- 1Academy of Natural Sciences of Drexel University, Philadelphia, PA, United States
- 2Department of Geological Sciences, University of South Carolina, Colombia, SC, United States
Pteropods have been hailed as the “canary in the coal mine” for ocean acidification, however, questions remain about their life history, habitat, and the environmental parameters that the isotopic composition of their shells reflect. In order to use pteropods as recorders of ocean chemistry, it is first necessary to understand where they calcify and how this may change through the year, whether this signal is affected by dissolution, and if shells are retained in the subfossil, and eventually fossil, record. Here we create the first annual record of the stable isotopic composition of shells of the pteropod Heliconoides inflatus in the Cariaco Basin, Venezuela utilizing samples and data from the CARIACO time series. Sixty-four H. inflatus specimens from 17 sediment trap samples between November 1996 and April 1998, and 22 specimens from the late Holocene-aged CAR2000-MC-2 core were analyzed for shell condition (an assessment of the amount of dissolution that a shell has experienced), size, and carbon and oxygen isotopic composition. Carbon isotopic measurements of juveniles (< 1mm) were more variable than those in adults (>1 mm), suggesting juvenile pteropods likely have a higher growth rate, and therefore different metabolic vital effects, and a more varied diet than adult pteropods. H. inflatus was found to have an apparent calcification depth of 51.2 ± 34.0 m, suggesting they calcify at the shallowest part of their diurnal migration in the mixed layer (10–35 m in the Cariaco Basin). H. inflatus shell calcification will therefore only be impacted by changes in water chemistry at mixed layer depths. The shell condition did not impact the stable isotopic composition of the shells in either the sediment trap or core sample, suggesting the potential for using the isotopic composition of pteropod shells as oceanographic proxies when they are preserved. Comparisons between sediment trap and core sample show a 0.5°C warming that is marginally significant and a significant 0.45‰ decrease in δ13C between the late Holocene and the late 1990's. These measurements reflect changes in oceanic conditions linked to anthropogenic fossil fuel emissions known as the Suess effect, and lay the groundwork for establishing pteropods as paleoceanographic proxies in the future.
Introduction
Understanding the impact of ocean acidification on epipelagic ecosystems is inherently difficult. This ~100 m deep layer spans over 360-million square kilometers in area, from the poles to the equator, and is changing at rates unprecedented in the geologic record. Ocean chemistry and hydrographic properties are monitored at fixed mooring sites (e.g., Bailey et al., 2019) and using automated gliders [e.g., Argo floats (argo.ucsd.edu)]. However, these methods are designed to measure abiotic changes in ocean chemistry at a fixed location over time, or at random locations in the ocean at a designated time. To gain a more holistic view of the impact of ocean acidification on marine biota, recent studies have proposed the development of organisms as biological indicators of human-induced changes to ocean chemistry (Bednaršek et al., 2017, 2019; Gaylord et al., 2018; Marshall et al., 2019).
Marine calcifiers, such as foraminifera and mollusks, are widely used as proxies for ocean chemistry. The shell chemistry of foraminifera and mollusks, such as mussels and oysters, record seawater conditions (e.g., Epstein et al., 1953; Anderson and Arthur, 1983; Grossman and Ku, 1986; Kucera, 2007; McConnaughey and Gillikin, 2008; Katz et al., 2010). However, despite the wealth of information drawn from these groups, they have their limitations, especially for interpreting conditions in the mixed layer. Planktic foraminifera have lifespans on the scale of weeks to months, and their blooms are often seasonal (Tedesco and Thunell, 2003; Jonkers and Kučera, 2015). The chemistry of their shells therefore records a snapshot of ocean conditions. Benthic marine mollusks are most abundant in coastal settings, and although their larvae are found in surface waters, their lack of larval diagnostic features and the limited understanding of their early life stages restricts their utility for deciphering upper ocean conditions (Parker et al., 2013; León et al., 2019).
Pteropods are a group of planktonic gastropods that, despite their abundance and global distribution (Bednaršek et al., 2012), are underdeveloped as oceanographic proxies. They form their thin shells from aragonite, a polymorph of calcium carbonate that is 1.5 times more soluble than calcite (Mucci, 1983). For this reason, they have been used as biological indicators of ocean acidification linked to anthropogenic (Busch et al., 2014; Bednaršek et al., 2019) and natural (Manno et al., 2019) forcings, and to assess seasonal and multi-decadal climatic shifts (Mohan et al., 2006; Thibodeau and Steinberg, 2018). Pteropods are more abundant in open ocean settings than other mollusks and it has been hypothesized that the pteropod Heliconoides inflatus could be the most abundant gastropod species in the world (Lalli and Gilmer, 1989). Pteropods live for about 1 year, a relatively long lifespan compared to other planktonic calcifiers (Wells, 1976; Gannefors et al., 2005; Wang et al., 2017). Like most mollusks (e.g., Grossman and Ku, 1986; Wefer and Berger, 1991; Schöne and Surge, 2012; Steinhardt et al., 2016; García-Escárzaga et al., 2019), pteropods, and specifically the group Limacinidae, have been found to calcify in near oxygen isotopic equilibrium with seawater (Grossman et al., 1986; Fabry and Deuser, 1992; Juranek et al., 2003), at depths of between 50 and 650 m (Fabry and Deuser, 1992; Jasper and Deuser, 1993; Fischer et al., 1999; Juranek et al., 2003; Keul et al., 2017). There have been no studies on the average or seasonal isotopic variability of H. inflatus shells in the Cariaco Basin. Furthermore, none of the pteropod isotope studies to date have assessed the impact of shell dissolution on isotopic composition of shell material, an essential variable to understand if pteropods are to be used as oceanographic proxies. Developing pteropods as oceanographic proxies would give new insights into the response of multicellular planktonic organisms, a previously understudied group. Pteropods contribute substantially to carbon cycling and carbonate biomass (Hunt et al., 2008; Bednaršek et al., 2012), with pteropod shells contributing 33% to shallow calcium carbonate export, and 1% to CaCO3 export at 4,000 m (Buitenhuis et al., 2019). Changes in pteropod populations therefore have the potential to affect the marine carbon cycle as a whole.
The Cariaco Basin is a tectonic depression on the Venezuelan shelf in the southwest Caribbean Sea. Seasonal changes in basin hydrography are controlled by the position of the Inter Tropical Convergence Zone (ITCZ). From December to April, the ITCZ moves south and Ekman transport, triggered by the strengthening of easterly trade winds, causes the upwelling of cold, nutrient rich waters to the surface. From August to November, the ITCZ moves north, rainy conditions prevail, and surface waters become warm and oligotrophic (Astor et al., 2013; Muller-Karger et al., 2019) (Supplementary Figure 1). The CARIACO (CArbon Retention In A Colored Ocean) program ran from 1995–2017 with the aim to monitor and document the physical and biological processes occurring in this unique basin. Sediment trap samples were collected every 2 weeks, supplemented by measurements of hydrography and water chemistry on monthly oceanographic cruises. The planktic foraminifera from the CARIACO timeseries have been used extensively to study seasonal and interannual population changes, and changes in the isotopic composition, temperature, and carbonate concentration of seawater (e.g., Black et al., 2001; Tedesco and Thunell, 2003; Tedesco et al., 2007; McConnell et al., 2009; Marshall et al., 2013, 2015; Davis et al., 2019), however, there has only been one study on pteropods from the Cariaco Basin (Oakes and Sessa, 2020). The wealth of samples, hydrographic measurements, and previous studies of calcareous plankton, makes the Cariaco Basin the ideal place to develop pteropods as oceanographic proxies.
Here we establish an annual record of the stable isotopes of oxygen and carbon in the shells of H. inflatus pteropods in the Cariaco Basin. These data are compared to established oxygen isotopic records of six planktic foraminifera species from the same sediment trap cups the pteropods were collected from Tedesco et al. (2007). Average stable isotopic values of specimens from the sediment trap samples are compared to specimens from a nearby core to assess the viability of using isotopes from pteropod shells from subfossil sediments as recorders of oceanographic conditions (Figure 1). This study is the first to establish an annual record of stable isotopic composition of pteropod shells in the Cariaco Basin, and to compare pteropod shells from sediment traps to those from surficial sediment samples.
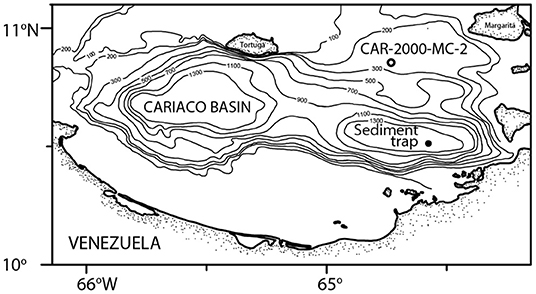
Figure 1. Location of the sediment trap (filled circle) and the CAR2000-MC-2 core (open circle) where specimens from this study were collected.
Materials and Methods
Sediment Trap Collection
The samples in this study came from a sediment trap deployed in the Cariaco Basin, off the coast of Venezuela (10° 30′ N, 64° 38.5′ W) as part of the CARIACO Time Series (Figure 1). The trap was at 150 m water depth (also known as the A trap) and samples were collected continuously for two-week intervals. Trap cups were filled with borate-buffered formalin prior to deployment to prevent degradation of the material after collection (Thunell et al., 2000). Sediment trap cups from between November 1996 and April 1998 were processed as described in Thunell et al. (2000) and Tedesco and Thunell (2003). Unfortunately samples were not collected from late March-April 1997 as the sediment trap was clogged (Tedesco et al., 2007). A quarter split was washed with deionized water over a 150-micron sieve and calcareous plankton were wet picked and then dried in an oven at 40°C for 24 h, before being stored for faunal analysis.
Core
Multicore CAR2000-MC-2 was taken on October 31, 2000 from the Cariaco Basin (10° 51′ N, 64° 44′ W; 192 m water depth). Pteropod specimens were picked from the 0.5–1.0 cm interval and later identified to the species level. Whole shells of the planktic foraminifera Globigerinoides sacculifer were also picked from this interval for carbon dating (see section Carbon Dating).
Specimen Selection
Pteropod shells were present in 17 out of 36 trap samples. We sampled 64 H. inflatus specimens from 17 trap samples collected between November 1996 and April 1998 (Table 1). A further 66 H. inflatus specimens were sampled from the CAR2000-MC-2 core: 22 for carbon and oxygen isotopic analysis, and 44 for carbon dating.
