- 1College of Public Health, Medical and Veterinary Sciences, James Cook University, Townsville, QLD, Australia
- 2College of Science and Engineering, James Cook University, Townsville, QLD, Australia
- 3Marineholmen RASLab AS, Bergen, Department of Biosciences, Faculty of Mathematics and Natural Sciences, University of Bergen, Bergen, Norway
- 4Leigh Marine Laboratory, The University of Auckland, Leigh, New Zealand
It is hard to find a definition of gill health in the literature although there is a lot of information on changes to gill structure as a result of infectious and non-infectious challenge. How these changes relate to overall fish health is sometimes not clear. Interaction between the gill, the fish, and a range of anticipated changes in the environment will have a currently unknown effect on marine health and aquaculture production. To a degree, fish will likely be able to ameliorate certain changes, such as compensating for slightly elevated carbon dioxide; however, these actions may come at the cost of compromising other functions such as osmoregulation. Compensation will also depend on gill epithelial health and other environmental factors like external nitrogen and ammonia sources which can rise depending on the direction future culture and levels of eutrophication take. Fish can also remodel gill structure in response to salinity, hypoxia, or acidification but it appears that increased temperatures may be associated with increased pathology observable in the gill, and certain fishes may be more susceptible to change. There is a need for more targeted research into climate change-specific gill physiology and a need to recognise gill health as being a key component of food security and not just fish health.
Introduction
Gill diseases represent a significant challenge in marine and freshwater finfish aquaculture globally (Iva et al., 1999; Adams and Nowak, 2003; Teruo et al., 2005; Griffin et al., 2009; Lovy et al., 2011; Mitchell and Rodger, 2011; Kim et al., 2016; Oldham et al., 2016; Bloecher et al., 2018), and may involve infectious or non-infectious agents (Rodger et al., 2011; Shi et al., 2015). Fish gills are responsible for a number of critical functions in addition to respiration: osmoregulation, excretion of nitrogenous waste, pH regulation, and hormone production (Herrero et al., 2018). Anticipated climatic change represents a big unknown in many respects, and it is not fully understood how infectious agents will be impacted by predicted oceanic changes or how agents will interact with their hosts with changing environmental parameters and natural phenomena, such as jellyfish swarms and phytoplankton blooms (Rodger et al., 2011; Vezzulli et al., 2013; Yao and Somero, 2014). In particular, the effect of predicted climate change on gill health is not understood. Temperature change, or climate change on a broad scale, is also thought likely to impact aquaculture through changes in the supply of fishmeal, fish oil, and ingredients derived on land as well as potentially increasing rates of eutrophication, harmful algal blooms, storminess, acidification, and disease (Klinger et al., 2017). Other anticipated impacts, around the British Isles, for example, include risks to infrastructure as a result of changes in the frequency and strength of storms, shifting shoreline morphology if sea levels rise, and altered rainfall patterns affecting turbidity and nutrient loading of rivers that, in turn, may trigger harmful algal blooms (Callaway et al., 2012) and presumably risk gill disease and subsequent fish health. Indeed, emergence, translocation, and virulence of diseases, parasites, and pathogens are some of the more serious but least predictable effects of climate change (Callaway et al., 2012; Vezzulli et al., 2013). There is growing concern over the role of jellyfish in gill disease with multiple reports of fish kills from several species and from biofouling organisms (Purcell et al., 2007; Baxter et al., 2011a, b, 2012; Marcos-López et al., 2016; Bosch-Belmar et al., 2017). Although there are likely to be multifactorial causes to population changes in jellyfish, such as anthropogenic “fishing down the food chain” and changes in eutrophication, there seems to be evidence in Japan, the Mediterranean, and the Irish Sea that, as a result of warming conditions and climate change, some species of jellyfish are becoming more abundant in some areas although not in others (Richardson et al., 2009). The United Nations describes climate change as the defining issue of our time…[with] weather patterns that threaten food production (Anon, 2019). The projected increase of the average temperature could also warm oceans to depths of at least 3000 m, which may have direct and indirect effects on aquatic organisms, such as their metabolism and migration to other geographical areas, as well as the formation of harmful algal blooms that could form marine biotoxins and contaminate, for example, mussels used for food production (Miraglia et al., 2009). The same United Nations report warns of predicted sea level rises of 24–30 cm by 2065 compared to pre-Industrial Revolution levels and reports an increase in global temperature of 0.85°C in the period from 1880 to 2012. By 2100, the concentration of atmospheric carbon dioxide is expected to exceed 500 parts per million with global temperatures expected to rise by at least 2°C (Hoegh-Guldberg et al., 2007). The authors report these values significantly exceed those of at least the past 420,000 years during which most extant marine organisms evolved. What effect this will have on gill health and subsequently on fish health remains to be seen.
What is Gill Health?
In order to begin to understand the effect of anticipated climate change on a fish’s health, understanding what gill health is and what affects it is probably a good start. The central tenet of homeostasis in most health sciences is that physiological function in the face of stressors should be maintained within safe operational limits. How important the gill is to fish and how far limits can be stretched before health is compromised is not well understood. Aristotle (350 Bc), within his work Historia Animalium, noted that the fish gill was an “exceptional organ,” but it was not until the late 1800s and early 1900s that the true multifunctional nature of the fish gill was established. Fish are the most diverse vertebrate group on Earth with approximately 25,000 species, all with marked anatomical and physiological differences at different latitudes and in different niches, and this review cannot generalize the situation for all fish that have evolved diverse gill function for different modes of life, active or sedentary, and physicochemical environments, such as temperature, oxygen, salinity, turbidity, and ammonia.
Attributing fish health to gill health is not always immediately obvious, and measuring the health of the gill can be problematic. Obtaining a definition of health itself in veterinary medicine is surprisingly difficult. A very simple definition of health in veterinary medicine might essentially be a dichotomous one, the presence or absence of disease (Gunnarsson, 2006)—a view popular in epidemiological studies in which disease frequency is often calculated based on whether the animal or population has or has not got the disease in question. This is, in turn, subject to case definitions, inclusion and exclusion criteria, and subsequent sensitivity and specificity impacts. Within the context of veterinary medicine, Gunnarsson found definitions of health in only 8% of 500 relevant publications. He described five categories of health definition: health as normality, as biological function, as homeostasis, as physical and psychological well-being, and as productivity including reproduction. Defining gill health itself is more elusive.
Although there are many excellent descriptions of lesions encountered in gills and how branchial tissue responds to infectious and non-infectious injury, the concept of gill health is not explicitly defined, but rather assumed based on reference made to normal and abnormal anatomical structure, disruption of branchial function, and further effects on the individual and population including altered production parameters (Wildgoose, 2002; Ferguson, 2006; Noga, 2010; Roberts, 2012). Presence of detectable change is more clearly described than the health of the gill per se, and based on current descriptions, gill health might, therefore, be framed around the presence or absence of detectable change—an opinion consistent with health as a function of normality and presumably biological function that Gunnarsson (2006) describes. However, gill disease should also be interpreted through a combination of behavior, clinical signs, gross pathology, histopathology (Mitchell et al., 2012), and other laboratory findings, an approach familiar to veterinary and fish health care practitioners. Other data have also been used to assess overall health status, including Fulton’s condition factor (CF), hepatic somatic index (HSI), and hematocrit and also gill histopathology (Saraiva et al., 2015). Histological indicators of gill disease include hypertrophy and hyperplasia of the epithelial and mucus cells, presence or absence of mast cells, lymphocytic and eosinophilic granular cell (EGC) infiltration, hyperemia and necrosis of gill tissue, and sequential progression of histopathology lesions (Ferguson et al., 1992; Dyková and Novoa, 2001; Bermingham and Mulcahy, 2004; Andrews et al., 2010; Saraiva et al., 2015). A disadvantage of histopathology is the subjective nature of interpretation, and a number of workers have devised evaluation schemes similar to those used to assess gross lesions to transform qualitative histological observations into semi-quantitative indices (Bernet et al., 1999; Mitchell et al., 2012; de Lima Cardoso et al., 2018). Behavioral changes and clinical signs, such as “coughing,” gasping, and excessive mucus production can indicate direct branchial tissue irritation or damage, and flared opercula and gilling can indicate a reaction to adverse environmental conditions (Roberts and Smith, 2011). Inspecting grossly apparent pathology allows the examiner to assess changes in anatomical structure at the macroscopic level and to avoid the subjective nature of interpretation; attempts to standardize and semi-quantify lesions with scoring regimes that can be non-specific or specific to a particular infection, like amoebic gill disease (AGD), have been created (Adams and Nowak, 2001; Wildgoose, 2002; Ferguson, 2006; Taylor et al., 2009; Noga, 2010; Mitchell and Rodger, 2011; Rodger et al., 2011; Roberts, 2012; Saraiva et al., 2015; Bloecher et al., 2018). Macroscopic or gross examination is commonly used to assess the need to treat significant gill conditions like AGD (Taylor et al., 2009) and is taken as an indicator of gill health and as a proxy for fish health—at least in the early stages of disease progression; in the latter stages of “disease,” there will likely be a number of other signs contributing to the overall assessment of fish health. In addition, diagnostic tools, like quantitative PCR, are used for initial diagnosis and as a method of “scoring” the severity of infection as in the case of Paramoeba peruans, Ca. Brachiomonas cisticola, and Desmozoon lepeophtherii, which, together and in combination with harmful algal and zooplankton, have given rise to the term “complex gill disease” (CGD) (Gjessing et al., 2019). In the case of CGD, macroscopic gill scoring may not necessarily reflect the gill pathology associated with any individual agent. Thus, although only semi-quantitative, tools like PCR are useful. Other technologies, like shape recognition, expression of inflammatory cytokines and gill mucus proteins, and assessment of immune status, have also been used at a laboratory level to assess health of the animal by the presence or absence of changes in the gill (Powell et al., 2014; Valdenegro-Vega et al., 2014; Saraiva et al., 2015; Shi et al., 2015; Sweidan et al., 2015). In channel catfish Ictalurus punctatus, gill pathology associated with Henneguya spp. appeared to be secondary to pre-inflammatory destruction of collagen and gill cartilage (Lovy et al., 2011), suggesting that, at least in some cases, gill health may already be compromised before the observable changes we assume have been caused by the identifiable agent. Ironically, the initial presence of Paramoeba perurans could be taken as a sign of a healthy gill because the ameba are known to infect or re-infect healthy tissue only (Adams and Nowak, 2003). The effect of various lesions, including hyperplasia and hypertrophy, is not always easy to translate into a meaningful assessment of branchial function, and the multifactorial nature of determinants causing gill disease appears highly likely. Because correct clinical interpretation of findings is complex and includes recognizing that a healthy gill condition is not just characterized by the complete absence of any histopathological changes, it appears that healthy gills do not always have to be completely “normal” in appearance, and apparently, clinically healthy fish may have gills displaying moderate alterations, such as epithelial hyperplasia or mild inflammatory reactions or even the presence of a primary determinant of disease (Adams and Nowak, 2003; Bernet et al., 2004; Wiik-Nielsen et al., 2017). It seems intuitive that visible changes to gill tissue are going to be influenced by and, in some cases, caused by the environment the fish is raised in and that this, in turn, must be affected by any climate change. The influence of environment also depends largely on the method of culture, meaning production methods in aquaculture affect gill health and, presumably, as a result, fish health.
Fish in Fisheries and Aquaculture
Fish culture techniques vary widely but can be described as involving the use of open systems (including wild fish capture and ranching, pond aquaculture, and net-pen cage systems), semi-closed systems (enclosed net-bag, sea-tank systems, pump-ashore flow-through tank systems), and fully closed recirculating aquaculture systems (RAS). These types of production impose different demands on fish welfare, stress, and physiology. For example, open systems typically use relatively low stocking densities of approximately < 20 kg/m3 in marine net-pens compared with > 50 kg/m3 in closed RAS (Figure 1).
