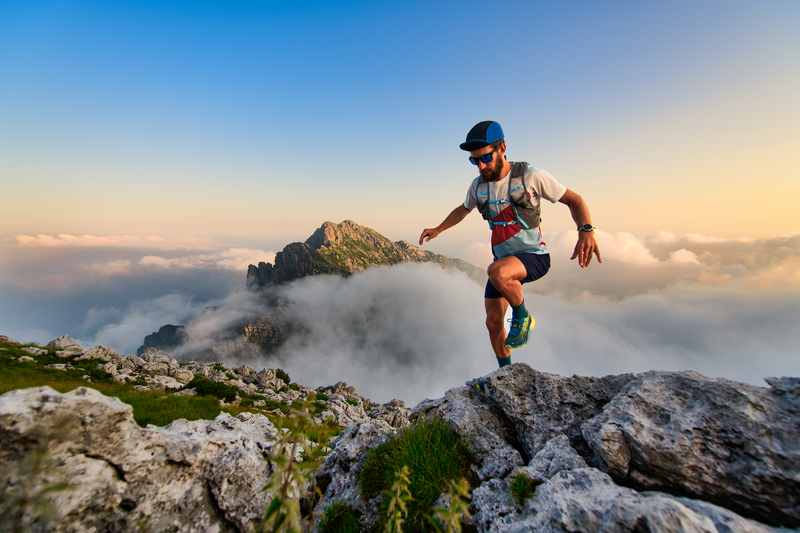
95% of researchers rate our articles as excellent or good
Learn more about the work of our research integrity team to safeguard the quality of each article we publish.
Find out more
BRIEF RESEARCH REPORT article
Front. Mar. Sci. , 24 April 2020
Sec. Global Change and the Future Ocean
Volume 7 - 2020 | https://doi.org/10.3389/fmars.2020.00200
This article is part of the Research Topic Extreme Benthic Communities in the Age of Global Change View all 14 articles
The risk assessment of seafloor massive sulfide (SMS) mining on meiobenthic organisms, specifically on soft-sediment meiofauna, is impeded by a lack of knowledge on the biology and ecology of these communities. In this study, we investigated sediment samples taken in proximity of active vents at Trans-Atlantic Geotraverse (TAG) and Snake Pit, two hydrothermal vent fields of the Mid-Atlantic Ridge, in order to explore metazoan meiofauna, particularly nematode community, and its relation to organic carbon, total nitrogen, total sulfur, and dissolved oxygen. Organic carbon and nitrogen contents were low at both sites. High concentrations of total sulfur and low oxygen penetration were found at Snake Pit compared to TAG. Snake Pit showed approximately four times higher meiofauna and nematode density compared to TAG, as well as a dissimilar nematode community composition. We hypothesize that high sulfur concentrations at Snake Pit may support high microbial growth, which represents one of the main food source for nematodes. Moreover, TAG nematode community mostly consisted of persisters (K-strategists), whereas Snake Pit one was composed by both persisters (Desmoscolecidae family) and colonizers (r-strategists Metalinhomoeus and Halomonhystera), whose presence can be facilitated by the bioturbation effect of polychaetes observed on the sediment surface. Therefore, food availability, geochemical settings, and biotic interactions seem to drive the local meiofauna and nematode community. Our study also draws attention to the opportunity of including meiofauna and specifically nematodes in impact studies conducted in this area in order to assess and monitor the impact of SMS mining.
The demand for mineral raw materials is increasing nowadays as a result of constant population growth, rising living standards, urbanization, technological advance, and, more recently, the transition to a low-carbon economy (Murton et al., 2019). Therefore, alternative sources of minerals are currently being sought, such as deep-sea seafloor massive sulfide (SMS) (Boschen et al., 2013). Seafloor massive sulfide deposits form through hydrothermal vent activity, which generates areas of hard substrate enriched with high content of base metals (zinc, iron, lead, and copper), sulfides, and rare elements, such as gold, silver, cobalt, and platinum (Hoagland et al., 2010). Once the flow of chemically reduced hydrothermal fluid ceases, sulfide deposits are no longer hydrothermally active, thus becoming inactive (exinctSMS or eSMS) (Van Dover, 2019). These deposits are likely accessible to future mining and are far more abundant than active ones, but their localization can be impeded by pelagic sediment, which slowly covers the deposits (Murton et al., 2019). For instance, according to Murton et al. (2019), the Trans-Atlantic Geotraverse (TAG) (Figure 1), which is one of the most studied hydrothermal sites of the Mid-Atlantic Ridge (MAR), is smeared with deposits that extend sub-seafloor and are actually larger than what previous studies estimated. Indeed, whereas Hannington et al. (1998) reported a deposit tonnage of 2.7 Mt, Murton et al. (2019) recently estimated TAG sulfides to be around 26 Mt. Thus, TAG has been defined as potentially more attractive for mining activities than it was believed so far. Moreover, SMS deposits located on a slow-spreading ridge, such as TAG and Snake Pit (Figure 1), are bigger, and their venting activity more stable, compared to fast-spreading ridges. They are thus more suitable sites for mining (Fouquet and Scott, 2009; Boschen et al., 2013).
Figure 1. Map of Trans-Atlantic Geotraverse (TAG) (26°08′ N) and Snake Pit (23°23′ N) vent fields in the Mid-Atlantic Ridge (a); map of Snake Pit sampling site, pink star = dive 1920-14 (b); map of TAG sampling site, pink star = dive 1913-07 (c), red triangle = nearest active mound (b,c); Snake Pit oxygen profile and penetration depth with evidences of bioturbation (d); TAG oxygen profile and penetration depth (e).
