- 1Biology Department, University of Massachusetts Dartmouth, Dartmouth, MA, United States
- 2Department of Environment and Natural Resources, Government of Bermuda, Crawl, Bermuda
- 3School of Marine Science and Technology, University of Massachusetts Dartmouth, New Bedford, MA, United States
Over the past three decades, lionfish (Pterois volitans and Pterois miles) from the Indo-Pacific have invaded the northwest Atlantic Ocean, Caribbean Sea, and the Gulf of Mexico. As generalist predators with a broad diet, they could pose a major threat to economically and ecologically important species and, therefore, the overall health of coral reef ecosystems and the communities that rely on them. In Bermuda, the lionfish invasion appears to be progressing at a slower rate than elsewhere in the invaded range, providing an opportunity to study their ecological impact at an early stage. This study used stable isotope analysis of lionfish, their major prey, and their competitors to investigate the feeding ecology of lionfish in Bermuda and provide a more complete evaluation of their potential impacts on community structure and trophic interactions. Results suggest that lionfish in Bermuda primarily derive resources from the plankton-based food web, with only a small contribution from food chains supported by macroalgae. Further, it appears that lionfish resource use overlaps substantially with other similarly sized sympatric mesopredators, in particular the coney grouper (Cephalopholis fulva), a species commonly targeted by local commercial fisheries. This visualization of Bermuda’s demersal ecosystem in two-dimensional isotope space, the first of its kind, will help track the ecological impact of lionfish over time, predict potential changes in community structure, and better inform developing control strategies for this invasive species.
Introduction
Since lionfish (Pterois volitans and Pterois miles) were first documented in the western Atlantic Ocean in 1985 (Whitfield et al., 2002), growing evidence suggests that they could have a substantial ecological impact across their invaded range (Albins and Hixon, 2008; Lesser and Slattery, 2011; Green et al., 2012). The generalist and opportunistic feeding habits of lionfish are well-recognized (Peake et al., 2018), and their broad diet, comprising a wide variety of teleost and crustacean species, could lead to ecosystem impacts via predation. The ecological impacts that lionfish may cause through resource competition have been explored to a lesser degree, as have other ecological characteristics such as trophic position and niche (but see Muñoz et al., 2011; Layman and Allgeier, 2012; Albins and Hixon, 2013; O’Farrell et al., 2014).
During the past several decades, ecologists have been using stable isotope analysis (SIA) to gain valuable insights into the diet and trophic relationships of populations, communities, and even entire ecosystems (Fry, 2006; Boecklen et al., 2011). Stable isotope ratios (e.g., 13C/12C and 15N/14N) in predators directly reflect those of their prey, often in a predictable manner (Peterson and Fry, 1987; Hobson and Clark, 1992; Hobson, 1999), so these isotope ratios can be used to better understand trophodynamics and predator–prey interactions across diverse systems. The relative ratio of 15N to 14N (δ15N) typically increases in a stepwise fashion with each trophic level (DeNiro and Epstein, 1981; Minagawa and Wada, 1984) and has thus been used to estimate the relative trophic position of a species within a community or ecosystem (DeNiro and Epstein, 1981; Peterson and Fry, 1987). In contrast, the relative ratio of 13C to 12C (δ13C), which can vary substantially among primary producers (Layman et al., 2007), has been used to reliably determine the source of primary production at the foundation of an animal’s specific trophic web (DeNiro and Epstein, 1978; Peterson and Fry, 1987).
More recently, SIA has proven to be an effective complement to stomach content analysis (SCA) in studies that aim to determine complex trophic relationships (Boecklen et al., 2011). Because an animal’s isotopic composition reflects its diet over a long period of time, SIA provides a more integrated view of an animal’s trophic ecology when compared to the simple “snapshot” of its feeding history that SCA provides (Hesslein et al., 1993). In addition, SIA will also more accurately reflect the contribution of rapidly digesting or otherwise unidentifiable diet items that might go unrecorded in SCA (Muñoz et al., 2011).
Recognizing that the isotopic ratios found in tissues represent the long-term accumulated feeding behavior of an animal (Hobson and Clark, 1992; Hesslein et al., 1993), SIA has been used to investigate a species’ trophic niche (Bearhop et al., 2004; Newsome et al., 2007), which represents the overall trophic role of a species through the accumulation of its trophic interactions (Elton, 1927; Leibold, 1995; Layman et al., 2007). The trophic niche space of a species, reflecting both (δ15N) and (δ13C) in two-dimensional isotopic space (i.e., δ-space; Newsome et al., 2007), has been used to describe community-wide trophic relationships by comparing isotopic niche space and position relative to other species in a given food web (Layman et al., 2007; Turner et al., 2010). Taken together, the isotopic niche overlap among species provides a novel approach to explore dietary similarity among consumers (Jackson et al., 2012), which can, to some degree, be used to indicate competition.
Despite being the first location outside of United States waters where non-indigenous lionfish were reported, overall lionfish densities in Bermuda are typically lower than those in other locations within the invaded range (Eddy, 2016), suggesting that the invasion is progressing more slowly there than elsewhere. This provides an important opportunity to investigate the ecological characteristics of lionfish (e.g., trophic position and niche) at an early stage of the invasion, before a population “explosion” occurs and potential impacts on community structure arise. As reported throughout the western Atlantic (Peake et al., 2018), the diet of lionfish in Bermuda is known to be diverse (Eddy et al., 2016), which may ultimately have an impact on Bermuda’s reef ecosystem. Recognizing that SIA provides an opportunity to evaluate the degree to which their diverse prey contributes to the isotopic values of lionfish, this study measured the δ15N and δ13C values for lionfish, a suite of other consumers (including potential lionfish competitors and known prey species), and primary producers, to determine the trophic structure of the invasive lionfish population and the community of coral reef fishes along Bermuda’s coastline as a means to illustrate overall community structure and explore potential impacts.
Materials and Methods
Sample Collection
Samples of lionfish, lionfish prey, and potential lionfish competitors were collected from multiple locations on the Bermuda platform from 2012 through 2015 by fishermen, snorkelers, SCUBA divers, researchers, and commercial fishermen using pole spears, collecting nets, experimental lionfish traps, and as bycatch in commercial lobster traps (Figure 1). Each specimen was measured for total length (TL), weighed, then either placed on ice to be dissected the same day or frozen whole (−10°C) for up to 4 weeks before being thawed and dissected. To prepare samples for SIA, myotomal muscle samples (∼2 g, taken from the area immediately posterior to the operculum) from lionfish and potential lionfish competitors were collected, placed in 2-ml centrifuge tubes, and frozen (−10°C). Samples of teleost prey species were prepared similarly where possible, while smaller fish and non-teleost prey species were placed in 2-ml centrifuge tubes and frozen whole (−10°C). Minimally digested prey items (i.e., easily and rapidly identifiable) found in the stomach contents of lionfish were also collected, placed in 2-ml centrifuge tubes, and immediately frozen (−10°C). Prey items were identified in a concurrent SCA (Eddy et al., 2016). Potential lionfish competitors were considered to be any species whose size, habitat use, and feeding preferences (as indicated by previous studies) are similar to those of lionfish in Bermuda (Eddy, 2016; Eddy et al., 2016). While searching for lionfish, SCUBA divers collected samples (∼2 g) of two common macroalgal genera (Dictyota spp. and Laurencia spp.), which were rinsed with fresh water, placed into 50-ml tubes, and frozen (−10°C) prior to processing and analysis. Stable isotope ratios for seagrass and other macroalgae in Bermuda were taken from Fourqurean et al. (2015) and Burgett et al. (2018). Stable isotope ratios for plankton1, dissolved nutrients, and particulate matter were taken from Altabet (1989), Gruber et al. (2002), Knapp et al. (2005), Landrum et al. (2011), and McMahon et al. (2013).
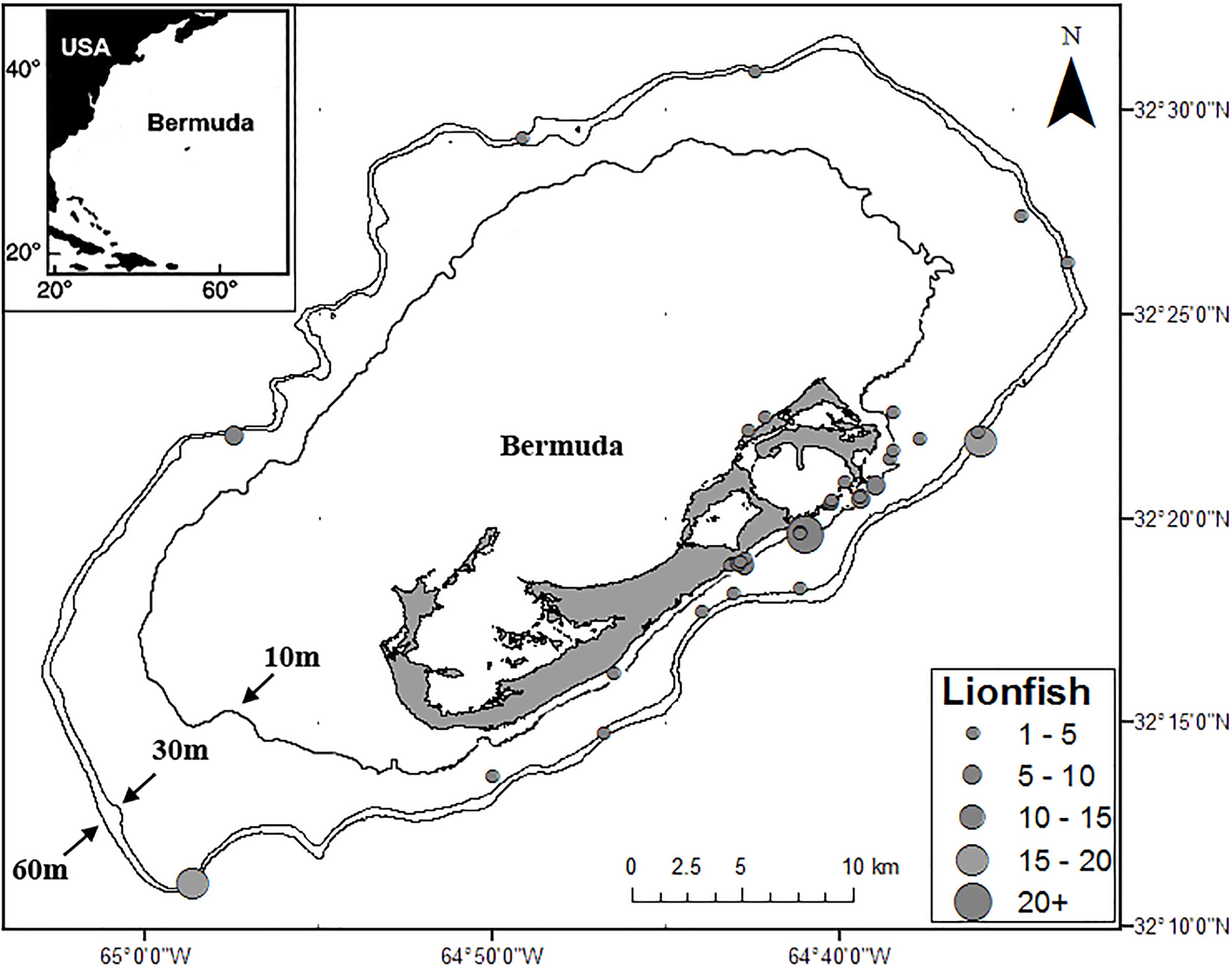
Figure 1. Locations and relative numbers of lionfish captured for this study. Depth contour lines at 10, 30, and 60 m are also shown.