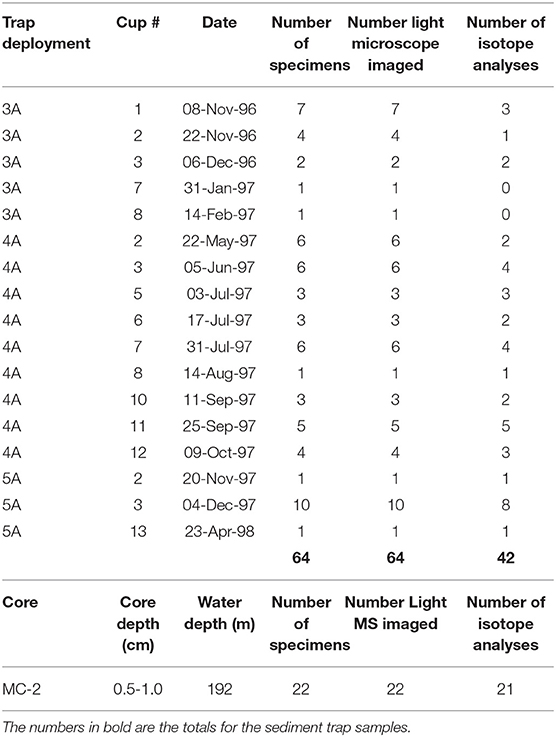
Table 1. Sample information about the specimens from the sediment trap and the core top used in this study.
Light Microscopy
Light Microscopy Image Collection
All 64 specimens from the sediment trap, and the 22 specimens from the core that were analyzed for stable isotopes, were imaged in the Malacology Imaging Center at the Academy of Natural Sciences of Drexel University, Philadelphia. Pteropods were positioned in apical view in a well slide with a black base. Images were taken using a circa 1950 Bausch & Lomb compound microscope attached via an eyepiece adaptor to a Nikon D5100 DSLR camera. Specimens were lit by a DynaLite 500XL power pack with two reversed studio heads and diffusers. A Carl Zeiss calibrated slide was photographed at the same magnification as the images in order to create a scale bar. Focus stacking was used to create an image with more depth of focus than is possible with a single exposure. The focus stack was created manually by taking 15–30 images per specimen at different depths of focus depending on the z-height of the shell. Images were compiled into a focus stack using the software Helicon Focus v. 6.7.2 with the pyramid rendering method (method C). All light microscope images of specimens from the sediment trap and the core are available in the Supplementary Materials (Supplementary Figure 2).
Assessment of Shell Condition and Life Stage
The condition of pteropod shells is used as an indicator of the amount of dissolution the shell has undergone; pristine shells are glassy and transparent, and highly dissolved shells are white and lusterless (Almogi-Labin et al., 1986; Gerhardt and Henrich, 2001). All shells from the sediment trap and the core were assessed using the Limacina Dissolution Index (LDX) (Gerhardt and Henrich, 2001) following the findings of Oakes and Sessa (2020) [Data in Supplementary Table 1 (sediment trap), and Supplementary Table 2 (core)].
To assess whether life stage affected isotopic composition, shells were split into juvenile (<1 mm) and adult (>1 mm) specimens based on the size differences found in these H. inflatus life stages off Barbados (Lalli and Wells, 1978). Shell size was assessed using shell diameter, measured in pixels at the widest part of the shell (Lischka et al., 2011) in ImageJ (Schneider et al., 2012). The image of the scale bar was used to convert the number of pixels to length.
Measurement of Stable Isotopic Composition (δ18O and δ13C) of Pteropod Shells
Pteropod shells were gently crushed with tweezers and any residual organic (sediment trap) or sediment (core) material was removed. Shell fragments were triple washed in borate-buffered deionized water. Oxygen and carbon isotopes were analyzed on a GV IsoPrime ratio mass spectrometer at the University of South Carolina. All results are reported relative to Vienna Pee Dee Belemnite (VPDB), and precision based on replicate analyses of an in-house Carrera marble standard during these analyses was 0.05‰ (1σ) for δ18O and 0.03‰ (1σ) for δ13C. In most cases, single pteropod specimens were analyzed, however when individual masses were below 12 μg, smaller shells were combined to meet a minimum mass for analysis of 12 μg. The number of specimens per analysis is clearly marked on all figures. All data are presented in Table 2. All δ18O terms used in this study are summarized in Table 3.
Seawater Composition
Contemporaneous measurements of the stable isotopic composition of seawater (δ13CSW, δ18OSW) are not available. The oxygen isotopic composition of seawater was therefore calculated from seawater salinity measurements (S) from discrete bottle samples collected between September 1996 and June 1998. Hydrographic measurements were made on monthly cruises at 19 discrete depths (1, 7, 15, 25, 35, 55, 75, 100, 130, 160, 200, 250, 300, 350, 400, 500, 750, 1,000, 1,200 m): data are publicly available at http://imars.marine.usf.edu/WebPageData_CARIACO/Master_Hydrography/. The salinity data were input into three formulas: the LeGrande and Schmidt (2006) Tropical Atlantic calibration (δ18OSW = 0.15*S−4.61), the McConnell et al. (2009) Cariaco Basin monthly calibrations (see Supplementary Table 3 for full list of equations), and the McConnell et al. (2009) Cariaco Basin annual calibration (δ18OSW = 0.34*S−11.48). The LeGrande and Schmidt (2006) equation is used throughout the study (see section Oxygen Isotopes) and has a δ18OSW error of ± 0.164‰.
Discrete bottle samples were also analyzed for nutrient concentrations and carbonate chemistry parameters (Astor et al., 2011). Water column properties were interpolated between sample days, and between bottle sample depths, using the interp function in the “akima” package (Akima and Gebhardt, 2016) in the computing language R, version 3.6.0 (R. Core Team, 2019), using RStudio (RStudio Team, 2016). The depth and date of sampling are clearly marked on Figures 5, 9.
Pteropod Calcification Depths
The apparent calcification depth (ACD) of pteropods was calculated following the findings of other studies that pteropods calcify in near equilibrium with the δ18O of seawater (Grossman et al., 1986; Fabry and Deuser, 1992; Jasper and Deuser, 1993). In H. inflatus, the majority of the shell volume is comprised of the final, or body, whorl, which represents the most recently calcified material (Fabry and Deuser, 1992). Shell isotopic measurements will therefore be dominated by this most recently calcified whorl (Keul et al., 2017). The δ18O of seawater, δ18OSW, for each discrete bottle sample was calculated using the LeGrande and Schmidt (2006) calibration. The theoretical δ18O of an aragonitic shell calcified in equilibrium with δ18OSW was determined from these calculated δ18O seawater values and CTD-derived temperature measurements (T) following the equation from Böhm et al. (2000) (δ18Oarag = (T-20)/(−4.42) + δ18OSW). Measured δ18O of pteropod shells (δ18Optero) were compared to full water column δ18O (δ18Oarag) (i.e., 0–1,300 m); the ACD was assigned as the depth in the water column on the date the sediment trap opened where δ18Optero and δ18Oarag were equal. As all pteropods were found to calcify in the top 200 m, the figures only present the top 200 m of the water column.
Carbon Dating
In order to determine the age of the core samples, 3.1 mg of pteropod (H. inflatus) shells and 8.1 mg of whole planktic foraminifera (Globigerinoides sacculifer) tests from core CAR2000-MC-2 were carbon dated at the Keck-Carbon Cycle AMS facility at the University of California, Irvine. Both pteropods and foraminifera were measured since the carbon ages of foraminifera tests are commonly analyzed in oceanographic and paleoceanographic work whereas pteropods are less well-studied. The foraminifera G. sacculifer has an average calcification depth of 25 m in the Cariaco Basin (Wejnert et al., 2013) so will be affected by similar water column properties as H. inflatus. Samples were leached 10% with dilute HCl prior to hydrolysis with 85% phosphoric acid. Measurements have been corrected for isotopic fractionation according to the conventions of Stuiver and Polach (1977), with δ13C values measured on prepared graphite using the AMS spectrometer.
Statistical Methods
Simple linear models were used to test the relationship between two parameters. Normality was tested using a Shapiro-Wilks test. The difference in variance between two normally distributed groups was tested using an F-test, and between two non-normally distributed groups using a Fligner-Killeen's test. The difference in means between two normally distributed groups was tested using Welch's t-test and between two non-normally distributed groups using the Wilcoxon rank-sum test. All statistical analyses were performed in R version 3.6.0 (R. Core Team, 2019) using the RStudio Interface (RStudio Team, 2016).
Results
Pteropod Shell Condition
Pteropod shell condition, ranked using the LDX (Gerhardt and Henrich, 2001), varied between 0 (pristine, transparent shell with smooth surface) and 2.5 (opaque, white shell that is beginning to lose surface sheen) in specimens from the sediment trap (Figure 2, Supplementary Table 1) and between 0.5 and 2.5 in specimens from the core (Supplementary Figure 4, Supplementary Table 2). There was no correlation between the amount of time pteropod shells spent in the collecting cups and their shell condition (p = 0.507, R2 = 0.011) (Supplementary Figure 3), similar to the findings of another sediment trap study in the Cariaco Basin (Oakes and Sessa, 2020). Similarly, there was no correlation between the shell condition of the pteropods from the sediment trap or the core and the δ18O or δ13C of shell material (δ18Otrap: p = 0.791, R2 = 0.002; δ13Ctrap: p = 0.944, R2 = 0.0001; δ18Ocore: p = 0.742, R2 = 0.006; δ13Ccore: p = 0.309, R2 = 0.054) (Figure 2, Supplementary Figure 4).
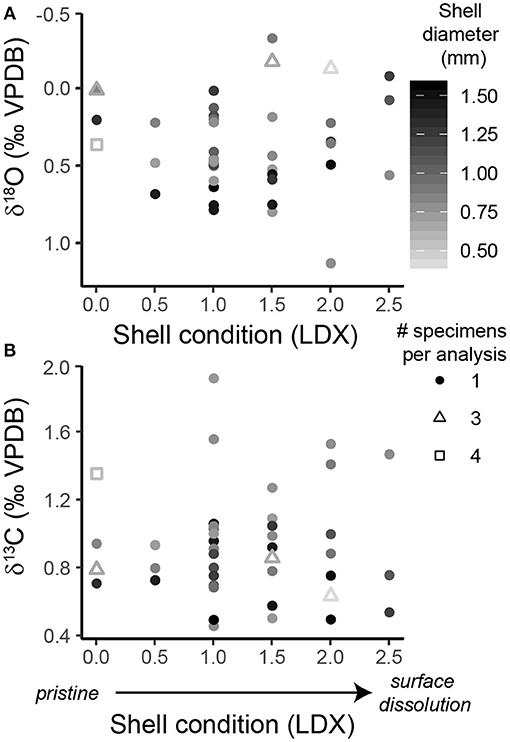
Figure 2. Scatter plots of the shell condition of H. inflatus shells from the sediment trap, assessed using the Limacina Dissolution Index (LDX: 0 = pristine, 5 = highly altered), and (A) the δ18O and (B) δ13C of the shells. Following convention, the δ18O axis is inverted. The color of the points corresponds to the shell diameter. The shape of the point represents the number of specimens pooled per isotopic analysis (see key in figure).
