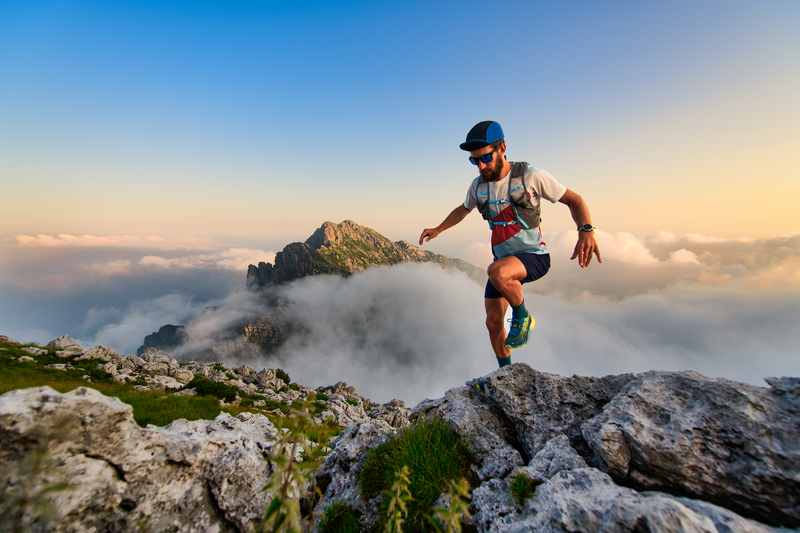
95% of researchers rate our articles as excellent or good
Learn more about the work of our research integrity team to safeguard the quality of each article we publish.
Find out more
ORIGINAL RESEARCH article
Front. Mar. Sci. , 13 November 2020
Sec. Aquatic Physiology
Volume 7 - 2020 | https://doi.org/10.3389/fmars.2020.544582
Chinese coastal waters are subject to eutrophication because of rapid economic development and population growth. To study the effects of nutrient enrichment on the growth and quality of Pyropia haitanensis, the thalli of a red-brown (Z-61) strain and a green (Z-26) strain of P. haitanensis were cultured semi-continuously for 15 days in four media with different nitrogen and phosphorus concentrations. High-nutrient conditions had no significant effect on the growth rate of Z-26 and Z-61. Higher concentrations of nutrients increased the chlorophyll a content of Z-61 and Z-26 by 116.7 and 34.2%, respectively. High concentrations of nutrients also significantly increased the average phycoerythrin and phycocyanin contents by 57.4 and 56.0%, respectively (strain Z-61); and by 37.7 and 44.7%, respectively (strain Z-26). As the nitrogen and phosphorus concentrations increased, the mean contents of total protein, essential amino acids, and flavor amino acids increased by 130.0, 102.5, and 341%, respectively, for strain Z-61, and by 25.1, 23.9, and 497%, respectively, for strain Z-26. Under high-nutrient conditions, the mean total amino acids content was 40.7% higher in Z-26 than in Z-61; the total flavor amino acids content was 117% higher in Z-61 than in Z-26; but there was no significant difference in the total essential amino acids content between the two strains. Interestingly, under low-nutrient conditions, Z-26 had a higher nutritional value than Z-61, but Z-61 tasted better than Z-26. Based on the results of these laboratory experiments, nutrient enrichment might enhance the market value of P. haitanensis by significantly improving its food quality.
Although marine macroalgae are restricted to a narrow area of the ocean, they contribute approximately 10% of the ocean’s primary productivity. Thus, they play an important role in the biological carbon pump and in sustaining marine aquaculture ecosystems (Israel et al., 2010). In addition to their ecological value, macroalgae are used to produce medicines, food, cosmetics, biofuels, and other products. At present, there are approximately 100 economic macroalgal species (Gao et al., 2018). Pyropia, formerly known as Porphyra, is not only rich in protein, carbohydrates, amino acids and vitamins, but also contains many minerals. Among the seaweeds cultivated on a large scale, Pyropia has the highest nutritional value. The history of Pyropia consumption in Asia goes back approximately 1,000 years. The thalli of Pyropia are used in soups, sushi, and other dishes (Mouritsen et al., 2018). Thus, Pyropia is the most valuable seaweed in the world and has been an important marine crop in east and southeast Asia for thousands of years (Wells et al., 2017; He et al., 2018). The total value of Pyropia in 2017 was approximately $2 billion (FAO, 2019). Two kinds of Pyropia species, i.e., Pyropia yezoensis and Pyropia haitanensis, are widely cultivated in China. P. haitanensis is traditionally cultivated in south China and has been transplanted to north China in recent years. The production of Pyropia reached ∼200,000 tons (dry weight) in 2018, and the production of P. haitanensis in Fujian Province reached ∼74,000 tons (dry weight) in 2018, accounting for 37% of the total Pyropia yield in China (Xu et al., 2019). In addition to extensive domestic sales, Pyropia products are also exported to southeast Asia and other countries around the world (He et al., 2018).
The color, flavor, and nutritional value are very significant characteristics in determining the food quality, and thus, the market potential of P. haitanensis (He et al., 2018). The contents of flavor amino acids (FAA), including sweet amino acids (SAA) and umami amino acids (UAA) determine the taste of Pyropia, so the FAA content is an important index of food quality (Nishimura and Kato, 1988). High-quality fresh thalli are characterized by a deep red color, which in P. haitanensis is conferred by photosynthetic pigments including chlorophyll, phycoerythrin (PE), phycocyanin (PC), and allophycocyanin (APC) (He et al., 2018). Dried sheets of P. haitanensis with a deeper color and better taste usually have a higher market value. The food value of P. haitanensis lies in its high nutritional content, including large amounts of proteins, vitamins, carbohydrates, minerals, dietary fiber, and especially amino acids, because they are involved in a wide range of physiological and metabolic activities (Noda, 1993). Further, the proportions of essential amino acids (EAA) that cannot be synthesized by humans and must be obtained from food to maintain human health are very similar between P. haitanensis and the amino acid reference pattern of the Food and Agriculture Organization (FAO) (Zeng et al., 1991).