Sample Analysis
Myotomal muscle samples for lionfish, potential competitors, and prey were dehydrated at 60°F for at least 48 h (Muñoz et al., 2011; Layman and Allgeier, 2012). Small prey specimens, collected either from stomach contents or using collecting nets, were dehydrated whole at 60°F for at least 48 h after their heads and viscera were removed. Dehydrated muscle samples and whole animals were homogenized using a mortar and pestle, and ∼0.85 mg of each was sealed separately in tin capsules. Dried macroalgae were cut into small pieces (∼1 mm3) using scissors, and ∼0.85 mg of each was also sealed separately in tin capsules.
Samples were processed by continuous-flow isotope-ratio mass spectrometry (CF-IRMS) using a GV IsoPrime to determine 15N/14N and 13C/12C ratios. These ratios (R) were transformed to δ15N and δ13C values by:
where X is the heavy isotope of nitrogen (δ15N) or carbon (δ13C), Rsample is the isotopic ratio of the sample, and Rstandard is the ratio of an accepted reference material [i.e., Pee Dee Belemnite (PDB) for δ13C and atmospheric N2 gas for δ15N]. The mass spectrometer was interfaced to an elemental analyzer for automated online combustion and purification of sample nitrogen and carbon. Every 10th sample was repeated, and a standard sample of dehydrated and homogenized mahi-mahi (Coryphaena hippurus) myotomal muscle (∼0.85 g) was analyzed every 12th sample as a quality control measure and to monitor and compensate for potential analyzer drift. The estimated analytical precision for the isotopic measurements was better than ±0.1%. The minimal lipid content (C:N < 3.5) of all samples precluded any potential bias due to a high lipid content, thus lipids were not extracted and the data were not normalized (Post et al., 2007).
The ratio of stable carbon isotopes (δ13C) differs between pelagic and benthic systems and was used to indicate the base of the lionfish food web (France, 1995; Post, 2002). Following Post (2002), relative trophic position (TP) was estimated using δ15N values by:
where λ is the TP of the organism used to estimate δ15Nbase, Δn is the lionfish-specific enrichment in 15N per trophic level [i.e., trophic discrimination factor (TDF)] calculated experimentally (Eddy, 2019), δ15Nconsumer is the direct measurement of δ15N for the target species (i.e., lionfish), and δ15Nbase was obtained from juvenile Atlantic creolefish (Paranthias furcifer), a sympatric zooplanktivore (i.e., primary consumer) with an assumed TP of 2.25 (Jacobsen and Bennett, 2013) and an important diet item of lionfish in Bermuda (Eddy et al., 2016).
Back-Calculation of Resource Pools
Experimentally derived TDFs (Eddy, 2019), annotated as Δ15N and Δ13C, were used to back-calculate the estimated position in δ-space where lionfish may derive their resources (i.e., the base of the trophic web). These estimated resource pools were compared to those of primary and secondary consumers, but lacking species-specific TDFs, and the back-calculation for consumers was done using global averages of Δ15N and Δ13C (Post, 2002: ΔN = 3.4‰, ΔC = 0.4‰; Caut et al., 2009: ΔN = 2.8‰, ΔC = 0.8) and to mean values calculated specifically from myotomal muscle samples of marine teleosts (ΔN = 3.2‰, ΔC = 2.2‰; data provided in Supplementary Table S1 of Caut et al., 2009).
Data Analysis
Differences in δ15N and δ13C values between lionfish and competitors, as well as among competitors, were tested using non-parametric tests (i.e., Kruskal–Wallace H-test and Dunn’s t-test). Isotopic space (i.e., δ-space) for the lionfish food web was visualized by plotting the mean isotope values of each species’ δ15N (y-axis) and δ13C (x-axis) (Genner et al., 1999; Newsome et al., 2007). Variations in diet as a function of habitat (i.e., depth) and total length (i.e., ontogenetic change) were analyzed using linear regressions, ANOVAs, and/or t-tests. The size and position of isotopic niche space among lionfish, potential competitors, prey species, and primary producers were compared by generating standard ellipses (i.e., two-dimensional equivalents of isotope value standard deviations) using SIBER in the statistical package R (Jackson et al., 2011). These ellipses (SEAc = Standard Ellipse Area, corrected for sample size) were then plotted in δ-space, as representations of isotopic niche, to investigate niche overlap and the potential for competition between lionfish and other mesopredators (i.e., small- to medium-sized predators) occupying similar habitats. Several metrics of community trophic structure (e.g., niche position and niche width) were determined following calculations outlined in Layman et al. (2007) and statistically analyzed following Turner et al. (2010) using the statistical software R.
Results
Lionfish Isotope Ecology
The δ15N values for lionfish ranged from 8.4 to 11.1‰ (mean ± SE: 9.7 ± 0.04, n = 219), and the δ13C values ranged from -18.2 to -14.3‰ (mean ± SE: -17.0 ± 0.04, n = 219; Figure 2). The highest δ13C values (i.e., >-15‰), which fell outside of the standard ellipse area, were found in lionfish captured in shallow, inshore areas. The isotopic niche width for lionfish was 1.62 (Figure 2).
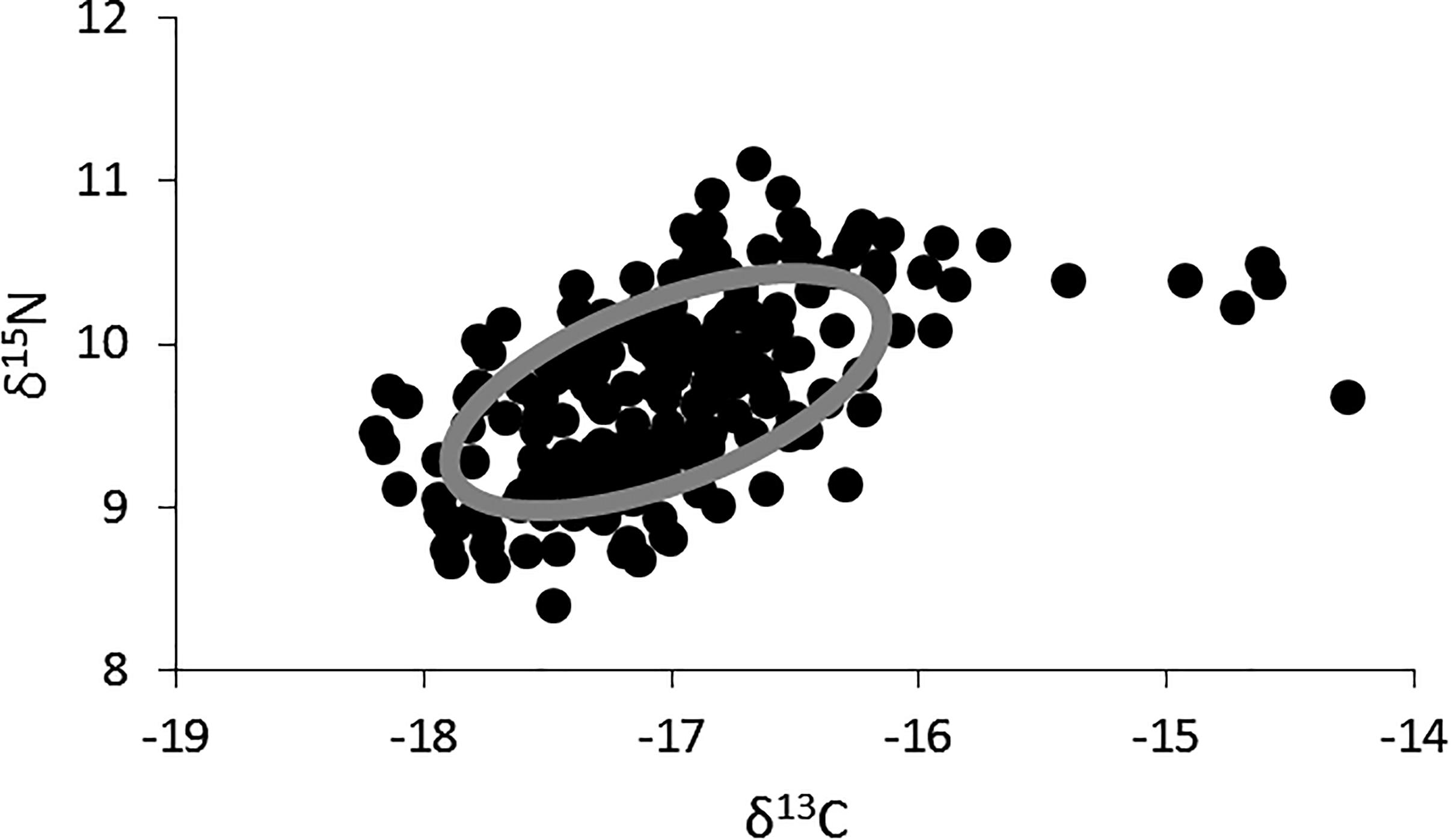
Figure 2. Two-dimensional plot of δ15N and δ13C values for all individual lionfish (black dots, n = 218) and the overall isotopic niche for lionfish in Bermuda (gray ellipse; SEAc, Standard Ellipse Area, corrected for sample size).
The relative position of lionfish in δ-space varied by the depth at which they were captured (Figure 3). The δ15N values of lionfish captured at 60 m were significantly different from the δ15N values of lionfish captured at all other depths (Student’s t-test, p < 0.001). The δ13C values of lionfish captured at 60 m were significantly different from those captured at 10 and 20 m (Student’s t-test, p < 0.001), and the δ13C values of lionfish captured at 45 m were significantly different from those captured at 10 m (Student’s t-test, p < 0.001). Overall, the isotopic position of lionfish captured at 60 m was significantly different from those of lionfish captured at all other depths (i.e., 45, 30, 20, and 10 m; Hotelling’s T2-test; p < 0.05) and the isotopic position of lionfish captured at 45 m was significantly different from that of lionfish at 10 m (Hotelling’s T2-test; p < 0.05). Although there was overlap in the isotopic niches of lionfish at different depths, the positions of isotopic niches moved toward lower δ15N and δ13C values with depth (Figure 3). However, there was no significant linear relationship between depth and either stable isotope [Figure 4; r2 = 0.39 and 0.31, respectively, ANOVA: F(1,215) = 3.88, p > 0.05].