To further explore whether the extent of dissolution affected shell isotopic composition, specimens were split into two groups: those that had undergone minor dissolution (LDX 0–1.5), and those that had undergone moderate dissolution (LDX 2-3). This threshold was chosen because shells with an LDX of 2 and higher have become fully opaque and therefore have, “truly experienced initial dissolution on the surface” (Gerhardt and Henrich, 2001, p. 2059). The δ18O and δ13C measurements were not significantly different between shells which had experienced minor vs. moderate dissolution, in either the sediment trap or core samples (δ18Otrap: p = 0.458, W = 186; δ13Ctrap: p = 0.836, W = 167; δ18Ocore: p = 0.689, W = 43; δ13Ccore: p = 0.197, W = 67) (Figure 2; Supplementary Figure 4).
Sediment Trap
Oxygen Isotopes
Oxygen isotope measurements of specimens from the sediment trap samples ranged from −0.32 to 1.13‰ (1.45‰ range) with a median value of 0.44‰ (Figure 3A, Table 2). The highest values were measured in specimens sampled during the summer (July–September 1997), and the lowest values were measured in specimens from October and November 1997 (Figure 3A). The range in ACDs for pteropod shells measured from the same sediment trap cup varies between 2 and 90 m with greater variability occurring in the non-upwelling conditions (Figure 5, Supplementary Table 4). There was not a significant relationship between the oxygen isotopic composition of pteropod shells and their shell diameter (p = 0.068, R2 = 0.081) (Figure 4A). The variability in δ18Optero measurements of adult (>1 mm diameter) and juvenile (<1 mm diameter) shells, corresponding to the transition from juvenile to male for this species (Lalli and Wells, 1978), were not significantly different (F = 1.334, p = 0.547) (Figure 4A).
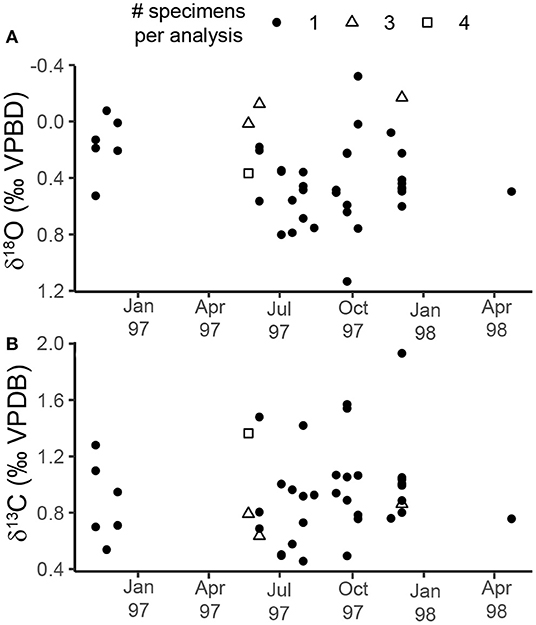
Figure 3. Scatter plots showing the changes in: (A) δ18O, and (B) δ13C throughout the study period for the sediment trap. The shape of the point represents the number of specimens pooled per isotopic analysis (see key at top of figure). Following convention, the δ18O axis is inverted. The breaks in the record correspond to times when pteropods were not found in the faunal splits from the sediment trap samples.
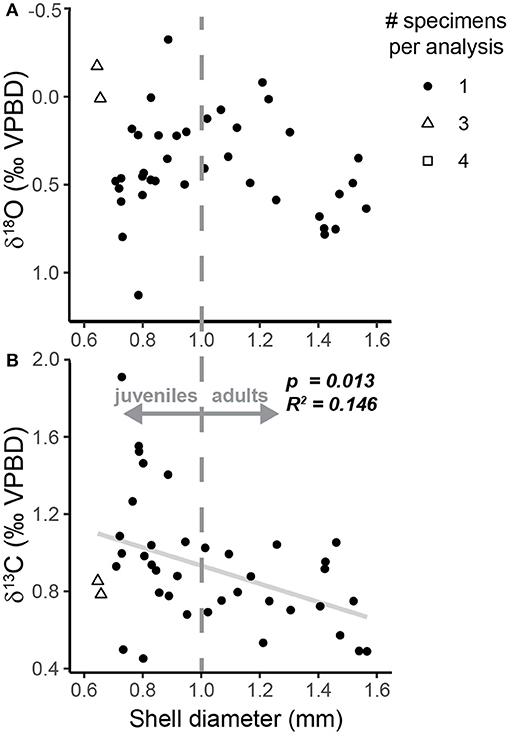
Figure 4. Scatter plots of H. inflatus shell diameter relative to the (A) δ18O and (B) δ13C of shells from the sediment trap. Following convention, the δ18O axis is inverted. The shape of the point represents the number of specimens pooled per isotopic analysis (see key in figure). The gray dashed line represents the break between juvenile and adult pteropod shells following the work of Lalli and Wells (1978). The relationship between δ18O and shell diameter is not significant (p = 0.068, R2 = 0.081). The relationship between δ13C and shell diameter is significant (p = 0.013, R2 = 0.146; trendline plotted in gray solid line), and δ13C measurements of adult shells (>1.0 mm) are significantly less variable (F = 3.60, p = 0.009) than those in juvenile shells (<1.0 mm).
H. inflatus pteropods were found to calcify at an average ACD of 51.2 ± 34.0 m using the LeGrande and Schmidt (2006) calibration (1 standard deviation (s.d.) range = 1–153 m) (Figure 5). If we were to apply the month-specific (Supplementary Table 3), and yearly average calibrations from McConnell et al. (2009) average ACDs would be 38.5 ± 33.2 m (1 s.d.) and 40.3 ± 31.9 m (1 s.d.) respectively (Supplementary Figure 5). The relevant calibrations from McConnell et al. (2009) are sometimes limited to 100 m depth, rather than covering the full range of pteropod ACDs, and are therefore likely to be less reliable. The calibration from LeGrande and Schmidt (2006) has therefore been used in all following calculations.
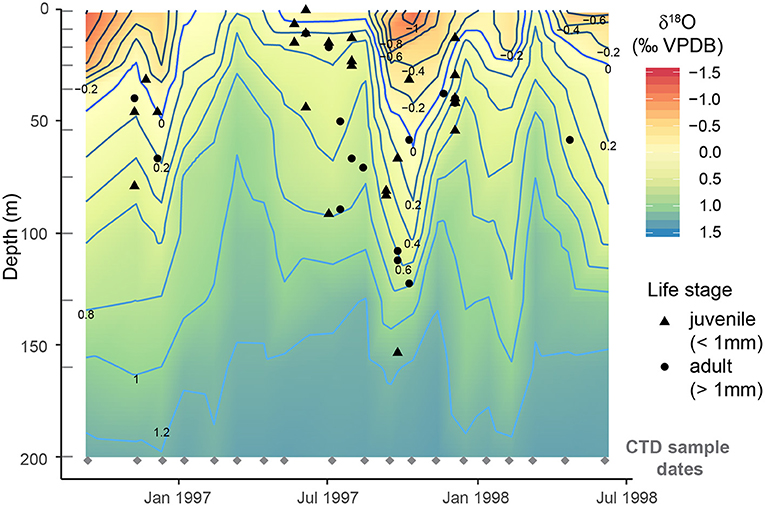
Figure 5. Calcification depths of H. inflatus determined by comparing δ18O measurements of shells to δ18O of the water column calculated from CTD sampled bottle measurements of salinity using the LeGrande and Schmidt (2006) Tropical Atlantic calibration (two additional calibrations are presented in Supplementary Figure 4). The average calcification depth was 50.2 ± 33.5 m (1 s.d.). The shape of the point represents the life stage of the individual: juveniles (<1 mm), adults >1 mm (Lalli and Wells, 1978). There is not a significant correlation between calcification depth and shell size (p = 0.090, R2 = 0.067). CTD collection dates are marked by gray diamonds and CTD bottle depths are marked by gray lines; δ18OSW values between these points have been interpolated.
Carbon Isotopes
Carbon isotope measurements of sediment trap specimens ranged from 0.46 to 1.91‰ (1.45‰ range) with a median value of 0.88‰ (Figure 3B, Table 2). The highest values were measured in specimens from September and December 1997 and the lowest values were measured in specimens from July 1997 (Figure 3B). There is a significant relationship between the carbon isotopic composition of pteropods shells and their shell diameter (p = 0.013, R2 = 0.146) (Figure 4B). The variability of δ13Cptero in juvenile shells (<1 mm diameter) is significantly higher than in adult shells (>1 mm diameter) (p = 0.009, F = 3.60), however there is not a significant relationship between the ACD and δ13C (p = 0.215, R2 = 0.038). There is not a strong or significant relationship between the δ18O and δ13C of the shell material (p = 0.074, R2 = 0.077) (Figure 6).
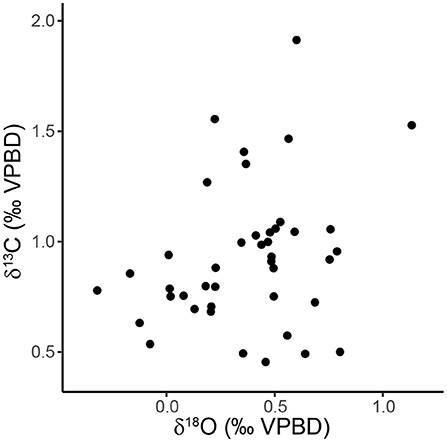
Figure 6. Scatter plot showing the relationship between δ18O and δ13C of H. inflatus shells from the sediment trap samples (p = 0.074, R2 = 0.077).
Core
The radiocarbon dates from foraminifera (G. sacculifer) and pteropods (H. inflatus) varied greatly: planktic foraminifera yielded a date of 1740 ± 15 BP, while pteropods gave a date of 860 ± 15 BP, a difference of 880 years (Table 4). Applying a marine surface reservoir correction for the Cariaco Basin of 420 years (Hughen et al., 1996), the sediment core is dated to either 1,340 ± 15 BP if G. sacculifer is used and 440 ± 15 BP if H. inflatus is used.

Table 4. 14C ages of Globigerinoides sacculifer (planktic foraminifera), and Heliconoides inflatus (pteropod) from the CAR2000-MC-2 core in the Cariaco Basin.