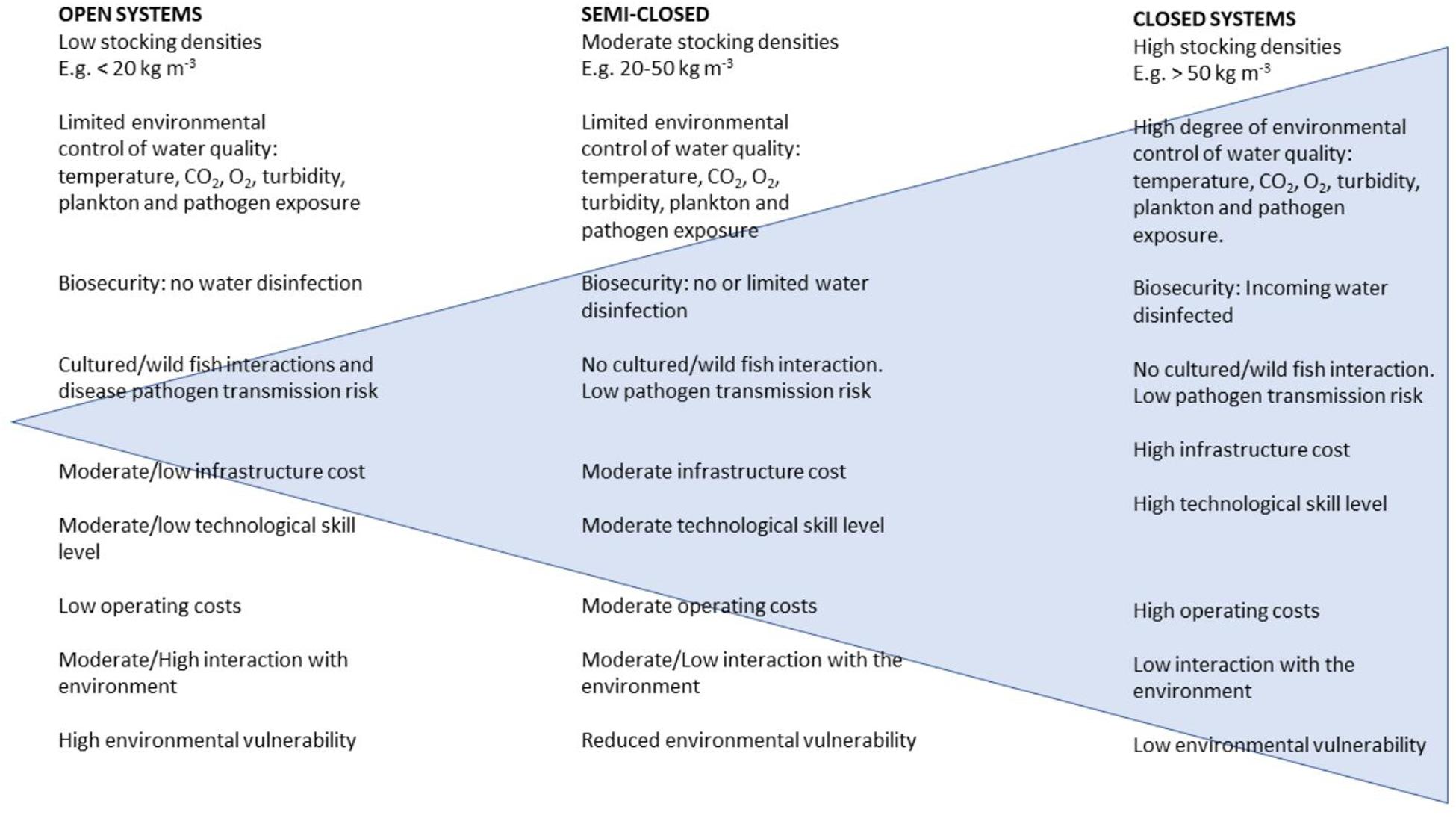
Figure 1. Qualitative comparison of the characteristics of open, semi-closed, and closed aquaculture systems with the qualitative range of complexity indicated by the blue triangle (used with permission, M. Powell).
There are different demands for water flow and water quality mitigation (filtration, nitrification, oxygenation, and disinfection) in closed systems compared to open systems in which water quality is a direct function of the environment. In terms of gill pathology, closed systems are more likely to be affected by water quality issues rather than external influences, such as plankton or pathogens (Holan et al., 2019). Consequently, RAS-based aquaculture is generally thought to be less susceptible to climate change impacts that might affect plankton or pathogen loads externally. Interactions between aquacultured and wild fish and their environment are a significant challenge in marine farming, but it should be emphasized that open-system farming represents both a risk to the (wild fish) environment and from the (wild fish) environment although the full import of that argument is beyond this review. Land-based farms are not always able to escape either. Wiik-Nielsen et al. (2017) find that the intake of seawater in an enclosed (land-based) system might be a route of entry for gill bacterial infections, such as Candidatus Branchiomonas cysticola, which can then be horizontally transmitted within the system. This translocated pathogen then has welfare and economic effects later in the cycle because it primarily affects Atlantic salmon pre-smolts and impacts smolt “quality” and future survival once transferred to sea, where a plethora of challenges and stressors await them. Although total bacterial loading on its own has not been directly linked to specific gill health issues, the presence of specific bacterial communities does appear to be important for larval fish performance, at least, with attendant repercussions to welfare and economic return (Munro et al., 1994; Salvesen et al., 1999; Verner-Jeffreys et al., 2003). In freshwater, conditions of poor water quality due to poor hygiene, increased temperature, and reduction in oxygen levels are significant risk factors for bacterial gill disease (BGD) caused by Flavobacterium branchiophilum, resulting in the proliferation of gill tissue and mucus that compromise gas exchange (Speare and Ferguson, 2006; Good et al., 2015). In marine systems, on the other hand, Tenacibaculum maritimum infections are acutely necrotic and often opportunistic, invading mechanically damaged gills, including those compromised by algae (Powell et al., 2004, 2005; Rodger et al., 2011). A rise in environmental temperature and organic carbon or eutrophication (Cochrane et al., 2009; De Silva and Soto, 2009; Koehn et al., 2011) seems likely to increase organic load and algae communities and could, therefore, result in further increases in total bacterial communities, which may increase levels of certain pathogens and disease outbreaks. Although T. maritimum is tolerant of a wide salinity range (10–35 ppt) and rarely occurs at temperatures below 15°C (Avenano-Herrera et al., 2006), the effect of environmental change on the progression of such infections and the consequence of their observable lesions is unknown. In light of this, and as an alternative to open production systems, mitigating the effects of an uncertain climate, such as temperature change, seems to be feasible through the use of highly intensive land-based facilities equipped with recirculation aquaculture technology (Ahmed et al., 2019). This would appear less applicable to open systems where environmental impacts of climatic change are likely to have a significant effect on production with the need for fish to be raised in locations of good water quality with lower levels of phyto- and zooplankton blooms. The environmental effect of having aquaculture in these zones with lower levels of phyto- and zooplankton blooms may be increased eutrophication—a problem thought likely to occur already with anticipated change, reducing the benefit of such pristine sites (Townhill et al., 2018). This is being addressed in some sectors of the aquaculture industry with the implementation of semi-closed and fully closed aquaculture systems, particularly for high-value cold-water species such as salmonids. However, for more temperate or tropical species, reliance remains on open systems. Semi-closed systems in marine aquaculture are driven by the need for a required freshwater stage in a semi-controlled environment (e.g., Atlantic salmon smolts, barramundi fry). If time held at sea can be reduced, the impact of infection from ectoparasites, such as the sea louse Lepeophtheirus salmonis, Caligus rogercressi, and P. perurans, can be somewhat reduced. Although closed systems appear to provide a distinct advantage as discussed previously, they may result in environments that facilitate severe gill pathology and fish mortality (Mouton et al., 2013). Closed systems may exclude agents, such as sea lice; however, they pose a risk for entrapment of other agents (including P. perurans) and harmful algae and jellyfish that are already a significant cause of gill morbidity and will presumably be more so as a result of environmental changes (O’Neil et al., 2012). An alternative strategy in order to escape the pathogens and harmful agents associated with inshore open-system aquaculture has been to move operations offshore into open ocean culture (Klinger et al., 2017). Unlike land-based production systems, aquaculture is constrained by variables that are less predictable and are influenced by climate change, including temperature and oceanographic parameters. However, despite this and depending on geographical location, open-ocean farming in the face of climate change predictions may remain a commercially viable option although, in order to exploit the benefits of offshore farming and circumnavigate climate change stressors in coastal waters, substantially larger and more robust infrastructure are required (Klinger et al., 2017). This could be in the form of larger structures capable of holding more fish, and the infrastructure capital cost per ton of fish produced will decrease. Unlike inshore aquaculture in relatively sheltered and, in some cases, shallow bays, where the maximum permitted biomass is regulated as a function of the impact on the sea bed, offshore environments with deep water and strong currents, are likely to be less impacted by stringent environmental regulation because fish-borne sediments are more likely dispersed with, presumably less environmental impact.
The Economic Sensitivity of Fish Production to Climate Change: Open Versus Closed Production
Although RAS technology is economically feasible and widely used in a number of different fish production environments, it is not without significant operational and economic risk. Perhaps the biggest limitations are the scale of production, poor design, overambitious production scheduling, and ineffective operations that compromise financial sustainability and longevity (DeIonno et al., 2006; Jeffrey et al., 2011). The extent to which RAS can replace open-ocean farming is currently being debated. Estimating the feasibility and cost of replacing open-ocean net-pen aquaculture with land-based RAS, therefore, makes for an interesting comparison (Table 1). The intensification of production on land leads to a significant increase in the cost of production compared to open-ocean net-pen farming, and this is a significant vulnerability (Warrer-Hansen, 2015) with potential social welfare connotations. However, a significant benefit of RAS-based culture is allowing fish to be farmed in a range of environments not limited to the coastal regions or to the natural range of a given species. Tvete (2016) reported that the costs for a marine farm of 5000-ton capacity in Norway would be in the range of 325–470 million NOK ($42.7–$53.5 million USD), and a land-based facility with the same level of production would cost, on average, slightly less at 300–450 NOK ($34.1–$51.2 million USD). After performing a modeled analysis of land-based salmon farming, Bennich (2015) concluded that, under ideal conditions, the production cost was approximately 21 NOK/kg, 8 NOK lower than the equivalent net-pen sea culture cost, meaning that production on land was viable. However, if production costs exceeded net-pen production by 8 NOK/kg (to 35 NOK/kg), then earnings before interest and taxes were negative, and the cost of recovering initial investments was not possible. Land-based production is, therefore, very sensitive to the cost of production, and this is heavily influenced by the need to maintain optimal water quality within the production system. This is important because unforeseen costs could arise from fish gill health in land-based operations in the form of treatment costs, production losses, and intangible costs, and these could tip the balance of optimality from land-based operations back to open production systems with all the increased susceptibility to oceanic changes that entails. Gill health is, therefore, an important component within the economics of fish food production and climate change mitigation potentially, and this should not be ignored. In addition, poor food conversion from impaired gill function, increased mortalities, cost of disposal, the cost of medication if required, and altered management practices can become significant (Shinn et al., 2015; Rodger, 2016; Herrero et al., 2018). In the case of P. paramoeba in particular, it is thought that increased temperature may affect the host response and favor higher attachment/growth capacity of the amebae (Benedicenti et al., 2019) influencing the development of AGD and possible progression of CGD. In addition to the significant cost of AGD, parasitism, such as amyloodiniosis, kudoa, Myxobolus spp., and Pleistophora spp., are known to have major economic impacts exacerbated by temperature increases (Shinn et al., 2015). How climate change impacts these costs will depend on many parameters, but as described above, it will also be influenced heavily by the production system, which needs to be taken into account. The role of gill health in the production of fish under climate change scenarios in different production systems is central and complex, but what do we know about how predicted change will impact gill function?
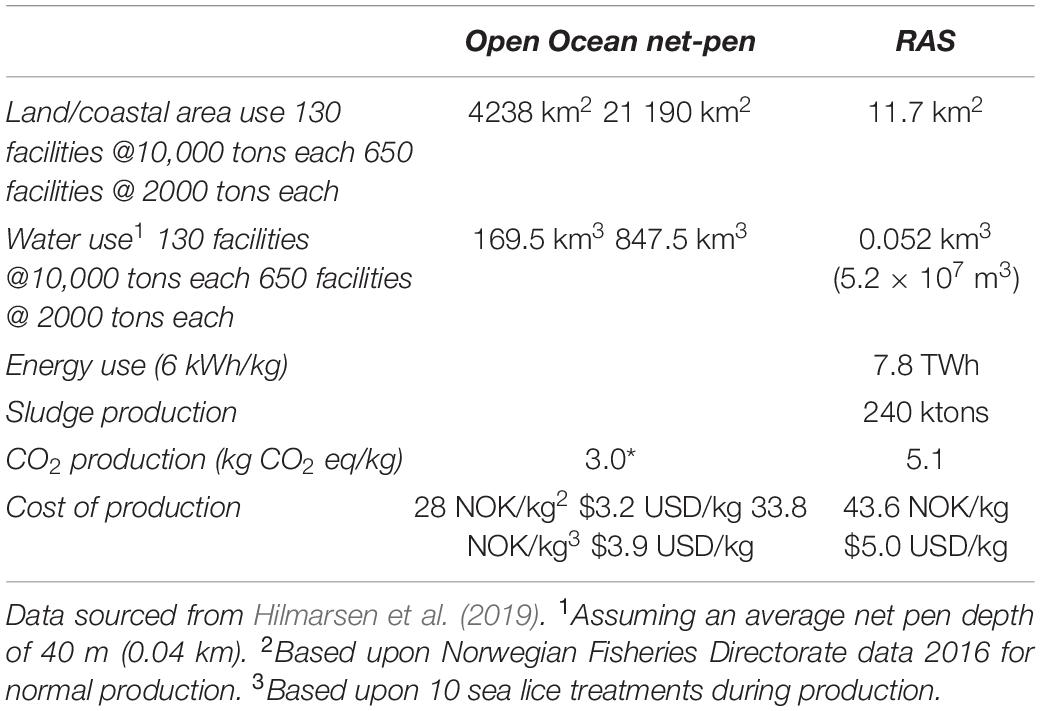
Table 1. Comparison of production requirements for 1.3 M tons of Atlantic salmon (Salmo salar), currently produced in Norway in ocean net-pen farming with that same production in recirculating aquaculture systems (RAS).
