- 1Department of Botany, University of British Columbia, Vancouver, BC, Canada
- 2Climate Change Cluster, University of Technology Sydney, Ultimo, NSW, Australia
- 3School of Biological Earth and Environmental Sciences, University of New South Wales, Kensington, NSW, Australia
- 4New South Wales Department of Primary Industries, Fisheries, National Marine Science Centre, Coffs Harbour, NSW, Australia
Species inhabiting warm-edge populations of their distribution are suggested to be at the forefront of global warming due to reduced fitness, limited gene flow and living close to their physiological thermal limits. Determining the scale that governs thermal niche and the functional responses of habitat-forming species to environmental stressors is critical for successful conservation efforts, particularly as coastal ecosystems are impacted by global change. Here, we examine the susceptibility of warm-edge populations to warming, in the habitat-forming macroalga, Hormosira banksii, from south-eastern Australia. We use a quantitative breeding design to quantify intraspecific variation in thermal performance (growth, ontogenic development and photosynthetic efficiency) of different genotypes sourced from sites at the equatorward distributional edge (warm-edge) and those toward the center of its distribution (non-edge). The genetic diversity and structure of H. banksii was also examined using microsatellite markers amongst the same sites. Our results found variable responses in thermal performance for growth and development. Warm-edge germlings grew optimally in lower temperatures tested and had narrower thermal breadth compared to non-edge germlings which grew in higher and more broader temperatures. Warm-edge germlings however, showed greater plasticity to tolerate high light indicated by a greater proportion of energy being dissipated as regulated non-photochemical quenching [Y(NPQ)] than non-regulated non-photochemical quenching [Y(NO)]. Overall genetic diversity was lower at the warm-edge location with evidence of increased structuring and reduced gene flow in comparison to the non-edge location. Evidence of genetic structuring was not found locally between high and low shore within sites. Together, these data suggest that non-edge populations may be “thermally buffered” from increased temperatures associated with ocean warming. Warm-edge populations of H. banksii, however, may be vulnerable to warming, due to narrower thermal breadth and sensitivity to higher temperatures, with genetic impoverishment through loss of individuals likely to further reduce population viability.
Introduction
Anthropogenic mediated climate change is already having profound impacts on the physiology and distribution of many species worldwide (Pecl et al., 2017; IPCC, 2018). By the end of this century, anthropogenic increases in atmospheric greenhouse gases will have increased ocean and air temperatures by 1.5–2°C with global mean surface temperatures already warmed by 0.87°C during the decade 2006–2015 (IPCC, 2018). In the last decade, the prevalence of extreme climate events (heatwaves, droughts, floods, cold spells, and storms) has caused further loss of populations and poleward shifts in distribution as species are being pushed past their physiological thresholds (Hawkins et al., 2009; Burrows et al., 2014; Poloczanska et al., 2016; Smale et al., 2019). Persistence in the face of a warming climate will require physiological plasticity and adequate genetic diversity for natural selection to act upon (Sgrò and Hoffmann, 2004; Reusch et al., 2005; Hoffmann and Sgrò, 2011; Wernberg et al., 2018; Gurgel et al., 2020).
Understanding how global warming and future extreme climate events will impact species requires knowledge of species’ thermal niche and underlying genetic diversity across its distribution. Thermal niche is developed through acclimation and adaptation to temperatures experienced throughout a species life history which can vary with space and time (for a review, see Bennett et al., 2015). For instance, thermal limits will differ for central and marginal populations as thermal regimes vary across a species geographical range (Sunday et al., 2012; Bennett et al., 2019). Thermal breadth is also influenced by the range of temperatures experienced throughout a species life history (Sunday et al., 2012). The climate variability hypothesis suggests that a positive relationship exists between thermal breadth of an organism and climate variability with increasing latitude. Therefore, populations in higher latitudes will have a greater thermal breadth as individuals experience a greater range in temperatures, than those closer to the equator (Stevens, 1989). Thus, cooler populations are suggested to be more resistant to warming as they have a broader thermal breadth compared to warmer populations (however see Bennett et al., 2015). As thermal limits often govern species range boundaries, and individuals are pushed beyond their physiological limits, the fitness of individuals therefore diminish toward distributional limits (Sagarin and Gaines, 2002; Thomas et al., 2004; Hampe and Petit, 2005; Pearson et al., 2009).
Reduced fitness at range margins often coincides with reduced gene flow and connectivity, habitat fragmentation (local separation of populations) and reduced effective population sizes. These can result in decreased genetic diversity (the range of functional responses provided by genotypes and phenotypes) and increase genetic differentiation between populations (Hampe and Petit, 2005; Eckert et al., 2008; Coleman et al., 2011a; Wernberg et al., 2018). The decrease in genetic diversity toward range limits has been documented extensively in plants and animals (Hampe and Petit, 2005; for a review see Eckert et al., 2008). Such patterns may also exist at the local scale over strong, but small-scale environmental gradients, where individuals live in habitat mosaics or where they may be spatially or temporally segregated (Helmuth et al., 2006; Harley, 2008). Species inhabiting edge distributions are at the forefront of climate change, as they are already restricted by environmental factors and living close to their physiological limits (Parmesan, 2006; Smale and Wernberg, 2013; Pecl et al., 2017). Without adequate genetic diversity, potential selection for tolerant genotypes may be limited.
Investigating the spatial scale at which variation in environmental stressors will most strongly influence fitness is needed to determine impacts on biological systems (Helmuth et al., 2014). Species’ distributions can often span thousands of kilometers and individuals can be exposed to wide variation in environmental regimes at different spatial scales; regionally among latitudes, and locally, among habitats within a single location. Factors such as local climate and topography within a habitat can translate to mosaics of “hotspots” and “coldspots” (Helmuth et al., 2006). For sessile organisms, morphological differences among individuals add to local habitat topography and daily fluctuations in exposure, all of which can shape differing physiological thresholds (Helmuth and Hofmann, 2001; Harley, 2008; Clark et al., 2018). Studies have also suggested that variation at the scale of an individual can have a greater effect on physiology than broad scale differences observed over kilometers (Helmuth et al., 2002; Helmuth, 2009). Consequently, species declines in response to increasing environmental stress may not occur evenly across their range (Helmuth et al., 2006; Pearson et al., 2009; Miller et al., 2019), yet understanding intraspecific variation in tolerance is required to predict future species distributions.
The rocky intertidal has been suggested to be a sentinel habitat for global warming, primarily due to resident organisms being already close to their thermal limits (Stillman and Somero, 2000; Somero, 2005). Habitat-forming macroalgae are particularly important primary producers in this habitat due to their role as ecosystem engineers through modifying local environmental conditions and providing resources (Dayton, 1972; Jones et al., 1994) that can strongly facilitate associated biodiversity (Schiel, 2006; Bishop et al., 2009). As macroalgae are sessile organisms, they cannot move to avoid heat stress so must physiologically tolerate, adapt or perish in the face of climate change. Studies on the effects of temperature in governing species distribution and warm-edge ranges are becoming more apparent in macroalgal dominated communities (Pearson et al., 2009; Martínez et al., 2012; Ferreira et al., 2014; Bennett et al., 2015; Mota et al., 2018; King et al., 2019). Temperature is a fundamental determinant of algal fitness as it regulates photosynthesis as well as enzymes that govern metabolic activity (Allakhverdiev et al., 2008; Falkowski and Raven, 2013). Due to this, photosynthetic health has been widely used to assess thermal tolerance in photosynthetic organisms including many plants and macroalgae (Pearson et al., 2009; Smolina et al., 2016; Wernberg et al., 2016). Photosynthetic performance, in turn, can be evaluated by chlorophyll-a fluorescence measurements, specifically the maximum quantum yield (FV/FM) and investigation of energy dissipation pathways (Genty et al., 1989; Kramer et al., 2004; Schreiber, 2004).
Variation in thermal response among marine macroalgae has been mostly studied in the context of determining how lethal temperatures set distributional limits both across species ranges and vertically on the shore (Schonbeck and Norton, 1978; Hartnoll and Hawkins, 1985; Davison and Pearson, 1996). On regional scales, individuals inhabiting warm range-edge populations closer to the equator have been shown to have greater thermal tolerances due to exposure to higher temperatures throughout their life history (Mota et al., 2018). Photosynthetic health of macroalgae at warm-range limits also reflect greater thermal tolerances indicating greater ability of warm-edge thalli to maintain maximum quantum yield of PSII (FV/FM) in higher temperatures than in cool-edge populations (Mota et al., 2018). Warm-edge populations are often fragmented and reduced in size (Coleman et al., 2011a, b; Zardi et al., 2015), suggesting that these populations are physiologically stressed toward range limits (Araújo et al., 2011) and may be less resilient to prolonged exposure to extreme climate events (Wernberg et al., 2016; Mota et al., 2018). Variation in thermal response on the local scale, among vertical heights on the shore, is well known and is related to daily tidal regimes and topography, and the ability to effectively photosynthesize during periods of increased desiccation and thermal stress (Schonbeck and Norton, 1978; Dring and Brown, 1982; Davison and Pearson, 1996; Williams and Dethier, 2005). Less well understood is the contribution of heritable genetic variation in thermal tolerance, required if there is to be any local adaptation to a given thermal regime or evolution of increased tolerance with increasing temperatures. Relatively few studies have experimentally identified heritable genetic variation in seaweeds with families showing the potential for adaptation to changes in environment associated with climate change (Clark et al., 2013; Al-Janabi et al., 2019; Mabin et al., 2019).
Southeastern Australia has been identified as a climate change hotspot (Lough and Hobday, 2011; Hobday and Pecl, 2014) and is therefore an ideal location to study the effects of global warming on the genetic diversity and physiology of marine macroalgae. The east coast of Australia follows a north (equatorward) to south (poleward) thermal gradient with a natural warm to cold water transition, and serves as the main conduit for gene flow for many sessile marine species within coastal ecosystems (Coleman et al., 2011b). Hormosira banksii, is a dominant, habitat-forming macroalga found on rocky shores in Australia and New Zealand and spans > 3000 km of coastline from the northern distributional edge Skennars Head in New South Wales (Figure 1) to Albany in Western Australia (Womersley, 1987; Huisman, 2019) as well Tasmania, and the North and South Island of New Zealand (Nelson, 2013). Toward the equatorward distributional limits, percent cover decreases from 80% at Minnie Water to 20% at Angourie and 25% at Skennars Head, with H. banksii mostly found in rockpools at the distributional limits (personal observation). It is dioecious and has a monophasic life cycle with oogonium development found in mature conceptacles in every season, producing gametes potentially every low tide (Osborn, 1948). Further, gamete dispersal is less than 10 m (Bellgrove et al., 1997), and has been documented to have limited gene flow (Coleman et al., 2011a, 2019) with local adaptation recently identified in thermally different regions on the south coast of Australia (Miller et al., 2019). Previous research on thermal performance of H. banksii is limited, but has identified increased thermal sensitivity through decreased photosynthetic yield of PSII, smaller morphology and lower percent coverage of thalli (∼20%) in adult thalli of warm-edge populations of H. banksii (Clark et al., 2018). Less is known about how populations of H. banksii within the equatorward range edge of this species will respond to elevated temperatures and extreme climate events, given limited gene flow and inhabiting physiological stressful environments.
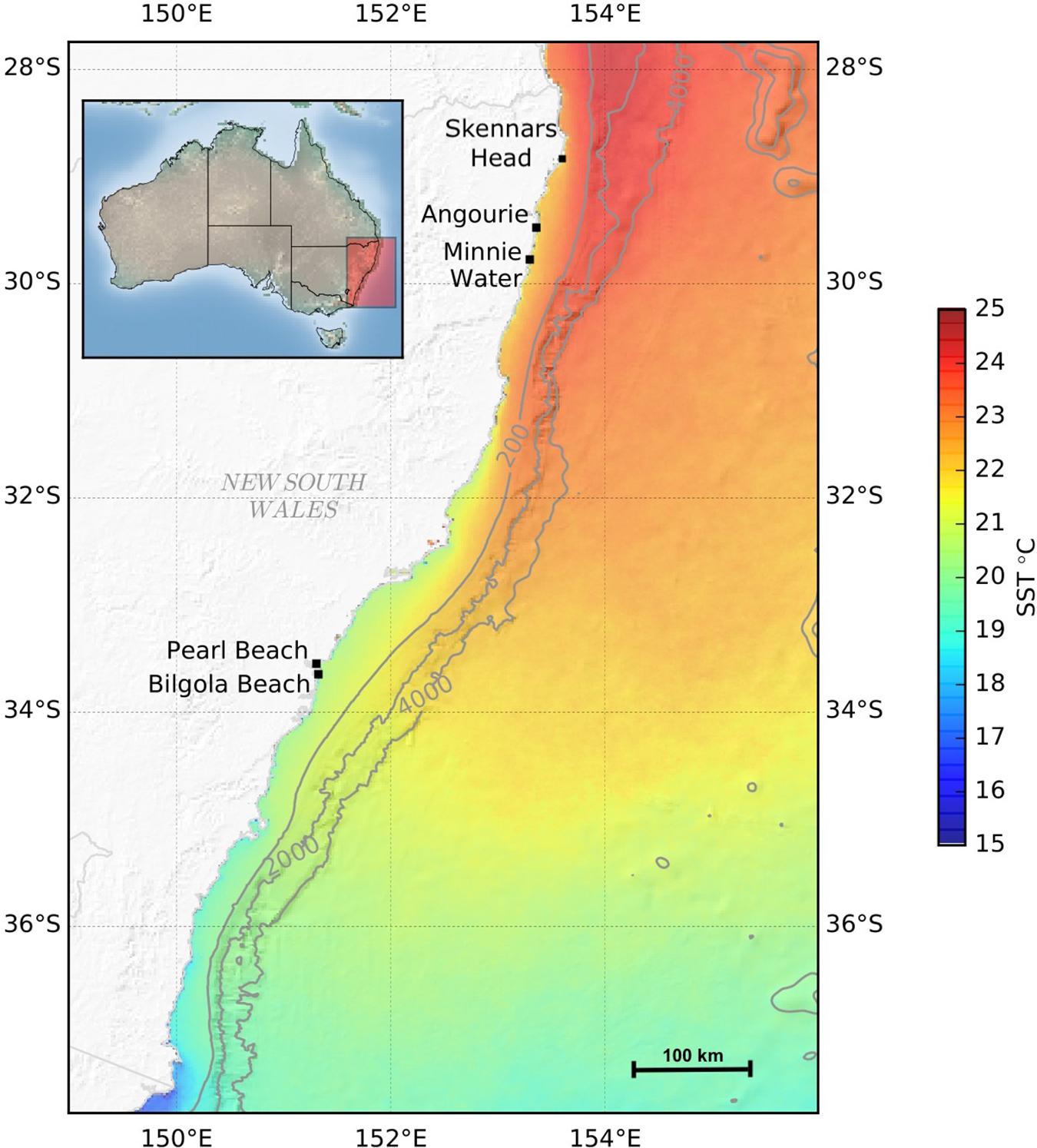
Figure 1. Map of locations from which H. banksii populations were collected from southeastern coast of Australia. Skennars Head is the current equatorward distributional range edge. Sea surface Temperature (SST) data reflect average SST taken from 2003 to 2015 from the Integrated Marine Observing System (IMOS) and used to illustrate coastal temperatures.
In this study, multiple performance traits of the ecologically and functionally important intertidal macroalga, Hormosira banksii, were quantified to assess variation in thermal tolerance and genetic structuring at three nested spatial scales: among locations, among heights on the shore and among individual genotypes within a shore, to determine the vulnerability of warm-edge populations to climate warming. We aimed to (1) assess the thermal performance of individuals (2) determine whether genetic variation in traits are heritable, and (3) determine the genetic diversity and connectivity of warm-edge versus non-edge populations of H. banksii to assess the role of thermal history and adaptation in providing resilience to global warming.
