- 1Department of Primary Industries, NSW Fisheries, National Marine Science Centre, Coffs Harbour, NSW, Australia
- 2National Marine Science Centre, Southern Cross University, Coffs Harbour, NSW, Australia
- 3University of Western Australia Oceans Institute and School of Biological Sciences, University of Western Australia, Crawley, WA, Australia
- 4Centre for Marine Science and Innovation, School of Biological Earth and Environmental Sciences, University of New South Wales, Kensington, NSW, Australia
- 5Sydney Institute of Marine Science, Mosman, NSW, Australia
- 6Institute of Marine Research, His, Norway
- 7ARC Centre of Excellence in Synthetic Biology, Department of Molecular Sciences, Macquarie University, North Ryde, NSW, Australia
- 8Department of Primary Industries, Elizabeth Macarthur Agricultural Institute, Menangle, NSW, Australia
- 9School of Life and Environmental Sciences, Coastal and Marine Ecosystems, The University of Sydney, Sydney, NSW, Australia
- 10Singapore Centre for Environmental Life Sciences Engineering, Nanyang Technological University, Singapore, Singapore
- 11Department of Science and Environment, Roskilde University, Roskilde, Denmark
Global habitat deterioration of marine ecosystems has led to a need for active interventions to halt or reverse the loss of ecological function. Restoration has historically been a key tool to reverse habitat loss and restore functions, but the extent to which this will be sufficient under future climates is uncertain. Emerging genetic technologies now provide the ability for restoration to proactively match adaptability of target species to predicted future environmental conditions, which opens up the possibility of boosting resistance to future stress in degraded and threatened habitats. As such, the choice of whether to restore to historical baselines or anticipate the future remains a key decision that will influence restoration success in the face of environmental and climate change. Here, we present an overview of the different motives for restoration – to recover or revive lost or degraded habitats to extant or historical states, or to reinforce or redefine for future conditions. We focus on the genetic and adaptive choices that underpin each option and subsequent consequences for restoration success. These options span a range of possible trajectories, technological advances and societal acceptability, and represent a framework for progressing restoration of marine habitat forming species into the future.
Introduction
Habitat deterioration and destruction threaten global ecological functions and result in significant loss of social and economic values (Hoffmann et al., 2010; Venter et al., 2016; Powers and Jetz, 2019). Recognizing this threat, the UN has declared 2021–2030 the “decade of restoration” (FAO, 2019) with the aim to restore 350 million hectares of degraded ecosystems, and massively scale up restoration efforts to promote resilience to climate and anthropogenic change and reverse biodiversity loss. In particular, there is an urgent need for marine restoration initiatives to combat and reverse existing habitat loss (e.g., Krumhansl et al., 2016; Filbee-Dexter and Wernberg, 2018), and even pre-empt future habitat loss (Gattuso et al., 2015; Hughes et al., 2017). As such, both preventive (passive) and adaptive (active) restoration measures are globally supported as viable options under future climates (IPCC, 2019).
Successful, large scale restoration efforts will require robust, science-based practices that consider the fundamental question: “to what time point should we restore?” Historically, restoration has sought to replicate what was lost and to recover properties of populations or communities (species, structure, ecosystem services) to historic states that are putatively adapted to extant environmental conditions. However, ongoing habitat deterioration and climate change is outpacing the ability of many species to adapt, challenging the assumption that restoration to historic states will be sufficient to ensure persistence into the future (Hobbs et al., 2009; van Oppen et al., 2015; Perring et al., 2015; Breed et al., 2018, 2019; Gurgel et al., 2020). Instead, improving or redesigning properties of lost habitats to withstand predicted future conditions may confer greater restoration success. Moreover, restoration could also include anticipatory actions (prior to loss) to proactively boost resilience and adaptive capacity of extant populations to predicted future conditions (assisted adaptation, Aitken and Whitlock, 2013; van Oppen et al., 2017). Thus, the decision whether to restore to extant or historical baselines, versus some predicted but uncertain future state is likely to be central to restoration success into the future.
Given that habitat resilience (the capacity to resist or recover from perturbation) and adaptive capacity to cope with environmental change will be influenced by underlying genetic properties of populations (genetic diversity, composition of genes and alleles; Wernberg et al., 2018), determining provenance (the origin and diversity of donor individuals) and thus, choosing an historic versus unknown future genetic baseline is a key consideration in contemporary restoration science (Breed et al., 2018). Although genetic baselines are not static and change through time, here we define a genetic baseline as the level of the genetic diversity and structure chosen and initially replicated in a restoration program through provenance decisions. Current best practice recommends local provenance for restoration, that is, that donor adults or propagules are sourced within contemporary extant genetic boundaries to maintain locally adapted genotypes and avoid maladaptation and genetic pollution (e.g., SERA, 2017). Moreover, characterizing and replicating extant levels of genetic diversity and structure is recommended to ensure sufficient diversity for adaptation (Bischoff et al., 2010; Wood et al., in review). Unfortunately, restoration, particularly in marine systems, has historically been conducted in the absence of formal genetic assessments, which may contribute to poor outcomes to date (McKay et al., 2005; Mijangos et al., 2015; Crouzeilles et al., 2016). Although current restoration efforts are increasingly incorporating empirical assessments of genetic provenance into practice1 (e.g., Evans et al., 2018; Wood et al., in review), the extent to which this will confer success under future scenarios of climate and anthropogenic stress remains a critical uncertainty (Weeks et al., 2011; Perring et al., 2015).
Predicted increases in climate and anthropogenic stress have prompted calls for “future-proof” restoration practices that reinforce or even redesign historic and extant genetic baselines to confer increased resilience to future conditions and stressors in restored populations (van Oppen et al., 2017; Breed et al., 2018, 2019; Ralls et al., 2018; Wood et al., 2019; Sgrò et al., 2011). Critically, both reinforcing and redefining existing genetic baselines can be applied similarly through traditional restoration programs after loss or degradation, or as a preventative measure prior to any impact occurring. Reinforcing genetic baselines could be achieved via increasing genetic diversity in restored populations to provide sufficient adaptive capacity to cope with future change. Another strategy involves matching predicted environmental and anthropogenic conditions to the ability of individuals and populations to adapt through addition of resilient genotypes identified through experimentation (Breed et al., 2019) or genome wide association studies (GWAS; van Oppen et al., 2015; Rinkevich, 2019). Completely redefining genetic baselines and population resilience is now possible with emerging genetic technologies (Popkin, 2018). For example, synthetic biology and gene editing using tools such as CRISPR/CAS9 can be harnessed to create or spread novel or engineered beneficial genetic elements within restored or vulnerable populations (Coleman and Goold, 2019) and allow bespoke restoration or assisted adaptation programs to be designed for specific stressors of interest.
Here, we focus on kelp forests, critical marine habitats in decline, to present a framework to guide the design of restoration initiatives as a function of four possible motives: Recover, Revive, Reinforce, and Redefine (Figure 1). Importantly, this framework does not only apply to kelp forests but to restoration efforts more broadly. Recover and revive center on contemporary, reactive restoration practices that seek to return already degraded habitat to historic or extant baselines. In contrast, reinforce and redefine seek to proactively anticipate future conditions and boost resilience in lost, degraded or vulnerable habitats. This framework spans a range of possible trajectories, technological advances and societal acceptability and represents a platform for progressing marine restoration into the future. We discuss techniques for estimating appropriate genetic provenance in cases where prior genetic data is limited and pathways to develop preventative strategies that anticipate and boost resilience to future stress.
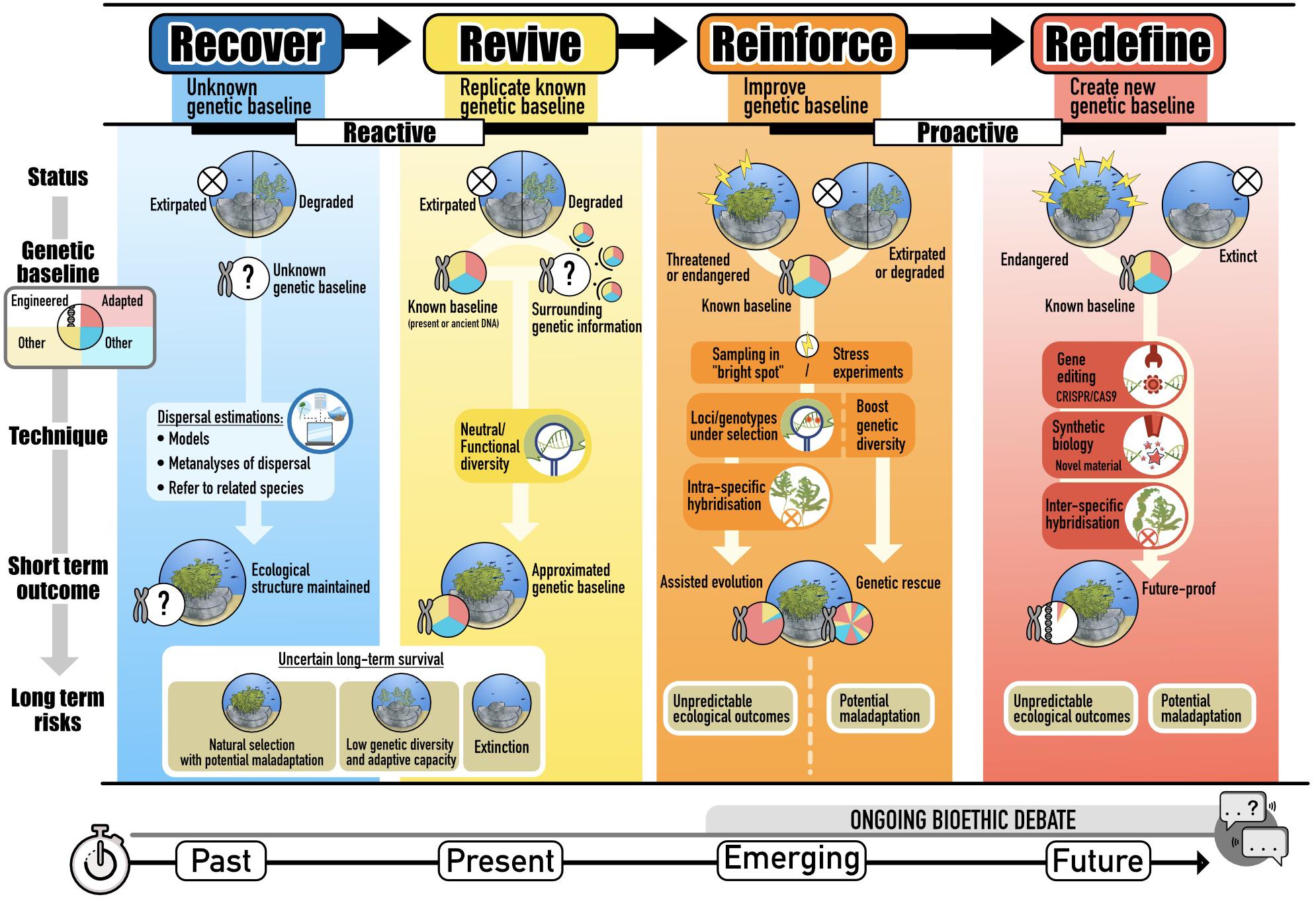
Figure 1. Different motives for restoration. Whether to recover or revive historic and extant genetic baselines or reinforce and redefine to unknown baselines for future conditions, is a critical choice in restoration programs that determines possibilities for adaptability and persistence under future environmental conditions and climates.
