- 1Department of Environmental Biology and Fisheries Science, National Taiwan Ocean University, Keelung, Taiwan
- 2Institute of Oceanography, National Taiwan University, Taipei, Taiwan
- 3Institute of Earth Sciences, Academia Sinica, Taipei, Taiwan
- 4Department of Earth Sciences, National Cheng Kung University, Tainan, Taiwan
- 5Earth Dynamic System Research Center, National Cheng Kung University, Tainan, Taiwan
- 6Department of Life Science, National Tsing Hua University, Hsinchu, Taiwan
- 7Institute of Systems Neuroscience, National Tsing Hua University, Hsinchu, Taiwan
- 8Center of Excellence for the Oceans, National Taiwan Ocean University, Keelung, Taiwan
Cuttlebone is a hard calcified structure that supports the cuttlefish body and aids in the regulation of buoyancy. The calcification rate of cuttlebone is high and is close to the growth rate of the cuttlefish mantle. The growth rate of the body mantle may strongly influence the incorporation of elements into the cuttlebone; however, the process has not been well studied. This is the first study to examine trace element incorporation into cuttlebone in detail. Controlled laboratory experiments and analyses of wild-caught cuttlefish revealed that both temperature and growth rate influence the elemental ratios of Li/Ca and Sr/Ca in cuttlebone. The variation in the elemental ratio was influenced the most by growth rates. After controlling for the minor influence of temperature, cuttlebone Li/Ca ratios appeared to be a potential proxy of cuttlefish growth. The relationship between growth rate and temperature-corrected Li/Ca ratios is positive and linear, and the trend does not vary between the sexes or across most of the life stages. In addition, the Li/Ca ratio is a promising growth rate proxy for evaluating differences among wild populations of cuttlefish, and the proxy could facilitate studies on cuttlefish biology and cuttlefish fisheries management.
Introduction
Elemental compositions of biogenic carbonate have been used extensively as environmental proxies for various factors, such as temperature, salinity, and water mass, and are usually applied in studies on foraminifera (Lea, 2003), coral (Lea, 2003; Corrège, 2006), fish otoliths (Bath et al., 2000), and mollusk shells (Schöne et al., 2011). It is now apparent that an vital effect is one of controlling factors of Me/Ca (Minor and trace metal element/Calcium) ratio, biasing Me/Ca ratio as an environmental proxy (Gillikin et al., 2005; Schöne et al., 2011; Sturrock et al., 2012; Chang and Geffen, 2013; Grammer et al., 2017; D’Olivo et al., 2018). Consequently, controlling for the biological factor would enable the calibration of carbonate Me/Ca ratios and facilitate the accurate determination of environmental conditions, particularly temperature (Zhao et al., 2017). Conversely, carbonate Me/Ca ratios can also be a source of information on physiological conditions by acting as a proxy for biological characteristics, leading to an understanding of the influence of physiological factors on biokinetics (Sturrock et al., 2015).
Me/Ca ratios of biogenic carbonate often reflect salinity, environmental temperature, and water chemistry conditions (Bath et al., 2000; Lea, 2003; Corrège, 2006; Schöne et al., 2011) and have been reported to be highly related to carbonate growth rate. For example, growth rates of biogenic carbonates showed a positive relationship with Li/Ca ratio in mollusk shells (Thébault et al., 2009), Mg/Ca ratio in otoliths (Sturrock et al., 2015), and Sr/Ca ratio in mollusk shells (Gillikin et al., 2005), but a negative trend in the Sr/Ca ratio of otoliths (Sturrock et al., 2015). However, the trace element chemistry of cuttlebone carbonate, the inner shell of cuttlefish, has been relatively understudied, although a few research have reported population or geographic differences in cuttlebone trace element chemistry (Ikeda et al., 1999; Turan and Yaglioglu, 2010).
Cuttlefish possess an internal shell, the cuttlebone, which is embedded in a cuttlebone sac. The size of the cuttlebone corresponds to mantle length of cuttlefish for supporting the body structure and controlling their buoyancy (Birchall and Thomas, 1983; Sherrard, 2000). Structurally, the cuttlebone comprises inner and outer cones. The outer cone, or dorsal shield, is thick, non-porous, and calcified and acts as a cover to seal off the lamellae. Conversely, the inner cone consists of a number of lamellae (Figure 1), and each layer is comprised of a single septum with a number of vertical pillars. The components of lamellae are based on an organic framework filled with calcium carbonate in aragonite form (Florek et al., 2009). Previous studies on cuttlebone structure have focused on the number of lamellae, each of which is formed over approximately 1.75 days; thus, they offer a mechanism for estimating the age of cuttlefish (Bettencourt and Guerra, 2001; Chung and Wang, 2013). Cuttlebone growth during ontogenetic development is through continuous lamellar formation. Although one layer does not follow the 1-day rule (Bettencourt and Guerra, 2001; Chung and Wang, 2013), the cuttlebone still records environmental and internal physiological signals over its lifetime.
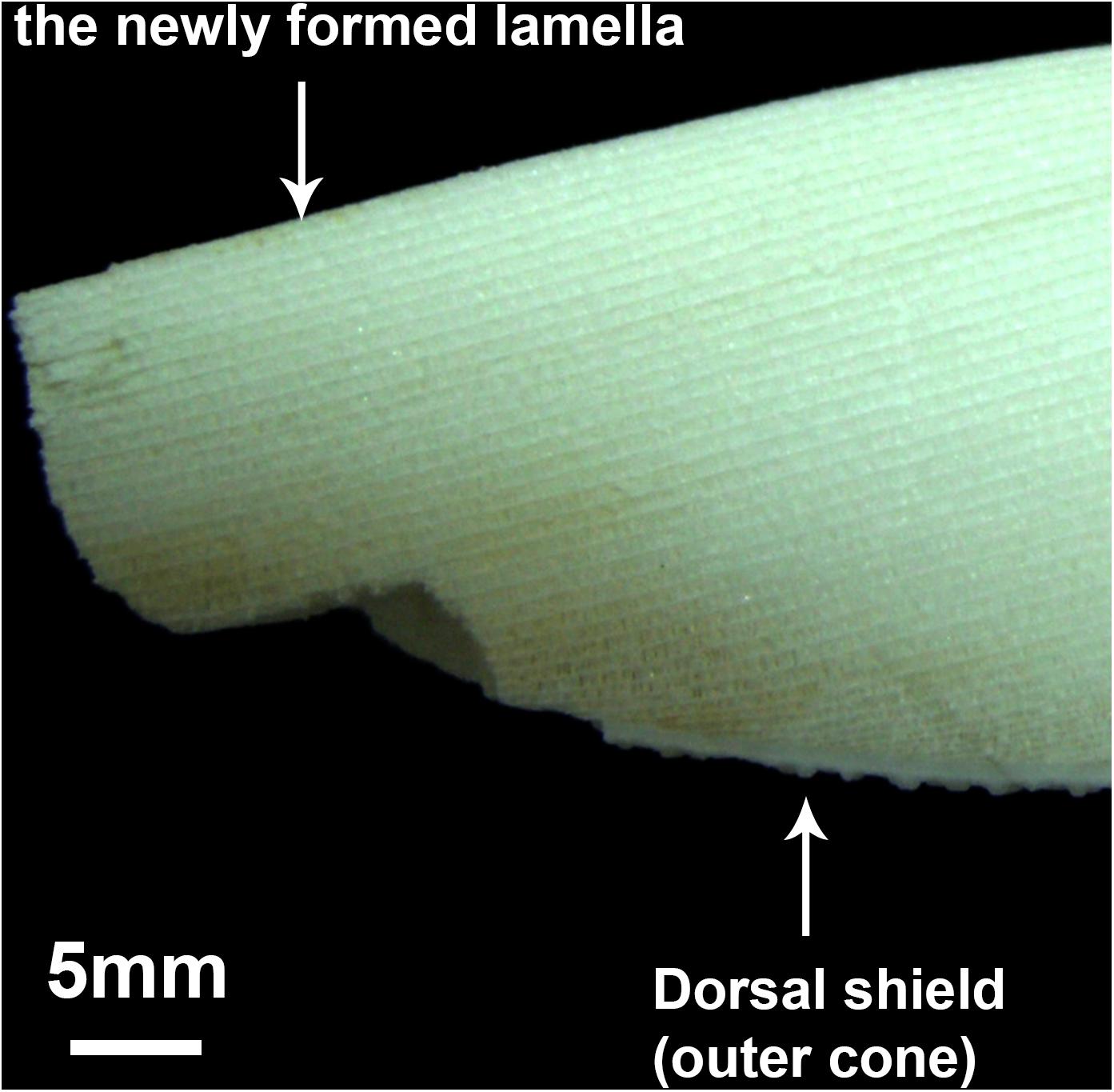
Figure 1. Longitudinal transection of a cuttlebone. The layers represent the structural units, lamellae, of the inner cone of a cuttlebone.