Materials and Methods
Study Locations and Collection of Model Organism
For genetic diversity analysis, H. banksii populations were sampled during the austral autumn (April–May 2014) in two eastern Australian regions: a warm-edge region encompassing two locations, Angourie (29°28′41.51″ S, 153°21′49.53″ E) and Minnie Water (29°46′34.23″ S, 153°18′07.43″ E) at the northern warm-edge of its distribution, and a non-edge region within the center of its range, encompassing two locations, Pearl Beach (33°32′57.70″ S, 151°18′32.36″ E) and Bilgola Beach (33°38′54.48″ S, 151°19′39.59″ E), approximately 460 km further south (Figure 1). For thermal response experiments, H. banksii was collected from two populations one within each of the mentioned regions (Minnie Water and Pearl Beach). At the time of sampling, H. banksii was the dominant, intertidal macroalgal species (60–80% cover) in all locations except Angourie which had 20% cover.
Thermal Exposure at Different Spatial Scales
Data from multiple sources was used to demonstrate average air and sea surface temperatures (SST) experienced at each warm-edge (Minnie Waters) and non-edge (Pearl Beach) location (Supplementary Figure S2). SST detected by satellite (MODIS-Aqua), was obtained via GIOVANNI (NASA GES DISC) using a 4 km2 area (Minnie Water −29.5°S, 29.0°S 153.4°E 153.9°E; Pearl Beach −33.844°S, −33.441°S, 151.296°E, 151.671°E) for years between 2003 and 2014. Local weather stations were used to estimate average minimum, maximum air temperature as well as mean number of days above 35°C at each location [Bureau of Meteorology (BOM), Australian Government1; Terry Hills station from 1954 to 2014 and Yamba from 1877 to 2014)]. Data reflect average temperatures recorded from when station first became operational. To document the temperature variability locally within H. banksii beds at each location, single HOBO® pendant loggers (Onset®, United States) were drilled into the substrate at high and low tidal heights and temperatures were recorded between April and July 2014 (Supplementary Figure S3). Data only overlapped for 7 days in June 2014 due to some loggers going missing, therefore were only used to view the temperature variability within each shore height at each location and were not compared between locations.
Assessment of Genetic Diversity
To assess genetic structure of populations, 32 thalli (approximately 1 m apart) were haphazardly sampled from the low and high shore at each location within each of the regions. High shore thalli were selected based on tidal exposure, local topography and drainage patterns to ensure they contrasted with low shore thalli, which were immediately adjacent to the water’s edge. Collection at low and high tidal heights were separated horizontally by ∼5–10 m above the low tide mark. Extraction of genomic DNA was conducted for a total of 235 individuals. Samples comprised of unfouled apical segments that were washed in freshwater to remove salts and epiphytes, snapped frozen in liquid nitrogen before storing in a −80°C freezer until use. Before DNA extraction, samples were freeze-dried overnight. Genomic DNA was isolated from 20–30 mg of freeze-dried tissue using the Nucleospin® 96 Plant II DNA extraction kit (Machery-Nagel, AGRF). Individuals were genotyped using 10 microsatellite loci as described in Bellgrove et al. (2017). Each 11 μL PCR reaction was set up in which consisted of 5 μL 2 × Multiplex Mastermix (Qiagen), 4 μL Primer mastermix and 2 μL of 1 in 20 diluted genomic DNA. Primer mastermix consisted of 10 μM reverse primer, 10 μM forward primer and 10 μM unique fluorophores (FAM, VIC, NED, PET) which tagged the flanking regions of the microsatellites.
Multiplex PCR reactions were run on a Veriti 96-well thermal cycler (Applied Biosystems) with the PCR conditions of 95°C for 15 min for denaturing, followed by 40 cycles of 94°C for 30 s, 59°C for 90s, 72°C 60 s, and a final elongation step at 60°C for 30 min as per the protocol described in Blacket et al. (2012). PCR products were checked for amplification using 1.5% agarose gel before fragment separation was conducted using ABI Genescan 3730 using the size standard LIZ500 (AGRF). Polymorphisms and allele sizes were visualized and determined manually using GeneMapper (v 4.0, Applied Biosystems).
Effects of Temperature on Phenotypic Traits
To assess how functional thermal responses differed within and across populations, quantitative breeding designs were set up to partition variance amongst different genotypes (families), amongst different vertical heights on the shore and between the warm and non-edge location. Adult thalli were collected 2 h before absolute low tide to prevent desiccation-induced spawning (Gunthorpe et al., 1995). Thalli were collected from the low shore, directly adjacent to the seaward edge of the rock platform, and the high shore, in the upper intertidal, 5–10 m vertically distance from low shore region. Thalli were transported on ice and gametes extracted within 48 h.
To induce spawning, thalli were gently agitated in tap water (room temperature), blotted dry, placed into individual containers and allowed to desiccate at room temperature (Doblin and Clayton, 1995). After 20 min, gametes were released from conceptacles through osmotic stress and desiccation and the sex of the thallus identified by the color of its gametes: olive green for females and orange for males (Osborn, 1948). As in Clark et al. (2013), three males and three females from each shore height within each location were used in a North Carolina II breeding design (Lynch and Walsh, 1998), where each male was cross fertilized with each corresponding female in a fully factorial design, yielding nine unique genotypes. Each egg and sperm solution were filtered through nylon mesh (100 μm for egg solution and 40 μm for sperm solution) to filter out debris and larger algal material before being mixed to initiate fertilization. Aliquots of each egg and sperm solution were distributed amongst multiple petri dishes filled with 0.7 μm (Whatman GFF) filtered seawater containing eight glass coverslips for zygotes to attach to. Petri dishes with settled zygotes were then randomly allocated to each of six temperatures; 22, 24, 26, 28, 30, and 32°C in a Climatron Plant Growth Chamber (Thermoline Scientific, Australia). These temperatures are representative of temperatures experienced by both populations (from climate weather and HOBO® pendants) as well as designed to be physiologically stressful to test thermal performance of intertidal macroalgae in populations at the warmer end of the distribution. To examine thermal responses under more realistic fluctuating environments and hence estimate realized rather than fundamental thermal reaction curves, ±5°C diel cycle was implemented (Paaijmans et al., 2013). This temperature regime was determined from examining field data from the HOBO® pendant loggers in a pilot study. Germlings were incubated in a 12:12 h light cycle at 30 ± 5 μmol photons m–2s–1.
At 120 h after fertilization, germlings growing on coverslips were removed from temperature treatments, wet mounted on a microscope slide and photographed using a light microscope (Olympus BX50, Japan) with AnalySIS imaging software (v 5.0, Japan). Total germling length (defined as extension along the primary rhizoid axis) was calculated from digital images using Image J (National Institutes of Health, United States, V1.6.0_24). To examine stages of development, germlings were scored into five ontogenetic stages: 0 = fertilization through condensation of the chloroplasts; 1 = protrusion of germling cell wall to create a pear shape which later develops into the rhizoid; 2 = division of the germling germinating cell and elongation of a single rhizoid; 3 = elongation of the rhizoid coupled with secondary and tertiary rhizoid development; and 4 = paraphysis development (apical hairs) on top of the germinating cell. These equate to stages 1, 2, 3–5, and 6 (a–d), respectively, in Clarke and Womersley (1981).
A pulse amplitude modulated fluorometer (Microscope Imaging-PAM, Walz GmbH, Germany) was used to examine photophysiological traits of multiple germlings in one field of view while returning individual measurements from each individual. The system comprises a modified epi-fluorescence microscope (AxioScope.A1, Zeiss) equipped with a modulated LED light source and a photomultiplier for detection of modulated chlorophyll-a fluorescence. Germlings attached to cover slips (120 h post fertilization) were wet mounted and scanned under green light (non-stimulatory for photosynthesis). Selection of germlings for measurement involved maximizing the number of germlings in a field of view, as coverslips were discarded after each fluorescence assay. To ensure we obtained high-quality data across all treatments and limit a potential diel effect in photophysiological assessments, we had to restrict our photophysiological measurements to one assay per coverslip. Therefore, the coverslip was briefly scanned to find a field of view where there were numerous germlings sufficiently close together for simultaneous assessment. Although coverslips had the same zygote density before being randomly allocated to temperature treatments, the germling density differed at the time of measurement (120 h), varying between two and eight. Gain settings were adjusted so that base fluorescence (Ft) was between 0.1 and 0.3 for each germling, ensuring fluorescence signals were detectable but not too high to cause saturation during measurements. To use Ft as a proxy for pigment content, values collected at different gain settings were standardized to a single gain setting to make them comparable amongst all germlings. This was done by recording the base fluorescence (Ft) of 120 h old germlings determined using the full range of gain settings. The increase in Ft with gain was then fitted with a log linear regression model (R2 > 0.90) so that Ft could be estimated amongst all germlings, no matter what gain setting was used to perform the steady-state analysis (Kramer et al., 2004).
The measurement protocol involved dark adapting germlings for 5 min before a single saturating pulse of blue light (blue Zeiss LED-Module 470 nm; pulse duration = 0.6 s; pulse intensity > 3000 μmol photons m–2 s–1; FM determination), followed by a two-step steady-state light curve. The steady state analysis included consecutive 5 min exposures to actinic blue light of 32 and 113 μmol photons m–2s–1 (sub-saturating and saturating irradiance, respectively, previously determined in a steady state P vs. I fluorescence curve). Saturating pulses of blue light were spaced 30 s apart to monitor FM’ and Ft. Due to the length of time needed for measurements, photosynthetic traits were only determined for 24, 28, and 32°C (three of the six temperature treatments) and represented the highest and stressful temperature for both populations with other two temperatures equally spanning the thermal gradient.
Maximum quantum yield of photosystem II (PSII), FV/FM, was calculated according to the equation (FM − FO)/FM (Schreiber, 2004). FV/FM is the measure of photosynthetic efficiency of PSII after dark acclimation of photosystems (Genty et al., 1989) with greater values (0–1) equating to greater number of photosystems available for light capture. Effective quantum yield of PSII, ΔF/FM’, was calculated using (FM’ − Ft)/FM’. The proportions of energy being used in photochemistry Y(PSII), regulated non-photochemical quenching Y(NPQ), i.e., energy dissipation through the rapid conversion of xanthophyll pigments) and unregulated non-photochemical quenching of excitation energy [Y(NO); i.e., heat dissipation] were calculated for each actinic light level assuming Y(PSII) + Y(NPQ) + Y(NO) = 1 according to (Kramer et al., 2004). To estimate the capacity of germlings to deal with high light, the relative NPQ between high light (HL) and low light (LL) steps was calculated: [HLY(NPQ)/LLY(NPQ)]. A value less than one means there is less NPQ under high light and the xanthophyll cycle has exceeded its capacity to deal with excess energy.
Statistical Analyses
In situ Temperature Regimes
The difference in temperature variability between high and low shores at each location, temperature data obtained by HOBO® was tested using Levene’s test of variance. SST recorded for each location (MODIS-Aqua satellite 2003–2014) was analyzed with a two-factor ANOVA to test for differences between location and months.
Estimates of Genetic Diversity and Structure
Prior to analyses, genotyping errors such as null alleles, stuttering, dropped alleles, and typographic errors were checked using MICROCHECKER (Van Oosterhout et al., 2004). Estimates of allelic frequencies, observed (HO) and expected (HE) heterozygosity and departures from Hardy–Weinberg equilibrium were conducted in GENETIX (v 4.05.2, Belkhir et al., 2000). For each measure of genetic variation, univariate analyses of variances (ANOVA) were conducted to test for differences between locations with between regions (warm-edge and non-edge) as well as between heights on the shore using PRIMER-E with PERMANOVA (v 6.1.16).
FIS, the proportion of genetic variance contained in an individual (I) relative to the variance contained in a subpopulation (S), and FST, the proportion of genetic variance contained in a subpopulation relative to total genetic variance (T) were estimated using the program FSTAT (v 2.9.3.2, Goudet, 1995) where Weir and Cockerham’s estimates of FST and pairwise comparisons of shore heights were calculated within each location. FST estimates the genetic differentiation among populations and ranges from 0 to 1. FST values of 0–0.05 indicate little differentiation, 0.05–0.25 indicate moderate genetic differentiation and values over 0.25 represent pronounced levels of genetic differentiation (Freeland et al., 2011). FIS estimates the amount of selfing or inbreeding occurring within a population and ranges between −1 and 1, where negative values represent an excess of heterozygotes and positive values represent an excess of homozygotes. FIS estimates were tested for significance using GENETIX. Linkage equilibrium was tested using 1000 permutations in FSTAT. FST estimates among all pairs of populations were calculated in FSTAT and significance levels of pairwise comparisons were corrected using Bonferroni correction (Rice, 1989).
Analysis of molecular variance (AMOVA) was performed using ARLEQUINN (v 3.5.22, Excoffier et al., 2007) which calculated the percentage of genetic variation attributed among and within each location. This analysis was conducted twice to determine variation amongst regions, among locations within regions and within locations (among individuals). FST estimates indicated no significant differences between shore heights, therefore shore heights were pooled for the AMOVA analysis. Isolation by distance was tested using Mantel tests in IBD WebService2 (Jensen et al., 2005) which tests the null hypothesis of no correlation between pairwise geographic distance and genetic distance matrices.
Effects of Temperature on Phenotypic Traits
Physiological responses (variation in germling length and ontogenic development) were analyzed with permutational ANOVA, with location (warm-edge and non-edge), height on the shore (low and high) and temperature (six levels) as fixed factors, and male and female identity as a random factor nested within each combination of height on the shore and location. For significant location × temperature or shore height × temperature interactions, main effects were tested with a reduced two-factor ANOVA to compare each temperature. Tukey’s HSD post hoc comparisons were conducted on significant interactions to determine temperature effects. Thermal breadth was obtained by arbitrarily setting a threshold of 80% of maximum germling length for each height on the shore and location combination. Photophysiological traits were analyzed using ANOVA with location, height on the shore and temperature as fixed factors (with the low fecundity of some combinations requiring that males and females were pooled). Univariate ANOVAs were conducted in the PERMANOVA routine of Primer-E (v6) and the proportion of variance explained by each factor calculated by least square estimates of variance components (Anderson et al., 2008). Data was visualized using package ‘ggplot2’ (Wickham, 2009) and post hoc comparisons were conducted in R Studio (version 1.2.5019) using R [R Core Team, 2020, version 3.6.2 (2019-12-12)].
Results
Thermal Exposure
Mean maximum monthly air temperatures recorded at local weather stations were similar at both locations, however minimum temperatures were lower at the non-edge location. The warm-edge location experienced a narrower temperature range, 9.7 to 26.7°C compared to 4.8 to 27.6°C at the non-edge location (Supplementary Figures S1A,B)—a difference between max and min air temperatures of 17.0°C and 22.8°C, at each location respectively (Supplementary Figures S1A,B). The warm-edge location experienced fewer days per year where air temperature exceeded 35°C than the non-edge location (on average ∼1 vs. ∼8 d y–1, respectively; Supplementary Figure S1C). Sea surface temperature recorded between 2003 and 2014 by satellite (MODIS-Aqua3), ranged from 19.4 to 26.7°C at the warm-edge location versus 16.3 to 24.4°C at the non-edge location and were significantly different between locations (F1 = 1619, P < 0.001; Supplementary Figures S1A,B) and months (F11 = 325, P < 0.001; Supplementary Figures S1A,B).
At the local scale, HOBO® sensors recorded similar mean temperatures on the low and high shore at the warm-edge location (21.9 ± 2.8°C vs. 21.5 ± 3.6°C, respectively; Supplementary Figures S2A,C) as well as the non-edge location (16.0 ± 2.4°C vs. 15.3 ± 3.5°C, respectively; Supplementary Figures S2B,D). High shore temperatures were, however, more variable than low shore temperatures at both locations (warm-edge: F1,2074 = 55.89, P = 0.001; non-edge: F1,1960, P = 0.001).