Mining activities will directly affect benthic communities of the SMS deposits target areas mainly because of the removal of substrate that harbors the organisms, but also because of sediment turbidity and toxic plumes (Boschen et al., 2013). The risk assessment of eSMS mining is, however, impeded by a lack of knowledge on the biology and ecology of communities associated with eSMS, and particularly so for the soft-sediment communities (Van Dover, 2019). The few studies that sampled hydrothermal sediments or sediments in the vicinity of hydrothermal vents highlighted the patchiness of the distribution, as well as the heterogeneity in the structure and composition of meiofaunal and macrofaunal assemblages (Vanreusel et al., 1997; Levin et al., 2009). In the North Fiji Basin, Vanreusel et al. (1997) reported higher meiofauna densities in active than inactive hydrothermal sediments as well as a lower diversity and different nematode species in hydrothermal compared to background sediments. The hydrothermal sediments, sampled with a TV grab, were hardly quantitative, but it remains, to date, the best comparison of meiofaunal assemblages between hydrothermally active and inactive sediments.
During the BICOSE 2 cruise (Cambon-Bonavita, 2018), patches of sediment were quantitatively sampled at the periphery of TAG and Snake Pit, two hydrothermal vent fields of the MAR, with the aim of describing meiofauna communities and understanding the drivers of variations in community structure and composition in different geochemical contexts. The meiofauna and nematode assemblages were investigated in soft metalliferous sediments in relation with organic carbon (OC), total nitrogen (N), total sulfur (S), and dissolved oxygen (O2).
TAG (26°08′ N) and Snake Pit (23°23′ N) vent sites (Figure 1) are located on two different segments of the slow-spreading (22 mm/year) MAR (Kleinrock and Humphris, 1996) and are separated by a linear distance of about 300 km (Van Dover, 1995).
The TAG area holds several extinct SMS deposit and one high-temperature SMS deposit (i.e., TAG active mound) (Murton et al., 2019). Trans-Atlantic Geotraverse active mound is located at 3,670-m depth and 3 km east of the ridge axis. It consists in a 250-m diameter and 50-m-high deposit topped with black smoker chimneys (350°C) (Rona et al., 1986). Metalliferous red-brown oxide sediments have been observed up to 125 m away from the base of the deposit talus, specifically a 7-cm-thick layer of Fe-rich red-brown mud (20–40% Fe) overlying a 4-cm-thick layer of carbonate ooze (5–70% CaCO3), which also contains up to 32% Fe (German, 1993). Trans-Atlantic Geotraverse active mound formed about 50,000 years ago and still shows hydrothermal activity (Lalou et al., 1990; German, 1993).
The Snake Pit vent field is located at the ridge axis at a water depth between 3,480 and 3,570 m, and it consists of three elongate parallel ridges (Thompson et al., 1988). The flat areas between the ridges are covered by metalliferous sediment composed of dark granular sulfides, mainly pyrrhotite, chalcopyrite, marcasite, and pyrite, and minor sphalerite (Thompson et al., 1988; Sudarikov and Galkin, 1995). Several small SMSs are known in the field, including the Beehive, Nail, Moose, Firtree, and the Cliff deposits. These mounds are 20–60 m in diameter and 20–25 m in height (Fouquet et al., 1993). High-temperature activity and related black smoker chimneys (325°-330°C) is restricted to the Beehive and Cliff hydrothermal sites (Fouquet et al., 1993).
Samples were collected at the MAR during the BICOSE2 cruise (2018) on board the RV Pourquoi Pas?. Soft sediment samples were taken through the human-operated vehicle (HOV) Nautile during the dive 1913-07 for TAG and dive 1920-14 for Snake Pit, from January 27 to March 11, 2018 (Figure 1). These two sampling sites will thereafter be named TAG and Snake Pit for simplification. Trans-Atlantic Geotraverse sampling site was located 90 m to the northwest of the active mound. Snake Pit sampling site was located 70 m to the northeast of the black smoker “Moose.” Four push cores (CT) (5.5-cm diameter, 24-cm2 sampling area) were retrieved per site; three were used for meiofauna, and one was used for O2 profiling and OC, N, and total S contents. Samples for meiofauna were sliced vertically on board according to depth in five layers of 1 cm each (0–1, 1–2, 2–3, 3–4, and 4–5 cm). Each layer was fixed in 4% formalin. The sediment core for geochemistry was first used for O2 profiling and then sliced horizontally every centimeter. The sediment was kept frozen at −20°C until freeze dried, grinded, and analyzed back at the laboratory.
All samples were sieved on 1 mm, 300 μm, and 32 μm in order to separate fauna by body size. Meiofauna was extracted by Ludox centrifugation according to Heip et al. (1985) and then stained with fuchsine and fixed in 4% formalin. All meiobenthic animals retained in both 32- and 300-μm sieves were counted and classified to high taxonomic level under a stereomicroscope Olympus SZX16 (Olympus Corporation, Tokyo, Japan). All nematodes were mounted on permanent slides following the formalin–ethanol–glycerol technique described by Seinhorst (1959) and then identified at genus level with a Leica DM2500 LED microscope (Leica Microsystems GmbH, Wetzlar, Germany) according to Platt and Warwick (1988), WoRMS Editorial Board (2020), Bezerra et al. (2020), Bain et al. (2014).