Kelp Forests – Critical Habitats in Decline
Kelp forests are highly productive seascapes dominated by large brown seaweeds (Wernberg and Filbee-Dexter, 2019). They are particularly prominent in temperate to polar environments where they are the foundations for immense biodiversity and valuable ecological services such as important recreational and commercial fisheries (Wernberg et al., 2019b). The best available evidence suggests that 40–60% of the world’s kelp forests have been in decline over the past 50 years (Krumhansl et al., 2016; Wernberg et al., 2019b) as a consequence of a variety of direct and indirect stressors including warming, marine heatwaves, eutrophication and increasing herbivory from range-shifting warm-water herbivores (Vergés et al., 2014; Filbee-Dexter and Wernberg, 2018). For example, in Australia, kelp forests of laminarian kelp and fucoids (e.g., Ecklonia radiata, Scyothalia dorycarpa, Phyllospora comosa) are found throughout the Great Southern Reef along the southern coastline of the continent (Coleman and Wernberg, 2017; Wernberg et al., 2019a). In recent decades, almost every part of this unique large-scale ecosystem has experienced localized to regional decline and loss of kelp forests due to a range of processes including eutrophication, over grazing, warming, and marine heatwaves (Coleman et al., 2008; Connell et al., 2008; Ling et al., 2009; Vergés et al., 2016; Wernberg et al., 2016; Carnell and Keough, 2019). In almost all cases in Australia and globally, kelp loss has been persistent with no signs of natural recovery. Instead, kelp forests have been replaced by alternate habitats including turf algae (Filbee-Dexter and Wernberg, 2018) or urchin barrens (Filbee-Dexter and Scheibling, 2014; Ling et al., 2015) which create strong reinforcing feedbacks that prevent natural recovery of kelp. This provides strong impetus and motivation to apply interventions such as restoration, especially where the initial stressor no longer occurs (e.g., Coleman et al., 2008) or can be controlled (e.g., Sanderson et al., 2015; Layton et al., 2020).
The first published studies on kelp forest restoration stem from the 1960s–1970s in Japan and North America (Carlisle et al., 1964; North, 1976; Kuwahara et al., 2006). Although the number of kelp restoration attempts has increased exponentially since then, such efforts are generally of limited duration (<2 years), small in scale (<0.1 ha) and have had limited success (Eger et al., 2019). Moreover, these efforts have generally lacked empirical data of underlying patterns of genetic diversity and structure (particularly functional genetic diversity), and often have not considered demographic history and ecological processes influencing kelp populations, all of which may have contributed to poor restoration outcomes. There are however some notable ongoing projects that address some of these concerns. For example, the Wheeler North Reef in southern California has successfully established a giant kelp forest community at a large scale (70 hectares of artificial reef structure) to compensate for the loss of natural giant kelp forests due to impacts from a nuclear power station (Schroeter et al., 2018). The Korean government has also developed a major marine seaweed foresting program and has already restored over 3,000 hectares of seaweed forests since 2009 (Lee, 2019). In Sydney, Australia, a project is also ongoing that aims to re-establish lost forests of P. comosa at the scale of the initial degradation −70 km of metropolitan coastline1 (Campbell et al., 2014; Wood et al., in review). The projects that have seen sustained success have generally been well financed, have often been coordinated by regulatory bodies, carried out over a sustained periods of time, or harnessed the power of local community engagement to deliver lasting results (Eger et al., 2019; DeAngelis et al., 2020; Layton et al., 2020). While these projects are currently in the minority, interest in kelp restoration is accelerating and we are at the point where we can adequately learn from our past mistakes and enhance restoration of our underwater forests (Eger et al., 2019). Central to the success of these future efforts, however, is determining which environmental conditions to restore to and, therefore, whether to recover or revive genetic baselines, or reinforce and redefine them. These issues are common not only to kelp forests restoration but also to marine and terrestrial restoration more broadly.
Recover – Restoration That Replicates Unknown Genetic Baselines
Marine restoration initiatives have historically operated in the absence of empirical genetic data, instead focusing on restoring community and habitat structure, functions and biodiversity (e.g., Campbell et al., 2014; Marzinelli et al., 2016; Verdura et al., 2018). This is partly a reflection of the historically high costs, complexity and inaccessibility of genomic techniques to assess baseline genetic diversity and structure using high throughput methods. Nonetheless, restoration practices have typically informally considered genetic baselines through available scientific literature on related taxa, knowledge of direct dispersal distances where measurable, incorporation of general genetic principles into practice (e.g., mixing populations to avoid inbreeding and ensure diversity) or through expert opinion. For example, global metanalyses for marine algae have shown that scales of dispersal and population connectivity are generally limited to ∼50 km (Durrant et al., 2014), which can be used as a general rule of thumb for provenance when empirical data for the species of interest is lacking. Alternatively, estimates of oceanographic dispersal distance relative to properties of propagules (Gaylord et al., 2006) can sometimes be used to infer appropriate provenance in the absence of data (e.g., Coleman et al., 2011b; Coleman et al., 2013), with consideration of potential barriers to dispersal or genetic breaks (e.g., Coleman and Brawley, 2005; Coleman and Kelaher, 2009; Alberto et al., 2010; Durrant et al., 2018).
The risk associated with restoring populations in the absence of empirical genetic knowledge is that restored populations will inadvertently lack diversity or appropriate adaptive capacity to cope with extant or future conditions (e.g., Williams, 2001), which may be particularly pertinent for species that exhibit small scale dispersal and are therefore susceptible to reduced gene flow, increased inbreeding or asexual propagation (e.g., Guillemin et al., 2008; Coleman et al., 2011a, 2019; Coleman and Wernberg, 2018; Miller et al., 2019). This risk is exacerbated given increasing habitat fragmentation and deterioration often characterizes the seascapes from which donor adults or propagules must be sourced for restoration (Coleman and Kelaher, 2009). Inadvertently sourcing donor plants from outside locally adapted populations could cause maladaptation and decreased fitness relative to appropriate provenance (Sexton et al., 2011), which may contribute to the general lack of successes in marine restoration to date (e.g., see Rinkevich, 2014 for a coral example). Obtaining genetic baselines is, however, now within reach of most restoration programs due to the increasing sophistication and reduced cost of modern genetic techniques (e.g., sequencing) that allow for assessments of population genetic structure without lengthy development stages (Narum et al., 2013). We argue that such assessments should now be planned and budgeted for prior to implementation in future restoration initiatives by adapting existing frameworks (e.g., Hoffmann et al., 2015).
Revive – Restoring Extant or Historic Genetic Baselines
Contemporary restoration programs should aim, at a minimum, to replicate natural genetic baselines informed by empirical genetic data. Given that “before” data collected prior to loss rarely exist (Grant et al., 2017), population genetic diversity and structure should be assessed within surrounding, putatively non-impacted populations. This can then be replicated within restored populations through careful selection of donor populations and individuals (Figure 2). Such genetic assessments can now be done with less cost and effort than previously with the advent and accessibility of high throughout sequencing and should be included as “best-practice” in restoration programs. Moreover, modern genomic techniques (e.g., genotyping by sequencing of single nucleotide polymorphisms; SNPs) allow both neutral and functional or adaptive genetic diversity to be characterized simultaneously, an advance that is set to improve restoration outcomes through refined provenance decisions. While there are no current examples of its use in the literature in the context of restoration, genotyping by sequencing of tens of thousands of SNP loci, along with reference genomes to identify functions, is providing detailed extant baselines for key foundation species of kelp (e.g., E. radiata), that will soon allow replication (or bespoke manipulation, see section “Reinforce – Improving Genetic Baselines for Future Conditions”) of neutral and functional diversity and structure in restoration programs.