Genetic Diversity and Structure
Null alleles were found in warm-edge populations, Minnie Water (MW) and Angourie (ANG) at low and high tidal heights for locus HB3 but not at any other locations or loci. All analyses were run with and without HB3 and results were consistent, therefore the locus HB3 was kept in subsequent analyses. Linkage disequilibrium was not found for any locus and all loci were in Hardy–Weinberg equilibrium (Supplementary Table S1). Amongst the 235 individuals collected, a total of 28 different alleles were genotyped across 10 loci. Total mean (±SD) number of alleles across all locations and shore heights sampled was 26.00 ± 1.18 where unique alleles were found at ANG high shore (two alleles), MW low shore (one allele), and BB low shore (two alleles) populations (Table 1). The total number of alleles in any single population varied from 24 to 28 and was similar between regions (F1,79 = 0.055, P = 0.820) amongst all locations (F3,79 = 0.106, P = 0.967), and between heights on the shore (Table 1; F1,79 = 0.053, P = 0.814).
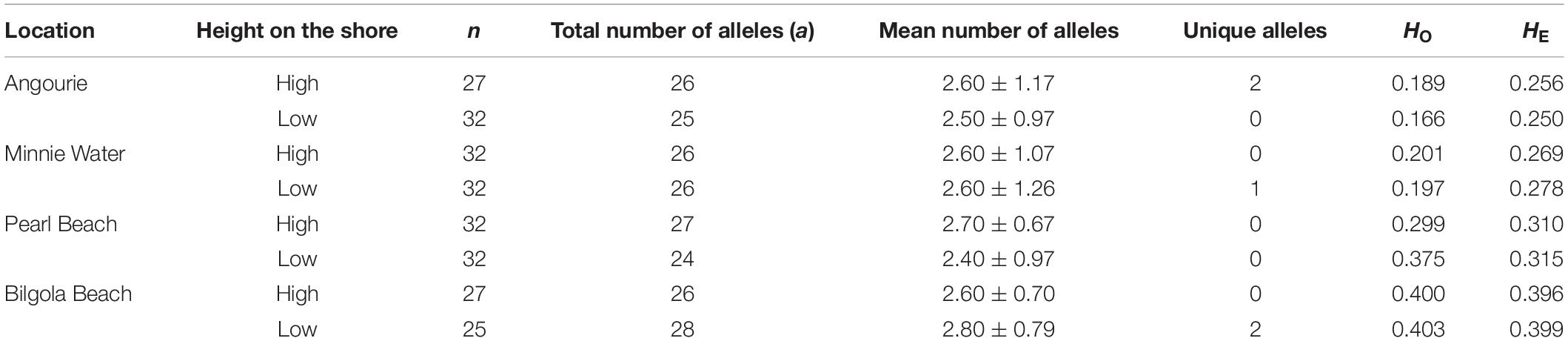
Table 1. The number of individuals sampled (n), total number of alleles (a), mean number of alleles (±SD), unique alleles, observed heterozygosity (HO) and expected heterozygosity (HE) for Hormosira banksii for each height on the shore within each of the four locations.
Genetic diversity was determined by expected heterozygosity (HE) which was lower in warm-edge populations (Table 1 and Supplementary Table S1). A trend for positive (but not significant) FIS values indicated some selfing or inbreeding at both warm-edge and non-edge populations but a significant deviation from random mating (negative FIS value) was only found in low shore populations at Pearl Beach, indicating an excess of heterozygotes (Supplementary Table S2).
The overall FST estimate amongst all spatial scales tested was 0.256 indicating high genetic structure, but genetic structuring diminished from regional to local scales (Table 2). There was pronounced and significant levels of genetic structuring between pairs of non-edge (Bilgola and Pearl Beach) and warm-edge populations (Minnie Water and Angourie) with FST values ranging between 0.274 and 0.413 (Table 2). Among non-edge locations there was moderate and significant genetic structure with pairwise FST values ranging from 0.150 to 0.190 (Table 2). Similarly, there was low but significant genetic structure between locations within the warm-edge region [Minnie Water and Angourie; FST = 0.066 and 0.105 (Table 2)]. Pairwise FST estimates between vertical shore heights were not significantly different at any location (Table 2).
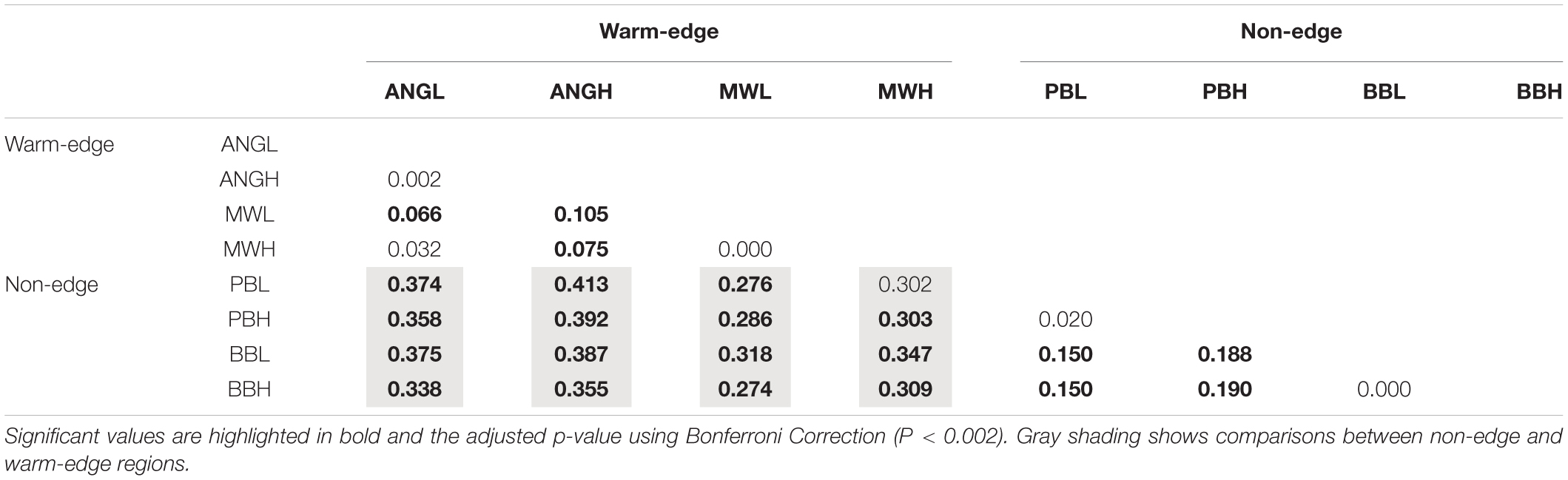
Table 2. Pairwise FST estimates between all pairs of heights on the shore (L and H) and locations Bilgola Beach (BB), Pearl Beach (PB), Minnie Water (MW), and Angourie (ANG).
There was a large (25.7%) and significant amount of genetic variation explained by differences in regions (warm-edge vs. non-edge). Similarly, a large and significant amount of genetic variation (8.0%) occurred between locations within each region, with the largest amount of genetic variation (66.3%) amongst individuals within each location (FST = 0.337, P < 0.001). When shore heights were pooled within each location, a separate AMOVA revealed greater variation was explained at the scale of locations (28.0%) and amongst individuals within each location (72.5%; FST = 0.275, P < 0.001). There were strong significant relationships between geographic and genetic distance across all locations (Mantel test: Z = 2620.41, r = 0.924, P = 0.002, Supplementary Figure S3) but not within non-edge (Mantel test: Z = 7.56, r = 0.976, P = 0.113, Supplementary Figure S3) or warm-edge regions (Mantel test: Z = 6.90, r = 0.831, P = 0.250, Supplementary Figure S3).
Effects of Temperature on Germling Growth and Ontogenesis
Temperature had a strong effect on germling growth, resulting in variable thermal performance curves at each location and height on the shore (Figure 2). Overall, there was a significant location × temperature interaction (15% of the total variation in germling length explained by this interaction) as well as a significant height × temperature interaction (3% of total variation; Table 3) and the interaction between male-female × temperature contribution was 2%. Separate two-factor ANOVAs found significant differences for the main effects of location and height on the shore for each temperature (Supplementary Table S3) with location × height interactions found for germling length at 22, 24, 26, and 30°C (Supplementary Table S3 and Figure 2). Among these interactions, Tukey’s post hoc comparisons were significant between all location × height combinations at each temperature except for some combinations at 22, 26, and 30°C (Table 4).
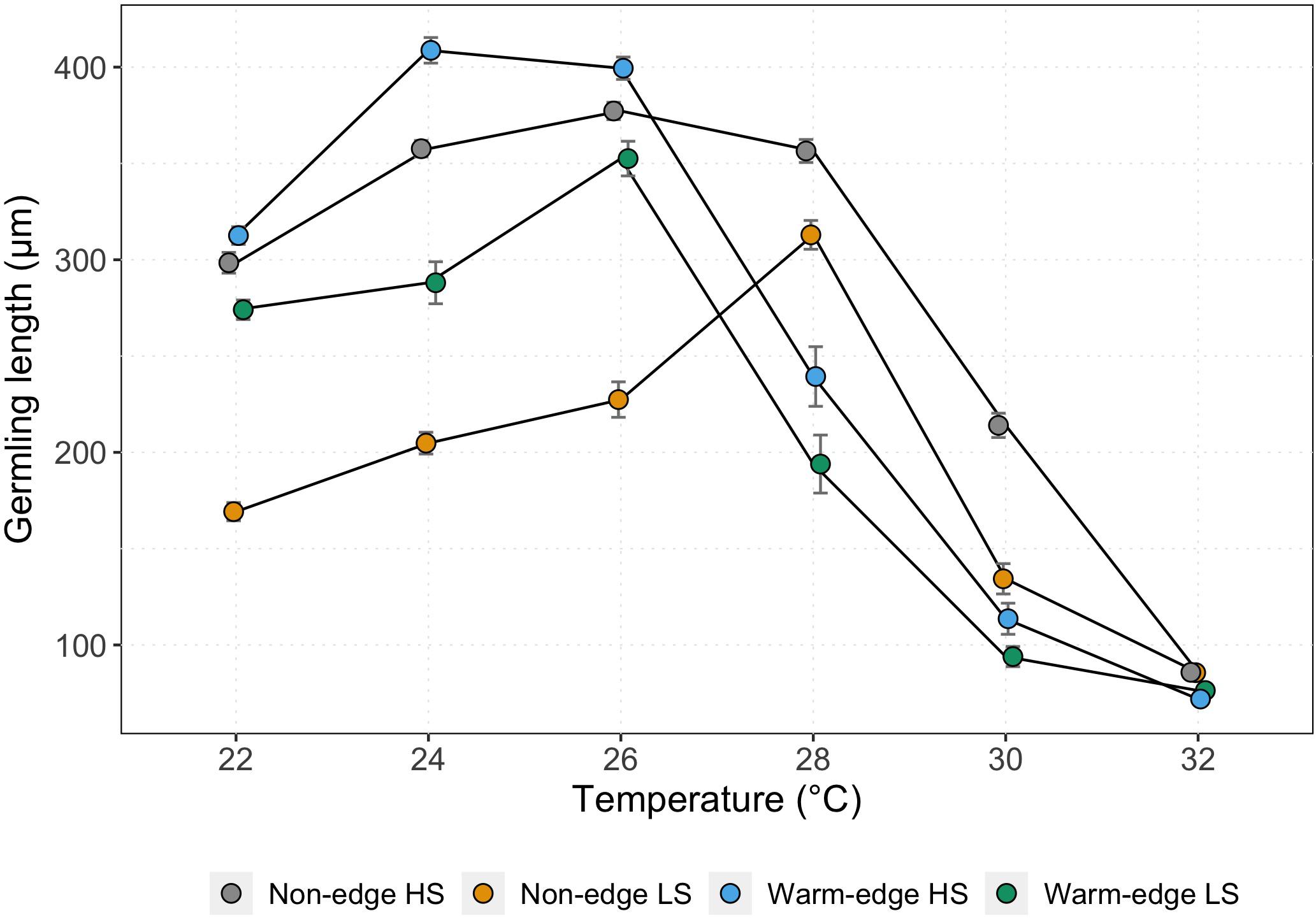
Figure 2. Mean (±SE) germling length after 5 days at six different temperatures (22, 24, 26, 28, 30, and 32°C) from the warm-edge and non-edge populations. Data from all crosses are pooled (n = 54).
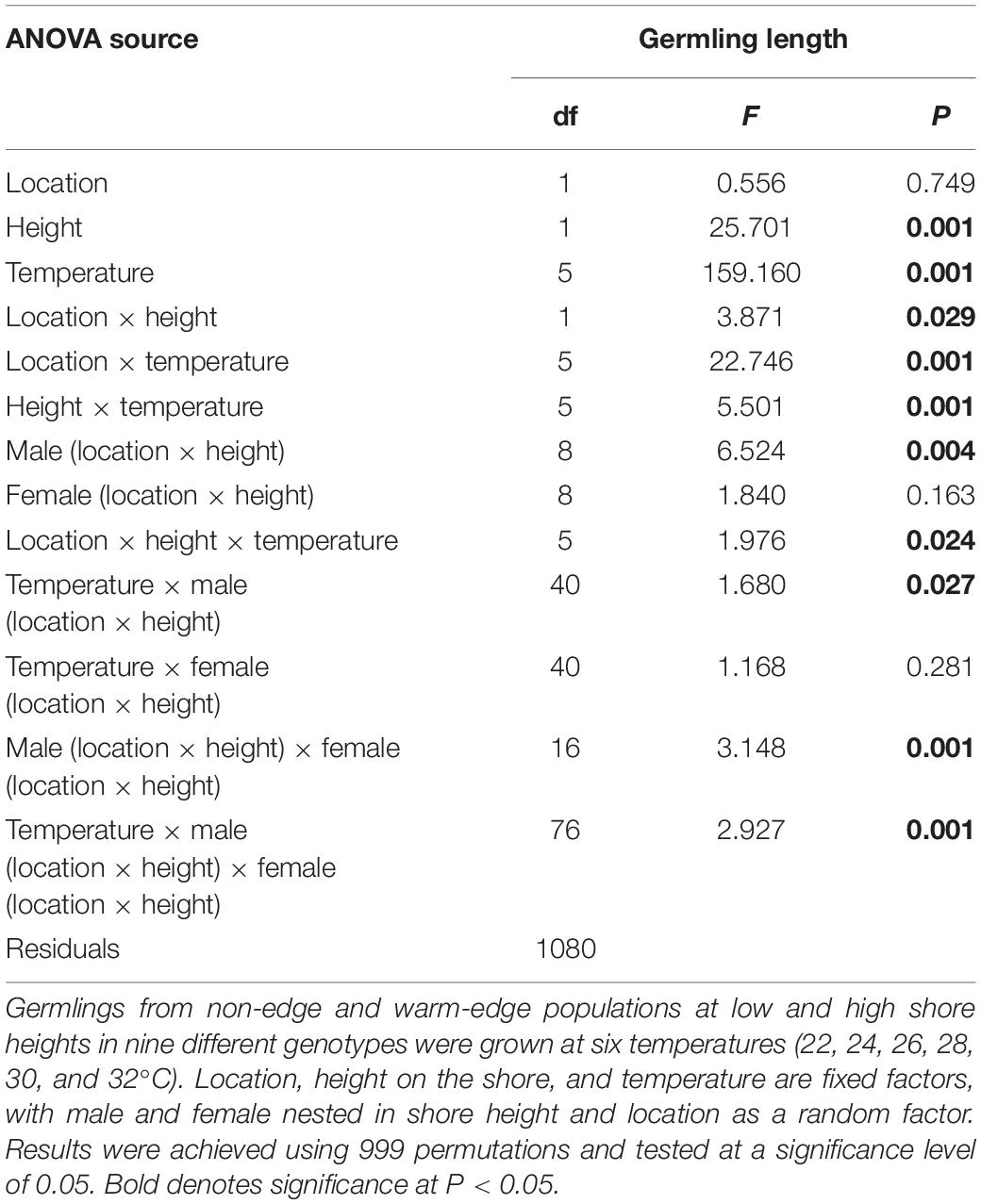
Table 3. Results of analysis of variance of Hormosira banksii germling length 5 days (120 h) post fertilization.
