- Institute of Aquaculture, University of Stirling, Stirling, United Kingdom
Whilst osmoregulation in the adult teleost fish has been extensively studied and significant advances have been made in recent years, much less information exists regarding osmoregulation during the early stages of development of teleosts. Adult fish maintain their blood osmolality in a narrow physiological range, i.e., ≈ 280–360 mOsm kg–1, through the combined osmoregulatory capabilities of several sites i.e., branchial chambers, skin, digestive system and urinary organs. However, embryonic and post-embryonic stages maintain their blood osmolality in a less narrow range of ≈ 240–540 mOsm kg–1 and osmoregulatory capacity is restricted to the cutaneous ionocytes located on the tegument with a transference in osmoregulatory function occurring during the early life stages to the developing digestive tract, the urinary organs and the developing branchial tissues and the ionocytes which they support. This review will discuss the development of osmoregulatory capacity that occurs throughout early life stages of teleosts and its role in conserving physiological homeostasis, focusing on the form and function of related mechanisms, i.e., the ionoregulatory cell or ionocyte, outlining the different roles and functions of different ionocyte types relative to their environment, i.e., freshwater or seawater, their plasticity and discuss spatio-temporal changes in ionocyte distribution that occur during ontogeny.
Introduction
Prunet and Bornancin (1989; p. 92) describe teleost fishes as “an open system in dynamic equilibrium with aquatic surroundings.” As osmoregulators, teleosts are homeo-isosmotic, i.e., able to regulate the concentration of solutes and their total osmolarity of their internal fluids at levels different to their external environment. Hence their body fluids remain relatively constant in spite of alterations to their external medium. They are, therefore, able to maintain their blood osmolality in a 280–360 mOsm kg–1 range, at the equivalent of 10–12 ppt (Evans et al., 2005). Hyper-osmotic regulators (most freshwater teleosts), subject to passive osmotic influx of water and diffusional loss of ions, mainly Na+ and Cl–, maintain body fluid concentration above that of their external surroundings. Hypo-osmotic regulators (most marine teleosts), subject to passive osmotic loss of water and diffusive gain of ions, maintain body fluid concentration below that of their external medium (Figure 1). Therefore, when faced with variations in external salinity, fishes must compensate for body fluid disturbances with a regulative capacity to adapt their osmoregulatory and ion transport strategies dependant on their surrounding environment (Ruiz-Jarabo et al., 2015).
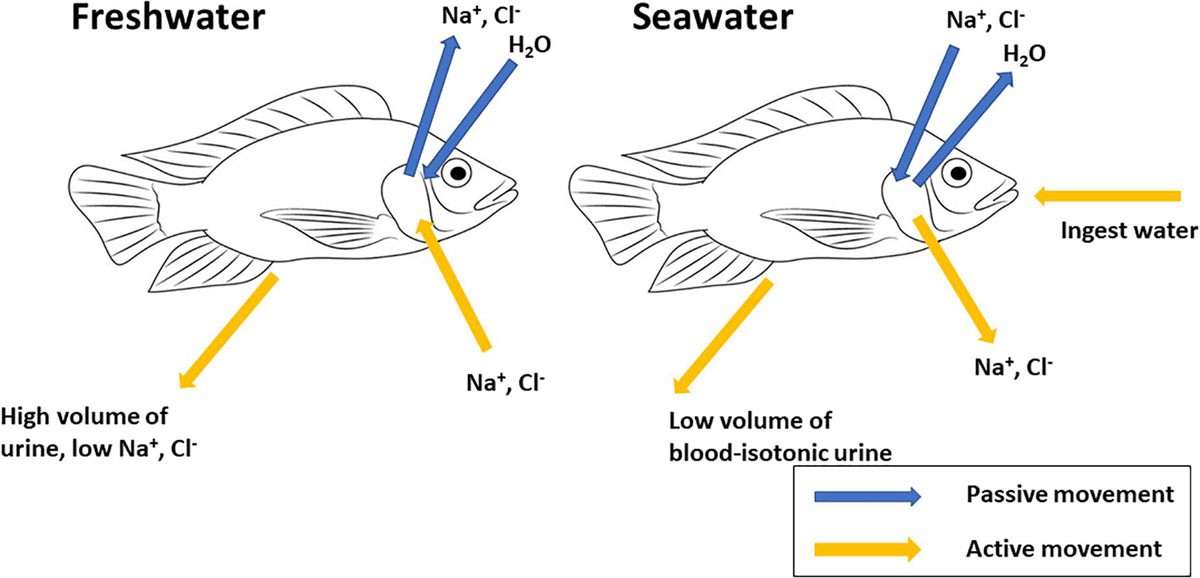
Figure 1. Generalized schematic representation of mechanisms of osmoregulation in teleost fishes. Freshwater fishes are hyper-osmotic to their aquatic environment; compensatory mechanisms include active uptake of NaCl across gill epithelium, intestinal absorption of NaCl and excretion of large volumes of dilute urine. Marine teleosts are hypo-osmotic to their aquatic environment; compensatory mechanisms include active secretion of NaCl across gill epithelium, ingestion of seawater, intestinal absorption of NaCl and water and excretion of small volumes of blood-isotonic urine.
Fishes are the most taxonomically diverse group of vertebrates with >32,400 identified species (Froese and Pauly, 2012) and have evolved to occupy almost all types of natural waters, ranging from low-ionic strength fresh waters to those of salinities of 80–142 ppt (Kinne, 1964; Parry, 1966; Griffith, 1974; Alderdice, 1988). The question of whether fish evolved in seawater or freshwater is still subject to debate. Nelson’s (2006) study argued that fishes evolved in a marine environment, invaded freshwater habitats, and subsequently reinvaded marine environments, based on their level of phylogenetic advancement, i.e., the most primitive orders consisting almost entirely of strictly marine species, the next moderately advanced orders consisting mostly of freshwater species and the remaining orders mostly composed of marine species. Conversely, Evans and Claiborne (2009) argued that the considerably lower osmolality of the internal fluids of teleosts (equivalent to 9 ppt) when compared to that of the marine environment, represented evidence for the origins of this clade of fishes from a freshwater or brackish water environment. This is supported by Vega and Wiens (2012) trait reconstructions based on living and fossil taxa. These authors suggested that all extant actinopterygians (ray-finned) fishes were derived from a freshwater ancestor. Inconclusive fossil records of fishes shed no further light (Halstead, 1985; Evans and Claiborne, 2009).
Some fishes are restricted to living in a narrow range of salinity (stenohaline) while others can adapt to and tolerate broad ranges of salinity (euryhaline). It is estimated that c. 40% (c. 15,000) of these inhabit freshwater during at least one phase of their life cycle (Bond, 1996). This euryhalinity can range from either compulsory, migratory events in the lifecycle of a fish, e.g., catadromous fishes which spend their pre-adult life in freshwater and return to spawn in the sea or, conversely, anadromous fishes which grow and mature in sea water but return to freshwater to spawn, to less clearly defined movements of fishes that occupy estuarine waters or coastal habitats and undergo regular and frequent variations in the salinity of the medium in which they inhabit. This ability to cope with salinity changes depends on their capacity to osmoregulate and plays an important role in defining species and developmental stage-specific distribution (Schreiber, 2001).
It is well established that teleost embryos and larvae are able to maintain osmotic and ionic gradients between their internal and external environments (Guggino, 1980a,b; Alderdice, 1988; Kaneko et al., 1995), although full adult osmoregulatory capacity is not reached in these early developmental stages as organs are under-developed or absent (Varsamos et al., 2005). Compared to adult teleosts, larvae are able to maintain their blood osmolality in a less narrow range of ≈ 240–540 mOsm kg–1, and this adaptive ability is accomplished by an early acquisition of osmoregulatory mechanisms that are different from those in adult fish. The ontogenetic development of osmoregulatory capacity, moving from a somewhat limited trans-membrane particle exchange at a cellular level in the embryonic blastular stage, to the fully-functioning regulatory tissues in juvenile and adult, such as the renal complex, the gut and the branchial epithelium, is described succinctly by Alderdice (1988; p.225) as a process which displays “continuity, with increasing complexity.” As part of this ontogenic process, teleost embryos and post-embryonic larvae are able to maintain osmotic and ionic gradients between their internal and external environments due to the presence of numerous extrabranchial, cutaneous ionocytes commonly observed on the abdominal epithelium of the yolk-sac and other body surfaces of fish embryos and larvae.
While osmoregulation in the adult teleost fish has been extensively studied and significant advances have been made in recent years (Evans, 1999; McCormick et al., 2013), much less information, however, exists regarding osmoregulation in the early stages of development (Holliday, 1965; Alderdice, 1988; Tytler et al., 1993; Schreiber, 2001; Evans et al., 2005; Varsamos et al., 2005; Bodinier et al., 2010). Recently, the availability of precisely staged young fish, due to both the improved rearing methods by aquaculture and less stressful capture techniques for wild populations, has contributed to developments in the field (Evans et al., 2005). In addition, the development and application of new immunological techniques allowing visualization of delicate early life stages, has allowed the progression of ontogenetic studies.
The Ionoregulatory Cell or Ionocyte
Introduction
As opposed to movement of gases, ion movements require specific carriers and this “metabolic machinery” (Rombough, 2004) is found in a specific cell type, the ionocyte. Numerous work, dedicated to the study of their form and function in the adult teleost, has established that these cells are the primary extra-renal site responsible for the trans-epithelial transport of ions in adults and juvenile teleosts (Laurent and Dunel, 1980; Laurent, 1984; Perry et al., 1992; McCormick, 1995; Evans, 1999; Evans et al., 2005; Hiroi and McCormick, 2012).
Large spherical cells with eosinophilic granules were first described by Keys and Willmer (1932) of the Physiological Laboratory, Cambridge (United Kingdom) as “chloride-secreting cells,” based on observations of the chloride secretory activity of gills of the adult eel (Anguilla anguilla) in seawater. The abbreviated name “chloride cell” is probably attributable to Copeland (1948) and was later clarified by Foskett and Scheffey (1982), who confirmed active transport of chloride ions by these cells using vibrating probe experiments on the opercular epithelium of sea-water adapted tilapia Oreochromis mossambicus. The term “mitochondria-rich cells” was first introduced by Lee et al. (1996), in order to emphasize the multifunctionality of the cells, i.e., they do more than just excrete chloride ions in seawater adapted fish. The term “ionocytes” was first introduced by Watrin and Mayer-Gostan (1996) in their study of ionoregulatory sites in the turbot (Scophthalmus maximus) and is currently accepted to be the most widely applicable term. Therefore, throughout this review, the term “ionocyte” will be used.
General Structure of Ionocytes
There are extensive reviews dealing with structure of ionocytes, e.g., Pisam and Rambourg (1991), McCormick (1995), Evans et al. (2005), Hwang and Lin (2013), Marshall (2011), and Dymowska et al. (2012). They have a number of common features that distinguish them from surrounding cells, sharing a specific complement of transporter or channels on the apical and basolateral membranes that allow directional movement of ions (Dymowska et al., 2012).
Ionocytes are highly specialized, polarized cells of large, columnar/ovoid shape displaying distinct ultra-structural features characteristic of ion-transporting cells, i.e., large numbers of mitochondria and a dense, tubular network that is continuous with the basolateral membrane causing extensive invagination (Doyle and Gorecki, 1961; Philpott, 1966). This tubular-vesicular system extends throughout most of the cytoplasm, and is closely associated with the mitochondria (Philpott, 1980; Laurent, 1984; Wilson et al., 2000a,b). It results in a large surface area for the placement of transport proteins, most importantly the ion-translocating enzyme Na+/K+-ATPase or “sodium pump” (García-Ayala et al., 1997) that has a role in both ion uptake and salt secretion in ionocytes in the teleost gill (Hiroi and McCormick, 2012). Early experiments confirmed labeled Na+ and Cl– efflux activity in live eels with the use of radioactive ouabain (a Na+/K+-ATPase inhibitor), thus inferring a basolateral location for the transporter protein Na+/K+-ATPase in mitochondria-rich cells (Silva et al., 1977). Subsequent work established that fish gill epithelia expressed large quantities of Na+/K+-ATPase (NKA) whose activity was usually proportional to the external salinity (De Rengis and Bornancin, 1984; McCormick, 1995). This has been attributed to an increased α-subunit mRNA abundance (Madsen et al., 1995; Singer et al., 2002) and protein amount (Lee et al., 2000; Tipsmark et al., 2002; Lin et al., 2003) or both (D’Cotta et al., 2000; Lin and Hwang, 2004).