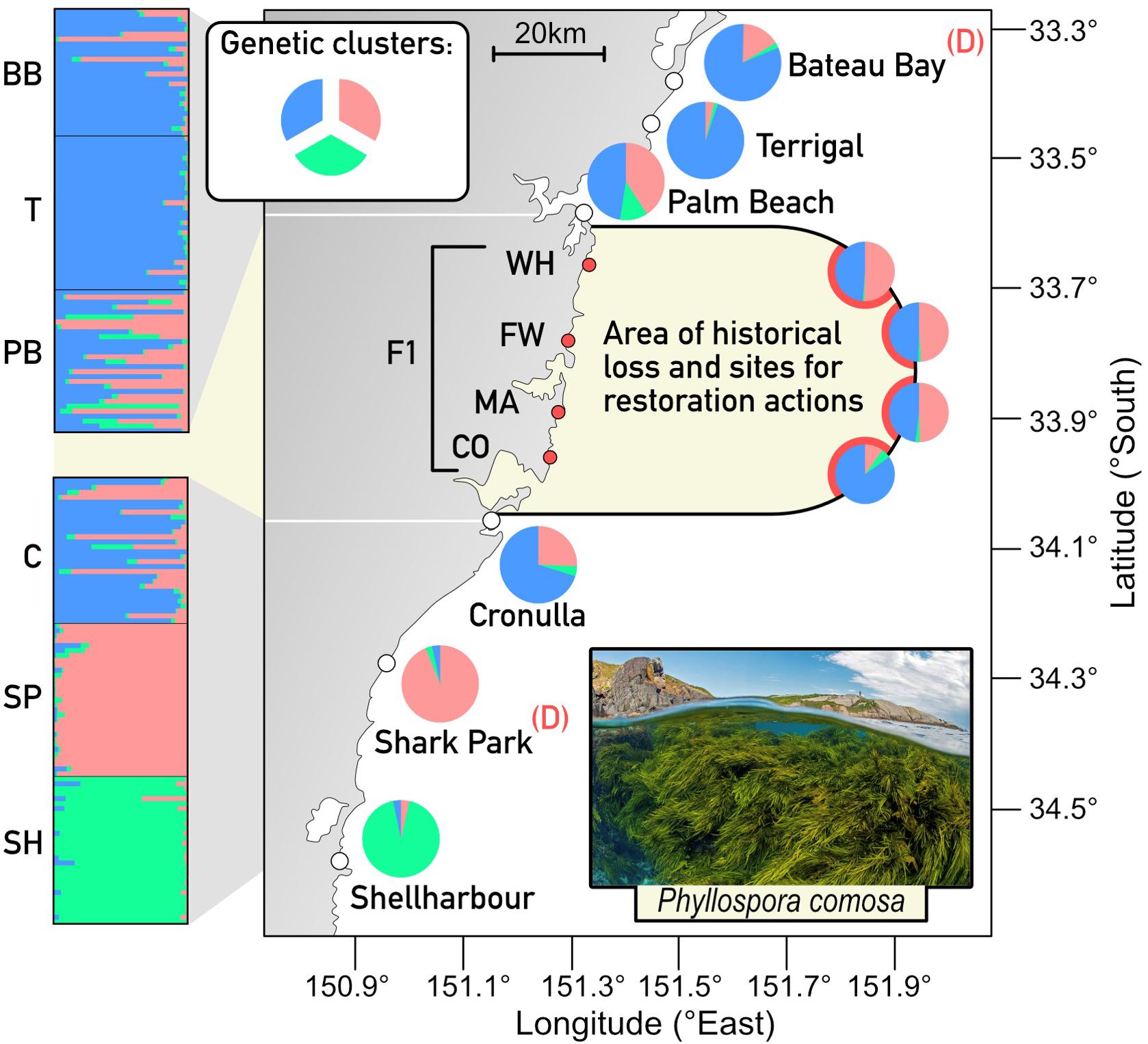
Figure 2. Restoration of crayweed (P. comosa) at four sites within the 70 km gap in distribution where it was locally extinct (shaded area), was informed by genetic structure of six extant surrounding populations. Restored sites were a mix of plants from two donor populations (D) and the resulting F1 generation mimicked natural, extant genetic baselines. Adapted from Wood et al. (in review).
Restoration informed by underlying patterns of genomic diversity and structure was recently implemented for one of the largest kelp restoration programs globally, Operation Crayweed1 (Wood et al., in review). Prior to restoration, population genetic diversity and structure of the endemic crayweed (P. comosa) was characterized throughout its entire distribution and within 180 km either side of the intended restoration areas (Figure 2; Coleman et al., 2008; Coleman and Kelaher, 2009; Wood et al., in review). Natural level of genetic diversity and structure were mimicked in restoration programs by sourcing and mixing adult donor plants from two sites that represented the genetic clusters that occur within an 80 km radius of where Phyllospora was lost (Figure 2; Wood et al., in review). This avoided mixing distant genetic clusters that were not representative of the region and was also a practical distance to ensure donor plant survival during transplantation. The success of this approach was evidenced by rapid recruitment and an F1 generation that had near identical genetic properties to donor plants and sites (Figure 2; Wood et al., in review). This is among the most successful restoration programs globally and there are now self-sustaining crayweed populations with likely F3–4 generations in some restored sites.
To ensure empirical genetic data is utilized to facilitate informed provenance decisions, data on genetic diversity and structure and their links to environmental conditions should be made publicly available to stakeholders and non-experts including community groups and governments who often implement restoration programs. For example, the restore and renew website for terrestrial plants2 (Rossetto et al., 2019) allows users to define a site to be restored, choose appropriate provenance within defined genetic populations and even provides provenance options to improve resilience (see “Revive – Restoring Extant or Historic Genetic Baselines”). No such platforms exist for marine systems but development of new marine restoration methods that will increase accessibility of marine restoration to diverse user groups and over large scales, will necessitate similar initiatives to ensure scientifically informed provenance decisions are made within the decade of restoration.
Dynamic Baselines – Why We Should Not Replicate What Was Lost
In rare cases baseline genetic data from lost or vulnerable populations are available and can theoretically be replicated. For example, rare “before” and “after” data has revealed massive change in baseline levels of genetic diversity and structure in three species of kelps that were impacted by a heatwave off Western Australia (Coleman et al., 2011b; Wernberg et al., 2018; Gurgel et al., 2020). Under a scenario of restoration that seeks to replicate what was lost, such “before” estimates can be used as a guide for provenance. This may be desirable where legislation dictates the necessity for a baseline (McAfee et al., 2019) or there are concerns surrounding genetic pollution (Potts et al., 2003). However, these studies have also revealed that genetic “baselines” are not static but are naturally dynamic properties of populations that can change rapidly (within a few months), due to redistribution of existing genetic variants through dispersal or selection (Torda et al., 2017; Gurgel et al., 2020).
This raises the important question of whether it is desirable to replicate exactly what was lost, or to embrace the dynamic nature of baselines and make informed decisions to reinforce or redefine them to an unknown future state (Figures 1, 3). Kelp loss and change in genetic baselines may naturally enhance resilience to future stress through selection. Thus, restoring kelp forests using past genetic baselines may, therefore, actually create populations that are more vulnerable to the events or stressors that caused loss in the first place providing a mechanistic basis and impetus for reinforcing as a restoration goal. Predicting future genetic response can be enhanced by the incorporation of neutral and functional genetic variation into species distribution models under projected future environmental scenarios (Bay et al., 2017; Razgour et al., 2019). Given naturally shifting baselines and environmental changes that are increasingly overwhelming the intrinsic capacity of organisms to adapt and survive in parts of their range (Deutsch et al., 2015; Segan et al., 2016) it is imperative to explore the potential to enhance or reinforce the resilience of ecosystems through restoration (Hobbs et al., 2017) as well as proactively reinforce resilience in habitats that will be threatened under future scenarios of change.