The similar lengths of the cuttlebone and mantle imply a coupling of the calcification rate of the cuttlebone to the growth rate of the cuttlefish. This means that biological factors may influence the incorporation of elements into the cuttlebone. In addition, the rate of lamellar formation can be as high as 1.5 mm every 1.75 days in Sepia pharaonis (Chung and Wang, 2013), which is much more rapid in comparison with other biogenic aragonite carbonate observed for example, in statoliths, otoliths, corals, and bivalve shells. Therefore, further studies on Me/Ca ratios are required, particularly Li/Ca, Mg/Ca, and Sr/Ca ratios, with regard to the effects of growth rate on elemental incorporation in cuttlebone.
The pharaoh cuttlefish, S. pharaonis, is the species of interest in the present study. The species is widely distributed in the Indo-Pacific Ocean and harvested heavily by coastal fisheries. Overfishing in the region highlights the urgent need for the protection of their juveniles (Mehanna et al., 2014). In the coastal waters around Taiwan, the species is relatively larger than other cuttlefish species, implying that they have a more rapid growth rate (Lu and Chung, 2017). Although the species has a short lifespan and generally exhibits rapid growth, few studies have explored its growth rate, growth phases, and growth trends across life stages and among different populations (Minton, 2004), which potentially hampers fishery resource management efforts. If the elemental ratios observed in cuttlebone could be validated as a useful proxy, it could be a powerful tool for monitoring cuttlefish growth in the field and improve upon the traditional approach, lamellar measurement. Because lamellar formation rate is inconsistent under different environments, across different cuttlefish life stages, and between cuttlefish species (Chung and Wang, 2013), its use for measurement may bias the determination of growth rate in wild cuttlefish.
To evaluate elemental incorporation in S. pharaonis cuttlebone, we applied three strategies to investigate the relationship between cuttlebone Me/Ca ratios and growth rate and temperature. The first method was a controlled laboratory experiment in which cuttlefish hatchlings were reared for 1 month at three different temperatures (20, 25, and 30°C) to evaluate the relationships between cuttlebone elemental incorporation and temperature and cuttlebone growth rate. The second method involved evaluating the effect of growth rate and temperature on Me/Ca ratios in wild cuttlefish that were captured monthly in the course of a year. Then, maturity and sex were assessed as potential physiological factors influencing the results. The third method involved using relative growth condition factor (Kn) values, which were compared with the Me/Ca ratio to evaluate whether there is a relationship between cuttlefish size and cuttlebone chemistry in wild populations. The Kn value indicates the individual body mass above (Kn > 1) or below (Kn < 1) the population average at a given mantle length (Bello, 2006). The results of these three tests provided information on cuttlebone chemistry and demonstrated potential for the application of Me/Ca ratios as a geochemical proxy.
Materials and Methods
Laboratory-Controlled Experiment
The rearing experimental design is described in detail in Chung and Wang (2013), and cuttlebones used in the present study were from the previous study. In short, egg capsules and hatchlings of cuttlefish, S. pharaonis, were reared in a temperature-controlled environment at 20, 25, and 30°C. After hatching, the cuttlefish were immersed immediately in seawater containing alizarin complexone (25 ppm) for 3 h before being reared in controlled environments for 1 month. At 1-month, 10–11 individuals were selected at random from each of the three temperature treatments. Mantle length and body weight were recorded for each hatchling. The cuttlebone was dissected from each capsule, ultrasonically cleaned with 10% H2O2 to remove adherent tissues, and washed three times with MilliQ water. The samples were dried overnight in the oven at 40°C before being stored in acid-cleaned vials. Carbonate growth during the rearing period was estimated by measuring the alizarin complexone staining band to the end of the last septum, after which the growth rate was obtained by dividing the increase in length by 30 (the number of rearing days).
Rearing seawater was prepared by using a commercial product of sea salt. The rearing seawater was collected directly from the rearing tank with acid-washed syringes and immediately filtered using 0.22-μm filters, acidified through the addition of ultrapure concentrated HNO3 (J. T. Baker) to maintain pH at 1, and then stored in a freezer. Rearing seawater was collected at least once a week during the rearing period. Samples were diluted in a series of concentrations up to 500-fold and analyzed using inductively coupled plasma optical emission spectrometry (ICP-OES, Thermo Fisher Scientific) at the Isotope Geochemistry Lab, Department of Earth Sciences, National Cheng Kung University, Taiwan. An in-house standard with multiple elements (Li, Mg, Ca, Sr) was prepared by using single element standards (HPS High-Purity Standards, United States) and measured in comparison with a reference material, NIST SRM 1640 (Trace Elements in Natural Water, National Institute of Standards and Technology, United States). The validation of in-house standard was through repeating measurements over 6 months, and the measurements for all elements showed a relative standard deviation lower than 3%. In addition, a percentage error for all element was lower than 3% with respect to NIST SRM 1640 (the difference between a measured and certified value, divided by the certified value and then multiplied by 100%). Then, the in-house standard was used to generate calibration curves for four selected elements. NIST SRM 1643e (Trace Elements in Water, National Institute of Standards and Technology, United States) was used in the samples analysis procedure to determine accuracy of analysis, which was observed to be lower than 2% of percentage error for all elements (Li: 1.9%; Mg: 0.8%; Ca: 0.5%; Sr: 1.2%). In addition, the matrix effect on elemental concentrations was monitored and calibrated by an in-house seawater standard. The limit of detection (LOD) and limit of quantification (LOQ) ranged from 5 to 15 μg/L for four elements in the analyses (see details in Supplementary Material). Me/Ca ratios were calculated and applied in the subsequent statistical analyses and discussions.
Cuttlebone growth patterns are discriminative, and the outermost portion of cuttlebone was obtained for chemical analyses. Organic matter in the lamellar microstructure (Florek et al., 2009), which cannot be degraded in the cleaning procedure using H2O2, may bias the chemical concentration of cuttlebone carbonate. Therefore, pieces of cuttlebone were immersed overnight in reagent grade 6% NaClO to degrade structural organic material. After removing the supernatant solution, the cuttlebone sample that remained in the vials was immersed in MilliQ water for ultrasonic cleaning for 1 min. The samples were washed in MilliQ water three times, and then the cuttlebone samples were transferred to a new acid-washed vial and subjected to three additional MilliQ cleanings of 1 min each using an ultrasonic cleaner. After cleaning, the cuttlebone was dissolved in 0.3 N HNO3 and diluted to 100 ppm Ca. High-resolution inductively coupled plasma mass spectrometry (HR-ICP-MS; Thermo Fisher Scientific, Element 2) was used to analyze samples at the Isotope Geochemistry Lab, Department of Earth Sciences, National Cheng Kung University, Taiwan. The aragonite reference material, FEBS-1 (Otolith-certified reference material for trace metals, National Research Council, Canada) was used to determine the element concentrations in samples, and JCp-1 (coral standards) was measured every nine samples as an internal standard to monitor the instrument drift. The measured elemental concentration of JCp-1 was also checked against certified values to ensure that the analysis was accurate and precise. Four isotopes were analyzed in low-resolution mode: 7Li, 25Mg, 43Ca, and 86Sr. The LOD and LOQ ranged from 0.03 to 5 μg/L, depending on the element (see details in Supplementary Material). Precision of analyses (RSD) was Li/Ca: 5.2%, Mg/Ca: 4.0%, Sr/Ca: 3.8%, and accuracy (% error) was Li/Ca: 9.9%, Mg/Ca: -4.7%, Sr/Ca:4.6%.
