- 1Department of Biology, School of Sciences and Engineering, University of Crete, Heraklion, Greece
- 2Institute of Oceanography, Hellenic Centre for Marine Research, Heraklion, Greece
Excessive primary productivity due to nutrient inputs is a potential problem in coastal areas when resulting in high organic matter sedimentation rates. Microphytobenthos and heterotrophic bacteria are two components of the benthic ecosystem that contribute to nutrient cycling and decomposition of organic matter. In this context, the effects of nutrient addition and the associated in situ produced organic matter on microphytobenthos community composition and benthic bacterial viability were assessed in a mesocosm experiment for 58 days. The experimental setup included triplicate mesocosms filled with sediment and water under three levels of nutrient addition (“control,” “low,” and “high”). Benthic algal community composition was assessed using chemotaxonomy and bacterial viability was estimated using flow cytometry and a double-staining protocol. Multivariate analysis detected a significant effect of treatment and time on microphytobenthic community composition indicating a difference between control and low mesocosms and also between low and high treatments at Days 12 and 24 of the experiment. Nonetheless, microphytobenthos implied high resistance and redundancy of benthic algae to disturbance as all three treatments showed no significant difference in community structure between Days 0 and 58. Bacterial viability responded quickly to the high nutrient addition and was significantly lower than in the “control” and “low” treatments at Days 6 and 12. Both pelagic and benthic environmental variables were correlated to these changes in benthic community.
Introduction
Marine sediments represent perhaps the most complex habitats on Earth and also constitute an important element in marine biogeochemical cycles (Gray and Elliott, 2009). Benthic fauna, algae, and bacteria are only a few examples of taxa that live in marine sediments and perform different functions in this ecosystem, including degradation of organic matter, sediment ventilation, nitrification, etc. (Kristensen, 1988; Holmer and Kristensen, 1994; Nielsen et al., 2010; Lake and Brush, 2011; Quero et al., 2015). As part of the benthic ecosystem, microphytobenthos, the unicellular eukaryotic algae, and Cyanobacteria that grow on the sediments, represent a key component of the carbon budget accounting for more than 50% of the total primary production in coastal areas where the euphotic zone reaches the sediment surface (Cahoon, 1999). These communities can radically modify the sediment function (Baustian et al., 2011, 2013). For example, photosynthesis and nutrient assimilation by microphytobenthos have strong impacts on the mineralization pathways and the nutrient fluxes at the sediment interface (Larson and Sundbäck, 2008). In addition, microphytobenthos plays a significant role in the benthic food web. For example, biomass and the production of extracellular polymeric substances by these organisms represent an important source of fresh organic matter, which can fuel heterotrophic bacteria (Middelburg et al., 2000).
Heterotrophic bacteria also constitute an important element of the benthic ecosystem, contributing to nutrient cycling and the decomposition of organic matter (e.g., Danovaro et al., 1999). Despite the high abundance of benthic bacteria in sediments, the bacterial activity therein is often low, meaning that only a small proportion of bacteria are responsible for the bacterial productivity in the benthic environment (Haglund et al., 2003; Lamy et al., 2006). The bacterial biomass can contain a large fraction of dead cells or cells in a dormant and/or starvation-survival state and this fraction is not involved in bacterial productivity (Lamy et al., 2006).
In the past decades, the nutrient discharge into coastal areas has increased due to human activities (Coll et al., 2012; Karydis and Kitsiou, 2012). Nutrient inputs can become a problem when they result in excessive primary productivity that may cause eutrophication and high organic matter sedimentation rates (Edwards et al., 2003; Dimitriou et al., 2017b). With increasing eutrophication, pelagic production is favored and microphytobenthos is affected due to shading of the sediments (Meyer-Reil and Köster, 2000). Microphytobenthic community composition could also be altered as different algal groups, e.g., species resilient to hypoxic conditions, will take advantage of the conditions prevailing in the sediments (Cibic et al., 2019). Furthermore, eutrophication is correlated with bacterial abundance and activity but only until a certain critical point (Meyer-Reil and Köster, 2000). Further increases in organic matter are not paralleled by corresponding increases in microbial biomass and activity indicating that different states of eutrophication are characterized by certain relationships between organic carbon and microbial biomass and activity (Meyer-Reil and Köster, 2000; Lamy et al., 2006).
Although the impacts of nutrient and organic matter enrichment in the water column and sediment, respectively, have been studied extensively, a holistic consideration of benthic–pelagic coupling has been the subject of publications only in recent years (Ubertini et al., 2012; Dimitriou et al., 2015, 2017a). Benthic–pelagic coupling comprises all processes that link the benthic and pelagic zones through the exchange of energy, mass, and nutrients (Griffith et al., 2017). In marine ecosystems, sediment suspension due to physical processes or biological activities (bioturbation and bioirrigation) is responsible for erosion of the sediment, leading to sediment resuspension in the water column. In turn, pelagic processes such as phytoplankton primary productivity affect the organic matter content in benthic habitats through sedimentation.
The effects of nutrient input on pelagic productivity and sedimentation rate have been commonly studied in water column mesocosm experiments without sediment (Vidal and Duarte, 2000; Svensen et al., 2001). Adding sediment in mesocosm studies increases the realism of the experiments by including more biogeochemical cycling processes and more types of organisms, especially in the study of eutrophication that affects both the pelagic and benthic environments (Dimitriou et al., 2017a). The mesocosm setup containing both water column and sediment described by Dimitriou et al. (2017a) offered new opportunities for benthic-pelagic experiments. This setup acts as an intermediate link between large-scale sea mesocosms and smaller benthocosms, combining better control of the experimental manipulation and also allowing the study of the water column due to its larger volume compared to a small-scale benthocosm experiment.
The effects of nutrient addition and the associated in situ produced organic matter in different components of pelagic and benthic ecosystem were previously studied using the aforementioned mesocosm setup. This mesocosm experiment included the assessment of eukaryotic and prokaryotic plankton community changes (Santi et al., 2019), benthic macroinvertebrate community changes (Dimitriou et al., 2017c), and sediment geochemical response (Dimitriou et al., 2017b), providing useful insights into the subject. Specifically, the results of this mesocosm experiment indicated eukaryotic and prokaryotic plankton community changes due to nutrient addition (Santi et al., 2019) and an increase in pelagic primary productivity. This increase in primary productivity caused an increase in organic matter sedimentation rate and consequently, a delayed sediment geochemical response (Dimitriou et al., 2017b), i.e., increasing total organic carbon (TOC) concentration and hypoxic conditions which in turn, led to benthic macroinvertebrate community changes (Dimitriou et al., 2017c). In this context, the present study as part of the same mesocosm experiment aimed to supplement this knowledge by presenting the response of microphytobenthos and benthic bacterial viability to the eutrophication process described above. Specifically, the objectives were to test: (i) how different levels of nutrient addition to the water column could change the microphytobenthos community composition via changes in the organic matter sedimentation rates, (ii) the response time of microphytobenthos to different nutrient addition inputs, (iii) if benthic heterotrophic bacterial viability was affected by nutrient additions, and (iv) if these changes in microphytobenthos and bacterial viability were mainly driven by pelagic or benthic abiotic factors. Microphytobenthic community composition changes were assessed through changes in the relative abundance of major algal groups and also photosynthetic pigments, while bacterial viability was estimated as the change in the ratio of active to inactive bacterial abundance. It is hypothesized that both microphytobenthos and bacterial viability would respond to the nutrient addition in the water column and to the associated in situ produced organic matter since they are two ecosystem components that are involved in nutrient cycling and decomposition of organic matter.
Materials and Methods
Experimental Setup and Sampling Design
The present study was part of a large mesocosm experiment conducted in the framework of the “HYPOXIA” project (GSRT 5381). This project was focused on the effect of nutrient addition in the pelagic and benthic environment. The mesocosm experimental setup used took place at the CretaCosmos facilities of the Hellenic Centre for Marine Research (HCMR) in Crete. A detailed description of and information about the experimental setup are provided in Dimitriou et al. (2017a). Briefly, it included nine cylindrical mesocosms (0.7 m diameter) containing a water column of 4 m depth and 1.5 m3 total volume, and at the bottom, an undisturbed coastal sediment layer of 0.6 m diameter, 0.3 m height, and 85 l total volume. In order to induce eutrophic conditions representative of the eastern Mediterranean, two levels of nutrient addition (“low” and “high” treatment) were created intended to increase ambient N:P ratio (3.2) in the water column 1.5 and 2 times, for “low” and “high” treatment, respectively, with one single addition of dissolved nutrients (N and P) at the beginning of the experiment. Freshly prepared solutions of KH2PO4 and KNO3 at appropriate concentrations were used for the nutrient amendment. This sudden and extreme increase in nutrient concentrations attempts to simulate events such as severe precipitations and land floods leading to enormous riverine inputs in the sea (Madsen et al., 2014). The experiment also included triplicate control mesocosms with no nutrient addition. The water used in the experiment was pumped from the HCMR coastal area in Heraklion (35° 20′ 05.14″ N; 25° 16′ 50.44″ E) from 2 m depth and was immediately transferred into the mesocosms. The sediment that filled the mesocosms was collected from the port of Heraklion, Crete (35° 20′ 36.0″ N; 25° 08′ 11.6″ E). The sediment silt/clay content is 40% and OM content is 10%, housing a benthic community with an average of 25 (±3.4) different species (detailed information in Dimitriou et al., 2017a). Polychaeta was the major group found in the mesocosms, specifically, Paraonidae, Cirratulidae, and Maldanidae families.