Ion Transport in Seawater
Morphology
As a general rule, ionocytes in seawater or seawater-adapted fishes have the following morphological characteristics: the apical membrane is recessed below the surface of the surrounding pavement cells to form a concave pore or “crypt” that can be shared by accessory cells (ACs) (Karnaky, 1986), often forming “multi-cellular complexes” with cytoplasmic processes of accessory cells (ACs) extending into the apical cytoplasm of ionocytes to form complex interdigitations (Laurent, 1984; Wilson and Laurent, 2002) (see Section “Accessory Cells” below). These two types of cells share a single-stranded, “shallow” junction, suggesting a “leaky” pathway is present between the cells (Laurent, 1984; Hwang, 1988), thus providing a paracellular route for sodium extrusion (Sardet et al., 1979; Laurent, 1984).
Accessory Cells (ACs)
Hootman and Philpott (1980) first named the undifferentiated ionocytes found beside mature ionocytes in seawater flounder “accessory cells” or ACs. They appeared to be structurally analogous to ionocytes, in that they possessed large amounts of mitochondria and a labyrinthal tubular system, but were smaller and less developed than ionocytes with a less developed tubular system and lower expression of Na+/K+-ATPase relative to mature ionocytes. It has been reported that either a single or more than one accessory cell (AC), cluster around an ionocyte forming a “multi-cellular complex” (MCC) with a shared apical crypt (Hwang, 1988). ACs are small, semi lunar or pear-shaped cells with lateral cytoplasmic processes that extend from the ACs to penetrate the apical portion of the adjacent ionocyte, sharing the apical cavity. ACs share a single-stranded, shallow junction with an ionocyte, suggestive of a “leaky” paracellular pathway thus giving additional paracellular pathways for the secretion of excess Na+ from body fluids (Evans, 1999).
Na-Cl Secretion
Studies on mechanisms of Na-Cl secretion in seawater type ionocytes have been studied for several decades (Hsu et al., 2014) and the current and well accepted model for active NaCl transport by ionocytes in seawater adapted teleosts consists of three major ion-transporting proteins, i.e., Na+/K+-ATPase (NKA), Na+/K+/2Cl– co-transporter 1 (NKCC1) and a Cl- channel homologous to the human cystic fibrosis transmembrane receptor (CFTR) (Evans, 1999; Hirose et al., 2003; Evans et al., 2005; Hwang and Lin, 2013).
Briefly; basolateral Na+/K+-ATPase driven extrusion of three Na+ from the cell to the plasma and entry of two K+ into the cell then generates an electrochemical gradient that drives Na+, coupled with Cl– and K+, back from the plasma into the cell’s cytoplasm, via the Na+/K+/2Cl– co-transporter or (NKCC). NKCC therefore mediates the movements of Na+, K+ and Cl– across the basolateral membrane of ionocytes and has a key role in cell volume homeostasis, maintenance of the electrolyte content and transepithelial ion and water movement in polarized cells (Cutler and Cramb, 2002). K+ therefore enters the cell basolaterally both via the Na+/K+-ATPase and the NKCC co-transporter and is removed basolaterally from the cell via the potassium or K+ channel. Cl– exits the cell via an apical Cl– anion channel or CFTR (cystic fibrosis transmembrane receptor).
Na+ moves through the leaky paracellular pathway between ionocytes and ACs via a cation-selective paracellular pathway (Degnan and Zadunaisky, 1980), due to the negative potential created by transcellular Cl– flux (Sardet et al., 1979). This transepithelial electrical potential across the gill epithelium drives Na+ across leaky junction between ionocytes and accessory cells (Hsu et al., 2014) (see section “Accessory Cells (ACs)” above).
Ion Transport in Freshwater
Morphology
Ionocytes in freshwater usually lack an apical crypt and have their apical surfaces forming microvilli above the adjacent PVCs, which is consistent with their ion absorptive nature (Hwang, 1988; Perry et al., 1992; Marshall et al., 1997). However, an invaginated, crypt-like structure has been reported in ionocytes of the euryhaline Mangrove killifish (Rivulus marmoratus) in 1 ppt (King et al., 1989) and a slightly invaginated apical opening in the β – ionocytes in the freshwater adapted guppy (Lebistes reticulatus) (Pisam et al., 1987) and the loach (Cobitis taenia) and the gudgeon (Gobio gobio) (Pisam et al., 1990). This has similarly, been reported in freshwater adapted Tilapiine species e.g., the Mozambique tilapia (Oreochromis mossambicus) (Lee et al., 1996; van der Heijden et al., 1997; Uchida et al., 2000; Inokuchi et al., 2009) and the Nile tilapia (Oreochromis niloticus) (Pisam et al., 1993). The basolateral tubular system is less well developed in freshwater than in seawater adapted ionocytes, and they form extensive tight, multi-stranded junctions with adjacent PVC cells (Hwang, 1988).
Uptake Mechanisms
The ion-uptake mechanisms in freshwater fishes are more complicated, and both mechanism and ionocyte sub-types appear to vary amongst species (Hwang and Lin, 2013), remaining a source of contention (Dymowska et al., 2012; Hiroi and McCormick, 2012; Hwang and Lin, 2013; Hsu et al., 2014; Breves et al., 2020).
Several ion pumps, transporters and channels which are selectively expressed, either apically or basolaterally in the cell, are responsible for the ion-transport functions of the ionocytes (Hiroi and McCormick, 2012). It would be true to say that, over the last decades, a number of types and sub-types have been proposed, many of them unique to the species in which they were investigated, i.e., in various teleosts species (Doyle and Gorecki, 1961); guppy (Lebistes reticulatus) (Pisam et al., 1987); brown bullhead (Ictalurus nebulosus) (Goss et al., 1992; Goss and Perry, 1994); Japanese eel (Anguilla japonica) (Wong and Chan, 1999); Rainbow trout (O. mykiss) (Galvez et al., 2002; Reid et al., 2003); Medaka (Oryzias latipes) (Kang et al., 2008, 2010; Wu et al., 2010; Lin et al., 2012; Hsu et al., 2014); Zebrafish (Danio rerio) (Lin et al., 2006; Wang et al., 2009; Chang and Hwang, 2011; Hwang et al., 2011; Dymowska et al., 2012; Chang et al., 2013; Hwang and Chou, 2013; Hwang and Lin, 2013); tilapia (Oreochromis spp.) (Chang et al., 2001, 2003; Hiroi et al., 2005, 2008; Inokuchi et al., 2008, 2009); Nile tilapia (O. niloticus) (Fridman et al., 2013b); Mozambique tilapia (O. mossambicus) (Lee et al., 1996, 2000); killifish (Fundulus heteroclitus) (Copeland, 1948; Burns and Copeland, 1950; Wood and Marshall, 1994; Marshall et al., 1997; Katoh et al., 2001, 2003; Wood and Laurent, 2003; Hiroi et al., 2005, 2008; Laurent et al., 2006; Breves et al., 2020); Japanese seabass (Lateolabrax japonicus) (Inokuchi et al., 2017); seabass (Dicentrarchus labrax) (Blondeau-Bidet et al., 2019).
These different models can be seen to reflect the evolution of multiple ion uptake strategies from distinct species (Hwang and Lin, 2013) from fluctuating and diverse freshwater environments with varying ion compositions (Dymowska et al., 2012; Takei et al., 2014; Yan and Hwang, 2019) as well as the criteria used to identify and/or techniques used to visualize and functionally analyze the ionocytes and their transporters (Hsu et al., 2014). It is for this reason that the ability to define a definitive or comprehensive model of ion uptake mechanism in teleost is difficult (Hwang et al., 2011; Hwang and Lin, 2013).
Plasticity in Ion Transporting Function of Ionocytes
It is well established that changes in environmental salinity causes replacement of pre-existing ionocytes by newly differentiated ionocytes with a different ion-transport function (Wilson et al., 2000a; Tang et al., 2011; Christensen et al., 2012; Hiroi and McCormick, 2012; Breves et al., 2020). Recent advances in immunohistochemistry and complementary imaging techniques has identified not only freshwater and seawater specific isoforms of the Na+K+-ATPase alpha subunit but also the various ion-transporting proteins in the apical and basolateral membranes of ionocytes, leading to the understanding that ionocytes display a plasticity of function in terms of ion transport which is determined by the localization of the various ion-transporting proteins in the apical and basolateral membranes (Inokuchi et al., 2017). This has been correlated with studies showing the plasticity of genes encoding sub-cellular effectors of ion transport expressed during salinity acclimation (Scott et al., 2004; Fiol and Kültz, 2007).
Ionic and Osmotic Balance in Eggs and During Gastrulation
Leading up to ovulation, the transfer of nutrients and ions occur through the contact between oocyte and follicular cell microvilli and, therefore, their ionic and osmotic control are a function of the parental regulatory system (Alderdice, 1988). At ovulation or release from the follicular cells, the mature eggs become free in the ovary of the adult and, surrounded by ovarian fluid, are still under the control of the adult regulatory system. During this period their plasma membrane appears to be relatively permeable to water and responds to changes in the ovarian fluid (Sower and Schreck, 1982); osmotically the ovarian fluid is very similar to the blood plasma (Hirano et al., 1978) and the blood plasma is in physiological balance with the external environment (Sower and Schreck, 1982).
At spawning, the mature eggs are hypotonic to sea water and hypertonic to fresh water. Independent regulatory capacity is first evident with activation of the embryo occurring in teleosts at metaphase II, the stage of meiosis following the extrusion of the polar body. During activation, the cortical alveoli, underlying the oocyte plasma membrane, discharge their contents into the presumptive perivitelline space between the chorion and the plasma membrane, by a process called cortical alveolar exocytosis, causing an uptake of water from the external environment across the chorion, lifting it away from the plasma membrane by displacement and blocking the micropyle therefore preventing polyspermy. Subsequent regulation and maintenance of the integrity of the egg appears to be achieved by the resistive maintenance of a tight plasma membrane and limited trans-membrane water and ion fluxes (Bennett et al., 1981).
Following this is the transitory developmental blastula stage, characterized by the formation and development of the blastoderm or overgrowth of the yolk by a single layer of cells called a blastomere, which spreads out as a flat plate over the upper surface of the yolk mass. There is little evidence to suggest that there is much control over water and ion exchange between egg and external environment at this stage and any regulatory capacity that does exist is presumed to arise from low trans-membrane fluxes and appears to be “neither modulated nor selective” (Alderdice, 1988; p. 241). Indeed, Alderdice (1988) concludes that the establishment of osmotic or systemic regulation, begins during gastrulation, and is in place by yolk-plug closure; an increase in the permeability of the plasma membrane during gastrulation coincides with the appearance of integumental or cutaneous ionocytes on the epithelium of the body surface and yolk-sac of the developing embryo, marking the start of the selective restriction of ions and water transfer or active ionoregulation (Guggino, 1980b). A recent study by Dahlke et al. (2020) of homeostatic regulation in embryo Atlantic cod (G. morhua) implies that the gastrulation period represents a critical transition from maternal control to active ionic regulation. Epiboly, or cellular overgrowth of the yolk and pericardial regions of the embryo, occurs when the developing ectodermal layer of the blastoderm, along with the marginal ridge of the blastodisc and its inner layer or “germ ring” grows to form an epiblast. This, combined with the periblast, which is the initial covering of the yolk, forms the yolk sac. The opening called the yolk-plug or blastopore overgrows when gastrulation is complete.