Gill Functions Impacted by Climate Change
The epithelial surface area of the fish gill is similar to that of the total skin area, and in some species, it is larger (Roberts, 2012). The gill is, therefore, extremely important to fish because it is known to be the dominant site for (i) gas exchange, (ii) osmoregulation, (iii) acid–base regulation, and (iv) excretion of nitrogenous waste (Evans, 1993; Evans et al., 2005) and is likely to have a profound influence over fish physiological performance during any climate change. The research literature for climate change has increased dramatically in recent years, but it usually ignores the effect of simultaneous stressors that have compounding impacts and/or complex interactions (e.g., Heuer and Grosell, 2014; Kreiss et al., 2015; Huth and Place, 2016; Lefevre, 2016; Michael et al., 2016; Nilsson and Lefevre, 2016). Experiments examining stressors in isolation do not, therefore, represent real-world conditions with multiple stressors, such as warming seas that are also acidified, particularly when stress is presented acutely and usually well above tolerance limits or end-of-century projections (Bresolin de Souza et al., 2014; Heuer and Grosell, 2014; Lefevre, 2016). Published experiments, however, do highlight potential areas of biological sensitivity and important pathways for future focus with respect to fish gill function; gill function in gas exchange, osmoregulation, acid–base regulation, and excretion of nitrogenous waste is reviewed extremely well elsewhere (Heisler, 1990; Evans, 1993; Brauner and Randall, 1996; Claiborne et al., 2002; Perry and Gilmour, 2002; Evans et al., 2005), so this section only attempts to distill key facts and unknowns with respect to fish gill function under the two main and direct climate change stressors of warming and acidification.
The Role of the Gills in Gas Exchange
Fish gills have an elaborate structure–function relationship with the environment and are usually the main gas-exchange organ where oxygen is taken up into the body and carbon dioxide is removed via diffusion, creating useable ATP energy through aerobic metabolic pathways, meaning the gills serve as an important conduit for these processes to occur. Fish gills though are multifunctional, and even under optimal conditions, marine fish face an osmo-respiratory compromise in which a balance must be struck between diffusing more gases (oxygen and carbon dioxide) across a highly perfused gill against the efflux of water and ion gain in saltwater fish. Climate change stressors place an increasing demand on this balancing act as rising temperature raises metabolic rates and requires more gill perfusion for the transfer of oxygen and carbon dioxide, possibly even more so when fish gills are remodeled with greater lamellar surface area (Sollid et al., 2005; Sollid and Nilsson, 2006). Rising temperature also causes ionoregulatory disturbances on its own (Gonzalez and McDonald, 2000). At the same time, ocean acidification leads to marked acid–base disturbances and is thought to place an increasing demand on osmoregulatory processes (ion exchange) at the gills (Evans, 1986; Heuer and Grosell, 2014). The interplay of all these functions in the context of climate change stressors remains relatively unstudied, but it is clear that the role of the fish gill and the response of different species to stressors is highly complex with multifunctional processes in terms of gas exchange, osmoregulation, acid–base regulation, and nitrogenous waste excretion all interlinked (McDonald et al., 1991). There is also the additional challenge of dealing with stressors, such as metal exposure not directly related to climate change (Dornelles Zebral et al., 2019). Fish physiologists, therefore, face the challenge of disentangling the multidimensional impacts of multiple climate change stressors across the myriad different fish species.
Susceptibility of Gill Gas Exchange to Rising Temperature
Most fish are poikilothermic, so global climate change and the associated rise in temperature will raise their rate of metabolism (Schulte, 2015). This not only means that oxygen and carbon dioxide need to move across the gills in larger volumes at a faster rate, but the extra oxygen demand occurs at a time of reduced oxygen availability in the environment due to the lower oxygen solubility of water at elevated temperatures (Schulte, 2015). Rising temperatures will, therefore, inevitably place pressure on gaseous exchange across the gills. Subtle compensatory adjustments to heart rate and opercular ventilation under normal conditions can maintain the level of oxygen and carbon dioxide in the blood and, thus, also maintain acid–base status within tight controlled limits (Perry and Gilmour, 2002). However, the peak level of whole body oxygen uptake, notably aerobic metabolic scope (AMS)—the difference between basal and maximal rates of oxygen uptake (Lefevre, 2016) is apparently reduced in some species at elevated temperatures (Khan et al., 2014; Rodgers et al., 2019). This concept of “oxygen and capacity limited thermal tolerance (OCLTT)” that dictates whether fish survive and perform optimally at elevated temperatures, particularly if temperatures approach lethal limits (Pörtner and Knust, 2007), is now not thought to apply to all species or conditions (Lefevre, 2016). For example, barramundi (Lates calcarifer) continue to increase AMS with increasing temperatures right up to its critical thermal maxima (Norin et al., 2014)—evidence that this species is not limited in its ability to extract oxygen across its gills under critically warm aquatic conditions up to its maximum tolerance. Although some authors report major gill histopathology such as aneurism, lamellar fusion, and gill oxidative stress (Jeffries et al., 2013; Madeira C. et al., 2016; Madeira D. et al., 2016; Rodgers et al., 2019) in response to elevated temperatures, it seems that factors other than oxygen uptake capacity across the gills influence survival of fish approaching their upper thermal limits. Fish hearts, for example, appear to fail well before oxygen flux becomes limiting during thermal stress, suggesting that cardiac health is potentially more important than respiratory and gill health under global warming scenarios (Iftikar and Hickey, 2013). Pauly and Cheung (2017) also recently proposed that fish body size will be reduced under conditions of ocean warming because the functional surface area of the gills cannot keep pace with the oxygen demand of large, three-dimensional bodies [Gill-Oxygen Limitation Theory (GOLT)]. However, this requires further testing to validate (Lefevre et al., 2017; Pauly and Cheung, 2018). Given that oxygen uptake across the gills may be limited in a warming world, possibly lowering fish growth and threatening food security, further scrutiny should be prioritized.
Susceptibility of Gill Gas Exchange to Ocean Acidification
Ocean acidification creates acid–base disturbances, which are usually fully compensated for in fish (Esbaugh et al., 2012), often at a cost to osmoregulation (Heuer and Grosell, 2014). The effect on osmoregulation is thought to alter metabolic oxygen consumption by increasing the resting basal metabolic rate (BMR; loading stress) or reducing the maximal metabolic rate (MMR; performance-limiting stress). However, evidence from the literature is not entirely supportive of either (Heuer and Grosell, 2014; Lefevre, 2016), and measuring the whole-animal oxygen consumption rates of fish is notoriously difficult. The rate at which oxygen is extracted across the gills from an acidified environment will not be fully understood until respirometry methods are improved and standardized across different studies.
The Role of the Gills in Acid–Base Regulation
Metabolic enzymes are highly sensitive to pH change and are controlled through acid–base regulation (Heisler, 1990; Claiborne et al., 2002). Aerobic and anaerobic metabolism yields carbon dioxide and acid–base relevant end products, such as H+ and lactic acid, and these must be regulated (eliminated) so that toxic accumulation is avoided and a steady state between pH (H+), pCO2, and HCO–3 is maintained (Esbaugh et al., 2012). Exogenous factors, such as temperature (global warming), carbon dioxide (ocean acidification), and hypoxia, all place a demand on acid–base regulation (Tresguerres and Hamilton, 2017), so assessing these factors in light of climate change would seem to be prudent. The mechanisms available for fish acid–base regulation include adjusting the partial pressure of carbon dioxide, pCO2, by a change in ventilation although this may be of less importance to climate change as small environmental-arterial pCO2 differences exist for fish in their normal aquatic environments (Claiborne et al., 2002). Fish can and do minimize transient pH changes within the body quite effectively through the use of non-bicarbonate buffers that transfer surplus H+ ions into a non-dissociated state. However, the tightest control of body pH is arguably achieved via the transfer of acid–base relevant ions (i.e., H+, OH–, and HCO–3) across the epithelial surface of the fish gill (McDonald et al., 1989; Heisler, 1990; Claiborne et al., 2002).
Susceptibility of Gill Acid–Base Regulation to Rising Temperature
Fish are subject to temperature-dependent changes to intracellular buffering characteristics and pH with body pH declining with increasing body temperature. A new steady state in plasma pH, pCO2, and HCO–3 will inevitably be reached and have to be maintained by poikilothermic fish facing climate warming. The temperature-dependent drop in plasma pH is associated with an increase in pCO2 and an efflux of HCO–3 across the gill epithelia into the water. The efflux of HCO–3 in exchange for Cl– is not immediate, occurring over hours, and any temperature change associated with climate change will occur over a much greater time scale, precluding the need for fast compensation (Heisler, 1993). According to Heisler (1993), the pH optima of energy-producing enzymes is apparently wide enough to support modest short-term disruptions to plasma pH. Each fish species presumably has a low-end limit to plasma pH, beyond which metabolic enzymes are unable to operate optimally, but to our knowledge, the plasma pH “tipping point” for different fish species is not known. Nor is it known when any temperature-dependent change in acid–base status has severe disrupting effects on other functions, such as osmoregulation or nitrogenous waste excretion, presuma bly due to conflict in the direction of acid–base ion movements across the gill epithelia. With the prospect of raised temperatures, long-term thermal acclimation studies are clearly required to address this issue.
Susceptibility of Gill Acid–Base Regulation to Ocean Acidification
Elevated ambient carbon dioxide either reduces or reverses the pCO2 gradient between the environment and fish body reducing the ability to excrete it. In the absence of compensation, the buildup of carbon dioxide will acidify the tissue fluid compartment (Heuer and Grosell, 2014; Tresguerres and Hamilton, 2017). For fish experiencing severe, acute environmental hypercapnia, carbon dioxide is generally transferred straight across the gill to the fish resulting in a similar increase in pCO2 with a correlated decrease in plasma pH. However, this decline in blood pH usually returns to normal levels due to an elevation in bicarbonate concentration, which compensates for, or even completely negates, the initial rise in pCO2 (Heisler, 1993; Esbaugh et al., 2012). This bicarbonate appears to be mainly supplied from the environment as a result of acid–base ion transporters on the gill epithelia (Esbaugh et al., 2012). Full pH compensation is remarkably efficient in fish and can be achieved even under an extreme hypercapnia level exceeding 10,000 μatm via the uptake or retention of HCO–3 and/or via the net excretion of acid (Heuer and Grosell, 2014). This is clearly in excess of the 1000 μatm level predicted in the year 2100 (Bernie et al., 2010), suggesting that some fish at least should be able to cope with rising carbon dioxide levels. Fish are remarkable acid–base regulators, but such stringent control and shift in ion regulatory capacity probably comes at a cost to osmoregulation and, therefore, potential gas exchange and metabolism (Deigweiher et al., 2008, 2010). Acid–base regulation during hypercapnia, for example, has a potential conflict with osmoregulation as ion transport typically needs to run in the opposite direction (e.g., Cl–/HCO–3 and Na+/H+ exchange in marine teleosts). However, as most studies have generally utilized extreme levels of hypercapnia, how these processes will be impacted at the end of the century (i.e., 1000 μatm level of carbon dioxide) remains to be seen.
The Role of the Gills in Osmoregulation
The gills of all fish are integral to the process of osmoregulation; branchial epithelium is generally the site for osmosis and ionic diffusion and is also the site of active ion transport. The role of the gill differs across different species: conformers versus strict osmoregulators versus partial osmoregulators, for example, and particularly between freshwater and marine species for which the salt and water gradients across the body are opposing. Further complexity exists for diadromous species. Freshwater fish generally gain water via osmosis and lose salts via diffusion, so the mitochondrion-rich cells (MRCs) or “chloride cells” of the branchial epithelium are active in the process of transporting ions back into the body against a concentration gradient via Na+/H+ and Cl–/HCO–3 exchange in the MRC. Water loss through osmosis and extreme salt gain is the problem faced by marine species, so again, the MRCs of the branchial epithelium play a major role in expelling excess ions, particularly the monovalent salts Na+ and Cl–. Basolateral Na+/K+-ATPase generates an electrochemical gradient that pulls Cl– into the MRC from the blood via a Na–K–Cl cotransporter (NKCC channel) and out to seawater via apical channels. The electrochemical gradient created by Cl– efflux from the MRC is what drives Na+ out of the blood to seawater via leaky, paracellular junctions in the branchial epithelium (Evans et al., 2005). As osmoregulation is a highly energetic process comprising approximately 20–50% of total energetic expenditure (Boeuf and Payan, 2001), there is great potential for climate change stressors to place extra metabolic demands on fish as a result of disturbed gill function.
Susceptibility of Gill Osmoregulatory Function to Rising Temperature
Acute rising temperature has been shown to affect gill osmoregulatory functions of fish differently with freshwater fish experiencing greater Na+ efflux and marine damselfish, for example, showing higher levels of plasma osmolality—both of which indicate higher gill permeability and a passive diffusion of ions (Gonzalez and McDonald, 2000; Tang et al., 2014). Interestingly, Gonzalez and McDonald (2000) showed that the stenothermal rainbow trout (Oncorhynchus mykiss) recovered ion balance after a 2-week period, whereas the eurythermal shiner (Notropis cornutus) did not. This questions whether different species need to defend osmolality and ionic composition around a strict steady-state set point in order to survive. The ramifications of ionoregulatory disturbances with climate change stressors may, therefore, need careful assessment. With respect to the effect of temperature on ion transporters, gill Na+/K+-ATPase activity was raised in response to an acute temperature increase in Atlantic cod (Gadus morhua) and a stenothermal Antarctic Nototheniid species (Kreiss et al., 2015; Michael et al., 2016; Kandalski et al., 2018). This correlated with improved osmoregulation in warmed cod but not for the Nototheniids. In another study, Na+/K+-ATPase protein activity was downregulated and presumed to downregulate energetic costs in heat-shocked, wild-caught adult pink (Oncorhynchus gorbuscha) and sockeye (Oncorhynchus nerka) salmon (Jeffries et al., 2013). Marked species-specific effects in the gill osmoregulatory response to temperature would seem to exist, and this would need further exploration in the context of climate change.