The elemental partition coefficient was calculated as follows:
An average value from each temperature-controlled tank was applied to the Me/Carearing seawater value to determine individual DMe/Ca values. First, we used the Shapiro–Wilk test to evaluate the normality of data and Levene’s test to examine the equality of variances among temperature treatments. A one-way analysis of variance (ANOVA), a Welch’s ANOVA (for heterogeneity), or a Kruskal–Wallis test (for non-normal distribution) was conducted to evaluate the effect of water temperature on rearing seawater Me/Ca, DMe/Ca, and cuttlebone growth rate. A follow-up Pearson’s correlation test was performed to examine the relationship between DMe/Ca and cuttlebone growth rate.
Wild Cuttlefish
Sepia pharaonis were collected predominantly at depths of between 30 and 60 m off Donggang, on Taiwan’s southwestern coast (DG population) and between depths of 2 and 10 m around the Penghu Islands in the Taiwan Strait (PH population) from September 2009 to December 2010. The samples were collected by trawling, and latitude, longitude, and depth data were recorded. The 208 captured cuttlefish were weighed, their mantle lengths measured, and their stage of maturity estimated based on the Workshop on Sexual Maturity Staging of Cephalopods (ICES, 2010) criteria. One hundred sixty-four specimens from the DG population were used for the evaluation of the effects of temperature and growth rates on Me/Ca ratios as well as for assessing relationships between male and female cuttlefish and between different maturity stages (1 to 3a; 1: immature, 2a: developing, 2b: maturing, and 3a: mature). In the case of the PH population, only individuals that had reached stage 3a (n = 40) were selected for comparison with the DG population on the basis of Me/Ca ratios. In both populations, growth condition was assessed after calculating Kn according to the following equation (Bello, 2006):
where Wi is the individual body mass (g), ML is the mantle length, and α and β are the parameters estimated from the weight–length relationships of different populations (DG population, α: 3.71 × 10–4 and β: 2.74; PH population: α: 5.66 × 10–4 and β: 2.65).
After dissection from the mantle, cuttlebones were cleaned using 10% H2O2 to remove clinging tissue and washed three times with MilliQ water. They were then dried in an oven at 40°C for 48 h before being cut longitudinally. The width of the final lamella of each cuttlebone was measured to assess the growth rate of cuttlebone carbonate at the time of capture, considering one lamella was consistently formed approximately every 1.75 days from the juvenile stage (Chung and Wang, 2013). The carbonate powder of the last increment was sampled using a dissecting needle. Powder samples were immersed in reagent grade 6% NaClO and maintained overnight to degrade structural organic matter, washed three times with MilliQ water, transferred to a new acid-washed vial, and washed three more times with MilliQ water. All procedures were similar to the steps applied for the reared cuttlefish. The cleaned powder was dissolved in 0.3 N HNO3 and diluted to a concentration of 100 ppm Ca. Samples were analyzed using HR-ICP-MS (Thermo Fisher Scientific, Element XR) at the Institute of Earth Sciences, Academia Sinica, Taiwan. The Me/Ca ratios in the cuttlebone were measured using an in-house standard, the Me/Ca ratios of which had been certified. Repeated measurements of FEBS-1 indicated the precision and accuracy of the analysis. Four isotopes were analyzed in low-resolution mode: 7Li, 25Mg, 43Ca, and 86Sr. The LOD and LOQ ranged from 0.1 to 15 μg/L, depending on the element (see details in Supplementary Material). RSD values were 3.2, 7.2, and 2.1% for Li/Ca, Mg/Ca, and Sr/Ca, respectively, and accuracies (% error) were -3.1, -3.8, and -0.3%, respectively.
Natural seawater was collected regularly from October 2009 to November 2010 in the DG population area and from November 2009 to November 2010 in the PH population area. The daily sea surface temperature at the sampling locations was derived from the database of the Central Weather Bureau, Taiwan1. The collected water samples were filtered with acid wash syringes and 0.22-μm filters, acidified through the addition of ultrapure concentrated HNO3 (J. T. Backer) to maintain the pH at 1, and then stored under freezing conditions. NASS-6 (Seawater reference material for trace metals, National Research Council Canada) was used to determine water elemental concentrations in a series of dilutions from 100 to 300-fold and measured after every nine samples to monitor instrumental drift. The measurement of 200-fold diluted CASS-4 (Nearshore seawater certified reference material for trace metals and other constituents, National Research Council Canada) provided the accuracy measurements of the elemental concentrations (% error: -8.3, 9.1, 4.6, and -1.4% for Li, Mg, Sr, and Ca, respectively. Samples were diluted 200-fold and analyzed using HR-ICP-MS (Thermo Fisher Scientific, Element XR) at the Institute of Earth Sciences, Academia Sinica, Taiwan. The LOD and LOQ ranged from 0.03 to 10 μg/L, depending on the element (see details in Supplementary Material).
We first evaluated the variation of Me/Ca in natural seawater in a time series using an ordinary least squares estimation. Where seawater Me/Ca ratio was demonstrated to not be significantly different in the course of the sampling period, cuttlebone Me/Ca ratios were used directly in subsequent statistical analyses instead of the elemental partition coefficient. Subsequently, the effect of temperature and growth rate, as well as growth rate nested in temperature, were assessed using a multiple linear regression model with backward selection. The model with the best fit was selected based on the lowest Akaike information criterion. The third step was to execute multiple linear regression models to examine the effect of sex and maturity on the relationship between cuttlebone Me/Ca and growth rate. All statistics were performed in R (R Core Team, 2018), and figures were made using the ggplot2 package in R (Wickham, 2009).
Results
Laboratory-Controlled Experiment
The average temperatures recorded for the ideal range in an experimental design were 20.3 ± 0.2°C, 25.4 ± 0.3°C, and 30.6 ± 0.7°C (using a WTW COND 3310 Multi-Mode Water Meter, Kenelec Scientific). The influence of temperature on hatchling growth was significant, with mantle length and body weight both being larger at lower temperatures (one-way ANOVA. Mantle length: n = 31, df = 2, F-value = 116, p < 0.01. Body weight: n = 31, df = 2, F-value = 180, p < 0.01; Table 1). Similarly, cuttlebone grew significantly faster toward lower temperatures (one-way ANOVA, n = 31, df = 2, F-value = 76.7, p < 0.01; Table 1).
Sr/Carearing seawater and Mg/Carearing seawater values varied significantly between temperature treatments (Kruskal–Wallis test. Sr/Carearing seawater: df = 2, chi-squared = 10.62, p < 0.01; Mg/Carearing seawater: df = 2, chi-squared = 6.62, p = 0.03); however, no differences were observed for Li/Carearing seawater values (Kruskal–Wallis test: df = 2, chi-squared = 2.03, p = 0.36; details shown in Table 1). After Me/Carearing seawater had been calibrated and elemental partition coefficients had been determined, DLi/Ca and DMg/Ca showed a decreasing trend with temperature increases (one-way Welch’s ANOVA, DLi/Ca: df = 2, F-value = 639, p < 0.01; DMg/Ca: df = 2, F-value = 59.6, p < 0.01; Figure 2). Contrariwise, DSr/Ca showed a significantly increasing trend with an increase in temperature (one-way Welch’s ANOVA, df = 2, F-value = 34.9, p < 0.01; Figure 2). Different growth rates were observed among temperature groups, and they were significantly correlated with the elemental partition coefficients, with a positive trend for DLi/Ca and DMg/Ca and a negative trend for DSr/Ca (Pearson’s correlation, DLi/Ca: df = 23, t = 11.2, p < 0.01, r = 0.919; DMg/Ca: df = 26, t = 8.31, p < 0.01, r = 0.852; DSr/Ca: df = 27, t = -5.73, p < 0.01, r = -0.741; Figure 2).
