) events (Aronson et al., 2002; McGowan and Theobald, 2017). The atmospheric component of the UVic model, however, is modulated by an energy–moisture equation, and therefore it is not able to simulate dynamic wind feedbacks as well as large-scaled air-sea coupling. Consequently, we cannot capture climate phenomena such as ENSO, which can trigger marine heat waves in some regions. While the development of ENSO under future climate changes is still uncertain (Guilyardi, 2006; Wang et al., 2019), the majority of research tends to suggest an increase of its intensity and frequency in the future (Cai et al., 2015; Wang, 2018). Moreover, simulating the detailed impacts of ENSO is also challenging for the climate modeling community, especially in our selected regions (Collins et al., 2010; Cai et al., 2015). In this study, we prepared model simulations for more than 50 years to generate SST deviations that resemble marine heat waves thus capable of triggering mass bleaching. By this we can provide meaningful results that help to identify the overall potential utility of AU in mitigating coral bleaching as well as knowledge gaps and side effects that need to be addressed by future studies.
Impact of AU on Salinity, Ω-Arag, and Nitrate Concentration
The model control run predicted a change of less than 0.1 PSU in area-averaged annual mean sea surface salinity (aa-SSS) by the year 2099 (Figure 4), resulting in 34.65 (GBR), 33.55 (SCS) and 35.17 (HWI) PSU. The effect of AU on aa-SSS varied greatly among the three test regions and four model runs in response to the different halocline structures and AU settings. For all three regions in year 2020, aa-SSS at the sea surface, 130 and 550 m depth, were (i) 34.80, 35.13, and 35.04 PSU respectively for GBR, (ii) 33.75, 34.16, and 34.61 PSU for SCS, and (iii) 35.16, 35.14 and 34.43 PSU for HWI (Supplementary Figure S5). Consequently, AU implementation increased aa-SSS in GBR and SCS by up to 0.78 PSU, and decreased aa-SSS in HWI by up to 0.52 PSU over the 80-year period (Figure 4). Furthermore, in consequence of such halocline features, salinity changes were stronger under AU simulations with water from 130 m than with water from 550 m depth in GBR and HWI, while the case turned opposite in SCS (Figure 4).
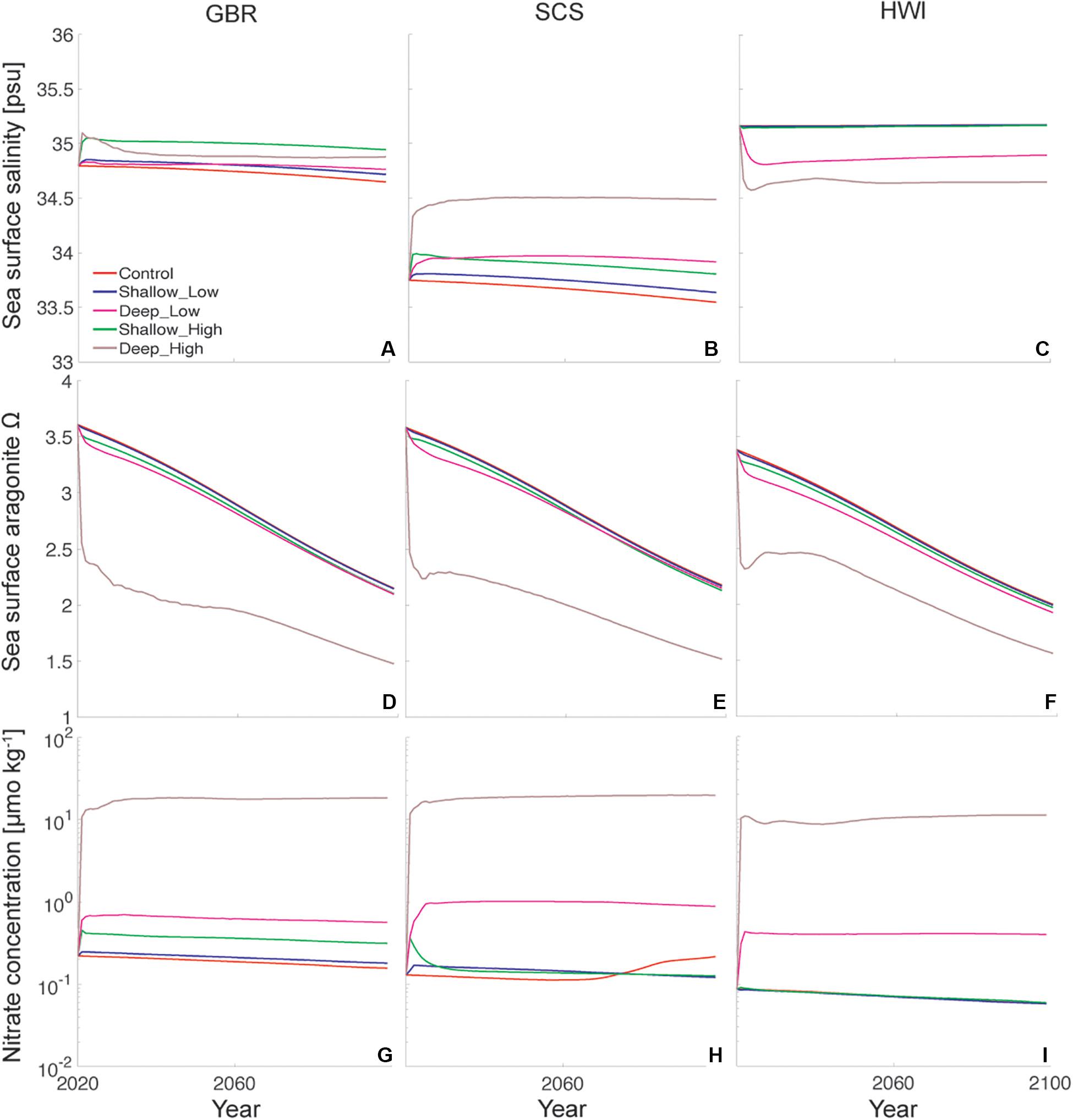
Figure 4. For the Great Barrier Reef (GBR), South China Sea (SCS), and Hawaii (HWI) regions, the time series of area-averaged annual mean sea surface salinity (A–C), aragonite saturation level (Ω-Arag, D–F), and nitrate concentration (G–I), where artificial upwelling was implemented.