Susceptibility of Gill Osmoregulatory Function to Ocean Acidification
Heuer and Grosell (2014) proposed that environmental hypercapnia could theoretically interfere with osmoregulation and increase basal metabolic costs as a result of several processes (Deigweiher et al., 2010; Kreiss et al., 2015). Exposure to elevated carbon dioxide appears to increase the drinking rate of marine teleosts, compounding uptake of salt into the body, and second, the abundance and activity of branchial transporters (e.g., Na+/K+-ATPase) appears to increase in a range of carbon dioxide-exposed fish species, either responding to the need to excrete excess ions across the branchial epithelium and/or to maintain acid base status via Na+/H+, Cl–/HCO–3, and Na+/HCO–3 exchange (Heuer and Grosell, 2014; Esbaugh et al., 2016). Carbon dioxide exposure did not, however, affect the osmoregulatory ability of Atlantic salmon smolts in their transition from fresh water to seawater (McCormick and Regish, 2018). There is still a paucity of literature examining the effects of acidification on osmoregulation (Kreiss et al., 2015; Michael et al., 2016), and the situation remains complex because of the entwined processes involved in osmo- and acid–base regulation (Esbaugh et al., 2016). Focused research in the future will, we hope, disentangle the impact of climate change on these functions.
The Role of Gills in Nitrogenous Waste Excretion
When amino acids from food are catabolized, the amino group (–NH2) must either be released by deamination or transferred by transamination to another molecule for removal or reuse. If the amino groups are not recycled into amino acids, they must be dissolved in water and then excreted to avoid a toxic buildup of nitrogenous waste in the body (Evans et al., 2005; Randall, 2011). Most fish are considered ammonotelic, so >80% of nitrogenous waste is excreted across the gills, predominantly as ammonia, and indeed, up to 60% of nitrogen intake is excreted across the gills within 24 h, highlighting the crucial and active role the gills serve in this process (Evans et al., 1989; Wood, 1993; Wilkie, 2002; Evans et al., 2005). For the majority of teleosts, ammonia excretion is linked with pH because the unionized ammonia molecule (NH3) in solution typically combines with a proton to form an ammonium ion (NH4+), and more NH4+ will form under acidic conditions (Evans et al., 2005; Randall, 2011). However, at normal physiological levels of pH in living tissues, a plentiful amount of NH3 still exists, and the gaseous form is free to diffuse and be excreted across the gills (Wilkie, 2002; Randall, 2011).
Susceptibility of Nitrogenous Waste Excretion to Climate Change Stressors
Acidified waters enhance the ability of fish to excrete ammonia because NH3 diffusing into the environment across the gill readily combines with a proton and is trapped as NH4+ (Randall, 2011). Ammonium is unable to diffuse back across the gill into the fish. In this scenario, ocean acidification as a result of climate change may actually help to maintain the gradient for NH3 movement across the gill as a result of “acid trapping” (Randall, 2011). While acidified waters might not pose a major threat to NH3 excretion, active NH4+ excretion could theoretically occur with Na+ uptake, which may lead to a conflict between osmoregulation and waste excretion in some marine teleosts. Examining the costs associated with this interaction and whether Na+/NH4+ exchange is a more likely mode of ammonia excretion for fish facing global climate change is a fruitful area for future research.
To our knowledge, there is no specific literature that examines the impact of elevated temperatures on nitrogenous waste excretion, but because temperature elevates the rate of metabolism of ectothermic fish, it is likely that the catabolism of dietary and/or structural protein would increase, leading to heightened levels of ammonia production and excretion. Whether fish can raise their rate of ammonia excretion in the face of other interacting climate change stressors, such as ocean acidification, remains unstudied but it appears that rising temperatures combined with acidified waters is unlikely to threaten nitrogenous waste excretion across the gill of fish in a major way. This is not the case for ammonia loading in the environment, for example, as a result of sewerage breakdown, because high environmental ammonia provides the biggest obstacle for nitrogenous waste excretion across the fish gill (Randall, 2011). Elevated ammonia in the environment reduces the rate of NH3 excretion and can lead to toxic buildup as a result of NH3 uptake into the body (Randall, 2011). Some fish, such as mudskippers, are adapted to this condition (Randall et al., 1999; Evans et al., 2005) but, for the majority of species, gill function may be threatened by climate change stressors, particularly with an increase in fish density and high nitrogen loading—a situation likely to be commonplace in future aquaculture under climate change.
Gill Remodeling in Response to Climate Change
Gill remodeling may play an important role in the resilience and acclimation of fish to future environmental conditions given the link between gill morphology and gill function (Laurent and Perry, 1991; Evans et al., 2005). Gill remodeling relates to changes in the abundance and distribution of epithelial cell populations in the gills in response to endogenous (e.g., oxygen demand) and exogenous factors (e.g., rising temperatures).
Gill Remodeling in Response to Rising Temperatures
Some species display a remarkable capacity to remodel key morphological traits that influence gill function, such as gill surface area and gas diffusion distance (i.e., the distance between water and blood sinus, Figure 2). A large gill surface area paired with a small gas diffusion distance is generally associated with enhanced diffusion of gas and ions across the branchial epithelium (Bindon et al., 1994; Lappivaara et al., 1995, reviewed in Sakuragui et al., 2003; Gonzalez, 2011; Gilmour and Perry, 2018). Crucian carp (Carassius carassius), in particular, are well known to use the growth of epithelial cells to modify their gill surface area and gas diffusion distance to regulate gill function (Sollid et al., 2005). At low temperatures (10–20°C), crucian carp grow an “inter-lamellar cell mass” in between their lamellae, leading to the fusion of adjacent lamellae, the reduction of gill surface area, and an increase in gas diffusion distance (Sollid et al., 2005; Gilmour and Perry, 2018). This reduction in surface area reduces diffusive ion loss across the branchial epithelium and allows crucian carp to reduce energetic costs associated with osmoregulation at times when oxygen demand is low (Sollid et al., 2005; Gilmour and Perry, 2018). This process can be reversed to where the inter-lamellar cell mass is rapidly shed in response to rising temperatures in the summer (>25°C) thereby increasing gill surface area by up to 7.5-fold and enhancing oxygen uptake (Sollid et al., 2005). Over a dozen species to date have been found to exhibit a similar capacity for gill remodeling like crucian carp (e.g., LeBlanc et al., 2010; Blair et al., 2016). Although those species have been found to have specific adaptations allowing them to tolerate extreme reductions in gill surface area and associated reductions in oxygen uptake, numerous other species have also been observed to undergo modifications in gill morphology in response to environmental changes, albeit to a lesser extent (e.g., Wu et al., 2007; Uliano et al., 2010; Anni et al., 2016; Esbaugh et al., 2016). The majority of studies, however, have focused on describing morphological changes observed in response to pollution (including toxins, heavy metals, and suspended particulate matter), parasites, and pathogens (e.g., Mueller et al., 1991; Ahmed et al., 2013; Zarha and Mobarak, 2015; Marcon et al., 2016), and little is known about whether gill remodeling may also occur in response to rising temperatures in these species. It is important to note, however, that modest changes in gill surface area or gas diffusion distances may not directly translate into changes in oxygen uptake and carbon dioxide excretion rates or other gill functions as these processes are influenced by numerous other factors as well (Robertson et al., 2015; Gilmour and Perry, 2018, see also Cumming and Herbert, 2016; Esbaugh et al., 2016; Hess et al., 2017). Findings in coral reef fish indicate that fish living at low latitudes and high average summer temperatures may generally have little capacity for gill remodeling in response to rising temperatures as many species already exhibit small gas diffusion distances, leaving little room for further decreases (Bowden et al., 2014). Further, it appears that elevated (>30°C) temperatures may increase the occurrence of gill damage with observed changes including necrosis, aneurysms, and disorganization of lamellae (Rojas et al., 2013; Liu et al., 2015; Takata et al., 2018; Rodgers et al., 2019). Gill damage may negate any benefits of morphological changes that occur to regulate gill function in response to rising temperatures, and it will, thus, be important to better understand the potential impacts of elevated temperatures on gill tissues.
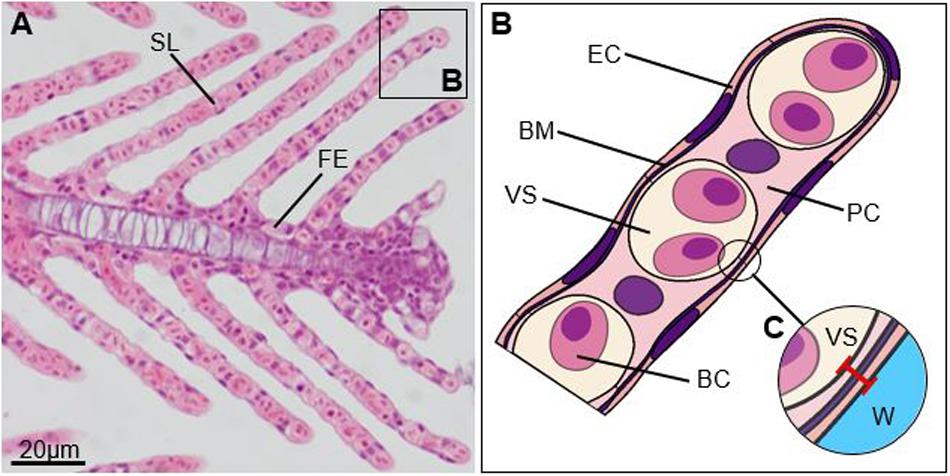
Figure 2. Gill filament. (A) Micrograph of a primary lamella, sagittal section through cartilaginous support (Bouin’s solution, H&E), (B) scheme of the tip of a secondary lamella, and (C) gas diffusion distance (red line). SL, secondary lamella; FE, filament epithelium; EC, epithelial cell; BM, basal membrane; VS, vascular space; PC, pillar cell; BC, blood cell; W, water.
Gill Remodeling in Response to Ocean Acidification
Gill remodeling has been suggested to play a role in the acclimation of acid–base regulation to ocean acidification (Esbaugh et al., 2012, 2016). As discussed previously, environmental hypercapnia reduces the carbon dioxide diffusion gradient across the branchial epithelium, leading to a reduced capacity for carbon dioxide excretion and potential acid–base disturbances (Strobel et al., 2012; Green and Jutfelt, 2014). In red drum (Sciaenops ocellatus), a 32% reduction in gas diffusion distance has been observed following exposure to 1000 μatm for 14 days (Esbaugh et al., 2016). A reduction in gas diffusion distance would be expected to enhance carbon dioxide excretion and may, thus, potentially compensate for a reduction in the carbon dioxide diffusion gradient. However, although a 30% reduction in plasma carbon dioxide was observed in red drum following 14 days exposure compared to acutely challenged fish (which did not have the chance to undergo gill remodeling), this difference was not statistically significant nor sufficient to fully offset the observed acid–base disturbance (Esbaugh et al., 2016). Identifying other species that may use gill remodeling to respond to ocean acidification and quantifying its benefits, if any, to acid–base regulation will be an interesting avenue for future research.
Fish have been observed to remodel MRC populations in the gills in response to hypercapnia, which may contribute to the acclimation of osmoregulatory functions to ocean acidification. As already discussed, the capacity for active ion transport at the gills has been linked to the fractional surface area or the proportion of the total gill surface area occupied by the MRCs (Goss et al., 1992; Bindon et al., 1994; Chang et al., 2001). The MRC fractional surface area is well known to be actively regulated by fish in response to various stressors via changes in the density of MRCs (Pandey et al., 2008; Paulino et al., 2012; Pereira et al., 2013), altering individual apical surface area folding or flattening of microridges in the apical membrane (Laurent et al., 1995; Matey et al., 2008; Aldoghachi et al., 2016), and exposure of MRCs to water (Rind et al., 2017; Carmo et al., 2018). The latter is modified by the migration of MRCs within the filament epithelium (e.g., from deeper cell layers to the surface; Carmo et al., 2018) and by neighboring pavement cells shifting or expanding to cover or uncover MRCs (Laurent et al., 1995; Baker et al., 2009). In freshwater fish, studies have documented decreases in density and individual apical surface area of MRCs in response to environmental hypercapnia (Goss et al., 1992; Goss and Perry, 1993; Baker et al., 2009) while the opposite has been observed in marine fish (Hayashi et al., 2013). These changes reflect the different compensation strategies of freshwater and marine fish (Hayashi et al., 2013). Although what remodeling of MRC populations means for osmoregulation has not been determined, the results highlight that changes in MRC populations in the gills and the effects of disease on the gills should be considered when examining the underlying physiological responses of fish to ocean acidification.