It is predicted that, due to the development of the coastal economy, total nitrogen and phosphorus inputs will increase by 30–200% in the coastal seas of China between 2,000 and 2,050 (Strokal et al., 2014). Consistent with this prediction, a recent study that showed that the nutrient loading from the eight major rivers of China into the coastal waters strongly increased from 2006 to 2012 (Tong et al., 2015). In general, the nutrient content in offshore waters is low and is maintained at a stable level (relative to that in coastal waters) by physical, chemical, and biological processes. In coastal waters, approximately two-thirds of dissolved inorganic phosphorus and dissolved inorganic nitrogen is originated from anthropogenic sources, such as riverine sources, atmospheric deposition, agriculture, and fossil fuel combustion (Dentener et al., 2006). Enrichment of the growth medium with nutrients such as inorganic nitrogen and phosphorus yields varying results with regard to changes in algal productivity and growth (Lobban and Harrison, 2004; Glibert, 2017). In general, high concentrations of nitrogen and phosphorus promote the growth of both microalgae and macroalgae. Thus, the overabundance of nutrients in water can have many harmful effects on human health and the environment, especially through the development of harmful algal blooms (Heisler et al., 2008). The negative effects of eutrophication on marine ecosystems have been extensively studied and have attracted attention worldwide (Conley et al., 2009). However, eutrophication is a continuing problem that will likely worsen in the future.
Seaweed aquaculture has the potential to relieve eutrophication. Culturing economic seaweeds in eutrophic zones could help to inhibit the formation of harmful algal blooms and balance the coastal ecosystem (Yang et al., 2015; He et al., 2018). Thus, the large-scale cultivation of P. haitanensis could not only supply food for human consumption, but may also improve the coastal environment by reducing eutrophication. To date, however, little is known about the comprehensive influence of nutrient enrichment on the growth and food quality of P. haitanensis. In this study, we cultured two strains of P. haitanensis in media with four different concentrations of nitrogen and phosphorus and investigated its physiological performance and food quality. The results of this study provide insights into how predicted nutrient enrichment in the future will affect the quality and potential use of P. haitanensis for food.
The conchocelis phases of a red-brown strain (Z-61) and a green strain (Z-26) of P. haitanensis were obtained from the seaweed germplasm bank in Fujian Province, China. Both strains were grown in enriched seawater medium (Provasoli’s enrichment solution; PES) (Starr and Zeikus, 1993), which contains sodium nitrate, disodium hydrogen phosphate dodecahydrate, and trace elements (iron, zinc, manganese, cobalt). The conchocelis filaments were ripened at 29°C under light intensity of 10–20 μmol/(m2⋅s) and an 8-h light:16-h dark photoperiod. Then, the conchocelis filaments were transferred to seawater medium at 21°C and aerated to promote the release of conchospores that developed into thalli.
The thalli were grown in semi-continuous culture using natural seawater with vitamins and trace metals added according to the protocol for PES medium, except for the NO3– and PO43– concentrations. The nitrogen:phosphorus molar concentration ratio of the four media (designated as N0P0, N1P1, N2P2 and N3P3) was adjusted to about 16:1. The NO3– and PO43– concentrations were adjusted by adding NaNO3 and NaH2PO4. The NO3– and PO43– concentrations in natural seawater in this study were 0.61 mg/L and 0.09 mg/L, respectively. The medium without added NO3– and PO43– was designated as N0P0. The media with added NO3– and PO43– were designated as N1P1, N2P2, and N3P3, respectively. The measured NO3– and PO43– concentrations were 1.81 and 0.19 mg/L in N1P1; 5.29 and 0.65 mg/L in N2P2, and 13.16 and 1.34 mg/L in N3P3 (Table 1).
To avoid the formation of precipitates, the media were only boiled for 1 min, and were then cooled to growth temperature before use. The cultures were aerated with filter-sterilized air and the culture medium was replaced every 3 days. The growth temperature was 21°C, the light intensity was 50–60 μmol/(m2⋅s), and the photoperiod was 12 h light:12 h dark. The culture experiments were carried out at approximately 35 days after the conchospores developed into thalli. The thalli with a length of ∼1 cm (with a fresh weight of ∼0.0003 g) were selected for the 15-day growth experiments. Three thalli were randomly chosen and placed in a 1-L flask, and four replicates were prepared for each treatment.
The length and fresh weight (FW) of thalli were measured to estimate growth. The final length and weight of the blades was measured to avoid any interference during incubation. Thalli were taken from the culture flasks and placed in a sterilized tray with a length scale, and the length was measured from the base to the tip (Li and Yan, 2015) after gently straightening the blade using two tweezers. Eight layers of absorbent gauze were used to remove the surface water from the blades and then FW was determined using an analytical balance. The fresh thalli were then dried in an oven at 50°C to constant weight, which was recorded as dry weight (DW), and the moisture content (MC) was calculated from the DW. The specific growth rate was calculated according to the following formula: SGR (d–1) = ln (Lf/L0)/15, where L0 and Lf refer to the initial and final length (or FW) after 15 days of culture, respectively.