The model control run for area-averaged annual mean sea surface Ω-Arag (aa-Ω) predicted a decrease by more than 1.3 units for 80 years’ course, reaching 2.15 (GBR), 2.17 (SCS), and 2 (HWI) in 2099. The implementation of AU decreased aa-Ω by < 0.2 units in the “shallow_low”, “shallow_high” and “deep_low” runs, and by > 0.5 units in the “deep_high” run relative to the control in all three regions in 2099 (Figure 4). Namely, the aa-(s in year 2099 for runs “shallow_low”, “shallow_high”, “deep_low”, and “deep_high” were: 2.15, 2.10, 2.10, and 1.48, respectively, in GBR; 2.17, 2.13, 2.15, and 1.52, respectively, in SCS; and 2.00, 1.97, 1.93, and 1.56, respectively, in HWI. Water from the deeper ocean layer in our test regions had lower (-Arag than the surface water (Supplementary Figure S5), which explains why the surface (-Arag decreased near AU sites.
The model control run for area-averaged annual mean sea surface nitrate concentration (aa-N) decreased by < 0.1 μmol/l in 80 years, reaching 0.157 (GBR), 0.218 (SCS), and 0.058 μmol/l (HWI) in 2099. Due to a higher nitrate concentration in the deep compared to the shallow, the AU model runs “shallow_low”, “shallow_high”, “deep_low”, and “deep_high” increased aa-N to 0.182, 0.315, 0.563, and 18.4 μmol/l, respectively, in GBR; 0.122, 0.127, 0.892, and 19.9 μmol/l, respectively, in SCS; and 0.058, 0.059, 0.398, and 11.2 μmol/l, respectively, in HWI (Figure 4). The magnitudes of the perturbations in those three variables from “shallow_low,” “shallow_high,” and “deep_low” were well within the seasonal variability for local inorganic nitrate. The “deep_high” run, however, increased surface nutrient concentration by two orders of magnitude, which was far beyond natural nutrient fluctuations (Supplementary Figures S6–S8).
Corals, along with other marine biota, can be sensitive to changes in oceanic salinity, Ω-Arag and nutrient levels, and AU could impose harmful impacts on ecosystems if changes of these parameters are too strong. With respect to concomitant occurrences of salinity changes and temperature increase as shown by our result e.g., from “deep_high,” the threat of thermal stress likely surpasses the stress imposed by salinity anomalies in corals (Buddemeier and Fautin, 1993; Reynaud et al., 2004; Chavanich et al., 2009). AU-induced changes in salinity are relatively small (<1PSU) and may therefore pose a minimal risk for corals (Hoegh-Guldberg and Smith, 1989; Humphrey et al., 2008). However, these changes in salinity may have a stronger effect on water density distribution and hence flow patterns. Along with thermal stress, ocean acidification (i.e., reductions in Ω-Arag), driven by progressing climate change, is considered a major global challenge to coral reefs and other marine ecosystems (Orr et al., 2005). This is because reductions in Ω-Arag suppresses calcification in corals and other marine calcifiers and thereby weaken their skeleton or other stabilizing structures (Gattuso et al., 1998; Ricke et al., 2013; Wittmann and Poertner, 2013). The decline in Ω-Arag of up to 1.3 units over the next 80 years (as demonstrated in the model control run) could transgress the Ω-Arag safe level of 2.82 for corals (Guan et al., 2015) and is therefore a major concern for the persistence of coral reefs (Orr et al., 2005; Hoegh-Guldberg et al., 2007). The further declines of Ω-Arag caused in particular by rather extreme AU scenarios (e.g., “deep_high” scenario) will intensify ocean acidification thus likely making environments inhabitable to corals (Kleypas et al., 1999). Moderate increases in inorganic nutrient supply (e.g., nitrate) may potentially lower the negative effects of ocean acidification, by increasing phytoplankton growth and hence the availability of energy (through feeding) for increased investment into calcification (Koop et al., 2001; Bongiorni et al., 2003). Increased heterotrophy in corals through increased food supply has previously been hypothesized to be the reason for increasing coral calcification despite decreasing Ω-Arag in Bermuda over the past two decades (Bates, 2017). Increased inorganic nutrient supply in general is known to either be neutral or beneficial for corals, since they can promote zooxanthellae growth and hence primary production and energy supply to corals (Fabricius, 2005). Negative effects, however, may still occur under extreme nutrient enrichment (e.g., “deep_high” AU scenario), where nitrate concentrations are well above the suggested upper threshold for coral reefs of 4.51 μmol/l (Guan et al., 2015). Negative effects on organism (coral) level can include (i) a loss of control of the coral host over the zooxanthellae population (Falkowski et al., 1993), and (ii) increased susceptibility to coral diseases and bleaching (Vega Thurber et al., 2013) in particular, if nutrient supply is unbalanced (N:P ratio; Wiedenmann et al., 2012). Negative effects on ecosystem level include, for example, (i) hypoxic conditions during mass die-off of algal biomass (Gray et al., 2002; Keller et al., 2014), and (ii) shifts in benthic community structures toward algae dominated ecosystems in cases of low herbivore abundance (e.g., due to overfishing,; McCook, 1999; Szmant, 2002). Therefore, we conclude that AU implementation causing a moderate inorganic nutrient enrichment of surface waters, represented by cases of “shallow_low,” “shallow_high,” and “deep_low” (below 4.51 μmol/l) might be tolerable or even beneficial for coral reefs, while AU implementations causing strong enrichment in coral reefs and beyond shown by “deep_high” is likely damaging and should therefore be avoided.