Recent evidence suggests the aquaporins, small, hydrophobic integral membrane channel proteins that aid the passive movement of water across bilaminar membranes against an osmotic gradient (Cerdà and Finn, 2010), have a vital adaptive role in maintain homeostasis during oocyte development and embryogenesis (see Cerdà et al., 2017).
Ionocytes During Early Life Stages
The Cutaneous or Extrabranchial Ionocyte
After hatch, post-embryonic larvae are able to live in media whose osmolality differs from their own blood osmolality, and this tolerance is based on ability to osmoregulate. This is due to the presence of numerous cutaneous ionocytes commonly observed in the yolk-sac membrane and other body surfaces of fish embryos and larvae, i.e., head, trunk and fins. These extrabranchial cells are considered to play a definitive role in osmoregulation during early development by secreting and absorbing ions in seawater and freshwater environments, respectively, until the time when gills become fully developed and branchial ionocytes become functional (Kaneko and Shiraishi, 2001). The flat surface that these sites offer has allowed repeated morphological analyses or analysis of ion fluxes that branchial surfaces, with their complex three-dimensional nature, have precluded and offer a convenient experimental substitution for branchial ionocytes thus shedding light on their morphology, function and differentiation (Hiroi and McCormick, 2012).
The first report of localization of ionoregulation to the integument of teleost larvae was that of Shelbourne (1957) who investigated chloride regulation sites in marine plaice larvae (P. platessa). Subsequent and similar reports are summarized in Table 1.
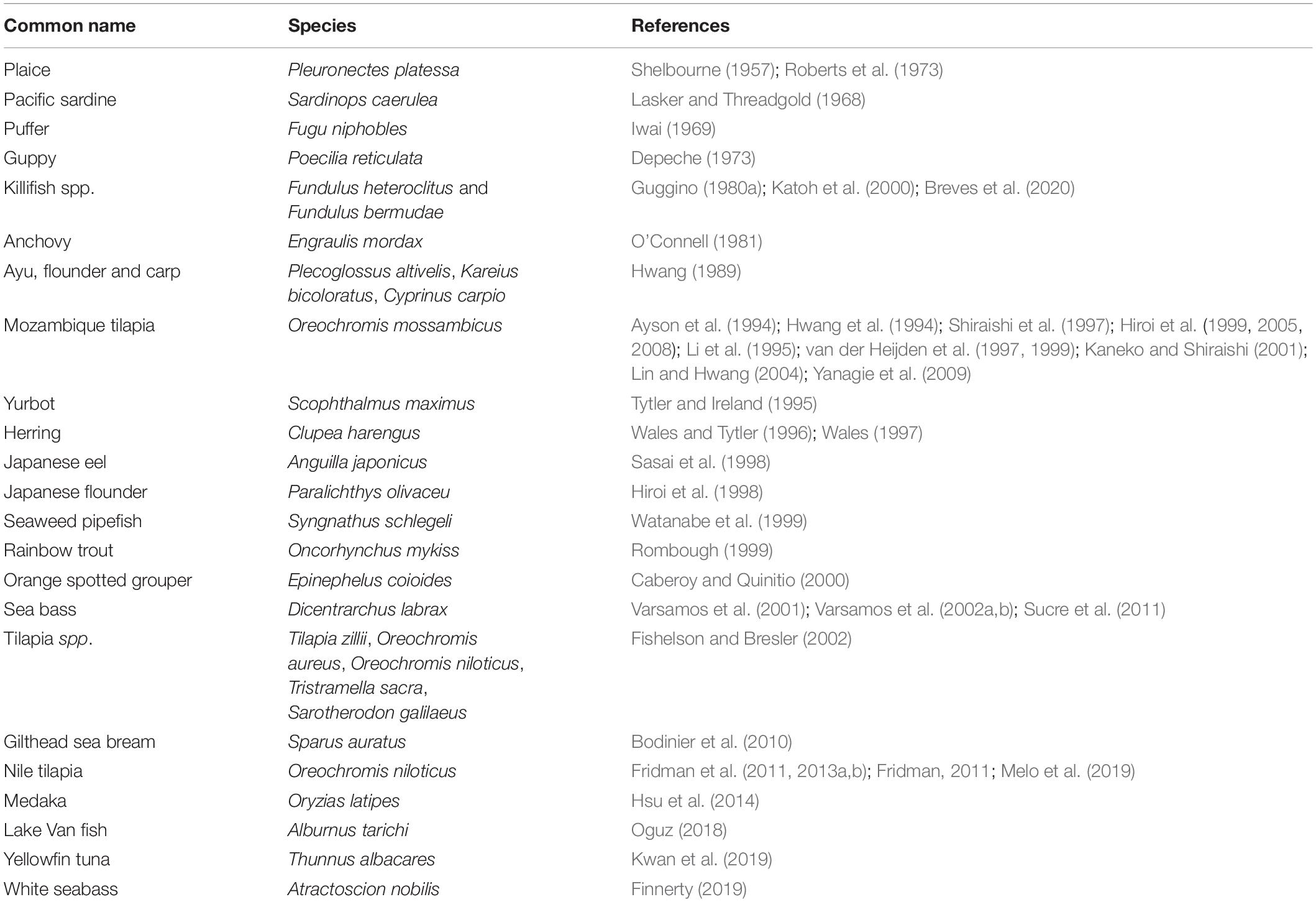
Table 1. Reports on the presence of extrabranchial ionocytes during embryonic and post-embryonic stages of commercially important teleosts.
In general, embryonic and larval integumental ionocytes appear structurally and biochemically similar to adult branchial ionocytes (Figure 2). Ayson et al. (1994) using transmission electron microscope to examine ionocytes in the yolk-sac membrane of freshwater and seawater-adapted O. mossambicus tilapia embryos and larvae noted a similarity with ionocytes in branchial and opercular epithelium of the adult fish; the cytoplasm of the ionocytes was seen to contain numerous mitochondria and Na+/K+- ATPase located on the extensive and well-developed tubular system. In addition, SEM indicated clear changes in the size and structure of apical openings in integumental ionocytes as a response to changes in salinity, as displayed in adults (Figure 3). Correspondingly, van der Heijden et al. (1999), using immunostaining of cross sections of whole tilapia larvae (O. mossambicus) with an antibody against α -subunit of Na+/K+- ATPase, found extrabranchial ionocytes (from 24 h post-hatch onward) in both freshwater and seawater adapted larvae to be ultrastructurally similar to that of ionocytes in the branchial epithelium of adult fish. In addition, Shiraishi et al. (1997) reported the presence of MCCs (see Section “Accessory Cells” below) in the yolk-sac membrane of seawater–adapted tilapia larvae O. mossambicus.
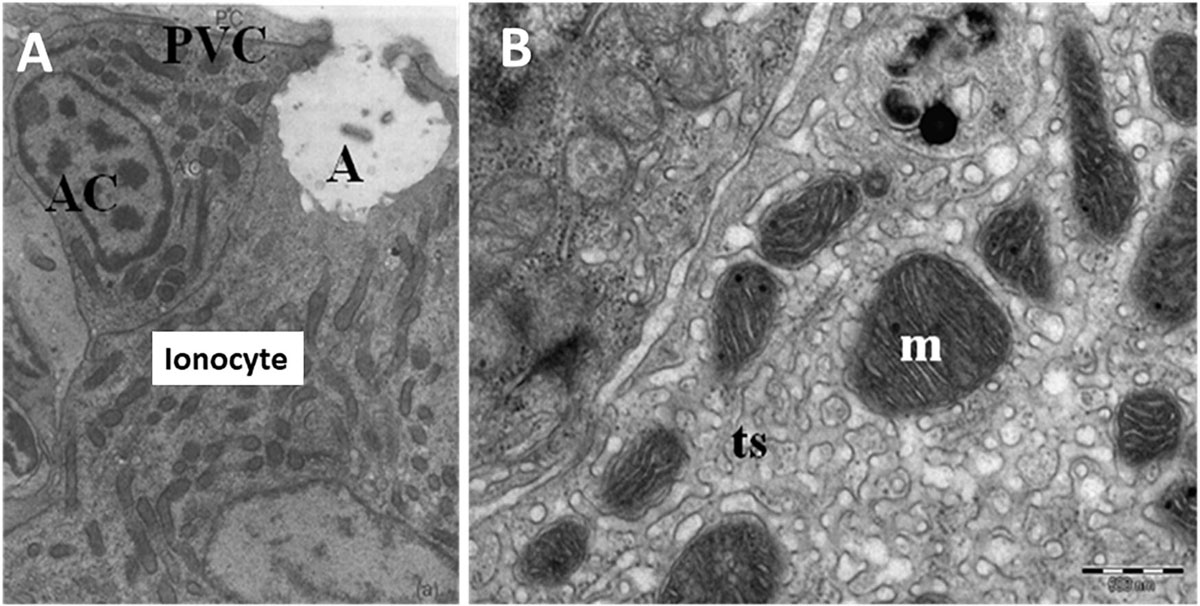
Figure 2. Ultrastructure of ionocytes in freshwater adapted Oreochromis niloticus larvae. (A) A multicellular complex (MCC) formed by a mature ionocyte and an accessory cell (AC) sharing a single apical crypt (A) lying beneath a pavement cell (PVC). Reduced osmium staining; x 11,900 and (B) Detail of mitochondria with tubular system (m; mitochondria, ts; tubular system) (Bar = 500 nm) (Fridman, 2011).
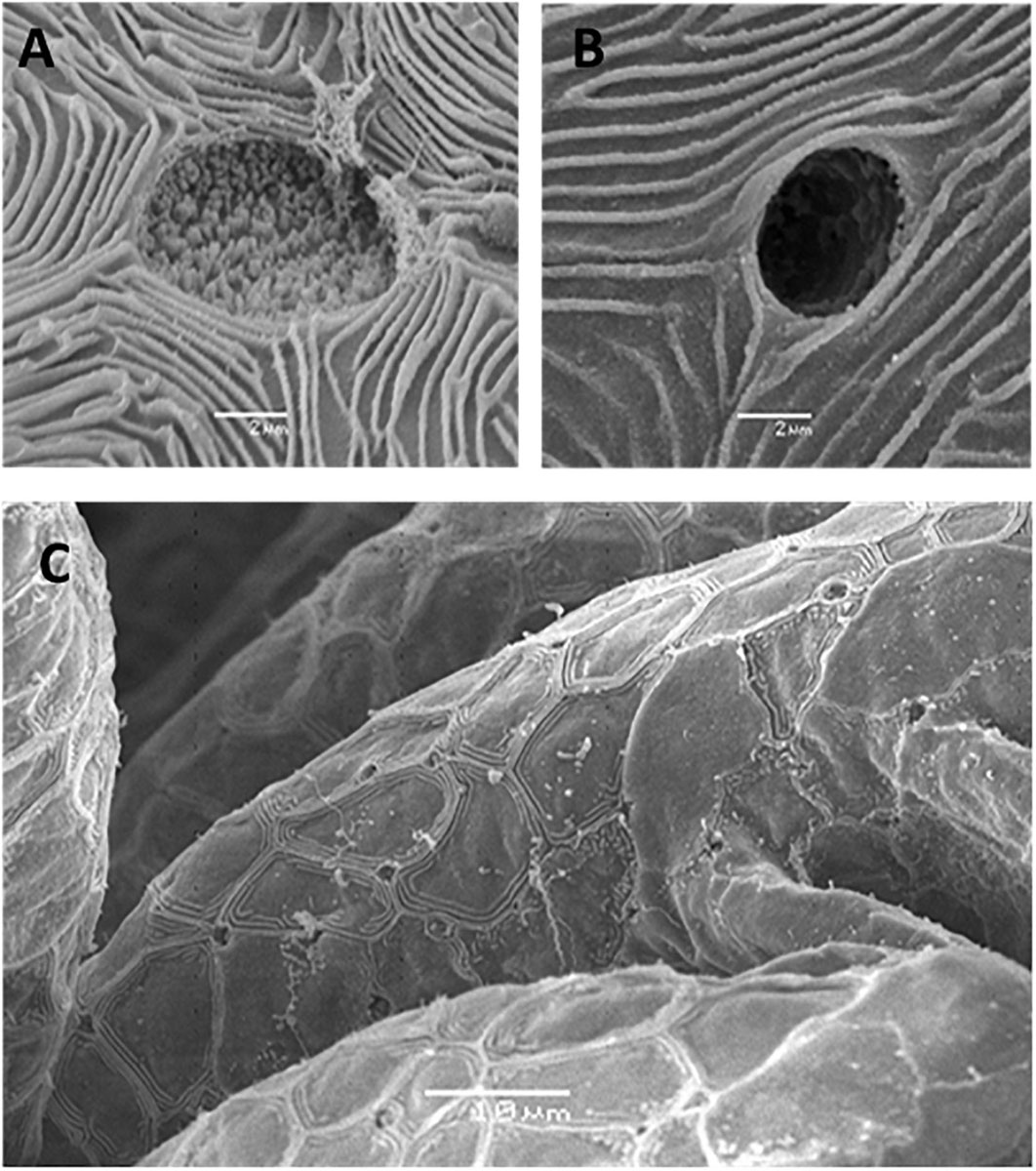
Figure 3. Scanning electron micrographs of external morphology of ionocytes during early life stages. (A) Apical opening of an ionocytes on yolk-sac epithelia of Nile tilapia in freshwater adapted larvae at hatch (Bar = 2 μm), (B) Apical opening of an ionocyte on yolk-sac epithelia of Nile tilapia in brackish water adapted larvae at hatch (Bar = 2 μm) and (C) Lower magnification of apical openings of an ionocyte on gill filaments of freshwater larvae at 3 dph (Bar = 10 μm) [from Fridman et al. (2011)].