Oxygen profiles were performed ex situ using Clark-type microelectrodes (Revsbech, 1989). The sediment core was kept at in situ temperature during profiling, and surface water was gently bubbled with an air diffusor to ensure a well-mixed overlying water. Linear calibration was achieved between an air-saturated seawater titrated using the Winkler technique (Grasshoff et al., 1983) and an O2-free water obtained by adding sodium sulfite (Na2SO3). The averaged concentrations were then calculated for each layer in which meiofauna was retrieved (basically every cm down to 5 cm when O2 was detected).
Total S, N, and carbon were determined using a TruMac CNS from LECO (LECO, St. Joseph, MI, USA) (Kowalenko, 2001). Total S and N were analyzed at 1,450°C using COMCAT (LECO, St. Joseph, MI, USA) as a combustion accelerator (Kowalenko, 2001). Organic carbon was measured at 1,350°C after removing carbonates overnight at a temperature of 60°C with 1 M HCl. Samples were then rinsed twice with Milli-Q water and dried before their introduction in the analyzer (Brodie et al., 2011 and references therein).
Richness (number of taxonomic groups), density (number of individuals per 10 cm2), and community composition for metazoan meiofauna were analyzed. For nematodes, richness (number of genera) and density (number of individuals per 10 cm2) were considered. Kruskal–Wallis test was conducted to assess differences in richness and density between sites. Differences in meiofauna and nematode community composition between the two studied sites were tested using Ružička (abundance) matrix through permutational analysis of variance (PERMANOVA). Ružička index was calculated through the function “beta” of the R package vegan v. 2.2-1 (Oksanen, 2015), and PERMANOVA was performed using distance matrices calculated with the function “adonis” included in the R package vegan v. 2.2-1 (Oksanen, 2015). Principal component analysis (PCA) conducted by densities were performed to visualize community composition variations between sites using the Hellinger distance for data transformation, functions “rda” and “decostand” of the R package vegan v. 2.2-1 (Oksanen et al., 2018). Moreover, the Shannon diversity index (H′) and the Pielou's evenness index (J) were calculated for both meiofauna and nematode with the software PRIMER v6 (PRIMER-e, Clarke and Gorley, Auckland, New Zealand) (Clarke and Gorley, 2006).
At Snake Pit, a thin layer of reddish sediments at the top of the core was draping darker brownish sediments. At the sediment surface, numerous hesionid polychaetes were observed crawling among tubes of ampharetid polychaetes.
The upper layer of the sediment core taken at TAG showed a reddish color, reflecting the typical TAG iron oxyhydroxide minerals (German, 1993). No macrofauna was visually observed at the surface of the sediment during the dive.
Oxygen concentration was decreasing with depth at both sites (Table S1, Figure 1). Oxygen penetration depth was 0.7 ± 0.5 cm at Snake Pit, with visual evidences for bioturbation (increases in O2 concentrations at depth due to bioirrigation of burrows by polychaetes; Figure 1, Table S1), whereas O2 concentration was still >100 μM at 7-cm depth at TAG, with no visible signs of bioturbation. The O2 concentrations averaged per sediment layers (from 0 to 5 cm) are displayed in Table S1. Organic carbon contents were <0.5% at both sites, with values slightly decreasing with depth between 0 and 1 cm. Nitrogen contents were very low, ranging from 0.06 to 0.11% at Snake Pit and from 0.03 to 0.1% at TAG. Total S contents were 26 to 31% at Snake Pit and only 0.7 to 1.0% at TAG in the top 5 cm of the core.
Snake Pit has the highest average meiofauna density (138 ± 34 ind/10 cm2) compared to TAG (32 ± 16 ind/10 cm2) (Figure 2), whereas the same taxonomic richness was observed at both sites (a total of 7 taxa per site) (Table 1). The highest meiofauna densities were found in the first 2 cm of sediment at both TAG (95%) and Snake Pit (97%), with a sharp decrease from the first to the second layer (from 90 to 6% at TAG and from 80% to 17% at Snake Pit). According to the Kruskal–Wallis test, the only statistically significant difference between TAG and Snake Pit was found in meiofauna density (p = 0.049). Nematodes account for 70% of the total meiofauna at TAG and for 62% at Snake Pit, thus being the highest density taxon, immediately followed by copepods and nauplii, together accounting for 27% at TAG and 34% at Snake Pit. Gastrotricha, Tantulocarida, Ostracoda, and Halacarida account each for <2%, whereas only at Snake Pit, polychaeta account for 3.4%. Only Nematoda were found in the deeper layers (3–5 cm) of TAG cores, whereas also Copepoda, Nauplii, and Ostracoda were present in those layers in Snake Pit samples (Figure S1). Moreover, Tantulocarida and Halacarida were exclusive taxa from TAG and Snake Pit, respectively (Figure S1). Permutational analysis of variance was conducted, but no statistically significant difference between sites was detected. Similarly, PCA did not identify a discrimination in taxa composition between the two sites (Figure 3). Both Shannon's diversity and Pielou's evenness indices were lower for TAG (H′ = 0.91, J = 0.47) than for Snake Pit (H′ = 1.11, J = 0.57) (Table 1).
Figure 2. Meiofauna density boxplot (A), meiofauna richness boxplot (B), nematode density boxplot (C), nematode richness boxplot (D). The boxplots represent the median value (horizontal thicker line within the box), the distributions of 50% of the data (the box), and the highest and lowest values of the distribution for both Snake Pit (in blue) and TAG (in yellow).
Table 1. The density (ind/10 cm2) per core of each meiofauna taxa and nematode genera is given for TAG (on the left) and for Snake Pit (on the right); total density and total abundance per core are also given, together with an average density, and average abundance per site.