Oxygen isotope measurements of specimens from the core samples were higher than those from the sediment trap, ranging from 0.13 to 1.36‰ (1.23‰ range) with a median value of 0.51‰ (Figure 7A, Supplementary Table 5). Using the temperature calibration from Keul et al. (2017), this offset represents a 0.5°C difference between the median values of samples from the core and the sediment trap, however the difference was only significant at p = 0.071. Carbon isotope measurements of specimens from the core were higher than the specimens from the sediment trap sample, ranging from 0.80 to 1.75‰ (0.95‰ range) with a median value of 1.33‰ (Figure 7B, Supplementary Table 5). The median core δ13C is 0.45‰ higher than the median sediment trap value, which represents a statistically significant difference (p = 1.91 × 10−5).
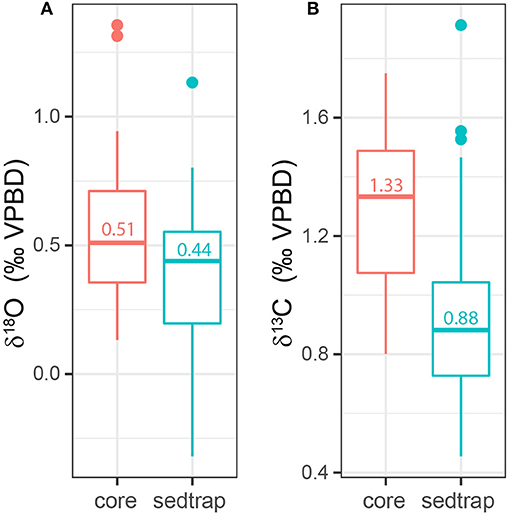
Figure 7. Box and whisker plots showing the difference between (A) the δ18O and (B) δ13C measurements of H. inflatus specimens from the sediment trap (blue) and the core (red). Both δ18O and δ13C values are higher in the core material than in the sediment trap. Median values are written inside each box.
Discussion
Fidelity of δ13C and δ18O Records From Pteropod Shells
Pteropods' fragile, aragonitic shells make them susceptible to dissolution, a concern when developing a proxy. The Cariaco Basin is anoxic below 300 m depth due to a shallow sill restricting exchange with other ocean basins (Hughen et al., 1996). The carbonate-rich waters in this shallow basin are supersaturated with respect to aragonite, even in the deepest part of the basin (1,300 m; Figure 1). The combination of anoxia and aragonite supersaturated seawater creates an ideal environment for the preservation of calcareous and siliceous microfossils, including pteropod shells (Peterson et al., 1991).
The chemical composition of mollusk shells, when sufficiently preserved, have been found to record the physical and chemical environmental conditions at the time of shell formation (e.g., Epstein et al., 1953; Anderson and Arthur, 1983; Grossman and Ku, 1986; McConnaughey and Gillikin, 2008). No previous studies have specifically investigated the relationship between pteropod shell condition and stable isotopic composition. Fabry and Deuser (1992) found that there was no change in the isotopic composition of pteropod shells between sediment trap samples from 500 to 3,200 m, implying minimal isotopic exchange occurred in the water column. In this study, there was no correlation between shell condition and δ13C or δ18O of shell material, despite visible alteration of pteropod shells from both sediment trap and core materials (Figure 2, Supplementary Figure 4). This demonstrates that in aragonite-supersaturated waters and in shallow subfossil sediment, the isotopic composition of H. inflatus pteropod shells is not altered during initial dissolution (at least up to an LDX score of 2.5), and shells in this preservational range are well-suited for use as oceanographic proxies.
Calculating Calcification Depth From δ18O
Based on comparison between the calculated δ18Oarag of seawater (LeGrande and Schmidt, 2006) and measured δ18O of pteropod shells, we found an ACD of 51.2 ± 34.0 m for H. inflatus in the Cariaco Basin (Figure 5). This falls within error of previously reported calcification depths of other pteropods within the same superfamily as H. inflatus; Limacina helicina from the Sargasso Sea (50 m, Fabry and Deuser, 1992), L. bulimoides from the North Pacific (75–125 m for large specimens, Grossman et al., 1986), and H. inflatus from the Atlantic Ocean (75 m, Keul et al., 2017), and is at the shallow end of the range proposed by Juranek et al. (2003) for H. inflatus from the Sargasso Sea (50–650 m). Unlike in L. bulimoides from the North Pacific (Grossman et al., 1986), we found no significant difference in the ACD of juvenile and adult specimens of H. inflatus in the Cariaco Basin.
Shell isotopic composition was compared to water chemistry on the day the sediment trap opened. Pteropods live for about a year (Wells, 1976; Fabry and Deuser, 1992), and although early studies on this species proposed constant growth rates over time (Wells, 1976), studies on other groups from the same superfamily have found that shell growth rates vary substantially depending on season, geographical region, and food availability (Dadon and de Cidre, 1992; Wang et al., 2017). Shell growth of H. inflatus in the Cariaco Basin has been found to vary associated with changes in upwelling, with larger, thicker shells forming during periods of wind-driven upwelling when nutrient concentrations, and food availability are higher (Oakes and Sessa, 2020). It is therefore likely that the individuals in this study experienced different water column conditions and therefore had different growth rates. As the growth rates of these specimens are unknown and there is not enough material to perform incremental isotopic measurements through the shell, the sediment trap opening date water chemistry is used as water chemistry at the time of calcification.
Tedesco et al. (2007) studied the seasonal and interannual variability of oxygen isotopes in six species of foraminifera from 1997 to 1999, sampled from the same sediment trap as this study. In order for the oxygen isotopic composition of aragonitic pteropods and calcitic planktic foraminifera to be compared, the aragonitic values are adjusted by 1.0‰ to account for the different fractionation factors between ambient seawater and calcite and aragonite (Böhm et al., 2000). When plotted in δ18Ocalcite space, H. inflatus fall in a similar range (−1.3 to 0.13‰, average −0.63‰) to the planktic foraminifera Orbulina universa (−0.14 to 0.03‰, average −0.37‰), a mixed-layer to thermocline dwelling organism which reaches its highest abundances at ~50 m in the east tropical Atlantic (Ravelo and Fairbanks, 1992; Tedesco et al., 2007) (Figure 8). The similarity of O. universa and H. inflatus oxygen isotopic values supports the calculated ACD for H. inflatus in the Cariaco Basin (51.2 ± 34.0 m).
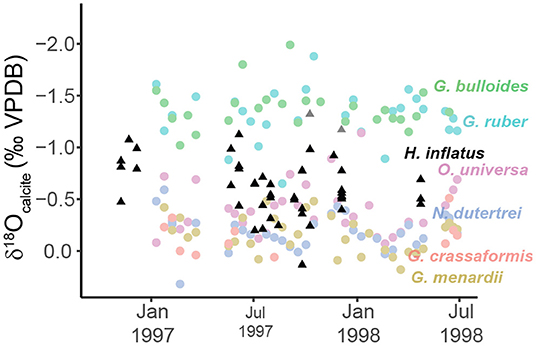
Figure 8. Scatter plot showing the oxygen isotopic composition of six species of foraminifera (colored circles) (Tedesco et al., 2007) and one species of pteropod (black triangles) (this study) from CARIACO sediment trap A (150 m water depth) between October 1996 and July 1998. Because aragonite and calcite have different fractionation factors relative to ambient seawater, the oxygen isotopic composition of pteropods (aragonite) are adjusted using the calibration from Böhm et al. (2000) (black triangles, 1‰ offset) to plot in calcite space.
The range of pteropod ACDs measured within one sample varies throughout the year, with smaller depth ranges during times of upwelling, and more varied ACDs during non-upwelling conditions (Figure 5, Supplementary Table 4). A greater range between minimum and maximum ACD can be interpreted as signifying an expanded vertical habitat, and likely intervals with a deeper mixed layer. We hypothesize that pteropods are recording this change in hydrography. The number of pteropods which reach the sediment trap cups varies with time, with some cups containing no pteropod shells. This could be the result of the inherent patchiness of pteropod distributions (Bednaršek et al., 2012; Thibodeau and Steinberg, 2018), or could reflect seasonality in the flux of shells, a hypothesis which could be tested with examination of a longer timeseries.
Previous studies have found that there are only small annual changes in the salinity in the Cariaco Basin [equivalent to ~0.10‰ δ18O (Tedesco et al., 2007)] therefore the majority of variation δ18OSW can be attributed changes in temperature. Using the only established palaeothermometry equation for H. inflatus (Keul et al., 2017) and CTD temperature profiles yields an average calcification depth of 15.9 ± 25.9 m (1 s.d.) (Supplementary Figure 6). This estimate is shallower than the ACD's calculated from seawater δ18O and comparisons with planktic foraminifera in this study, and shallower than previously published calcification estimates of H. inflatus (Fabry and Deuser, 1992; Juranek et al., 2003; Keul et al., 2017). In terms of δ18Optero, values are lower than would be expected if specimens had calcified at 50 m, the depth at which the paleothermometer was calibrated (Keul et al., 2017). One explanation for this offset could be that the specimens in this study span the whole year, whereas the specimens and water chemistry samples used to calibrate the paleothermometer were from single net samples taken between October and November 2012 (Keul et al., 2017), skewing the calibration toward cooler values.
Comparing ACDs to temperature profiles from CTD casts reveals 78% of individual H. inflatus calcify in water between 21 and 24°C, rather than calcifying at a fixed depth throughout the year (Figure 9). Calcification rates in other groups of marine calcifiers, such as bivalves (Kennish and Olsson, 1975; Jones et al., 1989), corals (Clausen and Roth, 1975; Bessat and Buigues, 2001), and coccolithophores (Buitenhuis et al., 2008), are temperature sensitive. H. inflatus in the Cariaco Basin may, therefore, be tracking their optimal temperature range for shell precipitation. Temperature can co-vary with other important parameters such as food and nutrient availability, light, and salinity, and therefore their calcification depth distribution may be controlled by a combination of these factors (Jonkers and Kučera, 2015).
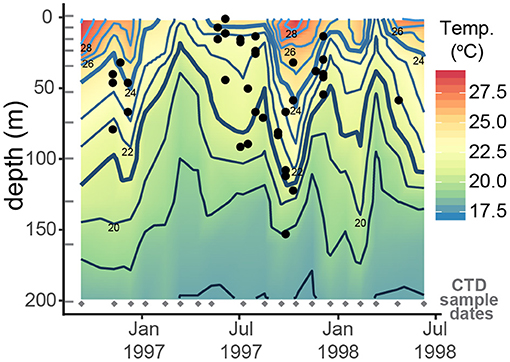
Figure 9. Apparent calcification depths of H. inflatus plotted relative to the seawater temperatures from CTD casts. CTD sampling days are marked by gray diamonds and depths are marked by gray dashes; δ18O values in between these points are interpolated. Over eighty percent of H. inflatus apparent calcification depths fall between the 21 and 24°C contours (bold lines), suggesting that H. inflatus is tracking this habitat throughout the year rather than calcifying at a fixed depth.