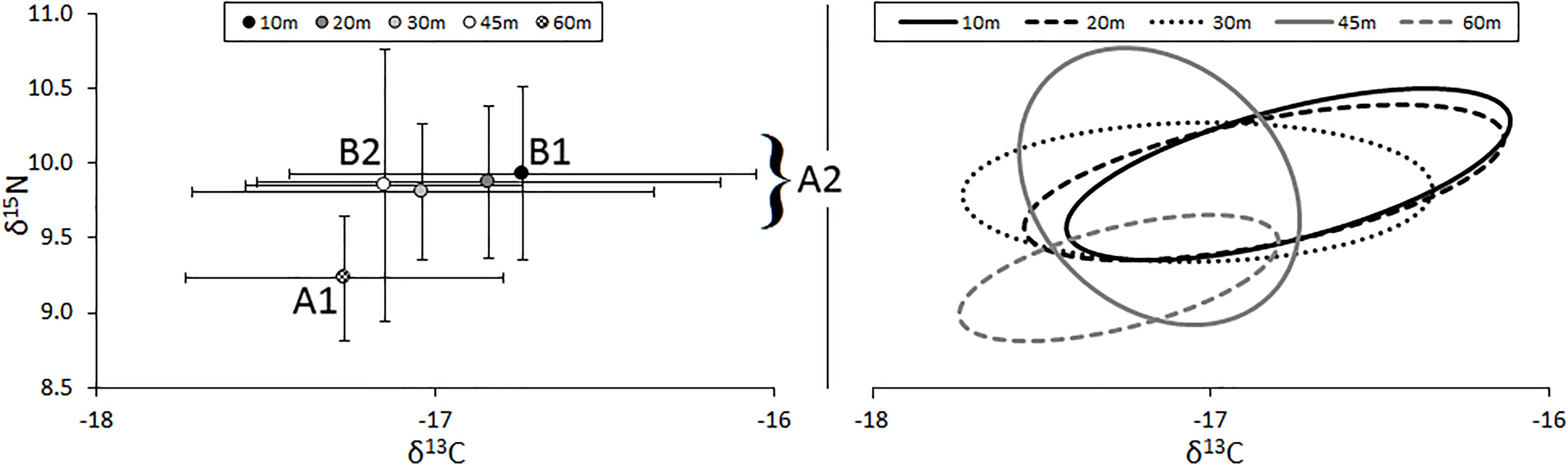
Figure 3. (Left) Mean (±SE) isotope values and (right) isotopic niches (SEAc) for lionfish captured at different depths. Sample sizes for 10-, 20-, 30-, 45-, and 60-m groups are 43, 18, 36, 30, and 55, respectively. Niche position was significantly different between lionfish captured at 60 m (A1) and all other depths (A2; Hotelling’s T2 test; p < 0.05) and between those captured at 10 m (B1) and 45 m (B2; Hotelling’s T2 test; p < 0.05).
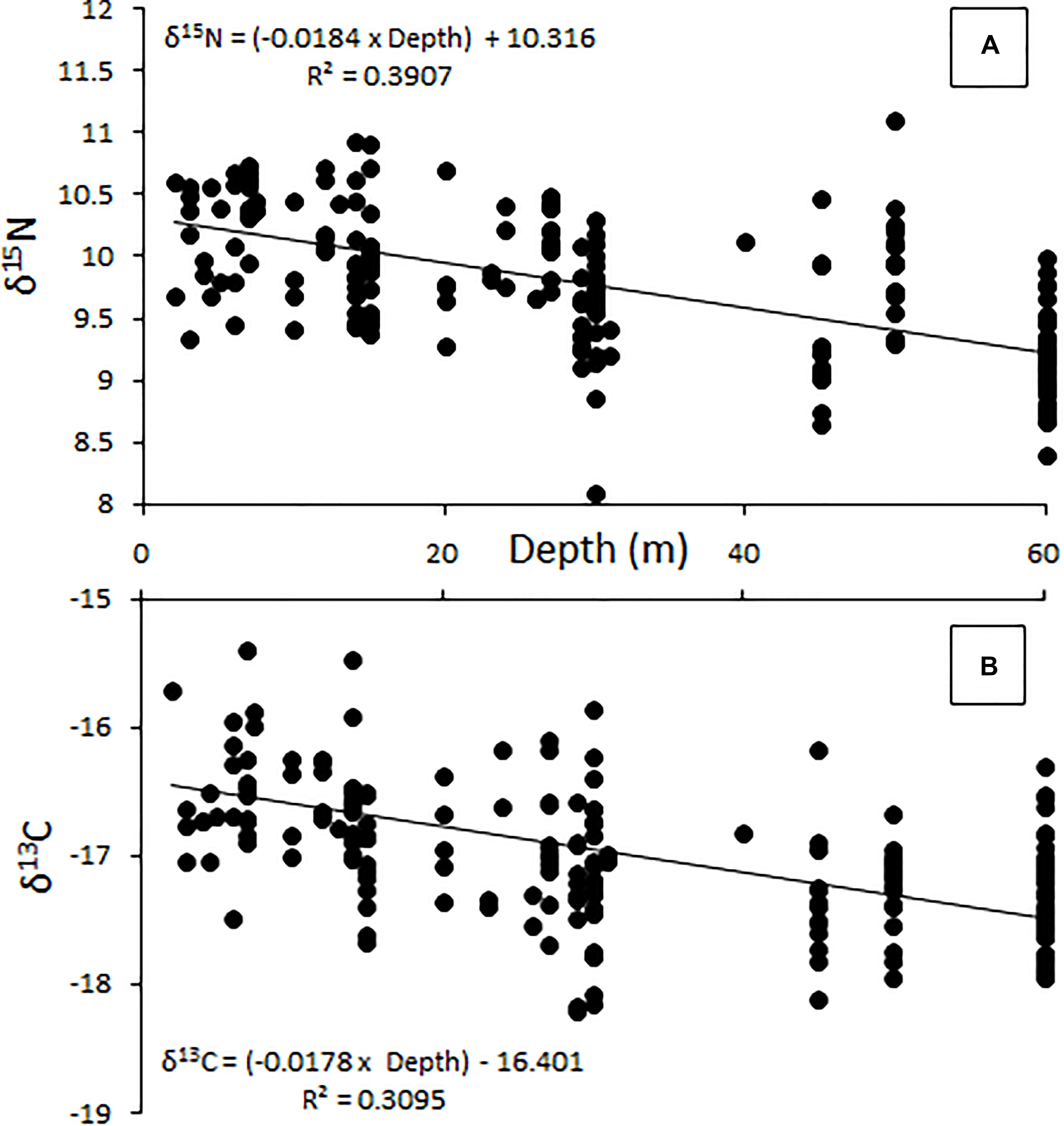
Figure 4. Change in δ15N (A) and δ13C (B) with increasing depth (m), showing a general decrease in both measures as depth increases. This relationship is not significant [ANOVA, F(1,215) = 3.88, p > 0.05].
The relative position of lionfish in δ-space was also affected by size, with larger size classes of lionfish generally having higher δ15N and δ13C values (Figure 5). However, the only significant difference in niche position was found between lionfish of 250–300 mm TL and those in the largest size classes (350–400 mm TL and >400 mm TL; Hotelling’s T2-test; p < 0.05); other comparisons using the Hotelling’s T2-test returned non-significant values. Further, there was no statistically significant relationship between either δ15N or δ13C and lionfish size overall (Figure 6). Some variation in niche width can be observed (Figure 5), with the smaller size classes exhibiting narrower niches.
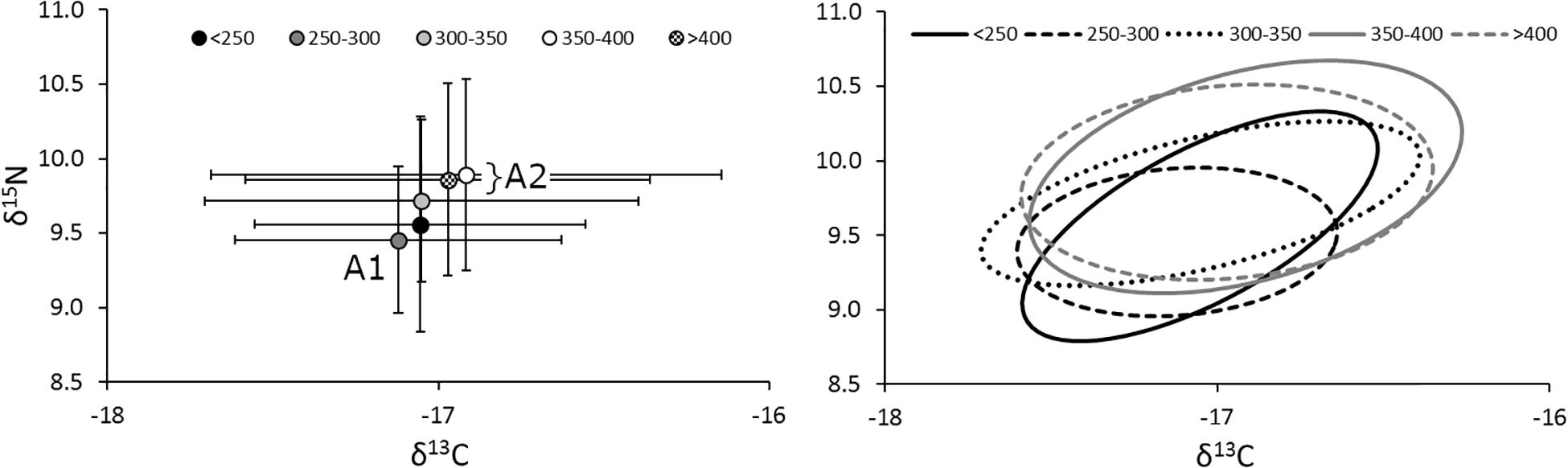
Figure 5. (Left) Mean (±SE) isotope values and (right) isotopic niches (SEAc) for lionfish in different size classes. Sample sizes for < 250-, 250– 300-, 300– 350-, 350– 400-, and >400-mm size classes are 9, 57, 59, 41, and 36, respectively. The niche position of 250–300-mm lionfish (A1) was significantly different from the two largest size classes (A2; Hotelling’s T2 test; p < 0.05), marginally different from the middle size class (300–350 mm; Hotelling’s T2 test; p = 0.06), but not significantly different from the smallest (<250 mm; Hotelling’s T2 test; p = 0.82).