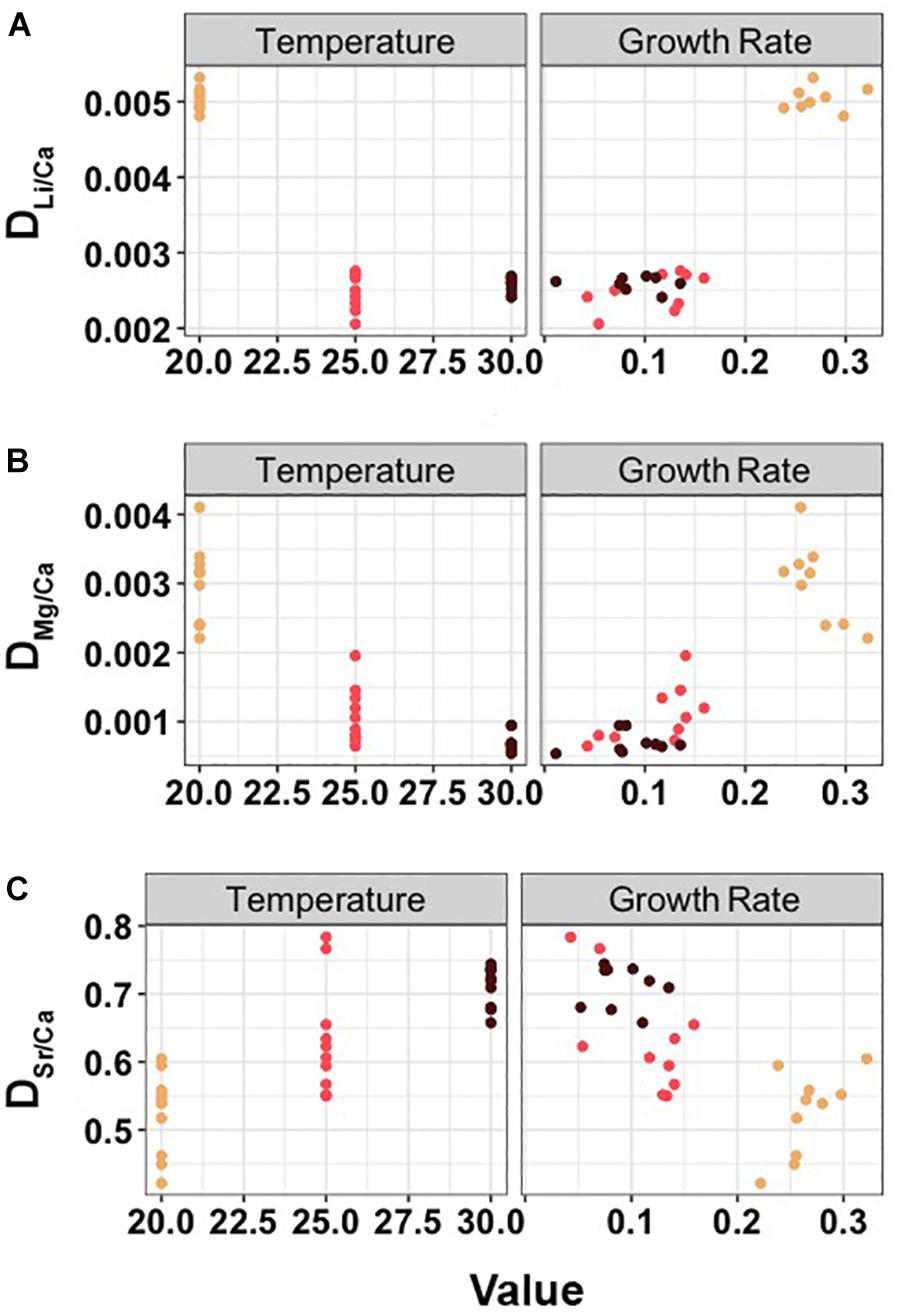
Figure 2. Partition coefficients of Li/Ca (A), Mg/Ca (B) and Sr/Ca (C) among temperature groups and in relation to cuttlebone growth rate.
Wild Cuttlefish
The 168 individual cuttlefish captured in the DG population comprised 96 females and 72 males (Table 2). Few were immature (maturity stage 1), and the largest proportion of specimens for both sexes was at stage 3. Both mantle length and body mass were generally larger in females at every maturity stage.
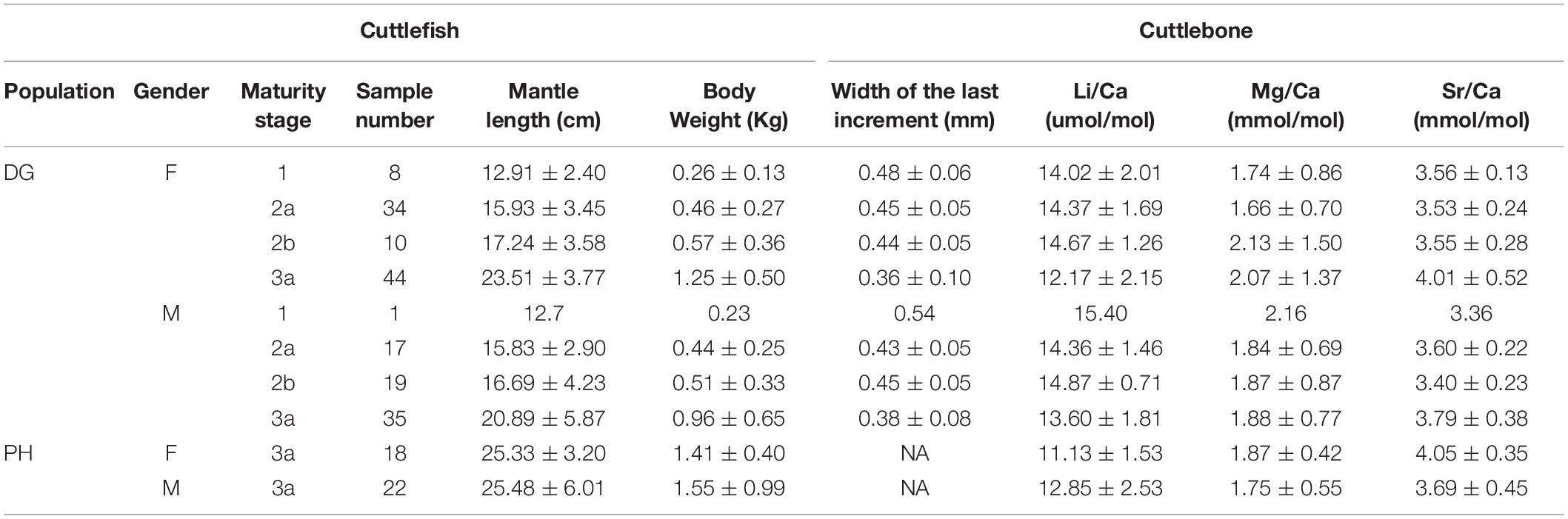
Table 2. Wild cuttlefish data used to evaluate cuttlebone Me/Ca (element/calcium) ratios (1, immature; 2a, developing; 2b, maturing; and 3a, mature).
Sea surface temperature during the sampling period ranged from 24 to 31°C, and seawater Me/Ca values were fairly consistent and not significantly different across months and years, with the average values being 2.38 ± 0.08, 28.0 ± 5.61, and 7.57 ± 0.92 mmol/mol for Li/Ca, Mg/Ca, and Sr/Ca, respectively (Table 3). There was negligible variation in seawater Me/Ca with time; therefore, instead of an element partition coefficient, cuttlebone Me/Ca values in wild cuttlefish were used in the subsequent statistical analyses.