Chlorophyll a (Chl a) was extracted from ∼0.05 g (FW) sample in 90% acetone at 4°C. After extraction in the dark for 24 h, the samples were centrifuged (5,000 × g, 4°C, 20 min) and the optical density of the supernatant was scanned from 400 to 800 nm with a spectrophotometer (UV-1800, Shimadzu, Japan). The Chl a concentration (CChl α; mg/g DW) was calculated based on Jensen (1978), as follows: CChl α = (A666 – A730) (10 × V)/890 × [FW(1-MC)], where A666 and A730 represent the absorbance at 666 and 730 nm, respectively.
To determine the contents of the three protein pigments (PE, PC, APC) approximately 0.01 g DW of thalli was thoroughly homogenized in 50 mL sodium phosphate buffer (0.05 mol/L, pH 6.8) at 4°C. After at least six rounds of freeze-thawing (-21°C to atmospheric temperature), the extracts were kept in the dark at 4°C for 12 h. The extracts were then centrifuged at 5,000 × g for 20 min at 4°C. The concentrations of phycobilisome (PBS) pigments were calculated from absorbance values according to method of Gao (1993) with slight modifications as described by Wang et al. (2019).
For total protein extraction, dried samples of thalli from the four different treatments were ground at 4°C with a mortar and pestle, and then lysed with NP40 lysis buffer (Solarbio, Beijing, China) containing 1% w/v protease inhibitor and 1% w/v phosphatase inhibitor. The mixture was then centrifuged at 12,000 × g for 15 min. The supernatant was collected and the total protein concentration was detected using a BCA Protein Assay Kit (Solarbio, Beijing, China). The amino acids and free amino acids were analyzed by the Qingdao Sci-tech Innovation Quality Testing Co., Ltd. using a high-performance liquid chromatograph (Agilent 1260 Series, San Francisco, California, USA) in accordance with the National Standard of the P. R. China (GB/T 30987-2014). The dried tissue was ground in liquid nitrogen with a mortar and pestle for about 3 min to obtain a fine powder. After hydrolysis of 100 mg dried thalli powder with 20 mL 6 mol/L HCl at 110°C for 24 h, the sample was cooled and then transferred to a 25 mL colorimetric tube. Then, 1 mL of this solution was dried in a water bath at 85°C and then re-dissolved in 1 mL deionized water. After adding 10 mL 0.02 mol/L HCl and shaking the mixture, 250 μL phenyl isothiocyanate acetonitrile (0.1 mol/L) and 250 μL 0.1 mol/L triethylamine acetonitrile were added to 500 μL sample solution and mixed. The solution was derivatized with 40 μL diethyl ethoxymethylenemalonate for 1 h. Samples were transferred to vials and 10 μL was injected into the HPLC. Chromatographic separations were performed on a SHISEIDO HP-C18 column (250 × 4.6 mm, 5 μm). The mobile phase consisted of solvent A (sodium acetate + acetonitrile, pH = 6.5) and solvent B (acetonitrile + water). The contents of amino acids in the mixture were quantified by comparison with standard peak values, and then used to calculate the concentration (g/kg DW) in the algal tissue.
Results are expressed as mean ± SD. Data were statistically analyzed by one-way analysis of variance using least significant difference at a significance level of 0.05. All statistical analyses were conducted with SPSS 19.0 (Chicago, IL, United States).
After 15 days of culture under the four different nutrient conditions, the length and FW of both Z-61 and Z-26 were significantly higher than the initial values (P < 0.05). The final lengths of Z-61 and Z-26 were 39.71–54.38 cm and 23.12–24.18 cm, respectively, while the FWs were 0.18–0.27 g and 0.11–0.14 g, respectively (Figures 1A,B). The blade length of Z-61 was not significantly different between N3P3 and N0P0 (Figure 1C; P > 0.05). The growth of Z-26 was similar in all treatments (Figure 1D, P > 0.05). The length and FW of Z-61 were greater than those of Z-26 in all treatments (Figures 1A,B). For example, in N3P3, the length of Z-61 was 33.0% higher than that of Z-26, and the FW of Z-61 was 46.9% higher than that of Z-26 (P < 0.05).
Figure 1. Length (black bars) and fresh weight (gray bars) of Z-61 (A) and Z-26 (B) thalli after 15 days of incubation. Specific growth rate of Z-61 (C) and Z-26 (D) cultured under different nutrient contents. From N0P0 to N3P3, the NO3– contents were 0.61, 1.81, 5.29, and 13.16 mg/L, while the PO43– contents were 0.09, 0.19, 0.65 and 1.34 mg/L, respectively. Values are means ± SD (n = 4). Different letters represent significant differences (P < 0.05) among treatments.
The Chl α contents of P. haitanensis Z-61 and Z-26 ranged from 3.30 to 7.15 mg⋅g–1 DW and 6.27 to 9.00 mg⋅g–1 DW, respectively (Figure 2). In general, the Chl α contents of both strains increased significantly with increasing nutrient concentrations in the medium (P < 0.05). With increasing nutrient contents from N0P0 to N3P3, the Chl α contents of Z-61 and Z-26 increased significantly by 116.7 and 34.2% (P < 0.05), respectively. In addition, the Chl α contents of Z-26 were significantly higher than that of Z-61 in the N0P0, N1P1, and N2P2 treatments (Figure 2; P < 0.05).
Figure 2. Chlorophyll a (Chl a) content of Z-61 (black bars) and Z-26 (gray bars) cultured under four different nutrient conditions. From N0P0 to N3P3, the NO3– contents were 0.61, 1.81, 5.29, and 13.16 mg/L, while the PO43– contents were 0.09, 0.19, 0.65 and 1.34 mg/L, respectively. Different letters represent significant differences (P < 0.05) among treatments (lowercase for Z-26 and capitalized for Z-61). * indicates significant difference between Z-61 and Z-26. Values are means ± SD (n = 4). DW: dry weight.