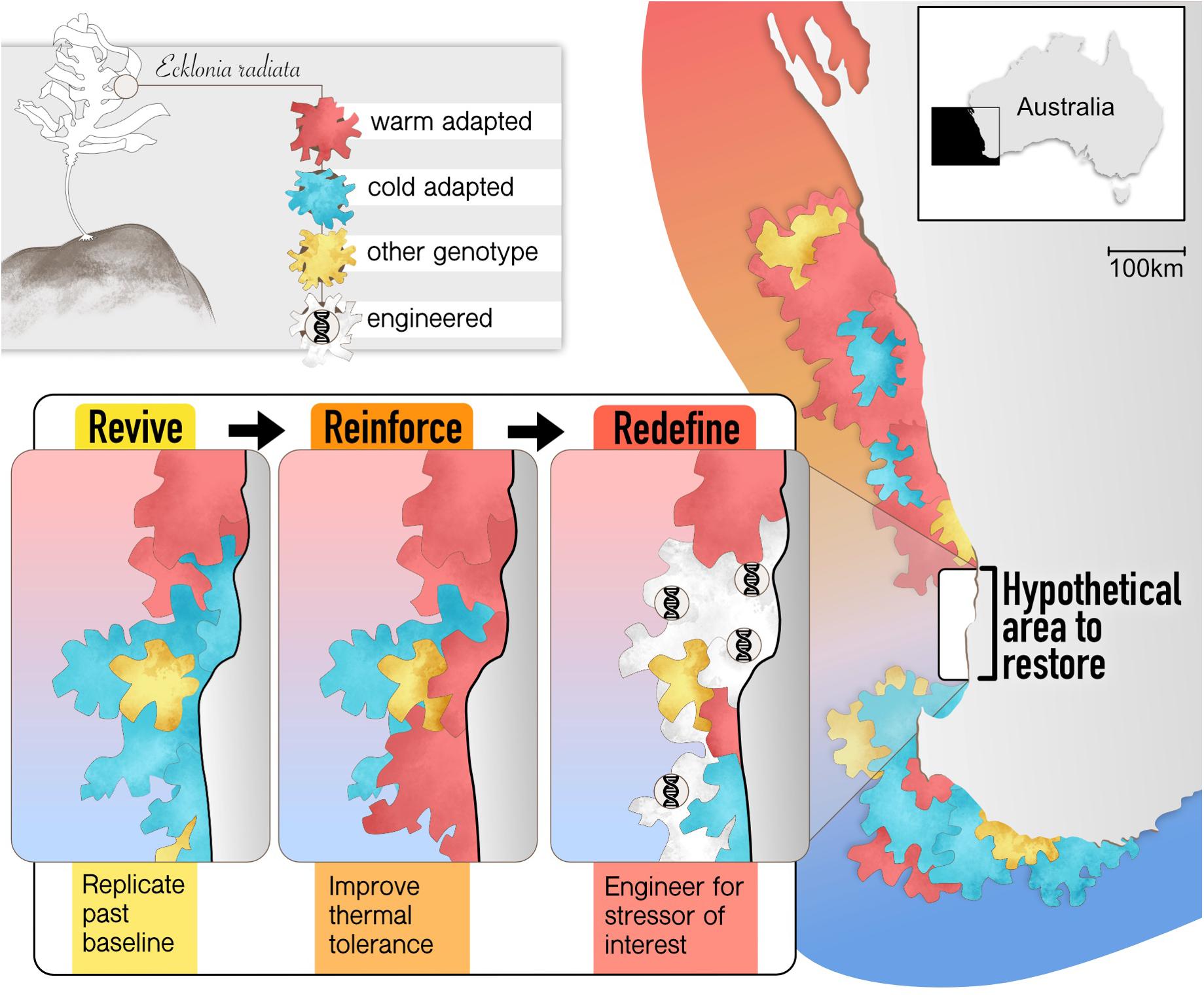
Figure 3. Potential scenarios for kelp restoration for a hypothetical area to be restored off Western Australia. Frequencies of putative warm (red) and cool (blue) adapted genotypes have been identified along a gradient of ocean temperature (shaded background) which could be utilized to revive or reinforce kelp forests against future thermal stress. Kelps with engineered genetic elements could one day be used to redefine the genetic baseline.
Reinforce – Improving Genetic Baselines for Future Conditions
Often termed “assisted adaptation” or “assisted evolution,” the idea of reinforcing genetic baselines is to introduce diversity or genotypes that will enhance resilience to future stressors in restored or threatened populations. While there are scientific and ethical challenges to adoption of such approaches (Coleman and Goold, 2019; Filbee-Dexter and Smajdor, 2019), the continued anthropogenic alteration of habitats and emergence of novel ecosystems place such interventions firmly at the forefront of restoration science.
The terms assisted adaptation or evolution capture numerous approaches for manipulating natural genetic properties of populations in order to increase their ability to adapt to changing environmental conditions. Such measures include moving resilient individuals to vulnerable populations to increase their capacity to resist or recover from disturbances (known as assisted gene flow or assisted migration; Figure 3). This could be achieved through targeted sourcing of donor plants using laboratory selection experiments or through identification of natural selection in the field (Zhang et al., 2011; Robinson et al., 2013; Gurgel et al., 2020). Another proposed strategy is “genetic rescue” whereby genetic diversity is enhanced in populations that have limited adaptive capacity, rather than entirely new genotypes introduced. Opting for boosting genetic diversity also reduces risks of negative fitness trade-offs by increasing the overall range of responses to various environmental conditions without aiming at improving one specific function. This approach has enhanced seagrass restoration success with greater productivity and biodiversity in experimental plots with increased genetic diversity (Reynolds et al., 2012). Similarly, higher genetic diversity in kelp forests may also confer greater resilience to climate stressors (Wernberg et al., 2018). Finally, resilience may be increased by utilizing intra-specific hybrid vigor or heterosis whereby crossing individuals from different populations (often not connected by contemporary gene flow) increases fitness relative to pure breds (e.g., Sexton et al., 2011), although this idea may be underpinned by mechanisms including increase in genetic diversity per se, addition of more resilient genes or epigenetic responses (Fujimoto et al., 2018).
Given the controversial nature of restoration strategies that seek to reinforce or improve extant genetic baselines, prioritization and careful selection of candidate species or sites is vital, as is experimentation to provide proof of concept that such strategies will work under a range of current and potential future stressors. It may be most appropriate to consider assisted adaptation in areas where species are already threatened or endangered, where projections of loss are severe or where impacts of loss will have widespread economic and ecological effects (e.g., foundation species; Baums, 2008; Aitken and Whitlock, 2013). Australian kelps meet all these criteria because a lack of poleward landmasses and warm currents along both coastlines create a unique scenario whereby species are locked into an ever narrowing thermal niche (Coleman et al., 2011b, 2017; Martínez et al., 2018). Projections for Australian kelps under climate change scenarios reveal an average loss of 78% of current distributions under the immediate RCP 6.0 scenario (Martínez et al., 2018), which may present logical targets for assisted adaptation and improving extant genetic baselines. This could be done through genomic identification of heritable loci under selection for certain stressors combined with manipulative stress experiments using multiple stressors that test the resilience of genotypes possessing such loci and assess potential trade-offs. Genotypes that perform well can then be cultured for enhanced seeding into restored populations (Figure 2; Weeks et al., 2011; Webster et al., 2017; Fredriksen et al., 2020). Given that such approaches, however, could lead to detrimental trade-offs (maladaptation) and decreased resilience to non-target stressors (Hereford, 2009; Anderson et al., 2014), a portfolio approach whereby assisted adaptation is paired with other approaches including enhancing diversity or connectivity, protecting a wide range of seascapes and minimizing stressors (Webster et al., 2017) may provide more security in uncertain futures.
Redefine – Create a Novel Genetic State
Scientific advances are providing never-before imagined solutions to emerging environmental problems, with synthetic biology and CRISPR/CAS9 gene editing tools among the fastest developing and transformative scientific fields (Lin and Qin, 2014; Wang et al., 2016; Piaggio et al., 2017). These technologies involve the creation of novel and engineered genetic variation that could be utilized in a restoration context to redefine extant genetic baselines and future resilience of species and populations to change (Figure 3; Coleman and Goold, 2019). The potential application of such technologies is vast, at times controversial, and technological advances have outpaced social, ethical and practical considerations. Here, we discuss some of the potential applications of synthetic biology and gene editing in restoration. Rather than advocate or oppose their use, we identify where and when they may play a role in restoration science. Regardless of whether these techniques will ever be socially acceptable or even necessary, this is a discussion that must be had early on.
Synthetic biology, or the engineered creation of novel genetic variation, is a fast developing and transformative scientific field. It can involve both genetic and metabolic engineering to create new functions in living cells or the creation of entirely new cells with synthetic components. Synthetic biology has been enabled through the decreasing costs of sequencing and synthesis of DNA, availability of extensive databases including information on sequences and functions, and the standardization of parts (genetic elements, proteins, organisms) which allows for increasing predictability in biological organisms, facilitating their use in a more designed approach as biological devices (Canton et al., 2008). Synthetic biology has also been enabled by development of the CRISPR/Cas9 genome editing tool (Pretorius, 2017) that cleaves viral double stranded DNA and allows for very precisely targeted changes to be made in a genome, as long as an organism is genetically tractable (Doudna and Charpentier, 2014). Examples of the use of CRISPR/Cas9 range from introducing a point mutation in a species genome to affect the quantity of metabolites (Shih et al., 2016), engineering speciation through designer karyotypes (Luo et al., 2018), or creating sequence specific anti-microbials through microbiome engineering (Bikard et al., 2014).
In conjunction with the availability of vast metagenomic data, it should be possible for scientists to map the genetic characteristics of resilient species or entire populations that are thriving despite stress, through natural and manipulative experiments. Resilient genetic elements could then help guide synthetic biology/genome engineering design principles. For example, traits from populations of marine organisms which have adapted to adverse conditions (e.g., polluted areas or warm range edges) could be introduced into related species or impacted populations to improve resilience to those stressors. The nature of these genetic elements could range from advantageous single nucleotide polymorphisms (SNPs; Doudna and Charpentier, 2014), to different genetic alleles/genes or even foreign/synthesized DNA (Williams et al., 2017), to larger duplications, inversions or deletions of entire chromosome arms (Luo et al., 2018). Synthetic biology could even be applied to restore extinct species. Such approaches are being considered in terrestrial contexts, with various groups attempting to resurrect extinct species (known as “de-extinction”), such as the great auk and the woolly mammoth (Corlett, 2017). Indeed, by extensive and rigorous bio-banking it may be possible, in the long term, to attempt to partially recreate extinct species and biomes using synthetic biology and store adaptive potential (Hodgins and Moore, 2016). Thus, to enable future restoration, bio-banking and ex situ conservation approaches should be promptly considered to allow the possibility for habitat recreation into the future.
Another technique that constitutes redefining extant genetic baselines is assisted evolution through inter-specific hybridization or heterosis. This utilizes the phenomenon of hybrid vigor, whereby species F1 hybrids display greater fitness than pure breds and is an emerging idea that has been suggested as a tool to enhance survival and persistence of foundation species under future climates (Rinkevich, 2014; van Oppen et al., 2015; Wood et al., 2019). In an assisted evolution context, inter-specific hybridization would be facilitated where it would otherwise not occur spatially, temporally (e.g., reproductive isolation) or within evolutionary time frames that would match the rapid pace of climate change. For example, hybrids of both kelp and coral have been shown to have greater thermal tolerance (Chan et al., 2018; Martins et al., 2019) which could be utilized in a restoration or assisted adaptation context that accepts redefining genetic baselines by mixing species gene pools.