The total duration of the experiment was 58 days (September–November). Sediment, sedimentation traps, and water samples were collected throughout the experiment at six time intervals, Day 0, Day 6, Day 12, Day 24, Day 44, and Day 58. No more than 10% of the total water or sediment volume was removed from each mesocosm during the entire duration of the experiment. Temperature and natural light illuminance have been monitored during the experiment.
Samples were collected with a corer sampler from the surface sediment (0–1 cm) at each time point and analyzed for bacterial viability and microphytobenthos community composition. Benthic environmental variables were also measured on the surface sediment (0–1 cm), including temperature, TOC and total organic nitrogen (TON) concentrations, redox potential (Eh), sulfide (S) concentration, and the refractory-to-labile organic matter ratio (ROM/LOM) as an indicator of the benthic organic matter degradation rate (Fodelianakis et al., 2016). Water column variables, namely dissolved oxygen (DO) and bottom water Chl-a concentration, were measured, as well as particulate organic carbon (POC flux) and nitrogen (PON flux) sedimentation rates. Sedimentation rates were calculated by accounting for the amount of POC and PON collected in the sediment traps at a specific time. Analytical protocols and methodologies used for the determination of the aforementioned environmental variables are described in Dimitriou et al. (2017a, b). Briefly, TOC and TON in sediment samples and POC and PON fluxes in water samples were determined by means of a Perkin Elmer 2400 CHN Elemental Analyzer (Hedges and Stern, 1984). Eh and S concentrations were measured using electrodes, as described in Wildish et al. (1999). The ROM/LOM was determined by measuring the percentage weight reduction after combustion for 16 h at 250 and 500°C of dried sediment samples (Loh et al., 2008). Chl-a measurements followed Yentsch and Menzel (1963) and DO was analyzed using the Winkler titration method.
Pigment Analysis
Microphytobenthic pigments were analyzed by means of high pressure liquid chromatography (HPLC). Photosynthetic pigments are commonly used for the quantitative chemotaxonomic analysis not only of phytoplankton functional groups (e.g., Wright et al., 1996; Lauridsen et al., 2011; Mendes et al., 2013) but also of microphytobenthos (e.g., Brotas and Plante-Cuny, 2003; Cibic et al., 2007a; Sañé et al., 2019). First, the benthic pigments were extracted from the sediment using the protocol described in Brotas and Plante-Cuny (2003). Approximately 1 g of freeze-dried sediment was mixed with 2–5 ml of methanol, buffered with 2% of ammonium acetate, and sonicated for 30 s at 30 W. The mixture was then incubated overnight at 4°C in the dark. The extract was filtered onto a Whatman GF/F filter and kept at −20°C prior to analysis. Filtered samples were injected into a Hewlett Packard 1100 Series HPLC system equipped with a column (C18 Gravity-SB, 4.6 × 250 mm, 5 μm particle size, Nucleodur, Macherey-Nagel, Germany) and a diode array detector. The injected volume was 50 μl and the flow rate was 0.6 ml min–1 for 35 min. Pigments were separated by applying the analytical gradient of Brotas and Plante-Cuny (2003). The identification of individual pigments was conducted as a combination of retention time and absorption spectra in relation to literature reports (Yacobi et al., 1990; Wright et al., 1991; Borrego and Garcia-Gil, 1994; Louda et al., 2000; Zapata et al., 2000; Brotas and Plante-Cuny, 2003). The chromatogram peaks were quantified by integration of the 430 nm trace and comparison to a calibration curve of commercial standards, namely chlorophylls a and b, fucoxanthin, diadinoxanthin, diatoxanthin, zeaxanthin, and lutein (DHI, Hørsholm, Denmark).
Pigment data were further processed with the CHEMTAX software (Mackey et al., 1996) in order to calculate the relative contribution of major benthic algae groups namely, Chlorophytes, Diatoms, Cyanobacteria, and Euglenophytes, to total microphytobenthic biomass. A pigment:Chl-a ratio matrix was defined based on literature data for microphytobenthos (Brotas and Plante-Cuny, 2003; Brotas et al., 2007). For optimization, 60 pigment ratio matrices were generated by adjusting each of the pigment ratios according to a random function (Wright et al., 2009). The best 10% of the outputs, based on lower root mean square (RMS) errors, were selected as starting matrices to determine microphytobenthic community change during the experiment.
Bacterial Viability
In order to estimate the number of live vs. dead benthic bacteria, a double-staining protocol based on the simultaneous use of two stains targeting the nucleic acid was used (Falcioni et al., 2008). The membrane integrity of bacterial cells was used as an indicator of cell viability, and the discrimination between live and dead cells was based on the different staining characteristics of two nucleic acid dyes being used simultaneously: SYBR Green I (SGI), being a membrane permeable dye, stains all cells (both live and dead), and propidium iodide (PI), a membrane impermeant dye, stains only cells with compromised membranes and considered dead.
Sediment samples were collected and processed immediately without prior preservation. Portions of 0.5 g of sediment were transferred to glass vials and mixed with 4.5 ml of filter-sterilized (0.2 μm) water (Haglund et al., 2003). A combination of mechanical treatment, including vigorous and manual shaking, and sonication for 1 min (30 W), were applied to the mixture. A 2.5 ml subsample was mixed with 2.5 ml of filter-sterilized (0.2 μm) water and further diluted 200 times. A 1:1 mixture of SGI and PI was added (50 μl) and the sample was incubated in the dark for 15 min. Cell counts were performed in a FACSCalibur flow cytometer (Becton Dickinson) equipped with an air-cooled laser at 488 nm and standard filters. One-μm-diameter fluorescent beads (Polysciences) were added to the stained sample immediately before analysis as an internal standard. Bacterial abundance was then calculated using the acquired cell counts and the respective flow rate. All flow cytometry data were gathered and processed with the Cell Quest Pro software. During the experiment, viable vs non-viable cells were clearly separated in the flow cytometric dots. Autotrophic bacteria were also separated, due to their different characteristics in red fluorescence from Chl-a, and excluded from the analysis. Hereafter, bacterial viability is referred to the live to dead bacterial abundance ratio.
Statistical Analysis
Changes in microphytobenthic pigments, bacterial viability and live bacterial abundance were tested in different treatments (control, low, and high) and at different times (Days 0 to 58). All variables were screened on whether they met the assumptions of normality (Shapiro–Wilk test) and homogeneity of variance (Levene’s test) while outliers were assessed using studentized residuals. Variability among treatments, temporal variability and the possible interaction between treatment and time were assessed by two-way repeated measures ANOVA (RM-ANOVA) using IBM SPSS version 23. In cases of significant treatment and time interaction, simple effects were assessed (variance between treatments at each level of time and variance over time for every treatment). Pairwise comparisons were performed using a post hoc test with the Bonferroni correction.
In order to evaluate changes in microphytobenthic community composition, permutational multivariate analysis (PERMANOVA) was performed on microphytobenthic groups derived by CHEMTAX, using Bray–Curtis similarity on log-transformed data (Clarke et al., 2014). The factors “treatment,” “time” were used as fixed factors and replicated mesocosms nested in “treatment” as random factor and permutation of residuals under a reduced model and 9,999 permutations were applied. Monte Carlo p-values were also considered for the significance of differences among factors. For these multivariate analyses, the Primer 7 statistical package was used (Clarke and Gorley, 2015).
To further detect the response levels of total benthic community (microphytobenthos and live bacteria) to nutrient input, a principal component analysis (PCA) was performed on standardized data by subtracting the mean and dividing by the standard deviation. Selected biotic variables including microphytobenthic taxa relative abundance and live bacteria relative abundance were used as independent variables in PCA, while environmental variables (TOC, TON, Eh, S, ROM/LOM, DO, POC and PON fluxes, and bottom water Chl-a) were added as supplementary variables in order to see how they were correlated with the biotic community. Specifically, PCA plot was done using the independent variables and the supplementary variables were projected onto this plot on the basis of their correlations to the ordination axes. Prior to this analysis, significant correlations among environmental variables were determined and variables strongly correlated (Spearman correlation, r > 0.9) to others were excluded from the analysis. Specifically, PON flux and bottom water Chl-a were excluded from the analysis due to correlation with POC flux, and also S due to correlation with Eh. PCA was performed in CANOCO 5 software (ter Braak and Smilauer, 2012).
Results
Pigment Analysis
Univariate analysis of benthic pigment concentration detected significant differences only for the factor “time” (Table 1). After nutrient addition, pigments decreased in both “low” and “high” mesocosms, followed by an increase at Days 12 and 24 when maximum pigment concentrations were recorded (Figure 1). This trend was also detected in the control mesocosms for most of the pigments, except for diatoxanthin and zeaxanthin pointing out the reason for the non-significant difference among treatments. Chl-b followed a different pattern of change throughout the experiment. Specifically, Chl-b concentration declined in the “control” and “low” mesocosms over time but showed an increase in the “high” treatment after Day 12, reaching maximum concentration at the end of the experiment. Nevertheless, these differences between treatments were statistically insignificant (Table 1).