Conclusion
It is apparent that fisheries and aquaculture, in particular, face challenges in any climate change scenario. Anthropogenic influence seems to be central to the discussion, and the complexity envisaged means it is not possible to predict with certainty what effects change will have. Eutrophication will reduce the ability of zooplankton to control algae because it leads to cyanobacteria dominance, which is harder to feed on and digest (Moss et al., 2011), and together, climate change and eutrophication will limit the ability of zooplankton to control algae, reinforcing the potential for harmful blooms that have already been demonstrated as an emerging problem in gill health and in fish health more generally. Reactions to agents that cause insult to the gill include hypertrophy and hyperplasia among others, and fishes may be able to maintain homeostasis regardless of surface area and thickness of the respiratory epithelium. Of more relevance to climate change, they may be able to cope with rising carbon dioxide levels to a degree, but it comes quite likely at the expense of osmoregulation. Although smaller fish with a smaller respiratory epithelium surface area will have less diffusive ion loss reducing osmoregulatory stress, oxygen uptake by these smaller fish will be less efficient because of that smaller surface area. If this is compounded by respiratory epithelial thickening encountered during disease, the outcome is unlikely to be positive—all of this, and there appears to be some, albeit non-validated, evidence that oxygen uptake across the gills may be limited in a warming world, possibly lowering fish growth and even threatening food security. Our lack of understanding of the multifactorial nature of gill disease and the role of gill health within the context of overall fish health means we may be missing the true influence of gill disease on health and production. This is compounded with no clear way of assessing fish health based on gill health other than overt clinical disease with altered function and production in latter stages of presentation. Mortalities arising from AGD may also be related to cardiac function rather than just the primary gill lesion, and cardiac health is potentially more important than gill health under global warming scenarios (Powell et al., 2002; Leef et al., 2005; Iftikar and Hickey, 2013). Despite being able to accurately describe gross and microscopic changes in the gills, interpreting those changes and any agent associated with those lesions requires the involvement of multiple disciplines. In addition to secondary infections by bacteria like T. maritimum, agents such as Nolandella sp. in the presence and absence of Nolandella perurans, and the consequence of various other potential pathogens in CGD remains challenging (Bowman and Nowak, 2004; English et al., 2019; Rozas-Serri, 2019). The role of Candidatus B. cysticola in gill disease in farmed Atlantic salmon was not clear despite being found in high densities with Desmozoan lepeophtherii, and only D. lepeophtherii and Ichthyobodo spp. were associated with poor-conditioned fish while Paramoeba spp. and other pathogens in the study were not (Gunnarsson et al., 2017). Merely identifying an organism does not necessarily answer any questions. Examination of the gill at the microscopic level is useful to reveal changes, such as chloride cell dynamics, and perhaps overall, histopathology is the most useful tool, particularly in the initial stages of assessing gill health (Mitchell et al., 2012). Nonetheless, in order to properly interpret results from histopathological evaluations, the literature shows that a healthy condition is not characterized by the complete absence of any histologically apparent traits, and an apparently healthy gill may display moderate alterations, such as minor structural disorders or mild inflammatory reactions (Bernet et al., 2004), prompting the question of what does a healthy gill look like? In addition to assessing the gill’s normal or abnormal physical function, physiological function of the gills is going to be a prime concern for fish production in a changing environment, especially in light of the four very important roles that the fish gill serves: gas exchange, osmoregulation, acid–base regulation, and nitrogenous waste excretion. Fish gill function is already subject to excellent reviews (Heisler, 1990; Evans, 1993; Brauner and Randall, 1996; Claiborne et al., 2002; Perry and Gilmour, 2002; Evans et al., 2005), but the specific impact of key climate change stressors on the physiological function of the fish gill remains relatively un-researched, especially under the relevant time scales of environmental change of decades to centuries and not hours, and when multiple stressors, such as warmed, acidified seas, and the influence of disease are combined. It is understood that eutrophication can have many effects in the anticipated future, but high environmental loading with nitrogenous compounds, such as ammonia and ammonium, will also affect osmoregulation, which would be expected to be exacerbated by gill tissue lesions. Investigation is still hampered by the lack of standardized respirometry methods, and the situation is further complicated by the fact that many fish gill functions are intertwined, a fact demonstrated by osmoregulation and acid–base regulation via the gills involving the same ion transporters but often working in opposite directions when environmental stress is applied. Several species are known to use extensive remodeling of cell populations at the gill epithelium to regulate gill function in response to environmental change, and modest changes in gill morphology are commonly observed among a wide variety of species. This raises the question of whether gill remodeling will play a role in resilience and acclimation of fish to future environmental conditions with a limited number of studies finding gill remodeling in response to rising temperatures and the ocean acidification predicted to occur by the end of the century. Unfortunately, potential benefits to the acclimation or resilience of gill functions remain unclear, and it will be important to consider the potential damaging effects of elevated temperatures and concurrent increases in the incidence of disease on gill morphology that may negate any beneficial effects of gill remodeling, leaving fish vulnerable.
It is clear that, in tropical zones, many aquacultured finfish species are farmed close to their thermal limit, leaving little room for a rising trend in oceanic temperatures. Fishes in these zones also have little room for gill remodeling that is likely to occur in parallel with increased levels of gill damage. Given the effect of temperature on host and pathogen metabolism, it is noted that progression of disease in the lower latitudes is more rapid and results in higher cumulative mortality (Leung and Bates, 2013) with tropical producers suffering relatively more losses in aquaculture during disease outbreaks and having less time to initiate control and preventative measures compared to other regions. That is not to say that finfish aquaculture in other areas would not suffer the consequences as well. Atlantic salmon farming in Tasmania already occurs near the thermal limit for that species, and with sea temperatures expected to rise in the North Atlantic summers (Bromley et al., 2018), salmon farming in northern Europe may see increasingly more unfavorable conditions for salmon rearing with a concomitant increase in infectious and non-infectious disease. Adaptability may be specific to the taxonomic group with piscivorous species possibly affected more by elevated metabolic costs compared to other species that may rely more heavily on phenotypic plasticity, and exploited fish populations may be affected more as their genetic diversity is reduced, decreasing their ability to adapt to changing conditions (Araújo et al., 2018). Consequently, anticipated changes are thought of as a serious threat to sea bream (Sparus aurata) populations. Alternative locations and open-ocean farming have already been discussed, but future mitigation may also have to include breeding for increased tolerance to thermal stress and an understanding of how other climate variables might affect production, including the effect on agriculture, land use, and extreme weather events among others.
One of the main aims of aquaculture is to provide wholesome, nutritious food, and aquaculture is key to food security in many parts of the world (Béné et al., 2016). Fish health experts in conjunction with producers and laboratory-based scientists must work closely anyway but, in the future, will have to combine their efforts even more so with those of other disciplines, including but not limited to meteorology, oceanography, and environmental and social sciences. This could be argued to be consistent with One Health’s interdisciplinarity approach (Zinsstag, 2012) and is even more relevant to a One Health approach when livelihoods that depend on aquaculture are taken into account. Indeed, all forms of intensive farming, terrestrial or aquatic, can negatively impact the environment, threatening sustainability. This supports an even stronger argument for a holistic One Health approach to aquaculture but is considered beyond the remit of this review. The incidence of some infectious and non-infectious diseases will increase with climate change, and mitigation strategies must take into account global distribution of disease and vulnerability in aquaculture and develop options to minimize impacts on food production (Bell et al., 2011; Leung and Bates, 2013). Understanding the multifactorial nature of gill health and how it is impacted by anticipated climate change is central to this, and elevates the importance and relevance of gill health in food security and human well-being and not just in fish health.
Author Contributions
All authors contributed to the content. KF and NH contributed toward final editing.
Funding
This work was supported by the 2018 Gill Health Symposium for Marine Fish and was funded by the World Universities Network (WUN) and The University of Sydney South East Asia Centre. Travel to the workshop for KF was funded by Professional Development Funding 2018, Centre for Sustainable Tropical Fisheries and Aquaculture, James Cook University, for SH by a Fisheries Society of the British Isles International Travel Grant, 2018.
Conflict of Interest
The authors declare that the research was conducted in the absence of any commercial or financial relationships that could be construed as a potential conflict of interest.
References
Adams, M. B., and Nowak, B. F. (2001). Distribution and structure of lesions in the gills of Atlantic salmon, Salmo salar L., affected with amoebic gill disease. J. Fish Dis. 24, 535–542. doi: 10.1046/j.1365-2761.2001.00330.x
Adams, M. B., and Nowak, B. F. (2003). Amoebic gill disease: sequential pathology in cultured Atlantic salmon, Salmo salar L. J. Fish Dis. 26, 601–614. doi: 10.1046/j.1365-2761.2003.00496.x
Ahmed, M. K., Habibullah-Al-Mamun, M., Parvin, E., Akter, M. S., and Khan, M. S. (2013). Arsenic induced toxicity and histopathological changes in gill and liver tissue of freshwater fish, tilapia (Oreochromis mossambicus). Exp. Toxicol. Pathol. 65, 903–909. doi: 10.1016/j.etp.2013.01.003
Ahmed, N., Thompson, S., and Glaser, M. (2019). Global aquaculture productive, environmental sustainability and climate change adaptability. Environ. Manag. 63, 159–172. doi: 10.1007/s00267-018-1117-3
Aldoghachi, M. A., Azirun, M. S., Yusoff, I., and Ashraf, M. A. (2016). Ultrastructural effects on gill tissues induced in red tilapia Oreochromis sp. by a waterborne lead exposure. Saudi J. Biol. Sci. 23, 634–641. doi: 10.1016/j.sjbs.2015.08.004
Andrews, M., Battaglene, S., Cobcroft, J., Adams, M., Noga, E., and Nowak, B. (2010). Host response to the chondracanthid copepod Chondracanthus goldsmidi, a gill parasite of the striped trumpeter, Latris lineata (forster), in Tasmania. J. Fish Dis. 33, 211–220. doi: 10.1111/j.1365-2761.2009.01107.x
Anni, I. S. A., Bianchini, A., Barcarolli, I. F., Junior, A. S. V., Robaldo, R. B., Tesser, M. B., et al. (2016). Salinity influence on growth, osmoregulation and energy turnover in juvenile pompano Trachinotus marginatus Cuvier 1832. Aquaculture 455, 63–72. doi: 10.1016/j.aquaculture.2016.01.010
Anon, U. N. (2019). Climate Change. Global Issues. Available online at: http://www.un.org/en/sections/issues-depth/climate-change/index.html (accessed June 9, 2020).
Araújo, J. E., Madeira, D., Vitorino, R., Repolho, T., Rosa, R., and Diniz, M. (2018). Negative synergistic impacts of ocean warming and acidification on the survival and proteome of the commercial sea bream, Sparus aurata. J. Sea Res. 139, 50–61. doi: 10.1016/j.seares.2018.06.011
Aristotle (350 BC). Animalia Historium. Book 2, part 13. The Internet Classics Archive. Translated by D’Arcy Wentworth Thompson. Available online at: http://classics.mit.edu//Aristotle/history_anim.html (accessed May 6, 2020).
Avenano-Herrera, R., Toranzo, A. E., and Magarinos, B. (2006). Tenacibaculosis infection in marine fish caused by Tenacibaculum maritimum: a review. Dis. Aquat. Organ. 71, 255–266. doi: 10.3354/dao071255
Baker, D. W., Matey, V., Huynh, K. T., Wilson, J. M., Morgan, J. D., and Brauner, C. J. (2009). Complete intracellular pH protection during extracellular pH depression is associated with hypercarbia tolerance in white sturgeon, Acipenser transmontanus. Am. J. Physiol. Regul. Integr. Compar. Physiol. 296, R1868–R1880.
Baxter, E. J., Rodger, H. D., McAllen, R., and Doyle, T. K. (2011a). Gill disorders in marine-farmed salmon: -investigating the role of hydrozoan jellyfish. Aquac. Environ. Interact. 1, 245–257. doi: 10.3354/aei00024
Baxter, E. J., Sturt, M. M., Ruane, N. M., Doyle, T. K., McAllen, R., Harman, L., et al. (2011b). Gill damage to Atlantic Salmon (Salmo salar) caused by the common jellyfish (Aurelia aurita) under experimental challenge. PLoS One 6:e18529. doi: 10.1371/journal.pone.0018529
Baxter, E. J., Sturt, M. M., Ruane, N. M., Doyle, T. K., McAllen, R., and Rodger, H. D. (2012). Biofouling of the hydroid Ectopleura larynx on aquaculture nets in Ireland: implications for finfish health. Fish Vet. J. 13, 18–30.
Bell, J. D., Johnson, J. E., and Hobday, A. J. (eds) (2011). Vulnerability of Tropical Pacific Fisheries and Aquaculture to Climate Change. Noumea: Pacific Community.
Béné, C., Arthur, R., Norbury, H., Allison, E. H., Beveridge, M., Bush, S., et al. (2016). Contribution of fisheries and aquaculture to food security and poverty reduction: assessing the current evidence. World Dev. 79, 177–196. doi: 10.1016/j.worlddev.2015.11.007
Benedicenti, O., Pottinger, T. G., Collins, C., and Secombes, C. J. (2019). Effects of temperature on amoebic gill disease development: Does it play a role? J. Fish Dis. 42, 1241–1258. doi: 10.1111/jfd.13047
Bennich, T. (2015). The Economic Sustainability of Landbased Aquaculture Systems: An Integrated Analysis. MSc Thesis, University of Bergen, Bergen.
Bermingham, M. L., and Mulcahy, M. F. (2004). Environmental risk factors associated with amoebic gill disease in cultured salmon, Salmo salar L., smolts in Ireland. J. Fish Dis. 27, 555–571. doi: 10.1111/j.1365-2761.2004.00574.x
Bernet, D., Schmidt, H., Meier, W., Burkhardt-Holm, P., and Wahli, T. (1999). Histopathology in fish: proposal for a protocol to assess aquatic pollution. J. Fish Dis. 22, 25–34. doi: 10.1046/j.1365-2761.1999.00134.x
Bernet, D., Schmidt-Posthaus, H., Wahli, T., and Burkhardt-Holm, P. (2004). Evaluation of two monitoring approaches to assess effects of waste water disposal on histological alterations in fish. Hydrobiologia 524, 53–66. doi: 10.1023/b:Hydr.0000036196.84682.27
Bernie, D., Lowe, J., Tyrrell, T., and Legge, O. (2010). Influence of mitigation policy on ocean acidification. Geophys. Res. Lett. 37:L15704. doi: 10.1029/2010GL043181
Bindon, S., Gilmour, K., Fenwick, J., and Perry, S. (1994). The effects of branchial chloride cell proliferation on respiratory function in the rainbow trout Oncorhynchus mykiss. J. Exp. Biol. 197, 47–63.