Implications for Real AU Practices
Our modeling results provide valuable information on the potential benefits and risks of AU on coral reefs and beyond. It shows that SST and thermal stress can be effectively reduced by AU, which, based on the reduction of DHW, either fully prevented heat-induced coral bleaching until the year 2099 (“shallow_high,” “deep_low,” and “deep_high” modes), or delayed the occurrence of bleaching and reduced its severity at a low upwelling intensity (“shallow_low”). In the “deep_high” run, AU induced perturbations that profoundly reshaped the hydrology and biogeochemistry, and triggered changes that were not seen in the preceding decades. Therefore, the AU settings in this model run are not practical. In terms of lowering coral bleaching threat, the “shallow_high” and “deep_low” runs produced similar results as the “deep_high” run, but with reduced adverse environmental impacts caused by temperature drops, salinity changes, Ω-Arag reductions and nutrient enrichment. These results imply that low to moderate upwelling rates and rather shallow upwelling depths are more practical to reduce undesirable effects while maintaining the efficiency of bleaching mitigation. Although “shallow_low” did not completely prevent bleaching for the entire duration of 80 years, it could effectively prevent BL_LV1 conditions before the year 2045 in SCS (Supplementary Figure S4). The delay in bleaching conditions caused by “shallow_low” run would thereby at least provide temporal refugia in which corals may adjust to increasing SST or in which other adaption technologies are developed. With respect to the temporal scale, the modeling results suggest that AU intensity should be adjusted over time following the predicted increase in SST (Meinshausen et al., 2011) and a predicted increase in the frequency of ENSO events entailing heat waves (Cai et al., 2014). Thus, AU intensity would need to be increased over time either by increasing the depth or the volume of upwelled water. At the same time, coral bleaching events usually occur in only abnormally warm summers, for several days to weeks. Therefore, AU may only be necessary for a given period of time when thermal threats occur. The continuous operation of AU runs as applied in our study, provides unnecessary upwelling throughout a large portion of the year, which most likely substantially exacerbating the overall negative side effects. Concerning the complexities in temporal patterns of the SST and heat stress under climate change, the ideal AU operation needs to be firmly guided by timely monitored and forecasted temperature profiles at targeted areas.
Although not tested in this study due to limitations of the Earth system model, another possible pathway to reduce the strong environmental impact seen in the “deep_high” run would be to reduce the geographical density of AU or to limit AU to locations close to the reef only. Indeed, the uniformly deployed arrays of AU water translocation pathways in the model covered large areas that were not inhabited by corals (Supplementary Figure S2). Such an approach will be difficult and undesirable to apply in practice, because of space constraints over the ocean and the enormous engineering costs (Matsuda et al., 1999; Kirke, 2003). At GBR, for example, coral reefs occurred only in less than 10% of the deployment area, while the excess of AU devices certainly contributed to the unintended environmental perturbations locally and elsewhere. It is more likely that the availability of the AU hardware, technology, and funds will allow very localized AU implementations, which will protect only a small fraction of reefs. Thus, if the AU pipes are deployed only at and near the reef sites, we might expect much lower overall environmental changes over the whole area even under intense upwelling scenario (“deep_high”).
The choice of the AU settings is not only a question of ecological benefits and risks, but also what is feasible from an engineering point of view in installation and operation. Advanced AU devices with stronger turbine, higher working efficiency and adiabatic pipelines could pump enough cold water to successfully prevent coral bleaching over larger areas, but they are very costly (Matsuda et al., 1999). Upwelling seawater from a depth beyond our proposed AU devices (from 130 m or 550 m Liu and Jin, 1995; Kirke, 2003;, see Supplementary Text S3 for technical details) is theoretically possible, however extending the upwelling pipe to deeper levels, will make the construction and maintenance of the devices more difficult (Kirke, 2003). In addition, many reef flats can extend to hundred kilometers horizontally without evidently stratified vertical sea temperature. In this situation, the installation of pipelines that connects an outlying deep water source, with coral living habitat might be the most expensive investment. When it comes to pumping rates we also need to consider energy demands and balance AU settings with its bleaching mitigation potential. In our rough estimation (Figure 5), increasing the AU vertical depth increases the energy demand to pump 1 m3 bottom water to sea surface almost linearly. However, increasing AU rates can increase the energy demand to upwell the same amount of water to the surface at a much lower rate (Figure 5). Therefore, for AU settings with similar cooling effects as demonstrated for “shallow_high” and “deep_low” runs, the “shallow_high” should be preferred for energy-saving causes (see Supplementary Text S4 for detailed calculations). Overall, the bleaching mitigation effectiveness and the costs of AU devices are both strongly determined by local circumstances and need careful balancing of benefits, risks and trade-offs.