At present, redefining extant genetic baselines in natural ecosystems is perhaps most palatable and ethically acceptable in the extreme case of stopping species extinction. Given the transformative nature and unpredictability of creating new genetic states, we suggest a starting point may be to prioritize species with little chance of persistence under future climate conditions. Again, Australian kelps provide the perfect example of such a situation because rapid warming, a narrowing thermal niche combined with high endemism (Phillips, 2001) create conditions that will see many species extinct within the next century (Martínez et al., 2018). For example, the endemic fucoid P. comosa (Coleman and Wernberg, 2017) is predicted to be completely lost by 2100 under future scenarios of climate change given extant temperature tolerances. Moreover, this species possesses a shallow history with low genetic diversity and structure (Coleman and Kelaher, 2009; Coleman et al., 2011a; Durrant et al., 2015), likely limiting its possible responses to change. Given that no other extant species seem to provide the same functions as P. comosa (Marzinelli et al., 2014, 2016), this warrants prompt discussion on the potential for genetic engineering of novel elements to boost thermal resilience as well as limit the effects of additional stressors (Coleman and Goold, 2019). Without such interventions, this key foundation species may be lost forever.
Assisted adaption, gene editing and hybridization raise complex ethical issues, which largely center on whether we should be deliberately introducing new genetic entities into natural ecosystems. From an ethical perspective, this shift in restoration focus from “revive” to “redefine” is significant (Camacho, 2010). Redefining genetic baselines can create problematic value judgments, such as prioritizing some species or properties over others, effectively deciding the winners and losers of the Anthropocene. These strategies to alter populations to withstand future stress also transform our role from guardians to engineers and designers of natural systems, which we do not fully comprehend, and can move ecosystems toward states that they have never been in before. As a result, we are determining the value of species and ecosystems based on the degree to which they match our current ideals of how things should be – targeting a more intact, familiar ecosystem that has been genetically manipulated to resist certain types of environmental stress, instead of an unpredictable and unfamiliar ecosystem that is transforming due to human activity. The use of adaptive or assisted evolution is also complicit with a worldview of environmental manipulation and commodification of natural systems that could perpetuate the damaging habits and dispositions which have caused the habitat loss in the first place (Sandler, 2013). Even if not morally wrong itself, adapted or assisted evolution may increase reliance on biotechnological intervention or even be used to justify continuing harmful practices in the future.
Conversely, inaction or passive decisions (i.e., not using all available tools to potentially save a species or habitat from disappearing) may also be unethical. If we have reason to predict that not acting will cause more harm than acting, then intervention seems to be the best course of action available. In an ideal world, we would reduce emissions and mitigate human impacts in time to remain in the “revive” space of conservation. Yet, we are in a state of crisis that we know will severely impact our environment and future generation (Gattuso et al., 2015). In light of this pressing reality, it could be argued that we have a moral responsibility to take risks we can reasonably predict will help to repair human-caused damage – as long as we are not reckless or negligent in doing so (Douglas, 2003). At a minimum, we should seek to thoroughly understand the potential impact of using all the tools available to us now, so that we will be in a position to choose these options should some catastrophic scenario arise in the future (e.g., “arm the future argument” outlined by Gardiner, 2010).
At a more practical level, there are several first steps we can take toward including ethical considerations in decisions to use assisted evolution tools. First, we can ensure that a minimum standard of risk assessment of potential impact on the environment is conducted. This could include controlled manipulative experiments on novel or engineered genotypes to assess their performance and interactions in natural settings. Second, that informed consent of stakeholders is obtained and that no conflicts of interest exist in the relationships between researchers/managers and local communities. Finally, we can develop policy and guidelines for the use of these tools in specific systems. Regardless, there is a pressing need for prompt collaboration and dialogue among geneticists, synthetic biologists, ecologists, and conservationists to identify opportunities for use of these transformative technologies and ensure that extant research directions are set on trajectories to allow these currently disparate fields to converge toward practical restoration solutions. While the application of such techniques to natural settings is currently controversial (Filbee-Dexter and Smajdor, 2019) they should remain at the forefront of discussions to future-proof marine ecosystems and restoration practices (Coleman and Goold, 2019).
Restore or Redefine: a Broadly Applicable Framework
While we have focused here on kelp forests, the framework presented is broadly applicable to any marine or terrestrial restoration program. Indeed, it may even be more easily applied and adopted for restoration of species that are more genetically tractable than kelps. Genomic techniques might be more rapidly developed in taxa for which DNA extraction and subsequent sequencing techniques are more easily applied. Further, genomic resources are more developed for key model species such as corals, making progress toward revive and redefine more tangible. Indeed, genetics and provenance are emerging considerations in coral reef restoration guidelines (Baums et al., 2019) and standard practices that are easily accessible to managers and practitioners (e.g., www.reefresilience.org). For such taxa, progress toward empirically incorporating appropriate genetic baselines to restoration programs should be more rapid. The goal to redefine genetic baselines in restoration programs may also be more tangible for taxa that have direct economic value (e.g., harvested species), where breeding programs often involve detailed genomic assessments linking performance and traits to environmental conditions. Regardless, restoration of foundation macrophytes (e.g., saltmarsh, seagrass, mangroves) and animals (e.g., oyster beds, corals) that underpin vast biodiversity of marine systems is gaining increasing traction, funding and sophistication with great potential toward achieving the aims of the United Nations decade of restoration.
Conclusion
Whether to recover and restore historic and extant genetic baselines, or reinforce and redefine them to some unknown future state, will fundamentally affect the resilience and adaptive capacity of restored populations (Hobbs et al., 2009; van Oppen et al., 2015; Breed et al., 2018, 2019; Wood et al., 2019) but is largely untested for marine systems. Here, we discuss the application of both traditional and modern genomic tools for characterizing (e.g., genotyping by sequencing) and manipulating (CRISPR/Cas9) the genetic composition of lost or degraded marine habitats. The increasing accessibility of these genomic techniques means that future marine restoration efforts can, and should, proceed with the best available genetic data and technologies. At a minimum, baseline empirical genetic data should inform provenance decisions and, where acceptable, incorporate assisted adaptation strategies.
More broadly, it is clear that restoration in the traditional sense of returning a system to a past state, is unlikely to be sufficient or effective under future climates (van Oppen et al., 2017). Instead, restoration should seek to reinforce and perhaps even redefine populations and species to withstand future environmental conditions and stressors. However, this raises profound and challenging management (to what baseline should we “restore?”), technical (how do we achieve that baseline in a practical sense?) and ethical (what right do we have to introduce novel genetic entities into the marine environment?) questions that will determine our ongoing relationship with nature. We argue for a worldwide move among marine managers and scientists toward prompt consideration of more interventionist approaches. The failure to consider and prepare for such approaches, despite ethical debates, is also an ethical decision with potentially serious environmental consequences of inaction.
Marine restoration will benefit from learnings from the history of biomedical fields, where technological developments and associated benefits have often outstripped the social and ethical dialogue necessary for implementation. Prompt dialogue is thus required among scientists, policy makers and the broader community on setting restoration targets, including the increasing need to restore for future conditions and the implications of using novel or engineered genetic entities (Coleman and Goold, 2019). Scientific agendas should be set on trajectories to provide the underpinnings for such decisions. Only then can we ensure that our valuable marine habitats continue to deliver ecosystem goods and services in the face of increasing environmental change.
Author Contributions
MC and AM conceived the idea and led the manuscript. All authors wrote sections and edited the manuscript. AM produced the figures.
Funding
This work was funded through the Australian Research Council Grants to TW, MC, and KF-D (DP190100058), KF-D (DE190100692), and PS, EM, AV, and MC (LP150100064). HG is embedded at the Synthetic Biology initiative at Macquarie University and is financially supported by an internal grant from the University and external grants from Bioplatforms Australia, the New South Wales (NSW) Chief Scientist and Engineer, and NSW Department of Primary Industries.
Conflict of Interest
The authors declare that the research was conducted in the absence of any commercial or financial relationships that could be construed as a potential conflict of interest.
Footnotes
References
Aitken, S. N., and Whitlock, M. C. (2013). Assisted gene flow to facilitate local adaptation to climate change. Annu. Rev. Ecol. Evol. Systemat. 44, 367–388. doi: 10.1146/annurev-ecolsys-110512-135747
Alberto, F., Raimondi, P. T., Reed, D. C., Coelho, N. C., Leblois, R., Whitmer, A., et al. (2010). Habitat continuity and geographic distance predict population genetic differentiation in giant kelp. Ecology 91, 49–56. doi: 10.1890/09-0050.1
Anderson, J. T., Lee, C.-R., and Mitchell-Olds, T. (2014). Strong selection genome-wide enhances fitness trade-offs across environments and episodes of selection. Evolution 68, 16–31. doi: 10.1111/evo.12259
Baums, I. B. (2008). A restoration genetics guide for coral reef conservation. Mol. Ecol. 17, 2796–2811. doi: 10.1111/j.1365-294x.2008.03787.x
Baums, I. B., Baker, A. C., Davies, S. W., Grottoli, A. G., Kenkel, C. D., Kitchen, S. A., et al. (2019). Considerations for maximizing the adaptive potential of restored coral populations in the western Atlantic. Ecol. Appl. 29, e01978.