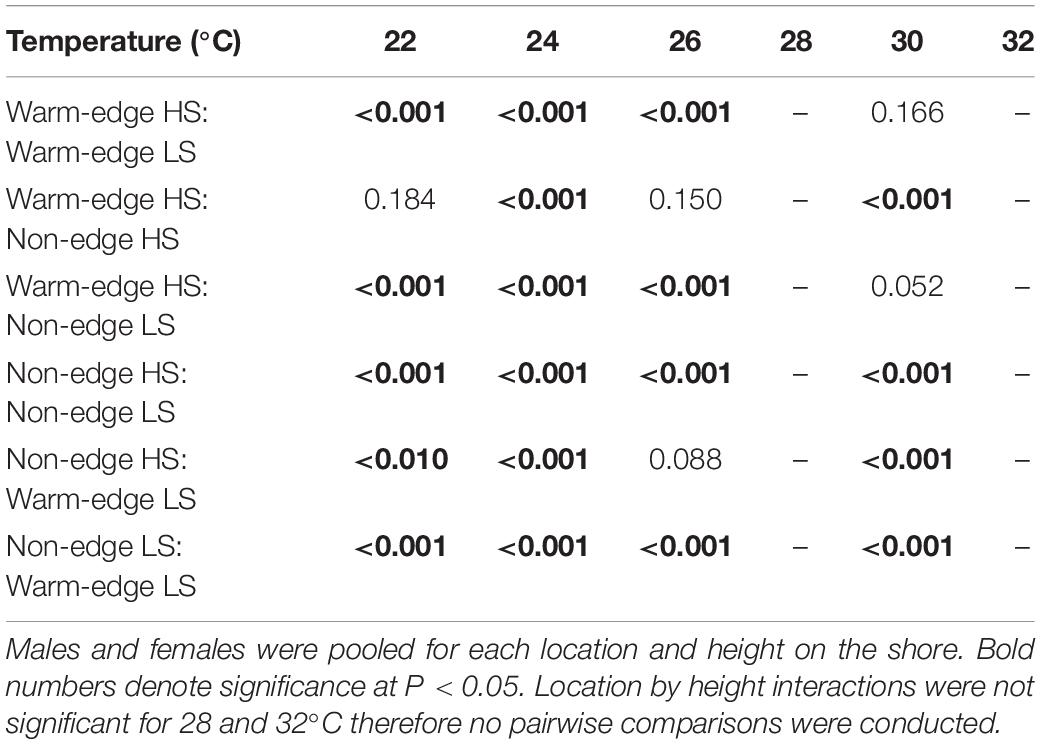
Table 4. Results of Tukey’s HSD post hoc comparisons of germling length (120 h) contrasted amongst different location and heights on the shore; high shore (HS) and low shore (LS) for each temperature.
Thermal breadth, defined as the temperature range in which germling length reached 80% of maximum germling length, varied at each shore height nested in each location. At the warm-edge population (MW) germling lengths from both low and high shore demonstrated greatest growth in the cooler range of temperatures tested (24–26°C). For overall length, warm-edge high shore germlings, were the longest at 24 and 26°C compared to all other heights on the shore tested (mean length ± SD; 408.73 ± 46.03 μm and 399.49 ± 42.61 μm respectively) and declined (decrease of 40%) significantly in temperatures beyond 26°C (22 < 24 = 26 > 28 > 30 > 32°C; P < 0.05, Supplementary Table S4 and Figure 2). Similarly, warm-edge low shore germlings grew optimally at 22 and 26°C (274.03 ± 37.21 μm – 352.52 ± 66.11 μm) with a significant decline (44% decrease) in growth after 26°C (22 = 24 = 26 > 28 > 30 = 32°C; P < 0.01, Supplementary Table S4 and Figure 2). For the non-edge population, germling growth was optimal in the warmer range of temperatures tested. Non-edge high shore germling growth was sustained across a wider thermal breadth between 22–28°C and low shore between 24 and 28°C (Figure 2). Non-edge high shore germlings were the greatest between 24 and 28°C (356.48 ± 43.84 μm – 377.24 ± 33.14 μm) and were significantly longer than other temperatures tested (22 < 24 = 26 = 28 > 30 > 32°C; P < 0.001, Supplementary Table S4 and Figure 2). Non-edge low shore germlings growth peaked at 28°C (204.78 ± 41.44 μm) and were significantly greater than germling length tested at other temperatures (22 < 24 = 26 < 28 > 30 > 32°C; P < 0.05, Supplementary Table S4 and Figure 2). Overall, high shore germlings from the warm-edge and non-edge location, grew faster than the low shore germlings. For all germlings despite location or height on the shore, temperatures beyond 28°C showed significantly reduced growth of germlings with substantial inhibitory effects at 30°C for warm-edge germlings. At the most extreme temperature, 32°C, germlings from the non-edge location grew three-fold to four-fold slower than at their maxima and those from the warm-edge location grew four-fold to five-fold slower.
At the level of the individual, the effect of temperature varied significantly with male identity for germling length for shore height and location (temperature × male interaction; Table 3), which provides evidence of heritable genetic variation in thermal tolerance in different populations of Hormosira banksii. The effect of temperature also varied with parental identity (a significant temperature × male × female interaction, Table 3) with ∼2% of the variance in growth of all germlings attributed to the variation in temperature effects among male/female combinations.
The effect of temperature on the ontogenic development of germlings varied among locations, heights on the shore and among genotypes (Figure 3). Overall, germlings from the warm-edge location developed more rapidly than those from the non-edge location (significant location × temperature interaction for stages 0, 3 and 4, Table 5), with up to 60% of warm-edge germlings reaching stage 3 or 4 after 5 days, in contrast to only 20% from non-edge (Figures 3A,C). The proportion of warm-edge germlings with delayed development (i.e., stage 0) increased steadily in temperatures that surpassed the temperatures optimal for growth (24–26°C) reaching ∼85% at 32°C. At the non-edge location, between 5 and 15% of germlings had delayed development across all temperatures except for high rates of ∼40% at 32°C (Figures 3B,D). Development at 32°C was characterized by enlargement of the germinating cell rather than through cell differentiation and rhizoid development.
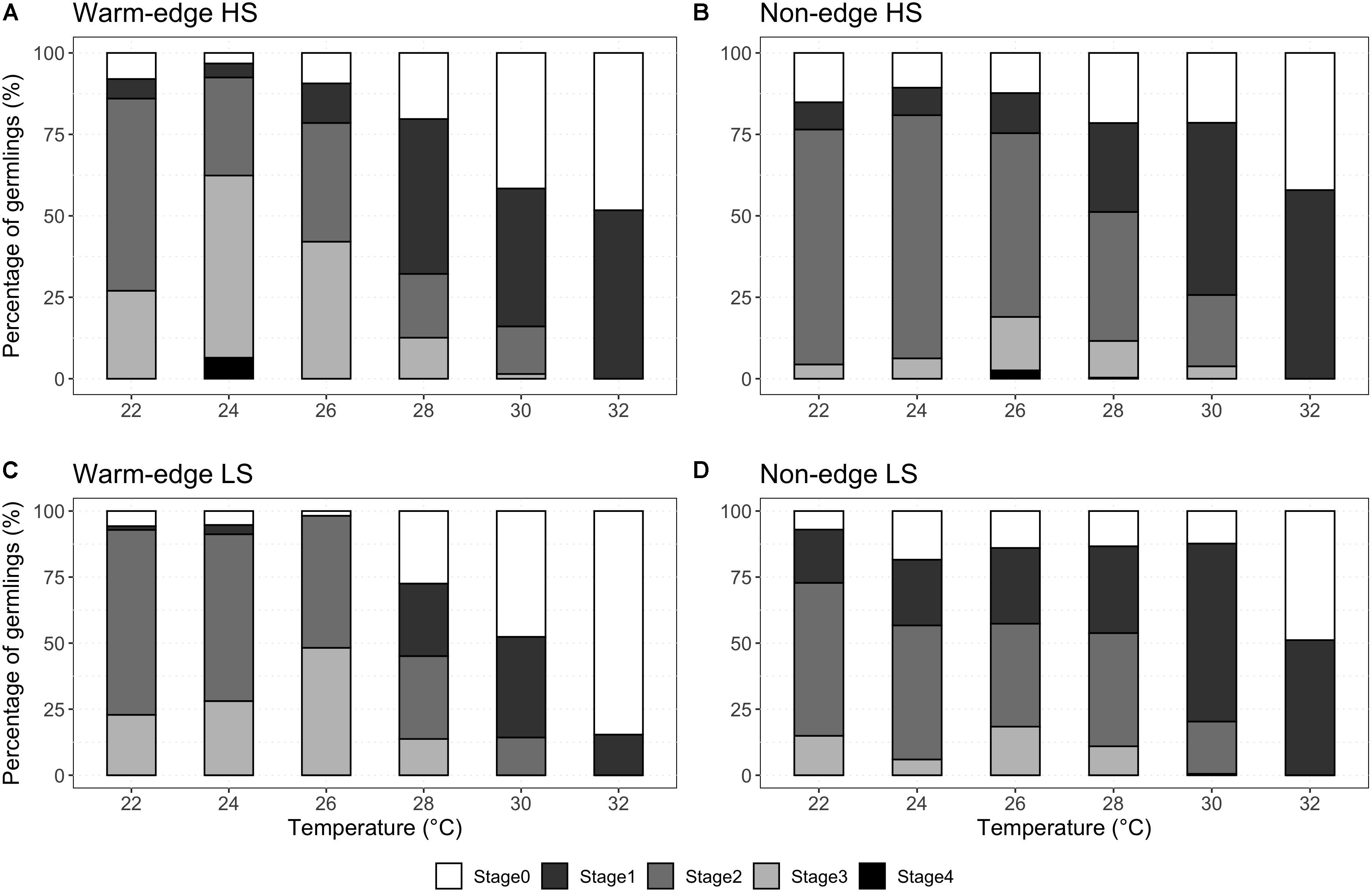
Figure 3. Percentage of H. banksii germlings reaching stage 1, 2, 3, and 4 or remaining in stage 0 after incubation at six different temperatures (22, 24, 26, 28, 30, and 32°C) for 5 days following fertilization. Germlings are from warm-edge (A,C) and non-edge (B,D) populations. White columns represent ontogenic stage 0 (fertilization through condensation of the chloroplasts); dark gray is stage 1 (protrusion of germling cell wall to create a pear shape which later develops into the rhizoid); medium gray is stage 2 (division of the germling germinating cell and elongation of a single rhizoid); light gray is stage 3 (elongation of the rhizoid coupled with secondary and tertiary rhizoid development); and black is stage 4 (paraphysis development on top of the germinating cell). Data represent pooled crosses (n = 40) amongst high shore (HS; A,B), low shore (LS; C,D).
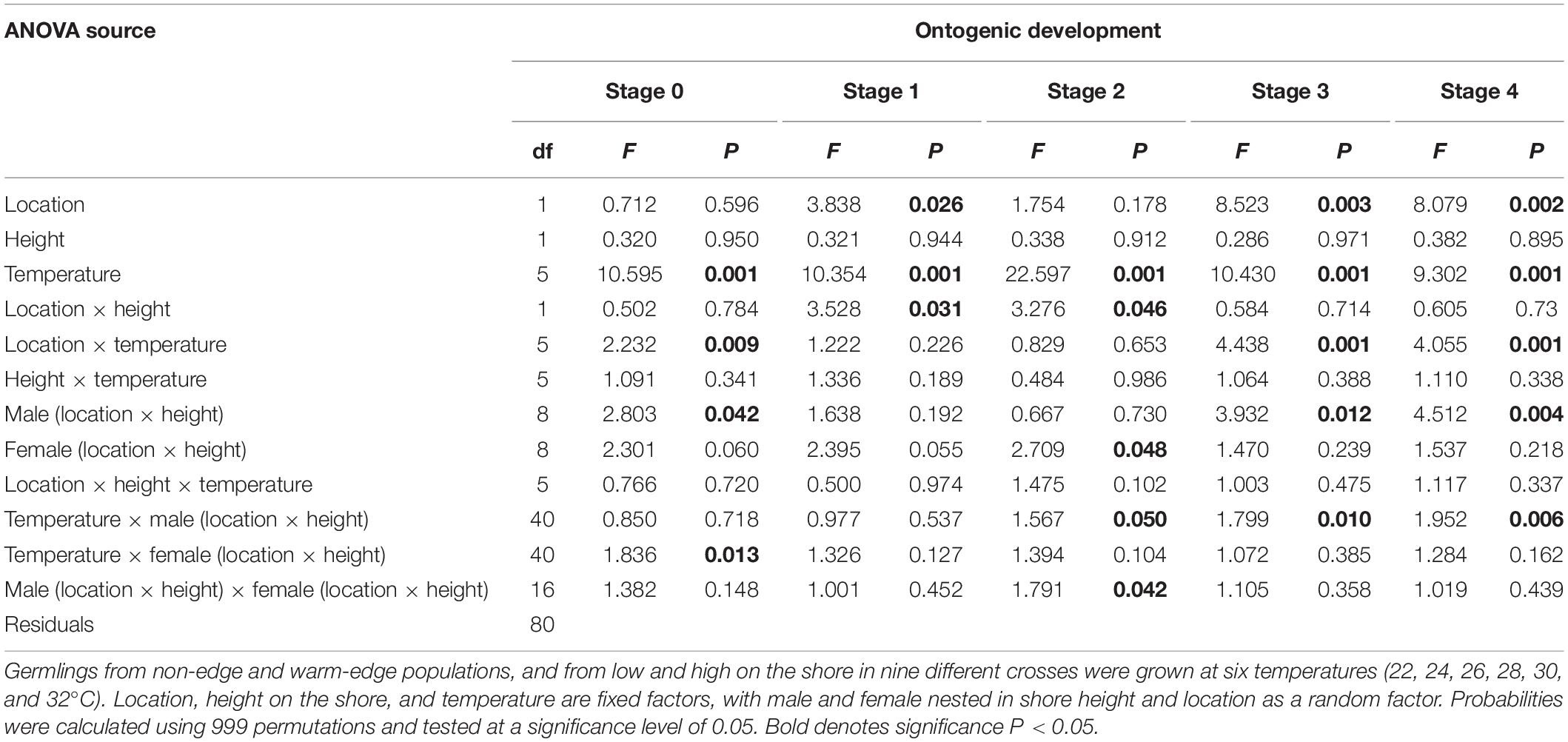
Table 5. Results of analysis of variance of the percent of Hormosira banksii germlings in each developmental stages, (stage 0–4) at 120 h after fertilization.
The proportion of germlings at any stage, or with delayed development in stage 0, did not vary with temperature and height on the shore in either location (non-significant temperature × height on shore interactions, Table 5). However, the effect of temperature varied significantly with male identity for the proportion of germlings in stages 2–4 (genotype by environment interaction; Table 5) indicating that there is heritable genetic variation in the effects of temperature on rates of ontogenic development.
Effects of Temperature on Photophysiological Traits
Temperature had a direct effect on the photochemical efficiency of PSII at a sub-saturating and saturating light intensities [LY(II), HY(II), respectively] amongst all germlings, but there were no significant interactions with location or heights on the shore (Table 6 and Figure 4). Germlings all showed similar photophysiological responses to increasing temperature, with maximum quantum yield and photosynthetic efficiency being relatively constant between 24°C and 28°C and decreasing at 32°C (Figure 4). Maximum quantum yield (FV/FM) was generally greater in warm-edge germlings compared to non-edge germlings but did not differ among heights on the shore or temperature (Figure 4 and Table 6).