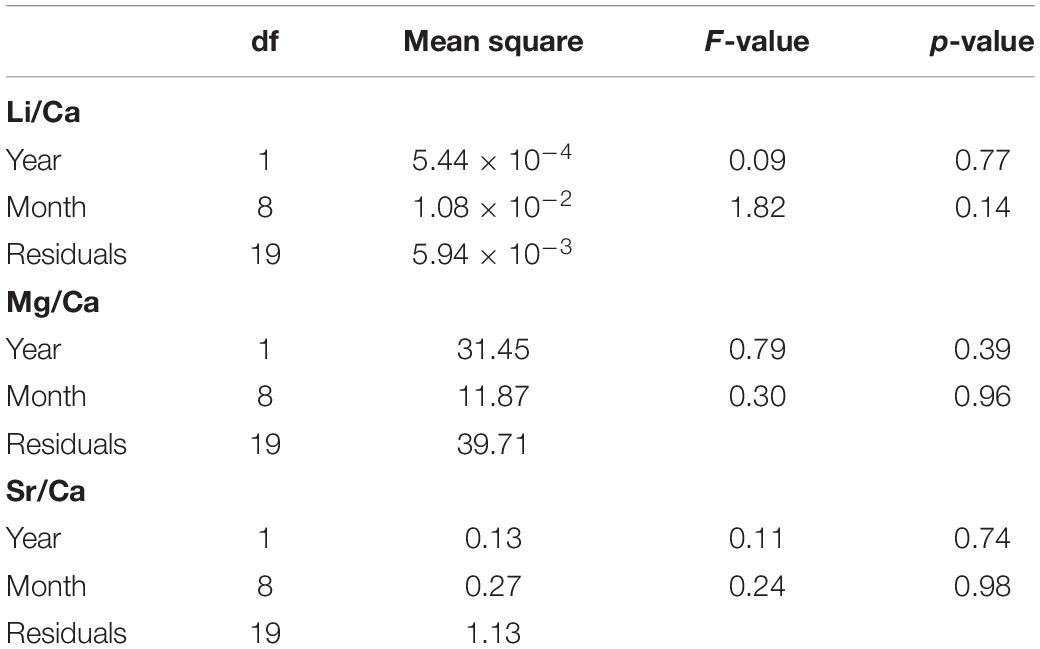
Table 3. Linear regression analysis (ordinary least squares) of natural seawater Me/Ca (element/calcium) in time series.
Multiple linear regression models with a backward selection method were applied to determine the best-fit model that described the levels of the effects of temperature and growth rate on Me/Ca ratios. For all three Me/Ca ratios, the best fit was explained by temperature and growth rate (increment width) with no interaction between the two factors, and the residuals in the best-fit model showed normality and homogeneity. Temperature and growth rate (increment width) explained 56% and 43% of the variation in cuttlebone Li/Ca and Sr/Ca ratios, respectively, but no significance of variables was observed in cuttlebone Mg/Ca. The model showed that growth rate contributed to more variation in the cuttlebone Li/Ca and Sr/Ca ratios than temperature. For example, a 1°C increase in temperature reduced the cuttlebone Li/Ca ratio by 0.11, and a 7°C difference in overall temperature records changed cuttlebone Li/Ca ratio by only 0.77. The difference was negligible when compared with the 7.6 to 18.4 cuttlebone Li/Ca ratio range. Similarly, temperature had a minor influence on cuttlebone Sr/Ca values, which fell by only 0.024 with each 1°C decrease in temperature. Therefore, we normalized the temperature at 24°C for both Li/Ca and Sr/Ca values and examined how Me/Ca and growth rate were related both between the sexes and at different maturity stages.
The temperature-corrected cuttlebone Li/Ca ratio increased linearly with an increase in growth rate, and there was no difference in slope between female and male cuttlefish. However, the intercept value was higher for the male cuttlefish (Table 4 and Figure 3). The linear relationship was consistent at most of the maturity stages and in either sex, with the exception of females at stage 2a and males at stage 2b. Conversely, the temperature-corrected cuttlebone Sr/Ca ratio decreased exponentially with an increase of growth rate, and the Sr/Ca ratio trend was similar between both sexes and in the maturity stages, with the exception of females at stage 2a (Table 4 and Figure 3).
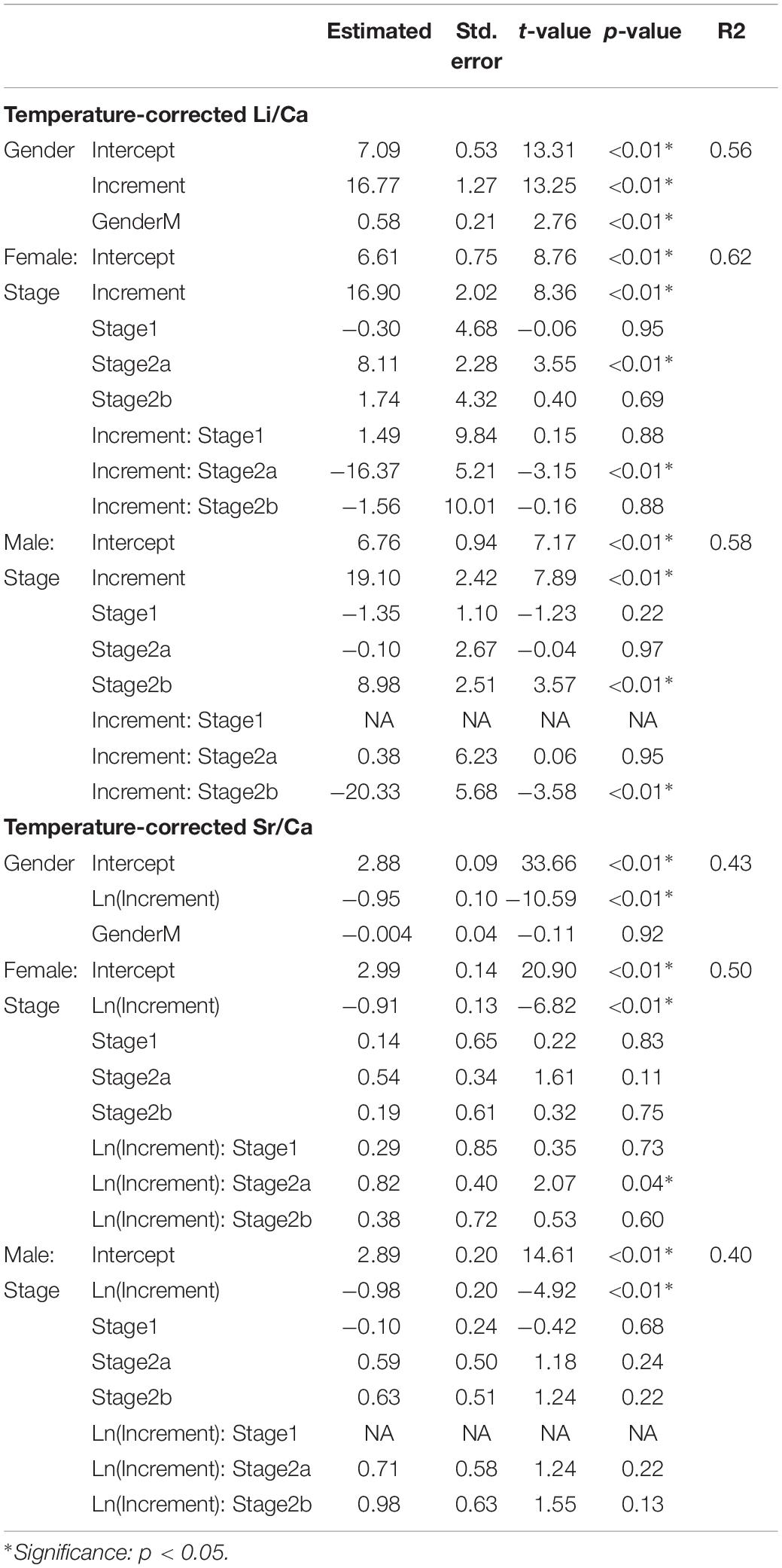
Table 4. Multiple linear regression analyses of temperature-corrected cuttlebone Me/Ca (element/calcium) between sexes and among maturity stages.