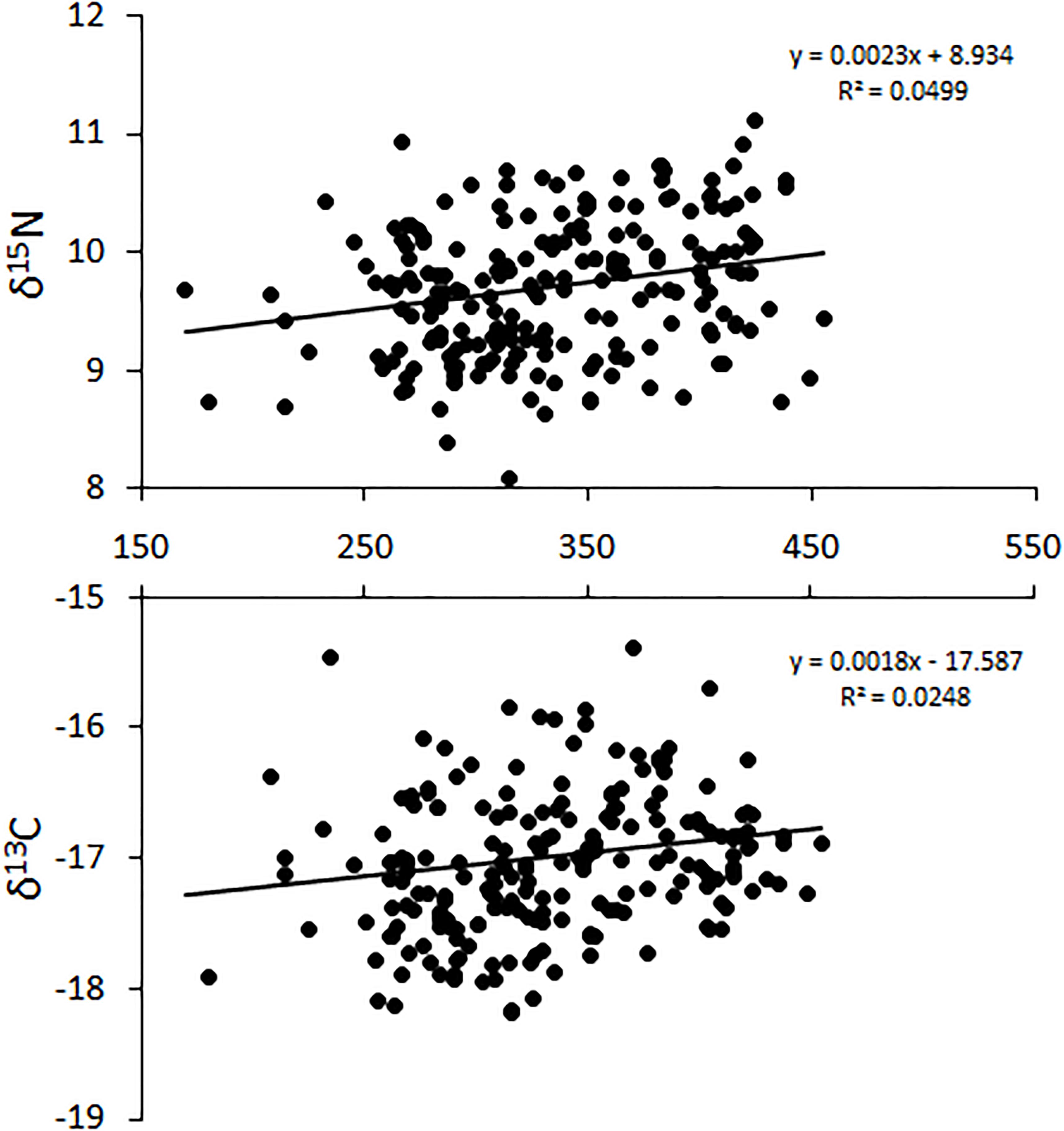
Figure 6. Change in δ15N and δ13C with increasing total length (TL) for all lionfish captured in Bermuda. Horizontal axis = total length (TL: mm). This relationship is not significant [ANOVA, F(1,126) = 3.91, p > 0.05].
Trophic Position
The trophic position of lionfish [using the Atlantic creolefish (P. furcifer) to represent the δ15Nbase, see above] was 3.4 when applying the lionfish-specific TDF (Eddy, 2019) and 3.2 when applying a global mean 15N TDF (Post, 2002). The experimentally derived TDF was also used to back-calculate the position of resource pools (i.e., the base of the trophic web), suggesting that resources were drawn primarily from a plankton-based food web with a small contribution from macroalgae (Figure 7; δ15N: ∼3.5 to 4.0‰; δ13C: ∼-21.1 to -19.5‰), a similar finding to that reached by applying other TDFs for muscle samples of marine teleosts (i.e., Caut et al., 2009). By contrast, the use of global mean TDFs from Post (2002) would indicate that resources were derived nearly equally from plankton and macroalgae (δ15N: ∼2.6 to 3.0‰; δ13C: ∼-19.1 to -17.5‰).
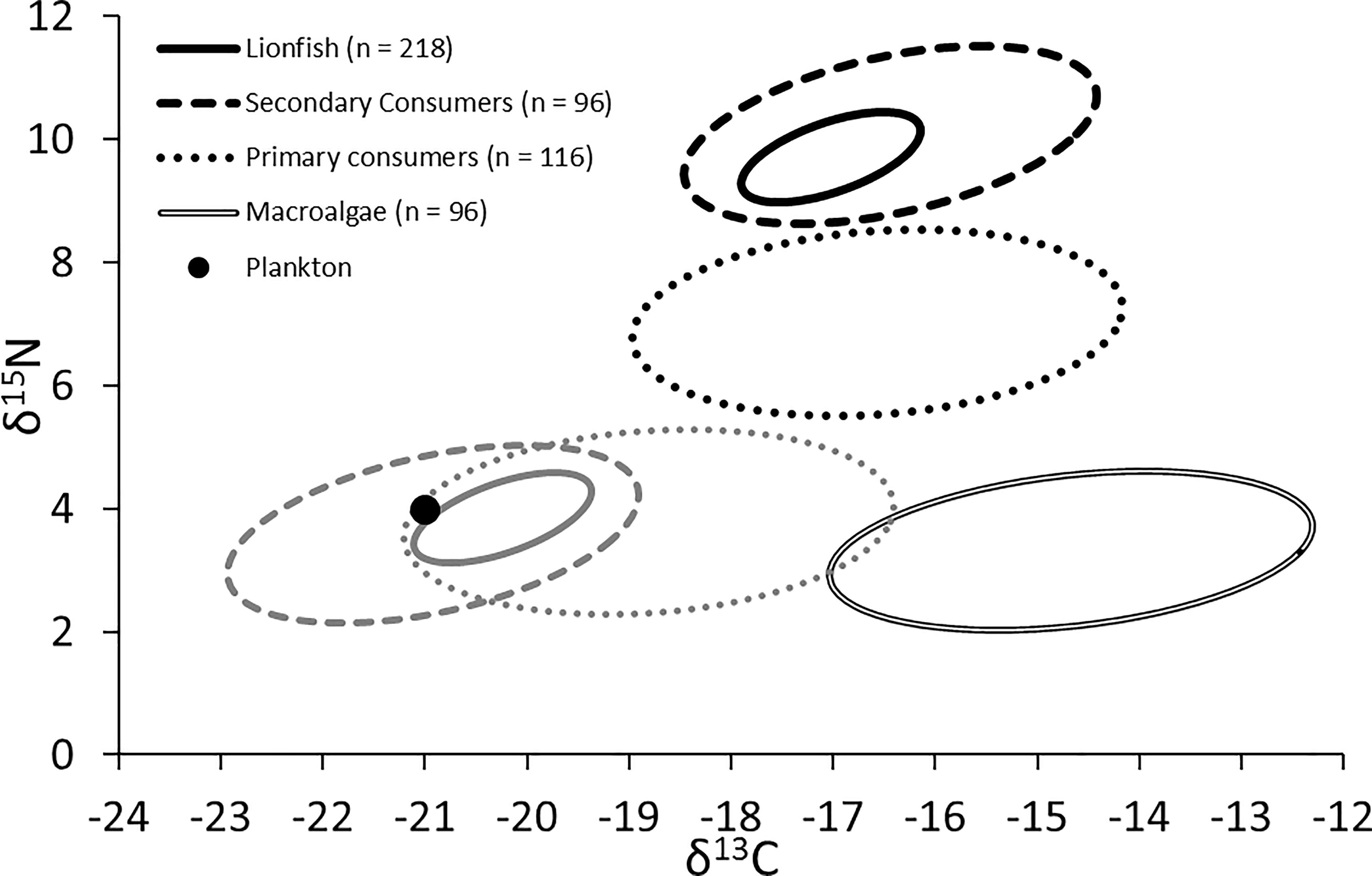
Figure 7. Black shapes: isotopic niche of lionfish, secondary consumers (competitors), primary consumers (prey), and primary producers (both plankton and macroalgae). Gray shapes: predicted resource pools of lionfish, secondary consumers, and primary consumers.
Community Structure
The isotopic position of secondary consumers, which may compete with lionfish for food, varied in δ-space (Figure 8A, with details in Supplementary Table S1), with mean δ15N values ranging from 8.1‰ for hogfish, Lachnolaimus maximus, to 10.7‰ for porgy, Calamus calamus, and mean δ13C values ranging from -18.6‰ for red hind, Epinephelus guttatus, to -13.2‰ for Lane snapper, Lutjanus synagris. Within a species, the largest variations of δ15N (± 2.2‰) and δ13C (± 2.2‰) were observed among juvenile Galapagos sharks, Carcharhinus galapagensis, and gray snapper, Lutjanus griseus, respectively, and the smallest variations in δ15N (±0.5‰) and δ13C (±0.6‰) were observed among red hinds and Lane snapper, respectively.