Blair, S. D., Matheson, D., He, Y., and Goss, G. G. (2016). Reduced salinity tolerance in the Arctic grayling (Thymallus arcticus) is associated with rapid development of a gill interlamellar cell mass: implications of high-saline spills on native freshwater salmonids. Conserv. Physiol. 4:cow010. doi: 10.1093/conphys/cow010
Bloecher, N., Powell, M., Hytterod, S., Gjessing, M., Wiik-Nielsen, J., Mohammad, S. N., et al. (2018). Effects of cnidarian biofouling on salmon gill health and development of amoebic gill disease. PLoS One 13:e0199842. doi: 10.1371/journal.pone.0199842
Boeuf, G., and Payan, P. (2001). How should salinity influence fish growth? Comp. Biochem. Physiol. 130C, 411–423. doi: 10.1016/s1532-0456(01)00268-x
Bosch-Belmar, M., Azzurro, E., Pulis, K., Milisenda, G., Fuentes, V., Kéfi-Daly Yahia, O., et al. (2017). Jellyfish blooms perception in Mediterranean finfish aquaculture. Mar. Policy 76, 1–7. doi: 10.1016/j.marpol.2016.11.005
Bowden, A. J., Gardiner, N. M., Couturier, C. S., Stecyk, J. A. W., Nilsson, G. E., Munday, P. L., et al. (2014). Alterations in gill structure in tropical reef fishes as a result of elevated temperatures. Compar. Biochem. Physiol. A Mol. Integr. Physiol. 175, 64–71. doi: 10.1016/j.cbpa.2014.05.011
Bowman, J. P., and Nowak, B. (2004). Salmonid gill bacteria and their relationship to amoebic gill disease. J. Fish Dis. 27, 483–492. doi: 10.1111/j.1365-2761.2004.00569.x
Brauner, C. J., and Randall, D. J. (1996). The interaction between oxygen and carbon dioxide movements in fishes. Comp. Biochem. Physiol. 113A, 83–90. doi: 10.1016/0300-9629(95)02062-4
Bresolin de Souza, K., Jutfelt, F., Kling, P., Förlin, L., and Sturve, J. (2014). Effects of increased CO2 on fish gill and plasma proteome. PLoS One 9:e102901. doi: 10.1371/journal.pone.0102901
Bromley, G., Putnam, A., Borns, H. Jr., Lowell, T., Sandford, T., and Barrell, D. (2018). Interstadial rise and younger dryas demise of scotland’s last ice fields. Paleoceanogr. Paleoclimatol. 33, 412–429. doi: 10.1002/2018pa003341
Callaway, R., Shinn, A. P., Grenfell, S. E., Bron, J. E., Burnell, G., Cook, E. J., et al. (2012). Review of climate change impacts on marine aquaculture in the UK and Ireland. Aquat. Conserv. Mar. Freshw. Ecosyst. 22, 389–421. doi: 10.1002/aqc.2247
Carmo, T. L., Azevedo, V. C., Siqueira, P. R., Galvão, T. D., Santos, F. A., Martinez, C. B., et al. (2018). Mitochondria-rich cells adjustments and ionic balance in the Neotropical fish Prochilodus lineatus exposed to titanium dioxide nanoparticles. Aquat. Toxicol. 200, 168–177. doi: 10.1016/j.aquatox.2018.05.006
Chang, I. C., Lee, T. H., Yang, C. H., Wei, Y. Y., Chou, F. I., and Hwang, P. P. (2001). Morphology and function of gill mitochondria-rich cells in fish acclimated to different environments. Physiol. Biochem. Zool. 74, 111–119. doi: 10.1086/319304
Claiborne, J. B., Edwards, S. L., and Morrisson-Shetlar, A. L. (2002). Acid-base regulation in fishes: cellular and molecular mechanisms. J. Exp. Zool. 293, 302–319. doi: 10.1002/jez.10125
Cochrane, K., De Young, C., Soto, D., and Bahri, T. (2009). Climate change implications for fisheries and aquaculture. FAO Fish. Aquac. Tech. Pap. 530:212.
Cumming, H., and Herbert, N. A. (2016). Gill structural change in response to turbidity has no effect on the oxygen uptake of a juvenile sparid fish. Conserv. Physiol. 4:cow033. doi: 10.1093/conphys/cow033
de Lima Cardoso, R., Carvalho-Neta, R. N. F., de Castro, A. C. L., Ferreira, C. F. C., Silva, M. H. L., de Jesus Azevedo, J. W., et al. (2018). Histological and genotoxic biomarkers in Prochilodus lacustris (Pisces, Prochilodontidae) for environmental assessment in a protected area in the Northeast of Brazil. Bull. Environ. Contam. Toxicol. 101, 570–579. doi: 10.1007/s00128-018-2464-8
De Silva, S. S., and Soto, D. (2009). Climate change and aquaculture: potential impacts, adaptation and mitigation. Climate change implications for fisheries and aquaculture: overview of current scientific knowledge. FAO Fish. Aquac. Tech. Pap. 530, 151–212.
Deigweiher, K., Hirse, T., Bock, C., Lucassen, M., and Pörtner, H. O. (2010). Hypercapnia induced shifts in gill energy budgets of Antarctic notothenioids. J. Comp. Physiol. B. 180, 347–359. doi: 10.1007/s00360-009-0413-x
Deigweiher, K., Koschnick, N., Pörtner, H. O., and Lucassen, M. (2008). Acclimation of ion regulatory capacities in gills of marine fish under environmental hypercapnia. Am. J. Physiol. Regul. Integr. Comp. Physiol. 295, R1660–R1670.
DeIonno, P., Wines, G. L., Jones, P. L., and Collins, R. O. (2006). A bioeconomic evaluation of a commercial scale recirculating finfish growout system – An Australian perspective. Aquaculture 259, 315–327. doi: 10.1016/j.aquaculture.2006.05.047
Dornelles Zebral, Y., Roza, M., da Silva Fonseca, J., Gomes Costa, P., Sturmer de Oliveira, C., Gubert Zocke, T., et al. (2019). Waterborne copper is more toxic to the killifish Poecilia vivipara in elevated temperatures: linking oxidative stress in the liver with reduced organismal thermal performance. Aquat. Toxicol. 209, 142–149. doi: 10.1016/j.aquatox.2019.02.005
Dyková, I., and Novoa, B. (2001). Comments on diagnosis of amoebic gill disease (AGD) in turbot, Scophthalmus maximus. Bull. Eur. Ass. Fish Pathol. 21:40.
English, C. J., Swords, F., Downes, J. K., Ruane, N. M., Botwright, N. A., Taylor, R. S., et al. (2019). Prevalence of six amoeba species colonising the gills of farmed Atlantic salmon with amoebic gill disease (AGD) using qPCR. Aquac. Environ. Interact. 11, 405–415. doi: 10.3354/aei00325
Esbaugh, A. J., Ern, R., Nordi, W. M., and Johnson, A. S. (2016). Respiratory plasticity is insufficient to alleviate blood acid-base disturbances after acclimation to ocean acidification in the estuarine red drum, Sciaenops ocellatus. J. Comp. Physiol. B 186, 97–109. doi: 10.1007/s00360-015-0940-6
Esbaugh, A. J., Heuer, R., and Grosell, M. (2012). Impacts of ocean acidification on respiratory gas exchange and acid-base balance in a marine teleost, Opsanus beta. J. Comp. Physiol. B. 182, 921–934. doi: 10.1007/s00360-012-0668-5
Evans, D. H. (1986). “The role of branchial and dermal epithelia in acid-base regulation in aquatic vertebrates,” in Acid-Base Regulation in Animals, ed. N. Heisler (Amsterdam: Elsevier-North Holland), 139–172.
Evans, D. H. (1993). “Osmotic and ionic regulation,” in The Physiology of Fishes, ed. D. H. Evans (Boca Raton, FL: CRC Press), 315–341.
Evans, D. H., More, K. J., and Robbins, S. L. (1989). Modes of ammonia transport across the gill epithelium of the marine teleost fish, Opsanus beta. J. Exp. Biol. 144, 339–356.
Evans, D. H., Piermarini, P. M., and Choe, K. P. (2005). The multifunctional fish gill: dominant site of gas exchange, osmoregulation, acid-base regulation, and excretion of nitrogenous waste. Physiol. Rev. 85, 97–177. doi: 10.1152/physrev.00050.2003
Ferguson, H. W. (2006). Systemic Pathology of Fish, A Text and Atlas of Normal Tissues in Teleosts and their Responses in Disease. London: Scotian Press.
Ferguson, H. W., Morrison, D., Ostland, V. E., Lumsden, J., and Byrne, P. (1992). Responses of mucus-producing cells in gill disease of rainbow trout (Oncorhynchus mykiss). J. Comp. Pathol. 106, 255–265. doi: 10.1016/0021-9975(92)90054-X
Gilmour, K. M., and Perry, S. F. (2018). Conflict and compromise: using reversible remodeling to manage competing physiological demands at the fish gill. Physiology 33, 412–422. doi: 10.1152/physiol.00031.2018
Gjessing, M. C., Steinum, T., Olsen, A.-B., Lie, K. I., Tavornpanich, S., Colquhoun, D. J., et al. (2019). Histopathological investigation of complex gill disease in sea farmed Atlantic salmon. PLoS One 14:e0222926. doi: 10.1371/journal.pone.0222926
Gonzalez, R. J. (2011). “The osmorespiratory compromise,” in Encyclopedia of Fish Physiology: From Genome to Environment, Vol. 2, eds A. P. Farrell, E. D. Stevens, J. J. Cech, and J. G. Richards (London: Academic Press), 1389–1394. doi: 10.1016/b978-0-12-374553-8.00211-2
Gonzalez, R. J., and McDonald, D. G. (2000). Ionoregulatory responses to temperature change in two species of freshwater fish. Fish. Physiol. Biochem. 22, 311–317.
Good, C., Davidson, J., Wiens, G. D., Welch, T. J., and Summerfelt, S. (2015). Flavobacterium branchiophilum and F. succinicans associated with bacterial gill disease in rainbow trout Oncorhynchus mykiss (Walbaum) in water recirculation aquaculture systems. J. Fish Dis. 38, 409–415.
Goss, G. G., and Perry, S. F. (1993). Physiological and morphological regulation of acid–base status during hypercapnia in rainbow trout (Oncorhynchus mykiss). Can. J. Zool. 71, 1673–1680. doi: 10.1139/z93-234
Goss, G. G., Perry, S. F., Wood, C. M., and Laurent, P. (1992). Mechanisms of ion and acid-base regulation at the gills of freshwater fish. J. Exp. Zool. 263, 143–159.
Green, L., and Jutfelt, F. (2014). Elevated carbon dioxide alters the plasma composition and behaviour of a shark. Biol. Lett. 10:20140538. doi: 10.1098/rsbl.2014.0538
Griffin, M. J., Pote, L. M., Camus, A. C., Mauel, M. J., Greenway, T. E., and Wise, D. J. (2009). Application of a real-time PCR assay for the detection of Henneguya ictaluri in commercial channel catfish ponds. Dis. Aquat. Organ. 86, 223–233. doi: 10.3354/dao02079
Gunnarsson, G. S., Karlsbakk, E., Blindheim, S., Plarre, H., Imsland, A. K., Handeland, S., et al. (2017). Temporal changes in infections with some pathogens associated with gill disease in farmed Atlantic salmon (Salmo salar L). Aquaculture 468, 126–134. doi: 10.1016/j.aquaculture.2016.10.011
Gunnarsson, S. (2006). The conceptualisation of health and disease in veterinary medicine. Acta Vet. Scand. 48:20. doi: 10.1186/1751-0147-48-20
Hayashi, M., Kikkawa, T., and Ishimatsu, A. (2013). Morphological changes in branchial mitochondria-rich cells of the teleost Paralichthys olivaceus as a potential indicator of CO2 impacts. Mar. Pollut. Bull. 73, 409–415. doi: 10.1016/j.marpolbul.2013.06.034
Heisler, N. (1990). Interaction between gas exchange, metabolism and ion transport in animals: an overview. Can. J. Zool. 67, 2923–2935. doi: 10.1139/z89-415
Heisler, N. (1993). “Acid-base regulation,” in The Physiology of Fishes, ed. D. Evans (Boca Raton, FL: CRC Press), 343–378.
Herrero, A., Thompson, K. D., Ashby, A., Rodger, H. D., and Dagleish, M. P. (2018). Complex gill disease: an emerging syndrome in farmed Atlantic Salmon (Salmo salar L.). J. Compar. Pathol. 163, 23–28. doi: 10.1016/j.jcpa.2018.07.004
Hess, S., Prescott, L. J., Hoey, A. S., McMahon, S. A., Wenger, A. S., and Rummer, J. L. (2017). Species-specific impacts of suspended sediments on gill structure and function in coral reef fishes. Proc. R. Soc. B 284:20171279. doi: 10.1098/rspb.2017.1279
Heuer, R. M., and Grosell, M. (2014). Physiological impacts of elevated carbon dioxide and ocean acidification on fish. Am. J. Physiol. 307, R1061–R1084.
Hilmarsen, Ø, Ambros Holte, E., Brendeløkken, H., Høyli, R., and Hognes, E. S. (2019). Konsekvense Av Landbasert Oppdrett Av Laks – Matfisk Og Post-Smolt. SINTEF Rapport OC2018 A-033.83. Trondheim: SINTEF.
Hoegh-Guldberg, O., Mumby, P. J., Hooten, A. J., Steneck, R. S., Greenfield, P., Gomez, E., et al. (2007). Coral reefs under rapid climate change and ocean acidification. Science 318:1737. doi: 10.1126/science.1152509
Holan, A. B., Good, C., and Powell, M. D. (2019). “Health management in RAS systems,” in Aquaculture Health Management, eds F. Kibenge and M. D. Powell (London: Elsevier).