Figure 3. Principal component analysis of meiofauna taxa composition (A) and nematode genera composition (B). Blue dots indicate Snake Pit samples, whereas yellow dots indicate TAG samples. For meiofauna (A), PC1 explains 76% of the variance, whereas PC2 explains 13%. For nematodes (B), only the most representative genera are shown in the plot, and PC1 explains 76% of the variance, whereas PC2 explains 12%.
Nematodes were found mostly in the first 2 cm of sediment for both Snake Pit (97% of the total population) and TAG (97%), with a sharper decrease from the first (94%) to the second (3%) cm at TAG than at Snake Pit (91% in the first cm, 6% in the second). Snake Pit has the highest average nematode density (86 ± 12 ind/10 cm2) compared to TAG (22 ± 7 ind/10 cm2) (Figure 2) and a slightly higher diversity (average of 14 genera, total of 24 genera) compared to TAG (average of 10 genera, total of 19 genera) (Table 1). According to the Kruskal–Wallis test, no statistically significant difference was found in terms of nematode diversity between the two sites, whereas the difference in nematode density is statistically significant (p = 0.049). The most abundant nematode genera at TAG are Desmoscolex (28% of the total density) and Microlaimus (18% of the total density), whereas at Snake Pit Metalinhomoeus accounts for 38% of the total density, and Halomonhystera accounts for 25% (Table 1). Three of the 24 and 19 genera observed at Snake Pit and TAG, respectively, were present at both sites: Cephalochaetosoma, Microlaimus, and Syringolaimus. Trans-Atlantic Geotraverse deeper layers (2–5 cm) host few nematodes: one specimen of Chromadorella, Cobbia, Retrotheristus, Sabatieria, and Syringolaimus (Figure S2). Snake Pit deeper layers (2–5 cm) host several nematode genera: two specimens of Camacolaimus, Metalinhomoeus, Sabatieria, and Syringolaimus; three of Halomonhystera; and only one of Cephalochaetosoma and Desmodora (Figure S2). Permutational analysis of variance analysis found a statistically significant difference in community composition between sites (p = 0.001; F = 2.73), which explains 13% of the variance. Moreover, PCA revealed a strong discrimination in genera composition between the two sites. Indeed, PC1 explains 78% of the variance, with Metalinhomoeus (score = 0.6) and Halomonhystera (score = 0.5) characterizing Snake Pit, whereas Desmoscolex (score = −0.5) typifies TAG (Figure 3). Both diversity and evenness indices were higher for TAG (H′= 2.04; J = 0.68) than for Snake Pit (H′= 1.92; J = 0.59) (Table 1).
The ecology of nematodes living in chemosynthetic environments has been reviewed by Vanreusel et al. (2010), who noted that the community structure was driven by the presence of soft substrate, geochemical settings, and the availability of food, whereas community composition was additionally driven by the spatial continuity of the habitat. In this study, sedimentation of particulate matter around the studied sites was mainly due to the erosion of ancient sulfides mounds and the settling of hydrothermal particles that precipitated within the plume. The solid phase composition shows a significant difference in total S, with a stronger influence of metalliferous sediment at Snake Pit (Table S1). Still, both sites exhibit similar and relatively low organic and N contents. Because O2 is generally correlated to OC, with an increasing penetration depth when OC decreases (Wenzhöfer and Glud, 2002), one could have expected a similar O2 penetration depth (OPD) between the sites. Nevertheless, while the OPD at TAG is what we expect from abyssal areas (i.e., several centimeters; Glud, 2008), the OPD at Snake Pit is much shallower, with evidences of bioturbation (Figure 1). This may have contributed to the observed differences in our nematodes community composition. While TAG nematode community is mostly composed by persisters with a K-type life strategy (low reproductive rates, low colonizing capability, and low levels of tolerance to disturbance) and no clear dominance pattern, Snake Pit community consists of both colonizers and persisters genera with a clear dominance of two taxa, Metalinhomoeus and Halomonhystera, representing together 63% of the total community. The dominance of these two tolerant genera and the presence of Sabatieria are consistent with the hypoxia of Snake Pit sediments. The two nematode genera Metalinhomoeus and Sabatieria are able to reach the deeper anoxic layers of Snake Pit sediments (5-cm depth) (Figure S2). Metalinhomoeus is one of the biggest and longest nematodes of our samples, a peculiar body shape of thiobiotic nematodes (deeper-living in the sediment) (Jensen, 1987). The pronounced body elongation and the increase in surface–volume ratio in thiobiotic species are an adaptive character related to low O2 partial pressure (Vanreusel et al., 2010). The increased body length in suboxic or anoxic conditions reflects an increased mobility, which allows nematodes to move easily among sediment layers, passing quickly from depleted to well-oxygenated zones (Jensen, 1986, 1987). Sabatieria has already been described as a resistant genus to anoxic conditions, able to survive periods of anoxia of up to 7 weeks, and it is often the only remaining species in strongly anoxic conditions (Jensen, 1984; Modig and Ólafsson, 1998; Boyd et al., 2000; Wetzel et al., 2002; Steyaert et al., 2005, 2007). The ovoviviparous strategy observed in Halomonhystera as a response to stressful or toxic conditions (Walker and Tsui, 1968; Luc et al., 1979; Chen and Caswell-Chen, 2003) may also enhance their probability to survive and make them more tolerant than other meiofauna taxa (Polz et al., 1992; Thiermann et al., 2000; Bellec et al., 2018). In contrast to what Vanreusel et al. (1997) found at the Fiji basin, specialized nematode genera seem to have been able to invade the Snake Pit vent fields surrounding sediments, where they coexist with genera less tolerant to harsh conditions. The presence of the latter may be facilitated by the activity of polychaetes that were observed on the sediment surface, because polychaetes act as biodiffusors and bioturbators, mixing particles and pore water through the sediment layers (Reible et al., 1996).