Integumental Ionocytes During Embryonic and Post-embryonic Stages
The first appearance of ionocytes in fish embryos was reported on the yolk-sac epithelia of dechorionated Mozambique tilapia (O. mossambicus) embryos as early as 26 h post-fertilization, but no apical crypt to indicate functionality was apparent until 48 h post-fertilization (Lin et al., 1999). Similarly, Ayson et al. (1994), using SEM and TEM, observed ionocytes distributed underneath the pavement cells on the yolk-sac epithelium of Mozambique tilapia (O. mossambicus) embryos at 30 h post-fertilization in both freshwater and seawater but were presumed to be not yet functional as no apical openings were noted. Apical openings of ionocytes were first observed, albeit at a low density, at 48 h post-fertilization or half-way to hatching.
The site of active ionoregulation in the integument of post-hatch or post-embryonic teleost larvae was first demonstrated by Shelbourne (1957) who investigated the chloride regulation sites in the European plaice larvae (P. platessa) and, since then, integumental ionocytes have been reported in the post-embryonic stages of many commercially important species (see Table 1). There exists a distinct spatial shift in ionocyte distribution in both freshwater and marine teleosts; it is generally accepted that integumental ionocytes are initially responsible for osmoregulation prior to development of the adult osmoregulatory organs in O. mossambicus (Ayson et al., 1994; Shiraishi et al., 1997; Hiroi et al., 1999), O. niloticus (Fridman et al., 2011; see Figure 4) killifish (F. heteroclitus) (Katoh et al., 2000) and gilthead seabream (S. auratus) (Bodinier et al., 2010). The extrabranchial integument that can potentially be occupied by larval ionocytes comprises the yolk-sac, head, trunk and fins (Varsamos et al., 2005). Distribution of ionocytes in the integuments can also clearly be seen to be species dependant (Varsamos et al., 2005) and vary ontogenetically (Wales and Tytler, 1996; Fishelson and Bresler, 2002; Janicke et al., 2007; Bodinier et al., 2010).
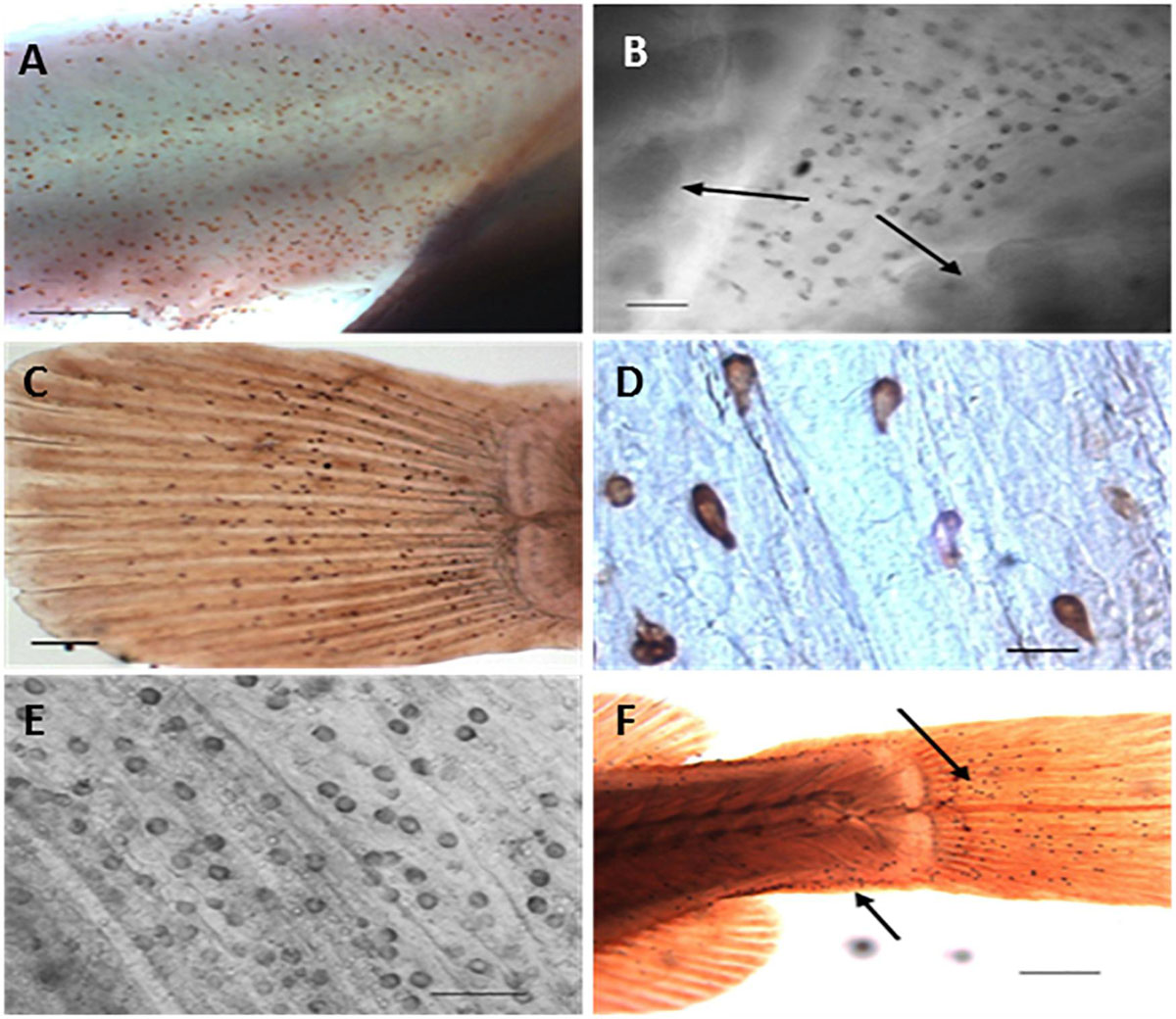
Figure 4. Distribution of ionocytes as revealed by anti-Na+/K+-ATPase antibody during post-embryonic development of Nile tilapia (Oreochromis niloticus) using light microscopy. (A) Detail of anal region of freshwater adapted larvae at 3 days post hatch (dph showing clustered immunoreactive ionocytes (Bar = 200 μm), (B) Ionocytes on ventral region of brackish water adapted larvae at 3 dph. Arrows indicates presence of gills underlying opercula (Bar = 30 μm), (C) Caudal fin of freshwater adapted larvae at 3 dph showing immunoreactive ionocytes (Bar = 200 μm) (LM), (D) Detail of immunoreactive ionocytes on caudal fin of brackish water adapted larvae at 3 dph (Bar = 20 μm), (E) Inner opercular area of freshwater adapted larvae at 5 dph showing immunoreactive ionocytes (Bar = 50 μm) (LM) and (F) Caudal extremity of brackish water adapted larvae at 7 dph. Arrows indicate location of clustered immunoreactive ionocytes (Bar = 300 μm) [from Fridman et al. (2011)].
Gill Development of the Role of Branchial Ionocytes During the Post-embryonic Period
A general feature of early fish larvae is the absence of fully developed gills (Segner et al., 1994), and the ontogeny of the gills forms an important part of the developmental process of the embryonic and larval fish. The sequence of gill development is described by Hughes (1984) as “continuous” with the epithelium that forms the surface of the gill arches becoming the surface of the filament and afterward the surface of the lamellae (Figure 5). Coinciding with this development is the maturation of other parts of the respiratory and cardiovascular system and coordination of the pumping systems for water and blood flow through the gills immediately prior to metamorphosis (Rombough, 2004; Figure 6).
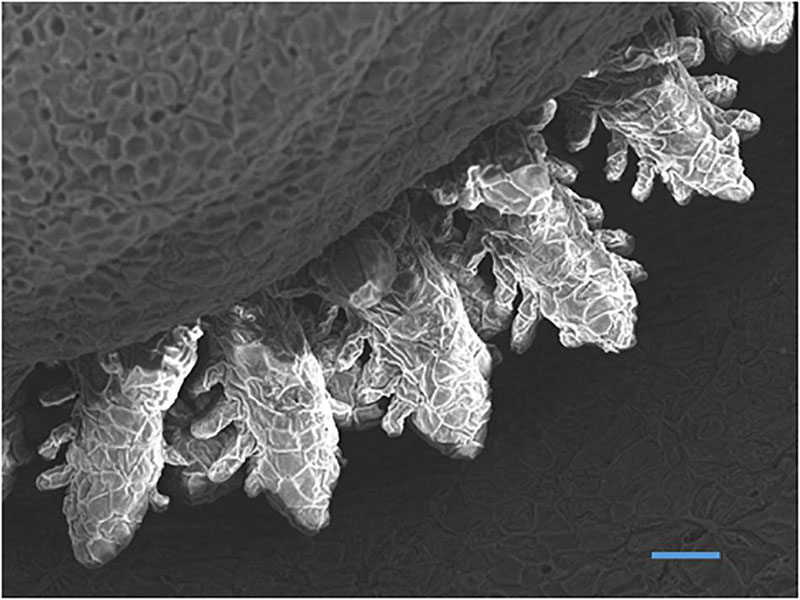
Figure 5. Scanning electron micrograph of developing gills in yolk-sac larvae of Nile tilapia at hatch showing filaments with budding secondary lamellae (Bar = 50 μm) (Fridman, 2011).