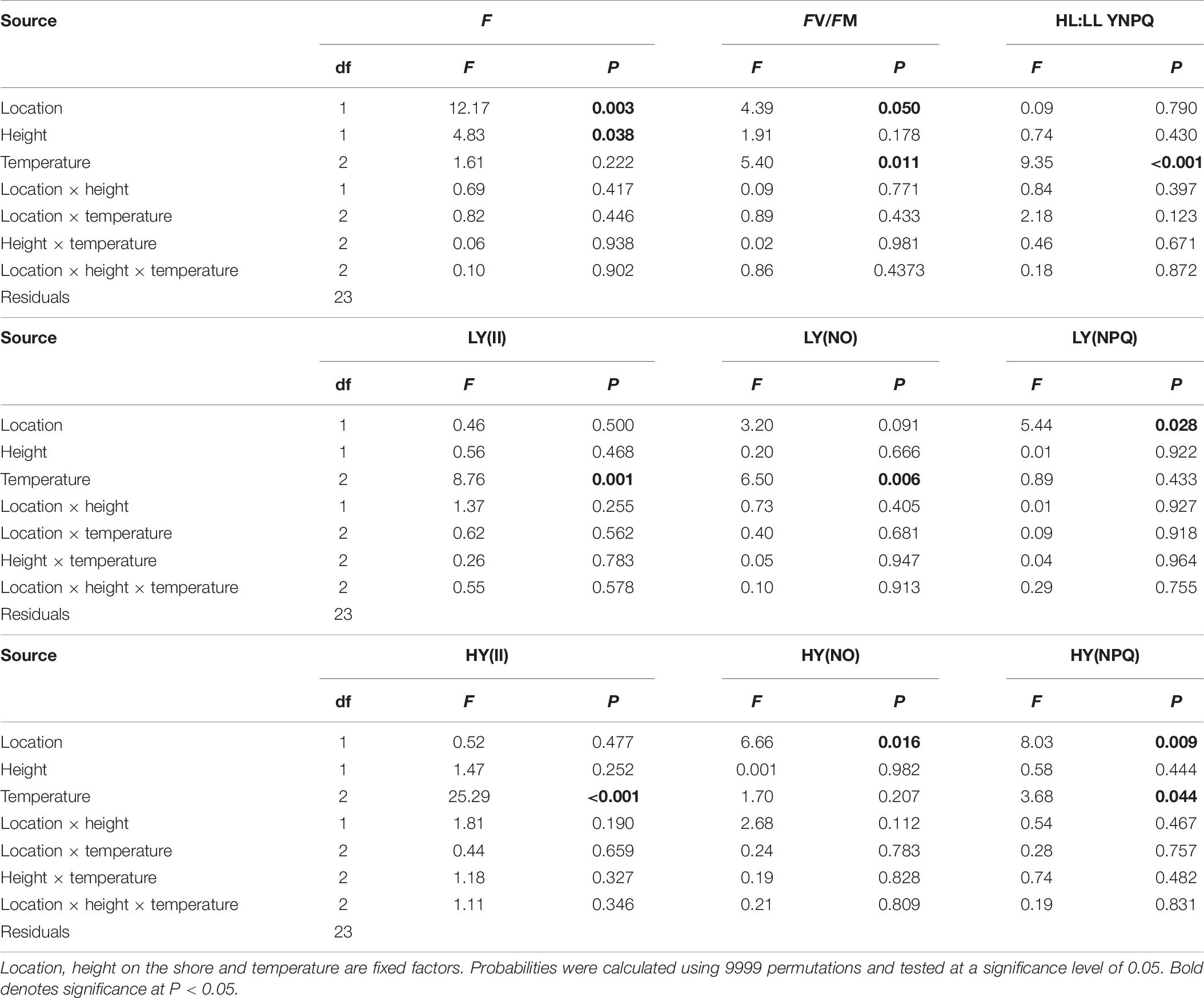
Table 6. Results of analysis of variance of the temperature effects on chlorophyll-a fluorescence F, maximum quantum yield (FV/FM), complementary photosynthetic pathways of photosynthesis Y(II), non-regulated non-photochemical quenching Y(NO), regulated non-photochemical quenching Y(NPQ) and high light (HL) to low light (LL) ratio of Y(NPQ).
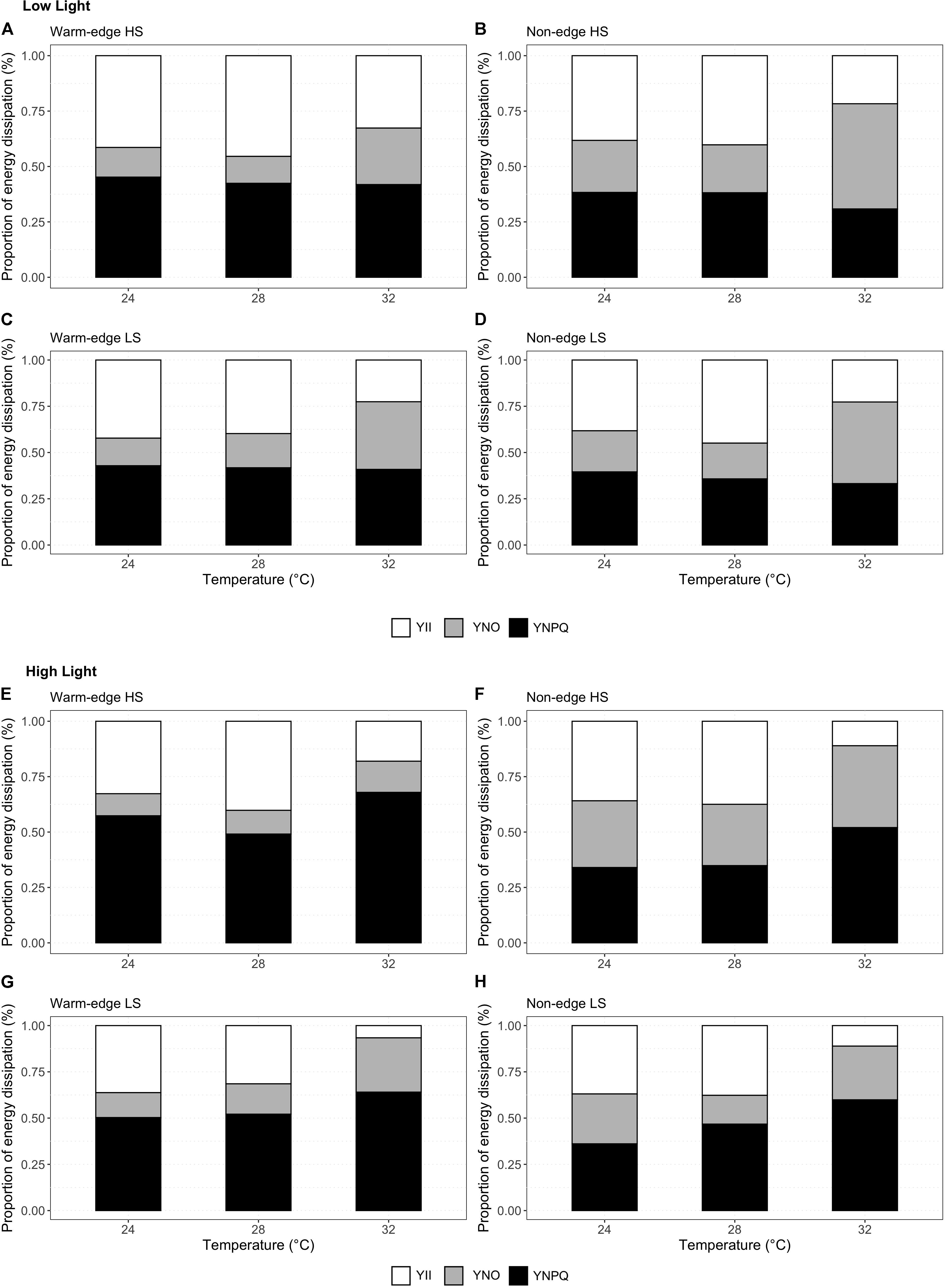
Figure 4. Mean proportion of light energy dissipated by H. banksii germlings amongst three complementary PSII pathways: photochemistry [white bars, Y(II); unregulated non-photochemical quenching, gray bars, Y(NO) or regulated non-photochemical quenching, black bars, Y(NPQ)] and potential photodamage, in germlings from warm-edge (A,C,E,G) and non-edge (B,D,F,H) populations from high on the shore (A,B,E,F) and low on the shore (C,D,G,H) after incubation for 120 h at three temperatures: 24, 28, and 32°C. Photophysiological measurements were made under two irradiances – low light: 32 μmol photons m–2 s–1 (A–D) and high light 113 μmol photons m–2 s–1 (E–H).
High and low shore germlings used regulated non-photochemical quenching [Y(NPQ)] as a means of photoprotection, with Y(NPQ) remaining similar under ambient and high light intensity (Figure 4 and Table 6). However, germlings from the warm-edge location diverted more energy proportionally to Y(NPQ) under high light intensities than those from the non-edge location (Figure 4 and Table 6). This was also evident with the ratio of regulated non-photochemical quenching under ambient and high light (HL: LL Y(NPQ), Figure 4 and Table 6) where the germlings from the warm-edge location had ratios above 1 (indicating increased regulated quenching of energy at saturating light intensity) compared to germlings from the non-edge location which had ratios below 1 (indicating increased unregulated quenching, or potential photodamage under high light). Baseline fluorescence (Ft), a proxy for photosynthetic pigment content, was significantly higher in the germlings from the non-edge location and those from high on the shore (Table 6). For all photophysiological traits, there were no significant interactions between temperature and location or height on the shore, indicating that germling responses to temperature did not vary at regional and local scales (Table 6).
Discussion
Populations inhabiting the warm range edge of distributions are suggested to be at the forefront of climate change. The increasing prevalence of extreme climate events such as heatwaves may challenge marine macroalgal dominated populations that have limited physiological plasticity to tolerate prolonged, elevated temperatures or reduced capacity to adapt (Wernberg et al., 2018; Gurgel et al., 2020). In this study, germlings of the dominant intertidal macroalga, Hormosira banksii, demonstrated variable thermal performance curves which was governed by the thermal environment from where they originated, locally on the vertical shore, rather than their relative distributional range origin. Warm-edge germlings had greater growth rate and development in lower and narrower range of temperatures tested and indicated sensitivity to higher temperatures compared to non-edge germlings, which grew optimally across a broader range of temperatures. Relative position on the vertical shore, had a greater influence on thermal physiology and breadth. This was demonstrated by variable thermal breadths across different heights on the shore and the ability of germlings to grow optimally in different temperatures. Warm-edge germlings, however, had greater capacity to regulate excess energy as non-photochemical quenching [Y(NPQ)] when exposed to greater temperature and light, suggesting they are less photophysiologically sensitive than non-edge population germlings. Evidence of heritable genetic variation (significant genotype by environment interaction) found for growth and development indicate that there is potential for adaptation in thermal tolerance traits. These physiological responses coincided with lower genetic diversity, restricted gene flow and evidence of inbreeding at warm-edge populations. This suggests that warm-edge germlings utilize physiological plasticity to tolerate short-term exposure (hours-days) to environmental stressors but over longer time scales (years) may potentially be less thermally buffered and at greater risk to global warming.
Thermal Effects of Physiology
Thermal history at study locations played an important role in governing thermal tolerances in H. banksii germlings. Warm-edge germlings grew optimally and developed faster in the cooler, narrower range of temperatures tested (22–26°C), compared to non-edge germlings that grew in a broader range of temperatures (22–28°C). Although previous research found that 90% of seaweeds displayed population level variation in upper thermal limits (King et al., 2017), which agrees with our findings, the result of increased thermal sensitivity to high temperatures for warm-edge germling growth is contrary to previous research. Many studies on marine macrophytes and invertebrates in lower latitudes were found to be more tolerant of higher temperatures, as they generally experience greater temperatures throughout their life history (Gerard and Du Bois, 1988; Stillman and Somero, 2000; Kelly et al., 2012; Sunday et al., 2012; Mota et al., 2018). Air temperature data collected from local meteorological stations demonstrated that the warm-edge population (Minnie Water) experience similar annual maximum monthly temperatures as the non-edge population (Pearl Beach), but warmer minimum monthly temperatures, less seasonal variation and fewer days over 35°C. This would suggest that the warm-edge population should also be more thermally tolerant. However, previous research on the effects of desiccation stress on adult thalli of H. banksii from the same warm-edge site (Minnie Water), adults were also more thermally sensitive to higher temperatures (Clark et al., 2018). Further, previous research also found a warm-edge macroalgal population was not more thermally tolerant than cooler populations and proposed that the warm-edge population was thermally maladapted (Pearson et al., 2009). The low gene flow and low genetic diversity found in the warm edge populations of this study may support local adaptation to conditions (discussed in section “Genetic Diversity and Structure”), however, physiological adaptation and local site effects may also play an important role in shaping thermal performance (discussed further in this section).
Our result of narrower thermal breadth of warm-edge population is consistent with the climate variability hypothesis of narrower thermal breadth toward lower latitudes (Stevens, 1989). However, a recent study of a non-edge population of a subtidal macroalgal species (Scythothalia dorycarpa) had similar thermal safety margins (defined as, ‘the temperature buffer between an organisms upper thermal-tolerance limit and the maximum ambient temperatures it experiences’) to warm-edge populations but different absolute temperature tolerances, which demonstrated that not all species at distributional limits have a narrower thermal breadth (Bennett et al., 2015). One explanation for our result of different thermal breadths for both populations is that intertidal species are exposed to dynamic environmental stress imposed by the terrestrial and marine environment, opposed to constantly being submerged, therefore differences such as emersion and air temperature variation may be more important in shaping thermal niche. The significant difference in thermal breadth in non-edge high and low shore germlings illustrates how local scale effects in the intertidal can influence thermal performance.
Despite locational differences in thermal regimes among locations, germlings of H. banksii demonstrated similar photophysiological responses to elevated temperatures. Divergence among locations in the ability to tolerate greater light intensity, however, was found for dissipation of excess energy. The lack of any interactions between temperature and location for photophysiological parameters in H. banksii suggests that germlings have a high degree of plasticity, and can adjust their photosystems to tolerate differences in light and temperature regimes. Despite significant reductions of growth at 28°C for warm-edge germlings and 30°C for non-edge germlings, H. banksii was still able to maintain a high level of PSII efficiency in ambient and high light intensities across 24 and 28°C, suggesting acclimation of photosystems (Major and Davison, 1998). This result is consistent with previous studies which also found no significant temperature interactions in photosynthetic response of macroalgae (Clark et al., 2013; McCoy and Widdicombe, 2019). The adjustment of photosystems to different temperatures and light intensities to optimize photosynthesis may be an important trait for intertidal macroalgae as temperature and light gradients can change rapidly with wave action and tidal cycles. Furthermore, in locations closer to the equator, light intensity is greater seasonally, therefore warm-edge germlings may be able to tolerate higher light intensity through phenotypic plasticity indicated by more energy being dissipated via Y(NPQ) rather than Y(NO), whereas non-edge germlings are more light sensitive indicated by the greater proportion of Y(NO). This may also explain the greater growth reduction at higher temperatures for germlings in the warm-edge location as more energy is being diverted toward photoprotection rather than to photochemistry. In sporophytes of the subtidal kelp Ecklonia radiata, physiological performance was maintained in higher temperatures through an increase in critical light demand (EC) (Staehr and Wernberg, 2009). This reduction allowed for similar levels of light limited photosynthesis to be achieved in warm and cool adapted populations found at different latitudes, consistent with this study.