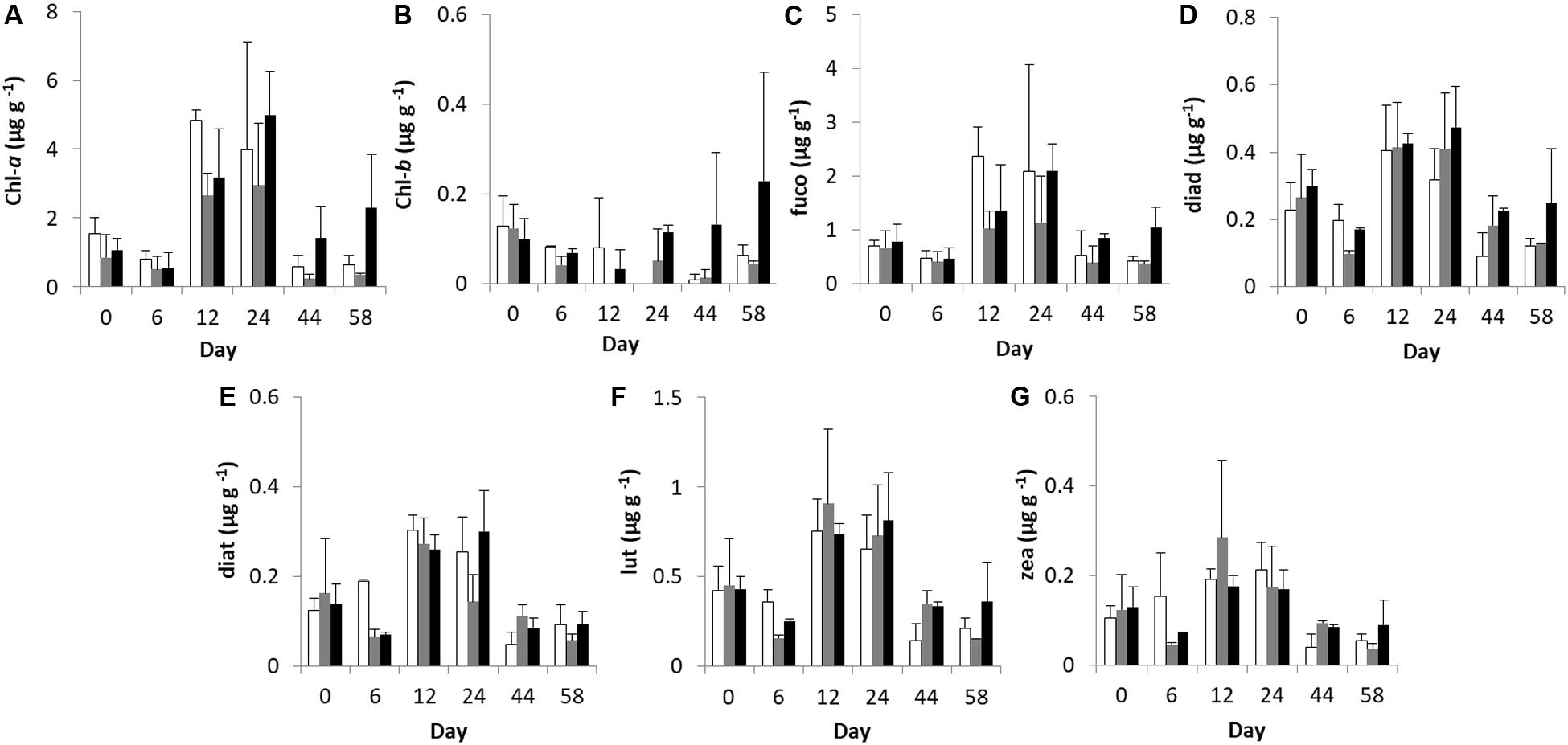
Figure 1. Temporal changes in microphytobenthic (A) Chlorophyll-a, (B) Chlorophyll-b, (C) fucoxanthin, (D) diadinoxanthin, (E) diatoxanthin, (F) lutein, and (G) zeaxanthin concentrations (μg g–1 of dry sediment) during the experiment in different treatments. In each diagram, white bars represent control values, gray bars represent “low” treatment and black bars represent “high” treatment. Error bars indicate standard deviation.
CHEMTAX Analysis of Microphytobenthic Groups
The set of pigments detected on the sediment samples were used as diagnostic pigments for different microphytobenthic groups. CHEMTAX analysis distinguished the relative contribution of four taxonomic groups – Chlorophytes, Diatoms, Cyanobacteria, and Euglenophytes – to total benthic Chl-a concentration. PERMANOVA detected a significant effect of “time” and a significant effect of “treatment” on microphytobenthic community composition (Table 2). No significant interaction effect of “time” and “treatment” was detected. The “low” nutrient addition treatment was significantly different from the control treatment. In addition, a significant difference between the “low” and “high” treatment was recorded indicating that community composition in these mesocosms were affected by the nutrient addition levels (low and high) during the experiment. In addition, significant changes in microphytobenthic community composition were detected in all treatments at Days 12 and 24. Specifically, Chlorophytes was the major algal group detected in all mesocosms at the beginning of the experiment followed by Diatoms, Euglenophytes, and Cyanobacteria which had the lowest contribution to total Chl-a. In the “low” treatment, nutrient addition initially caused an increase in Chlorophytes and Cyanobacteria but this was replaced later, after Day 24, by an increase in Diatoms and Euglenophytes (Figure 2). In the “high” treatment, an increase in Cyanobacteria was detected after Day 12 and continued until the end of the experiment (Figure 2).
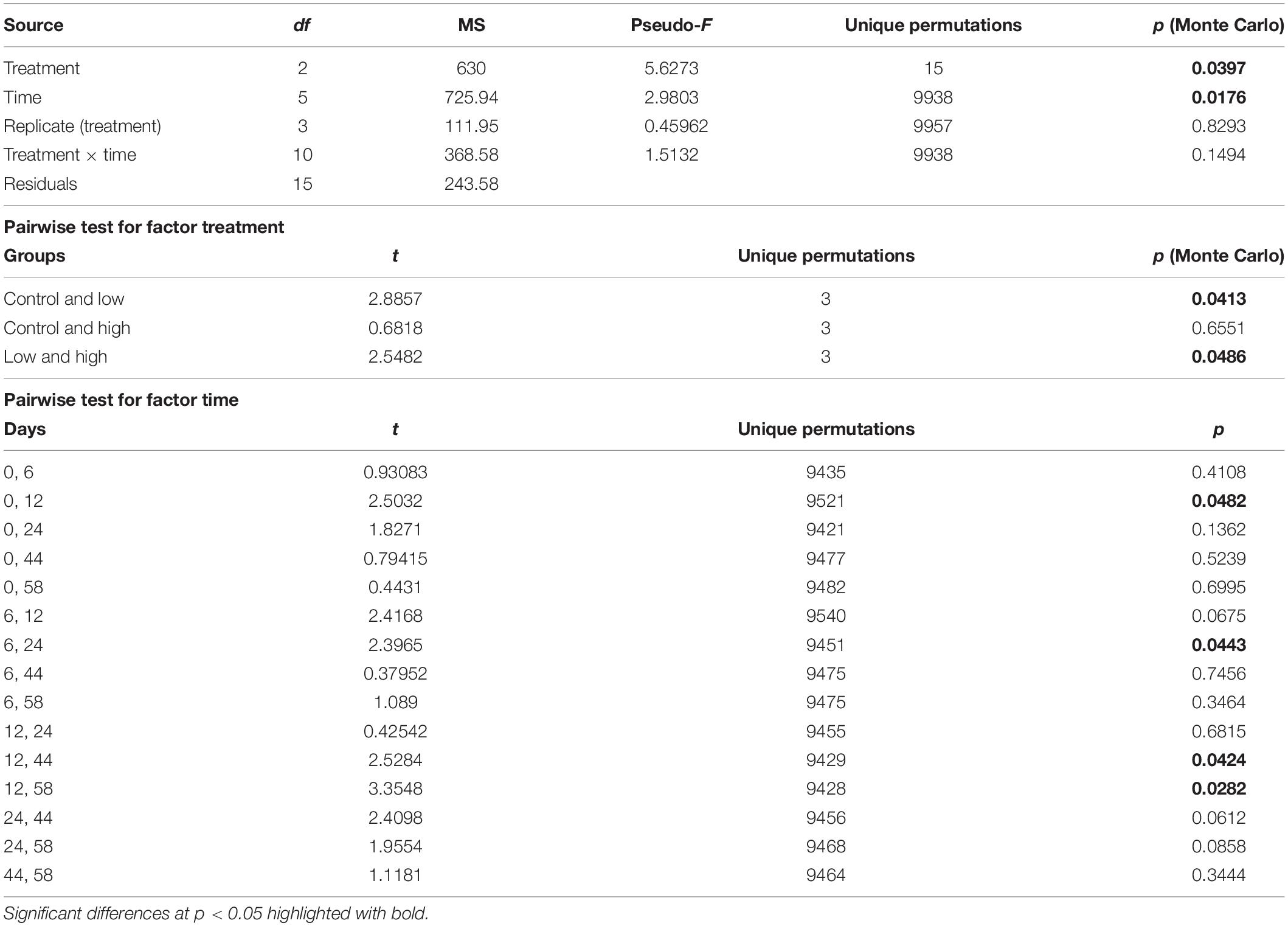
Table 2. Results of PERMANOVA and the resultant pairwise tests indicating differences in the microphytobenthic community across “treatment” and “time.”