Compared with N0P0, the N3P3 treatment significantly increased the PE contents of Z-61 and Z-26 by 57.4 and 37.7%, respectively, and increased the PC contents by 56.0 and 44%, respectively (Figure 3; P < 0.05). However, the APC content of Z-61 was not significantly affected by the nutrient content in the medium (P > 0.05). The APC content of Z-26 was significantly increased by 66.0% (P < 0.05) as the nutrient concentrations increased from N0P0 to N3P3. Except in the N3P3 treatment, the PC content was higher in Z-26 than in Z-61 (P < 0.05).
Figure 3. Contents of phycoerythrin (PE), phycocyanin (PC) and allophycocyanin (APC) in thalli of Z-61 (A) and Z-26 (B) cultured under four different nutrient levels. From N0P0 to N3P3, the NO3– contents were 0.61, 1.81, 5.29, and 13.16 mg/L, while the PO43– contents were 0.09, 0.19, 0.65, and 1.34 mg/L, respectively. Values are means ± SD (n = 4). DW: dry weight.
The total protein contents of Z-61 and Z-26 ranged from 15.9 to 36.6% and from 31.8 to 39.8%, respectively (Figure 4). Compared with N0P0, the N3P3 treatment significantly increased the total protein contents of Z-61 and Z-26 by 130.0 and 25.1% (P < 0.05), respectively. In N0P0, the total protein content of Z-26 was about double that of Z-61 (P < 0.05). We detected significant positive linear relationships between protein content and nutrient contents in the culture media. The protein contents in Z-61 and Z-26 increased significantly with increasing nitrogen or phosphorus concentrations in the growth media (Z-61: R2 = 0.58, Z-26: R2 = 0.64/0.68 P < 0.05, Figures 5A,B).
Figure 4. Total protein contents in thalli of Z-61 (A) and Z-26 (B) cultured at different nutrient levels. From N0P0 to N3P3, the NO3– contents were 0.61, 1.81, 5.29, and 13.16 mg/L, while the PO43– contents were 0.09, 0.19, 0.65, and 1.34 mg/L, respectively. Values are means ± SD (n = 4). Different letters represent significant differences (P < 0.05) among treatments. DW: dry weight.
Figure 5. Linear relationships between protein content in thalli and nitrogen (A) or phosphorus (B) concentrations in different culture media (blue dotted line, Z-61; red dotted line, Z-26).
The amino acid and free amino acids contents of P. haitanensis cultured under four different nutrient conditions are shown in Tables 2, 3, respectively. Regardless of the nutrient conditions, the most abundant amino acids in both strains were glutamic acid, proline, alanine, arginine, and aspartic acid. Culture under high-nutrient conditions increased the contents of almost all amino acids and thus increased the contents of total amino acids (TAA), total free amino acids (TFAA), non-essential amino acids (NEAA), and EAA. The P. haitanensis thalli contained seven EAA. From N0P0 to N3P3, the contents of total essential amino acids (TEAA) of Z-61 and Z-26 increased significantly by 102.5 and 23.9% (P < 0.05), respectively. Compared with N0P0, the N3P3 treatment significantly increased the total UAA (glutamic acid, aspartic acid) and total SAA (serine, glycine, alanine and proline) contents of Z-61 by 500 and 466% (P < 0.05), respectively, and those of Z-26 by 537% and 1102%, respectively (P < 0.05). In the N3P3 treatment, the TAA content was 40.7% higher in Z-26 than in Z-61; the TFAA content was 117% higher in Z-61 than in Z-26 (P < 0.05); and the TEAA content did not differ significantly between the two strains (P > 0.05). All samples were rich in EAA. In all treatments, the ratio of TEAA to total NEAA was greater than 0.3. The ratio of TEAA to total TAA was higher than 0.3 in Z-61 and higher than 0.2 in Z-26. The proportions of EAA were close to those of the FAO amino acid reference pattern.
Table 2. Amino acid contents (g⋅kg–1 dry weight) in thalli of Z-61 and Z-26 cultured under different nutrient levels.
Table 3. Free amino acid contents (g⋅kg–1 dry weight) in thalli of Z-61 and Z-26 cultured under different nutrient levels.
Nitrogen is an essential element that is required in large amounts for the growth of marine algae. This element plays a central role in plant metabolism as it is a constituent of proteins, chlorophylls, nucleic acids, phytohormones, coenzymes, and secondary metabolites (Taiz and Zeiger, 2010). Changes in nutrient concentrations in seawater are known to affect algal growth (Pedersen and Borum, 1996; Lobban and Harrison, 2004). In general, increasing nitrogen and phosphorus concentrations increase the growth of macroalgae, but other environmental factors can also influence algal growth. However, at least at the values tested in our experiments, increased nutrient concentrations had no significant influence on the SGR of Z-26 and Z-61 (Figure 1). In practice, farmers prefer to cultivate P. haitanensis in areas with a high seawater flow rate because of the potential for increased nutrient inputs (He et al., 2018). In the case of low nutrient supply in this study, aeration of the cultures ensured the even distribution of nitrogen and phosphorus in the culture medium, and the nitrogen and phosphorus contents were sufficient to maintain the growth rate with medium renewed every 3 days.