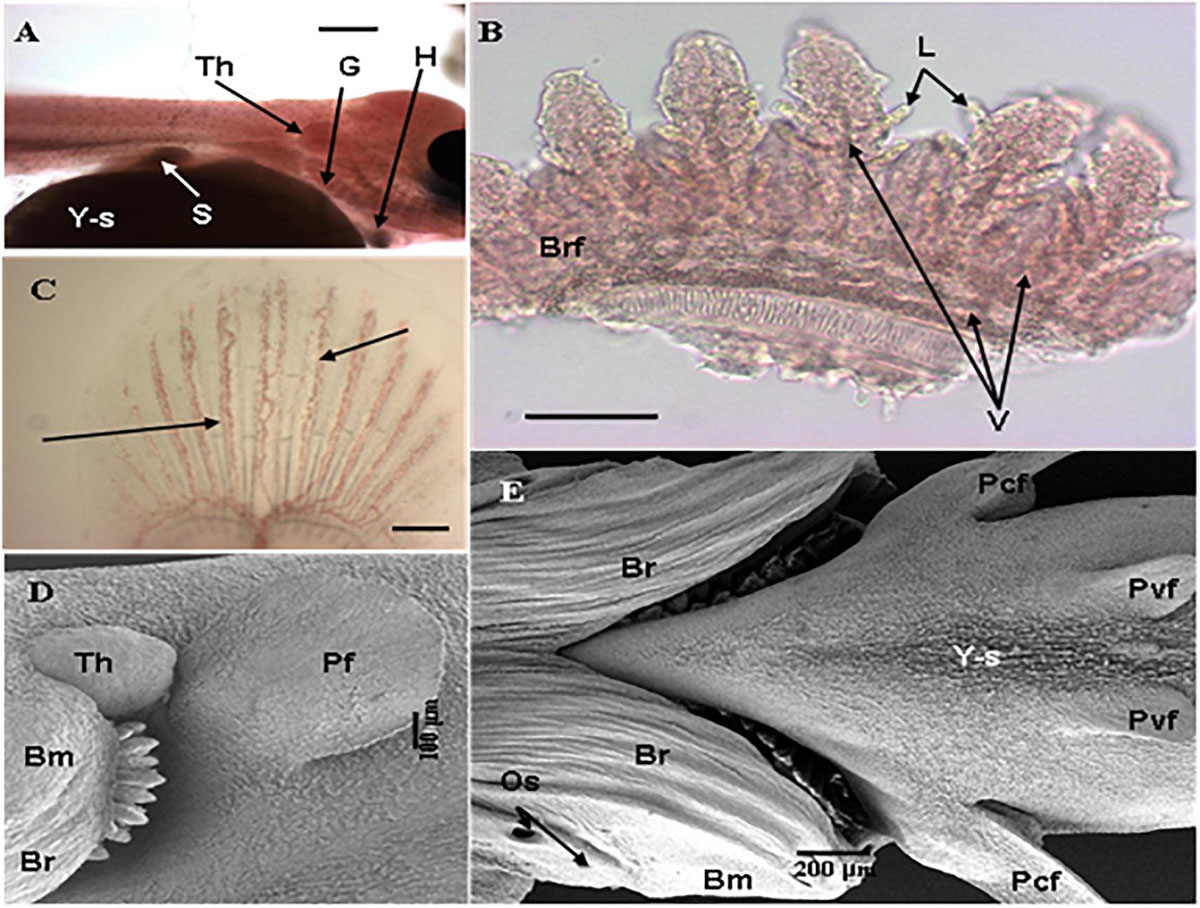
Figure 6. Development of branchial system and vasculature in Nile tilapia. (A) Freshwater adapted larvae at 1 dph showing gills (G), budding thymus (Th), heart (H), yolk-sac (Y-s) and stomach (S) (Bar = 500 μm) (LM), (B) Detail of branchial arch of freshwater adapted larvae at 1 dph showing pairs of hemibranchs or branchial filaments (Brf) with emergent lamellae (L) with clearly defined vasculature (V) (arrows) (Bar = 100 μm) (LM), (C) Developing caudal fin of larvae adapted to brackish water at 3 dph showing vasculature (arrow) (Bar = 200 μm) (LM), (D) Freshwater adapted larvae 3 dph showing pectoral fin (Pf), prominent thymus (Th) and branchiostegal membrane or operculum with visible branchiostegal rays (Br) partly covering gill arches and developing gills (Bar = 100 μm) (SEM) and (E) Underside of brackish water adapted larvae at 7 dph showing gills completely covered by the fully-defined branchiostegal membrane (Bm) with branchiostegal rays (Br), opercular spiracles (Os) and pectoral (Pcf) and pelvic fins (Pvf) developing on shrunken yolk-sac (Y-s) (Bar = 200 μm) (SEM) [from Fridman et al. (2011)].
It is widely accepted that gills in fish larvae have an ionoregulatory function before a respiratory function, however, the exact timing of ionocyte functionality in the fish gill is a matter of debate (Alderdice, 1988); less is known about the ontogeny of branchial ionocytes in fish larvae, with the majority of osmoregulatory studies in embryos and larvae focusing on integumental ionocytes. However, a clearly defined temporal staging of the appearance of ionocytes, conferring ability to cope with varying environmental conditions during early development, is evident throughout the yolk-sac period. An ontogenic transfer of regulative, osmoregulatory function from the integumental system to the developing branchial epithelial sites, culminating in the fully-functioning, branchial ionocytes has been widely reported, i.e., the sea water flounder (K. bicoloratus) (Hwang, 1989), the summer flounder (P. dentatus) (Schreiber and Specker, 1998), the rainbow trout O. mykiss (Gonzalez et al., 1996; Rombough, 1999), the trout (S. trutta) (Rojo et al., 1997), the Japanese flounder (P. olivaceus) (Hiroi et al., 1998), the guppy (P. reticulata) (Shikano and Fujio, 1998a,b), the Nile tilapia (O. niloticus) (Fridman et al., 2011, 2013a,b), Mozambique tilapia (O. mossambicus) (Li et al., 1995; van der Heijden et al., 1999) and the killifish (F. heteroclitus) (Katoh et al., 2000), sea bream (D. labrax) (Varsamos et al., 2005; Bodinier et al., 2009) and the gilthead seabream (S. auratus) (Bodinier et al., 2010).
Summary
This review has outlined the main physiological changes and adaptations in osmoregulatory capacity that occur throughout the early life stages of teleosts and the role of the ionoregulatory cell or ionocyte in conserving physiological homeostasis. It describes the different ionocyte types relative to their environment, i.e., freshwater or seawater, their plasticity in form and function and discusses spatio-temporal changes in integumental ionocyte distribution that occur during gastrulation and embryonic and post-embryonic stages, prior to transfer of full regulative function to the osmoregulatory organs of the adult teleost, i.e., branchial chambers, skin, digestive system and urinary organs.
Author Contributions
The author confirms being the sole contributor of this work and has approved it for publication.
Conflict of Interest
The authors declare that the research was conducted in the absence of any commercial or financial relationships that could be construed as a potential conflict of interest.
Acknowledgments
The author acknowledges that this work is an expansion of ideas originally presented in her Ph.D. thesis “Ontogeny of osmoregulation in the Nile tilapia (Oreochromis niloticus L.)”.
References
Alderdice, D. F. (1988). “Osmotic and ionic regulation in teleost eggs and larvae,” in Fish Physiology: vol. XIA, eds W. S. Hoar and D. J. Randall (New York: Academic Press), 163–251. doi: 10.1016/s1546-5098(08)60200-9
Ayson, F. G., Kaneko, T., Hasegawa, S., and Hirano, T. (1994). Development of mitochondria-rich cells in the yolk-sac membrane of embryos and larvae of tilapia, Oreochromis mossambicus, in fresh water and seawater. J. Exp. Zool. 270, 129–135. doi: 10.1002/jez.1402700202
Bennett, A. F., Dawson, W. R., and Putnam, R. W. (1981). Thermal environment and tolerance of embryonic Western Gulls. Physiol. Zool. 54, 146–154. doi: 10.1086/physzool.54.1.30155813
Blondeau-Bidet, E., Hiroi, J., and Lorin-Nebel, C. (2019). Ion uptake pathways in European sea bass Dicentrarchus labrax. Gene 692, 126–137. doi: 10.1016/j.gene.2019.01.006
Bodinier, C., Boulo, V., Lorin-Nebel, C., and Charmantier, G. (2009). Influence of salinity on the localization and expression of the CFTR chloride channel in the ionocytes of Dicentrarchus labrax during ontogeny. J. Anat. 214, 318–329. doi: 10.1111/j.1469-7580.2009.01050.x
Bodinier, C., Sucre, E., Lecurieux-Belfond, L., Blondeau-Bidet, E., and Charmantier, G. (2010). Ontogeny of osmoregulation and salinity tolerance in the gilthead sea bream Sparus aurata. Comp. Biochem. Physiol. A 157, 220–228. doi: 10.1016/j.cbpa.2010.06.185
Breves, J. P., Starling, J. A., Popovski, C. M., Doud, J. M., and Tipsmark, C. K. (2020). Salinity-dependent expression of ncc2 in opercular epithelium and gill of mummichog (Fundulus heteroclitus). J. Comp. Physiol. B 190, 219–230. doi: 10.1007/s00360-020-01260-x
Burns, J., and Copeland, D. E. (1950). Chloride excretion in the head region of Fundulus heteroclitus. Biol. Bull. 99, 381–385. doi: 10.2307/1538468
Caberoy, N., and Quinitio, G. (2000). Changes in Na+,K+-ATPase activity and gill chloride cell morphology in the grouper Epinephelus coioides larvae and juveniles in response to salinity and temperature. Fish Physiol. Biochem. 23, 83–94.
Cerdà, J., and Finn, R. N. (2010). Piscine aquaporins: an overview of recent advances. J. Exp. Zool. A Ecol. Genet. Physiol. 313, 623–650. doi: 10.1002/jez.634
Cerdà, J., Chauvigné, F., and Finn, R. N. (2017). “The physiological role and regulation of aquaporins in teleost germ cells,” in Aquaporins. Advances in Experimental Medicine and Biology, Vol. 969, ed. B. Yang (Dordrecht: Springer). doi: 10.1086/319304
Chang, I.C., Lee, T.H., Yang, C.H., Wei, Y.W., Chou, F.I., and Hwang, P.P. (2001). Morphology and function of gill mitochondria-rich cells in fish acclimated to different environments. Physiol. Biochem. Zool. 74, 111–119.
Chang, I. C., Wei, Y. Y., Chou, F. I., and Hwang, P. P. (2003). Stimulation of Cl- uptake and morphological changes in gill mitochondria-rich cells in freshwater tilapia (Oreochromis mossambicus). Physiol. Biochem. Zool. 76, 544–552. doi: 10.1086/375438
Chang, W. J., and Hwang, P. P. (2011). Development of zebra fish epidermis. Birth Defects Res. C 93, 205–214.
Chang, W. J., Wang, Y. F., Hu, H. J., Wang, J. H., Lee, T. H., and Hwang, P. P. (2013). Compensatory regulation of Na+ absorption by Na+/H+ exchanger and Na+ Cl- cotransporter in zebrafish (Danio rerio). Front. Zool. 10:46. doi: 10.1186/1742-9994-10-46
Christensen, A. K., Hiroi, J., Schultz, E. T., and Mc Cormick, S. D. (2012). Branchial ionocyte organisation and ion-transport protein expression in juvenile alewives acclimated to freshwater or seawater. J. Exp. Biol. 215, 642–652. doi: 10.1242/jeb.063057
Copeland, D. E. (1948). The cytological basis of chloride transfer in the gills of Fundulus heteroclitus. J. Morphol. 82, 201–227. doi: 10.1002/jmor.1050820204
Cutler, C. P., and Cramb, G. (2002). Branchial expression of an aquaporin 3 (AQP-3) homologue is downregulated in the European eel (Anguilla anguilla) following seawater acclimation. J. Exp. Biol. 205, 2643–2651.
Dahlke, F., Lucassen, M., Bickmeyerm, U., Wohlrab, S., Puvanendran, V., Mortensenm, A., et al. (2020). Fish embryo vulnerability to combined acidification and warming coincides with a low capacity for homeostatic regulation. J. Exp. Biol. 223(Pt 11), jeb212589. doi: 10.1242/jeb.212589
D’Cotta, H., Valotaire, C., le Gac, F., and Prunet, P. (2000). Synthesis of gill Na+/K+-ATPase in Atlantic salmon smolts: differences in α-mRNA and α-protein levels. Am. J. Physiol. 278, 101–110.