Bay, R. A., Rose, N., Barrett, R., Bernatchez, L., Ghalambor, C. K., Lasky, J. R., et al. (2017). Predicting responses to contemporary environmental change using evolutionary response architectures. Am. Nat. 189, 463–473. doi: 10.1086/691233
Bikard, D., Euler, C. W., Jiang, W., Nussenzweig, P. M., Goldberg, G. W., Duportet, X., et al. (2014). Exploiting crispr-cas nucleases to produce sequence-specific antimicrobials. Nat. Biotechnol. 32, 1146–1150. doi: 10.1038/nbt.3043
Bischoff, A., Steinger, T., and Müller-Schärer, H. (2010). The importance of plant provenance and genotypic diversity of seed material used for ecological restoration. Restor. Ecol. 18, 338–348. doi: 10.1111/j.1526-100x.2008.00454.x
Breed, M. F., Harrison, P. A., Bischoff, A., Durruty, P., Gellie, N. J. C., Gonzales, E. K., et al. (2018). Priority actions to improve provenance decision-making. BioScience 68, 510–516. doi: 10.1093/biosci/biy050
Breed, M. F., Harrison, P. A., Blyth, C., Byrne, M., Gaget, V., Gellie, N. J. C., et al. (2019). The potential of genomics for restoring ecosystems and biodiversity. Nat. Rev. Genet. 20, 615–628. doi: 10.1038/s41576-019-0152-0
Camacho, A. E. (2010). Assisted migration: redefining nature and natural resource law under climate change. Yale J. Regul. 27, 171.
Campbell, A. H., Marzinelli, E. M., Verges, A., Coleman, M. A., and Steinberg, P. D. (2014). Towards restoration of missing underwater forests. PLoS ONE 9:e84106. doi: 10.1371/journal.pone.0084106
Canton, B., Labno, A., and Endy, D. (2008). Refinement and standardization of synthetic biological parts and devices. Nat. Biotechnol. 26, 787–793. doi: 10.1038/nbt1413
Carlisle, J. G., Turner, C. H., and Ebert, E. E. (1964). Artificial Habitat in the Marine Environment. Sacramento, CA: Resources Agency of California.
Carnell, P. E., and Keough, M. J. (2019). Reconstructing historical marine populations reveals major decline of a kelp forest ecosystem in Australia. Estuar. Coasts 42, 765–778. doi: 10.1007/s12237-019-00525-1
Chan, W. Y., Peplow, L. M., Menéndez, P., Hoffmann, A. A., and van Oppen, M. J. H. (2018). Interspecific hybridization may provide novel opportunities for coral reef restoration. Front. Mar. Sci. 5:160. doi: 10.3389/fmars.2018.00160
Coleman, M. A., and Brawley, S. H. (2005). Spatial and temporal variability in dispersal and population genetic structure of a rockpool alga. Mar. Ecol. Prog. Ser. 300, 63–77. doi: 10.3354/meps300063
Coleman, M. A., Cetina-Heredia, P., Roughan, M., Feng, M., Sebille, E., and Kelaher, B. P. (2017). Anticipating changes to future connectivity within a network of marine protected areas. Global Change Biol. 23, 3533–3542. doi: 10.1111/gcb.13634
Coleman, M. A., Chambers, J., Knott, N. A., Malcolm, H. A., Harasti, D., Jordan, A., et al. (2011a). Connectivity within and among a network of temperate marine reserves. PLoS ONE 6:e20168. doi: 10.1371/journal.pone.0020168
Coleman, M. A., Roughan, M., Macdonald, H. S., Connell, S. D., Gillanders, B. M., Kelaher, B. P., et al. (2011b). Variation in the strength of continental boundary currents determines continent-wide connectivity in kelp. J. Ecol. 99, 1026–1032. doi: 10.1111/j.1365-2745.2011.01822.x
Coleman, M. A., Clark, J. S., Doblin, M. A., Bishop, M. J., and Kelaher, B. P. (2019). Genetic differentiation between estuarine and open coast ecotypes of a dominant ecosystem engineer. Mar. Freshw. Res. 70, 977–985.
Coleman, M. A., Feng, M., Roughan, M., Cetina-Heredia, P., and Connell, S. D. (2013). Temperate shelf water dispersal by Australian boundary currents: Implications for population connectivity. Limnol. Oceanogr. Fluids Environ. 3, 295–309. doi: 10.1215/21573689-2409306
Coleman, M. A., and Goold, H. (2019). Harnessing synthetic biology for kelp forest conservation. J. Phycol. 55, 745–751. doi: 10.1111/jpy.12888
Coleman, M. A., and Kelaher, B. P. (2009). Connectivity among fragmented populations of a habitat-forming alga, Phyllospora comosa (Phaeophyceae, Fucales) on an urbanised coast. Mar. Ecol. Prog. Ser. 381, 63–70. doi: 10.3354/meps07977
Coleman, M. A., Kelaher, B. P., Steinberg, P. D., and Millar, A. J. K. (2008). Absence of a large brown macroalga on urbanized rocky reefs around Sydney, Australia, and evidence for historical decline. J. Phycol. 44, 897–901. doi: 10.1111/j.1529-8817.2008.00541.x
Coleman, M. A., and Wernberg, T. (2017). Forgotten underwater forests: the key role of fucoids on Australian temperate reefs. Ecol. Evol. 7, 8406–8418. doi: 10.1002/ece3.3279
Coleman, M. A., and Wernberg, T. (2018). Genetic and morphological diversity in sympatric kelps with contrasting reproductive strategies. Aquat. Biol. 27, 65–73. doi: 10.3354/ab00698
Connell, S. D., Russell, B. D., Turner, D. J., Shepherd, S. A., Kildea, T., Miller, D., et al. (2008). Recovering a lost baseline: missing kelp forests from a metropolitan coast. Mar. Ecol. Prog. Ser. 360, 63–72. doi: 10.3354/meps07526
Corlett, R. T. (2017). A bigger toolbox: biotechnology in biodiversity conservation. Trends Biotechnol. 35, 55–65. doi: 10.1016/j.tibtech.2016.06.009
Crouzeilles, R., Curran, M., Ferreira, M. S., Lindenmayer, D. B., Grelle, C. E. V., and Rey Benayas, J. M. (2016). A global meta-analysis on the ecological drivers of forest restoration success. Nat. Commun. 7:11666.
DeAngelis, M. B., Sutton-Grier, E. A., Colden, A., Arkema, K. K., Baillie, J. C., Bennett, O. R., et al. (2020). Social factors key to landscape-scale coastal restoration: lessons learned from three U.S. case studies. Sustainability 12:869. doi: 10.3390/su12030869
Deutsch, C., Ferrel, A., Seibel, B., Pörtner, H., and Huey, R. (2015). Climate change tightens a metabolic constraint on marine habitats. Science 348, 1132–1135. doi: 10.1126/science.aaa1605
Doudna, J. A., and Charpentier, E. (2014). The new frontier of genome engineering with Crispr-Cas9. Science 346, 1258096. doi: 10.1126/science.1258096
Durrant, H. M. S., Barrett, N. S., Edgar, G. J., Coleman, M. A., and Burridge, C. P. (2015). Shallow phylogeographic histories of key species in a biodiversity hotspot. Phycologia 54, 556–565. doi: 10.2216/15-24.1
Durrant, H. M. S., Barrett, N. S., Edgar, G. J., Coleman, M. A., and Burridge, C. P. (2018). Seascape habitat patchiness and hydrodynamics explain genetic structuring of kelp populations. Mar. Ecol. Prog. Ser. 587, 81–92. doi: 10.3354/meps12447
Durrant, H. M. S., Burridge, C. P., Kelaher, B. P., Barrett, N. S., Edgar, G. J., and Coleman, M. A. (2014). Implications of macroalgal isolation by distance for networks of marine protected areas. Conserv. Biol. 28, 438–445. doi: 10.1111/cobi.12203
Eger, A. M., Marzinelli, E. M., Steinberg, P. D., and Vergés, A. (2019). Open Science Framework Portal (OSF). Available online at: osf.io/5bgtw
Evans, S. M., Sinclair, E. A., Poore, A. G. B., Bain, K. F., and Vergés, A. (2018). Assessing the effect of genetic diversity on the early establishment of the threatened seagrass Posidonia australis using a reciprocal-transplant experiment. Restor. Ecol. 26, 570–580. doi: 10.1111/rec.12595
FAO (2019). New United Nations Decade on Ecosystem Restoration Offers Unparalleled Opportunity for Job Creation, Food Security and Addressing Climate Change. Available online at: http://www.fao.org/news/story/en/item/1182090/icode/ (accessed December 3, 2019).
Filbee-Dexter, K., and Scheibling, R. E. (2014). Sea urchin barrens as alternative stable states of collapsed kelp ecosystems. Mar. Ecol. Prog. Ser. 495, 1–25. doi: 10.3354/meps10573
Filbee-Dexter, K., and Smajdor, A. (2019). Ethics of assisted evolution in marine conservation. Front. Mar. Sci. 6:20. doi: 10.3389/fmars.2019.00020
Filbee-Dexter, K., and Wernberg, T. (2018). Rise of turfs: a new battlefront for globally declining kelp forests. BioScience 68, 64–76. doi: 10.1093/biosci/bix147
Fredriksen, S., Filbee-Dexter, K., Norderhaug, K. M., Steen, H., Bodvin, T., Coleman, M. A., et al. (2020). Green gravel: a novel restoration tool to combat kelp forest decline. Sci. Rep. 10:3983.
Fujimoto, R., Uezono, K., Ishikura, S., Osabe, K., Peacock, W. J., and Dennis, E. S. (2018). Recent research on the mechanism of heterosis is important for crop and vegetable breeding systems. Breed. Sci. 68, 145–158. doi: 10.1270/jsbbs.17155
Gardiner, S. (2010). “Is’ arming the future’with geoengineering really the lesser evil? Some doubts about the ethics of intentionally manipulating the climate system,” in Climate Ethics: Essential Readings, eds S. Gardiner, S. Caney, D. Jamieson, and H. Shue (Oxford: Oxford University Press), 30.