Pteropods migrate diurnally, and in the Florida Strait H. inflatus have an average daytime depth of 230 m (spread = 177 m), and migrate upwards at night (Wormelle, 1962). If we assume that H. inflatus in the Cariaco Basin has a similar migratory pattern, the δ18Optero suggests that the majority of calcification occurs at night when H. inflatus reach the shallowest part of their migration. Night-time calcification, at the shallowest part of a pteropods diurnal migration, has been proposed in previous studies (Fabry and Deuser, 1992; Keul et al., 2017), hypothesized to be due to the lower energy requirements for calcification in warmer, more aragonite saturated, shallow waters.
Deciphering Pteropod δ13C
The δ13C of the shells of mollusks incorporate both respired CO2, derived from dietary organic carbon, and dissolved inorganic carbon (DIC) (e.g., Tanaka et al., 1986; McConnaughey, 1989; McConnaughey et al., 1997; Owen et al., 2002; Lorrain et al., 2004; McConnaughey and Gillikin, 2008; Butler et al., 2011). Carbon isotopes derived from a mixture of these sources (and of the intermediate calcification steps) can be difficult to disentangle (McConnaughey and Gillikin, 2008), but the proportion of respired carbon incorporated into the shells of aquatic (marine and freshwater) mollusks ranges between <10 and 90% (McConnaughey and Gillikin, 2008; Butler et al., 2011). Studies specifically focusing on δ13C in pteropod shells have investigated the effects of dissolved inorganic carbon and respired CO2 on δ13Cptero. In a study of six species of pteropods, including Limacina bulimoides, in the North Pacific Ocean, Grossman et al. (1986) found no shells precipitated in 13C equilibrium with dissolved bicarbonate. A study of “Limacina inflata” (now H. inflatus) from Bermuda concluded that δ13Cptero is influenced by metabolic incorporation of CO2 during shell growth as well as ambient seawater [] (Juranek et al., 2003). The carbon isotopic composition of pteropod shells in this study is discussed in the context of DIC, metabolic vital effects, and diet.
The δ13C of pteropod shells has been shown to record the concentration of carbonate ions in seawater (Keul et al., 2017). The calibration presented in Keul et al. (2017) is valid for δ13C of <1‰, equivalent to minimum carbonate ion concentrations of >200 μmol/ kgSW. These conditions are only found in the upper 50 m of the water column in the Cariaco Basin, shallower than the ACD of most pteropods. Despite the limitations, if the equation of Keul et al. (2017) is applied to this dataset, the ACD would be 38 ± 38 m (Supplementary Figure 7), within error of the ACD assessed by δ18O.
There are significant differences in δ13C variability between juvenile (<1 mm) and (>1 mm) adult pteropod shells (F = 3.60, p = 0.0087) which may indicate the presence of a metabolic vital effect (Figure 4B). The isotopic composition of scallop specimens displaying high growth rates has been found to deviate more from isotopic equilibrium than specimens with slower growth rates (Owen et al., 2002), and several studies have found that growth rates decrease and become less variable with age (Schöne et al., 2005; Butler et al., 2010; Mancuso et al., 2019). Assuming similar relationships occur in pteropods, the increased variability in δ13C of juvenile pteropods relative to adults could be attributed to higher growth rates in smaller specimens. Alternatively, Grossman et al. (1986) found two other species of pteropod, Cuvieria columnella and Diacria trispinosa, incorporated more metabolic carbon with age, which would cause the δ13C of adult shells to be less variable than juvenile shells.
Studies of other groups of planktonic calcifiers (Uhle et al., 1997; Doi et al., 2010) pelagic nekton (Gorokhova and Hansson, 1999), and mollusks (McConnaughey and Gillikin, 2008) have found that organism δ13C is linked to diet, and that diet can change with growth (Doi et al., 2010). There is not a significant relationship between δ13C and ACD, suggesting that H. inflatus of all sizes in this study (0.67–1.52 mm) were calcifying in the same water mass (Figure 5). The difference in δ13C variability between juvenile and adult pteropods suggests that pteropods may target different food sources dependent on their life stage. Pteropods feed on small crustaceans, amphipods, copepods, and phytoplankton (Lalli and Gilmer, 1989; Gilmer and Harbison, 1991). Gilmer and Harbison (1986, 1991) found that over 40% of the diet of large (i.e., adult) Limacina helicina pteropods in the Arctic Ocean was composed of juvenile pteropods and crustaceans, but suggested smaller L. helicina (i.e., those at an earlier lifestage) may be herbivorous, transitioning to omnivory as they grow. The lower variability in δ13C of adult pteropods may, therefore, be due to their diet being composed of few, large zooplankton while juvenile pteropods would need to consume a large number of smaller planktonic organisms such as diatoms, algae, and dinoflagellates to gain their energy. These smaller planktonic organisms span a wide range of δ13C values (Hansman and Sessions, 2016; Tiselius and Fransson, 2016) which would cause the δ13C of juvenile pteropod shells to be more variable than adults.
Using Pteropods as Proxies in the Shallow Sedimentary Record
Core specimens of H. inflatus were dated to 440 years BP. Interestingly, planktic foraminifera (G. sacculifer) from the same sample were dated to 1,340 years BP. Incongruent 14C ages from planktic foraminifera and pteropods have been documented in previous studies (Price et al., 1985; Ganssen et al., 1991; Mekik, 2014) and this offset has been attributed to differences in sedimentation rates, bioturbation, and winnowing between the two groups. In contrast to previous findings, the pteropods from the MC-2 core record a younger age than the planktic foraminifera sampled at the same stratigraphic level. Regardless of which date is correct, specimens are late Holocene in age, and therefore record δ13C marine reservoir and seawater temperatures from pre-1850 (i.e., prior to changes related to anthropogenic emissions) (Beveridge and Shackleton, 1994). Both the oxygen and carbon isotopic values of pteropod shells from the core material are higher than specimens from the sediment trap, although the change in δ18Optero is only significant at p = 0.071 (Δ18O = 0.07‰, Δ13C = 0.45‰) (Figure 7, Supplementary Table 5). Applying the calibration from Keul et al. (2017), the offset in δ18O represents an increase in median seawater temperatures recorded by pteropods of 0.5°C between the late Holocene and 1997. This is comparable to the amount of warming recorded by planktic foraminifera from the same basin between 1850 and 1990 (Black et al., 2007).
The 0.45‰ decrease in δ13C is of similar magnitude to the changes recorded in the planktic foraminifera Globigerinoides ruber (pink) from the same basin between 1750 and 1998 (0.5–0.6‰) (Black et al., 2011). The δ13C of marine carbonates, such as corals (Swart et al., 2010) and planktic foraminifera (Beveridge and Shackleton, 1994), have been shown to record the change in atmospheric and oceanic reservoirs of 13C associated with the combustion of 13C poor fossil fuels known as the 13C Suess Effect (Suess, 1955; Keeling, 1979). The 0.07‰ decrease in δ18O, and 0.45‰ decrease in δ13C between late Holocene samples and sediment trap samples from 1997 show that the H. inflatus shells from Cariaco Basin surface sediments record both the warming, and the 13C Suess Effect caused by increased combustion of fossil fuels since the industrial revolution.
Implication of Establishing Pteropods as Proxies
The shells of H. inflatus record seawater chemistry from mixed layer depths (51 ± 34 m), tracking a 21–24°C temperature range throughout the year. Understanding where this ocean acidification-susceptible species calcifies delineates a critical depth range for monitoring with regards to ocean acidification. Although the shells of other marine calcifiers, such as foraminifera and benthic mollusks, have been traditionally used for oceanographic analyses (see Urey et al., 1951; McConnaughey and Gillikin, 2008; Pearson, 2012), foraminifera are short-lived and influenced by seasonality, while benthic mollusks are orders of magnitude less common than pteropods in ocean cores. The development of proxies from pteropod shells gives a new insight into how these abundant, multicellular calcifying plankton respond to ocean chemistry, both on seasonal and annual time scales in the modern ocean, and potentially over decadal to millennial time scales in the fossil record.
Because of their size, pteropod shells settle relatively quickly through the water column at rates of 850–1,700 m/day (Bergan et al., 2017). Pteropod shells are therefore less likely to be transported post-mortem than smaller planktonic calcifiers (Kalberer et al., 1993), however, their delicate, aragonitic shells mean they are not well-preserved in all oceanographic settings, which has likely limited their development as oceanographic proxies. The pteropod fossil record reflects the specialized nature of their preservation: the oldest known pteropod is a single specimen preserved as a mold in Campanian (~82 Ma) sediments; there is then a 20 million year gap in their fossil record (Janssen and Goedert, 2016). Molds are the dominant preservation style, until the Late Oligocene (~27 Ma) when shell material is more commonly preserved (Janssen, 1990, 2012). We predict that pteropod fossils retaining original shell material will be found in ocean basins which have experienced aragonite-supersaturated conditions at depth, such as the Red Sea (Almogi-Labin et al., 1991) and the Cariaco Basin. Despite the geographically restricted nature of the pteropod fossil record, the promise of pteropods as oceanographic proxies, as outlined in this study, suggests there is a need for exploration of the pteropod fossil record in other ocean basins.
Conclusions
Pteropods' importance for monitoring ongoing changes in ocean chemistry positions them well to be developed as oceanographic proxies. Shells of H. inflatus record the stable isotopic composition at ~50 m depth, the shallow part of their diurnal migration. They appear to track a temperature range of 21–24°C. This delineates a focused range of water conditions that should be monitored to understand the impact of changing ocean chemistry on this ecologically relevant species. The isotopic composition of pteropod shells is not affected by moderate dissolution in this aragonite-supersaturated basin. Comparing pteropod shells from the sediment trap to surface sediment samples reveals a 0.5°C increase in temperature and 0.45‰ decrease in δ13C since the late Holocene, recording the warming and changing isotopic composition of carbon reservoirs associated with anthropogenic combustion of fossil fuels known as the Suess Effect. The data presented in this study highlight that pteropods are viable candidates to be developed as oceanographic and paleoceanographic proxies in environments where their shells are preserved. Developing this new proxy will enable investigations into how multicellular planktonic organisms have been impacted by past climatic changes on timescales from weeks to millennia.