Degnan, K. J., and Zadunaisky, J. A. (1980). Passive sodium movements across the opercular epithelium: the paracellular shunt pathway and ionic conductance. J. Membr. Biol. 55, 175–185. doi: 10.1007/bf01869459
Depeche, J. (1973). Infrastructure superficielle de la vesicule vitelline et dusac pericardique de l’embryon de Poecilia reticulata (Poisson Teleosteen). Z. Zellforsch. 141, 235–253. doi: 10.1007/bf00311356
De Rengis, G., and Bornancin, M. (1984). “Ion transport and gill ATPases,” in Fish Physiology Vol X, eds W. S. Hoar and D. J. Randall (London: Academic Press), 65–104. doi: 10.1016/s1546-5098(08)60182-x
Doyle, W. L., and Gorecki, D. (1961). The so-called chloride cell of the fish gill. Physiol. Zool. 34, 81–85. doi: 10.1086/physzool.34.2.30152686
Dymowska, A., Hwang, P. P., and Goss, G. G. (2012). Structure and function of ionocytes in the freshwater fish gill. Respir. Physiol. Neurobiol. 184, 282–292. doi: 10.1016/j.resp.2012.08.025
Evans, D. H. (1999). Ionic transport in the fish gill epithelium. J. Exp. Zool. 283, 641–652. doi: 10.1002/(sici)1097-010x(19990601)283:7<641::aid-jez3>3.0.co;2-w
Evans, D. H., and Claiborne, J. B. (2009). “Osmotic and ionic regulation in fishes,” in Osmotic and Ionic Regulation: Cells and Animals, ed. D. H. Evans (Boca Raton, FL: Taylor and Francis Group), 295–366. doi: 10.1201/9780849380525.ch8
Evans, D. H., Piermarini, P. M., and Choe, K. P. (2005). The multifunctional fish gill: dominant site of gas exchange, osmoregulation, acid-base regulation and excretion of nitrogenous waste. Physiol. Rev. 85, 97–117.
Finnerty, S. H. (2019). Tracking Ontogenetic Changes of Ionocyte Distribution and Morphology in Larval Seabass (Atractoscion nobilis). M.Sc. Thesis. San Francisco, CA: University of California.
Fiol, D. F., and Kültz, D. (2007). Osmotic stress sensing and signaling in fishes. FEBS J. 274, 5790–5798. doi: 10.1111/j.1742-4658.2007.06099.x
Fishelson, L., and Bresler, V. (2002). Comparative studies of the development and differentiation of chloride cells in tilapiine fish with different reproductive styles. J. Morph. 253, 118–131. doi: 10.1002/jmor.1115
Foskett, J. K., and Scheffey, C. (1982). The chloride cell: definitive identification as the salt-secretory cell in teleosts. Science 8, 164–166. doi: 10.1126/science.7053566
Fridman, S. (2011). Ontogeny of Osmoregulation in the Nile tilapia (Oreochromis niloticus L.). Ph.D. thesis. Stirling: University of Stirling.
Fridman, S., Bron, J. E., and Rana, K. J. (2011). Ontogenetic changes in location and morphology of chloride cells during early life stages of the Nile tilapia (Oreochromis niloticus (L.) adapted to freshwater and brackish water. J. Fish Biol. 79, 597–614. doi: 10.1111/j.1095-8649.2011.03043.x
Fridman, S., Rana, K. J., and Bron, J. E. (2013a). Confocal scanning laser microscopy with complementary 3-D image analysis allows quantitative studies of functional state of ionoregulatory cells in the Nile tilapia (Oreochromis niloticus) following salinity challenge. Microscope Res. Tech. 76, 412–418. doi: 10.1002/jemt.22181
Fridman, S., Rana, K. J., and Bron, J. E. (2013b). Structural differentiation of apical openings in active mitochondria-rich cells during early life-stages of Nile tilapia (Oreochromis niloticus) as a response to osmotic challenge. Fish Physiol. Biochem. 39, 1101–1114. doi: 10.1007/s10695-012-9767-1
Froese, R., and Pauly, D. (2012). FishBase.World Wide Web electronic publication. Available online at: www.fishbase.org, version 08/2012 (accessed July 27, 2020).
Galvez, F., Reid, S. D., Hawkings, G., and Goss, G. G. (2002). Isolation and characterization of mitochondria-rich cell types from the gill of freshwater rainbow trout. Am. J. Physiol. Regul. Integr. Comp. Physiol. 282, R658–R668.
García-Ayala, A., García-Hernández, M. P., Quesada, J. A., and Agulleiro, B. (1997). Immunocytochemical and ultrastructural characterization of prolactin, growth hormone, and somatolactin cells from the Mediterranean yellowtail (Seriola dumerilii, Risso 1810). Anat. Rec. 247, 395–404. doi: 10.1002/(SICI)1097-0185(199703)247:3<395::AID-AR11>3.0.CO;2-K
Gonzalez, M. E., Blanquez, M. J., and Rojo, C. (1996). Early gill development in the rainbow trout. Oncorhynchus mykiss. J. Morphol. 229, 201–217. doi: 10.1002/(sici)1097-4687(199608)229:2<201::aid-jmor5>3.0.co;2-3
Goss, G. G., Laurent, P., and Perry, S. F. (1992). Evidence for a morphological component in acid-base regulation during environmental hypercapnia in the brown bullhead (Ictalurus nebulosus). Cell Tissue Res. 268, 539–552. doi: 10.1007/bf00319161
Goss, G. G., and Perry, S. F. (1994). Different mechanisms of acidbase regulation in rainbow trout (Oncorhynchus mykiss) and American eel (Anguilla rostrata) during NaHCO3 infusion. Physiol. Zool. 67, 381–406. doi: 10.1086/physzool.67.2.30163854
Guggino, W. B. (1980b). Water balance in embryos of Fundulus heteroclitus and F. bermudae in seawater. Am. J. Physiol. 238, 36–41.
Guggino, W. B. (1980a). Salt balance in embryos of Fundulus heteroclitus and F. bermudae adapted to seawater. Am. J. Physiol. 238, 42–49.
Halstead, L. B. (1985). The vertebrate invasion of fresh water. Philos. Trans. R. Soc. B Biol. Sci. 309, 243–258. doi: 10.1098/rstb.1985.0085
Hirano, T., Morisawa, M., and Suzuki, K. (1978). Changes in plasma and ceolomic fluid composition of the mature salmon (Oncorhynchus keta) during freshwater adaptation. J. Comp. Biochem. Physiol. A 61, 5–8. doi: 10.1016/0300-9629(78)90266-9
Hiroi, J., Kaneko, T., Seikai, T., and Tanaka, M. (1998). Developmental sequence of chloride cells in the body skin and gills of Japanese flounder (Paralichthys olivaceus) larvae. Zool. Sci. 15, 455–460. doi: 10.2108/zsj.15.455
Hiroi, J., Kaneko, T., and Tanaka, M. (1999). In vivo sequential changes in chloride cell morphology in the yolk-sac membrane of Mozambique Tilapia (Oreochromis mossambicus) embryos and larvae during seawater adaptation. J. Exp. Biol. 202, 3485–3495.
Hiroi, J., McCormick, S. D., Ohtani-Kaneko, R., and Kaneko, T. (2005). Functional classification of mitochondrion-rich cells in euryhaline Mozambique tilapia (Oreochromis mossambicus) embryos, by means of triple immunofluorescence staining for Na+/K+-ATPase, Na+/K+/2Cl- cotransporter and CFTR anion channel. J. Exp. Biol. 208, 2023–2036. doi: 10.1242/jeb.01611
Hiroi, J., Yasumasu, S., McCormick, S. D., Hwang, P. P., and Kaneko, T. (2008). Evidence for an apical Na-Cl co-transporter involved in ion uptake in a teleost fish. J. Exp. Biol. 211, 2584–2599. doi: 10.1242/jeb.018663
Hiroi, J., and McCormick, S. D. (2012). New insights into gill ionocyte and ion transporter function in euryhaline and diadromous fish. Respir. Physiol. Neurobiol. 184, 257–268. doi: 10.1016/j.resp.2012.07.019
Hirose, S., Kaneko, T., Naito, N., and Takei, Y. (2003). Molecular biology of major components of chloride cells. Comp. Biochem. Physiol. Part B 136, 593–620. doi: 10.1016/s1096-4959(03)00287-2
Hootman, S. R., and Philpott, C. W. (1980). Accessory cells in teleost branchial epithelium. Am. J. Physiol. 238, 185–198.
Holliday, F. G. T. (1965). Osmoregulation in marine teleost eggs and larvae. Calif. Coop. Oceanic Fish. Invest. Rep. 10, 89–95.
Hsu, H. H., Lin, L. Y., Tseng, Y. C., Horng, J. L., and Hwang, P. P. (2014). A new model for fish ion regulation: identification of ionocytes in freshwater- and seawater-acclimated medaka (Oryzias latipes). Cell Tissue Res. 357, 225–243. doi: 10.1007/s00441-014-1883-z
Hughes, G. M. (1984). “General anatomy of the gills,” in Fish Physiology, vol. X(A), eds W. S. Hoar and J. Randall (London: Academic Press).
Hwang, P. P. (1988). Multicellular complex of chloride cells in the gills of freshwater teleosts. J. Morphol. 196, 15–22. doi: 10.1002/jmor.1051960103
Hwang, P. P. (1989). Distribution of chloride cells in teleost larvae. J. Morphol. 200, 1–8. doi: 10.1002/jmor.1052000102
Hwang, P. P., Tsai, Y. N., and Tung, Y. C. (1994). Calcium balance in embryos and larvae of the freshwater-adapted teleost Oreochromis mossambicus. Fish. Physiol. Biochem. 13, 325–333. doi: 10.1007/bf00003437
Hwang, P. P., Lee, T. H., and Lin, L. Y. (2011). Ion regulation in fish gills: recent progress in the cellular and molecular mechanisms. Am. J. Physiol. Regul. Integr. Comp. Physiol. 301, R28–R47.
Hwang, P. P., and Chou, M. Y. (2013). Zebrafish as an animal model to study ion homeostasis. Pflugers. Arch. 465, 1233–1247. doi: 10.1007/s00424-013-1269-1
Hwang, P. P., and Lin, L. Y. (2013). “Gill ionic transport, acid-base regulation, and nitrogen excretion,” in The Physiology of Fishes, 4th Edn, eds D. H. Evans and J. B. Claiborne (Boca Raton, FL: CRC Press), 205–233.
Inokuchi, M., Hiroi, J., Watanabe, S., Lee, K. M., and Kaneko, T. (2008). Gene expression and morphological localization of NHE3, NCC and NKCC1a in branchial mitochondria-rich cells of Mozambique tilapia (Oreochromis mossambicus) acclimated to a wide range of salinities. Comp. Biochem. Physiol. A 151, 151–158. doi: 10.1016/j.cbpa.2008.06.012
Inokuchi, M., Hiroi, J., Watanabe, S., Hwang, P. P., and Kaneko, T. (2009). Morphological and functional classification of ion-absorbing mitochondria-rich cells in the gills of Mozambique tilapia. J. Exp. Biol. 212, 1003–1010. doi: 10.1242/jeb.025957
Inokuchi, M., Nakamura, M., Miyanishi, H., Hiroi, J., and Kaneko, T. (2017). Functional classification of gill ionocytes and spatiotemporal changes in their distribution after transfer from seawater to freshwater in Japanese seabass. J. Exp. Biol. 220, 4720–4732. doi: 10.1242/jeb.167320
Iwai, T. (1969). On the chloride cells in the skin of larval puffer, Fugu niphobles (Jordan and Snyder). La Mer 72, 6–31.