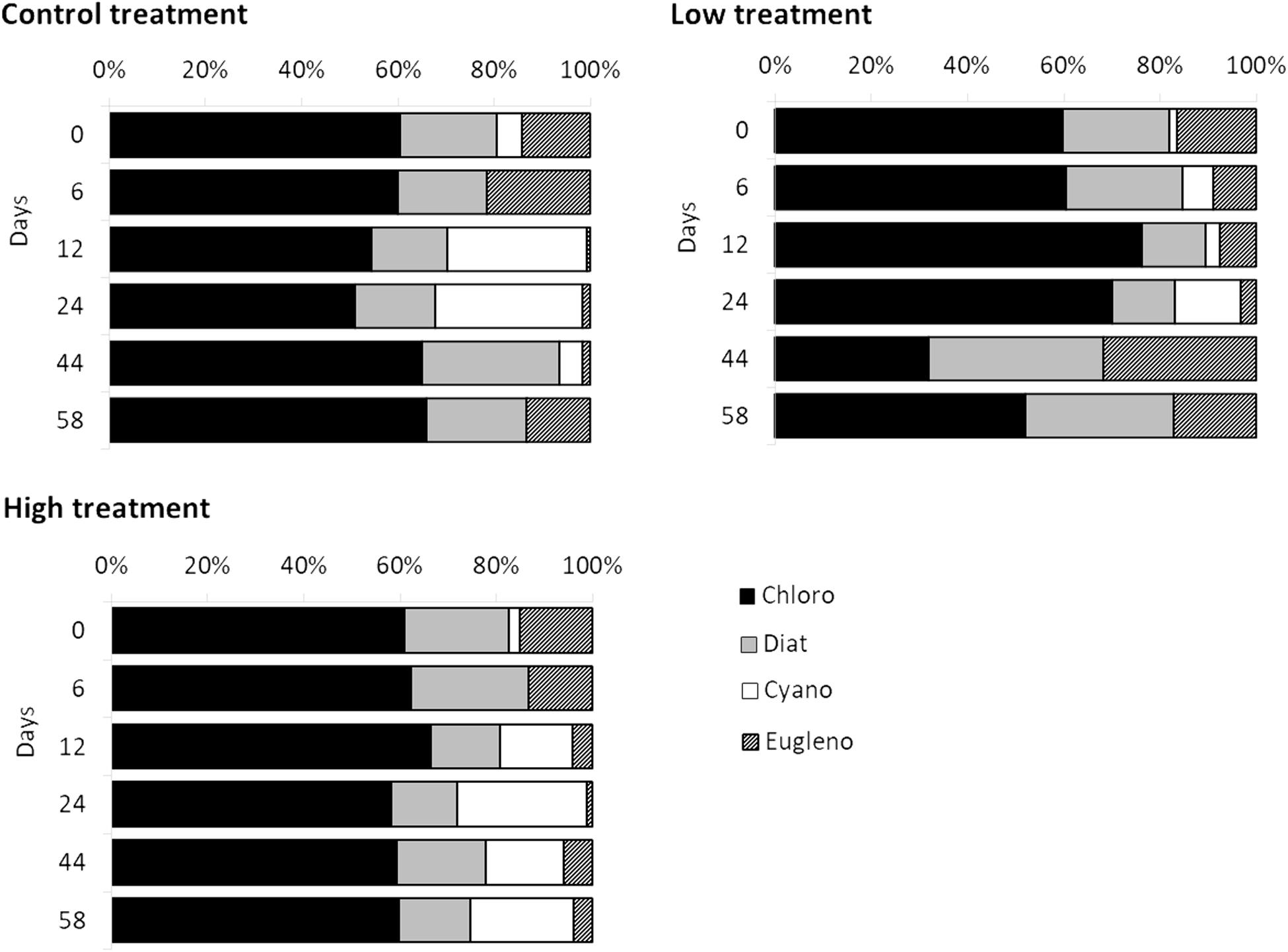
Figure 2. Temporal changes recorded in relative contribution of Chlorophytes (black bars), Diatoms (gray bars), Cyanobacteria (white bars), and Euglenophytes (diagonal patterned bars) to total Chl-a during the experiment in different treatments.
Bacterial Viability
Live bacterial abundance on the surface sediment changed in all mesocosms following a relatively similar trend among treatments (Figure 3). Only the factor “time” caused significant changes in the live bacterial abundance (RM-ANOVA; live bacteria: F = 6.169; p = 0.003) and post-hoc Bonferroni t-test separated the live bacterial abundance at Day 24 from Days 12 to 44. However, there was an interaction in the factors “time” and “treatment” (RM-ANOVA; bacterial viability: F = 7.619; p < 0.001) for the bacterial viability measurements and post-hoc comparisons indicated that bacterial viability was significantly lower in the “high” treatment than in the “control” and “low” treatments at Days 6 and 12 showing a significant response to the high nutrient addition effect.
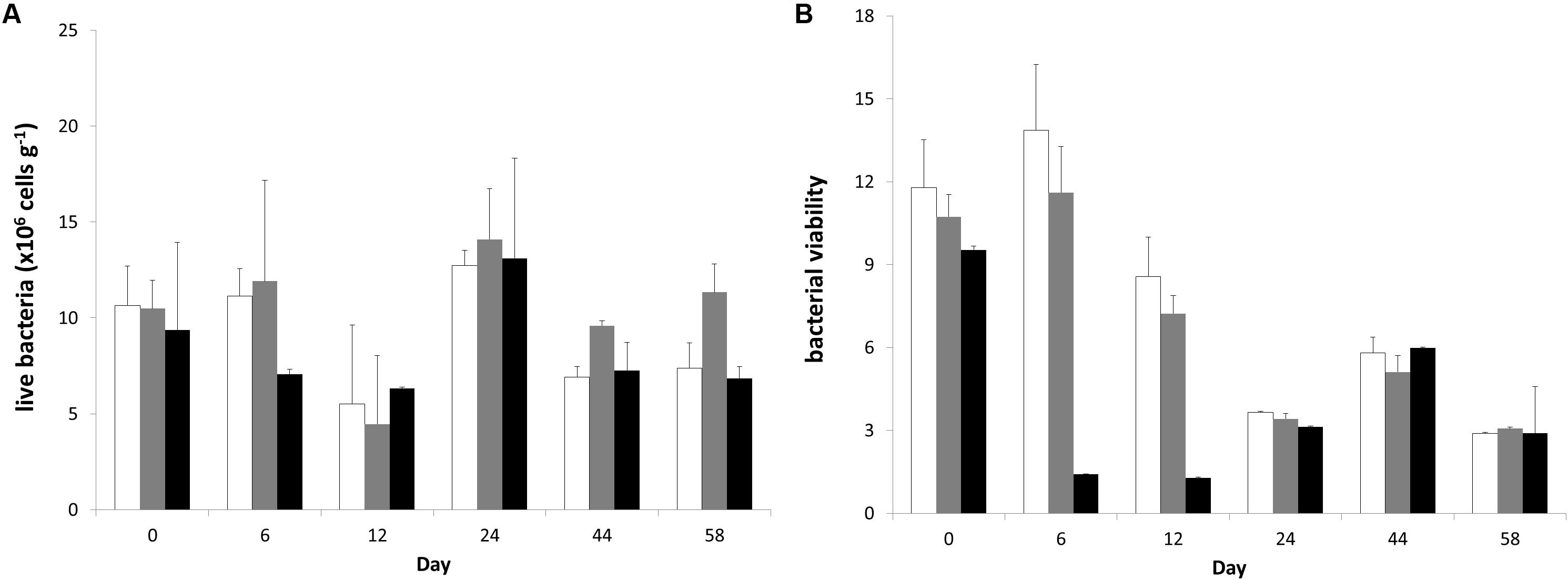
Figure 3. Temporal changes (A) live bacterial abundance and (B) bacterial viability during the experiment in different treatments. In each diagram, white bars represent control values, gray bars represent “low” treatment and black bars represent “high” treatment. Error bars indicate standard deviation.
Effect of Abiotic Variables on Benthic Bacterial Viability and Microphytobenthos
To better integrate all the considered variables in a comprehensive manner, a PCA was applied to the benthic community (microphytobenthos and live bacteria) data using abiotic variables as supplementary variables (Figure 4). In the PCA plot, the first two principal components explained 52.88 and 29.03% of the total variance of the benthic community, respectively. In general, samples from different treatments were clustered together until Day 6 when “high” treatment samples were clustered in the upper left quadrant, separate from “low” treatment samples along both axes.