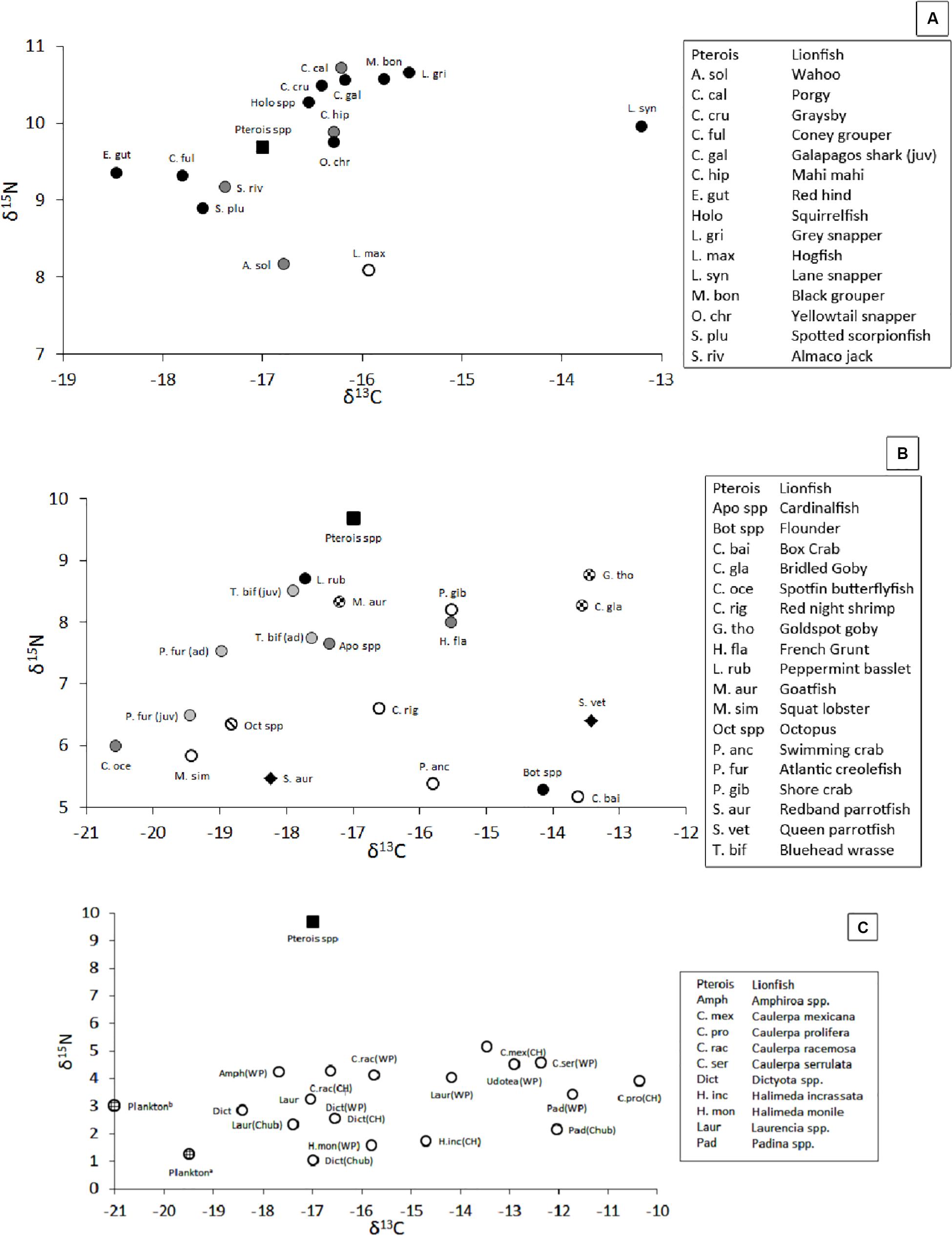
Figure 8. (A) Position of mesopredators in δ-space (i.e., potential competitors, pelagic piscivores, and demersal invertivores). Black square = lionfish. Black circle = demersal piscivores. Gray circle = pelagic piscivores. White circle = demersal invertivore. (B) Position of prey species, most of which are primary consumers, in δ-space. Black square = lionfish. Black circle = invertivore/piscivore. Dark gray circle = invertivore. Light gray circle = zooplanktivore. Checkered circle = detritivore/invertivore. Circle with diagonal line = Octopus. White circle = crustacean. Black diamond = herbivore. (C) Position of primary producers in δ-space (Fourqurean et al., 2015; Burgett et al., 2018). Black square = lionfish. White circle = macroalgae. Lined circle = plankton. WP = Walsingham Pond. CH = Castle Harbor. Chub = Chub Head. δ-values not shown: δ13C for dissolved inorganic carbon ranged from 1 to 1.6‰, δ15N for suspended particles and zooplankton were 2.0 and 3.8‰, respectively, and δ15N for total dissolved inorganic and organic nitrogen was ∼3.9‰. Sample sizes and standard errors for panels (A,B) are provided in Supplementary Table S1. Sample sizes and standard errors are not provided for panel (C) because the values were collected from the literature.
The position of primary consumers, many of which are lionfish prey (Eddy et al., 2016), also varied in δ-space (Figure 8B, with details in Supplementary Table S1), with δ15N values ranging from 5.2‰ for the box crab, Cryptosoma bairdii, to 8.7‰ for goldspot goby, Gnatholepis thompsoni, and mean δ13C values ranging from -13.5‰ for goldspot goby to -20.6‰ for spotfin butterflyfish, Chaetodon ocellatus. In contrast, mean (±SE) δ-values among all crustacean prey, invertivorous prey, and zooplanktivorous prey varied more narrowly, with δ15N values of 6.2‰ (±0.2‰, n = 25), 7.0‰ (±0.3‰, n = 20), and 6.9‰ (±0.2, n = 17) and mean δ13C values of -15.8‰ (±0.5‰, n = 25), -16.8‰ (±0.6‰, n = 20), and -18.9‰ (±0.2‰, n = 17), respectively.
The niche positions in δ-space for primary producers (Figure 8C), primary consumers, and secondary consumers were significantly different (Hotelling’s T2 test; p = 0.001), while the niche position of lionfish was contained with the overall niche for secondary consumers (Figure 7). The size of the niche (i.e., width) for primary producers was not significantly different from that for primary consumers (Hotelling’s T2 test, p = 0.529). The niche positions of most potentially competing secondary consumers in δ-space differed significantly from that of lionfish (Hotelling’s T2 test; p < 0.05; Table 1 and Figure 9). The size of isotopic niches among these potential competitors varied, and approximately half of them were significantly different in size from others (Table 2). Yellowtail snapper (Ocyurus chrysurus) had the widest isotopic niche (5.34) and Lane snapper had the narrowest (0.52). Among known prey species, the detritivorous red night shrimp (Cinetorhynchus rigens; Manning, 1961) had the widest niche (6.85) and the zoooplanktivorous juvenile Atlantic creolefish had the narrowest niche (0.16; Figure 9). The niche of macroalgae was very broad (9.17).
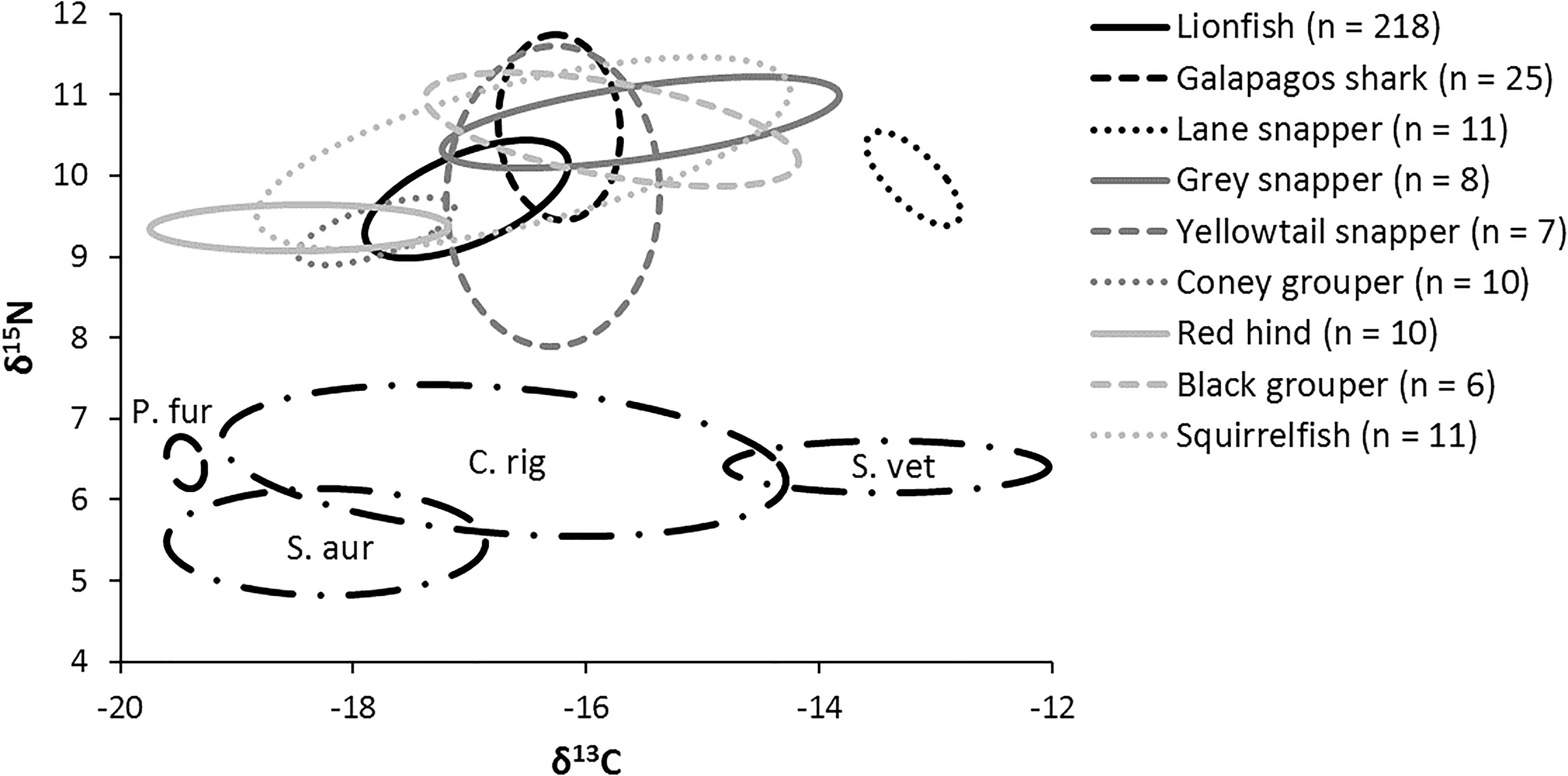
Figure 9. Isotopic niche of individual mesopredators and four primary consumers (black dashed lines). P. fur, Atlantic creolefish (n = 12); C. rig, Red night shrimp (n = 12); S. vet, Queen parrotfish (n = 10); S. aur, Redband parrotfish (n = 11).
Resource Competition
Mean δ15N values differed significantly between lionfish and likely competitors (Figure 8A, Supplementary Table S1; Kruskal–Wallis H-test: p < 0.001), and the pairwise comparisons between lionfish (9.7 ± 0.04‰) and most potential competitors (i.e., juvenile Galapagos shark: 10.6 ± 0.2‰; gray snapper: 10.7 ± 0.2‰; coney grouper: 9.3 ± 0.1‰; red hind: 9.4 ± 0.1‰; black grouper: 10.6 ± 0.3‰), as well as between several of these competitors, were significantly different (Table 3; Dunn’s t-test: p < 0.05).
Mean δ13C values also differed significantly between lionfish and potentially competing species (Figure 8A, Supplementary Table S1; Kruskal–Wallis H-test: p < 0.001), and the pairwise comparisons between lionfish (−17.0 ± 0.04‰), juvenile Galapagos shark, and all members of the grouper snapper complex examined were significantly different (Table 4; Dunn’s t-test: p < 0.05). The pairwise comparisons between several of these competitors were also significantly different (Table 4).
The overlap of isotopic niches of secondary consumers, a potential indicator of resource competition, varied considerably among mesopredators that occupy similar habitats as lionfish and presumably compete for both space and resources (Figure 10 and Table 5). The greatest overlap occurred between lionfish and coney grouper, where 54% of the coney grouper niche is included in that of lionfish, while there is no overlap between lionfish and Lane snapper (Figure 10 and Table 5). The niche of squirrelfishes overlapped 92% of the lionfish niche, but lionfish overlapped that of squirrelfishes by only 23% (Figure 10 and Table 5).