Janicke, M., Carney, T. J., and Hammerschmidt, M. (2007). Foxi3 transcription factors and Notch signaling control the formation of skin ionocytes from epidermal precursors of the zebrafish embryo. Dev. Biol. 307, 258–271. doi: 10.1016/j.ydbio.2007.04.044
Kang, C. K., Tsai, S. C., Lee, T. H., and Hwang, P. P. (2008). Differential expression of branchial Na+/K+-ATPase of two medaka species, Oryzias latipes and Oryzias dancena, with different salinity tolerances acclimated to freshwater, brackishwater and seawater. Comp. Biochem. Physiol. A 151, 566–575. doi: 10.1016/j.cbpa.2008.07.020
Kang, C. K., Tsai, H. J., Liu, C. C., Lee, T. H., and Hwang, P. P. (2010). Salinity dependent expression of a Na+, K +, 2Cl - cotransporter in gills of the brackish medaka Oryzias dancena: a molecular correlate for hyposmoregulatory endurance. Comp. Biochem. Physiol. A 157, 7–18. doi: 10.1016/j.cbpa.2010.05.013
Karnaky, K. J. (1986). Structure and function of the chloride cell of Fundulus heteroclitus and other teleosts. Am. Zool. 26, 209–224. doi: 10.1093/icb/26.1.209
Katoh, F., Shimizu, A., Uchida, K., and Kaneko, T. (2000). Shift of chloride cell distribution during early life stages in seawater-adapted killifish (Fundulus heteroclitus). Zool. Sci. 17, 11–18. doi: 10.2108/zsj.17.11
Katoh, F., Hasegawa, S., Kita, J., Takagi, Y., and Kaneko, T. (2001). Distinct seawater and freshwater types of chloride cells in killifish, Fundulus heteroclitus. Can. J. Zool. 79, 822–829. doi: 10.1139/z01-042
Katoh, F., Hyodo, S., and Kaneko, T. (2003). Vacuolar-type proton pump in the basolateral plasma membrane energizes ion uptake in branchial mitochondria-rich cells of killifish Fundulus heteroclitus, adapted to a low ion environment. J. Exp. Biol. 206, 793–803. doi: 10.1242/jeb.00159
Kaneko, T., Hasegawa, S., Takagi, Y., Tagawa, M., and Hirano, T. (1995). Hypo-osmoregulatory ability of eyed-stage embryos of chum salmon. Mar. Biol. 122, 165–170. doi: 10.1007/bf00349290
Kaneko, T., and Shiraishi, K. (2001). Evidence for chloride secretion from chloride cells in the yolk-sac membrane of Mozambique tilapia larvae adapted to seawater. Fish. Sci. 67, 541–543. doi: 10.1046/j.1444-2906.2001.00290.x
Keys, A. B., and Willmer, E. N. (1932). Chloride secreting cells in the gills of fishes with special reference to the common eel. J. Physiol. 76, 368–378. doi: 10.1113/jphysiol.1932.sp002932
King, J. A. C., Abel, D. C., and DiBona, D. R. (1989). Effects of salinity on chloride cells in the euryhaline cyprinodontid fish Rivulus marmoratus. Cell Tissue Res. 257, 367–377. doi: 10.1007/bf00261839
Kinne, O. (1964). The effects of temperature and salinity on marine and brackish water animals. II. Salinity and temperature salinity combinations. Oceanogr. Mar. Biol. 2, 281–339.
Kwan, G. T., Wexler, J. B., Wegner, N. C., and Tresguerres, M. (2019). Ontogenetic changes in cutaneous and branchial ionocytes and morphology in yellowfish tuna (Thunnus albacares) larvae. J. Comp. Physiol. B 189, 81–95. doi: 10.1007/s00360-018-1187-9
Lasker, R., and Threadgold, L. T. (1968). Chloride cells in the skin of the larval sardine. Exp. Cell Res. 52, 582–590. doi: 10.1016/0014-4827(68)90498-9
Laurent, P. (1984). “Gill internal morphology,” in Fish Physiology, Vol. X, eds W. S. Hoar and D. J. Randall (New York, NY: Academic Press), 73–183. doi: 10.1016/s1546-5098(08)60318-0
Laurent, P., and Dunel, S. (1980). Morphology of gill epithelia in fish. Am. J. Physiol. 238, 147–159.
Laurent, P., Chevalier, C., and Wood, C. M. (2006). Appearance of cuboidal cells in relation to salinity in gills of Fundulus heteroclitus, a species exhibiting branchial Na+ but not Cl- uptake in freshwater. Cell Tissue Res. 325, 481–492. doi: 10.1007/s00441-006-0187-3
Lee, T. H., Hwang, P. P., Lin, H. C., and Huang, F. L. (1996). Mitochondria- rich cells in the branchial epithelium of the teleost, Oreochromis mossambicus, acclimated to various hypotonic environments. Fish Physiol. Biochem. 15, 513–523. doi: 10.1007/bf01874924
Lee, T. H., Hwang, P. P., Shieh, Y. E., and Lin, C. H. (2000). The relationship between ‘deep-hole’ mitochondria-rich cells and salinity adaptation in the euryhaline teleost, Oreochromis mossambicus. Fish Physiol. Biochem. 23, 133–140.
Li, J., Eygensteyn, J., Lock, R. A. C., Verbost, P. M., van der Heijden, A. J. H., Wendelaar Bonga, S. E., et al. (1995). Branchial chloride cells in larvae and juveniles of freshwater tilapia Oreochromis mossambicus. J. Exp. Biol. 198, 2177–2184.
Lin, L. Y., Weng, C. F., and Hwang, P. P. (1999). Effects of cortisol on ion regulation in developing tilapia (Oreochromis mossambicus). Physiol. Biochem. Zool. 72, 397–404. doi: 10.1086/316682
Lin, L. Y., Chiang, C. C., Gong, H. Y., Cheng, C. Y., Hwang, P. P., and Weng, C. F. (2003). Cellular distributions of creatine kinase in branchia of euryhaline tilapia (Oreochromis mossambicus). Am. J. Physiol. Cell Physiol. 284, 233–241.
Lin, L. Y., and Hwang, P. P. (2004). Activation and inactivation of mitochondria-rich cells in tilapia larvae acclimated to ambient chloride changes. J. Exp. Biol. 207, 1335–1344. doi: 10.1242/jeb.00869
Lin, L. Y., Horng, J. L., Kunkel, J. G., and Hwang, P. P. (2006). Proton pump-rich cell secretes acid in skin of zebrafish larvae. Am. J. Physiol. Cell. Physiol. 290, C371–C378.
Lin, C. C., Lin, L. Y., Hsu, H. H., Thermes, V., Prunet, P., Horng, J. L., et al. (2012). Acid secretion by mitochondrion-rich cells of medaka (Oryzias latipes) acclimated to acidic freshwater. Am. J. Physiol. Regul. Integr. Comp. Physiol. 302, R283–R291.
Madsen, S. S., Jensen, M. K., Nøhr, J., and Kristiansen, K. (1995). Expression of Na+-K+-ATPase in the brown trout, Salmo trutta: in vivo modulation by hormones and seawater. Am. J. Physiol. 269, 1339–1345.
Marshall, W. S. (2011). Mechanosensitive signalling in fish gill and other ion-transporting epithelia. Acta Physiol. 202, 487–499. doi: 10.1111/j.1748-1716.2010.02189.x
Marshall, W. S., Bryson, S. E., Darling, P., Whitten, C., Patrick, M., Wilkie, M., et al. (1997). NaCl transport and ultrastructure of opercular epithelium from a freshwater-adapted euryhaline teleost, Fundulus heteroclitus. J. Exp. Zool. 27, 213–237.
McCormick, S. D. (1995). “Hormonal control of gill Na+,K+-ATPase and chloride cell function,” in Fish Physiology: Cellular and Molecular Approaches to Fish Ionic Regulation, Vol. 14, eds C. M. Wood and T. J. Shuttleworth (London: Academic Press), 285–315. doi: 10.1016/s1546-5098(08)60250-2
McCormick, S. D., Farrell, A. P., and Brauner, C. J. (2013). “Fish physiology,” in Euryhaline Fishes, Vol. 32, eds A. P. Farrell and C. J. Brauner (Oxford: Academic Press), 558.
Melo, L. H., Martins, Y. S., Melo, R. M. C., and Prado, P. S. (2019). Low salinity negatively affects early larval development of Nile tilapia, Oreochromis niloticus: insights from skeletal muscle and molecular biomarkers. Zygote 27, 375–381. doi: 10.1017/s0967199419000431
O’Connell, C. P. (1981). Development of organ systems in the northern anchovy Engraulis mordax and other teleosts. Am. Zool. 21, 429–446. doi: 10.1093/icb/21.2.429
Oguz, A. R. (2018). Development of osmoregulatory tissues int eh Lake van fish (Alburnus tarichi) during larval development. Fish Physiol. Biochem. 44, 227–233. doi: 10.1007/s10695-017-0427-3
Perry, S. F., Goss, G. G., and Laurent, P. (1992). The interrelationships between gill chloride cell morphology and ionic uptake in four freshwater teleosts. Can. J. Zool. 70, 1775–1786. doi: 10.1139/z92-245
Philpott, C. W. (1966). The use of horseradish peroxidase to demonstrate functional continuity between the plasmalemma and the unique tubular system of the chloride cell. J. Cell Biol. 31, 86–91.
Philpott, C. W. (1980). Tubular system membranes of teleost chloride cells: osmotic response and transport sites. Am. J. Physiol. 238, 171–184.
Pisam, M., Caroff, A., and Rambourg, A. (1987). Two types of chloride cells in the gill epithelium of a freshwater-adapted euryhaline fish: Lebistes reticulatus; their modifications during adaptation to saltwater. Am. J. Anat. 179, 40–50. doi: 10.1002/aja.1001790106
Pisam, M., Boeuf, G., Prunet, P., and Rambourg, A. (1990). Ultrastructural features of mitochondria-rich cells in stenohaline freshwater and seawater fishes. Am. J. Anat. 187, 21–31. doi: 10.1002/aja.1001870104
Pisam, M., and Rambourg, A. (1991). Mitochondria-rich cells in the gill epithelium of teleost fishes: an ultrastructural approach. Int. Rev. Cyt. 130, 191–232. doi: 10.1016/s0074-7696(08)61504-1
Pisam, M., Auperin, B., Prunet, P., Rentier-Delrue, F., Martial, J., and Rambourg, A. (1993). Effects of prolactin on alpha and beta chloride cells in the gill epithelium of the saltwater adapted tilapia Oreochromis niloticus. Anat. Rec. 235, 275–284. doi: 10.1002/ar.1092350211
Prunet, P., and Bornancin, M. (1989). Physiology of salinity tolerance in tilapia: an uptake on basic and applied aspects. Aqu. Living Resour. 2, 91–97. doi: 10.1051/alr:1989011
Reid, S. D., Hawkings, G. S., Galvez, F., and Goss, G. G. (2003). Localization and characterization of phenamil-sensitive Na+ influx inisolated rainbow trout gill epithelial cells. J. Exp. Biol. 206, 551–559. doi: 10.1242/jeb.00109
Riegel, J. A. (1998). Analysis of fluid dynamics in perfused glomeruli of the hagfish Eptatretus stouti (Lockington). J. Exp. Biol. 201, 3097–3104.
Roberts, R., Bell, M., and Young, H. (1973). Studies of the skin of plaice (Pleuronectes platessa L.): II. The development of larval plaice skin. J. Fish Biol. 5, 103–108. doi: 10.1111/j.1095-8649.1973.tb04435.x
Rojo, M. C., Blanquez, M. J., and Gonzalez, M. E. (1997). Ultrastructural evidence for apoptosis of pavement cells, chloride cells and hatching gland cells in the developing branchial area of the trout Salmo trutta. J. Zool. 243, 637–651. doi: 10.1111/j.1469-7998.1997.tb02807.x
Rombough, P. J. (1999). The gill of fish larvae. Is it primarily a respiratory organ or an ionoregulatory structure? J. Fish Biol. 55, 186–204. doi: 10.1111/j.1095-8649.1999.tb01055.x
Rombough, P. J. (2004). Gas exchange, ionoregulation and the functional development of the teleost gill. Am. Fish. Soc. Symp. 40, 47–83.