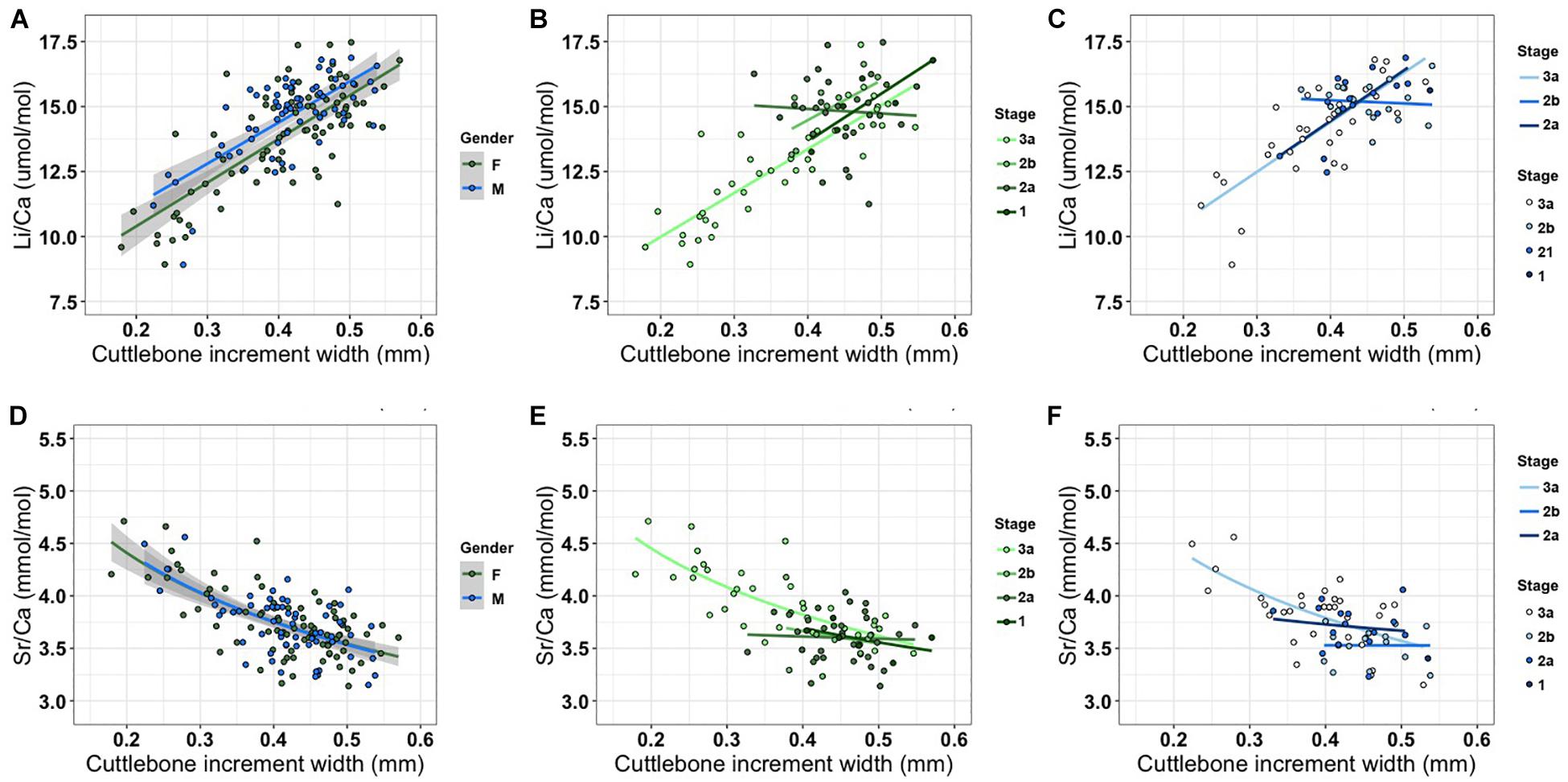
Figure 3. Relationship between temperature-corrected Li/Ca (A–C) and Sr/Ca (D–F) and the width of last increment in cuttlebone. Increment width refers to the growth rate in wild cuttlefish. Comparisons among maturity stages are given for females (B,E) and males (C,F). Results of multiple linear regression are presented in Table 4 (1, immature; 2a, developing; 2b, maturing; and 3a, mature).
Comparisons Between Two Populations From Different Areas
Forty cuttlefish at stage 3a were captured from the PH population, comprising 18 females and 22 males (Table 2). The females were smaller than the males, which was opposite to the trend observed in the cuttlefish from the DG population. Sea surface temperatures ranged from 19.5 to 26.3°C, and temperature correction of cuttlebone Li/Ca and Sr/Ca ratios followed the aforementioned approaches, where Li/Ca and Sr/Ca values were normalized at 24°C and calibrated as -0.11 and -0.024 per°C, respectively. We modeled temperature-corrected Me/Ca ratios with Kn between populations. Table 5 summarizes the model output and displays a significant positive relationship between temperature-corrected Li/Ca ratios and Kn. However, the slope was different between populations of females from each of the study sites. A reverse trend for Kn was revealed in the temperature-corrected Sr/Ca ratio, and the significance was only observed in females.
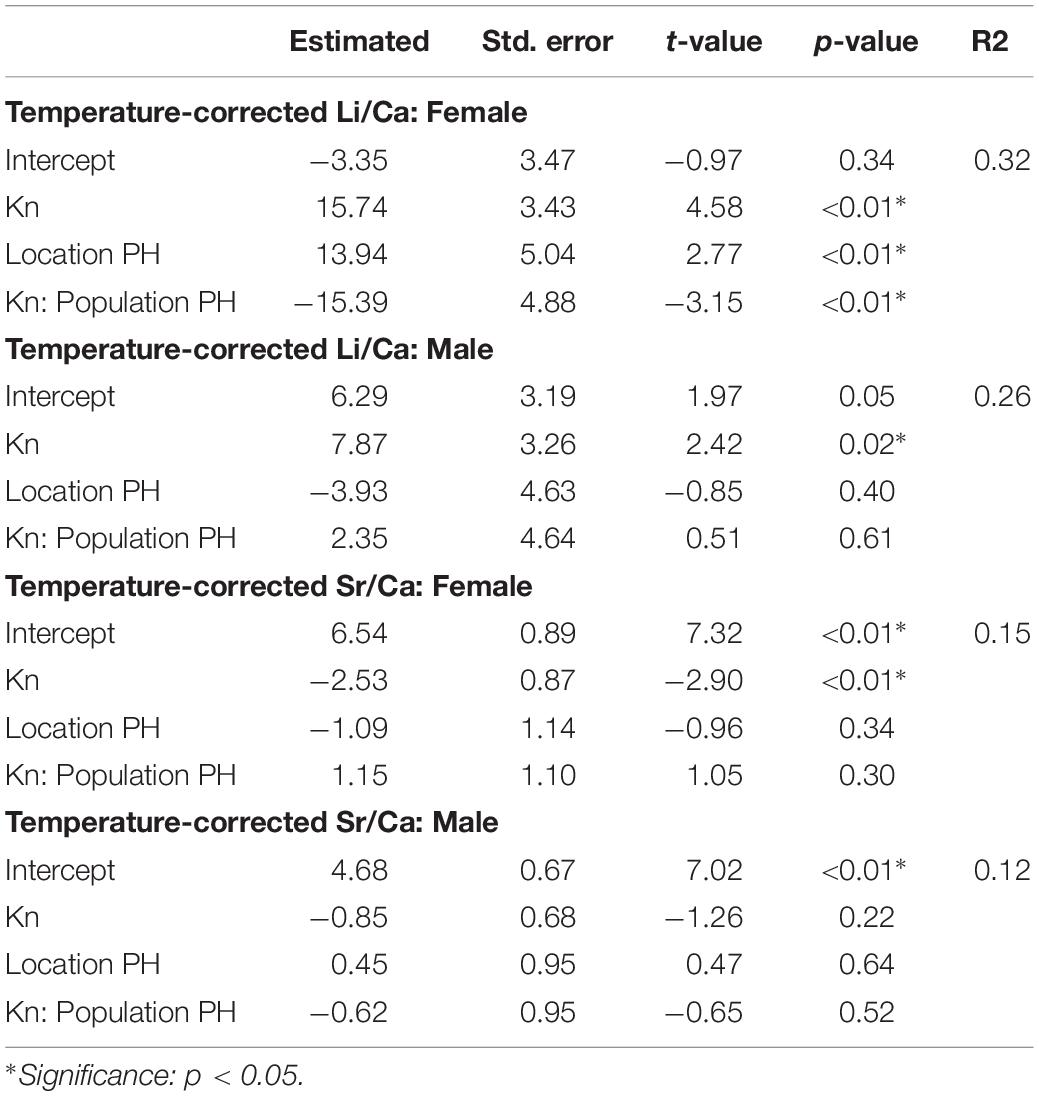
Table 5. Multiple linear regression analyses of temperature-corrected cuttlebone Me/Ca (element/calcium) with Kn (relative growth condition) values between two populations (DG and PH).