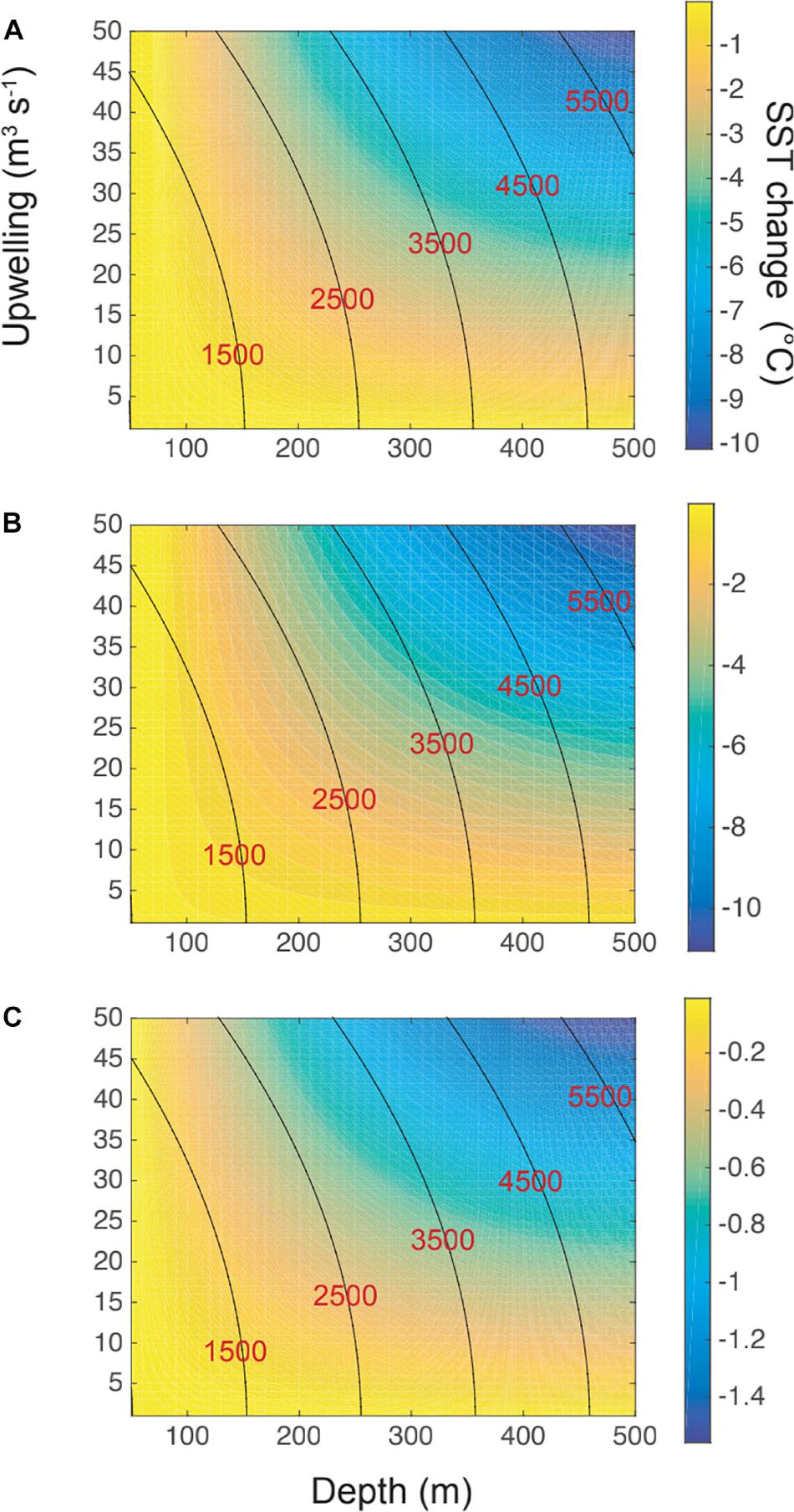
Figure 5. The quantitative analysis for required AU input by investigating the surface cooling effects after the first-year’s AU implementation. Area-mean SST differences between AU runs and control run are plotted against AU upwelling rate and AU depth for regions GBR (A), SCS (B), and HWI (C). To pump 1 m3 bottom water to sea surface adiabatically, required energy (unit: kJ) is plotted as isoclines over the SST fields.
Conclusion
Monitored heat stress and predicted future global warming have prompted the search for ways to protect coral reefs from severe bleaching. In this context, we have assessed the environmental impacts of virtually implemented water translocation, as an analogous process to AU, over large areas that included coral reefs with an Earth System modeling approach. We used constant upwelling rates over 80 years for 500 km2 regions. Although these scenarios are beyond of what would be temporally and spatially feasible and desirable in situ, the model results provide valuable information about the effect of AU on the perturbations of ocean heat and biogeochemistry. The results clearly show that AU has the potential to effectively reduce SST and DHW, and delay the occurrence of future coral bleaching events. However, the potential side effects of AU, such as surface overcooling, salinity fluctuations, surface acidification, and nutrient enrichment, can cause severe risks to corals, in particular, if water is upwelled from deep layer (e.g., 550 m) at high rates. Though it was not discussed in detail, model simulations also revealed changes of ocean circulation under the deployment of more severe AU scenarios, which can cause unexpected perturbations in ocean heat budget and biogeochemistry (Supplementary Text S5). In summary, our model evaluation indicates that even rather low upwelling rates of water derived from rather shallow depth (here 130 m) can be an effective measure to prevent coral bleaching during thermal stress, while at the same time minimizing undesirable environmental side effects.
In order to explore this topic further and to validate and refine our model results, future studies require regional models with a higher spatial and temporal resolution as well as experimental work. Although the development of regional models that allow simulations of AU scenarios is challenging, they are necessary to provide region-specific details about the biogeochemical and hydrographic consequences of AU, which may be strongly influenced by the local bathymetry and hydrographic conditions. In addition, experimental studies that investigate the effectiveness of different AU scenarios on different coral species in different regions to guide the selection of AU sites and operating strategies, are necessary. In this regard, performing a medium to long-term experiment with focus on analyzing heat-stressed corals’ physiological response to cool subthermocline water analogous to AU, can be a meaningful attempt. This can provide important insights for how AU can be operated to provide stress relief while reducing side effects (Sawall et al., 2020), and suggest further refined set-ups for modeling studies. Current technology would theoretically allow successful installation and operation of AU devices locally in shallow coral reef environment. The biggest technological challenge, however, is likely the accessibility of cool water. With oceanic deep water being distant to many shallow water corals, a possible solution to such natural constraint could be exploiting underground cool brine water from aquifer layers. Its effectiveness and potential risks, however, remain to be investigated. If climate change follows a business-as-usual scenario in the future and AU technology proves to be useful to mitigate heating stress, AU can probably buy corals some time, required that adequate funds, human power, infrastructure, and space are available. Although in the future the obstacles to the implementation of AU and its environmental side effects may be overcome with the development of new AU hardware and wisely planned preparations, it is still necessary to limit climate change to well below the 1.5°C warming, since human interventions will not be able to save coral reefs on a global scale.