The presence of numerous polychaetes and the higher density of meiofauna, together with the low OPD, would suggest an extra input of food sources at Snake Pit, despite the similar OC and N contents at both sites. Snake Pit sediments are characterized by sulfide-rich minerals, and Kato et al. (2018) suggested that chemolithoautotrophic bacteria may play a key role as primary producers in the sulfide deposits below the seafloor. This chemosynthetic system can be fueled by oxidized pyrite or pyrrhotite, which release Fe(II) and sulfide in oxygenated seawater (Schippers and Jorgensen, 2002). In these reactions, intermediate S species (ISS), such as elemental S, sulfite, and thiosulfate, are produced during electron transfer from sulfide to sulfate (Moses et al., 1987). Through the analysis of metagenome-assembled genomes from massive sulfide deposits, Kato et al. (2018) found that some yet-uncultivated bacteria are able to use sulfate or ISS as energy sources. Therefore, we hypothesize that high sedimentary S concentrations may result in an increase of bacterial density and, subsequently, in a raise of food inputs for the dominant meiofauna taxa, resulting in their flourishing (Van Gaever et al., 2009). Indeed, the most abundant nematode genera at Snake Pit (Metalinhomoeus and Halomonhystera) are nonselective deposit feeders (group 1B, Wieser, 1953), which can feed on bacteria as well (Moens and Vincx, 1997).
The observed differences in nematode community composition between TAG and Snake Pit could also be attributed to the distance between the sites (300 km) and the further separation due to the Kane Fracture Zone. As already suggested by Vanreusel et al. (1997), the dispersal ability of free-living nematodes is limited; thus, colonization of patchily distributed, and ephemeral hydrothermal vents comes from adjacent sediments rather than the long-range dispersal of a specialized fauna. In the Fiji basin indeed, the nematode genera composition was similar between vents and control sites with few dominating genera, of which Monhystera prevailed in the majority of samples (Vanreusel et al., 1997). However, this hypothesis will be further tested by a comparison with control sites in a future study.
At our sites, as the meiofauna community composition reflects the pattern reported in the literature for the deep sea, that is, nematodes as approximately 90% of the total abundance of meiofauna, it does not allow to determine a discrimination between TAG and Snake Pit (Heip et al., 1985; Vanreusel et al., 2010; Zeppilli et al., 2014). Instead, nematodes show a genus-specific response to variable geochemical characteristics of metalliferous sediments. Thus, although meiofauna may be a powerful bioindicator of anthropogenic impacts (Zeppilli et al., 2015), a higher taxonomic resolution is needed to reveal the response of meiobenthic communities to the heterogeneity of their environments (Zekely et al., 2006; Gollner et al., 2010; Degen et al., 2012; Sarrazin et al., 2015). This heterogeneity should be taken into account when predicting the effect of a mining event on the structure of meiobenthic community, knowing that the reduction in habitat heterogeneity may permanently alter the structure of benthic communities (Zeppilli et al., 2015) and recolonization relies mainly on near vent sites (Vanreusel et al., 1997; Zekely et al., 2006).
The soft-sediment meiofauna and nematode communities showed four times higher densities at the Snake Pit than the TAG vent fields. The composition of the meiofauna at a low taxonomic resolution reflects the pattern reported in the literature for the deep sea (i.e., nematodes contributing for 90% of the total abundance of meiofauna) (Vanreusel et al., 2010 and references therein; Zeppilli et al., 2014), but differences in the Nematoda community composition at genus level revealed the influence of the surrounding environment. The geochemical settings (mostly O2 and total S) and food availability are hypothesized to be the main drivers for the observed differences between the two study sites. Thus, our findings highlight the heterogeneity of hydrothermal sediments and the necessity of a high taxonomic resolution to reveal the response of meiofauna community to variations in biotic and abiotic factors and eventually mining impacts.
The datasets generated for this study are available on request to the corresponding author.
AS sorted meiofauna samples, identified the nematodes, and wrote the main body of the paper. NS designed the article, sorted meiofauna, ran the statistical analysis, and helped in nematodes identification. LP analyzed the cores for the geochemistry and contributed in defining the geochemical setting of the paper. LM designed the objectives of the study and the sampling strategy, supervised sampling on board during BICOSE2, and highly contributed to the statistical analysis. DZ was the chief of the project and contributed in the nematodes identification. Everybody contributed equally to the writing of the paper.
This work was supported by EQUINOR and IFREMER, which funded the postdoctoral fellowship of NS as part of the eCOREF project (Ecological connectivity and functional links between hydrothermal active and inactive sites in view of potential SMS mining in the deep-sea). We also thank Università Politecnica delle Marche and the Erasmus Traineeship Program for its contribution to AS first stay at Ifremer.
The authors declare that the research was conducted in the absence of any commercial or financial relationships that could be construed as a potential conflict of interest.
We wish to thank all participants and the staff of the RV Pourquoi Pas?, the HOV Nautile crew and all scientists and students who participated in the BICOSE2 cruise (doi: 10.17600/18000004). We specially want to thank Dr. Marie-Anne Cambon-Bonavita, chief scientist of the cruise and Ewan Pelleter for his advice.