Growth of germlings from low and high on the shore was also affected by differences in local temperatures, indicated by significant interactions between height on the shore and temperature. Temperatures recorded by HOBO pendants in the high shore at both locations were significantly more variable than low shore temperatures. These results are consistent with a growing body of research that suggests that local scale topography and environmental conditions may be more important in driving physiology and species’ distributions than larger regional effects of climate (Helmuth et al., 2002, 2006; Helmuth, 2009). For example, local scale topography and environmental conditions experienced by individuals of the intertidal mussel Mytilus californianus can result in body temperatures varying between 6 to 13°C within a population at a given time (Helmuth and Hofmann, 2001; Harley, 2008). Consequently, temperatures experienced by individuals may not be easily predicted by larger scale variation in temperatures (e.g., among latitudes), but instead be a mosaic of smaller scale hot and coldspots. In this study, the warm-edge location is characterized by large boulders that can shade H. banksii and trap small pools of water, potentially reducing the stress experienced by individual thalli in contrast with temperatures experienced on flatter rock platforms such as at the non-edge location. The shore topography at the warm-edge location could thus modify the thermal exposure of individuals and lead to similar growth rates of germlings from low and high on the shore as found in this study.
Maintaining thermal tolerance across broader temperatures can be physiologically costly, therefore germlings may not grow optimally across all temperatures (Huey et al., 2012). This is demonstrated by differences in optimal temperatures for germling growth and may reflect increased energy dissipation (i.e., non-photochemical quenching) and decreased photochemistry (YII) with increased temperatures and light intensities likely experienced for longer periods during low tide high on the shore (Davison and Pearson, 1996). In addition, the reduced growth and narrow thermal optima amongst low shore germlings in both populations may reflect light limited photosynthesis of adults as they experience longer periods spent submerged compared to those on the high shore, while optimizing growth within a narrow range of temperature that they most commonly experience (Huey et al., 2012). There were no significant interactions between temperature and height on the shore for photosynthetic parameters, suggesting phenotypic plasticity for these photophysiological traits. Given that intertidal macroalgae at different heights on the shore must contend with dynamic variation in light and temperature during daily tidal cycles, it suggests that photosystems need to be able to rapidly acclimatize to different light and temperature regimes (Hanelt et al., 1993). Over longer time scales, adaptation of the population at the local scale involving genotypes tolerant to the prevailing thermal and light regime may also be important (Hanelt et al., 1993; Al-Janabi et al., 2019).
The potential for adaptation in temperature tolerance traits amongst H. banksii germlings is indicated by a significant male × temperature interaction for germling length and ontogenic development. This is consistent with earlier investigations of this species (Clark et al., 2013) and suggests that as temperatures increase with global warming, genotypes that are better able to tolerate higher temperatures will be favored (Deutsch et al., 2008; Sunday et al., 2012; Fusi et al., 2015). This will be particularly important for populations that have limited gene flow such as the warm-edge population. A significant interaction between female identity and temperature was also found for the proportion of germlings that did not develop (stage 0), suggesting a role for either female genotype or non-genetic maternal effects in thermal responses. Maternal effects have been identified previously in different organisms (e.g., bryozoans, Marshall, 2008; terrestrial plants, Galloway et al., 2009; sea urchins, Foo et al., 2012; fish, Chambers and Leggett, 2015) and are potentially relevant in H. banksii where egg size differs among different females (Clark, 2016). Maternal environment may impact the resources available for reproduction which can affect egg size and growth trajectory of offspring (Wolf and Wade, 2009). The significant interaction between temperature and parental identity (i.e., male × female × temperature) suggests that different genotypes are more susceptible to different temperatures. There were no interactions between temperature and male or female identity for any of the photosynthetic parameters, suggesting that photosynthesis is highly regulated amongst individuals. This agrees with previous studies in which no heritable genetic variation was found in H. banksii photosynthetic traits (Clark et al., 2013).
Genetic Diversity and Structure
Consistent with previous studies (Coleman et al., 2011a, 2019; Miller et al., 2019) we found strong genetic structure between the warm-edge and non-edge regions (∼500 km apart) as well as isolation by distance suggesting that dispersal capacity is limited across long distances as well as between neighboring populations (>50 km). Moreover, trends for lower estimates of genetic diversity toward distributional edges found in this study is in accordance with previous studies of H. banksii across a longitudinal gradient (Miller et al., 2019) and other macroalgal species (Faugeron et al., 2004; Teixeira et al., 2016; King et al., 2017; Wernberg et al., 2018). The observed patterns of lower genetic diversity at warm-edge populations is suggested to be the result of reduced gene flow and connectivity which can create isolation among populations and reduce within population genetic diversity (Hampe and Petit, 2005). In addition, as distributional limits often represent the physiological limits of a species, environmental conditions can impose strong selection pressure resulting in decreased diversity as environmental conditions and habitat become suboptimal with only tolerant genotypes and phenotypes persisting at range edges. While we cannot tease apart these mechanisms with the neutral markers used here, the early life stages of H. banksii from warm-edge populations had a narrower range of thermal performance compared to populations found within the center of its distribution suggesting that reduced genetic diversity may constrain responses. With lower genetic diversity, the warm-edge population may not have the range of the functional responses such as greater tolerance for higher temperature, however, greater regulated non-photochemical protection in warm-edge germlings, suggests that this population may have greater phenotypic plasticity to tolerate dynamic light conditions.
Moderate gene flow is evident between neighboring H. banksii populations within each warm-edge and non-edge region separated by <50 km. Dispersal of gametes or zygotes is not a likely method of long-distance dispersal as fertilized zygotes sink to the substrate and adhere within hours of fertilization (Dimartino et al., 2015). Rather, rafting of buoyant dislodged adult thalli which drift with ocean currents with the aid of air bladders or vesicles has been suggested as the most likely method of long-distance dispersal and has been evident amongst different macroalgal species (Muhlin et al., 2008; Valero et al., 2011; Bussolini and Waters, 2015; Coleman et al., 2019). There is limited empirical evidence that supports whether floating thalli contribute to long distance gene flow in H. banksii, however, a recent study suggests that floating thalli can end up in estuaries where they can grow and survive (Coleman et al., 2019). The moderate but significant levels of genetic structure between neighboring populations within each non-edge and warm-edge region may show restriction in gene flow possibly due to the existence of physical barriers such as sandy beaches, and mouths of estuaries which may serve as barriers to gene flow in other macroalgae (Billot et al., 2003; Coleman, 2013).
Within smaller scales (within 5–10 m), gene flow of H. banksii was not restricted between vertical heights on the intertidal shore which agrees with other studies on macroalgae (Engel et al., 2004; Tatarenkov et al., 2005; Teixeira et al., 2016; Bellgrove et al., 2017). The intertidal is characterized by steep environmental gradients suggesting selection for stress-tolerant genotypes on high shores may be an important driver of genetic structure. This has been demonstrated amongst barnacles (Schmidt and Rand, 2001), gastropods (Johannesson et al., 1995) as well as amongst hybrids of the macroalgae Fucus vesiculosus and Fucus spiralis (Billard et al., 2010; Zardi et al., 2011). Nonetheless, the lack of small-scale genetic structure between shore heights found in this study, suggests that gene flow is unobstructed and that H. banksii zygotes and gametes may be readily dispersed across these smaller distances (Dudgeon et al., 2001). Studies on the attachment strength of H. banksii zygotes have found that adhesion to the substrate is not at maximum strength until 24 h after fertilization suggesting that zygotes could potentially be dislodged and recruit elsewhere (Dimartino et al., 2015). Specific habitat types related to strong environmental gradients within the intertidal have been found to influence phenotypic divergence independently of genetic structure (Engel et al., 2004; Zardi et al., 2013). Lack of differences at small scales suggest that H. banksii may survive living in different environmental gradients through phenotypic plasticity rather than genetic differentiation as documented in F. vesiculosus (Zardi et al., 2013). This suggests that thermal exposure within the intertidal may not necessarily select for different genotypes but perhaps genotypes that are highly plastic. An alternative explanation is that adaptive genetic differentiation between tidal heights may exist, but is not apparent in our neutral markers (which only show variation due to dispersal and connectivity, not selection). Testing this idea would require use of markers such as SNPs which examine portions of the genome under selection.
Genetic diversity was found to be lower at warm-edge populations. This is not surprising as these populations are at the edge of their equatorward distribution, where populations are more fragmented, conditions are not optimal and macroalgal populations are therefore at their physiological threshold. The lower genetic diversity found at these populations suggests that these populations may lack the potential to adapt to future warming and be particularly vulnerable to extreme climate events (i.e., heat waves). Previous studies have already shown local extinction in warm-edge populations of macroalgal populations with extreme climate events which may be a consequence of a smaller gene pool (Araújo and Williams, 2001; Smale and Wernberg, 2013; Wernberg et al., 2018).
Conclusion
The results of this study provide evidence that germlings of H. banksii inhabiting populations within the warm-edge of its distribution may at risk to increases in temperatures associated with global warming (Figure 5). The sensitivity to higher temperatures tested, narrower thermal breadth as well as relatively low genetic diversity and limited gene flow are all indications of populations that are vulnerable to warming (Pearson et al., 2009; Mota et al., 2018; King et al., 2019). Significant genotype by environment interactions found for growth and ontogenic development suggests that there is heritable genetic variation in growth and development under different temperatures, which could be important particularly for the warm-edge populations with lower genetic diversity and gene flow. Our experimental data show that these warm-edge populations may also be surviving through phenotypic plasticity by obtaining similar levels of photochemistry through greater levels of regulated non-photochemical quenching (photoprotection) at higher light and temperature than non-edge population. However, over the long-term the genetic impoverishment and reduced gene flow may be problematic as global warming and extreme climate events continue to push species past their physiological limits. The prevalence of greater number of hot days (days over 35°C) in non-edge populations, suggests that the non-edge population may be at risk to habitat fragmentation. Greater tolerance to higher temperatures as well as the significant genotype × environment interactions suggest that non-edge populations may be locally adapted to local environmental conditions and have heritable genetic variation in thermal tolerance traits. Further greater genetic diversity and gene flow suggests that the non-edge population have greater connectivity and therefore available for genetic rescue from surrounding populations.
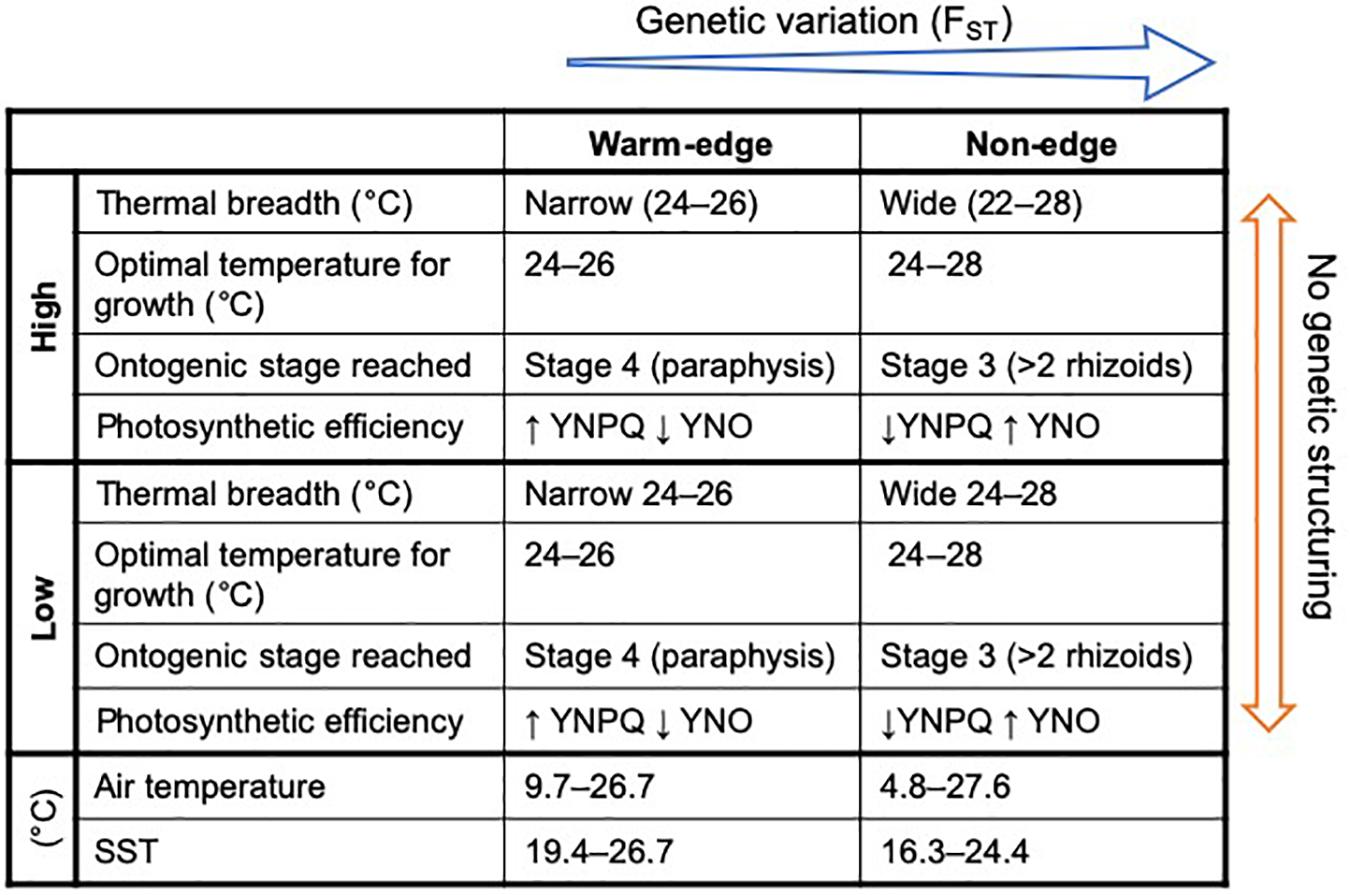
Figure 5. Conceptual model of summarized data indicating how physiological traits differed amongst high and low on the shore as well as between warm-edge and non-edge locations. Thermal breadth was determined through arbitrarily setting a threshold of 80% of maximum germling length. Optimal temperature for growth was determined through pairwise comparisons. Ontogenic staged reached was the furthest developmental stage reached within 120 h. Photosynthetic efficiency stated are the overall changes in the proportion of energy dissipated in to unregulated non-photochemical quenching [Y(NO)] or regulated non-photochemical quenching [Y(NPQ)] at both light intensities. Temperature data (air and SST) are presented for each location only.
Contrasting the relative magnitude of within-population variation to variation in thermal responses on larger spatial scales, this study shows that the interaction between temperature and location comprised an effect size of 15% of the total variation in growth, the interaction between temperature and heights on the shore had an effect size of 3%, and the interaction between temperature and male-female combination had an effect size of 2%. This within-population variation in thermal tolerance will be particularly important under a changing climate as populations with greater diversity will have a broader suite of tolerant genotypes for selection to act upon (Reusch, 2014; Wernberg et al., 2018). The results of this study and previous research on genetic diversity of H. banksii across its species distribution (Miller et al., 2019) has helped improve predictions of how this species will respond to ongoing warming and identified potentially sensitive populations. Conservation efforts such as transplanting tolerant individuals or reseeding to increase genetic diversity in genetically impoverished populations may aid in providing greater functional resilience to warming climates (Campbell et al., 2014; Wood et al., 2019; Fredriksen et al., 2020).
Data Availability Statement
The raw data supporting the conclusions of this article will be made available by the authors, without undue reservation.
Author Contributions
JC conceived the idea for the manuscript, collected the data, curated and formally analyzed the data, and wrote the original manuscript. MD, AP, and MC supervised the project, reviewed, and wrote and edited the manuscript. MD collected some of the data and provided resources for the project. All authors contributed to the article and approved the submitted version.
Funding
This research was supported by the University of Technology Sydney Climate Change Cluster (C3), and an Australian postgraduate award to JC. Samples were collected under the Department of the Environment and Heritage permit number P10/0057-2.0.
Conflict of Interest
The authors declare that the research was conducted in the absence of any commercial or financial relationships that could be construed as a potential conflict of interest.