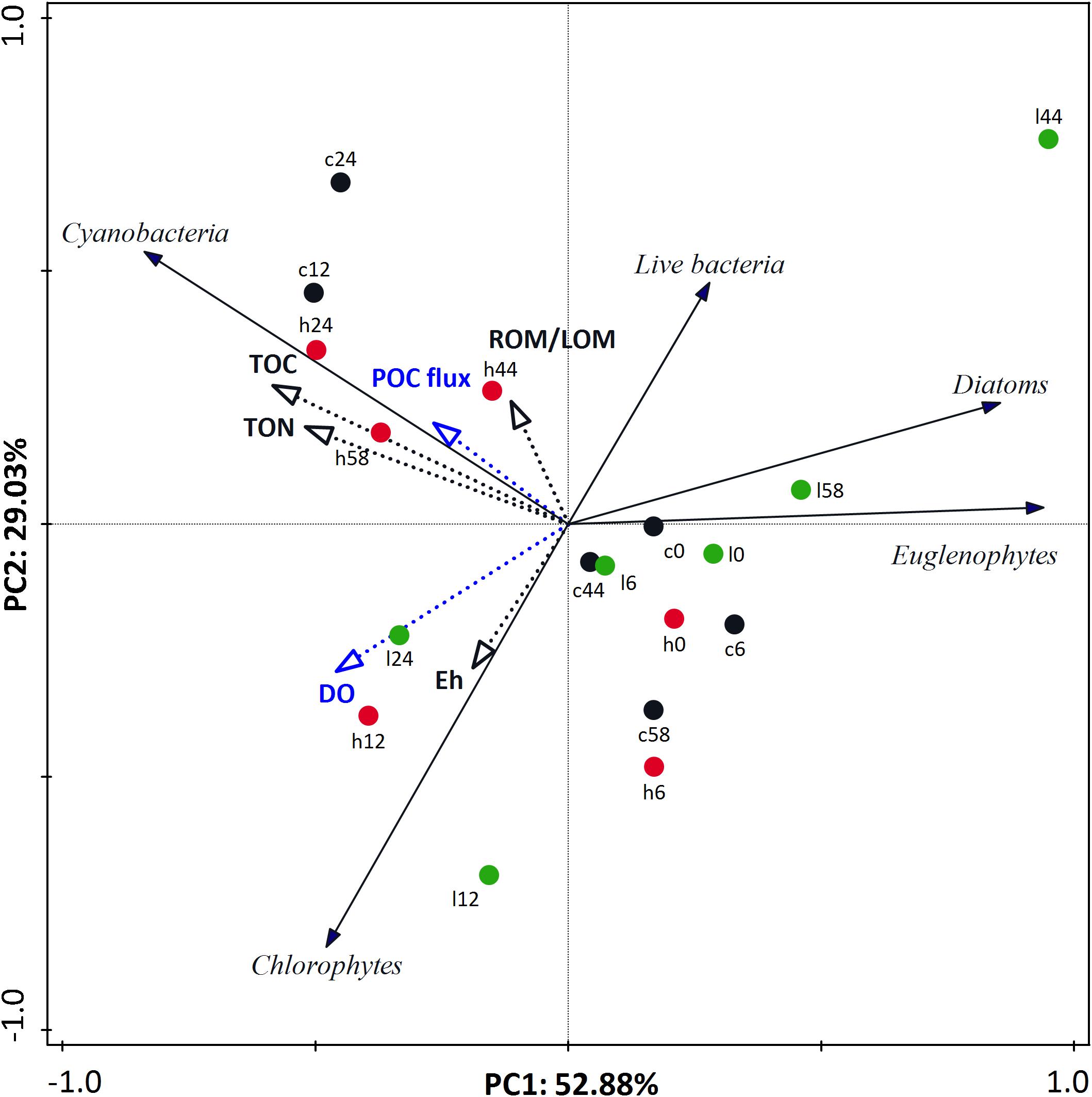
Figure 4. Principal component analysis (PCA) ordination tri-plot for benthic community data with environmental variables (black and blue dotted-line vectors) and biotic variables (black line vectors) represented as vectors. Each vector points in the direction of the steepest increase of values. Black, green, and red circles indicate “control,” “low,” and “high” treatment, respectively. Digits reflect experimental days.
Supplementary variables explained 44.42% of the total variance of benthic community. Abiotic variables best correlated to the ordination axes were Eh, sediment TOC and TON concentrations, and ROM/LOM but also variables that describe water column characteristics such as DO and POC sedimentation rate (Supplementary Table S1). The trends of these environmental variables during the experiment were described and interpreted in Dimitriou et al. (2017b). Briefly, among the sediment variables, Eh was significantly different among treatment safter Day 24, while TOC, TON, and ROM/LOM differed among treatments from Day 12 onward. DO was significantl ydifferent among treatments from Days 9 to 51 and POC sedimentation rate was significantly different in all treatments after Day 12 (Dimitriou et al., 2017b). It is also noticeable that different microphytobenthic groups correlated with different abiotic factors (Supplementary Table S2). In detail, the Cyanobacteria that characterized the “high” treatment were positively correlated with POC flux from the water column and also sediment TOC and TON. On the other hand, a relative abundance of Chlorophytes was correlated with water column DO and Eh as well while Diatoms and Euglenophytes were negatively correlated with almost every abiotic variable and took advantage of the hypoxic conditions that prevailed on the mesocosms. Finally, live bacteria was positively correlated with ROM/LOM and was higher in “low” nutrient addition mesocosms and negatively correlated with DO and Eh.
Discussion
The present study was part of a mesocosm experiment focused on the nutrient addition impact on several pelagic and benthic components, and on the benthic–pelagic coupling processes. Specifically, we assessed the effects of eutrophication on microphytobenthic community composition and benthic heterotrophic bacterial viability. This is the first study in which these two ecosystem components (benthic prokaryotes and microphytobenthos) have been investigated simultaneously on a relatively large in size experimental setup but under controlled conditions. The results indicated that both microphytobenthos community composition and benthic bacterial viability changed during the experiment under conditions of organic enrichment induced by nutrient addition in the water column and by the consequent increase in organic matter sedimentation from the pelagic zone. Specifically, microphytobenthic community composition fluctuated during the experiment but returned to the initial composition, while bacterial viability was only affected by high nutrient inputs.
In order to better interpret the results of this study, it should be pointed out that Santi et al. (2019), studying the effects of nutrient addition on prokaryotic and eukaryotic microbial phytoplankton communities in the same experiment, observed an increase in pelagic primary productivity and discovered two blooms per treatment, on Day 3 at both treatments; and on Day 9 for “low” and Day 12 for “high” treatment, respectively. Furthermore, Dimitriou et al. (2017b), also in the same mesocosm experiment, concluded that eutrophication affected sediment geochemical variables through increasing sedimentation of organic matter, resulting in increasing TOC concentration and hypoxic conditions (negative Eh values) in the benthic ecosystem; however, in both “low” and “high” treatments there was a time lag between pelagic trophic status and the response of the sediment geochemistry.
Microphytobenthic communities are subject to local environmental conditions that define their composition, such as nutrient and oxygen supply (Cibic et al., 2007b; Baustian et al., 2011). Since there was a change in benthic geochemical variables due to different levels of nutrient addition in the water column (Dimitriou et al., 2017b), it was expected that these changes would also affect microphytobenthos. In general, pigments that were quantified during this study were indicative of Diatoms, Cyanobacteria, Chlorophytes, and Euglenophytes (Riaux-Gobin et al., 1987; Klein and Riaux-Gobin, 1991; Bianchi et al., 1993; Brotas and Plante-Cuny, 1998, 2003) and their ratios to Chl-a were indicative of the relative contribution of different algal taxa to total Chl-a concentration (Mackey et al., 1996). During the experiment, changes in pigment concentrations indicated a significant shift in the relative abundance of dominant algal taxa that could cause changes in ecosystem functions. Many studies have determined the effects of organic enrichment and hypoxic conditions on microphytobenthos and most of them agreed that benthic phototrophic communities not only depend on environmental conditions but also affect them (Larson and Sundbäck, 2008; Cibic et al., 2019; Tsikopoulou et al., 2019). In our study, low and high nutrient inputs caused different responses in the microphytobenthic community composition. Specifically, in the “low” treatment, the initial increase in Chlorophytes and Cyanobacteria relative abundance was replaced by an increase in Diatoms and Euglenophytes after Day 24, while in the extreme nutrient disturbance, the increasing abundance of Cyanobacteria characterized the microphytobenthic community from Day 12 until the end of the experiment. It is noteworthy that most of these changes were observed right after the phytoplankton blooms reported in Santi et al. (2019) and could be the result of the sedimentation of organic matter indicating benthic–pelagic coupling (Dimitriou et al., 2017b).
Regardless of the aforementioned changes, microphytobenthic community maintained its initial composition at the end of the experiment and no significant changes between Days 0 and 58 were recorded for any treatment, indicating that the microphytobenthic community was resilient to disturbance during this study, as observed also in previous studies (Larson and Sundbäck, 2008; Franzo et al., 2014). Mechanisms influencing microphytobenthic community composition include nutrient availability, grazing and also competition among species (Sigmon and Cahoon, 1997; Underwood and Barnett, 2006; Cibic et al., 2007a; Komárek and Johansen, 2015). Diatoms, Cyanobacteria, and Euglenophytes all include organisms resistant to anoxic conditions, high levels of organic enrichment and r-selected life strategy species that could potentially benefit from extreme conditions. For example, Cibic et al. (2019) linked the high abundance of Cyanobacteria to high water column loadings in four lagoons in the Po River Delta system, and suggested that severe pollution led to the dominance of non-diatom species in microphytobenthic community. In agreement to this, our results implied that the environmental conditions prevailing in each treatment during the experiment favored different taxonomic groups of benthic algae, for example, Cyanobacteria after Day 12 in the most extreme disturbance (“high” mesocosms).