Data Availability Statement
Datasets (model simulations and computer codes to generate figures/tables) for this research are available in GEOMAR repository (https://ftp3.geomar.de/users/yfeng/CoralAU/).
Author Contributions
EF initiated the idea of the study, conducted the study, performed data analysis, and wrote the manuscript. YS, MW, ML, and YF contributed conception and design of the study. All authors contributed to manuscript revision, read and approved the submitted version.
Funding
This project was funded by the Cluster of Excellence “The Future Ocean” (CP1780), funded within the framework of the Excellence Initiative by the Deutsche Forschungsgemeinschaft (DFG, German Research Foundation).
Conflict of Interest
The authors declare that the research was conducted in the absence of any commercial or financial relationships that could be construed as a potential conflict of interest.
Supplementary Material
The Supplementary Material for this article can be found online at: https://www.frontiersin.org/articles/10.3389/fmars.2020.556192/full#supplementary-material
References
Aronson, R. B., Precht, W. F., Toscano, M. A., and Koltes, K. H. (2002). The 1998 bleaching event and its aftermath on a coral reef in Belize. Mar. Biol. 141, 435–447. doi: 10.1007/s00227-002-0842-5
Bates, N. R. (2017). Twenty years of marine carbon cycle observations at devils hole bermuda provide insights into seasonal hypoxia, coral reef calcification, and ocean acidification. Front. Mar. Sci. 4:36. doi: 10.3389/fmars.2017.00036
Bayraktarov, E., Pizarro, V., Eidens, C., Wilke, T., and Wild, C. (2013). Bleaching susceptibility and recovery of colombian caribbean corals in response to water current exposure and seasonal upwelling. PLoS One 8:e80536. doi: 10.1371/journal.pone.0080536
Bitz, C. M., and Liscomb, W. H. (1999). An energy-conserving thermodynamic model of sea ice. J. Geophys. Res. 104:15669. doi: 10.1029/1999JC900100
Bonan, G. B., and Doney, S. C. (2018). Climate, ecosystems, and planetary futures: the challenge to predict life in Earth system models. Science 359:eaam8328. doi: 10.1126/science.aam8328
Bongiorni, L., Shafir, S., Angel, D., and Rinkevich, B. (2003). Survival, growth and gonad development of two hermatypic corals subjected to in situ fish-farm nutrient enrichment. Mar. Ecol. Prog. Ser. 253, 137–144. doi: 10.3354/meps253137
Buddemeier, R. W., and Fautin, D. G. (1993). Coral bleaching as an adaptive mechanism. Bioscience 43, 320–326. doi: 10.2307/1312064
Cai, W., Borlace, S., Lengaigne, M., van Rensch, P., Collins, M., Vecchi, G., et al. (2014). Increasing frequency of extreme El Niño events due to greenhouse warming. Nat. Clim. Change 4:111. doi: 10.1038/nclimate2100
Cai, W., Santoso, A., Wang, G., Yeh, S.-W., An, S.-I., Cobb, K. M., et al. (2015). ENSO and greenhouse warming. Nat. Clim. Change 5:849. doi: 10.1038/nclimate2743
Chavanich, S., Viyakarn, V., Loyjiw, T., Pattaratamrong, P., and Chankong, A. (2009). Mass bleaching of soft coral, Sarcophyton spp. in Thailand and the role of temperature and salinity stress. ICES J. Mar. Sci. 66, 1515–1519. doi: 10.1093/icesjms/fsp048
Chollett, I., and Mumby, P. J. (2013). Reefs of last resort: Locating and assessing thermal refugia in the wider Caribbean. Biol. Conserv. 167, 179–186. doi: 10.1016/j.biocon.2013.08.010
Collins, M., An, S.-I., Cai, W., Ganachaud, A., Guilyardi, E., Jin, F.-F., et al. (2010). The impact of global warming on the tropical Pacific Ocean and El Niño. Nat. Geosci. 3:391. doi: 10.1038/ngeo868
Deloitte Access Economics (2013). Economic Contribution of the Great Barrier Reef. Townsville, QL: Deloitte Access Economics.
Fabricius, K. E. (2005). Effects of terrestrial runoff on the ecology of corals and coral reefs: review and synthesis. Mar. Pollut. Bull. 50, 125–146. doi: 10.1016/J.MARPOLBUL.2004.11.028
Falkowski, P. G., Dubinsky, Z., Muscatine, L., and McCloskey, L. (1993). Population control of symbiotic corals. Bioscience 43, 601–611.