The reviewer CL declared a past co-authorship with one of the authors MC to the handling editor.
Acknowledgments
The authors would like to thank Daniel Lewis for assistance in the field and sample collections and Kevin Davies for providing Figure 1.
Supplementary Material
The Supplementary Material for this article can be found online at: https://www.frontiersin.org/articles/10.3389/fmars.2020.00711/full#supplementary-material
Footnotes
References
Al-Janabi, B., Wahl, M., Karsten, U., Graiff, A., and Kruse, I. (2019). Sensitivities to global change drivers may correlate positively or negatively in a foundational marine macroalga. Sci. Rep. 9:14653. doi: 10.1038/s41598-019-51099-8
Allakhverdiev, S. I., Kreslavski, V. D., Klimov, V. V., Los, D. A., Carpentier, R., and Mohanty, P. (2008). Heat stress: an overview of molecular responses in photosynthesis. Photosynth. Res. 98, 541–550. doi: 10.1007/s11120-008-9331-0
Anderson, M., Gorley, R., Clarke, K., Anderson, M., Gorley, R., Clarke, K., et al. (2008). PERMANOVA+ for PRIMER. Guide to Software and Statistical Methods. Plymouth: PRIMER-E, Ltd.
Araújo, M. B., and Williams, P. H. (2001). The bias of complementarity hotspots toward marginal populations. Conserv. Biol. 15, 1710–1720. doi: 10.1046/j.1523-1739.2001.99450.x
Araújo, R., Serrão, E. A., Sousa-Pinto, I., and Åberg, P. (2011). Phenotypic differentiation at southern limit borders: the case study of two fucoid macroalgal species with different life-history traits: phenotypic differentiation at southern limit borders. J. Phycol. 47, 451–462. doi: 10.1111/j.1529-8817.2011.00986.x
Belkhir, K., Borsa, P., Goudet, J., Chikhi, L., and Bonhomme, F. (2000). GENETIX, version 4.05. Laboratoire Genome, Populations. Interactions, CNRS UPR 9060. Montpellier: Université de Montpellier II.
Bellgrove, A., Clayton, M. N., and Quinn, G. P. (1997). Effects of secondarily treated sewage effluent on intertidal macroalgal recruitment processes. Mar. Fresh. Res. 48:137. doi: 10.1071/MF96011
Bellgrove, A., van Rooyen, A., Weeks, A. R., Clark, J. S., Doblin, M. A., and Miller, A. D. (2017). New resource for population genetics studies on the Australasian intertidal brown alga, Hormosira banksii: isolation and characterization of 15 polymorphic microsatellite loci through next generation DNA sequencing. J. Appl. Phycol. 29, 1721–1727. doi: 10.1007/s10811-016-1015-0
Bennett, S., Duarte, C. M., Marbà, N., and Wernberg, T. (2019). Integrating within-species variation in thermal physiology into climate change ecology. Philos. Trans. R. Soc. B Biol. Sci. 374:20180550. doi: 10.1098/rstb.2018.0550
Bennett, S., Wernberg, T., Arackal Joy, B., de Bettignies, T., and Campbell, A. H. (2015). Central and rear-edge populations can be equally vulnerable to warming. Nat. Commun. 6:10280. doi: 10.1038/ncomms10280
Billard, E., Serrão, E., Pearson, G., Destombe, C., and Valero, M. (2010). Fucus vesiculosus and spiralis species complex: a nested model of local adaptation at the shore level. Mar. Ecol. Prog. Ser. 405, 163–174. doi: 10.3354/meps08517
Billot, C., Engel, C., Rousvoal, S., Kloareg, B., and Valero, M. (2003). Current patterns, habitat discontinuities and population genetic structure: the case of the kelp Laminaria digitata in the English Channel. Mar. Ecol. Prog. Ser. 253, 111–121. doi: 10.3354/meps253111
Bishop, M. J., Morgan, T., Coleman, M. A., Kelaher, B. P., Hardstaff, L. K., and Evenden, R. W. (2009). Facilitation of molluscan assemblages in mangroves by the fucalean alga Hormosira banksii. Mar. Ecol. Prog. Ser. 392, 111–122. doi: 10.3354/meps08247
Blacket, M. J., Robin, C., Good, R. T., Lee, S. F., and Miller, A. D. (2012). Universal primers for fluorescent labelling of PCR fragments-an efficient and cost-effective approach to genotyping by fluorescence: universal primers for fluorescent genotyping. Mol. Ecol. Resour. 12, 456–463. doi: 10.1111/j.1755-0998.2011.03104.x
Burrows, M. T., Schoeman, D. S., Richardson, A. J., Molinos, J. G., Hoffmann, A., Buckley, L. B., et al. (2014). Geographical limits to species-range shifts are suggested by climate velocity. Nature 507, 492–495. doi: 10.1038/nature12976
Bussolini, L. T., and Waters, J. M. (2015). Genetic analyses of rafted macroalgae reveal regional oceanographic connectivity patterns. J. Biogeogr. 42, 1319–1326. doi: 10.1111/jbi.12491
Campbell, A. H., Marzinelli, E. M., Vergés, A., Coleman, M. A., and Steinberg, P. D. (2014). Towards restoration of missing underwater forests. PLoS One 9:e84106. doi: 10.1371/journal.pone.0084106
Chambers, R. C., and Leggett, W. C. (2015). Maternal influences on variation in egg sizes in temperate marine fishes. Am. Zool. 36, 180–196. doi: 10.1093/icb/36.2.180
Clark, J. S. (2016). Assessing the Vulnerability of a Habitat Forming Macroalga to Climate Warming: Roles of Physiology, Ecology and Evolutionary Processes in Determining Resilience. PhD Dissertation. Sydney, NSW: University of Technology Sydney.
Clark, J. S., Poore, A. G. B., and Doblin, M. A. (2018). Shaping up for stress: physiological flexibility is key to survivorship in a habitat-forming macroalga. J. Plant Physiol. 231, 346–355. doi: 10.1016/j.jplph.2018.10.005
Clark, J. S., Poore, A. G. B., Ralph, P. J., and Doblin, M. A. (2013). Potential for adaptation in response to thermal stress in an intertidal macroalga. J. Phycol. 49, 630–639. doi: 10.1111/jpy.12067
Clarke, S., and Womersley, H. (1981). Cross-fertilization and hybrid development of forms of the brown alga Hormosira banksii (Turner) Decaisne. Austr. J. Bot. 29, 497–505.
Coleman, M. A. (2013). Connectivity of the habitat-forming kelp, Ecklonia radiata within and among estuaries and open coast. PLoS One 8:e64667. doi: 10.1371/journal.pone.0064667
Coleman, M. A., Chambers, J., Knott, N. A., Malcolm, H. A., Harasti, D., Jordan, A., et al. (2011a). Connectivity within and among a network of temperate marine reserves. PLoS One 6:e20168. doi: 10.1371/journal.pone.0020168
Coleman, M. A., Roughan, M., Macdonald, H. S., Connell, S. D., Gillanders, B. M., Kelaher, B. P., et al. (2011b). Variation in the strength of continental boundary currents determines continent-wide connectivity in kelp: boundary currents determine connectivity of kelp. J. Ecol. 99, 1026–1032. doi: 10.1111/j.1365-2745.2011.01822.x
Coleman, M. A., Clark, J. S., Doblin, M. A., Bishop, M. J., and Kelaher, B. P. (2019). Genetic differentiation between estuarine and open coast ecotypes of a dominant ecosystem engineer. Mar. Freshw. Res. 70:977. doi: 10.1071/MF17392
Davison, I. R., and Pearson, G. A. (1996). Stress tolerance in intertidal seaweeds. J. Phycol. 32, 197–211. doi: 10.1111/j.0022-3646.1996.00197.x
Dayton, P. K. (1972). Toward an Understanding of Community Resilience and the Potential Effects of Enrichments to the Benthos at McMurdo Sound, Antarctica. Lawrence, KS: Allen Press Lawrence, 81–96.
Deutsch, C. A., Tewksbury, J. J., Huey, R. B., Sheldon, K. S., Ghalambor, C. K., Haak, D. C., et al. (2008). Impacts of climate warming on terrestrial ectotherms across latitude. Proc. Natl. Acad. Sci. U.S.A. 105, 6668–6672. doi: 10.1073/pnas.0709472105
Dimartino, S., Mather, A. V., Alestra, T., Nawada, S., and Haber, M. (2015). Experimental and computational analysis of a novel flow channel to assess the adhesion strength of sessile marine organisms. Interface Focus 5:20140059. doi: 10.1098/rsfs.2014.0059
Doblin, M., and Clayton, M. (1995). Effects of secondarily-treated sewage effluent on the early life-history stages of two species of brown macroalgae: Hormosira banksii and Durvillaea potatorum. Mar. Biol. 122, 689–698. doi: 10.1007/bf00350691
Dring, M. J., and Brown, F. A. (1982). Photosynthesis of intertidal brown algae during and after periods of emersion: a renewed search for physiological causes of zonation. Mar. Ecol. Prog. Ser. 8, 301–308. doi: 10.3354/meps008301
Dudgeon, S., Kübler, J., Wright, W., Vadas, R. Sr., and Petraitis, P. S. (2001). Natural variability in zygote dispersal of Ascophyllum nodosum at small spatial scales. Funct. Ecol. 15, 595–604. doi: 10.1046/j.0269-8463.2001.00559.x
Eckert, C. G., Samis, K. E., and Lougheed, S. C. (2008). Genetic variation across species’ geographical ranges: the central–marginal hypothesis and beyond. Mol. Ecol. 17, 1170–1188. doi: 10.1111/j.1365-294X.2007.03659.x
Engel, C., Destombe, C., and Valero, M. (2004). Mating system and gene flow in the red seaweed Gracilaria gracilis: effect of haploid–diploid life history and intertidal rocky shore landscape on fine-scale genetic structure. Heredity 92, 289–298. doi: 10.1038/sj.hdy.6800407
Excoffier, L., Laval, G., and Schneider, S. (2007). Arlequin (version 3.0): an integrated software package for population genetics data analysis. Evol. Bioinform. 1, 47–50.
Falkowski, P. G., and Raven, J. A. (2013). Aquatic Photosynthesis. Princeton, NJ: Princeton University Press.
Faugeron, S., Martínez, E. A., Correa, J. A., Cardenas, L., Destombe, C., and Valero, M. (2004). Reduced genetic diversity and increased population differentiation in peripheral and overharvested populations of Gigartina skottsbergii (Rhodophyta, Gigartinales) in southern Chile. J. Phycol. 40, 454–462. doi: 10.1111/j.1529-8817.2004.03114.x
Ferreira, J. G., Arenas, F., Martínez, B., Hawkins, S. J., and Jenkins, S. R. (2014). Physiological response of fucoid algae to environmental stress: comparing range centre and southern populations. New Phytol. 202, 1157–1172. doi: 10.1111/nph.12749
Foo, S. A., Dworjanyn, S. A., Poore, A. G. B., and Byrne, M. (2012). Adaptive capacity of the habitat modifying sea urchin Centrostephanus rodgersii to ocean warming and ocean acidification: performance of early embryos. PLoS One 7:e42497. doi: 10.1371/journal.pone.0042497
Fredriksen, S., Filbee-Dexter, K., Norderhaug, K. M., Steen, H., Bodvin, T., Coleman, M. A., et al. (2020). Green gravel: a novel restoration tool to combat kelp forest decline. Sci. Rep. 10:3983. doi: 10.1038/s41598-020-60553-x
Freeland, J. R., Petersen, S. D., and Kirk, H. (2011). Molecular Ecology, 2nd Edn. Hoboken, NJ: Wiley-Blackwell.
Fusi, M., Giomi, F., Babbini, S., Daffonchio, D., McQuaid, C. D., Porri, F., et al. (2015). Thermal specialization across large geographical scales predicts the resilience of mangrove crab populations to global warming. Oikos 124, 784–795. doi: 10.1111/oik.01757
Galloway, L. F., Etterson, J. R., and McGlothlin, J. W. (2009). Contribution of direct and maternal genetic effects to life-history evolution. New Phytol. 183, 826–838. doi: 10.1111/j.1469-8137.2009.02939.x
Genty, B., Briantais, J.-M., and Baker, N. R. (1989). The relationship between the quantum yield of photosynthetic electron transport and quenching of chlorophyll fluorescence. Biochim. Biophys. Acta 990, 87–92. doi: 10.1016/S0304-4165(89)80016-9
Gerard, V. A., and Du Bois, K. R. (1988). Temperature ecotypes near the southern boundary of the kelp Laminaria saccharina. Mar. Biol. 97, 575–580. doi: 10.1007/BF00391054
Goudet, J. (1995). FSTAT (version 1.2): a computer program to calculate F-statistics. J. Hered. 86, 485–486. doi: 10.1093/oxfordjournals.jhered.a111627
Gunthorpe, L., Nottage, M., Palmer, D., and Wu, R. (1995). The development of a fertilisation inhibition assay using gametes of the brown alga Hormosira banksii. Austr. J. Ecotoxicol. 1, 25–31.
Gurgel, C. F. D., Camacho, O., Minne, A. J. P., Wernberg, T., and Coleman, M. A. (2020). Marine heatwave drives cryptic loss of genetic diversity in underwater forests. Curr. Biol. 30, 1199.e–1206.e. doi: 10.1016/j.cub.2020.01.051
Hampe, A., and Petit, R. J. (2005). Conserving biodiversity under climate change: the rear edge matters. Ecol. Lett. 8, 461–467. doi: 10.1111/j.1461-0248.2005.00739.x
Hanelt, D., Huppertz, K., and Nultsch, W. (1993). Daily course of photosynthesis and photoinhibition in marine macroalgae investigated in the laboratory and field. Mar. Ecol. Prog. Ser. 97, 31–37. doi: 10.3354/meps097031
Harley, C. (2008). Tidal dynamics, topographic orientation, and temperature-mediated mass mortalities on rocky shores. Mar. Ecol. Prog. Ser. 371, 37–46. doi: 10.3354/meps07711
Hartnoll, R. G., and Hawkins, S. J. (1985). Patchiness and fluctuations on moderately exposed rocky shores. Ophelia 24, 53–63. doi: 10.1080/00785236.1985.10426619
Hawkins, S., Sugden, H., Mieszkowska, N., Moore, P., Poloczanska, E., Leaper, R., et al. (2009). Consequences of climate-driven biodiversity changes for ecosystem functioning of North European rocky shores. Mar. Ecol. Prog. Ser. 396, 245–259. doi: 10.3354/meps08378
Helmuth, B. (2009). From cells to coastlines: how can we use physiology to forecast the impacts of climate change? J. Exp. Biol. 212, 753–760. doi: 10.1242/jeb.023861
Helmuth, B., Broitman, B. R., Blanchette, C. A., Gilman, S., Halpin, P., Harley, C. D. G., et al. (2006). Mosaic patterns of thermal stress in the rocky intertidal zone: implications for climate change. Ecol. Monogr. 76, 461–479. doi: 10.1890/0012-9615(2006)076[0461:mpotsi]2.0.co;2
Helmuth, B., Harley, C. D. G., Halpin, P. M., O’Donnell, M., Hofmann, G. E., and Blanchette, C. A. (2002). Climate change and latitudinal patterns of intertidal thermal stress. Science 298, 1015–1017. doi: 10.1126/science.1076814
Helmuth, B., Russell, B. D., Connell, S. D., Dong, Y., Harley, C. D., Lima, F. P., et al. (2014). Beyond long-term averages: making biological sense of a rapidly changing world. Clim. Change Respons. 1:6. doi: 10.1186/s40665-014-0006-0
Helmuth, B. S. T., and Hofmann, G. E. (2001). Microhabitats, thermal heterogeneity, and patterns of physiological stress in the rocky intertidal zone. Biol. Bull. 201, 374–384. doi: 10.2307/1543615
Hobday, A. J., and Pecl, G. T. (2014). Identification of global marine hotspots: sentinels for change and vanguards for adaptation action. Rev. Fish Biol. Fish. 24, 415–425. doi: 10.1007/s11160-013-9326-6
Hoffmann, A. A., and Sgrò, C. M. (2011). Climate change and evolutionary adaptation. Nature 470, 479–485. doi: 10.1038/nature09670
Huey, R. B., Kearney, M. R., Krockenberger, A., Holtum, J. A., Jess, M., and Williams, S. E. (2012). Predicting organismal vulnerability to climate warming: roles of behaviour, physiology and adaptation. Philos. Trans. R. Soc. B Biol. Sci. 367, 1665–1679. doi: 10.1098/rstb.2012.0005
IPCC (2018). “Summary for policymakers,” in Global Warming of 1.5°C. An IPCC Special Report on the Impacts of Global Warming of 1.5°C Above Pre-Industrial Levels and Related Global Greenhouse Gas Emission Pathways, in the Context of Strengthening the Global Response to the Threat of Climate Change, Sustainable Development, and Efforts to Eradicate Poverty, eds V. Masson-Delmotte, P. Zhai, H.-O. Portner, D. Roberts, J. Skea, P. R. Shukla, et al. (Geneva: World Meteorological Organization), 32.