Gattuso, J.-P., Magnan, A., Billé, R., Cheung, W. W. L., Howes, E. L., Joos, F., et al. (2015). Contrasting futures for ocean and society from different anthropogenic Co2 emissions scenarios. Science 349:aac4722. doi: 10.1126/science.aac4722
Gaylord, B., Reed, D. C., Raimondi, P. T., and Washburn, L. (2006). Macroalgal spore dispersal in coastal environments: mechanistic insights revealed by theory and experiment. Ecol. Monogr. 76, 481–502. doi: 10.1890/0012-9615(2006)076
Grant, P. R., Grant, B. R., Huey, R. B., Johnson, M. T. J., Knoll, A. H., and Schmitt, J. (2017). Evolution caused by extreme events. Philos. Trans. R. Soc. B-Biol. Sci. 372:20160146.
Guillemin, M.-L., Faugeron, S., Destombe, C., Viard, F., Correa, J. A., and Valero, M. (2008). Genetic variation in wild and cultivated populations of the haploid– diploid red alga gracilaria chilensis: how farming practices favor asexual reproduction and heterozygosity. Evolution 62, 1500–1519. doi: 10.1111/j.1558-5646.2008.00373.x
Gurgel, C. F. D., Camacho, O., Minne, A. J. P., Wernberg, T., and Coleman, M. A. (2020). Marine heatwave drives cryptic loss of genetic diversity in underwater forests. Curr. Biol. [Epub ahead of print].
Hereford, J. (2009). A quantitative survey of local adaptation and fitness trade-offs. Am. Nat. 173, 579–588. doi: 10.1086/597611
Hobbs, R. J., Higgs, E., and Harris, J. A. (2009). Novel ecosystems: implications for conservation and restoration. Trends Ecol. Evol. 24, 599–605. doi: 10.1016/j.tree.2009.05.012
Hobbs, R. J., Higgs, E. S., and Hall, C. M. (2017). Expanding the portfolio: conserving nature’s masterpieces in a changing world. BioScience 67, 568–575. doi: 10.1093/biosci/bix043
Hodgins, K. A., and Moore, J. L. (2016). Adapting to a warming world: Ecological restoration, climate change, and genomics. Am. J. Bot. 103, 590–592. doi: 10.3732/ajb.1600049
Hoffmann, A., Griffin, P., Dillon, S., Catullo, R., Rane, R., Byrne, M., et al. (2015). A framework for incorporating evolutionary genomics into biodiversity conservation and management. Clim. Change Responses 2:1.
Hoffmann, M., Hilton-Taylor, C., Angulo, A., Böhm, M., Brooks, T. M., Butchart, S. H. M., et al. (2010). The impact of conservation on the status of the world’s vertebrates. Science 330:1503.
Hughes, T. P., Barnes, M. L., Bellwood, D. R., Cinner, J. E., Cumming, G. S., Jackson, J. B. C., et al. (2017). Coral reefs in the Anthropocene. Nature 546, 82–90.
IPCC (2019). Summary for Policymakers. Ipcc Special Report on the Ocean and Cryosphere in a Changing Climate. Geneva: IPCC.
Krumhansl, K. A., Okamoto, D. K., Rassweiler, A., Novak, M., Bolton, J. J., Cavanaugh, K. C., et al. (2016). Global patterns of kelp forest change over the past half-century. Proc. Natl. Acad. Sci. U.S.A. 113, 13785–13790.
Kuwahara, H., Watanuki, A., Aota, T., Ando, W., Kawai, T., Terawaki, T., et al. (2006). Trends in literature on seaweed restoration techniques on barren grounds in Japan. J. Fish. Eng. 43, 81–87.
Layton, C., Coleman, M. A., Marzinelli, E. M., Steinberg, P. D., Swearer, S. E., Vergés, A., et al. (2020). Kelp forest restoration in Australia. Front. Mar. Sci. 7:74. doi: 10.3389/fmars.2020.00074
Lee, S. G. (2019). “Marine stock enhancement, restocking, and sea ranching in Korea,” in Wildlife Management – Failures, Successes and Prospects, eds J. R. Kideghesho and A. A. Rija (London: IntechOpen).
Lin, H., and Qin, S. (2014). Tipping points in seaweed genetic engineering: scaling up opportunities in the next decade. Mar. Drugs 12, 3025–3045. doi: 10.3390/md12053025
Ling, S. D., Johnson, C. R., Frusher, S. D., and Ridgway, K. R. (2009). Overfishing reduces resilience of kelp beds to climate-driven catastrophic phase shift. Proc. Natl. Acad. Sci. U.S.A. 106, 22341–22345. doi: 10.1073/pnas.0907529106
Ling, S. D., Scheibling, R. E., Rassweiler, A., Johnson, C. R., Shears, N., Connell, S. D., et al. (2015). Global regime shift dynamics of catastrophic sea urchin overgrazing. Philos. Trans. R. Soc. B Biol. Sci. 370, 20130269. doi: 10.1098/rstb.2013.0269
Luo, J., Sun, X., Cormack, B. P., and Boeke, J. D. (2018). Karyotype engineering by chromosome fusion leads to reproductive isolation in yeast. Nature 560, 392–396. doi: 10.1038/s41586-018-0374-x
Martínez, B., Radford, B., Thomsen, M. S., Connell, S. D., Carreño, F., Bradshaw, C. J. A., et al. (2018). Distribution models predict large contractions of habitat-forming seaweeds in response to ocean warming. Divers. Distribut. 24, 1350–1366. doi: 10.1111/ddi.12767
Martins, N., Pearson, G. A., Gouveia, L., Tavares, A. I., Serrão, E. A., and Bartsch, I. (2019). Hybrid vigour for thermal tolerance in hybrids between the allopatric kelps Laminaria digitata and L. pallida (Laminariales, Phaeophyceae) with contrasting thermal affinities. Eur. J. Phycol. 54, 548–561. doi: 10.1080/09670262.2019.1613571
Marzinelli, E. M., Campbell, A. H., Verges, A., Coleman, M. A., Kelaher, B. P., and Steinberg, P. D. (2014). Restoring seaweeds: does the declining fucoid Phyllospora comosa support different biodiversity than other habitats? J. Appl. Phycol. 26, 1089–1096. doi: 10.1007/s10811-013-0158-5
Marzinelli, E. M., Leong, M. R., Campbell, A. H., Steinberg, P. D., and Verges, A. (2016). Does restoration of a habitat-forming seaweed restore associated faunal diversity? Restor. Ecol. 24, 81–90. doi: 10.1111/rec.12292
McAfee, D., Doubleday, Z. A., Geiger, N., and Connell, S. D. (2019). Everyone loves a success story: optimism inspires conservation engagement. BioScience 69, 274–281. doi: 10.1093/biosci/biz019
McKay, J. K., Christian, C. E., Harrison, S., and Rice, K. J. (2005). “How local is local?”—A review of practical and conceptual issues in the genetics of restoration. Restor. Ecol. 13, 432–440. doi: 10.1111/j.1526-100x.2005.00058.x
Mijangos, J. L., Pacioni, C., Spencer, P. B. S., and Craig, M. D. (2015). Contribution of genetics to ecological restoration. Mol. Ecol. 24, 22–37. doi: 10.1111/mec.12995
Miller, A., Coleman, M., Clark, J., Cook, R., Naga, Z., Doblin, M., et al. (2019). Local thermal adaptation and limited gene flow constrain future climate responses of a marine ecosystem engineer. Evol. Appl. 1–17.
Narum, S. R., Buerkle, C. A., Davey, J. W., Miller, M. R., and Hohenlohe, P. A. (2013). Genotyping-by-sequencing in ecological and conservation genomics. Mol. Ecol. 22, 2841–2847. doi: 10.1111/mec.12350
North, W. J. (1976). Aquacultural techniques for creating and restoring beds of giant kelp, Macrocystis spp. J. Fish. Res. Board Canada 33, 1015–1023. doi: 10.1139/f76-129
Perring, M. P., Standish, R. J., Price, J. N., Craig, M. D., Erickson, T. E., Ruthrof, K. X., et al. (2015). Advances in restoration ecology: rising to the challenges of the coming decades. Ecosphere 6:art131. doi: 10.1890/es15-00121.1
Phillips, J. A. (2001). Marine macroalgal biodiversity hotspots: why is there high species richness and endemism in southern Australian marine benthic flora? Biodivers. Conserv. 10, 1555–1577.
Piaggio, A. J., Segelbacher, G., Seddon, P. J., Alphey, L., Bennett, E. L., Carlson, R. H., et al. (2017). Is it time for synthetic biodiversity conservation? Trends Ecol. Evol. 32, 97–107.
Popkin, G. (2018). Can a transgenic chestnut restore a forest icon? Science 361, 830–831. doi: 10.1126/science.361.6405.830
Potts, B. M., Barbour, R. C., Hingston, A. B., and Vaillancourt, R. E. (2003). Genetic pollution of native eucalypt gene pools-identifying the risks. Austr. J. Bot. 51, 1–25.