Fanning, A. F., and Weaver, A. J. (1996). An atmospheric energy-moisture balance model: Climatology, interpentadal climate change, and coupling to an ocean general circulation model. J. Geophys. Res. 101:15111. doi: 10.1029/96JD01017
Feng, E. Y., Keller, D. P., Koeve, W., and Oschlies, A. (2016). Could artificial ocean alkalinization protect tropical coral ecosystems from ocean acidification? Environ. Res. Lett. 11:074008. doi: 10.1088/1748-9326/11/7/074008
Frölicher, T. L., and Laufkötter, C. (2018). Emerging risks from marine heat waves. Nat. Commun. 9:650. doi: 10.1038/s41467-018-03163-6
Gattuso, J.-P., Frankignoulle, M., Bourge, I., Romaine, S., and Buddemeier, R. W. (1998). Effect of calcium carbonate saturation of seawater on coral calcification. Glob. Planet. Change 18, 37–46. doi: 10.1016/s0921-8181(98)00035-6
Gray, S. J., Wu, R., and Or, Y. Y. (2002). Effects of hypoxia and organic enrichment on the coastal marine environment. Mar. Ecol. Prog. Ser. 238, 249–279. doi: 10.3354/meps238249
Guan, Y., Hohn, S., and Merico, A. (2015). Suitable environmental ranges for potential Coral reef habitats in the tropical ocean. PLoS One 10:e0128831. doi: 10.1371/journal.pone.0128831
Guilyardi, E. (2006). El Niño–mean state–seasonal cycle interactions in a multi-model ensemble. Clim. Dyn. 26, 329–348. doi: 10.1007/s00382-005-0084-6
Hoegh-Guldberg, O., and Fine, M. (2004). Low temperatures cause coral bleaching. Coral Reefs 23:444. doi: 10.1007/s00338-004-0401-2
Hoegh-Guldberg, O., Mumby, P. J., Hooten, A. J., Steneck, R. S., Greenfield, P., Gomez, E., et al. (2007). Coral reefs under rapid climate change and ocean acidification. Science 318, 1737–1742. doi: 10.1126/science.1152509
Hoegh-Guldberg, O., and Smith, G. J. (1989). The effect of sudden changes in temperature, light and salinity on the population density and export of zooxanthellae from the reef corals Stylophora pistillata Esper and Seriatopora hystrix Dana. J. Exp. Mar. Biol. Ecol. 129, 279–303. doi: 10.1016/0022-0981(89)90109-3
Howells, E. M. J., Berkelmans, R., Oppen, M. J. H., van Willis, B. L., and Bay, L. K. (2013). Historical thermal regimes define limits to coral acclimatization. Ecology 94, 1078–1088. doi: 10.1890/12-1257.1
Hughes, T. P., Anderson, K. D., Connolly, S. R., Heron, S. F., Kerry, J. T., Lough, J. M., et al. (2018). Spatial and temporal patterns of mass bleaching of corals in the Anthropocene. Science 359, 80–83. doi: 10.1126/science.aan8048
Hughes, T. P., Kerry, J. T., Álvarez-noriega, M., Álvarez-romero, J. G., Anderson, K. D., Baird, A. H., et al. (2017). Global warming and recurrent mass bleaching of corals. Nature 543, 373–377. doi: 10.1038/nature21707
Humphrey, C., Weber, M., Lott, C., Cooper, T., and Fabricius, K. (2008). Effects of suspended sediments, dissolved inorganic nutrients and salinity on fertilisation and embryo development in the coral Acropora millepora (Ehrenberg, 1834). Coral Reefs 27, 837–850. doi: 10.1007/s00338-008-0408-1
Jiménez, C., Cortés, J., León, A., and Ruíz, E. (2001). Coral bleaching and mortality associated with the 1997-98 El Nino in an upwelling envrionment in the eastern Pacific (Gulf of Papagayo. Coasta Rica). Bull. Mar. Sci. 69, 151–169.
Jokiel, P. L., and Brown, E. K. (2004). Global warming, regional trends and inshore environmental conditions influence coral bleaching in Hawaii. Glob. Change Biol. 10, 1627–1641. doi: 10.1111/j.1365-2486.2004.00836.x
Keller, D. P., Feng, E. Y., and Oschlies, A. (2014). Potential climate engineering effectiveness and side effects during a high carbon dioxide-emission scenario. Nat. Commun. 5, 1–11. doi: 10.1038/ncomms4304
Kemp, D. W., Oakley, C. A., Thornhill, D. J., and Laura, A. (2011). Catastrophic mortality on inshore coral reefs of the Florida Keys due to severe low-temperature stress. Glob. Change Biol. 17, 3468–3477. doi: 10.1111/j.1365-2486.2011.02487.x
Kirke, B. (2003). Enhancing fish stocks with wave-powered artificial upwelling. Ocean Coast. Manag. 46, 901–915. doi: 10.1016/S0964-5691(03)00067-X
Kleypas, A. J. (1997). Modeled estimates of global reef habitat and carbonate production since the last glacial maximum. Paleoceanography 12, 533–545. doi: 10.1029/97pa01134
Kleypas, J. A., MacManus, J. W., and Menez, L. A. B. (1999). Environmental limits to coral reef development: where do we draw. Ameri. Zool. 39, 146–159. doi: 10.1093/icb/39.1.146
Koop, K., Booth, D., Broadbent, A., Brodie, J., Bucher, D., Capone, D., et al. (2001). ENCORE: The effect of nutrient enrichment on coral eeefs: synthesis of results and conclusions. Mar. Pollut. Bull. 42, 91–120. doi: 10.1016/S0025-326X(00)00181-8
Landschützer, P., Gruber, N., Bakker, D. C. E., and Schuster, U. (2014). Recent variability of the global ocean carbon sink. Glob. Biogeochem. Cycles 28, 927–949. doi: 10.1002/2014GB004853
Lessin, G., Artioli, Y., Almroth-rosell, E., Blackford, J. C., Queirós, A. M., Rabouille, C., et al. (2018). Modelling marine sediment biogeochemistry: current knowledge gaps, challenges, and some methodological advice for advancement. Front. Mar. Sci. 5:19. doi: 10.3389/fmars.2018.00019
Li, S., Yu, K. F., Chen, T. R., Shi, Q., and Zhang, H. L. (2011). Assessment of coral bleaching using symbiotic zooxanthellae density and satellite remote sensing data in the Nansha Islands, South China Sea. Chin. Sci. Bull. 56, 1031–1037. doi: 10.1007/s11434-011-4390-6
Lirman, D., and Manzello, D. (2009). Patterns of resistance and resilience of the stress-tolerant coral Siderastrea radians (Pallas) to sub-optimal salinity and sediment burial. J. Exp. Mar. Biol. Ecol. 369, 72–77. doi: 10.1016/j.jembe.2008.10.024
Lirman, D., Schopmeyer, S., Manzello, D., Gramer, L. J., Precht, W. F., Muller-Karger, F., et al. (2011). Severe 2010 cold-water event caused unprecedented mortality to corals of the Florida reef tract and reversed previous survivorship patterns. PLoS One 6:e23047. doi: 10.1371/journal.pone.0023047
Liu, C., and Jin, Q. (1995). Artificial upwelling in regular and random waves. Ocean Eng. 22, 337–350. doi: 10.1016/0029-8018(94)00019-4
Liu, G., Strong, A. E., Skirving, W. J., and Arzayus, L. F. (2006). “Overview of NOAA Coral Reef Watch program’s near-real-time satellite global coral bleaching monitoring activities,” in Proceedings of the 10th International Coral Reef Symposium, Okinawa, 1783–1793.