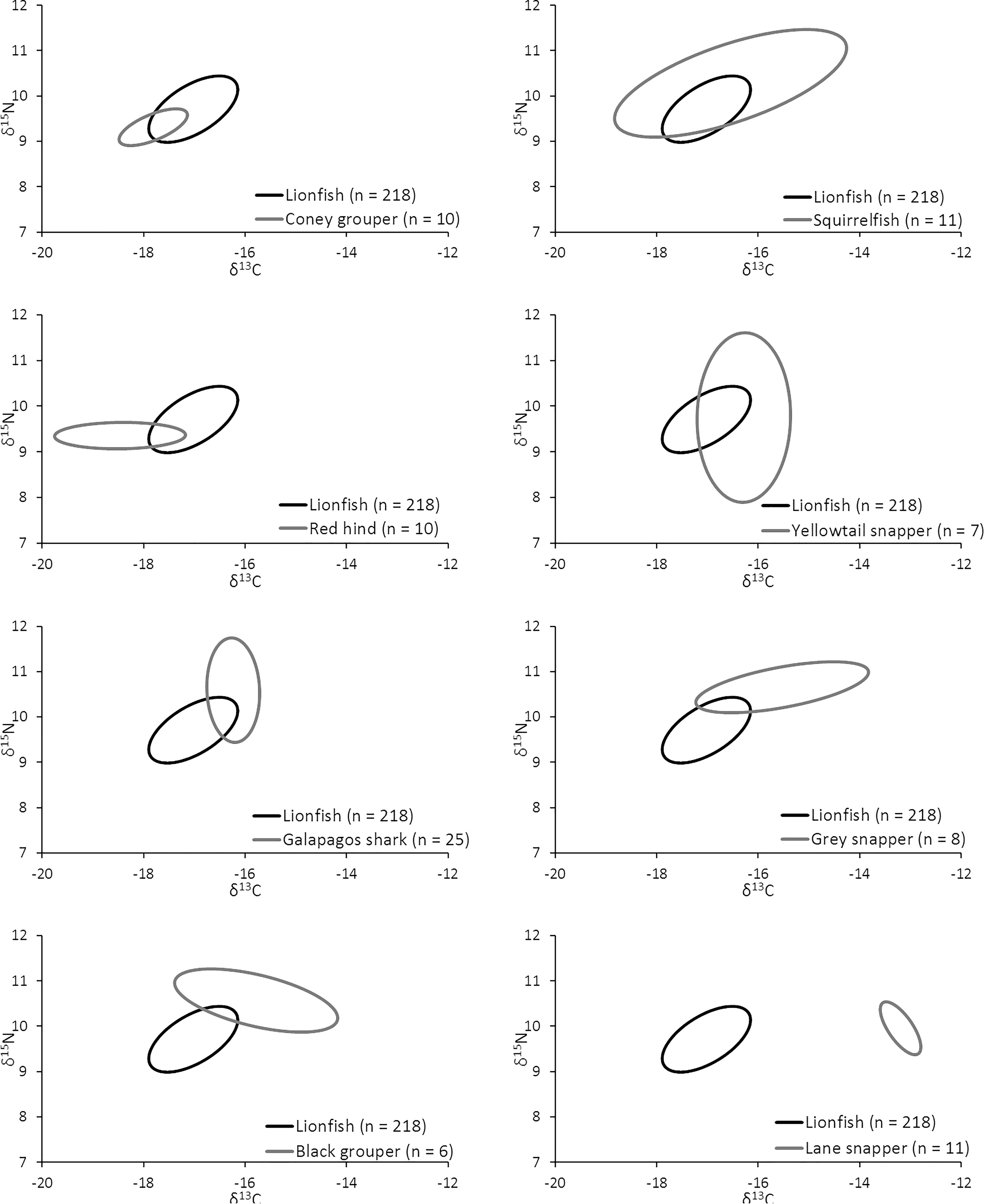
Figure 10. Overlap in isotopic niche potentially indicating a degree of resource overlap (i.e., competition) among eight important predators.
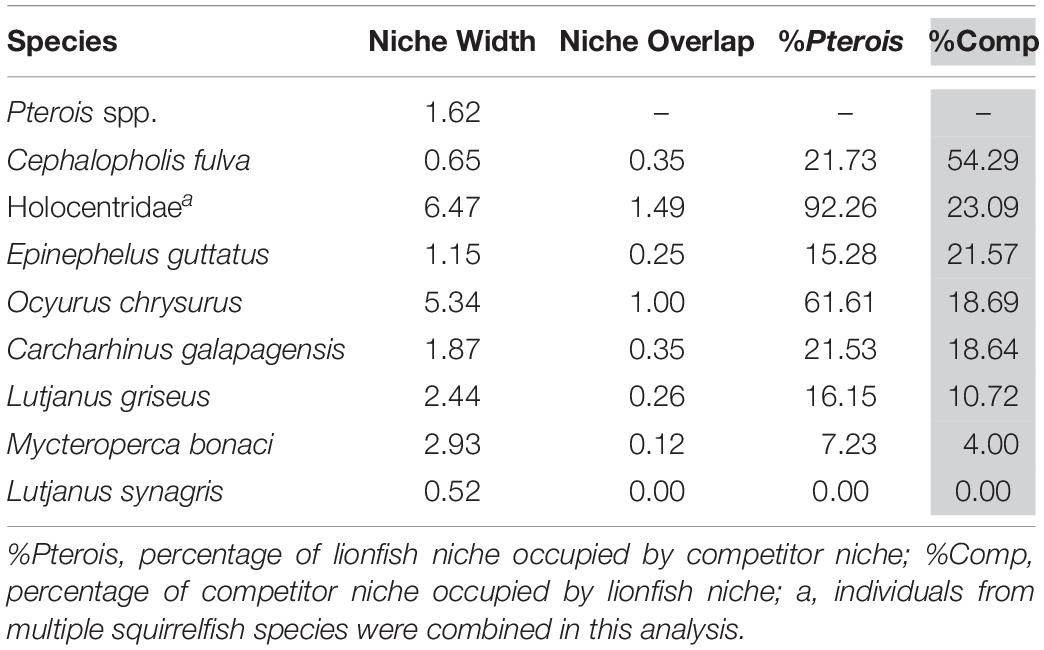
Table 5. Isotopic niche width of eight potential competitors and the overlap in niche with lionfish.
Discussion
By applying isotope measures of community structure (Layman et al., 2007; Turner et al., 2010), we offer the first glimpse of Bermuda’s marine ecosystem in two-dimensional isotopic space (i.e., δ-space). This approach allows the investigation of isotopic niche position and size and the opportunity to explore dietary resource use and competition between the invasive lionfish and native species.
Among demersal piscivores of similar size, lionfish are a top predator in Bermuda and occupy a trophic position of 3.4. The wide δ-space occupied by lionfish highlights a broad isotopic niche characteristic of a generalist predator feeding from a varied resource base, in agreement with stomach contents analyses (Peake et al., 2018) and similar work using SIA (Muñoz et al., 2011; Layman and Allgeier, 2012; O’Farrell et al., 2014). Stomach contents analyses also typically show a subtle dietary shift through ontogeny (Morris and Akins, 2009; Eddy et al., 2016; Peake et al., 2018). While our new findings do not show a significant change in either δ15N or δ13C with increased body length, the niche position of the second smallest size class was significantly different from all larger size classes, and a significant difference in the niche position of the smallest size class (<250 mm; n = 9) might have been detected if a larger sample size were available. Further, given that lionfish, at least at the population level, are generalist predators feeding opportunistically upon dozens of species (Eddy et al., 2016; Peake et al., 2018), their diet is unlikely to change in any distinct way with additional growth after maturity.
The isotopic composition of Bermuda’s lionfish varied more noticeably with depth. Lionfish from mesophotic reefs at 60 m had lower δ15N and δ13C than those from shallow reefs (10–20 m), with fish from 45 m showing overlapping characteristics. These differences between depth zones reflect changes in overall reef community structure across a range of taxa (Goodbody-Gringley et al., 2019; Stefanoudis et al., 2019a, b) and thus in the prey items available to an opportunistic generalist predator such as the lionfish. For example, juvenile Atlantic creolefish (P. furcifer) and small squat lobster (Munida simplex), both common prey items for lionfish (Eddy et al., 2016), are common at 60 m but absent at shallow depths (Sterrer, 1985; Eddy, 2016). Importantly, as macroalgae and seagrass are less common in deep water around Bermuda (Manuel et al., 2013), and those macroalgal species that are present at depth tend to be less palatable (Goodbody-Gringley et al., 2019; Stefanoudis et al., 2019b), δ13C values among teleosts and crustaceans decrease with depth as the carbon sources become more planktonic and pelagic. Although horizontal distances between deep water (i.e., 60 m) and shallow water areas (i.e., 10 m) around Bermuda can be as little as 1.5 km, this is still enough to segregate shallow carbon sources from deep carbon sources. This is particularly true for species with small ranges, such as lionfish (Jud and Layman, 2012; Tamburello and Côté, 2015), at least on the temporal scales at which stable isotopes are integrated by predators.
The predictable changes in δ15N and δ13C between trophic levels (i.e., isotope enrichment rates or TDFs; DeNiro and Epstein, 1978; DeNiro and Epstein, 1981; Minagawa and Wada, 1984; Peterson and Fry, 1987; McCutchan et al., 2003; Caut et al., 2009) provide an opportunity to estimate the relative isotopic position of the resource pools from which lionfish and other consumers within the reef community derive their energy, thus identifying the theoretical base of these animals’ food webs. Considering the diversity of known lionfish prey items and a general lack of isotopic data by which to describe them, it would not be appropriate to use a multiple-source mixing model (i.e., Vander Zanden and Rasmussen, 2001) to reconstruct the diet of lionfish. Therefore, back-calculating resource pools using TDFs is a more suitable approach. However, the use of different TDFs generates considerable variability in the identification of carbon resource pools. Using global mean TDFs from Post (2002) suggested that lionfish food webs derived resources nearly equally from plankton and macroalgae. However, species- and location-specific TDFs are thought to provide more accurate and reliable results. Our approach, using global means (Post, 2002) for 1.0 trophic level [i.e., the number of trophic steps between primary producers (TP = 1) and consumers] together with lionfish-specific TDFs (Eddy, 2019) for 1.2 trophic levels (i.e., the number of trophic steps between primary consumers and lionfish), suggests most of the resources used by lionfish in Bermuda are derived from a plankton-based food web, with a less substantial contribution from macroalgae. Use of mean values for other marine teleosts (i.e., Caut et al., 2009) produces a similar finding for potential competitors, indicating that this applies more broadly across reef fish species in Bermuda, at least among the species included in this study. Indeed, planktonic productivity is important in Bermuda’s oligotrophic mid-oceanic location where terrestrial nutrient inputs to reef environments are relatively low (Smith et al., 2013). The importance of planktonic production to the base of demersal foods webs has also been described by Davenport and Bax (2002) for a reef ecosystem off southeastern Australia.
As lionfish are a recognized threat to marine ecosystems across their invaded range, with demonstrated ecological impacts (Albins and Hixon, 2008; Green et al., 2012; Lesser and Slattery, 2011), it is important to understand the various mechanisms through which these impacts occur. While the impact of lionfish predation is well established, the impact caused by resource competition is less understood, but the use of stable isotope ecology provides new ways to investigate community structure and explore how lionfish may affect the resource use of other teleosts. This approach shows substantial overlap between the isotopic niches of lionfish and certain ecologically and economically important native meso-predators (Figure 10), which may be indicative of resource competition.