Powers, R. P., and Jetz, W. (2019). Global habitat loss and extinction risk of terrestrial vertebrates under future land-use-change scenarios. Nat. Clim. Change 9, 323–329. doi: 10.1038/s41558-019-0406-z
Pretorius, I. S. (2017). Synthetic genome engineering forging new frontiers for wine yeast. Crit. Rev. Biotechnol. 37, 112–136. doi: 10.1080/07388551.2016.1214945
Ralls, K., Ballou, J. D., Dudash, M. R., Eldridge, M. D. B., Fenster, C. B., Lacy, R. C., et al. (2018). Call for a paradigm shift in the genetic management of fragmented populations. Conserv. Lett. 11:e12412. doi: 10.1111/conl.12412
Razgour, O., Forester, B., Taggart, J. B., Bekaert, M., Juste, J., Ibáñez, C., et al. (2019). Considering adaptive genetic variation in climate change vulnerability assessment reduces species range loss projections. Proc. Natl. Acad. Sci. U.S.A. 116, 10418–10423. doi: 10.1073/pnas.1820663116
Reynolds, L. K., Mcglathery, K. J., and Waycott, M. (2012). Genetic diversity enhances restoration success by augmenting ecosystem services. PLoS ONE 7:e38397. doi: 10.1371/journal.pone.0038397
Rinkevich, B. (2014). Rebuilding coral reefs: does active reef restoration lead to sustainable reefs? Curr. Opin. Environ. Sustain. 7, 28–36. doi: 10.1016/j.cosust.2013.11.018
Rinkevich, B. (2019). Coral chimerism as an evolutionary rescue mechanism to mitigate global climate change impacts. Global Change Biol. 25, 1198–1206. doi: 10.1111/gcb.14576
Robinson, N., Winberg, P., and Kirkendale, L. (2013). Genetic improvement of macroalgae: status to date and needs for the future. J. Appl. Phycol. 25, 703–716. doi: 10.1007/s10811-012-9950-x
Rossetto, M., Bragg, J., Kilian, A., Mcpherson, H., Van Der Merwe, M., and Wilson, P. D. (2019). Restore and Renew: a genomics-era framework for species provenance delimitation. Restor. Ecol. 27, 538–548. doi: 10.1111/rec.12898
Sanderson, J. C., Ling, S. D., Dominguez, J. G., and Johnson, C. R. (2015). Limited effectiveness of divers to mitigate ‘barrens’ formation by culling sea urchins while fishing for abalone. Mar. Freshw. Res. 67, 84–95.
Sandler, R. L. (2013). “Environmental virtue ehtics,” in Internatioal Encyclopedia of Ethics, ed. H. Lafollette (Oxford: Blackwell Publishing).
Schroeter, S. C., Reed, D. C., and Raimondi, P. (2018). Artificial reefs to mitigate human impacts in the marine environment: the Wheeler North Reef as a test case. Am. Fish. Soc. Symp. 86, 197–213.
Segan, D., Murray, K., and Watson, J. (2016). A global assessment of current and future biodiversity vulnerability to habitat loss–climate change interactions. Glob. Ecol. Conserv. 5, 12–21. doi: 10.1016/j.gecco.2015.11.002
Sera. (2017). National Standards for the Practice of Ecological Restorationin Australia, Second Edn. New South Wales: Society for Ecological Restoration Australasia.
Sexton, J. P., Strauss, S. Y., and Rice, K. J. (2011). Gene flow increases fitness at the warm edge of a species’ range. Proc. Natl. Acad. Sci. U.S.A. 108, 11704–11709. doi: 10.1073/pnas.1100404108
Sgrò, C. M., Lowe, A. J., and Hoffmann, A. A. (2011). Building evolutionary resilience for conserving biodiversity under climate change. Evol. Appl. 4, 326–337. doi: 10.1111/j.1752-4571.2010.00157.x
Shih, P. M., Vuu, K., Mansoori, N., Ayad, L., Louie, K. B., Bowen, B. P., et al. (2016). A robust gene-stacking method utilizing yeast assembly for plant synthetic biology. Nat. Commun. 7:13215.
Torda, G., Donelson, J. M., Aranda, M., Barshis, D. J., Bay, L., Berumen, M. L., et al. (2017). Rapid adaptive responses to climate change in corals. Nat. Clim. Change 7, 627–636. doi: 10.1038/nclimate3374
van Oppen, M. J. H., Gates, R. D., Blackall, L. L., Cantin, N., Chakravarti, L. J., Chan, W. Y., et al. (2017). Shifting paradigms in restoration of the world’s coral reefs. Glob. Change Biol. 23, 3437–3448.
van Oppen, M. J. H., Oliver, J. K., Putnam, H. M., and Gates, R. D. (2015). Building coral reef resilience through assisted evolution. Proc. Natl. Acad. Sci. U.S.A. 112, 2307–2313. doi: 10.1073/pnas.1422301112
Venter, O., Sanderson, E. W., Magrach, A., Allan, J. R., Beher, J., Jones, K. R., et al. (2016). Sixteen years of change in the global terrestrial human footprint and implications for biodiversity conservation. Nat. Commun. 7:12558.
Verdura, J., Sales, M., Ballesteros, E., Cefalì, M. E., and Cebrian, E. (2018). Restoration of a canopy-forming alga based on recruitment enhancement: methods and long-term success assessment. Front. Plant Sci. 9:1832. doi: 10.3389/fpls.2018.01832
Vergés, A., Doropoulos, C., Malcolm, H. A., Skye, M., Garcia-Pizá, M., Marzinelli, E. M., et al. (2016). Long-term empirical evidence of ocean warming leading to tropicalization of fish communities, increased herbivory, and loss of kelp. Proc. Natl. Acad. Sci. U.S.A. 113, 13791–13796. doi: 10.1073/pnas.1610725113
Vergés, A., Steinberg, P. D., Hay, M. E., Poore, A. G. B., Campbell, A. H., Ballesteros, E., et al. (2014). The tropicalization of temperate marine ecosystems: climate-mediated changes in herbivory and community phase shifts. Proc. R. Soc. B-Biol. Sci. 281∗Google Scholar
Wang, H., La Russa, M., and Qi, L. S. (2016). Crispr/Cas9 in genome editing and beyond. Annu. Rev. Biochem. 85, 227–264.
Webster, M. S., Colton, M. A., Darling, E. S., Armstrong, J., Pinsky, M. L., Knowlton, N., et al. (2017). Who should pick the winners of climate change? Trends Ecol. Evol. 32, 167–173. doi: 10.1016/j.tree.2016.12.007
Weeks, A. R., Sgro, C. M., Young, A. G., Frankham, R., Mitchell, N. J., Miller, K. A., et al. (2011). Assessing the benefits and risks of translocations in changing environments: a genetic perspective. Evol. Appl. 4, 709–725. doi: 10.1111/j.1752-4571.2011.00192.x
Wernberg, T., Bennett, S., Babcock, R. C., De Bettignies, T., Cure, K., Depczynski, M., et al. (2016). Climate-driven regime shift of a temperate marine ecosystem. Science 353, 169–172. doi: 10.1126/science.aad8745
Wernberg, T., Coleman, M., Babcock, R. B., Sy, Bolton, J., Connell, S., et al. (2019a). “Biology and ecology of the globally significant kelp Ecklonia radiata,” in Oceanography and Marine Biology: An Annual Review, eds M. Barnes, R. N. Gibson, and R. N. Gibson (Boca Raton, FL: CRC Press).
Wernberg, T., Krumhansl, K. A., Filbee-Dexter, K., and Pedersen, M. (2019b). “Status and trends for the world’s kelp forests,” in World Seas: An Environmental Evaluation, ed. C. Sheppard (London: Elsivier).
Wernberg, T., Coleman, M. A., Bennett, S., Thomsen, M. S., Tuya, F., and Kelaher, B. P. (2018). Genetic diversity and kelp forest vulnerability to climatic stress. Sci. Rep. 8:1851.
Wernberg, T., and Filbee-Dexter, K. (2019). Missing the marine forest for the trees. Mar. Ecol. Prog. Ser. 612, 209–215. doi: 10.3354/meps12867
Williams, S. L. (2001). Reduced genetic diversity in eelgrass transplantations affects both population growth and individual fitness. Ecol. Appl. 11, 1472–1488. doi: 10.1890/1051-0761(2001)011
Williams, T. C., Xu, X., Ostrowski, M., Pretorius, I. S., and Paulsen, I. T. (2017). Positive-feedback, ratiometric biosensor expression improves high-throughput metabolite-producer screening efficiency in yeast. Synth. Biol. 2:ysw002.
Wood, G., Marzinelli, E. M., Coleman, M. A., Campbell, A. H., Santini, N. S., Kajlich, L., et al. (2019). Restoring subtidal marine macrophytes in the Anthropocene: trajectories and future-proofing. Mar. Freshw. Res. 70, 936–951.
Keywords: assisted adaptation, provenance, kelp, climate change, evolution, synthetic biology
Citation: Coleman MA, Wood G, Filbee-Dexter K, Minne AJP, Goold HD, Vergés A, Marzinelli EM, Steinberg PD and Wernberg T (2020) Restore or Redefine: Future Trajectories for Restoration. Front. Mar. Sci. 7:237. doi: 10.3389/fmars.2020.00237
Received: 03 December 2019; Accepted: 26 March 2020;
Published: 17 April 2020.
Edited by:
Brian Silliman, Duke University, United StatesReviewed by:
Andrew M. Fischer, University of Tasmania, AustraliaMatthew David Tietbohl, King Abdullah University of Science and Technology, Saudi Arabia
Copyright © 2020 Coleman, Wood, Filbee-Dexter, Minne, Goold, Vergés, Marzinelli, Steinberg and Wernberg. This is an open-access article distributed under the terms of the Creative Commons Attribution License (CC BY). The use, distribution or reproduction in other forums is permitted, provided the original author(s) and the copyright owner(s) are credited and that the original publication in this journal is cited, in accordance with accepted academic practice. No use, distribution or reproduction is permitted which does not comply with these terms.
*Correspondence: Melinda Ann Coleman, TWVsaW5kYS5jb2xlbWFuQGdtYWlsLmNvbQ==; bWVsaW5kYS5jb2xlbWFuQGRwaS5uc3cuZ292LmF1