Huth, T. J., and Place, S. P. (2016). Transcriptome wide analyses reveal a sustained cellular stress response in the gill tissue of Trematomus bernacchii after acclimation to multiple stressors. BMC Genomics 17:127. doi: 10.1186/s12864-016-2454-3
Iftikar, F. I., and Hickey, A. J. R. (2013). Do mitochondria limit hot fish hearts? Understanding the role of mitochondrial function with heat stress in Notolabrus celidotus. PLoS One 8:e64120. doi: 10.1371/journal.pone.0064120
Iva, D. Â, Antonio, F., and Beatriz, N. (1999). Epizoic amoebae from the gills of turbot Scophthalmus maximus. Dis. Aquat. Organ. 38, 33–38. doi: 10.3354/dao038033
Jeffrey, K., Stinton, N., and Ellis, T. (2011). A Review of the Land-Based, Warm-Water Recirculation Fish Farm Sector in England and Wales. FES220: Centre for Environment, Fisheries & Aquaculture Science. Available online at: http://www. fishfrom. com/index. php/fishfromdocuments/guidance/10-advantages-and-disadvantages/file
Jeffries, K., Hinch, S. G., Sierocinski, T., Pavlidis, P., and Miller, K. M. (2013). Transcriptomic responses to high water temperature in two species of Pacific salmon. Evol. Appl. 7, 286–300. doi: 10.1111/eva.12119
Kandalski, P. K., Dmengeon, M. R., Pedreiro de Souza, M. R. D., Herrerias, T., Machado, C., Zaleski, T., et al. (2018). Effects of short-term thermal stress on the plasma biochemical profiles of two Antarctic nototheniid species. Rev. Fish Biol. Fish. 28, 925–940. doi: 10.1007/s11160-018-9535-0
Khan, J. R., Pether, S., Bruce, M., Walker, S. P., and Herbert, N. A. (2014). Optimum temperatures for growth and feed conversion in cultured hapuku (Polyprion oxygeneios) – Is there a link to aerobic metabolic scope and final temperature preference? Aquaculture 430, 107–113. doi: 10.1016/j.aquaculture.2014.03.046
Kim, W. S., Kong, K. H., Kim, J. O., and Oh, M. J. (2016). Amoebic gill infection in coho salmon Oncorhynchus kisutch farmed in Korea. Dis. Aquat. Organ. 121, 75–78. doi: 10.3354/dao03037
Klinger, D. H., Levin, S. A., and Watson, J. R. (2017). The growth of finfish in global open-ocean aquaculture under climate change. Proc. R. Soc. B Biol. Sci. 284:20170834. doi: 10.1098/rspb.2017.0834
Koehn, J. D., Hobday, A. J., Pratchett, M. S., and Gillanders, B. M. (2011). Climate change and Australian marine and freshwater environments, fishes and fisheries: synthesis and options for adaptation. Mar. Freshw. Res. 62, 1148–1164. doi: 10.1071/MF11139
Kreiss, C. M., Michael, K., Lucassen, M., Jutfelt, F., Motyka, R., and Dupont, S. (2015). Ocean warming and acidification modulate energy budget and gill ion regulatory mechanisms in Atlantic cod (Gadus morhua). J. Comp. Physiol. B 185, 767–781. doi: 10.1007/s00360-015-0923-7
Lappivaara, J., Nikinmaa, M., and Tuurala, H. (1995). Arterial oxygen tension and the structure of the secondary lamellae of the gills in rainbow trout (Oncorhynchus mykiss) after acute exposure to zinc and during recovery. Aquat. Toxicol. 32, 321–331. doi: 10.1016/0166-445x(94)00097-a
Laurent, P., Maina, J. N., Bergman, H. L., Narahara, A., Walsh, P. J., and Wood, C. M. (1995). Gill structure of a fish from an alkaline lake: effect of short-term exposure to neutral conditions. Can. J. Zool. 73, 1170–1181. doi: 10.1139/z95-139
Laurent, P., and Perry, S. F. (1991). Environmental effects on fish gill morphology. Physiol. Zool. 64, 4–25. doi: 10.1086/physzool.64.1.30158511
LeBlanc, D. M., Wood, C. M., Fudge, D. S., and Wright, P. A. (2010). A fish out of water: gill and skin remodeling promotes osmo-and ionoregulation in the mangrove killifish Kryptolebias marmoratus. Physiol. Biochem. Zool. 83, 932–949. doi: 10.1086/656307
Leef, M. J., Harris, J. O., Hill, J., and Powell, M. D. (2005). Cardiovascular responses of three salmonid species affected with amoebic gill disease (AGD). J. Compar. Physiol. B 175, 523–532. doi: 10.1007/s00360-005-0020-4
Lefevre, S. (2016). Are global warming and ocean acidification conspiring against marine ectotherms? A meta-analysis of the respiratory effects of elevated temperature, High CO2, and their interaction. Cons. Physiol. 4:cow009. doi: 10.1093/conphys/cow009
Lefevre, S., McKenzie, D. J., and Nilsson, G. E. (2017). Model projecting the fate of fish populations under climate change need to be based on valid physiological mechanisms. Glob. Change Biol. 23, 3449–3459. doi: 10.1111/gcb.13652
Leung, T. L. F., and Bates, A. E. (2013). More rapid and severe disease outbreaks for aquaculture at the tropics: implications for food security. J. Appl. Ecol. 50, 215–222. doi: 10.1111/1365-2644.12017
Liu, Y., Ma, D., Xiao, Z., Xu, S., Wang, Y., Wang, Y., et al. (2015). Histological change and heat shock protein 70 expression in different tissues of Japanese flounder Paralichthys olivaceus in response to elevated temperature. Chinese J. Oceanol. Limnol. 33, 11–19. doi: 10.1007/s00343-015-4028-7
Lovy, J., Goodwin, A. E., Speare, D. J., Wadowska, D. W., and Wright, G. M. (2011). Histochemical and ultrastructural analysis of pathology and cell responses in gills of channel -catfish affected with proliferative gill disease. Dis. Aquat. Organ. 94, 125–134. doi: 10.3354/dao02322
Madeira, C., Madeira, D., Diniz, M. S., and Cabral, H. N. (2016). Thermal acclimation in clownfish: an integrated biomarker response and multi-tissue experimental approach. Ecol. Indic. 71, 280–292. doi: 10.1016/j.ecolind.2016.07.009
Madeira, D., Vinagre, C., and Diniz, M. S. (2016). Are fish in hot water? Effects of warming on oxidative stress metabolism in the commercial species Sparus aurata. Ecol. Indic. 63, 324–331. doi: 10.1016/j.ecolind.2015.12.008
Marcon, L., Lopes, D. S., Mounteer, A. H., Goulart, A. M. A., Leandro, M. V., and Dos Anjos Benjamin, L. (2016). Pathological and histometric analysis of the gills of female Hyphessobrycon eques (Teleostei: Characidae) exposed to different concentrations of the insecticide Dimilin®. Ecotoxicol. Environ. Saf. 131, 135–142. doi: 10.1016/j.ecoenv.2016.05.016
Marcos-López, M., Mitchell, S. O., and Rodger, H. D. (2016). Pathology and mortality associated with the mauve stinger jellyfish Pelagia noctiluca in farmed Atlantic salmon Salmo salar L. J. Fish Dis. 39, 111–115. doi: 10.1111/jfd.12267
Matey, V., Richards, J. G., Wang, Y., Wood, C. M., Rogers, J., Davies, R., et al. (2008). The effect of hypoxia on gill morphology and ionoregulatory status in the Lake Qinghai scaleless carp, Gymnocypris przewalskii. J. Exp. Biol. 211, 1063–1074. doi: 10.1242/jeb.010181
McCormick, S. D., and Regish, A. M. (2018). Effects of ocean acidification on salinity tolerance and seawater growth of Atlantic salmon Salmo salar smolts. J. Fish Biol. 93, 560–566. doi: 10.1111/jfb.13656
McDonald, D. G., Cavdek, V., and Ellis, R. (1991). Gill design in freshwater fishes: interrelationships amongst gas exchange, ion regulation and acid-base regulation. Physiol. Zool. 64, 103–123. doi: 10.1086/physzool.64.1.30158515
McDonald, D. G., Tang, Y., and Boutilier, R. G. (1989). Acid and ion transfer across the gills of fish: mechanisms and regulation. Can. J. Zool. 67, 3046–3054. doi: 10.1139/z89-428
Michael, K., Kreiss, C. M., Hu, M. Y., Koschnick, N., Bickmeyer, U., Dupont, S., et al. (2016). Adjustments of molecular key components of branchial ion and pH regulation in Atlantic cod (Gadus morhua) in response to ocean acidification and warming. Comp. Biochem. Physiol. 193B, 33–46. doi: 10.1016/j.cbpb.2015.12.006
Miraglia, M., Marvin, H. J. P., Kleter, G. A., Battilani, P., Brera, C., Coni, E., et al. (2009). Climate change and food safety: an emerging issue with special focus on Europe. Food Chem. Toxicol. 47, 1009–1021. doi: 10.1016/j.fct.2009.02.005
Mitchell, S., Baxter, E., Holland, C., and Rodger, H. D. (2012). Development of a novel histopathological gill scoring protocol for assessment of gill health during a longitudinal study in marine-farmed Atlantic salmon (Salmo salar). Aquac. Int. 20, 813–825. doi: 10.1007/s10499-012-9504-x
Mitchell, S. O., and Rodger, H. D. (2011). A review of infectious gill disease in marine salmonid fish. J. Fish Dis. 34, 411–432. doi: 10.1111/j.1365-2761.2011.01251.x
Moss, B., Kosten, S., Meerhoff, M., Battarbee, R. W., Jeppesen, E., Mazzeo, N., et al. (2011). Allied attack: climate change and eutrophication. Inland waters 1, 101–105. doi: 10.5268/IW-1.2.359
Mouton, A., Crosbie, P. B. B., Cadoret, K., and Nowak, B. F. (2013). First record of amoebic gill disease caused by Neoparamoeba perurans in South Africa. J. Fish Dis. 37, 407–409. doi: 10.1111/jfd.12133
Mueller, M. E., Sanchez, D. A., Bergman, H. L., McDonald, D. G., Rhem, R. G., and Wood, C. M. (1991). Nature and time course of acclimation to aluminum in juvenile brook trout (Salvelinus fontinalis). II. Gill histology. Can. J. Fish. Aquat. Sci. 48, 2016–2027. doi: 10.1139/f91-240
Munro, P. D., Barbour, A., and Birkbeck, T. H. (1994). Comparison of the gut bacterial-flora of start-feeding larval turbot reared under different conditions. J. Appl. Bacteriol. 77, 560–566. doi: 10.1111/j.1365-2672.1994.tb04402.x
Nilsson, G. E., and Lefevre, S. (2016). Physiological challenges to fishes in a warmer and acidified future. Physiology 31, 409–417. doi: 10.1152/physiol.00055.2015
Noga, E. (2010). “Fish Disease,” in Diagnosis and Treatment, Second Edn, ed. E. J. Noga (Iowa: Blackwell Publishing).
Norin, T., Malte, H., and Clark, T. D. (2014). Aerobic scope does not predict the performance of a tropical eurythermal fish at elevated temperatures. J. Exp. Biol. 217, 244–251. doi: 10.1242/jeb.089755
Oldham, T., Rodger, H., and Nowak, B. F. (2016). Incidence and distribution of amoebic gill disease (AGD) — An epidemiological review. Aquaculture 457, 35–42. doi: 10.1016/j.aquaculture.2016.02.013
O’Neil, J. M., Davis, T. W., Burford, M. A., and Gobler, C. J. (2012). The rise of harmful cyanobacteria blooms: the potential roles of eutrophication and climate change. Harmful Algae 14, 313–334. doi: 10.1016/j.hal.2011.10.027
Pandey, S., Parvez, S., Ansari, R. A., Ali, M., Kaur, M., Hayat, F., et al. (2008). Effects of exposure to multiple trace metals on biochemical, histological and ultrastructural features of gills of a freshwater fish, Channa punctata Bloch. Chem. Biol. Interact. 174, 183–192. doi: 10.1016/j.cbi.2008.05.014
Paulino, M. G., Sakuragui, M. M., and Fernandes, M. N. (2012). Effects of atrazine on the gill cells and ionic balance in a neotropical fish, Prochilodus lineatus. Chemosphere 86, 1–7. doi: 10.1016/j.chemosphere.2011.08.033
Pauly, D., and Cheung, W. W. L. (2017). Sound physiological knowledge and principles in modelling shrinking of fishes under climate change. Glob. Change Biol. 24, e743–e744.
Pauly, D., and Cheung, W. W. L. (2018). On confusing cause and effect in oxygen limitation of fish. Glob. Change Biol. 24, e15–e26.