Jensen, J. L., Bohonak, A. J., and Kelley, S. T. (2005). Isolation by distance, web service. BMC Genet. 6:13. doi: 10.1186/1471-2156-6-13
Johannesson, K., Johannesson, B., and Lundgren, U. (1995). Strong natural selection causes microscale allozyme variation in a marine snail. Proc. Natl. Acad. Sci. U.S.A. 92, 2602–2606. doi: 10.1073/pnas.92.7.2602
Jones, C. G., Lawton, J. H., and Shachak, M. (1994). Organisms as ecosystem engineers. Oikos 69:373. doi: 10.2307/3545850
Kelly, M. W., Sanford, E., and Grosberg, R. K. (2012). Limited potential for adaptation to climate change in a broadly distributed marine crustacean. Proc. R. Soc. B Biol. Sci. 279, 349–356. doi: 10.1098/rspb.2011.0542
King, N. G., McKeown, N. J., Smale, D. A., and Moore, P. J. (2017). The importance of phenotypic plasticity and local adaptation in driving intraspecific variability in thermal niches of marine macrophytes. Ecography 41, 1469–1484. doi: 10.1111/ecog.03186
King, N. G., McKeown, N. J., Smale, D. A., Wilcockson, D. C., Hoelters, L., Groves, E. A., et al. (2019). Evidence for different thermal ecotypes in range centre and trailing edge kelp populations. J. Exp. Mar. Biol. Ecol. 514–515, 10–17. doi: 10.1016/j.jembe.2019.03.004
Kramer, D. M., Johnson, G., Kiirats, O., and Edwards, G. E. (2004). New fluorescence parameters for the determination of Q A redox state and excitation energy fluxes. Photosynth. Res. 79:209. doi: 10.1023/b:pres.0000015391.99477.0d
Lough, J. M., and Hobday, A. J. (2011). Observed climate change in Australian marine and freshwater environments. Mar. Freshw. Res. 62, 984–999. doi: 10.1071/MF10272
Lynch, M., and Walsh, B. (1998). Genetics and Analysis of Quantitative Traits. Sunderland, MA: Sinauer Associates, Inc.
Mabin, C., Johnson, C., and Wright, J. (2019). Physiological response to temperature, light, and nitrates in the giant kelp Macrocystis pyrifera, from Tasmania, Australia. Mar. Ecol. Prog. Ser. 614, 1–19. doi: 10.3354/meps12900
Major, K., and Davison, I. (1998). Influence of temperature and light on growth and photosynthetic physiology of Fucus evanescens (Phaeophyta) embryos. Eur. J. Phycol. 33, 129–138. doi: 10.1080/09670269810001736623
Marshall, D. J. (2008). Transgenerational plasticity in the sea: context-dependent maternal effects across the life history. Ecology 89, 418–427. doi: 10.1890/07-0449.1
Martínez, B., Arenas, F., Rubal, M., Burgués, S., Esteban, R., García-Plazaola, I., et al. (2012). Physical factors driving intertidal macroalgae distribution: physiological stress of a dominant fucoid at its southern limit. Oecologia 170, 341–353. doi: 10.1007/s00442-012-2324-x
McCoy, S. J., and Widdicombe, S. (2019). Thermal plasticity is independent of environmental history in an intertidal seaweed. Ecol. Evol. 9, 13402–13412. doi: 10.1002/ece3.5796
Miller, A. D., Coleman, M. A., Clark, J., Cook, R., Naga, Z., Doblin, M. A., et al. (2019). Local thermal adaptation and limited gene flow constrain future climate responses of a marine ecosystem engineer. Evol. Appl. 13, 918–934. doi: 10.1111/eva.12909
Mota, C. F., Engelen, A. H., Serrao, E. A., Coelho, M. A. G., Marbà, N., Krause-Jensen, D., et al. (2018). Differentiation in fitness-related traits in response to elevated temperatures between leading and trailing edge populations of marine macrophytes. PLoS One 13:e0203666. doi: 10.1371/journal.pone.0203666
Muhlin, J. F., Engel, C. R., Stessel, R., Weatherbee, R. A., and Brawley, S. H. (2008). The influence of coastal topography, circulation patterns, and rafting in structuring populations of an intertidal alga. Mol. Ecol. 17, 1198–1210. doi: 10.1111/j.1365-294X.2007.03624.x
Osborn, J. E. (1948). The structure and life history of Hormosira banksii (Turner) Decaisne. Trans. R. Soc. N. Z. 77, 47–71.
Paaijmans, K. P., Heinig, R. L., Seliga, R. A., Blanford, J. I., Blanford, S., Murdock, C. C., et al. (2013). Temperature variation makes ectotherms more sensitive to climate change. Glob. Change Biol. 19, 2373–2380. doi: 10.1111/gcb.12240
Parmesan, C. (2006). Ecological and evolutionary responses to recent climate change. Annu. Rev. Ecol. Evol. Syst. 37, 637–669. doi: 10.1146/annurev.ecolsys.37.091305.110100
Pearson, G. A., Lago-Leston, A., and Mota, C. (2009). Frayed at the edges: selective pressure and adaptive response to abiotic stressors are mismatched in low diversity edge populations. J. Ecol. 97, 450–462. doi: 10.1111/j.1365-2745.2009.01481.x
Pecl, G. T., Araújo, M. B., Bell, J. D., Blanchard, J., Bonebrake, T. C., Chen, I.-C., et al. (2017). Biodiversity redistribution under climate change: impacts on ecosystems and human well-being. Science 355:eaai9214. doi: 10.1126/science.aai9214
Poloczanska, E. S., Burrows, M. T., Brown, C. J., García Molinos, J., Halpern, B. S., Hoegh-Guldberg, O., et al. (2016). Responses of marine organisms to climate change across oceans. Front. Mar. Sci. 3:62. doi: 10.3389/fmars.2016.00062
R Core Team (2020). R: A Language and Environment for Statistical Computing. Vienna: R Foundation for Statistical Computing.
Reusch, T. B. H. (2014). Climate change in the oceans: evolutionary versus phenotypically plastic responses of marine animals and plants. Evol. Appl. 7, 104–122. doi: 10.1111/eva.12109
Reusch, T. B. H., Ehlers, A., Hämmerli, A., and Worm, B. (2005). Ecosystem recovery after climatic extremes enhanced by genotypic diversity. PNAS 102, 2826–2831. doi: 10.1073/pnas.0500008102
Rice, W. R. (1989). Analyzing tables of statistical tests. Evolution 43, 223–225. doi: 10.1111/j.1558-5646.1989.tb04220.x
Sagarin, R. D., and Gaines, S. D. (2002). The “abundant centre” distribution: to what extent is it a biogeographical rule? Ecol. Lett. 5, 137–147. doi: 10.1046/j.1461-0248.2002.00297.x
Schiel, D. R. (2006). Rivets or bolts? When single species count in the function of temperate rocky reef communities. J. Exp. Mar. Biol. Ecol. 338, 233–252. doi: 10.1016/j.jembe.2006.06.023
Schmidt, P. S., and Rand, D. M. (2001). Adaptive maintenance of genetic polymorphism in an intertidal barnacle: habitat-and life-stage-specific survivorship of Mpi genotypes. Evolution 55, 1336–1344. doi: 10.1111/j.0014-3820.2001.tb00656.x
Schonbeck, M., and Norton, T. A. (1978). Factors controlling the upper limits of fucoid algae on the shore. J. Exp. Mar. Biol. Ecol. 31, 303–313. doi: 10.1016/0022-0981(78)90065-5
Schreiber, U. (2004). “Pulse-amplitude-modulation (PAM) fluorometry and saturation pulse method: an overview,” in Chlorophyll a Fluorescence, eds G. C. Papageorgiou and Govindjee (Dordrecht: Springer), 279–319. doi: 10.1007/978-1-4020-3218-9_11
Sgrò, C. M., and Hoffmann, A. A. (2004). Genetic correlations, tradeoffs and environmental variation. Heredity 93, 241–248. doi: 10.1038/sj.hdy.6800532
Smale, D. A., and Wernberg, T. (2013). Extreme climatic event drives range contraction of a habitat-forming species. Proc. R. Soc. B Biol. Sci. 280:20122829. doi: 10.1098/rspb.2012.2829
Smale, D. A., Wernberg, T., Oliver, E. C. J., Thomsen, M., Harvey, B. P., Straub, S. C., et al. (2019). Marine heatwaves threaten global biodiversity and the provision of ecosystem services. Nat. Clim. Change 9, 306–312. doi: 10.1038/s41558-019-0412-1
Smolina, I., Kollias, S., Jueterbock, A., Coyer, J. A., and Hoarau, G. (2016). Variation in thermal stress response in two populations of the brown seaweed, Fucus distichus, from the Arctic and subarctic intertidal. R. Soc. Open Sci. 3:150429. doi: 10.1098/rsos.150429
Somero, G. N. (2005). Linking biogeography to physiology: evolutionary and acclimatory adjustments of thermal limits. Front. Zool. 2:1. doi: 10.1186/1742-9994-2-1
Staehr, P. A., and Wernberg, T. (2009). Physiological responses of Ecklonia radiata (Laminariales) to a latitudinal gradient in ocean temperature. J. Phycol. 45, 91–99. doi: 10.1111/j.1529-8817.2008.00635.x
Stevens, G. C. (1989). The latitudinal gradient in geographical range: how so many species coexist in the tropics. Am. Nat. 133, 240–256. doi: 10.1086/284913
Stillman, J. H., and Somero, G. N. (2000). A comparative analysis of the upper thermal tolerance limits of eastern pacific porcelain crabs, genus Petrolisthes: influences of latitude, vertical zonation, acclimation, and phylogeny. Physiol. Biochem. Zool. 73, 200–208. doi: 10.1086/316738
Sunday, J. M., Bates, A. E., and Dulvy, N. K. (2012). Thermal tolerance and the global redistribution of animals. Nat. Clim. Change 2, 686–690. doi: 10.1038/nclimate1539
Tatarenkov, A., Bergström, L., Jönsson, R. B., Serrão, E. A., Kautsky, L., and Johannesson, K. (2005). Intriguing asexual life in marginal populations of the brown seaweed Fucus vesiculosus: clonality in marginal fucoid populations. Mol. Ecol. 14, 647–651. doi: 10.1111/j.1365-294X.2005.02425.x
Teixeira, S., Pearson, G., Candeias, R., Madeira, C., Valero, M., and Serrão, E. (2016). Lack of fine-scale genetic structure and distant mating in natural populations of Fucus vesiculosus. Mar. Ecol. Prog. Ser. 544, 131–142. doi: 10.3354/meps11607
Thomas, C. D., Cameron, A., Green, R. E., Bakkenes, M., Beaumont, L. J., Collingham, Y. C., et al. (2004). Extinction risk from climate change. Nature 427, 145–148. doi: 10.1038/nature02121
Valero, M., Destombe, C., Mauger, S., Ribout, C., Engel, C. R., Daguin-Thiebaut, C., et al. (2011). Using genetic tools for sustainable management of kelps: a literature review and the example of Laminaria digitata. Cah. Biol. Mar. 52, 467–483.
Van Oosterhout, C., Hutchinson, W. F., Wills, D. P. M., and Shipley, P. (2004). Micro-checker: software for identifying and correcting genotyping errors in microsatellite data. Mol. Ecol. Notes 4, 535–538. doi: 10.1111/j.1471-8286.2004.00684.x
Wernberg, T., Coleman, M. A., Bennett, S., Thomsen, M. S., Tuya, F., and Kelaher, B. P. (2018). Genetic diversity and kelp forest vulnerability to climatic stress. Sci. Rep. 8:1851. doi: 10.1038/s41598-018-20009-9
Wernberg, T., de Bettignies, T., Joy, B. A., and Finnegan, P. M. (2016). Physiological responses of habitat-forming seaweeds to increasing temperatures: seaweed responses to increasing temperature. Limnol. Oceanogr. 61, 2180–2190. doi: 10.1002/lno.10362
Williams, S. L., and Dethier, M. N. (2005). High and dry: variation in net photosynthesis of the intertidal seaweed Fucus gardneri. Ecology 86, 2373–2379. doi: 10.1890/04-1569
Wolf, J. B., and Wade, M. J. (2009). What are maternal effects (and what are they not)? Philos. Trans. R. Soc. B Biol. Sci. 364, 1107–1115. doi: 10.1098/rstb.2008.0238
Womersley, H. B. S. (1987). The Marine Benthic Flora of Southern Australia. Part II. Adelaide: South Australian Government Printing Division.
Wood, G., Marzinelli, E. M., Coleman, M. A., Campbell, A. H., Santini, N. S., Kajlich, L., et al. (2019). Restoring subtidal marine macrophytes in the Anthropocene: trajectories and future-proofing. Mar. Freshw. Res. 70, 936–951. doi: 10.1071/MF18226
Zardi, G. I., Nicastro, K., Costa, J. F., Serrão, E., and Pearson, G. (2013). Broad scale agreement between intertidal habitats and adaptive traits on a basis of contrasting population genetic structure. Estuar. Coast. Shelf Sci. 131, 140–148. doi: 10.1016/j.ecss.2013.08.016
Zardi, G. I., Nicastro, K. R., Canovas, F., Costa, J. F., Serrão, E. A., and Pearson, G. A. (2011). Adaptive traits are maintained on steep selective gradients despite gene flow and hybridization in the intertidal zone. PLoS One 6:e19402. doi: 10.1371/journal.pone.0019402
Keywords: thermal performance, climate change, photosynthesis, microsatellites, genetic diversity, quantitative breeding designs, seaweed, Hormosira banksii
Citation: Clark JS, Poore AGB, Coleman MA and Doblin MA (2020) Local Scale Thermal Environment and Limited Gene Flow Indicates Vulnerability of Warm Edge Populations in a Habitat Forming Macroalga. Front. Mar. Sci. 7:711. doi: 10.3389/fmars.2020.00711
Received: 31 March 2020; Accepted: 04 August 2020;
Published: 27 August 2020.
Edited by:
Christopher Edward Cornwall, Victoria University of Wellington, New ZealandReviewed by:
Thibaut de Bettignies, Muséum National d’Histoire Naturelle, FranceCayne Layton, University of Tasmania, Australia
Copyright © 2020 Clark, Poore, Coleman and Doblin. This is an open-access article distributed under the terms of the Creative Commons Attribution License (CC BY). The use, distribution or reproduction in other forums is permitted, provided the original author(s) and the copyright owner(s) are credited and that the original publication in this journal is cited, in accordance with accepted academic practice. No use, distribution or reproduction is permitted which does not comply with these terms.
*Correspondence: Jennifer S. Clark, SmVubmlmZXIuQ2xhcmtAYm90YW55LnViYy5jYQ==