The Supplementary Material for this article can be found online at: https://www.frontiersin.org/articles/10.3389/fmars.2020.00200/full#supplementary-material
Bain, O., Baldwin, J. G., Beveridge, I., Campinas Bezerra, T., Braeckman, U., Coomans, A., et al. (2014). Nematoda. Berlin; Boston, MA: De Gruyter. doi: 10.1515/9783110274257
Bellec, L., Cambon-Bonavita, M. A., Cueff-Gauchard, V., Durand, L., Gayet, N., and Zeppilli, D. (2018). A nematode of the mid-atlantic ridge hydrothermal vents harbors a possible symbiotic relationship. Front Microbiol. 9:2246. doi: 10.3389/fmicb.2018.02246
Bezerra, T. N., Decraemer, W., Eisendle-Flöckner, U., Hodda, M., Holovachov, O., Leduc, D., et al. (2020). Nemys: World Database of Nematodes. doi: 10.14284/366
Boschen, R. E., Rowden, A. A., Clark, M. R., and Gardner, J. P. A. (2013). Mining of deep-sea seafloor massive sulfides: a review of the deposits, their benthic communities, impacts from mining, regulatory frameworks and management strategies. Ocean Coast. Manag. 84, 54–67. doi: 10.1016/j.ocecoaman.2013.07.005
Boyd, S. E., Rees, H. L., and Richardson, C. A. (2000). Nematodes as sensitive indicators of change at dredged material disposal sites. Estuar. Coast. Shelf Sci. 51, 805–819. doi: 10.1006/ecss.2000.0722
Brodie, C. R., Leng, M. J., Casford, J. S. L., Kendrick, C. P., Lloyd, J. M., Yongqiang, Z., et al. (2011). Evidence for bias in C and N concentrations and δ13C composition of terrestrial and aquatic organic materials due to pre-analysis acid preparation methods. Chem. Geol. 282, 67–83. doi: 10.1016/j.chemgeo.2011.01.007
Chen, J., and Caswell-Chen, E. P. (2003). Why Caenorhabditis elegans adults sacrifice their bodies to progeny. Nematology 5, 641–645. doi: 10.1163/156854103322683355
Degen, R., Riavitz, L., Gollner, S., Vanreusel, A., Plum, C., and Bright, M. (2012). Community study of tubeworm-associated epizooic meiobenthos from deep-sea cold seeps and hot vents. Mar. Ecol. Prog. Ser. 468, 135–148. doi: 10.3354/meps09889
Fouquet, Y., and Scott, S. D. (2009). “SS: ocean mining: the science of seafloor massive sulfides (SMS) in the modern ocean - a new global resource for base and precious metals,” in Offshore Technology Conference. doi: 10.4043/19849-MS
Fouquet, Y., Wafik, A., Cambon, P., Mevel, C., Meyer, G., and Gente, P. (1993). Tectonic setting and mineralogical and geochemical zonation in the snake pit sulfide deposit (mid-Atlantic ridge at 23°N). Econ. Geol. 88, 2018–2036. doi: 10.2113/gsecongeo.88.8.2018
German, C. R. (1993). A geochemical study of metalliferous sediment from the TAG hydrothermal mound, 26°08′N, Mid-Atlantic Ridge. J. Geophys. Res. 98, 9683–9692. doi: 10.1029/92JB01705
Glud, R. N. (2008). Oxygen dynamics of marine sediments. Mar. Biol. Res. 4, 243–289. doi: 10.1080/17451000801888726
Gollner, S., Riemer, B., Arbizu, P. M., le Bris, N., and Bright, M. (2010). Diversity of meiofauna from the 9°50′N east pacific rise across a gradient of hydrothermal fluid emissions. PLoS ONE 5:e12321. doi: 10.1371/journal.pone.0012321
Grasshoff, K., Ehrhardt, M., and Kremling, K. (1983). Methods of Seawater Analysis, 2nd edn. Weinheim: Verlag Chemie GmbH.
Hannington, M. D., Galley, A. G., Gerzig, P. M., and Petersen, S. (1998). Comparison of the TAG mound and stockwork complex with Cyprus-type massive sulfide deposits. Proc. Ocean Drill. Progr. Sci. Results 158, 389–415. doi: 10.2973/odp.proc.sr.158.217.1998
Heip, C., Vincx, M., and Vranken, G. (1985). The ecology of marine nematodes. Oceanogr. Mar. Biol. 23, 399–489.