Organisms, including macroalgae, can alter their metabolism under nutrient-limited conditions. For example, many plants convert fixed carbon into carbon-rich storage products (carbohydrates, polysaccharides) under nutrient-deficient conditions (Taiz and Zeiger, 2010). Diatoms were shown to switch from protein synthesis to carbohydrate synthesis under nutrient-limited conditions (Fernández et al., 1992). Similarly, macroalgae have been found to accumulate storage polysaccharides under nitrogen-limited conditions, and then resume protein and pigment synthesis under nitrogen-sufficient conditions (Chopin et al., 1995; Smit et al., 1996). In this study, less protein accumulated in the N0P0 treatment than in the treatments with higher nutrient concentrations. This may represent a strategy to save energy and resources for the synthesis of carbohydrates under low-nutrient conditions.
As a key component of photosynthesis, chlorophyll is involved in light harvesting and is thus related to the growth and yield of photoautotrophs (Taiz and Zeiger, 2010). Nitrogen is a component of Chl a, so nitrogen deficiency can reduce the chlorophyll content in plants. Although phosphorus is not a constituent of Chl a, higher phosphorus supply can stimulate the activity of enzymes involved in Chl a synthesis (Von Wettstein et al., 1995). Similar to higher plants, two Ulva species (Ulva rotundata and Ulva curvata) showed positive correlations between Chl a contents and nitrogen contents in the tissue (Vergara et al., 1997). In our study, we also observed that nutrient enrichment had a positive effect on Chl a synthesis (Figure 2).
We detected a positive correlation between PBP contents and nutrient concentrations in this study. This is consistent with a previous study in which the PE contents of two species of intertidal red macroalgae were found to be closely related to the nitrogen content in seawater (Hiroyuki, 2002). These findings suggest that nitrogen supply affects pigment synthesis. In algae, PBP functions not only as a light-harvesting pigment-protein, but also as a nitrogen reserve. Under nutrient-sufficient conditions, algae absorb nutrients from the environment to synthesize sufficient PBP to survive even if they are utilized and/or degraded in the absence of nutrients (Gantt et al., 2003; He et al., 2018). This hypothesis was supported by the finding that more pigments were synthesized under high nitrogen and phosphorus conditions than under low nitrogen and phosphorus conditions (Rowan, 1989). In another study, pigment concentrations were found to decrease in parallel with nitrogen or phosphorus starvation (Latasa and Berdalet, 1994). Consistent with those findings, we found that the PE and PC contents of Z-61 and Z-26 increased with increasing nutrient concentrations in the growth media.
In P. haitanensis, PBP are key natural pigments with biological activity. They also play an important role in conferring the different colors of various strains. A recent study found that the PE content determined the color of P. haitanensis. That is, the PE contents were higher in red and yellow strains than in green strains, but the Chl a content was not significantly different among the differently colored strains (Lian, 2019). To date, only red strains of P. haitanensis are accepted in the market, so only red strains are used in aquaculture. Compared with thalli cultured in oligotrophic areas, those cultured in fertile areas had a deeper red color, and a prolonged lack of nitrogen caused greening disease (He et al., 2018). When the internal nitrogen reserves of algae were deficient, the deep red color gradually disappeared so that thalli became yellow and stop growing (He et al., 2018). The colors of Z-61 and Z-26 are red-brown and green, respectively, and are determined by the relative contents of PBP and chlorophyll. In this study, under low-nutrient conditions, the color of the algal blades gradually became lighter with prolonged culture time.
With a wide range of applications and high development and utilization value, PBPs have been applied in biotechnological fields where they are used in fluorescent reagents, biomolecule labeling, and immunochemistry. In addition, PBPs are used in food, cosmetics, dyes, and medicines (Sekar and Chandramohan, 2008; Harnedy and FitzGerald, 2011). Our study demonstrates that nutrient enrichment may enhance the market value of P. haitanensis via stimulating the synthesis of PBPs.
Nutrients are essential for protein synthesis. A previous study found higher tissue nitrogen concentrations in P. haitanensis cultured in areas with higher nitrate levels (Zeng et al., 1985). Thus, it is generally suggested that P. haitanensis should be cultured in areas with higher nutrient levels and/or with high flow rates to replenish the nutrient supply. This is supported by our finding that protein contents in P. haitanensis were positively correlated with nutrient concentrations (Figure 5). For example, in the N3P3 treatment, the protein content of both strains increased to 40%. Similarly, a previous study found the content of total soluble proteins in Hypnea cervicornis increased by 67% when the concentrations of nitrate and phosphate in the culture medium were increased by 200% (Ribeiro et al., 2012). The protein content in Heterocapsa sp. cells decreased significantly under phosphorus-limited conditions (Berdalet et al., 1994). Thus, it can be concluded that nutrient enrichment stimulates protein synthesis in P. haitanensis, which could improve its quality as a food.
Higher nutrient levels increased the contents of most amino acids and FAA in P. haitanensis, thus increasing the contents of TAA, TFAA, TEAA, UAA, and SAA (Tables 2, 3). The TAA and TFAA contents differed significantly between the two P. haitanensis strains. Particularly, under the highest nutrient conditions, the TAA and TFAA contents of Z-61 were lower and higher, respectively, than those of Z-26. This result indicates that the relative proportions of amino acids differs between the two strains. Ji et al. (2011) found that the TAA content of wild P. haitanensis sampled from eight sites along the coast of Fujian Province was about 180∼265 g kg–1, lower than the levels detected in Z-26 under nutrient-enriched conditions, comparable to the levels detected in Z-61 under nutrient enriched conditions, but higher the levels detected in Z-61 in the N0P0 treatment. The differences in amino acid levels between thalli cultured in laboratory and natural conditions may be because of the different strains used. In addition, water movement could relieve nutrient limitation, although the NO3– and PO43– concentrations in coastal waters are comparable to those in the N0P0 treatment (Zeng et al., 1985).