Discussion
Elemental incorporation into inorganic carbonates should adhere to thermodynamic and kinetic laws, but elemental signals recorded in biogenic carbonates deviate from the scheme due to the influence of physiological factors. Me/Ca ratios in biogenic aragonite carbonate have been studied in bivalve shells, coral, and fish otoliths. However, relatively few studies have been conducted on cephalopod hard structures such as statoliths and cuttlebone. To the best of our knowledge, this represents the first study to assess Li/Ca, Mg/Ca, and Sr/Ca ratios in cuttlebone carbonate. Its functional structure makes cuttlebone unique among biogenic aragonite carbonates because growth or lamellar formation is closely correlated with an increase in mantle length. Our results show that both temperature and growth rates influence elemental incorporation, with some variations across the elements studied. We compared the results of controlled experiments in the laboratory with data obtained from wild cuttlefish in the field to comprehensively evaluate the factors influencing trace element incorporation.
Li/Ca
The relationship between Li/Ca and temperature is not consistent among all biogenic carbonates. Thermodynamics would predict an increase in carbonate Li/Ca with an increase in temperature (Hall and Chan, 2004), and that is the case in the shells of some bivalve species (Thébault et al., 2009). However, temperature has a negative effect on the Li/Ca ratio in corals, brachiopods, benthic foraminifera (Marriott et al., 2004b; Raddatz et al., 2013) and cuttlebone in the present study. According to the exponential curve of the temperature-dependent relationship demonstrated by Marriott et al. (2004a), cuttlebone DLi/Ca decreases 6.6% per°C, which is a higher rate than in inorganic calcite (4.6% per°C, Marriott et al., 2004a) and in Porites coral (4.9% per°C, Marriott et al., 2004a) but not as high as in cold water coral (Lophelia pertusa, 7.3% per°C, Raddatz et al., 2013). However, the effect of temperature on cuttlebone DLi/Ca values was not evaluated based on similar growth rate. Therefore, the effect could be overestimated when an increasing growth rate in the warmer environment increases the DLi/Ca values.
Growth rate and temperature are both important factors that contribute to the variation in cuttlebone Li/Ca ratios in wild cuttlefish. The range of temperature in natural habitats is 7°C, which is less than the laboratory test range of 10°C. In addition, wild specimens exhibit more variable growth rates. Therefore, we evaluated the growth rate in wild cuttlebone by correcting the influence of temperature on the wild cuttlebone Li/Ca ratio. After temperature correction, the Li/Ca ratio presented a convincing linear and positive relationship with cuttlebone growth rates (Figure 3). The positive relationship between carbonate Li/Ca values and calcification rates has been observed in synthetic aragonite (Gabitov et al., 2011) and mollusk shells (Thébault et al., 2009; Thébault and Chauvaud, 2013). In addition, the positive relationships further indicate that cuttlebone Li/Ca is a potential proxy for cuttlefish growth based on increases in cuttlebone Li/Ca values.
Before using cuttlebone Li/Ca as a proxy for growth rate, we evaluated the Li/Ca relationship between sexes and among maturity stages. There were no differences in the slopes between cuttlebone Li/Ca ratios and growth rates at maturity between females and males, excluding females at the 2a stages and males at the 2b stages (Figure 3). Uncoupling of cuttlebone Li/Ca and increased width may be due to a change in the frequency of lamellar formation, which could be associated with life stage (Chung and Wang, 2013), food availability (le Goff et al., 1998; Martínez et al., 2011), breeding (le Goff et al., 1998), or mechanical buoyancy control (Denton and Gilpin-Brown, 1961). Overall, the cuttlebone Li/Ca ratio could be applied as a growth proxy in most maturation stages; however, further investigations into the uncoupling trends observed in the present study are required.
Mg/Ca
There has been a lot of discussion on the application of carbonate Mg/Ca values in paleotemperature reconstructions. Abiotic aragonite obtained from a precipitation experiment showed that the DMg/Ca values were negative and decreased exponentially with a decrease in temperature (Gaetani and Cohen, 2006). However, planktonic foraminifera (Lea, 2003), coral (Gagnon et al., 2007; Reynaud et al., 2007), and mollusk shell Mg/Ca (Klein et al., 1996; Freitas et al., 2006) exhibited a positive relationship with temperature, although the slope varies based on taxa and species. The physiological regulation associated with growth or calcification rate potentially alters the partition coefficient of Mg incorporated into biogenic carbonate. For example, Reynaud et al. (2007) reported a direct temperature effect, temperature-induced growth, and light-induced growth as the three major factors influencing coralline Mg/Ca. Our controlled laboratory experiment confirmed that cuttlebone DMg/Ca varies based on temperature and growth rate. We observed a negative trend between Mg/Ca and temperature in cuttlebone, which is consistent with the observation in abiotic aragonite. However, our results also revealed a growth rate associated with the variation in cuttlebone Mg/Ca. The mechanical underpinning of the growth rate effect on Mg/Ca in biogenic carbonate is unclear. For example, the Mg/Ca value of biogenic carbonate is positively correlated with growth rate in corals (Reynaud et al., 2007), but negatively correlated with growth rate in otoliths (Martin and Thorrold, 2005). The effect of growth rate is even species dependent in bivalve shells (Carré et al., 2006; Schöne et al., 2011). Furthermore, there is no clear cuttlebone Mg/Ca incorporation trend in wild cuttlefish, either in relation to temperature or in relation to growth rate. The complex mechanism of the incorporation of Mg into biogenic carbonates has recently been explained by a metabolic control (DiMaria et al., 2010; Schöne et al., 2011). However, our results are limited to explain the metabolic effect on Mg/Ca ratio in cuttlebone and do not suggest that cuttlebone Mg/Ca is a proxy for any environmental or physiological condition with regard to wild cuttlefish.
Sr/Ca
According to thermodynamics, Sr/Ca ratios exhibit a negative relationship with temperature in inorganic aragonite (Kinsman and Holland, 1969) as well as in biogenic aragonite, such as corals (Goodkin et al., 2005; Gagnon et al., 2007; Reynaud et al., 2007) and squid statolith (Ikeda et al., 1998; Arkhipkin et al., 2004). Nevertheless, temperature may not influence cuttlefish statoliths (Zumholz et al., 2007) or may exhibit reverse influences on cuttlefish statoliths between prehatching and posthatchling (Gillanders et al., 2013). We also observed a contrary effect of temperature on cuttlebone Sr/Ca values, which was positive for reared cuttlefish but negative for wild cuttlefish. The observation could indicate other factors beyond the effect of temperature on cuttlebone Sr/Ca ratio.
Several studies have reported a considerable influence of calcification rate on biogenic carbonate Sr/Ca; for example, in corals (Reynaud et al., 2007) and in bivalve shells (Gillikin et al., 2005; Carré et al., 2006; Schöne et al., 2011). The influence of calcification rate on biogenic carbonate Sr/Ca could suppress the influence of temperature. In our controlled laboratory experiment, the positive relationship between temperature and cuttlebone DSr/Ca is inconsistent with what has been observed in other biogenic carbonates as well as with theoretical predictions. When growth rate is taken into account in the evaluation of wild cuttlefish using a multiple linear regression model, the influence exerted by temperature on cuttlebone Sr/Ca is negative (linear relationship, -24 μmol/mol per°C), which is comparable to the observation for inorganic aragonite (linear relationship, -44 μmol/mol per°C; Gagnon et al., 2007). The finding reinforces the view that Sr/Ca ratio evaluation should consider growth rate.
However, the effect of growth rate on carbonate Sr/Ca values is not equal across all taxa and species because the effect could be positive, negative, or have no effect on shells in different bivalve species (Gillikin et al., 2005; Carré et al., 2006; Reynaud et al., 2007; Schöne et al., 2011; Zhao et al., 2017). Our findings in S. pharaonis cuttlebone are consistent with the results obtained for the shell of Arctica islandica (Schöne et al., 2011) but are inconsistent with the results of a precipitation experiment on abiotic aragonite (Gaetani and Cohen, 2006). According to Sturrock et al. (2015), blood Sr/Ca values and related physiological characteristics explain the reduced fish otolith Sr/Ca values while otolith growth increases. In such cases, physiological processes and body fluid chemistry influence the relationship between carbonate Sr/Ca and calcification rate in a way that could explain differences between taxa and species. Therefore, it would be inappropriate to use cuttlebone Sr/Ca as a proxy for growth rate currently due to the inconsistent findings in the literature and because the mechanism by which Sr/Ca in biogenic carbonate is influenced physiologically remains unclear.