Data Availability Statement
The original contributions presented in the study are included in the article/Supplementary Material, further inquiries can be directed to the corresponding author/s.
Author Contributions
RO, CD, and JS conceived the idea. RO identified specimens, took light microscope images, performed statistical analyses, made figures, and wrote the initial manuscript draft. CD ran isotopic analyses. RO and CD ran initial data analyses and drafted the manuscript. All authors read and edited the manuscript and approved the submitted version.
Funding
RO was supported by the John J. & Anna H. Gallagher Fellowship, The Academy of Natural Sciences of Drexel University.
Conflict of Interest
The authors declare that the research was conducted in the absence of any commercial or financial relationships that could be construed as a potential conflict of interest.
Acknowledgments
The authors would like to thank Aida Stevenson (University of South Carolina) for picking pteropods from the faunal splits, Paul Callomon (Academy of Natural Sciences of Drexel University) for setting up a fantastic light microscope imaging system, Michelle Gannon (Academy of Natural Sciences of Drexel University) for her thoughtful discussion of the controls on the stable isotopic composition of mollusk shells, and Eric Tappa (University of South Carolina) for his help running the isotopes, and for information on sample processing. The CAR2000-MC-2 core is archived at the University of South Carolina. Thanks to Nina Keul (Kiel University) for an extremely helpful methods discussion before we started our analysis, and to Max Christie (University of Illinois at Urbana-Champaign) for helping us make our R code do what we wanted it to. Thanks to everyone who visited RO poster at AGU 2019 and OSM 2020–your feedback has helped to improve the clarity of this work. Finally, we would like to thank the two reviewers for their constructive reviews which helped improve this manuscript.
Supplementary Material
The Supplementary Material for this article can be found online at: https://www.frontiersin.org/articles/10.3389/fmars.2020.553104/full#supplementary-material
References
Akima, H., and Gebhardt, A. (2016). akima: Interpolation of Irregularly and Regularly Spaced Data. R package version 0.6-2. Available online at: https://CRAN.R-project.org/package=akima (accessed November 5, 2020).
Almogi-Labin, A., Hemleben, C., Meischner, D., and Erlenkeuser, H. (1991). Paleoenvironmental events during the last 13,000 years in the central Red Sea as recorded by pteropoda. Paleoceanography 6, 83–98. doi: 10.1029/90PA01881
Almogi-Labin, A., Luz, B., and Duplessy, J.-C. (1986). Quaternary paleo-oceanography, pteropod preservation and stable-isotope record of the Red Sea. Palaeogeogr. Palaeoclimatol. Palaeoecol. 57, 195–211. doi: 10.1016/0031-0182(86)90013-1
Anderson, T. F., and Arthur, M. (1983). “Stable isotopes of oxygen and carbon and their application to sedimentologic and palaeoenvironmental problems,” in Stable Isotopes in Sedimentary Geology, eds M. A. Arthur, T. F. Anderson, I. R. Kaplan, J. Veizer, and L and, L. S. Land (Tulsa, OK: Society of Economic Paleontologists and Mineralogists Short Course), 1–151. doi: 10.2110/scn.83.01.0000
Astor, Y. M., Fanning, K., Guzman, L., Li, X., Lorenzoni, L., Masserini, R., et al. (2011). Handbook of methods for the analysis of oceanographic parameters at the Cariaco Time-Series Station. Available online at: http://imars.marine.usf.edu/sites/default/files/project/cariaco/publications/CARIACO_Methods_Manual.pdf (accessed November 1, 2020).
Astor, Y. M., Lorenzoni, L., Thunell, R., Varela, R., Muller-Karger, F., and Troccoli, L. (2013). Deep-sea research II interannual variability in sea surface temperature and fCO2 changes in the Cariaco Basin. Deep. Res. II 93, 33–43. doi: 10.1016/j.dsr2.2013.01.002
Bailey, K., Steinberg, C., Davies, C., Galibert, G., Hidas, M., McManus, M. A., et al. (2019). Coastal mooring observing networks and their data products: recommendations for the next decade. Front. Mar. Sci. 6:180. doi: 10.3389/fmars.2019.00180
Bednaršek, N., Feely, R. A., Howes, E. L., Hunt, B. P. V., Kessouri, F., León, P., et al. (2019). Systematic review and meta-analysis toward synthesis of thresholds of ocean acidification impacts on calcifying pteropods and interactions with warming. Front. Mar. Sci. 6:227. doi: 10.3389/fmars.2019.00227
Bednaršek, N., Klinger, T., Harvey, C. J., Weisberg, S., McCabe, R. M., Feely, R. A., et al. (2017). New ocean, new needs: application of pteropod shell dissolution as a biological indicator for marine resource management. Ecol. Indic. 76, 240–244. doi: 10.1016/j.ecolind.2017.01.025
Bednaršek, N., MoŽina, J., Vogt, M., O'Brien, C., and Tarling, G. A. (2012). The global distribution of pteropods and their contribution to carbonate and carbon biomass in the modern ocean. Earth Syst. Sci. Data 5, 167–186. doi: 10.5194/essd-4-167-2012
Bergan, A. J., Lawson, G. L., Maas, A. E., and Wang, Z. A. (2017). The effect of elevated carbon dioxide on the sinking and swimming of the shelled pteropod Limacina retroversa. ICES J. Mar. Sci. 74, 1–13. doi: 10.1093/icesjms/fsx008
Bessat, F., and Buigues, D. (2001). Two centuries of variation in coral growth in a massive Porites colony from Moorea (French Polynesia): a response of ocean-atmosphere variability from south central Pacific. Palaeogeogr. Palaeoclimatol. Palaeoecol. 175, 381–392. doi: 10.1016/S0031-0182(01)00381-9
Beveridge, N. A. S., and Shackleton, N. J. (1994). Carbon isotopes in recent planktonic foraminifera: a record of anthropogenic CO2 invasion of the surface ocean. Earth Planet. Sci. Lett. 126, 259–273. doi: 10.1016/0012-821X(94)90111-2
Black, D., Thunell, R., Wejnert, K., and Astor, Y. (2011). Carbon isotope composition of Caribbean Sea surface waters: response to the uptake of anthropogenic CO2. Geophys. Res. Lett. 38, 1–5. doi: 10.1029/2011GL048538
Black, D. E., Abahazi, M. A., Thunell, R. C., Kaplan, A., Tappa, E. J., and Peterson, L. C. (2007). An 8-century tropical Atlantic SST record from the Cariaco Basin: baseline variability, twentieth-century warming, and Atlantic hurricane frequency. Paleoceanography 22, 1–10. doi: 10.1029/2007PA001427
Black, D. E., Thunell, R. C., and Tappa, E. J. (2001). Planktonic foraminiferal response to the 1997-1998 El Niño: a sediment-trap record from the Santa Barbara Basin. Geology 29, 1075–1078. doi: 10.1130/0091-7613(2001)029<1075:PFRTTE>2.0.CO;2
Böhm, F., Joachimski, M. M., Dullo, W. C., Eisenhauer, A., Lehnert, H., Reitner, J., et al. (2000). Oxygen isotope fractionation in marine aragonite of coralline sponges. Geochim. Cosmochim. Acta 64, 1695–1703. doi: 10.1016/S0016-7037(99)00408-1
Buitenhuis, E. T., Le Quéré, C., Bednaršek, N., and Schiebel, R. (2019). Large contribution of pteropods to shallow CaCO3 export. Global Biogeochem. Cycles 33, 458–468. doi: 10.1029/2018GB006110
Buitenhuis, E. T., Pangerc, T., Franklin, D. J., Le Quéré, C., and Malin, G. (2008). Growth rates of six coccolithophorid strains as a function of temperature. Limnol. Oceanogr. 53, 1181–1185. doi: 10.4319/lo.2008.53.3.1181
Busch, D. S., Maher, M., Thibodeau, P., and McElhany, P. (2014). Shell condition and survival of Puget Sound pteropods are impaired by ocean acidification conditions. PLoS ONE 9:e0105884. doi: 10.1371/journal.pone.0105884
Butler, P. G., Richardson, C. A., Scourse, J. D., Wanamaker, A. D., Shammon, T. M., and Bennell, J. D. (2010). Marine climate in the Irish Sea: analysis of a 489-year marine master chronology derived from growth increments in the shell of the clam Arctica islandica. Quat. Sci. Rev. 29, 1614–1632. doi: 10.1016/j.quascirev.2009.07.010
Butler, P. G., Wanamaker, A. D., Scourse, J. D., Richardson, C. A., and Reynolds, D. J. (2011). Long-term stability of δ13C with respect to biological age in the aragonite shell of mature specimens of the bivalve mollusk Arctica islandica. Palaeogeogr. Palaeoclimatol. Palaeoecol. 302, 21–30. doi: 10.1016/j.palaeo.2010.03.038
Clausen, C. D., and Roth, A. A. (1975). Effect of temperature and temperature adaptation on calcification rate in the hermatypic coral Pocillopora damicornis. Mar. Biol. 33, 93–100. doi: 10.1007/BF00390713
Dadon, J. R., and de Cidre, L. L. (1992). The reproductive cycle of the Thecosomatous pteropod Limacina retroversa in the western South Atlantic. Mar. Biol. 114, 439–442. doi: 10.1007/BF00350035
Davis, A. N., Davis, C. V., Thunell, R. C., Osbourne, E. B., Black, D. E., and Benitez-Nelson, C. R. (2019). Reconstructing 800 Years of carbonate ion concentration in the Cariaco basin using the area density of planktonic foraminifera shells. Paleoceanogr. Paleoclimatol. 34, 2129–2140. doi: 10.1029/2019PA003698
Doi, H., Yurlova, N. I., Kikuchi, E., Shikano, S., Yadrenkina, E. N., Vodyanitskaya, S. N., et al. (2010). Stable isotopes indicate individual level trophic diversity in the freshwater gastropod Lymnaea stagnalis. J. Molluscan Stud. 76, 384–388. doi: 10.1093/mollus/eyq020
Epstein, S., Buchsbaum, R., Lowenstam, H. A., and Urey, H. C. (1953). Revised carbonate-water isotopic temperature scale. Bull. Geol. Soc. Am. 64, 1315–1326. doi: 10.1130/0016-7606(1953)64[1315:RCITS]2.0.CO;2
Fabry, V. J., and Deuser, W. G. (1992). Seasonal changes in the isotopic compositions and sinking fluxes of Euthecosomatous pteropod shells in the Sargasso sea. Paleoceanography 7:195. doi: 10.1029/91PA03138
Fischer, G., Kalberer, M., Donner, B., and Wefer, G. (1999). “Stable isotopes of pteropod shells as recorders of sub-surface water conditions: comparison to the record of G.ruber and to measured values,” in Use of Proxies in Paleoceanography: Examples from the South Atlantic (Berlin Heidelberg: Springer-Verlag), 191–206. doi: 10.1007/978-3-642-58646-0_7
Gannefors, C., Böer, M., Kattner, G., Graeve, M., Eiane, K., Gulliksen, B., et al. (2005). The Arctic sea butterfly Limacina helicina: lipids and life strategy. Mar. Biol. 147, 169–177. doi: 10.1007/s00227-004-1544-y
Ganssen, G. M., Troelstra, S. R., Van Der Borg, K., and De Jong, A. M. F. (1991). Late quaternary pteropod preservation in eastern North Atlantic sediments in relation to changing climate. Radiocarbon 33, 277–282. doi: 10.1017/S0033822200040297
García-Escárzaga, A., Gutiérrez-Zugasti, I., Schöne, B. R., Cobo, A., Martín-Chivelet, J., and González-Morales, M. R. (2019). Growth patterns of the topshell Phorcus lineatus (da Costa, 1778) in northern Iberia deduced from shell sclerochronology. Chem. Geol. 526, 49–61. doi: 10.1016/j.chemgeo.2018.03.017
Gaylord, B., Rivest, E., Hill, T., Sanford, E., Shukla, P., and Ninokawa, A. (2018). California Mussels as Bio- Indicators of the Ecological Consequences of Global Change: Temperature, Ocean Acidification, and Hypoxia. California's Fourth Climate Change Assessment. Davis, CA: California Natural Resources Agency; Bodega Marine Laboratory, University of California, Davis.