Even though there were changes in benthic community composition (microphytobenthos and live bacteria) during the experiment in all mesocosms, “control” and “low” treatments seemed to return to their initial conditions at the end of the experiment. In contrast, in the “high” nutrient addition mesocosms, the initial community differed from the final one, following the pattern recorded previously in sediment geochemical variables (Dimitriou et al., 2017b), in which it was observed that only the “high” treatment did not recover during the experiment, while the “low” treatment showed signs of recovery at the end of the experiment.
Among the environmental variables used to explain the benthic microbial community during the experiment, six abiotic variables that characterized both the sediment and the water column were best correlated to community composition changes. It is noteworthy that these variables were indicative of eutrophic conditions and hypoxia. POC fluxes from the water column and also sediment TOC and TON were positively correlated with the increase in Cyanobacteria relative abundance in the “high” treatment. On the other hand, the relative abundance of Chlorophytes was favored by water column DO and Eh, as well. These two findings agreed with the results of previous studies that suggest that Cyanobacteria and Chlorophytes are influenced by water column nutrient loadings (Baustian et al., 2011; Cibic et al., 2019). The presence of benthic Cyanobacteria under the extreme nutrient disturbance is indicative of their ability to survive in varying environments (Baustian et al., 2011; Tsikopoulou et al., 2019). Diatoms and Euglenophytes detected at high abundances after Day 24 in “low” treatment were negatively correlated with benthic organic enrichment and also took advantage of the hypoxic conditions along with moderate nutrient inputs prevailing in the “low” mesocosms. Although, microphytobenthos displays a significant correlation with nutrient concentrations (Facca et al., 2002), benthic Diatoms show high resistance to fluctuating environments. Specifically, they can cope with organic enrichment (Franzo et al., 2014), anoxic conditions (Larson and Sundbäck, 2008), and other pollutants (Potapova et al., 2016) since they include diverse assemblages and occur in all types of aquatic environments (Weckstrom and Juggins, 2005; Cibic and Blasutto, 2011; Cibic et al., 2012). In addition, Euglenophyte species were also found to correlate with sediment characteristics, especially water content and organic matter (Scholz and Liebezeit, 2012).
Benthic heterotrophic bacteria responded faster to the nutrient addition in the water column than microphytobenthos. Many studies have suggested that a variable yet highly productive portion of bacteria is responsible for the remineralization of the phytoplankton-derived material, particularly during the post-bloom conditions (Lamy et al., 2006; Rauch et al., 2008). On the contrary, our study indicated that the abrupt increase in nutrient concentrations caused a simultaneous decrease in bacterial viability, which was significant in the case of “high” treatment. The higher bacterial viability found in “low” nutrient addition mesocosms is indicative of different mechanisms controlling the microbial community. Specifically, in the “high” nutrient addition treatment, the biogeochemical processes may have been shifted from a microbially driven functioning to a more faunistic one where microbial density was mainly controlled by bacterivore species. Lamy et al. (2006) suggested that live bacterial abundance were mainly controlled by predation rather than the organic matter supply. Our hypothesis was also supported by the results of Dimitriou et al. (2017c) during our mesocosm experiment which indicated that in high nutrient addition, the increased availability of organic matter in the sediment caused differences in macrofaunal community structure compared to the “control” and “low” treatments by favoring deposit-feeding species with high bioturbation ability. The bioturbation potential of the new community contributed to the oxygenation of the sediment within “high” mesocosms, preventing the creation of hypoxic conditions in the sediment.
The organic matter dynamics over time could be indicative of a change in ecosystem functioning (Fodelianakis et al., 2016). In a well-functioning benthic bacterial community, LOM is degraded more rapidly than ROM because it is more bioavailable. In this study, bacterial viability was lower in “high” nutrient addition treatments and live bacterial abundance among others was also correlated with ROM/LOM and DO. This suggests that in the “high” nutrient mesocosms the microbial community consumes the labile organic matter slower than the addition of organic matter through sedimentation from the water column. Moreover, it indicates differences in the microbial community responses under moderate and extreme nutrient disturbance and supports the aforementioned results which suggest that the degradation of organic matter was mostly performed by bacteria in “low” treatment and by eukaryotic organisms in “high” treatment, as also proposed by Furukawa et al. (2004).
Conclusion
During a nutrient addition mesocosm experiment that included water column and sediment, microphytobenthos, and bacterial viability responded quickly to the nutrient addition, indicating a significant level of benthic–pelagic coupling since both benthic and pelagic variables affected the benthic community composition. The changes recorded in microphytobenthos implied a high resistance and redundancy of benthic algae to disturbance. This plasticity provided mechanisms to imply a rapid restoration of the benthic community at least in moderate nutrient inputs. In case of high disturbance due to nutrient addition, bacterial viability decreased indicating that microbial density was mainly controlled by bacterivore species and microbial degradation of precipitated organic matter was probably replaced by a more faunistic one.
Data Availability Statement
The datasets generated for this study are available on request to the corresponding author.
Author Contributions
IK, PP, PD, and NP designed the mesocosm experiment. IT, IS, PD, and NP performed geochemical analyses. IS performed flow cytometer analyses. IT wrote the first version of the manuscript, performed microphytobenthic and benthic bacterial viability analyses, interpreted, and statistically analyzed the data. All co-authors contributed to revisions.
Funding
This work was funded by the European Union and Greek national funds through the Operational Program “Education and Lifelong Learning” of the National Strategic Reference Framework (NSRF) – ARISTEIA II (HYPOXIA project, No. 4705).
Conflict of Interest
The authors declare that the research was conducted in the absence of any commercial or financial relationships that could be construed as a potential conflict of interest.
Acknowledgments
The authors would like to thank the participants of the mesocosm experiment for their significant help during experimental setup and sampling. Special thanks are also attributed to Dr. A. Kouvarakis and Prof. S. A. Pergantis for providing access to the Environmental Chemical Processes Laboratory (E.C.P.L.) and for their valuable advising during chromatography analyses and Mrs. de Wilde for her assistance on the English language.
Supplementary Material
The Supplementary Material for this article can be found online at: https://www.frontiersin.org/articles/10.3389/fmars.2020.00270/full#supplementary-material
References
Baustian, M. M., Rabalais, N. N., Morrison, W. L., and Turner, R. E. (2011). Seasonal microphytobenthos on the hypoxic northern Gulf of Mexico continental shelf. Mar. Ecol. Prog. Ser. 436, 51–66. doi: 10.3354/meps09262
Baustian, M. M., Rabalais, N. N., Morrison, W. L., Turner, R. E., Johnson, V. R., Brownlee, C., et al. (2013). The contribution of microphytobenthos to total productivity in upper Narragansett Bay, Rhode Island. Estuar. Coast. Shelf Sci. 6, 1813–1824. doi: 10.1016/j.ecss.2011.09.005
Bianchi, T. S., Dibb, J. E., and Findlay, S. (1993). Early diagenesis of plant pigments in hudson river sediments. Estuar. Coast. Shelf Sci. 36, 517–527. doi: 10.1006/ecss.1993.1031
Borrego, C. M., and Garcia-Gil, L. J. (1994). Separation of bacteriochlorophyll homologues from green photosynthetic sulfur bacteria by reversed-phase HPLC. Photosynth. Res. 41, 157–164. doi: 10.1007/BF02184156
Brotas, V., Mendes, C. R., and Cartaxana, P. (2007). Microphytobenthic biomass assessment by pigment analysis: comparison of spectrophotometry and High Performance Liquid Chromatography methods. Hydrobiologia 587, 19–24. doi: 10.1007/s10750-007-0680-z
Brotas, V., and Plante-Cuny, M. R. (1998). Spatial and temporal patterns of microphytobenthic taxa of estuarine tidal flats in the Tagus Estuary (Portugal) using pigment analysis by HPLC. Mar. Ecol. Prog. Ser. 171, 43–57.
Brotas, V., and Plante-Cuny, M. R. (2003). The use of HPLC pigment analysis to study microphytobenthos communities. Acta Oecologica 24, 109–115. doi: 10.1016/S1146-609X(03)00013-4
Cahoon, L. B. (1999). The role of benthic microalgae in neritic ecosystems. Oceanogr. Mar. Biol. Annu. Rev. 37, 47–86.
Cibic, T., and Blasutto, O. (2011). “Living marine benthic diatoms as indicators of nutrient enrichment: a case study in the Gulf of Trieste,” in Diatoms: Ecology and Life Cycle, ed. J. C. Compton (Hauppauge, NY: Nova Science Publishers, Inc), 169–184.