Locarnini, R. A., Mishonov, A. V., Antonov, J. I., Boyer, T. P., Garcia, H. E., Baranova, O. K., et al. (2013). “NOAA Atlas NESDIS 62 World Ocean Atlas 2013,” in Temperature, Vol. 1, ed. S. Levitus (Silver Spring: NOAA Atlas NESDIS), doi: 10.1182/blood-2011-06-357442
Marshall, P. A., and Baird, A. H. (2000). Bleaching of corals on the Great Barrier Reef: differential susceptibilities among taxa. Coral Reefs 19, 155–163. doi: 10.1007/s003380000086
Matsuda, F., Szyper, J., Takahashi, P., and Vadus, J. (1999). The ultimate ocean ranch. Sea Technol. 40, 17–26.
McCook, L. J. (1999). Macroalgae, nutrients and phase shifts on coral reefs: scientific issues and management consequences for the Great Barrier Reef. Coral Reefs 18, 357–367. doi: 10.1007/s003380050213
McGowan, H., and Theobald, A. (2017). ENSO weather and coral bleaching on the great barrier reef. Australia. Geophys. Res. Lett. 10:607. doi: 10.1002/2017GL074877
Meinshausen, M., Smith, S. J., Calvin, K., Daniel, J. S., Kainuma, M. L. T., Lamarque, J.-F., et al. (2011). The RCP greenhouse gas concentrations and their extensions from 1765 to 2300. Clim. Change 109, 213–241. doi: 10.1007/s10584-011-0156-z
Meissner, K. J., Weaver, A. J., Matthews, H. D., and Cox, P. M. (2003). The role of land surface dynamics in glacial inception: a study with the UVic earth system model. Clim. Dyn. 21, 515–537. doi: 10.1007/s00382-003-0352-2
Mongin, M., and Baird, M. (2014). The interacting effects of photosynthesis, calcification and water circulation on carbon chemistry variability on a coral reef flat: a modelling study. Ecol. Model. 284, 19–34. doi: 10.1016/j.ecolmodel.2014.04.004
Ncrw. (2000). NOAA Coral Reef Watch Operational 50-km Satellite Coral Bleaching Degree Heating Weeks Product. Silver Spring, ML: NCRW.
Orr, J. C., Fabry, V. J., Aumont, O., Bopp, L., Doney, S. C., Feely, R. A., et al. (2005). Anthropogenic ocean acidification over the twenty-first century and its impact on calcifying organisms. Nature 437, 681–686. doi: 10.1038/nature04095
Oschlies, A., Pahlow, M., Yool, A., and Matear, R. J. (2010). Climate engineering by artificial ocean upwelling: Channelling the sorcerer’s apprentice. Geophys. Res. Lett. 37:L04701. doi: 10.1029/2009GL041961
Pacanowski, R. C. (1996). MOM2: Documentation, user’s guide and reference manual. GFDL Ocean Tech. Rep. 2:329.
Pan, Y., Li, Y., Fan, W., Zhang, D., Qiang, Y., Jiang, Z., et al. (2019). A sea trial of air-lift consept artificial upwelling in east China sea. J. Atmos. Oceanic Technol. 36, 2191–2204. doi: 10.1175/jtech-d-18-0238.1
Pat Grandelli, P. E., Rochelleau, G., Hamrick, J., Church, M., and Powell, B. (2012). Modeling the Physical and Biochemical Influence of Ocean Thermal Energy Conversion Plant Discharges Into Their Adjacent Waters. Technical Report Po Box 1206. Kailua: U.S. Department of Energy.