Gerhardt, S., and Henrich, R. (2001). Shell preservation of Limacina inflata (Pteropoda) in surface sediments from the Central and South Atlantic Ocean: a new proxy to determine the aragonite saturation state of water masses. Deep. Res. I 48, 2051–2071. doi: 10.1016/S0967-0637(01)00005-X
Gilmer, R. W., and Harbison, G. R. (1986). Morphology and field behavior of pteropod molluscs: feeding methods in the families Cavoliniidae, Limacinidae and Peraclididae (Gastropoda: Thecosomata). Mar. Biol. 91, 47–57. doi: 10.1007/BF00397570
Gilmer, R. W., and Harbison, G. R. (1991). Diet of Limacina helicina (Gastropoda: Thecosomata) in Arctic waters in midsummer. Mar. Ecol. Prog. Ser. 77, 125–134. doi: 10.3354/meps077125
Gorokhova, E., and Hansson, S. (1999). An experimental study on variations in stable carbon and nitrogen isotope fractionation during growth of Mysis mixta and Neomysis integer. Can. J. Fish. Aquat. Sci. 56, 2203–2210. doi: 10.1139/f99-149
Grossman, E. L., Betzer, P. R., Dudley, W. C., and Dunbar, R. B. (1986). Stable isotopic variation in pteropods and atlantids from North Pacific sediment traps. Mar. Micropaleontol. 10, 9–22. doi: 10.1016/0377-8398(86)90022-8
Grossman, E. L., and Ku, T.-L. (1986). Oxygen and carbon isotope fractionation in biogenic aragonite: temperature effects. Chem. Geol. 59, 59–74. doi: 10.1016/0168-9622(86)90057-6
Hansman, R. L., and Sessions, A. L. (2016). Measuring the in situ carbon isotopic composition of distinct marine plankton populations sorted by flow cytometry. Limnol. Oceanogr. Methods 14, 87–99. doi: 10.1002/lom3.10073
Hughen, K. A., Overpeck, J. T., Peterson, L. C., and Anderson, R. F. (1996). The nature of varved sedimentation in the Cariaco Basin, Venezuela, and its paleoclimatic significance. Geol. Soc. Spec. Publ. 116, 171–183. doi: 10.1144/GSL.SP.1996.116.01.15
Hunt, B. P. V., Pakhomov, E. A., Hosie, G. W., Siegel, V., Ward, P., and Bernard, K. (2008). Pteropods in Southern Ocean ecosystems. Prog. Oceanogr. 78, 193–221. doi: 10.1016/j.pocean.2008.06.001
Janssen, A. W. (1990). Pteropoda (Gastropoda, Euthecosomata) from the Australian Cainozoic. Scr. Geol. 91, 1–75.
Janssen, A. W. (2012). Late quaternary to recent holoplanktonic mollusca (Gastropoda) from bottom samples of the eastern Mediterranean sea: systematics, morphology. Boll. Malacol. 48, 1–105.
Janssen, A. W., and Goedert, J. L. (2016). Notes on the systematics, morphology and biostratigraphy of fossil holoplanktonic Mollusca, 24. First observation of a genuinely late Mesozoic thecosomatous pteropod. Basteria 80, 59–63.
Jasper, J. P., and Deuser, W. G. (1993). Annual cycles of mass flux and isotopic composition of pteropod shells settling into the deep Sargasso Sea. Deep Sea Res. I Oceanogr. Res. Pap. 40, 653–669. doi: 10.1016/0967-0637(93)90064-A
Jones, D. S., Arthur, M. A., and Allard, D. J. (1989). Sclerochronological records of temperature and growth from shells of Mercenaria mercenaria from Narragansett Bay, Rhode Island. Mar. Biol. 102, 225–234. doi: 10.1007/BF00428284
Jonkers, L., and Kučera, M. (2015). Global analysis of seasonality in the shell flux of extant planktonic Foraminifera. Biogeosciences 12, 2207–2226. doi: 10.5194/bg-12-2207-2015
Juranek, L. W., Russell, A. D., and Spero, H. J. (2003). Seasonal oxygen and carbon isotope variability in euthecosomatous pteropods from the Sargasso sea. Deep Sea Res. I Oceanogr. Res. Pap. 50, 231–245. doi: 10.1016/S0967-0637(02)00164-4
Kalberer, M., Fischer, G., Pätzold, J., Donner, B., Segl, M., and Wefer, G. (1993). Seasonal sedimentation and stable isotope records of pteropods off Cap Blanc. Mar. Geol. 113, 305–320. doi: 10.1016/0025-3227(93)90024-P
Katz, M. E., Cramer, B. S., Franzese, A., Hönisch, B., Miller, K. G., Rosenthal, Y., et al. (2010). Traditional and emerging geochemical proxies in foraminifera. J. Foraminifer. Res. 40, 165–192. doi: 10.2113/gsjfr.40.2.165
Keeling, C. D. (1979). The suess effect: 13Carbon-14Carbon interrelations. Environ. Int. 2, 229–300. doi: 10.1016/0160-4120(79)90005-9
Kennish, M. J., and Olsson, R. K. (1975). Effects of thermal discharges on the microstructural growth of Mercenaria mercenaria. Environ. Geol. 1, 41–64. doi: 10.1007/BF02426940
Keul, N., Peijnenburg, K. T. C. A., Andersen, N., Kitidis, V., Goetze, E., and Schneider, R. R. (2017). Pteropods are excellent recorders of surface temperature and carbonate ion concentration. Sci. Rep. 7, 1–11. doi: 10.1038/s41598-017-11708-w
Kucera, M. (2007). “Chapter six planktonic foraminifera as tracers of past oceanic environments,” in Developments in Marine Geology, eds C. Hillaire–Marcel, A. De Vernal (New York, NY: Elsevier), 213–262. doi: 10.1016/S1572-5480(07)01011-1
Lalli, M., and Wells, F. E. (1978). Reproduction in the genus Limacina (Opistobranchia: Thecosomata). J. Zool. 186, 95–108. doi: 10.1111/j.1469-7998.1978.tb03359.x
LeGrande, A. N., and Schmidt, G. A. (2006). Global gridded data set of the oxygen isotopic composition in seawater. Geophys. Res. Lett. 33, 1–5. doi: 10.1029/2006GL026011
León, P., Bednaršek, N., Walsham, P., Cook, K., Hartman, S. E., Wall-Palmer, D., et al. (2019). Relationship between shell integrity of pelagic gastropods and carbonate chemistry parameters at a Scottish coastal observatory monitoring site. ICES J. Mar. Sci. 77, 436–450. doi: 10.1093/icesjms/fsz178
Lischka, S., Büdenbender, J., Boxhammer, T., and Riebesell, U. (2011). Impact of ocean acidification and elevated temperatures on early juveniles of the polar shelled pteropod Limacina helicina: mortality, shell degradation, and shell growth. Biogeosciences 8, 919–932. doi: 10.5194/bg-8-919-2011
Lorrain, A., Paulet, Y. M., Chauvaud, L., Dunbar, R., Mucciarone, D., and Fontugne, M. (2004). δ13C variation in scallop shells: increasing metabolic carbon contribution with body size? Geochim. Cosmochim. Acta 68, 3509–3519. doi: 10.1016/j.gca.2004.01.025
Mancuso, A., Stagioni, M., Prada, F., Scarponi, D., Piccinetti, C., and Goffredo, S. (2019). Environmental influence on calcification of the bivalve Chamelea gallina along a latitudinal gradient in the Adriatic sea. Sci. Rep. 9, 1–11. doi: 10.1038/s41598-019-47538-1
Manno, C., Rumolo, P., Barra, M., D'Albero, S., Basilone, G., Genovese, S., et al. (2019). Condition of pteropod shells near a volcanic CO2 vent region. Mar. Environ. Res. 143, 39–48. doi: 10.1016/j.marenvres.2018.11.003
Marshall, B. J., Thunell, R. C., Henehan, M. J., Astor, Y., and Wejnert, K. E. (2013). Planktonic foraminiferal area density as a proxy for carbonate ion concentration: a calibration study using the Cariaco basin ocean time series. Paleoceanography 28, 363–376. doi: 10.1002/palo.20034
Marshall, B. J., Thunell, R. C., Spero, H. J., Henehan, M. J., Lorenzoni, L., and Astor, Y. (2015). Morphometric and stable isotopic differentiation of Orbulina universa morphotypes from the Cariaco Basin, Venezuela. Mar. Micropaleontol. 120, 46–64. doi: 10.1016/j.marmicro.2015.08.001
Marshall, D. J., Abdelhady, A. A., Wah, D. T. T., Mustapha, N., Gödeke, S. H., De Silva, L. C., et al. (2019). Biomonitoring acidification using marine gastropods. Sci. Total Environ. 692, 833–843. doi: 10.1016/j.scitotenv.2019.07.041
McConnaughey, T. (1989). 13C and 18O isotopic disequilibrium in biological carbonates: I. Patterns. Geochim. Cosmochim. Acta 53, 151–162. doi: 10.1016/0016-7037(89)90282-2
McConnaughey, T. A., Burdett, J., Whelan, J. F., and Paull, C. K. (1997). Carbon isotopes in biological carbonates: Respiration and photosynthesis. Geochim. Cosmochim. Acta 61, 61l−622. doi: 10.1016/S0016-7037(96)00361-4
McConnaughey, T. A., and Gillikin, D. P. (2008). Carbon isotopes in mollusk shell carbonates. Geo-Marine Lett. 28, 287–299. doi: 10.1007/s00367-008-0116-4
McConnell, M. C., Thunell, R. C., Lorenzoni, L., Astor, Y., Wright, J. D., and Fairbanks, R. (2009). Seasonal variability in the salinity and oxygen isotopic composition of seawater from the Cariaco Basin, Venezuela: implications for paleosalinity reconstructions. Geochem. Geophys. Geosyst. 10:Q06019. doi: 10.1029/2008GC002035
Mekik, F. (2014). Radiocarbon dating of planktonic foraminifer shells: a cautionary tale. Paleoceanography 29, 13–29. doi: 10.1002/2013PA002532