Cibic, T., Blasutto, O., Falconi, C., and Fonda Umani, S. (2007a). Microphytobenthic biomass, species composition and nutrient availability in sublittoral sediments of the Gulf of Trieste (northern Adriatic Sea). Estuar. Coast. Shelf Sci. 75, 50–62. doi: 10.1016/j.ecss.2007.01.020
Cibic, T., Blasutto, O., Hancke, K., and Johnsen, G. (2007b). Microphytobenthic species composition, pigment concentration, and primary production in sublittoral sediments of the Trondheimsfjord (Norway). J. Phycol. 43, 1126–1137. doi: 10.1111/j.1529-8817.2007.00405.x
Cibic, T., Fazi, S., Nasi, F., Pin, L., Alvisi, F., Berto, D., et al. (2019). Natural and anthropogenic disturbances shape benthic phototrophic and heterotrophic microbial communities in the Po River Delta system. Estuar. Coast. Shelf Sci. 222, 168–182. doi: 10.1016/j.ecss.2019.04.009
Cibic, T., Franzo, A., Celussi, M., Fabbro, C., and Del Negro, P. (2012). Benthic ecosystem functioning in hydrocarbon and heavy-metal contaminated sediments of an Adriatic lagoon. Mar. Ecol. Prog. Ser. 458, 69–87. doi: 10.3354/meps09741
Clarke, K. R., Gorley, R. N., Somerfield, P. J., and Warwick, R. M. (2014). Change in Marine Communities: An Approach to Statistical Analysis and Interpretation, 3rd Edn. Plymouth: PRIMER-E Ltd.
Coll, M., Piroddi, C., Albouy, C., Ben Rais Lasram, F., Cheung, W. W. L., Christensen, V., et al. (2012). The Mediterranean Sea under siege: spatial overlap between marine biodiversity, cumulative threats and marine reserves. Glob. Ecol. Biogeogr. 21, 465–480. doi: 10.1111/j.1466-8238.2011.00697.x
Danovaro, R., Marrale, D., Della Croce, N., Parodi, P., and Fabiano, M. (1999). Biochemical composition of sedimentary organic matter and bacterial distribution in the Aegean Sea: trophic state and pelagic-benthic coupling. J. Sea Res. 42, 117–129. doi: 10.1016/S1385-1101(99)00024-6
Dimitriou, P. D., Papageorgiou, N., Arvanitidis, C., Assimakopoulou, G., Pagou, K., Papadopoulou, K. N., et al. (2015). One step forward: benthic pelagic coupling and indicators for environmental status. PLoS One 10:e0141071. doi: 10.1371/journal.pone.0141071
Dimitriou, P. D., Papageorgiou, N., Geropoulos, A., Kalogeropoulou, V., Moraitis, M., Santi, I., et al. (2017a). A novel mesocosm setup for benthic-pelagic coupling experiments. Limnol. Oceanogr. Methods 15, 349–362. doi: 10.1002/lom3.10163
Dimitriou, P. D., Papageorgiou, N., Geropoulos, A., Kalogeropoulou, V., Moraitis, M., Santi, I., et al. (2017b). Benthic pelagic coupling in a mesocosm experiment: delayed sediment responses and regime shifts. Sci. Total Environ. 605–606, 637–645. doi: 10.1016/j.scitotenv.2017.06.239
Dimitriou, P. D., Papageorgiou, N., and Karakassis, I. (2017c). Response of benthic macrofauna to eutrophication in a mesocosm experiment: ecosystem resilience prevents hypoxic conditions. Front. Mar. Sci. 4:391. doi: 10.3389/fmars.2017.00391
Edwards, V. R., Tett, P., and Jones, K. J. (2003). Changes in the yield of chlorophyll a from dissolved available inorganic nitrogen after an enrichment event - Applications for predicting eutrophication in coastal waters. Cont. Shelf Res. 23, 1771–1785. doi: 10.1016/j.csr.2003.06.003
Facca, C., Sfriso, A., and Socal, G. (2002). Changes in abundance and composition of phytoplankton and microphytobenthos due to increased sediment fluxes in the Venice lagoon, Italy. Estuar. Coast. Shelf Sci. 54, 773–792. doi: 10.1006/ecss.2001.0848
Falcioni, T., Papa, S., and Gasol, J. M. (2008). Evaluating the flow-cytometric nucleic acid double-staining protocol in realistic situations of planktonic bacterial death. Appl. Environ. Microbiol. 74, 1767–1779. doi: 10.1128/AEM.01668-07
Fodelianakis, S., Moustakas, A., Papageorgiou, N., Manoli, O., Tsikopoulou, I., Michoud, G., et al. (2016). Modified niche optima and breadths explain the historical contingency of bacterial community responses to eutrophication in coastal sediments. Mol. Ecol. 26, 2006–2018. doi: 10.1111/mec.13842
Franzo, A., Cibic, T., Del Negro, P., and Solidoro, C. (2014). Microphytobenthic response to mussel farm biodeposition in coastal sediments of the northern Adriatic Sea. Mar. Pollut. Bull. 79, 379–388. doi: 10.1016/j.marpolbul.2013.11.002
Furukawa, Y., Smith, A. C., Kostka, J. E., Watkins, J., and Alexander, C. R. (2004). Quantification of macrobenthic effects on diagenesis using a multicomponent inverse model in salt marsh sediments. Limnol. Oceanogr. 49, 2058–2072. doi: 10.4319/lo.2004.49.6.2058
Gray, J. S., and Elliott, M. (2009). Ecology of Marine Sediments. New York, NY: Oxford University press. doi: 10.1007/s13398-014-0173-7.2
Griffith, G. P., Strutton, P. G., and Semmens, J. M. (2017). Climate change alters stability and species potential interactions in a large marine ecosystem. Glob. Chang. Biol. 90–100. doi: 10.1111/gcb.13891
Haglund, A. L., Lantz, P., Törnblom, E., and Tranvik, L. (2003). Depth distribution of active bacteria and bacterial activity in lake sediment. FEMS Microbiol. Ecol. 46, 31–38. doi: 10.1016/S0168-6496(03)00190-9
Hedges, J. I., and Stern, J. H. (1984). Carbon and nitrogen determinations of carbonate-containing solids1. Limnol. Oceanogr. 29, 657–663. doi: 10.4319/lo.1984.29.3.0657
Holmer, M., and Kristensen, E. (1994). Coexistence of sulfate reduction and methane production in an organic-rich sediment. Mar. Ecol. Prog. Ser. 107, 177–184. doi: 10.3354/meps107177
Karydis, M., and Kitsiou, D. (2012). Eutrophication and environmental policy in the Mediterranean Sea: a review. Environ. Monit. Assess. 184, 4931–4984. doi: 10.1007/s10661-011-2313-2
Klein, B., and Riaux-Gobin, C. (1991). Algal pigment diversity in coastal sediments from Kerguelen (sub-Antarctic Islands) reflecting local dominance of green algae, euglenoids and diatoms. Polar Biol. 11, 439–448. doi: 10.1007/BF00233079
Komárek, J., and Johansen, J. R. (2015). Filamentous Cyanobacteria. doi: 10.1016/B978-0-12-385876-4.00004-9
Kristensen, E. (1988). “Benthic fauna and biogeochemical processes in marine sediments: microbial Activites and Fluxes,” in Nitrogen Cycling in Coastal Marine Environments, ed. T. I. H. I. Blackburn (Hoboken, NJ: John Wiley & Sons Ltd), 301–341.
Lake, S. J., and Brush, M. J. (2011). The contribution of microphytobenthos to total productivity in upper Narragansett Bay, Rhode Island. Estuar. Coast. Shelf Sci. 95, 289–297.
Lamy, D., Artigas, L. F., Jauzein, C., Lizon, F., and Cornille, V. (2006). Coastal bacterial viability and production in the eastern English Channel: a case study during a Phaeocystis globosa bloom. J. Sea Res. 56, 227–238. doi: 10.1016/j.seares.2006.04.003
Larson, F., and Sundbäck, K. (2008). Role of microphytobenthos in recovery of functions in a shallow-water sediment system after hypoxic events. Mar. Ecol. Prog. Ser. 357, 1–16. doi: 10.3354/meps07426
Lauridsen, T. L., Schlüter, L., and Johansson, L. S. (2011). Determining algal assemblages in oligotrophic lakes and streams: comparing information from newly developed pigment/chlorophyll a ratios with direct microscopy. Freshw. Biol. 56, 1638–1651. doi: 10.1111/j.1365-2427.2011.02588.x
Loh, P. S., Miller, A. E. J., Reeves, A. D., Harvey, S. M., Overnell, J., and Systems, E. (2008). Assessing the biodegradability of terrestrially-derived organic matter in Scottish sea loch sediments. Hydrol. Earth Syst. Sci. 12, 811–823.