Hoagland, P., Beaulieu, S., Tivey, M. A., Eggert, R. G., German, C., Glowka, L., et al. (2010). Deep-sea mining of seafloor massive sulfides. Mar. Policy 34, 728–732. doi: 10.1016/j.marpol.2009.12.001
Jensen, P. (1984). Ecology of benthic and epiphytic nematodes in brackish waters. Hydrobiologia 108, 201–217. doi: 10.1007/BF00006329
Jensen, P. (1986). Nematode fauna in the sulfide-rich brine seep and adjacent bottoms of the east flower garden northwestern gulf of mexico iv. Ecol Aspects. Mar. Biol. 92, 489–503. doi: 10.1007/BF00392509
Jensen, P. (1987). Differences in microhabitat, abundance, biomass and body size between oxybiotic and thiobiotic free-living marine nematodes. Oecologia 71, 564–567. doi: 10.1007/B.F.00379298
Kato, S., Shibuya, T., Takaki, Y., Hirai, M., Nunoura, T., and Suzuki, K. (2018). Genome-enabled metabolic reconstruction of dominant chemosynthetic colonizers in deep-sea massive sulfide deposits. Environ. Microbiol. 20, 862–877. doi: 10.1111/1462-2920.14032
Kleinrock, M. C., and Humphris, S. E. (1996). Structural asymmetry of the TAG rift valley: evidence from a near-bottom survey for episodic spreading. Geophys. Res. Lett. 23, 3439–3442. doi: 10.1029/96GL03073
Kowalenko, C. G. (2001). Assessment of Leco CNS-2000 analyzer for simultaneously measuring total carbon, nitrogen, and sulphur in soil. Commun. Soil Sci. Plant Anal. 32, 2065–2078. doi: 10.1081/CSS-120000269
Lalou, C., Thompson, G., Arnold, M., Brichet, E., Druffel, E., and Rona, P. A. (1990). Geochronology of TAG and Snake Pit hydrothermal fields; MAR - Witness to a long ans complex hydrothermal history. Earth Planet. Sci. Lett. 97, 113–128. doi: 10.1016/0012-821X(90)90103-5
Levin, L. A., Mendoza, G. F., Konotchick, T., and Lee, R. (2009). Macrobenthos community structure and trophic relationships within active and inactive Pacific hydrothermal sediments. Deep. Res. Part II Top. Stud. Oceanogr. 56, 1632–1648. doi: 10.1016/j.dsr2.2009.05.010
Luc, M., Taylor, D. P., and Netscher, C. (1979). On endotokia matricida and intra-uterine development and hatching in nematodes. Nematologica 25, 268–274. doi: 10.1163/187529279X00299
Modig, H., and Ólafsson, E. (1998). Responses of Baltic benthic invertebrates to hypoxic events. J. Exp. Mar. Bio. Ecol. 229, 133–148. doi: 10.1016/S0022-0981(98)00043-4
Moens, T., and Vincx, M. (1997). Observations on the feeding ecology of estuarine nematodes. J. Mar. Biol. Ass. U.K. 77, 211–227. doi: 10.1017/S0025315400033889
Moses, C. O., Kirk Nordstrom, D., Herman, J. S., and Mills, A. L. (1987). Aqueous pyrite oxidation by dissolved oxygen and by ferric iron. Geochim. Cosmochim. Acta 51, 1561–1571. doi: 10.1016/0016-7037(87)90337-1
Murton, B. J., Lehrmann, B., Dutrieux, A. M., Martins, S., de la Iglesia, A. G., Stobbs, I. J., et al. (2019). Geological fate of seafloor massive sulphides at the TAG hydrothermal field (Mid-Atlantic Ridge). Ore Geol. Rev. 107, 903–925. doi: 10.1016/j.oregeorev.2019.03.005
Oksanen, J. (2015). Vegan: Community Ecology Package. R package version 2.4-3. Available online at: https://CRAN.R-project.org/package=vegan. (accessed February 28, 2020).
Oksanen, J., Blanchet, F. G., Friendly, M., Kindt, R., Legendre, P., McGlinn, D., et al. (2018). vegan: Community Ecology Package. R package version 2.5-2. CRAN R.
Platt, H. M., and Warwick, R. M. (1988). Free-Living Marine Nematodes, Part II British Chromadorids. Netherlands: Brill, Leiden.
Polz, M., Felbeck, H., Novak, R., Nebelsick, M., and Ott, J. A. (1992). Chemoautotrophic, sulfur-oxidizing symbiotic bacteria on marine nematodes: morphological and biochemical characterization. Microb. Ecol. 24, 313–329. doi: 10.1007/BF00167789
Reible, D. D., Popov, V., Valsaraj, K. T., Thibodeaux, L. J., Lin, F., Dikshit, M., et al. (1996). Contaminant fluxes from sediment due to Tubificid oligochaete bioturbation. Water Res. 30, 704–714. doi: 10.1016/0043-1354(95)00187-5
Revsbech, N. P. (1989). An oxygen microsensor with a guard cathode. Limnol. Oceanogr. 34, 474–478. doi: 10.4319/lo.1989.34.2.0474
Rona, P. A., Klinkhammer, G., Nelsen, T. A., Trefry, J. H., and Elderfield, H. (1986). Black smokers, massive sulphides and vent biota at the Mid-Atlantic ridge. Nature 321, 33–37. doi: 10.1038/321033a0
Sarrazin, J., Legendre, P., de Busserolles, F., Fabri, M. C., Guilini, K., Ivanenko, V. N., et al. (2015). Biodiversity patterns, environmental drivers and indicator species on a high-temperature hydrothermal edifice, Mid-Atlantic Ridge. Deep. Res. Part II Top. Stud. Oceanogr. 121, 177–191. doi: 10.1016/j.dsr2.2015.04.013
Schippers, A., and Jorgensen, B. B. (2002). Biogeochemistry of pyrite and iron sulfide oxidation in marine sediments. Geochim. Cosmochim. Acta 66, 85–92. doi: 10.1016/S0016-7037(01)00745-1
Seinhorst, J. W. (1959). A rapid method for the transfer of nematodes from fixative to anhydrous glycerin. Nematologica 4, 67–69. doi: 10.1163/187529259X00381
Steyaert, M., Moodley, L., Nadong, T., Moens, T., Soetaert, K., and Vincx, M. (2007). Responses of intertidal nematodes to short-term anoxic events. J. Exp. Mar. Bio. Ecol. 345, 175–184. doi: 10.1016/j.jembe.2007.03.001
Steyaert, M., Moodley, L., Vanaverbeke, J., Vandewiele, S., and Vincx, M. (2005). Laboratory experiments on the infaunal activity of intertidal nematodes. Hydrobiologia 140, 217–223. doi: 10.1007/s10750-004-7145-4
Sudarikov, S. M., and Galkin, S. V. (1995). Geochemistry of the Snake Pit vent field and its implications for vent and non-vent fauna. Geol. Soc. Spec. Publ. 87, 319–327. doi: 10.1144/GSL.SP.1995.087.01.24
Thiermann, F., Vismann, B., and Giere, O. (2000). Sulphide tolerance of the marine nematode Oncholaimus campylocercoides - A result of internal sulphur formation? Mar. Ecol. Prog. Ser. 193, 251–259. doi: 10.3354/meps193251
Thompson, G., Humphris, S. E., Schroeder, B., Sulanowska, M., and Rona, P. A. (1988). Active vents and massive sulfides at 26°N (TAG) and 23°N (Snake Pit) on the Mid-Atlantic Ridge. Can. Mineral. 26, 697–711.