Petoukhov, V., Claussen, M., Berger, A., Crucifix, M., Eby, M., Eliseev, A. V., et al. (2005). EMIC intercomparison project (EMIP-CO2): comparative analysis of EMIC simulations of climate and of equilibrium and transient responses to atmospheric CO2 doubling. Clim. Dyn. 25, 363–385. doi: 10.1007/s00382-005-0042-3
Raftery, A. E., Zimmer, A., Frierson, D. M. W., Startz, R., and Liu, P. (2017). Less than 2 °C warming by 2100 unlikely. Nat. Clim. Change 7, 637–641. doi: 10.1038/NCLIMATE3352
Reynaud, S., Hemming, N. G., Juillet-Leclerc, A., and Gattuso, J.-P. (2004). Effect of pCO2 and temperature on the boron isotopic composition of the zooxanthellate coral Acropora sp. Coral Reefs 23, 539–546. doi: 10.1007/s00338-004-0399-5
Ricke, K. L., Orr, J. C., Schneider, K., and Caldeira, K. (2013). Risks to coral reefs from ocean carbonate chemistry changes in recent earth system model projections. Environ. Res. Lett. 8:034003. doi: 10.1088/1748-9326/8/3/034003
Roth, M. S., Goericke, R., and Deheyn, D. D. (2012). Cold induces acute stress but heat is ultimately more deleterious for the reef-building coral Acropora yongei. Sci. Rep. 2:240. doi: 10.1038/srep00240
Sawall, Y., Harris, M., Lebrato, M., Wall, M., and Feng, E. Y. (2020). Discrete pulses of cooler deep water can decelerate coral bleaching during thermal stress: implications for artificial upwelling during heat stress events. Front. Mar. Sci. 7:720. doi: 10.3389/fmars.2020.00720
Saxby, T., Dennison, W. C., and Hoegh-guldberg, O. (2003). Photosynthetic responses of the coral Montipora digitata to cold temperature stress. Mar. Ecol.Prog. Ser. 248, 85–97. doi: 10.3354/meps248085
Schneider, K., and Erez, J. (2006). Seawater carbonate chemistry and processes during experiments with coral Acropora eurystoma, 2006. Limnol. Oceanogr. 51, 1284–1293. doi: 10.1594/PANGAEA.726914
Szmant, A. M. (2002). Nutrient enrichment on coral reefs: Is it a major cause of coral reef decline? Estuaries 25, 743–766. doi: 10.1007/BF02804903
Trench, M., The, I., Pacific, N., Thermal, O., and Conversion, E. (2004). Artificial upwelling of deep seawater using the perpetual salt fountain for cultivation of ocean desert. J. Oceanogr. 60, 563–568. doi: 10.1023/b:joce.0000038349.56399.09
Vega Thurber, R., Burkepile, D., Fuchs, C., Shantz, A., McMinds, R., and Zaneveld, J. (2013). Chronic nutrient enrichment causes increased coral disease prevalence and severity. Glob. Change Biol. 20, 544–554. doi: 10.1111/gcb.12450
Viúdez, Á, Fernández-Pedrera Balsells, M., and Rodríguez-Marroyo, R. (2016). Artificial upwelling using offshore wind energy for mariculture applications. Sci. Mar. 80, 235–248. doi: 10.3989/scimar.04297.06b
Waheed, Z., Benzoni, F., van der Meij, S. E. T., Terraneo, T. I., and Hoeksema, B. W. (2015). Scleractinian corals (Fungiidae, Agariciidae and Euphylliidae) of Pulau Layang-Layang, Spratly Islands, with a note on Pavona maldivensis. Zookeys 517, 1–37.
Wall, M., Putchim, L., Schmidt, G. M., Jantzen, C., Khokiattiwong, S., and Richter, C. (2015). Large-amplitude internal waves benefit corals during thermal stress. Proc. R. Soc. B 282:20140650. doi: 10.1098/rspb.2014.0650
Wang, C., Zhang, L., Lee, S., Wu, L., and Mechoso, C. R. (2014). A global perspective on CMIP5 climate model biases. Nat. Clim. Change 4, 201–205. doi: 10.1038/NCLIMATE2118
Wang, Y., Luo, Y., Lu, J., and Liu, F. (2019). Changes in ENSO amplitude under climate warming and cooling. Clim. Dyn. 52, 1871–1882. doi: 10.1007/s00382-018-4224-1
Weaver, A. J., Eby, M., Wiebe, E. C., Bitz, C. M., Duffy, P. B., Ewen, T. L., et al. (2001). The UVic earth system climate model: model description, climatology, and applications to past, present and future climates. Atmos. Ocean 39, 361–428. doi: 10.1080/07055900.2001.9649686
White, A., Björkman, K., Grabowski, E., Letelier, R., Poulos, S., Watkins, B., et al. (2010). An open ocean trial of controlled upwelling using wave pump technology. J. Atmos. Ocean. Technol. 27, 385–396. doi: 10.1175/2009JTECHO679.1
Wiedenmann, J., D’Angelo, C., Smith, E. G., Hunt, A. N., Legiret, F.-E., Postle, A. D., et al. (2012). Nutrient enrichment can increase the susceptibility of reef corals to bleaching. Nat. Clim. Change 3:160. doi: 10.1038/nclimate1661
Wittmann, A. C., and Poertner, H.-O. (2013). Sensitivities of extant animal taxa to ocean acidification. Nat. Clim. Change 3, 995–1001. doi: 10.1038/nclimate1982
Keywords: coral bleaching, ocean biogeochemical modeling, artificial upwelling, global warming, sea surface temperature rise, heat waves, earth system modeling
Citation: Feng EY, Sawall Y, Wall M, Lebrato M and Fu Y (2020) Modeling Coral Bleaching Mitigation Potential of Water Vertical Translocation – An Analogue to Geoengineered Artificial Upwelling. Front. Mar. Sci. 7:556192. doi: 10.3389/fmars.2020.556192
Received: 27 April 2020; Accepted: 04 September 2020;
Published: 26 November 2020.
Edited by:
Anastazia T. Banaszak, National Autonomous University of Mexico, MexicoReviewed by:
Mark Edward Baird, Commonwealth Scientific and Industrial Research Organisation (CSIRO), AustraliaValeria Pizarro, ECOMARES, Colombia
Copyright © 2020 Feng, Sawall, Wall, Lebrato and Fu. This is an open-access article distributed under the terms of the Creative Commons Attribution License (CC BY). The use, distribution or reproduction in other forums is permitted, provided the original author(s) and the copyright owner(s) are credited and that the original publication in this journal is cited, in accordance with accepted academic practice. No use, distribution or reproduction is permitted which does not comply with these terms.
*Correspondence: Ellias Y. Feng, ZmZweWNtQGhvdG1haWwuY29t; eWZlbmdAZ2VvbWFyLmRl