In the present study, the arginine and lysine contents increased with increased NO3– and PO43– concentrations in the media. Arginine is an essential amino acid for fetuses and neonates, and it can enhance the activity of arginine-metabolizing enzymes in the liver. All cereal proteins have a low lysine content, resulting in lysine deficiency in the human body (Wu, 2009). The rich lysine content in algal thalli can make up for the deficiency in cereals and legumes. Notably, when the concentrations of NO3– and PO43– increased from N2P2 to N3P3, the concentrations of most amino acids were unchanged but that of FAA almost doubled (Tables 2, 3). This suggested that the protein production was already saturated under the nutrient conditions in the N2P2 treatment (Figure 4B), but there was further potential for increased accumulation of FAA in the N3P3 treatment.
The pathway of amino acid synthesis in algal cells begins with the reduction of nitrate to nitrate in the cytosol. Nitrite is then further reduced to ammonium in the chloroplasts, and the ammonium is used for amino acid synthesis (Heldt and Piechulla, 2010). An increase in nitrogen content commonly stimulates amino acid synthesis. In previous studies on P. yezoensis, when the nitrogen concentration in the medium was increased, the TAA or TFAA contents significantly increased (Li et al., 2016; Gao et al., 2019). In a study on Ulva ohnoi, the TAA content of the green macroalga increased linearly with intracellular nitrogen content (Angell et al., 2014). Phosphorus also plays a critical role in the synthesis of amino acids. For instance, the addition of phosphorus was shown to stimulate the synthesis of amino acids including alanine and glycine (Barrett, 2012). Several phosphorus-containing co-enzymes, such as pyridoxal phosphate (which catalyzes transamination reactions) are also required for amino acid synthesis, indicating that the synthesis of amino acids could be affected by phosphorus content (Heldt and Piechulla, 2010). In our study, the contents of amino acids in thalli were significantly higher under high-phosphorus conditions than under low-phosphorus conditions.
Under low-nutrient conditions (N0P0), the total protein, aspartic acid, arginine and threonine contents in Z-26 were approximately double those in Z-61 (Figure 4 and Table 2). A previous study found that differences between strains might lead to differences in amino acid contents in P. yezoensis (Hu et al., 2015). Our results indicate that Z-26 is more tolerant than Z-61 to low-nutrient conditions. Under nutrient-limited conditions, Z-26 maintained a higher food quality, indicating that this strain is more suitable than Z-61 for broad applications. However, the TFAA content was lower in Z-26 than in Z-61 in all treatments, indicating that the flavor of Z-61 is superior to that of Z-26. The green color of Z-26 makes it less appealing to consumers than red strains, including Z-61, which might limit its acceptance in the market. Based on the results of this study, nutrient enrichment can increase the protein and amino acids contents of P. haitanensis. This may play a key role in the quality of P. haitanensis, as amino acids are required for the synthesis of enzymes, hormones, and other important components of the body, and participate in a wide range of physiological activities and metabolism (Wu, 2009).
To the best of our knowledge, there is limited understanding of the effects of nutrient enrichment on the growth, biomass yield, and food quality of P. haitanensis. In these laboratory experiments, nutrient enrichment showed some potential to improve the market value and food quality of P. haitanensis. The contents of pigments, proteins, and amino acids, including free amino acids, were increased in both strains under nutrient-enriched conditions. The results of this study provide important information on the effects of nutrient enrichment on the food quality of the marine crop P. haitanensis.
The raw data supporting the conclusions of this manuscript will be made available by the authors, without undue reservation to any qualified researcher.
NX and KX performed the experiments and analyzed the data. KX and CX conceived and designed the experiments. NX wrote the manuscript, prepared figures and tables. KX reviewed drafts of the manuscript. WW, YX, DJ, and CC performed the experiments and reviewed drafts of the manuscript. All authors contributed to the article and approved the submitted version.
The authors gratefully acknowledge funding from the National Key Research and Development Program of China (2018YFD0900702, 2018YFD0901506), the National Natural Science Foundation of China (41806183), and the China Agriculture Research System (CARS-50), and the Training Program of the National Foundation of Jimei University.
The authors declare that the research was conducted in the absence of any commercial or financial relationships that could be construed as a potential conflict of interest.
We thank Catherine Dandie, Ph.D., and Jennifer Smith, Ph.D., from Liwen Bianji, Edanz Editing China (www.liwenbianji.cn/ac), for editing the English text of drafts of this manuscript.
Angell, A. R., Mata, L., de Nys, R., and Paul, N. A. (2014). Variation in amino acid content and its relationship to nitrogen content and growth rate in Ulva ohnoi (Chlorophyta). J. Phycol. 50, 216–226. doi: 10.1111/jpy.12154
Barrett, G. (2012). Chemistry and Biochemistry of the Amino Acids. New York, NY: Springer Science & Business Media.