Mohan, R., Verma, K., Mergulhao, L. P., Sinha, D. K., Shanvas, S., and Guptha, M. V. S. (2006). Seasonal variation of pteropods from the Western Arabian sea sediment trap. Geo-Marine Lett. 26, 265–273. doi: 10.1007/s00367-006-0035-1
Mucci, A. (1983). The solubility of calcite and aragonite in seawater at various salinities, temperatures, and one atmosphere total pressure. Am. J. Sci. 283, 780–799. doi: 10.2475/ajs.283.7.780
Muller-Karger, F. E., Astor, Y. M., Benitez-Nelson, C. R., Buck, K. N., Fanning, K. A., Lorenzoni, L., et al. (2019). The scientific legacy of the CARIACO ocean time-series program. Ann. Rev. Mar. Sci. 11:annurev-marine-010318-095150. doi: 10.1146/annurev-marine-010318-095150
Oakes, R. L., and Sessa, J. A. (2020). Determining how biotic and abiotic variables affect the shell condition and parameters of Heliconoides inflatus pteropods from a sediment trap in the Cariaco basin. Biogeosciences 17, 1975–1990. doi: 10.5194/bg-17-1975-2020
Owen, R., Kennedy, H., and Richardson, C. (2002). Isotopic partitioning between scallop calcite and seawater: effect of shell growth rate. Geochim. Cosmochim. Acta 66, 1727–1737. doi: 10.1016/S0016-7037(01)00882-1
Parker, L. M., Ross, P. M., O'Connor, W. A., Pörtner, H. O., Scanes, E., and Wright, J. M. (2013). Predicting the response of molluscs to the impact of ocean acidification. Biology 2, 651–692. doi: 10.3390/biology2020651
Pearson, P. N. (2012). Oxygen isotopes in foraminifera: overview and historical review. Paleontol. Soc. Pap. 18, 1–38. doi: 10.1017/S1089332600002539
Peterson, L. C., Overpeck, J. T., Kipp, N. G., and Imbrie, J. (1991). A high-resolution Late Quaternary upwelling record from the anoxic Cariaco Basin, Venezuela. Paleoceanography 6, 99–119. doi: 10.1029/90PA02497
Price, B. A., Killingley, J. S., and Berger, W. H. (1985). On the pteropod pavement of the eastern Rio Grande Rise. Mar. Geol. 64, 217–235. doi: 10.1016/0025-3227(85)90106-9
Ravelo, A. C., and Fairbanks, R. G. (1992). Oxygen isotopic composition of multiple species of planktonic foraminifera: recorders of the modern photic zone temperature gradient. Paleoceanography 7, 815–831. doi: 10.1029/92PA02092
R. Core Team (2019). R: A language and environment for statistical computing. Vienna: R Foundation for Statistical Computing. Available online at: https://www.R-project.org/ (accessed November 5, 2020).
RStudio Team (2016). RStudio: Integrated Development for R. RStudio, Inc. Boston, MA. Available online at: http://www.rstudio.com/ (accessed November 5, 2020).
Schneider, C. A., Rasband, W. S., and Eliceiri, K. W. (2012). NIH Image to ImageJ: 25 years of image analysis. Nat. Methods 9, 671–675. doi: 10.1038/nmeth.2089
Schöne, B., and Surge, D. M. (2012). Treatise online no. 46: part N, revised, volume 1, chapter 14: bivalve sclerochronology and geochemistry. Treatise Online 0, 1–24. doi: 10.17161/to.v0i0.4297
Schöne, B. R., Houk, S. D., Castro, A. D. F., Fiebig, J., Oschmann, W., Kröncke, I., et al. (2005). Daily growth rates in shells of Arctica islandica: assessing sub-seasonal environmental controls on a long-lived bivalve mollusk. Palaios 20, 78–92. doi: 10.2110/palo.2003.p03-101
Steinhardt, J., Butler, P. G., Carroll, M. L., and Hartley, J. (2016). The application of long-lived bivalve sclerochronology in environmental baseline monitoring. Front. Marine Sci. 3:176. doi: 10.3389/fmars.2016.00176
Stuiver, M., and Polach, H. (1977). Discussion of 14C data. Radiocarbon 19, 355–363. doi: 10.1017/S0033822200003672
Suess, H. E. (1955). Radiocarbon concentration in modern wood. Science. 122, 415–417. doi: 10.1126/science.122.3166.415-a
Swart, P. K., Greer, L., Rosenheim, B. E., Moses, C. S., Waite, A. J., Winter, A., et al. (2010). The 13C suess effect in scleractinian corals mirror changes in the anthropogenic CO2 inventory of the surface oceans. Geophys. Res. Lett. 37, 1–5. doi: 10.1029/2009GL041397
Tanaka, N., Monaghan, M. C., and Rye, D. M. (1986). Contribution of metabolic carbon to mollusc and barnacle shell carbonate. Nature 320, 520–523. doi: 10.1038/320520a0
Tedesco, K., Thunell, R., Astor, Y., and Muller-Karger, F. (2007). The oxygen isotope composition of planktonic foraminifera from the Cariaco Basin, Venezuela: seasonal and interannual variations. Mar. Micropaleontol. 62, 180–193. doi: 10.1016/j.marmicro.2006.08.002
Tedesco, K. A., and Thunell, R. C. (2003). Seasonal and interannual variations in planktonic foraminiferal flux and assemblage composition in the Cariaco Basin, Venezuela. J. Foraminifer. Res. 33, 192–210. doi: 10.2113/33.3.192
Thibodeau, P. S., and Steinberg, D. K. (2018). Environmental controls on pteropod biogeography along the Western Antarctic Peninsula. Limnol. Oceanogr. 00, 1–17. doi: 10.1002/lno.11041
Thunell, R. C., Varela, R., Llano, M., Collister, J., Muller-Karger, F., and Bohrer, R. (2000). Organic carbon fluxes, degradation, and accumulation in an anoxic basin: sediment trap results from the Cariaco Basin. Limnol. Oceanogr. 45, 300–308. doi: 10.4319/lo.2000.45.2.0300
Tiselius, P., and Fransson, K. (2016). Daily changes in d15N and d13C stable isotopes in copepods: equilibrium dynamics and variations of trophic level in the field. J. Plankton Res. 38, 751–761. doi: 10.1093/plankt/fbv048
Uhle, M. E., Macko, S. A., Spero, H. J., Engel, M. H., and Lea, D. W. (1997). Sources of carbon and nitrogen in modern planktonic foraminifera: the role of algal symbionts as determined by bulk and compound specific stable isotopic analyses. Org. Geochem. 27, 103–113. doi: 10.1016/S0146-6380(97)00075-2
Urey, H. C., Lowenstam, H., a., Epstein, S., and McKinney, C. R. (1951). Measurement of Paleotemperatures and temperatures and the Southeastern United States. Bull. Geol. Soc. Am. 62, 399–416. doi: 10.1130/0016-7606(1951)62[399:MOPATO]2.0.CO;2
Wang, K., Hunt, B. P. V., Liang, C., Pauly, D., and Pakhomov, E. A. (2017). Reassessment of the life cycle of the pteropod Limacina helicina from a high resolution interannual time series in the temperate North Pacific. ICES J. Mar. Sci. 74, 1906–1920. doi: 10.1093/icesjms/fsx014
Wefer, G., and Berger, W. H. (1991). Isotope paleontology: growth and composition of extant calcareous species. Mar. Geol. 100, 207–248. doi: 10.1016/0025-3227(91)90234-U
Wejnert, K., Thunell, R. C., and Astor, Y. M. (2013). Comparison of species-specific oxygen isotope paleotemperature equations: sensitivity analysis using planktonic foramifera from the Cariaco Basin, Venezuela. Marine Micropaleontol. 101, 76–88. doi: 10.1016/j.marmicro.2013.03.001
Wells, F. E. (1976). Growth rate of four species of euthecosomatous pteropods occurring off Barbados, West Indies. Nautlius 90, 114–116.
Wormelle, R. (1962). A survey of the standing crop of plankton of the Florida Current. VI. A study of the distribution of the pteropods of the Florida current. Bull. Mar. Sci. Gulf Caribb. 12, 95–136. Available online at: http://www.ingentaconnect.com/content/umrsmas/bullmar/1962/00000012/00000001/art00006 (accessed December 21, 2020).
Keywords: pteropoda, stable isotopes, calcification, plankton, CARIACO, proxy
Citation: Oakes RL, Davis CV and Sessa JA (2021) Using the Stable Isotopic Composition of Heliconoides inflatus Pteropod Shells to Determine Calcification Depth in the Cariaco Basin. Front. Mar. Sci. 7:553104. doi: 10.3389/fmars.2020.553104
Received: 17 April 2020; Accepted: 08 December 2020;
Published: 14 January 2021.
Edited by:
Gordon T. Taylor, Stony Brook University, United StatesReviewed by:
Nina Keul, University of Kiel, GermanyMichaël Hermoso, UMR8187 Laboratoire d'océanologie et de géosciences (LOG), France
Copyright © 2021 Oakes, Davis and Sessa. This is an open-access article distributed under the terms of the Creative Commons Attribution License (CC BY). The use, distribution or reproduction in other forums is permitted, provided the original author(s) and the copyright owner(s) are credited and that the original publication in this journal is cited, in accordance with accepted academic practice. No use, distribution or reproduction is permitted which does not comply with these terms.
*Correspondence: Rosie L. Oakes, cm9zaWUubC5vYWtlc0BnbWFpbC5jb20=
†Present Address: Rosie L. Oakes, Met Office, Exeter, United Kingdom
Catherine V. Davis, Department of Earth and Planetary Sciences, Yale University, New Haven, CT, United States