Pereira, S., Pinto, A. L., Cortes, R., Fontaínhas-Fernandes, A., Coimbra, A. M., and Monteiro, S. M. (2013). Gill histopathological and oxidative stress evaluation in native fish captured in Portuguese northwestern rivers. Ecotoxicol. Environ. Saf. 90, 157–166. doi: 10.1016/j.ecoenv.2012.12.023
Perry, S. F., and Gilmour, K. M. (2002). Sensing and transfer of respiratory gases at the fish gill. J. Exp. Zool. 293, 249–263. doi: 10.1002/jez.10129
Pörtner, H. O., and Knust, R. (2007). Climate change affects marine fishes through the oxygen limitation of thermal tolerance. Science 315, 95–97. doi: 10.1126/science.1135471
Powell, M., Carson, J., and van Gelderen, R. (2004). Experimental induction of gill disease in Atlantic salmon (Salmo salar L.) with Tenacibaculum maritimum. Dis. Aquat. Organ. 61, 179–185. doi: 10.3354/dao061179
Powell, M. D., Harris, J. O., Carson, J., and Hill, J. V. (2005). Effects of gill abrasion and experimental infections with Tenacibaculum maritimum on the respiratory physiology of amoebic gill disease affected Atlantic salmon Salmo salar L. Dis. Aquat. Organ. 63, 169–174. doi: 10.3354/dao063169
Powell, M. D., Nowak, B. F., and Adams, M. B. (2002). Cardiac morphology in relation to amoebic gill disease history in Atlantic salmon, Salmo salar L. J. Fish Dis. 25, 209–215. doi: 10.1046/j.1365-2761.2002.00356.x
Powell, M. D., Yousaf, M. N., Rasmussen, K. J., Kollner, B., Zou, J., Secombes, C., et al. (2014). Immunohistochemical localization of inflammatory cells and cell cycle proteins in the gills of Loma salmonae infected rainbow trout (Oncorhynchus mykiss). Fish Shellf. Immunol. 40, 91–98. doi: 10.1016/j.fsi.2014.06.004
Purcell, J. E., Uye, S., and Lo, W. (2007). Anthropogenic causes of jellyfish blooms and their direct consequences for humans: a review. Mar. Ecol. Prog. Ser. 350, 153–174. doi: 10.3354/meps07093
Randall, D. J. (2011). “Excretion of ammonia,” in Encyclopedia of Fish Physiology. From Genome to Environment, ed. A. P. Farrell (London: Academic press), 1437–1443.
Randall, D. J., Wilson, J. M., Peng, K. W., Kok, T. W. K., Kuah, S. S. L., Chew, S. F., et al. (1999). The mudskipper, Periophthalmodon schlosseri actively secretes NH4+ against a concentration gradient. Am. J. Physiol. Regul. Integr. Comp. Physiol. 277, R1562–R1567.
Richardson, A. J., Bakun, A., Hays, G. C., and Gibbons, M. J. (2009). The jellyfish joyride: causes, consequences and management responses to a more gelatinous future. Trends Ecol. Evol. 24, 312–322. doi: 10.1016/j.tree.2009.01.010
Rind, K., Beyrend, D., Blondeau-Bidet, E., Charmantier, G., Cucchi, P., and Lignot, J.-H. (2017). Effects of different salinities on the osmoregulatory capacity of Mediterranean sticklebacks living in freshwater. J. Zool. 303, 270–280. doi: 10.1111/jzo.12491
Roberts, H. E., and Smith, S. A. (2011). Disorders of the respiratory system in pet and ornamental fish. Vet. Clin. North Am. Exotic Anim. Pract. 14, 179–206. doi: 10.1016/j.cvex.2011.03.004
Robertson, L. M., Kochhann, D., Bianchini, A., Matey, V., Almeida-Val, V. F., Val, A. L., et al. (2015). Gill paracellular permeability and the osmorespiratory compromise during exercise in the hypoxia-tolerant Amazonian oscar (Astronotus ocellatus). J. Comp. Physiol. B 185, 741–754. doi: 10.1007/s00360-015-0918-4
Rodger, H. D. (2016). “Fish disease causing economic impact in global aquaculture,” in Fish Vaccines, ed. A. Adams (Basel: Springer).
Rodger, H. D., Henry, L., and Mitchell, S. O. (2011). Non-infectious gill disorders of marine salmonid fish. Rev. Fish Biol. Fish. 21, 423–440. doi: 10.1007/s11160-010-9182-6
Rodgers, G. G., Rummer, J. L., Johnson, L. K., and McCormick, M. I. (2019). Impacts of increased ocean temperatures on a low-latitude coral reef fish – Processes related to oxygen uptake and delivery. J. Thermal Biol. 79, 95–102. doi: 10.1016/j.jtherbio.2018.12.008
Rojas, L.-M., Mata, C., Oliveros, A., and Salazar-Lugo, R. (2013). Histology of gill, liver and kidney in juvenile fish Colossoma macropomum exposed to three temperatures. Rev. Biol. Trop. 61, 797–806.
Rozas-Serri, M. (2019). Gill diseases in marine salmon aquaculture with an emphasis on amoebic gill disease. CAB Rev. Perspect. Agric. Vet. Sci. Nutr. Nat. Resour. 14, 1–15. doi: 10.1079/PAVSNNR201914032
Sakuragui, M. M., Sanches, J. R., and Fernandes, M. N. (2003). Gill chloride cell proliferation and respiratory responses to hypoxia of the neotropical erythrinid fish Hoplias malabaricus. J. Comp. Physiol. B 173, 309–317. doi: 10.1007/s00360-003-0337-9
Salvesen, I., Skjermo, J., and Vadstein, O. (1999). Growth of turbot (Scophthalmus maximus L.) during first feeding in relation to the proportion of r/k-strategists in the bacterial community of the rearing water. Aquaculture 175, 337–350.
Saraiva, A., Costa, J., Serrão, J., Eiras, J. C., and Cruz, C. (2015). Study of the gill health status of farmed sea bass (Dicentrarchus labrax L., 1758) using different tools. Aquaculture 441, 16–20. doi: 10.1016/j.aquaculture.2015.02.004
Schulte, P. M. (2015). The effects of temperature on aerobic metabolism: towards a mechanistic understanding of the responses of ectotherms to a changing environment. J. Exp. Biol. 218, 1856–1866.
Shi, L., Feng, L., Jiang, W. D., Liu, Y., Jiang, J., Wu, P., et al. (2015). Folic acid deficiency impairs the gill health status associated with the NF-kappaB, MLCK and Nrf2 signaling pathways in the gills of young grass carp (Ctenopharyngodon idella). Fish Shellfish Immunol. 47, 289–301. doi: 10.1016/j.fsi.2015.09.023
Shinn, A. P., Pratoomyot, J., Bron, J. E., Paladini, G., Brooker, E. E., and Brooker, A. J. (2015). Economic costs of protistan and metazoan parasites to global mariculture. Parasitology 142, 196–270. doi: 10.1017/S0031182014001437
Sollid, J., and Nilsson, G. E. (2006). Plasticity of respiratory structures – adaptive remodelling of fish gills induced by ambient oxygen and temperature. Resp. Physiol. Neurobiol. 154, 241–251.
Sollid, J., Weber, R. E., and Nilsson, G. E. (2005). Temperature alters the respiratory surface area of Crucian carp Carassius carassius and goldfish Carassius auratus. J. Exp. Biol. 208, 1109–1116.
Speare, D. J., and Ferguson, H. W. (2006). “Gills and pseudobranchs,” in Systemic Pathology of Fish, 2nd Edn, ed. H. W. Ferguson (Kentville: Scotian press), 24–63.
Strobel, A., Bennecke, S., Leo, E., Mintenbeck, K., Pörtner, H. O., and Mark, F. C. (2012). Metabolic shifts in the Antarctic fish Notothenia rossii in response to rising temperature and PCO2. Front. Zool. 9:28. doi: 10.1186/1742-9994-9-28
Sweidan, A. H., El-Bendary, N., Hegazy, O. M., Hassanien, A. E., and Snasel, V. (2015). Water pollution detection system based on fish gills as a biomarker. Proc. Comput. Sci. 65, 601–611. doi: 10.1016/j.procs.2015.09.004
Takata, R., Nakayama, C. L., e Silva, W. D. S., Bazzoli, N., and Luz, R. K. (2018). The effect of water temperature on muscle cellularity and gill tissue of larval and juvenile Lophiosilurus alexandri a Neotropical freshwater fish. J. Thermal Biol. 76, 80–88.
Tang, C. H., Leu, M. Y., Shao, K., Hwang, L. Y., and Chang, W. B. (2014). Short-term effects of thermal stress on the responses of branchial protein quality control and osmoregulation in a reef-associated fish, Chromis viridis. Zool. Studies. 53:21.
Taylor, R. S., Muller, W. J., Cook, M. T., Kube, P. D., and Elliott, N. G. (2009). Gill observations in Atlantic salmon (Salmo salar, L.) during repeated amoebic gill disease (AGD) field exposure and survival challenge. Aquaculture 290, 1–8. doi: 10.1016/j.aquaculture.2009.01.030
Teruo, M., Tadashi, I., and Hori, K. (2005). Histopathological and electron microscopy studies on sleepy disease of koi Cyprinus carpio koi in Japan. Dis. Aquat. Organ 65, 197–207.
Townhill, B. L., Tinker, J., Jones, M., Pitois, S., Creach, V., Simpson, S. D., et al. (2018). Harmful algal blooms and climate change: exploring future distribution changes. ICES J. Mar. Sci. 75, 1882–1893.
Tresguerres, M., and Hamilton, T. J. (2017). Acid-base physiology, neurobiology and behaviour in relation to CO2-induced ocean acidification. J. Exp. Biol. 220, 2136–2148.
Tvete, A. (2016). An Approach to Salmon Farming in Norway. A Future for Land Based Salmon Farming? Master’s thesis, Norwegian School of Economics, Bergen.
Uliano, E., Cataldi, M., Carella, F., Migliaccio, O., Iaccarino, D., and Agnisola, C. (2010). Effects of acute changes in salinity and temperature on routine metabolism and nitrogen excretion in gambusia (Gambusia affinis) and zebrafish (Danio rerio). Comp. Biochem. Physiol. A Mol. Integr. Physiol. 157, 283–290. doi: 10.1016/j.cbpa.2010.07.019
Valdenegro-Vega, V. A., Crosbie, P., Bridle, A., Leef, M., Wilson, R., and Nowak, B. F. (2014). Differentially expressed proteins in gill and skin mucus of Atlantic salmon (Salmo salar) affected by amoebic gill disease. Fish Shellfish Immunol. 40, 69–77. doi: 10.1016/j.fsi.2014.06.025
Verner-Jeffreys, D. W., Shields, R. J., and Birkbeck, T. H. (2003). Bacterial influences on Atlantic halibut Hippoglossus hippoglossus yolk-sac larval survival and start-feed response. Dis. Aquat. Orgs. 56, 105–113.
Vezzulli, L., Colwell, R. R., and Pruzzo, C. (2013). Ocean warming and spread of pathogenic vibrios in the aquatic environment. Microb. Ecol. 65, 817–825. doi: 10.1007/s00248-012-0163-2
Warrer-Hansen, I. (2015). Potential for Land Based Salmon Growout in Recirculating Aquaculture Systems (RAS) in Ireland. Netherlands: IFA Aquaculture.
Wiik-Nielsen, J., Gjessing, M., Solheim, H. T., Litlabo, A., Gjevre, A. G., Kristoffersen, A. B., et al. (2017). Ca. Branchiomonas cysticola, Ca. Piscichlamydia salmonis and Salmon Gill Pox Virus transmit horizontally in Atlantic salmon held in fresh water. J. Fish Dis. 40, 1387–1394. doi: 10.1111/jfd.12613
Wildgoose (2002). BSAVA Manual of Ornamental Fish. Glouscester: British Small Animal Veterinary Association.
Wilkie, M. P. (2002). Ammonia excretion and urea handling by fish gills: present understanding and future research challenges. J. Exp. Zool. 293, 284–301.
Wood, C. M. (1993). “Ammonia and urea metabolism and excretion,” in The Physiology of Fishes, ed. D. Evans (Boca Raton, FL: CRC Press), 379–425.
Wu, S. M., Shih, M. J., and Ho, Y. C. (2007). Toxicological stress response and cadmium distribution in hybrid tilapia (Oreochromis sp.) upon cadmium exposure. Comp. Biochem. Physiol. C Toxicol. Pharmacol. 145, 218–226.
Yao, C.-L., and Somero, G. N. (2014). The impact of ocean warming on marine organisms. Chinese Sci. Bull. 59, 468–479. doi: 10.1007/s11434-014-0113-0
Zarha, R. K., and Mobarak, Y. (2015). The effects of the pyrethroid pesticide cypermethrin on gills and kidneys (Trunk Mesonephroi) of guppy’s fish (Poecilia reticulata). Catrina Int. J. Environ. Sci. 11, 93–101.
Keywords: fish health, gill physiology, gill remodeling, fish physiology, gill plasticity, food security
Citation: Foyle KL, Hess S, Powell MD and Herbert NA (2020) What Is Gill Health and What Is Its Role in Marine Finfish Aquaculture in the Face of a Changing Climate? Front. Mar. Sci. 7:400. doi: 10.3389/fmars.2020.00400
Received: 06 February 2020; Accepted: 08 May 2020;
Published: 19 June 2020.
Edited by:
Youji Wang, Shanghai Ocean University, ChinaReviewed by:
Ahmet Regaib Oğuz, Yüzüncü Y ı l University, TurkeyBeaven Utete, Chinhoyi University of Technology, Zimbabwe
Jardel Nimet, State University of Maringá, Brazil
Copyright © 2020 Foyle, Hess, Powell and Herbert. This is an open-access article distributed under the terms of the Creative Commons Attribution License (CC BY). The use, distribution or reproduction in other forums is permitted, provided the original author(s) and the copyright owner(s) are credited and that the original publication in this journal is cited, in accordance with accepted academic practice. No use, distribution or reproduction is permitted which does not comply with these terms.
*Correspondence: Kevin L. Foyle, bGVvLmZveWxlQGpjdS5lZHUuYXU=