Berdalet, E., Latasa, M., and Estrada, M. (1994). Effects of nitrogen and phosphorus starvation on nucleic acid and protein content of Heterocapsa sp. J. Plankton Res. 16, 303–316. doi: 10.1093/plankt/16.4.303
Chopin, T., Gallant, T., and Davison, I. (1995). Phosphorus and nitrogen nutrition in Chondrus crispus(Rhodophyta):effects on total phosphorus and nitrogen content, carrageenan production, and photosynthetic pigments and metabolism. J. Phycol. 31, 283–293. doi: 10.1111/j.0022-3646.1995.00283.x
Conley, D. J., Paerl, H. W., Howarth, R. W., Boesch, D. F., Seitzinger, S. P., Havens, K. E., et al. (2009). Controlling Eutrophication:nitrogen and phosphorus. Science 323, 1014–1015. doi: 10.1126/science.1167755
Dentener, F., Drevet, J., Lamarque, J.-F., Bey, I., Eickhout, B., Fiore, A. M., et al. (2006). Nitrogen and sulfur deposition on regional and global scales: a multimodel evaluation. Glob. Biogeochem. Cycles 20, 1–20. doi: 10.1029/2005gb002672
Fernández, E., Serret, P., de Madariaga, I., Harbour, D. S., and Davies, A. G. (1992). Photosynthetic carbon metabolism and biochemical composition of spring phytoplankton assemblages enclosed in microcosms: the diatom-Phaeocystis sp. succession. Mar. Ecol. Prog. Ser. 90, 89–102. doi: 10.3354/meps090089
Gantt, E., Grabowski, B., and Cunningham, F. X. (2003). “Antenna systems of red algae: phycobilisomes with photosystem ll and chlorophyll complexes with photosystem I,” in Light-Harvesting Antennas in Photosynthesis, eds E. Gantt and B. Grabowski (Berlin: Springer), 307–322. doi: 10.1007/978-94-017-2087-8_10
Gao, G., Clare, A. S., Rose, C., and Caldwell, G. S. (2018). Ulva rigida in the future ocean: potential for carbon capture, bioremediation and biomethane production. Glob. Change Biol. Bioenergy 10, 39–51. doi: 10.1111/gcbb.12465
Gao, G., Gao, Q., Bao, M., Xu, J., and Li, X. (2019). Nitrogen availability modulates the effects of ocean acidification on biomass yield and food quality of a marine crop Pyropia yezoensis. Food Chem. 271, 623–629. doi: 10.1016/j.foodchem.2018.07.090
Gao, H. (1993). The variation in the contents of phycobilipro-teins from Porphyra haitanensis collected in different growing stages. Oceanol. Limnol. Sin. 24, 645–648.
Glibert, P. M. (2017). Eutrophication, harmful algae and biodiversity — Challenging paradigms in a world of complex nutrient changes. Mar. Pollut. Bull. 124, 591–606. doi: 10.1016/j.marpolbul.2017.04.027
Harnedy, P. A., and FitzGerald, R. J. (2011). Bioactive proteins, peptides, and amino acids from macroalgae. J. Phycol. 47, 218–232. doi: 10.1111/j.1529-8817.2011.00969.x
He, P., Zhang, Z., Zhang, X., and Ma, J. (2018). Seaweed Cultivation (in Chinese). Beijing: Science Press.
Heisler, J., Glibert, P. M., Burkholder, J. M., Anderson, D. M., Cochlan, W., Dennison, W. C., et al. (2008). Eutrophication and harmful algal blooms: a scientific consensus. Harmful Algae 8, 3–13. doi: 10.1016/j.hal.2008.08.006
Heldt, H.-W., and Piechulla, B. (2010). “Nitrate assimilation is essential for the synthesis of organic matter,” in Plant Biochemistry, ed. H. W. Heldt (San Diego: Academic Press), 273–306. doi: 10.1016/b978-0-12-384986-1.00010-7
Hiroyuki, M. (2002). Relationship between phycoerythrin and nitrogen content in Gloiopeltis furcata and Porphyra yezoensis. Algae 17, 89–93. doi: 10.4490/algae.2002.17.2.089
Hu, C., Lu, Q., Zhang, M., and Zhu, J. (2015). FAA composition and content Variation in different Pyropia yezoensis cultiivar in harvest period. Agric. Sci. Technol. 16, 2816–2820. doi: 10.16175/j.cnki.1009-4229.2015.12.052
Israel, A., Einav, R., and Seckbach, J. (2010). “Cellular origin, life in extreme habitats and astrobiology,” in Seaweeds and Their role in Globally Changing Environments, ed. A. Israel (Netherlands: Springer), 480.
Jensen, A. (1978). “Handbook of physiological methods: physiological and biochemical methods,” in Chlorophylls and Carotenoids, ed. A. Jensen (London: Cambridge University Press), 59–70.
Ji, D.-H., Xie, C.-T., and Shi, X.-Z. (2011). Analysis of the major quality traits in wild Porphyra haitanensis of Fujian Coast. J. Jimei Univ. 16, 16–18. doi: 10.19715/j.jmuzr.2011.06.001
Latasa, M., and Berdalet, E. (1994). Effect of nitrogen or phosphorus starvation on pigment composition of cultured Heterocapsa sp. J. Plankton Res. 16, 83–94. doi: 10.1093/plankt/16.1.83
Li, S., and Yan, X. (2015). Isolation and characterization of an improved strain of Pyropia dentat(Bangiales,Rhodophyta)after being irradiated by ~(60)Co-γray (in Chinese). Acta Oceanol. Sin. 37, 69–79. doi: 10.3969/j.issn.0253-4193.2015.10.007
Li, X., Xu, J., and He, P. (2016). Comparative research on inorganic carbon acquisition by the macroalgae Ulva prolifera (Chlorophyta) and Pyropia yezoensis (Rhodophyta). J. Appl. Phycol. 28, 491–497. doi: 10.1007/s10811-015-0603-8
Lian, Y. (2019). The color formation mechanism of the mutant of Pyropia haitanensis pigment. master’s thesis, Jimei University, Xiamen.