Louda, W. J., Loitz, J. W., Rudnick, D. T., and Baker, E. W. (2000). Early diagenetic alteration of chlorophyll-a and bacteriochlorophyll-a in a contemporaneous marl ecosystem; Florida Bay. Org. Geochem. 31, 1561–1580. doi: 10.1016/S0146-6380(00)00071-1
Mackey, M. D., Mackey, D. J., Higgins, H. W., and Wright, S. W. (1996). CHEMTAX - a Program for Estimating Class Abundances From Chemical Markers: application To HPLC measurements of phytoplankton. Mar. Ecol. Prog. Ser. 144, 265–283. doi: 10.3354/meps144265
Madsen, H., Lawrence, D., Lang, M., Martinkova, M., and Kjeldsen, T. R. (2014). Review of trend analysis and climate change projections of extreme precipitation and floods in Europe. J. Hydrol. 519, 3634–3650. doi: 10.1016/J.JHYDROL.2014.11.003
Mendes, C. R. B., Tavano, V. M., Leal, M. C., de Souza, M. S., Brotas, V., and Garcia, C. A. E. (2013). Shifts in the dominance between diatoms and cryptophytes during three late summers in the Bransfield Strait (Antarctic Peninsula). Polar Biol. 36, 537–547. doi: 10.1007/s00300-012-1282-4
Meyer-Reil, L.-A., and Köster, M. (2000). Eutrophication of marine waters: effects on benthic microbial communities. Mar. Pollut. Bull. 41, 255–263. doi: 10.1016/S0025-326X(00)00114-4
Middelburg, J. J., Barranguet, C., Boschker, H. T. S., Herman, P. M. J., Moens, T., and Heip, C. H. R. (2000). The fate of intertidal microphytobenthos carbon: An in situ 13 C-labeling study. Limnol. Oceanogr. 45, 1224–1234. doi: 10.4319/lo.2000.45.6.1224
Nielsen, L. P., Risgaard-Petersen, N., Fossing, H., Christensen, P. B., and Sayama, M. (2010). Electric currents couple spatially separated biogeochemical processes in marine sediment. Nature 463, 1071–1074. doi: 10.1038/nature08790
Potapova, M., Desianti, N., and Enache, M. (2016). Potential effects of sediment contaminants on diatom assemblages in coastal lagoons of New Jersey and New York States. Mar. Pollut. Bull. 107, 453–458. doi: 10.1016/j.marpolbul.2016.01.028
Quero, G. M., Cassin, D., Botter, M., Perini, L., and Luna, G. M. (2015). Patterns of benthic bacterial diversity in coastal areas contaminated by heavy metals, polycyclic aromatic hydrocarbons (PAHs) and polychlorinated biphenyls (PCBs). Front. Microbiol. 6:1053. doi: 10.3389/fmicb.2015.01053
Rauch, M., Denis, L., and Dauvin, J.-C. (2008). The effects of Phaeocystis globosa bloom on the dynamics of the mineralization processes in intertidal permeable sediment in the Eastern English Channel (Wimereux, France). Mar. Pollut. Bull. 56, 1284–1293. doi: 10.1016/j.marpolbul.2008.04.026
Riaux-Gobin, C., Llewellyn, C., and Klein, B. (1987). Microphytobenthos from two subtidal sediments from North Brittany. 11. Variations of pigment compositions and concentrations determined by HPLC and conventional techniques. Mar. Ecol. Prog. Ser. 40, 275–283.
Sañé, E., Valente, A., Fatela, F., Cabral, M. C., Beltrán, C., and Drago, T. (2019). Assessment of sedimentary pigments and phytoplankton determined by CHEMTAX analysis as biomarkers of unusual upwelling conditions in summer 2014 off the SE coast of Algarve. J. Sea Res. 146, 33–45. doi: 10.1016/j.seares.2019.01.007
Santi, I., Tsiola, A., Dimitriou, P. D., Fodelianakis, S., Kasapidis, P., Papageorgiou, N., et al. (2019). Prokaryotic and eukaryotic microbial community responses to N and P nutrient addition in oligotrophic Mediterranean coastal waters: novel insights from DNA metabarcoding and network analysis. Mar. Environ. Res. 150:104752. doi: 10.1016/j.marenvres.2019.104752
Scholz, B., and Liebezeit, G. (2012). Microphytobenthic dynamics in a Wadden Sea intertidal flat - Part II: seasonal and spatial variability of non-diatom community components in relation to abiotic parameters. Eur. J. Phycol. 47, 120–137. doi: 10.1080/09670262.2012.665251
Sigmon, D. E., and Cahoon, L. B. (1997). Comparative effects of benthic microalgae and phytoplankton on dissolved silica fluxes. Aquat. Microb. Ecol. 13, 275–284.
Svensen, C., Egge, J., and Stiansen, J. (2001). Can silicate and turbulence regulate the vertical flux of biogenic matter? A mesocosm study. Mar. Ecol. Prog. Ser. 217, 67–80. doi: 10.3354/meps217067
ter Braak, C. J. F., and Smilauer, P. (2012). Canoco Reference Manual and User’s Guide: Software for Ordination (version 5.0). Ithaca, NY: Microcomputer Power.
Tsikopoulou, I., Papageorgiou, N., Tsiavos, T., Fodelianakis, S., Kotzabasis, K., and Karakassis, I. (2019). Microphytobenthic response to organic matter enrichment: Does the same stressor lead to identical communities? Reg. Stud. Mar. Sci. 29:100682. doi: 10.1016/j.rsma.2019.100682
Ubertini, M., Lefebvre, S., Gangnery, A., Grangeré, K., Le Gendre, R., and Orvain, F. (2012). Spatial variability of benthic-pelagic coupling in an estuary ecosystem: consequences for Microphytobenthos Resuspension phenomenon. PLoS One 7:e44155. doi: 10.1371/journal.pone.0044155
Underwood, G. J. C., and Barnett, M. (2006). “What determines species composition in microphytobenthic biofilms?” in Functioning of Microphytobenthos in Estuaries, eds J. Komkamp, J. F. K. De Brouwer, G. F. Blanchard, R. M. Forster, and V. Creach (Amsterdam: Royal Netherlands Academy of Arts and Sciences), 123–140. doi: 10.1086/586980
Vidal, M., and Duarte, C. (2000). Nutrient accumulation at different supply rates in experimental Mediterranean planktonic communities. Mar. Ecol. Prog. Ser. 207, 1–11. doi: 10.3354/meps207001
Weckstrom, K., and Juggins, S. (2005). Coastal diatom-environment relationships form the Gulf of Finland, Baltic Sea. Phycol. Soc. Am. 42, 21–35.
Wildish, D. J., Akagi, H. M., Hamilton, N., and Hargrave, B. T. (1999). A recommended method for monitorig sediments to detect organic enrichment from mariculture in the Bay of Fundy. Can. Tech. Rept. Fish. Aquat. Sci. 2286:31.
Wright, S. W., Ishikawa, A., Marchant, H. J., Davidson, A. T., Van Den Enden, R. L., and Nash, G. V. (2009). Composition and significance of picophytoplankton in Antarctic waters. Polar Biol. 32, 797–808. doi: 10.1007/s00300-009-0582-9
Wright, S. W., Jeffrey, S. W., Mantoura, R. F. C., Llewellyn, C. A., Bjornland, T., Repete, D., et al. (1991). Improved HPLC method for the analysis of chlorophylls and carotenoids from marine phytoplankton. Mar. Ecol. Prog. Ser. 77, 183–196.
Wright, S. W., Thomas, D. P., Marchant, H. J., Higgins, H. W., Mackey, M. D., and Mackey, D. J. (1996). Analysis of phytoplankton of the Australian sector of the Southern Ocean: comparisons of microscopy and size frequency data with interpretations of pigment HPLC data using the “CHEMTAX” matrix factorisation program. Mar. Ecol. Prog. Ser. 144, 285–298. doi: 10.3354/meps144285
Yacobi, Y. Z., Eckert, W., Triiper, H. G., and Berman, T. (1990). High performance liquid chromatography detection of phototrophic bacterial pigments in aquatic environments abstract. Microb. Ecol. 19, 127–136.
Yentsch, C. S., and Menzel, D. W. (1963). A method for the determination of phytoplankton chlorophyll and phaeophytin by fluorescence. Deep Sea Res. Oceanogr. Abstr. 10, 221–231. doi: 10.1016/0011-7471(63)90358-9
Keywords: microphytobenthos, mesocosm experiment, benthic–pelagic coupling, eutrophication, bacterial viability
Citation: Tsikopoulou I, Santi I, Dimitriou PD, Papageorgiou N, Pitta P and Karakassis I (2020) Response of Microphytobenthos and Benthic Bacteria Viability to Eutrophication in a Benthic–Pelagic Coupling Mesocosm Experiment. Front. Mar. Sci. 7:270. doi: 10.3389/fmars.2020.00270
Received: 06 January 2020; Accepted: 03 April 2020;
Published: 30 April 2020.
Edited by:
Katherine Dafforn, Macquarie University, AustraliaReviewed by:
Giulia Filippini, Macquarie University, AustraliaDana Clark, Cawthron Institute, New Zealand
Sally Bracewell, University of New South Wales, Australia
Julie Anne Hope, The University of Auckland, New Zealand
Copyright © 2020 Tsikopoulou, Santi, Dimitriou, Papageorgiou, Pitta and Karakassis. This is an open-access article distributed under the terms of the Creative Commons Attribution License (CC BY). The use, distribution or reproduction in other forums is permitted, provided the original author(s) and the copyright owner(s) are credited and that the original publication in this journal is cited, in accordance with accepted academic practice. No use, distribution or reproduction is permitted which does not comply with these terms.
*Correspondence: Irini Tsikopoulou, aS50c2lrb3BvdWxvdUB1b2MuZ3I=