Ruiz-Jarabo, I., Herrera, M., Hachero-Cruzado, I., Vargas-Chacoff, L., Mancera, J. M., and Arjona, F. J. (2015). Environmental salinity and osmoregulatory processes in cultured flatfish. Aqua. Res. 46, 10–29. doi: 10.1111/are.12424
Sardet, C., Pisam, M., and Maetz, J. (1979). The surface epithelium of teleostean fish gills. Cellular and junctional adaptations of the chloride cell in relation to salt adaptation. J. Cell Biol. 80, 96–117. doi: 10.1083/jcb.80.1.96
Sasai, S., Kaneko, T., Hasegawa, S., and Tsukamoto, K. (1998). Morphological alteration in two types of gill chloride cells in Japanese eel (Anguilla japonica) during catadromous migration. Can. J. Zool. 76, 1480–1487. doi: 10.1139/cjz-76-8-1480
Schreiber, A. M., and Specker, J. L. (1998). Metamorphosis in the summer flounder (Paralichthys dentatus): influence of stage-specific thyroidal status on larval development and growth. Gen. Comp. Endocrinol. 111, 156–166. doi: 10.1006/gcen.1998.7095
Schreiber, A. M. (2001). Metamorphosis and early larval development of the flatfishes (Pleuronectiformes): an osmoregulatory perspective. Comp. Physiol. B 129, 587–595. doi: 10.1016/s1096-4959(01)00346-3
Scott, G. R., Richards, J. G., Forbush, B., Isenring, P., and Schulte, P. M. (2004). Changes in gene expression in gills of the euryhaline killifish Fundulus heteroclitus after abrupt salinity transfer. Am. J. Physiol. Cell. Physiol. 287, C300–C309.
Segner, H., Storch, V., Reinecke, M., and Kloas, W. (1994). The development of functional digestive and metabolic organs in turbot (Scophthalmus maximus). Mar. Biol. 11, 471–486. doi: 10.1007/bf00347544
Shelbourne, J. E. (1957). Site of chloride regulation in marine fish larvae. Nature 180, 920–922. doi: 10.1038/180920a0
Shikano, T., and Fujio, Y. (1998a). Immunolocalisation of Na++/K++-ATPase and morphological changes in two types of chloride cells in the gill epithelium during seawater and freshwater adaptation in a euryhaline teleost, Poecilia reticulata. J. Exp. Zool. 281, 80–89. doi: 10.1002/(sici)1097-010x(19980601)281:2<80::aid-jez2>3.0.co;2-6
Shikano, T., and Fujio, Y. (1998b). Relationships of salinity tolerance to immunolocalisation of Na+/K+-ATPase in the gill epithelium during seawater and freshwater adaptation of the guppy, Poecilia reticulata. Zool. Sci. 15, 35–41. doi: 10.2108/zsj.15.35
Shiraishi, K., Kaneko, T., Hasegawa, S., and Hirano, T. (1997). Development of multicellular complexes of chloride cells in the yolk-sac membrane of Tilapia (Oreochromis mossambicus) embryos and larvae in seawater. Cell Tissue Res. 288, 583–590. doi: 10.1007/s004410050844
Silva, P., Solomon, R., Spokes, K., and Epstein, F. (1977). Ouabain inhibition of gill Na-K-ATPase: relationship to active chloride transport. J. Exp. Zool. 199, 419–426. doi: 10.1002/jez.1401990316
Singer, T. D., Clements, K. M., Semple, J. W., Schulte, P. M., Bystriansky, J. S., Finstad, B., et al. (2002). Seawater tolerance and gene expression in two strains of Atlantic salmon smolts. Can. J. Fish. Aquat. Sci. 59, 125–135. doi: 10.1139/f01-205
Sower, S. A., and Schreck, C. B. (1982). Steroid and thyroid hormones during sexual maturation of coho salmon (Oncorhynchus kisutch) in seawater or fresh water. Gen. Comp. Endo. 47, 42–53. doi: 10.1016/0016-6480(82)90082-x
Sucre, E., Charmantier-Daures, M., Grousset, E., and Cucchi-Mouillot, P. (2011). Embryonic ionocytes in the European sea bass (Dicentrarchus labrax): structure and functionality. Dev. Growth Differ. 53, 26–36. doi: 10.1111/j.1440-169x.2010.01219.x
Takei, Y., Hiroi, J., Takahaski, H., and Sakamoto, T. (2014). Diverse mechanisms for body fluid regulation in teleost fishes. Am. J. Physiol. Integr. Comp. Physiol. 307, R778–R792.
Tang, C. H., Hwang, L. Y., Shen, I. D., Chiu, Y. H., and Lee, T. H. (2011). Immunolocalization of chloride transporters to gill epithelia of euryhaline teleosts with opposite salinity-induced Na+/K+-ATPase responses. Fish Physiol. Biochem. 37, 709–724. doi: 10.1007/s10695-011-9471-6
Tipsmark, C. K., Madsen, S. S., Seidelin, M., Christensen, A. S., Cutler, C. P., and Cramb, G. (2002). Dynamics of Na+/K+/2Cl- cotransporter and Na+/K+-ATPase expression in the branchial epithelium of brown trout (Salmo trutta) and Atlantic salmon (Salmo salar). J. Exp. Zool. 293, 106–118. doi: 10.1002/jez.10118
Tytler, P., Bell, M. V., and Robinson, J. (1993). “The ontogeny of osmoregulation in marine fish: effects of changes in salinity and temperature,” in Physiological and Biochemical Aspects of Fish Development, eds B. T. Walther and H. J. Fyhn (Bergen: University of Bergen), 249–258.
Tytler, P., and Ireland, J. (1995). The influence of temperature and salinity on the structure and function of mitochondria in chloride cells in the skin of the larvae of the turbot (Scophthalmus maximus). J. Therm. Biol. 20, 1–14. doi: 10.1016/0306-4565(94)00021-a
Uchida, K., Kaneko, T., Miyazaki, H., Hasegawa, H., and Hirano, T. (2000). Excellent salinity tolerance of Mozambique tilapia (Oreochromis mossambicus): elevated chloride cell activity in the branchial and opercular epithelia of the fish adapted to concentrated seawater. Zool. Sci. 17, 149–160. doi: 10.2108/zsj.17.149
van der Heijden, A. J. H., Verbost, P. M., Eygensteyn, J., Li, J., Wendelaar Bonga, S. E., and Flik, G. (1997). Mitochondria-rich cells in gills of tilapia (Oreochromis mossambicus) adapted to fresh water or sea water: quantification by confocal scanning laser microscopy. J. Exp. Biol. 200, 55–64.
van der Heijden, A. J. H., van der Meij, J. C. A., Flik, G., and Bonga, S. E. W. (1999). Ultrastructure and distribution dynamics of chloride cells in tilapia larvae in fresh water and sea water. Cell Tiss. Res. 297, 119–130. doi: 10.1007/s004410051339
Varsamos, S., Connes, R., Diaz, J. P., Barnabé, G., and Charmantier, G. (2001). Ontogeny of osmoregulation in the European sea bass Dicentrarchus labrax L. Mar. Biol. 138, 909–915. doi: 10.1007/s002270000522
Varsamos, S., Diaz, J. P., Charmantier, G., Blasco, C., Connes, R., and Flik, G. (2002a). Location and morphology of chloride cells during the post-embryonic development of the European sea bass, Dicentrarchus labrax. Anat. Embryol. 205, 203–213. doi: 10.1007/s00429-002-0231-3
Varsamos, S., Diaz, J. P., Charmantier, G., Flik, G., Blasco, C., and Connes, R. (2002b). Branchial chloride cells in sea bass (Dicentrarchus labrax) adapted to freshwater, seawater and doubly-concentrated seawater. J. Exp. Zool. 293, 12–26. doi: 10.1002/jez.10099
Varsamos, S., Nebel, C., and Charmantier, G. (2005). Ontogeny of osmoregulation in postembryonic fish: a review. Comp. Biochem. Physiol. A. 141, 401–429. doi: 10.1016/j.cbpb.2005.01.013
Vega, G. C., and Wiens, J. J. (2012). Why are there so few fish in the sea? Proc. R. Soc. B Biol. Sci. 279, 2323–2329. doi: 10.1098/rspb.2012.0075
Wales, B., and Tytler, P. (1996). Changes in chloride cell distribution during early larval stages of Clupea harengus. J. Fish Biol. 49, 801–814. doi: 10.1111/j.1095-8649.1996.tb00080.x
Wales, B. (1997). Ultrastructural study of chloride cells in the trunk epithelium of larval herring, Clupea harengus. Tissue Cell 29, 439–447. doi: 10.1016/s0040-8166(97)80030-4
Wang, Y. F., Tseng, Y. C., Yan, J. J., Hiroi, J., and Hwang, P. P. (2009). Role of SLC12A10.2, a Na+-Cl- cotransporter-like protein, in a Cl uptake mechanism in zebrafish (Danio rerio). Am. J. Physiol. Regul. Integr. Comp. Physiol. 296, R1650–R1660.
Watanabe, S., Kaneko, T., and Watanabe, Y. (1999). Immunocytochemical detection of mitochondria-rich cells in the brood pouch epithelium of the pipefish, Syngnathus schlegeli: structural comparison with mitochondria-rich cells in the gills and larval epidermis. Cell Tissue Res. 295, 141–149. doi: 10.1007/s004410051220
Watrin, A., and Mayer-Gostan, N. (1996). Simultaneous recognition of ionocytes and mucous cells in the gill epithelium of turbot and in the rat stomach. J. Exp. Zool. 276, 95–101. doi: 10.1002/(sici)1097-010x(19961001)276:2<95::aid-jez2>3.0.co;2-8
Wilson, J. M., Laurent, P., and Tufts, B. L. (2000a). NaCl uptake by the branchial epithelium in freshwater teleost fish: an immunological approach to ion-transport protein localization. J. Exp. Biol. 203, 2279–2296.
Wilson, J. M., Randall, D. J., Donowitz, M., Vogl, A. W., and Ip, Y. K. (2000b). Immunolocalisation of ion-transport proteins to branchial epithelium mitochondria-rich cells in the mudskipper (Periophthalmodon schlosseri). J. Exp. Biol. 203, 2297–2310.
Wilson, J. M., and Laurent, P. (2002). Fish gill morphology: inside out. J. Exp. Zool. 293, 192–213. doi: 10.1002/jez.10124
Wong, C. K. C., and Chan, D. K. (1999). Isolation of viable cell types from the gill epithelium of Japanese eel Anguilla japonica. Am. J. Physiol. 276, 363–373.
Wood, C. M., and Marshall, W. S. (1994). Ion balance, acid–base regulation, and chloride cell function in the common killifish, Fundulus heteroclitus, a euryhaline estuarine teleost. Estuaries 17, 34–52.
Wood, P., and Laurent, P. (2003). Na+ versus Cl- transport in the intact killifish after rapid salinity transfer. Biochem. Biophys. Acta 1618, 106–119. doi: 10.1016/j.bbamem.2003.08.014
Wu, S. C., Horng, J. L., Hwang, P. P., Wen, Z. H., Lin, C. S., and Lin, L. Y. (2010). Ammonium-dependent sodium uptake in mitochondrion-rich cells of medaka (Oryzias latipes) larvae. Am. J. Physiol. Integr. Comp. Physiol. 298, C237–C250.
Yan, J. J., and Hwang, P. P. (2019). Novel discoveries in acid-base regulation and osmoregulation: a review of selected hormonal actions in Zebrafish and Medaka. Gen. Comp. Endo. 277, 20–29. doi: 10.1016/j.ygcen.2019.03.007
Keywords: osmoregulation, adaptability, larvae, embryos, early life stages, salinity, chloride cell, mitochondria rich cell
Citation: Fridman S (2020) Ontogeny of the Osmoregulatory Capacity of Teleosts and the Role of Ionocytes. Front. Mar. Sci. 7:709. doi: 10.3389/fmars.2020.00709
Received: 27 January 2020; Accepted: 04 August 2020;
Published: 21 August 2020.
Edited by:
Neill Andrew Herbert, The University of Auckland, New ZealandReviewed by:
Claudio Agnisola, University of Naples Federico II, ItalyZhigang Shen, Huazhong Agricultural University, China
Copyright © 2020 Fridman. This is an open-access article distributed under the terms of the Creative Commons Attribution License (CC BY). The use, distribution or reproduction in other forums is permitted, provided the original author(s) and the copyright owner(s) are credited and that the original publication in this journal is cited, in accordance with accepted academic practice. No use, distribution or reproduction is permitted which does not comply with these terms.
*Correspondence: Sophie Fridman, ZnJpZG1hbnNvcGhpZUBnbWFpbC5jb20=