It appears that lionfish in Bermuda compete most with coney groupers (Cephalopholis fulva), squirrelfishes2 (Holocentridae), and red hind (Epinephelis guttatus), eclipsing 54, 23, 22%, respectively, of the niche space of these species. This is supported by the known feeding habits of these species, previously identified by SCA (Randall, 1967). Similarly, Hackerott et al. (2013) suggested coney grouper, red hind, and graysby (Cephalopholis cruentata) would be the most likely competitors of lionfish based on their similar foraging habitats and known prey items. As graysby is not abundant in Bermuda, we did not collect a sufficient number of samples to calculate a reliable estimate of its trophic niche (Jackson et al., 2011) and thus cannot comment on this potential interaction. In contrast, lionfish eclipse a smaller portion of the niches belonging to gray snapper (L. griseus, 11%) and Bermuda’s black grouper (Mycteroperca bonaci, 4%) and have no isotopic niche overlap with Lane snapper (L. synagris), a species that forages over open sandy areas where lionfish do not typically hunt (Biggs and Olden, 2011; C. Eddy, personal observation). At first glance, it may appear counterintuitive that lionfish eclipse 19% of the isotopic niche of juvenile Galapagos sharks (C. galapagensis). However, they are known to feed on similar species, including a variety of mid-sized herbivores and planktivores (C. Eddy, unpublished data), so although different size classes are targeted, the prey species in question are unlikely to undergo substantial ontogenetic shifts in diet or isotope profiles. Altogether, it should be recognized that competitive interactions between lionfish and native species across the invaded region will vary according to the species composition of a given community, which may vary spatially, both horizontally and with depth, and also seasonally.
Considering the degree of trophic overlap between lionfish and several co-occurring mesopredators (Figure 10 and Table 5), it is important to consider the possible impacts of such competition on ecosystem structure and function. Depletion of certain prey items by lionfish may cause changes in the diet of other mesopredators, which could respond by choosing lower quality prey and/or modifying their feeding behavior, which may in turn expose them to greater predation risks during novel feeding events (Hrabik et al., 1998; Jackson, 2002; Albins, 2013). Consequences could potentially include reductions in growth, reproduction, and overall fitness of these competing species, or increased mortality, all of which could then lead to a reduction in abundance (Hrabik et al., 1998; MacRae and Jackson, 2001; Jackson, 2002; Hixon and Jones, 2005; Albins, 2015). Additionally, Hrabik et al. (1998) suggests that competition may decrease the strength of entire year classes, with subsequent impacts on community structure as well as population size and dynamics. As mesopredators have a direct influence on the abundance of smaller reef fishes, any effect that lionfish may have upon them, via exploitative competition could very well disrupt community structure and function.
Our data suggest that, in Bermuda, the coney grouper is likely to sustain the greatest competitive impact from lionfish. This could be compounded by the fact that the annual fishery landings of coney grouper, and thus their mortality rates, have increased considerably since 1990 (Trott and Luckhurst, 2008). Future research to monitor potential changes in diet (including isotopic niche), growth, reproduction, and abundance of coney grouper, as well as other potentially competing mesopredators (e.g., squirrelfish and red hind), could indicate interspecific impacts and ecosystem effects of exploitative competition.
This study demonstrates that SIA and isotopic niches provide a valuable tool to recognize and highlight the ecological role of lionfish and the potential impact they may exert via resource competition. Recognizing this concern, our results provide a foundation for resource managers to estimate the impact of lionfish upon competitors, model ecosystem-wide effects of the invasion, and design or alter fisheries management plans to account for that. Furthermore, in the absence of baseline isotopic data for Bermuda prior to the arrival of lionfish, our results provide a benchmark by which future changes in trophic ecology and trophodynamics can be measured across the entire ecosystem.
Data Availability Statement
The datasets generated for this study are available on request to the corresponding author.
Ethics Statement
Ethical review and approval was not required for the animal study because all animals used in this research were collected and examined in Bermuda. Ethical review and approval were not required by Bermuda’s Department of Environment and Natural Resources, which does not have an animal ethics committee.
Author Contributions
CE and JP executed the fieldwork and collected data. CE, JL, and MA analyzed the data. All authors developed the concept for this study and contributed to writing of the manuscript.
Funding
This work was supported by the National Science Foundation (DGE-1144241); the UK Department for Environment, Food, and Rural Affairs’ Darwin Plus program (DPLUS001); and the University of Massachusetts Dartmouth; and the Bermuda Institute of Ocean Sciences.
Conflict of Interest
The authors declare that the research was conducted in the absence of any commercial or financial relationships that could be construed as a potential conflict of interest.
Acknowledgments
The authors gratefully acknowledge the logistical support from Bermuda’s Department of Environment and Natural Resources and the Bermuda Zoological Society. Among the numerous commercial fishermen and lionfish cullers who provided samples for this study, we particularly recognize Mark Outerbridge, Chris Cabral, Alex Davidson, Weldon Carl Wade, William Gillett, Jon Pedro, Adrienne Smatt, Andrew Conyers, Andrew de Melo, Adrian Kawaley-Lathan, Michael Sinclair, Tommy Sinclair, Joshua Hill, the Jedi Master Chris Flook, Tim Noyes, Mark Moran, Stephen Harrold, and Forrest Williams Jr. The authors are also grateful to John Logan from the Massachusetts Division of Marine Fisheries for his help reviewing and revising an early version of this manuscript.
Supplementary Material
The Supplementary Material for this article can be found online at: https://www.frontiersin.org/articles/10.3389/fmars.2020.00435/full#supplementary-material
TABLE S1 | Sample sizes, δ15N, δ13C, and standard errors for species mentioned in Figures 8A,B.
Footnotes
- ^ It is important to acknowledge here that plankton includes detritus and zooplankton and should not strictly be considered a source of primary production, but instead a primary food source.
- ^ Our analysis of squirrelfish is assumed to include multiple species because samples provided from fishermen often were only identified with the common name, “squirrelfish”. With that in mind, the isotopic niche of the family (Holocentridae) used in this study (6.47) should not be used to compare to a single competing species.
References
Albins, M. A. (2013). Effects of invasive Pacific red lionfish Pterois volitans versus a native predator on Bahamian coral-reef fish communities. Biol. Invasions. 15, 29–43. doi: 10.1007/s10530-012-0266-1
Albins, M. A. (2015). Invasive Pacific lionfish Pterois volitans reduce abundance and species richness of native Bahamian coral-reef fishes. Mar. Ecol. Prog. Ser. 522, 231–243. doi: 10.3354/meps11159
Albins, M. A., and Hixon, M. A. (2008). Invasive Indo-Pacific lionfish Pterois volitans reduce recruitment of Atlantic coral-reef fishes. Mar. Ecol. Prog. Ser. 367, 233–238. doi: 10.3354/meps07620
Albins, M. A., and Hixon, M. A. (2013). Worst case scenario: potential long-term effects of invasive predatory lionfish (Pterois volitans) on Atlantic and Caribbean coral-reef communities. Environ. Biol. Fishes 96, 1151–1157. doi: 10.1007/s10641-011-9795-1
Altabet, M. A. (1989). A time-series study of the vertical structure of nitrogen and particle dynamics in the Sargasso Sea. Limnol. Oceanogr. 34, 1185–1201. doi: 10.4319/lo.1989.34.7.1185
Bearhop, S., Adams, C. E., Waldron, S., Fuller, R. A., and MacLeod, H. (2004). Determining trophic niche width: a novel approach using stable isotope analysis. J. Anim. Ecol. 73, 1007–1012. doi: 10.1111/j.0021-8790.2004.00861.x
Biggs, C. R., and Olden, J. D. (2011). Multi-scale habitat occupancy of invasive lionfish (Pterois volitans) in coral reef environments of Roatan. Honduras. Aquat. Invasions 6, 447–453.
Boecklen, W. J., Yarnes, C. T., Cook, B. A., and James, A. C. (2011). On the use of stable isotopes in trophic ecology. Annu. Rev. Ecol. Evol. Syst. 42, 411–440.
Burgett, C. M., Burkholder, D. A., Coates, K. A., Fourqurean, V. L., Kenworthy, W. J., Manuel, S. A., et al. (2018). Ontogenetic diet shifts of green sea turtles (Chelonia mydas) in a mid-ocean developmental habitat. Mar. Biol. 165:33.
Caut, S., Angulo, E., and Courchamp, F. (2009). Variation in discrimination factors (Δ15N and Δ13C): the effect of diet isotopic values and applications for diet reconstruction. J. Appl. Ecol. 46, 443–453. doi: 10.1111/j.1365-2664.2009.01620.x
Davenport, S. R., and Bax, N. J. (2002). A trophic study of a marine ecosystem off southeastern Australia using stable isotopes of carbon and nitrogen. Can. J. Fish. Aquat. Sci. 59, 514–530. doi: 10.1139/f02-031
DeNiro, M. J., and Epstein, S. (1978). Influence of diet on the distribution of carbon isotopes in animals. Geochim. Cosmochim. Acta 42, 495–506. doi: 10.1016/0016-7037(78)90199-0
DeNiro, M. J., and Epstein, S. (1981). Influence of diet on the distribution of nitrogen isotopes in animals. Geochim. Cosmochim. Acta 45, 341–351. doi: 10.1016/0016-7037(81)90244-1
Eddy, C. (2016). An Investigation of the Biology and Ecology of the Invasive Lionfish (Pterois Volitans and P. Miles) to Explore their Ecological Impact and Inform Management in Bermuda’s Marine Ecosystem. PhD Dissertation, University of Massachusetts Dartmouth, Dartmouth, MA.
Eddy, C. (2019). Trophic discrimination factors for invasive lionfish (Pterois volitans and P. miles) in Bermuda. Biol Invasions 21, 3473–3477. doi: 10.1007/s10530-019-02078-7
Eddy, C., Pitt, J., Morris, J. A., Smith, S., Goodbody-Gringley, G., and Bernal, D. (2016). Diet of invasive lionfish (Pterois volitans and P. miles) in Bermuda. Mar. Ecol. Prog. Ser. 558, 193–206. doi: 10.3354/meps11838
Fourqurean, J. W., Manuel, S. A., Coates, K. A., Kenworthy, W. J., and Boyer, J. N. (2015). Water quality, isoscapes and stoichioscapes of seagrasses indicate general P limitation and unique N cycling in shallow water benthos of Bermuda. Biogeosci 12, 6235–6249. doi: 10.5194/bg-12-6235-2015
France, R. (1995). Critical examination of stable isotope analysis as a means for tracing carbon pathways in stream ecosystems. Can. J. Fish. Aquat. Sci. 52, 651–656. doi: 10.1139/f95-065
Genner, M. J., Turner, G. F., Barker, S., and Hawkins, S. J. (1999). Niche segregation among lake malawi cichlid fishes? Evidence from stable isotope signatures. Ecol. Lett. 2, 185–190. doi: 10.1046/j.1461-0248.1999.00068.x
Goodbody-Gringley, G., Noyes, T., and Smith, S. R. (2019). “Bermuda. Chapter 2,” in Mesophotic Coral Ecosystems, eds Y. Loya, K. A. Puglise, and T. C. L. Bridge (Berlin: Springer).