Van Dover, C. L. (1995). Ecology of Mid-Atlantic Ridge hydrothermal vents. Geol. Soc. London Spec. Publ. 87, 257–294. doi: 10.1144/GSL.SP.1995.087.01.21
Van Dover, C. L. (2019). Inactive sulfide ecosystems in the deep sea: a review. Front. Mar. Sci. 6:461. doi: 10.3389/fmars.2019.00461
Van Gaever, S., Olu, K., Derycke, S., and Vanreusel, A. (2009). Metazoan meiofaunal communities at cold seeps along the Norwegian margin: Influence of habitat heterogeneity and evidence for connection with shallow-water habitats. Deep. Res. Part I Oceanogr. Res. Pap. 56, 772–785. doi: 10.1016/j.dsr.2008.12.015
Vanreusel, A., de Groote, A., Gollner, S., and Bright, M. (2010). Ecology and biogeography of free-living nematodes associated with chemosynthetic environments in the deep sea: a review. PLoS ONE 5:e12449. doi: 10.1371/journal.pone.0012449
Vanreusel, A., Van Den Bossche, I., and Thiermann, F. (1997). Free-living marine nematodes from hydrothermal sediments: Similarities with communities from diverse reduced habitats. Mar. Ecol. Prog. Ser. 157, 207–219. doi: 10.3354/meps157207
Walker, J. T., and Tsui, R. K. (1968). Induction of ovoviviparity in rhabditis by sulfur dioxide. Nematologica 14, 148–149. doi: 10.1163/187529268X00769
Wenzhöfer, F., and Glud, R. N. (2002). Benthic carbon mineralization in the Atlantic: a synthesis based on in situ data from the last decade. Deep-Sea Res. I 49, 1255–1279. doi: 10.1016/S0967-0637(02)00025-0
Wetzel, M. A., Weber, A., and Giere, O. (2002). Re-colonization of anoxic/sulfidic sediments by marine nematodes after experimental removal of macroalgal cover. Mar. Biol. 141, 679–689. doi: 10.1007/s00227-002-0863-0
Wieser, W. (1953). Die beziehung zwischen mundhohlengestalt, ernahrungsweise und vorkommen be1 fre lebenden marmen nernatoden. Ark. Zool. 4, 439–484.
Zekely, J., Van Dover, C. L., Nemeschkal, H. L., and Bright, M. (2006). Hydrothermal vent meiobenthos associated with mytilid mussel aggregations from the Mid-Atlantic Ridge and the East Pacific Rise. Deep. Res. Part I Oceanogr. Res. Pap. 53, 1363–1378. doi: 10.1016/j.dsr.2006.05.010
Zeppilli, D., Bongiorni, L., Santos, R. S., and Vanreusel, A. (2014). Changes in nematode communities in different physiographic sites of the Condor Seamount (North-East Atlantic Ocean) and adjacent sediments. PLoS ONE 9:115601. doi: 10.1371/journal.pone.0115601
Keywords: meiobenthos, nematoda, Mid-Atlantic Ridge, seafloor massive sulfides, biodiversity
Citation: Spedicato A, Sánchez N, Pastor L, Menot L and Zeppilli D (2020) Meiofauna Community in Soft Sediments at TAG and Snake Pit Hydrothermal Vent Fields. Front. Mar. Sci. 7:200. doi: 10.3389/fmars.2020.00200
Received: 29 November 2019; Accepted: 13 March 2020;
Published: 24 April 2020.
Edited by:
Paulina Martinetto, Institute of Marine and Coastal Research (IIMyC), ArgentinaReviewed by:
Sabine Gollner, Royal Netherlands Institute for Sea Research (NIOZ), NetherlandsCopyright © 2020 Spedicato, Sánchez, Pastor, Menot and Zeppilli. This is an open-access article distributed under the terms of the Creative Commons Attribution License (CC BY). The use, distribution or reproduction in other forums is permitted, provided the original author(s) and the copyright owner(s) are credited and that the original publication in this journal is cited, in accordance with accepted academic practice. No use, distribution or reproduction is permitted which does not comply with these terms.
*Correspondence: Adriana Spedicato, YXNwZWRpY2FAaWZyZW1lci5mcg==
Disclaimer: All claims expressed in this article are solely those of the authors and do not necessarily represent those of their affiliated organizations, or those of the publisher, the editors and the reviewers. Any product that may be evaluated in this article or claim that may be made by its manufacturer is not guaranteed or endorsed by the publisher.
Research integrity at Frontiers
Learn more about the work of our research integrity team to safeguard the quality of each article we publish.