Lobban, C. S., and Harrison, P. J. (2004). Seaweed ecology and physiology. Biol. Plant. 38:396. doi: 10.1007/BF02896669
Mouritsen, O. G., Rhatigan, P., and Pérez-Lloréns, J. L. (2018). World cuisine of seaweeds: science meets gastronomy. Int. J. Gastron. Food Sci. 14, 55–65. doi: 10.1016/j.ijgfs.2018.09.002
Nishimura, T., and Kato, H. (1988). Taste of free amino acids and peptides. Food Rev. Int. 4, 175–194. doi: 10.1080/87559128809540828
Noda, H. (1993). Health benefits and nutritional properties of nori. J. Appl. Phycol. 5, 255–258. doi: 10.1007/BF00004027
Pedersen, M. F., and Borum, J. (1996). Nutrient control of algal growth in estuarine waters. Nutrient limitation and the importance of nitrogen requirements and nitrogen storage among phytoplankton and species of macroalgae. Mar. Ecol. Prog. Ser. 142, 261–272. doi: 10.3354/meps142261
Ribeiro, A. L. N. L., Tesima, K. E., Souza, J. M. C., and Yokoya, N. S. (2012). Effects of nitrogen and phosphorus availabilities on growth, pigment, and protein contents in Hypnea cervicornis J. Agardh (Gigartinales, Rhodophyta). J. Appl. Phycol. 25, 1151–1157. doi: 10.1007/s10811-012-9938-6
Sekar, S., and Chandramohan, M. (2008). Phycobiliproteins as a commodity: trends in applied research, patents and commercialization. J. Appl. Phycol. 20, 113–136. doi: 10.1007/s10811-007-9188-1
Smit, A. J., Robertson, B. L., and du Preez, D. R. (1996). Influence of ammonium-N pulse concentrations and frequency, tank condition and nitrogen starvation on growth rate and biochemical composition of Gracilaria gracilis. J. Appl. Phycol. 8, 473–481. doi: 10.1007/bf02186325
Starr, R. C., and Zeikus, J. A. (1993). UTEX - the culture collection of algae at the University of Texas at Austin 1993 list of culures. J. Phycol. 29, 1–106. doi: 10.1111/j.0022-3646.1993.00001.x
Strokal, M., Yang, H., Zhang, Y., Kroeze, C., Li, L., Luan, S., et al. (2014). Increasing eutrophication in the coastal seas of China from 1970 to 2050. Mar. Pollut. Bull. 85, 123–140. doi: 10.1016/j.marpolbul.2014.06.011
Tong, Y., Zhao, Y., Zhen, G., Chi, J., Liu, X., Lu, Y., et al. (2015). Nutrient loads flowing into coastal waters from the main rivers of China (2006–2012). Sci. Rep. 5:16678. doi: 10.1038/srep16678
Vergara, J. J., Pérez Lloréns, J. L., Peralta, G., Hernández, I., and Niell, F. X. (1997). Seasonal variation of photosynthetic performance and light attenuation in Ulava canopies from palmones river estuary. J. Phycol. 33, 773–779. doi: 10.1111/j.0022-3646.1997.00773.x
Von Wettstein, D., Gough, S., and Kannangara, C. G. (1995). Chlorophyll biosynthesis. Plant Cell 7:1039.
Wang, Y., Xu, K., Wang, W., Xu, Y., Ji, D., Chen, C., et al. (2019). Physiological differences in photosynthetic inorganic carbon utilization between gametophytes and sporophytes of the economically important red algae Pyropia haitanensis. Algal Res. 39:101436. doi: 10.1016/j.algal.2019.101436
Wells, M. L., Potin, P., Craigie, J. S., Raven, J. A., Merchant, S. S., Helliwell, K. E., et al. (2017). Algae as nutritional and functional food sources: revisiting our understanding. J. Appl. Phycol. 29, 949–982. doi: 10.1007/s10811-016-0974-5
Wu, G. (2009). Amino acids: metabolism, functions, and nutrition. Amino Acids 37, 1–17. doi: 10.1007/s00726-009-0269-0
Xu, L., Wu, F., Guo, Y., and Gao, H. (2019). China Fishery Statistical Yearbook (2019). Beijing: China Agricultural Press.
Yang, Y., Chai, Z., Wang, Q., Chen, W., He, Z., and Jiang, S. (2015). Cultivation of seaweed Gracilaria in Chinese coastal waters and its contribution to environmental improvements. Algal Res. 9, 236–244.
Zeng, C., Wang, S., and Liu, S. (1985). Seaweed Cultivation. Shanghai: Shanghai scientific & technical publishers.
Keywords: amino acid, eutrophication, phycobiliprotein, protein, Pyropia
Citation: Xu N, Xu K, Wang W, Xu Y, Ji D, Chen C and Xie C (2020) Nutrient Enrichment Improves Growth and Food Quality of Two Strains of the Economic Seaweed Pyropia haitanensis. Front. Mar. Sci. 7:544582. doi: 10.3389/fmars.2020.544582
Received: 21 March 2020; Accepted: 27 October 2020;
Published: 13 November 2020.
Edited by:
Leonardo Julián Magnoni, University of Porto, PortugalCopyright © 2020 Xu, Xu, Wang, Xu, Ji, Chen and Xie. This is an open-access article distributed under the terms of the Creative Commons Attribution License (CC BY). The use, distribution or reproduction in other forums is permitted, provided the original author(s) and the copyright owner(s) are credited and that the original publication in this journal is cited, in accordance with accepted academic practice. No use, distribution or reproduction is permitted which does not comply with these terms.
*Correspondence: Chaotian Xie, Y3R4aWVAam11LmVkdS5jbg==
†These authors share first authorship
Disclaimer: All claims expressed in this article are solely those of the authors and do not necessarily represent those of their affiliated organizations, or those of the publisher, the editors and the reviewers. Any product that may be evaluated in this article or claim that may be made by its manufacturer is not guaranteed or endorsed by the publisher.
Research integrity at Frontiers
Learn more about the work of our research integrity team to safeguard the quality of each article we publish.