Green, S. J., Akins, J. L. M., Maljkovic, A., and Côté, I. M. (2012). Invasive lionfish drive Atlantic coral reef fish declines. PLoS One 7:e32596. doi: 10.1371/journal.pone.0032596
Gruber, N., Keeling, C. D., and Bates, N. R. (2002). Interannual variability in the North Atlantic Ocean carbon sink. Sci 298, 2374–2378. doi: 10.1126/science.1077077
Hackerott, S., Valdivia, A., Green, S. J., Côté, I. M., Cox, C. E., Akins, L., et al. (2013). Native predators do not influence invasion success of Pacific lionfish on Caribbean reefs. PLoS One 8:e68259. doi: 10.1371/journal.pone.0068259
Hesslein, R. H., Hallard, K. A., and Ramlal, P. (1993). Replacement of sulfur, carbon, and nitrogen in tissue of growing broad whitefish (Coregonus nasus) in response to a change in diet traced by δ34S, δ13C, and δ15N. Can. J. Fish. Aquat. Sci. 50, 2071–2076. doi: 10.1139/f93-230
Hixon, M. A., and Jones, G. P. (2005). Competition, predation, and density-dependent mortality in demersal marine fishes. Ecology 86, 2847–2859. doi: 10.1890/04-1455
Hobson, K. A. (1999). Tracing origins and migration of wildlife using stable isotopes: a review. Oecologia 120, 314–326. doi: 10.1007/s004420050865
Hobson, K. A., and Clark, R. G. (1992). Assessing avian diets using stable isotopes I: turnover of 13C in tissues. Condor 181–188. doi: 10.2307/1368807
Hrabik, T. R., Magnuson, J. J., and McLain, A. S. (1998). Predicting the effects of rainbow smelt on native fishes in small lakes: evidence from long-term research on two lakes. Can. J. Fish. Aquat. Sci. 55, 1364–1371. doi: 10.1139/f98-032
Jackson, A. L., Inger, R., Parnell, A. C., and Bearhop, S. (2011). Comparing isotopic niche widths among and within communities: SIBER–stable isotope bayesian ellipses in R. J. Anim. Ecol. 80, 595–602. doi: 10.1111/j.1365-2656.2011.01806.x
Jackson, D. A. (2002). Ecological effects of micropterus introductions: the dark side of black bass. Am. Fish. Soc. Sympos. 31, 221–232.
Jackson, M. C., Donohue, I., Jackson, A. L., Britton, J. R., Harper, D. M., and Grey, J. (2012). Population-level metrics of trophic structure based on stable isotopes and their application to invasion ecology. PLoS One. 7:e31757. doi: 10.1371/journal.pone.0031757
Jacobsen, I. P., and Bennett, M. B. (2013). A comparative analysis of feeding and trophic level ecology in stingrays (Rajiformes; Myliobatoidei) and electric rays (Rajiformes: Torpedinoidei). PLoS One 8:e71348. doi: 10.1371/journal.pone.0071348
Jud, Z. R., and Layman, C. A. (2012). Site fidelity and movement patterns of invasive lionfish, Pterois spp., in a Florida estuary. J. Exp. Mar. Biol. Ecol. 414, 69–74. doi: 10.1016/j.jembe.2012.01.015
Knapp, A. N., Sigman, D. M., and Lipschultz, F. (2005). N isotopic composition of dissolved organic nitrogen and nitrate at the bermuda atlantic time-series study site. Global Biogeochem. Cycles 19, 1–15.
Landrum, J. P., Altabet, M. A., and Montoya, J. P. (2011). Basin-scale distributions of stable nitrogen isotopes in the subtropical North Atlantic Ocean: contribution of diazotroph nitrogen to particulate organic matter and mesozooplankton. Deep Sea Res. I 58, 615–625. doi: 10.1016/j.dsr.2011.01.012
Layman, C., and Allgeier, J. (2012). Characterizing trophic ecology of generalist consumers: a case study of the invasive lionfish in the Bahamas. Mar. Ecol. Prog. Ser./ 448, 131–141. doi: 10.3354/meps09511
Layman, C. A., Arrington, D. A., Montaña, C. G., and Post, D. M. (2007). Can stable isotope ratios provide for community-wide measures of trophic structure? Ecology 88, 42–48. doi: 10.1890/0012-9658(2007)88[42:csirpf]2.0.co;2
Leibold, M. A. (1995). The niche concept revisited: mechanistic models and community context. Ecology 76, 1371–1382. doi: 10.2307/1938141
Lesser, M. P., and Slattery, M. (2011). Phase shift to algal dominated communities at mesophotic depths associated with lionfish (Pterois volitans) invasion on a Bahamian coral reef. Biol. Invasions 13, 1855–1868. doi: 10.1007/s10530-011-0005-z
MacRae, P. S., and Jackson, D. A. (2001). The influence of smallmouth bass (Micropterus dolomieu) predation and habitat complexity on the structure of littoral zone fish assemblages. Can. J. Fish Aquat. Sci. 58, 342–351. doi: 10.1139/f00-247
Manuel, S. A., Coates, K. A., Kenworthy, W. J., and Fourqurean, J. W. (2013). Tropical species at the northern limit of their range: composition and distribution in Bermuda’s benthic habitats in relation to depth and light availability. Mar. Environ. Res. 89, 63–75. doi: 10.1016/j.marenvres.2013.05.003
McCutchan, J. H., Lewis, W. M., Kendall, C., and McGrath, C. C. (2003). Variation in trophic shift for stable isotope ratios of carbon, nitrogen, and sulfur. Oikos 102, 378–390. doi: 10.1034/j.1600-0706.2003.12098.x
McMahon, K. W., Hamady, L. L., and Thorrold, S. R. (2013). Ocean Ecogeochemistry - A review. Oceanogr. Mar. Biol. Annu. Rev. 51, 327–374.
Minagawa, M., and Wada, E. (1984). Stepwise enrichment of 15N along food chains: further evidence and the relation between δ15N and animal age. Geochim. Cosmochim. Acta 48, 1135–1140. doi: 10.1016/0016-7037(84)90204-7
Morris, J. A., and Akins, J. L. (2009). Feeding ecology of invasive lionfish (Pterois volitans) in the bahamian archipelago. Environ. Biol. Fishes 86, 389–398.
Muñoz, R. C., Currin, C. A., and Whitfield, P. E. (2011). Diet of invasive lionfish on hard bottom reefs of the Southeast USA: insights from stomach. Mar. Ecol. Prog. Ser. 432, 181–193. doi: 10.3354/meps09154
Newsome, S. D., Martinez del Rio, C., Bearhop, S., and Phillips, D. L. (2007). A niche for isotopic ecology. Front. Ecol. Environ. 5:429–436. doi: 10.1890/060150.1
O’Farrell, S., Bearhop, S., McGill, R. A., Dahlgren, C. P., Brumbaugh, D. R., and Mumby, P. J. (2014). Habitat and body size effects on the isotopic niche space of invasive lionfish and endangered Nassau grouper. Ecosphere 5, 1–11.
Peake, J., Bogdanoff, A. K., Layman, C. A., Castillo, B., Reale-Munroe, K., Chapman, J., et al. (2018). Feeding ecology of invasive lionfish (Pterois volitans and Pterois miles) in the temperate and tropical western Atlantic. Biol. Invasions 20, 2567–2597. doi: 10.1007/s10530-018-1720-5
Peterson, B. J., and Fry, B. (1987). Stable isotopes in ecosystem studies. Annu. Rev. Ecol. Syst. 18, 293–320. doi: 10.1146/annurev.es.18.110187.001453
Post, D. M. (2002). Using stable isotopes to estimate trophic position: models, methods, and assumptions. Ecology 83, 703–718. doi: 10.1890/0012-9658(2002)083[0703:usitet]2.0.co;2
Post, D. M., Layman, C. A., Arrington, D. A., Takimoto, G., Quattrochi, J., and Montana, C. G. (2007). Getting to the fat of the matter: models, methods and assumptions for dealing with lipids in stable isotope analyses. Oecologia 152, 179–189. doi: 10.1007/s00442-006-0630-x
Randall, J. E. (1967). Food Habits of Reef Fishes of the West Indies. Erdemli: Institute of Marine Sciences.
Smith, S. R., Sarkis, S., Murdoch, T. J. T., Weil, E., Croquer, A., Bates, N. R., et al. (2013). “Threats to coral reefs of bermuda. Chapter 13,” in Coral Reefs of the United Kingdom Overseas Territories, Coral Reefs of the World 4, ed. C. R. C. Sheppard (Berlin: Springer Science+Business Media Dordrecht), 173. doi: 10.1007/978-94-007-5965-7_13
Stefanoudis, P. V., Gress, E., Pitt, J. M., Smith, S. R., Kincaid, T., Rivers, M., et al. (2019a). Depth-dependent structuring of reef fish assemblages from the shallows to the rariphotic zone. Front. Mar. Sci. 6:307. doi: 10.3389/fmars.2019.00307
Stefanoudis, P. V., Rivers, M., Smith, S. R., Schneider, C. W., Wagner, D., Ford, H., et al. (2019b). Low connectivity between shallow, mesophotic and rariphotic zone benthos. R. Soc. Open sci. 6:190958. doi: 10.1098/rsos.190958
Tamburello, N., and Côté, I. M. (2015). Movement ecology of Indo-Pacific lionfish on Caribbean coral reefs and its implications for invasion dynamics. Biol. Invasions. 17, 1639–1653. doi: 10.1007/s10530-014-0822-y
Trott, T. M., and Luckhurst, B. E. (2008). “An evaluation of the status of the coney, Cephalopholis fulva, population in Bermuda,” in Proceedings of the Gulf and Caribbean Fisheries Institute, Vol. 60, 257–262. Punta Cana, Dominican Republic. Available online at: http://aquaticcommons.org/id/eprint/15431
Turner, T. F., Collyer, M. L., and Krabbenhoft, T. J. (2010). A general hypothesis-testing framework for stable isotope ratios in ecological studies. Ecology 91, 2227–2233. doi: 10.1890/09-1454.1
Vander Zanden, M. J., and Rasmussen, J. B. (2001). Variation in δ15N and δ13C trophic fractionation: implications for aquatic food web studies. Limnol Oceanogr. 46, 2061–2066. doi: 10.4319/lo.2001.46.8.2061
Keywords: lionfish, stable isotope analysis, community structure, feeding ecology, resource competition, invasive species, impacts of invasion
Citation: Eddy C, Pitt JM, Larkum J, Altabet MA and Bernal D (2020) Stable Isotope Ecology of Invasive Lionfish (Pterois volitans and P. miles) in Bermuda. Front. Mar. Sci. 7:435. doi: 10.3389/fmars.2020.00435
Received: 02 December 2019; Accepted: 18 May 2020;
Published: 26 June 2020.
Edited by:
Christos Dimitrios Arvanitidis, Hellenic Centre for Marine Research (HCMR), GreeceReviewed by:
Mauro Sinopoli, University of Naples Federico II, ItalyDanielle Denley, Simon Fraser University, Canada
Copyright © 2020 Eddy, Pitt, Larkum, Altabet and Bernal. This is an open-access article distributed under the terms of the Creative Commons Attribution License (CC BY). The use, distribution or reproduction in other forums is permitted, provided the original author(s) and the copyright owner(s) are credited and that the original publication in this journal is cited, in accordance with accepted academic practice. No use, distribution or reproduction is permitted which does not comply with these terms.
*Correspondence: Corey Eddy, Y29yZXllZGR5MUBnbWFpbC5jb20=