Cuttlebone Li/Ca as an Indicator of Growth Rate in Wild Cuttlefish Populations
The Kn value represents a relative growth condition in animals: values higher than 1 signify greater weight than other individuals of the same length in a population, and values lower than 1 signify the reverse (Bello, 2006). Comparing individuals at stage 3a in both the DG and PH populations, it revealed a positive correlation between Kn values and temperature-corrected Li/Ca, indicating that individuals with superior growth conditions had higher growth rates; moreover, sex and location influence the pattern. For example, female cuttlefish from the DG population exhibited an increasing Kn trend that was relatively sharp compared with the trend in the PH population. However, the Kn trend was similar in male cuttlefish between the two populations (Table 5 and Figure 4).
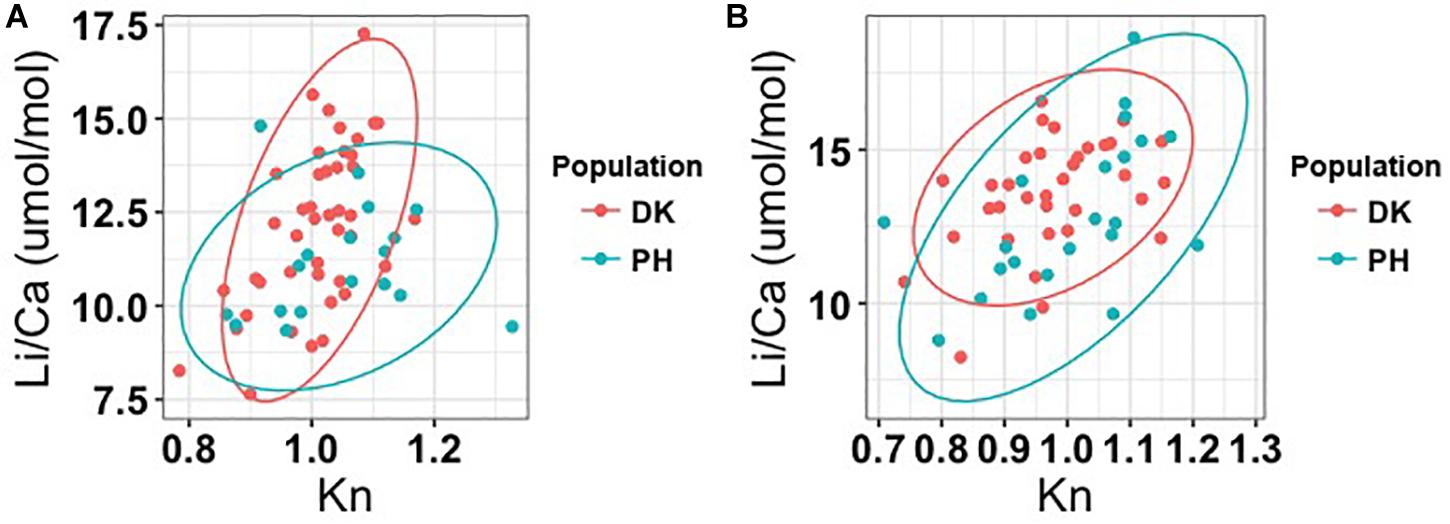
Figure 4. Relationship between temperature-corrected Li/Ca and Kn (relative growth condition) values between two study populations. Only individuals at stage 3a were used in the analysis. Comparisons are given for females (A) and males (B). Results of multiple linear regression are presented in Table 5.
These Li/Ca and Kn value trends between sexes and populations provide information that could facilitate fishery management. For example, capturing cuttlefish with higher Kn values (better growth conditions) would imply removing bigger and heavier individuals from the ecosystem. At the same time, individuals with higher growth rates (higher Li/Ca values) are removed from the ecosystem. Such a strategy may exert a stronger effect on the females in the DG population than on the females in the PH population, because DG population has higher growth rates than PH population does at the same level of growth conditions. Consequently, low growth rate parents left in the ecosystem may produce low growth rate offspring. This phenomenon will be more obvious in the females of the DG population. Although, more investigations should be conducted on the relationships between elemental ratios and cuttlefish growth condition before a comprehensive tool for the management of cuttlefish fisheries can be developed, our results provide additional information for management and policy making to cuttlefish fisheries.
Implications
This study demonstrated that cuttlebone Li/Ca is a potential geochemical proxy that could enhance our understanding of cuttlefish growth and ecology. The proxy has a consistent relationship with cuttlebone growth rate in terms of mantle length growth rate. The use of a geochemical proxy has numerous benefits for cuttlefish ecology. First, it would be possible to reconstruct the growth rate history of an individual based on the results of the elemental analysis of cuttlebone carbonate formed at different life stages. Second, the elemental signals recorded in cuttlebone can be used to discriminate between populations and stocks (Ikeda et al., 1999; Turan and Yaglioglu, 2010). Integrating a growth rate proxy and other trace element signals would also facilitate the assessment of growth rates among identified population and stocks. Third, cuttlebone oxygen isotope values could reveal temperature regimes (Bettencourt and Guerra, 1999; Rexfort and Mutterlose, 2006). Applying a multiple geochemical approach along a cuttlebone growth transection enables the reconstruction of the life history of a cuttlefish and an understanding of its growth conditions at different temperatures. Finally, it could be possible to apply such proxies to ancient cephalopods such as belemnites (Ullmann et al., 2013; Immenhauser et al., 2016) through validation from modern cephalopod species such as cuttlefish in the study.
Data Availability Statement
Publicly available datasets were analyzed in this study. All the data are presented in the manuscript.
Ethics Statement
Ethical review and approval was not required for the animal study because the study is using the biogenic carbonate structure, which was reserved by a previous published study.
Author Contributions
C-HW led this work. C-HW and M-TC designed and conducted the experiments. M-TC and K-FH conducted the elemental analyses. C-FY assisted in the elemental analyses. C-CC assisted the wild cuttlefish collections. All authors contributed to the manuscript preparation.
Funding
This study was supported by the Ministry of Science and Technology, Taiwan (MOST 98-2628-B-007-001-MY3 and MOST 102-2621-B-019-006-MY3).
Conflict of Interest
The authors declare that the research was conducted in the absence of any commercial or financial relationships that could be construed as a potential conflict of interest.
Acknowledgments
We would like to thank Hui-Lun Chen and Lu-Peng Wang for assistance with cuttlefish collection, cuttlebone preparation, and measurement of lamellar widths, Dr. Hou-Chun Liu for assistance in water sample analyses, and Dr. Amy Featherstone for helpful suggestions for the manuscript. This manuscript was edited by Wallace Academic Editing.
Supplementary Material
The Supplementary Material for this article can be found online at: https://www.frontiersin.org/articles/10.3389/fmars.2019.00796/full#supplementary-material
Footnotes
References
Arkhipkin, A. I., Campana, S. E., FitzGerald, J., and Thorrold, S. R. (2004). Spatial and temporal variation in elemental signatures of statoliths from the Patagonian longfin squid (Loligo gahi). Can. J. Fish. Aquat. Sci. 61, 1212–1224. doi: 10.1139/f04-075
Bath, G. E., Thorrold, S. R., Jones, C. M., Campana, S. E., McLaren, J. W., and Lam, J. W. H. (2000). Strontium and barium uptake in aragonitic otoliths of marine fish. Geochim. Cosmochim. Acta 64, 1705–1714. doi: 10.1016/S0016-7037(99)00419-6
Bello, G. (2006). Tentacle club length and body condition in the cuttlefishes Sepia elegans Blainville, 1827 and Sepia orbignyana Férussac, 1826 (Cephalopoda: Sepiidae). Zool. Anz. 244, 187–192. doi: 10.1016/j.jcz.2005.10.001
Bettencourt, V., and Guerra, A. (1999). Carbon- and oxygen-isotope composition of the cuttlebone of Sepia officinalis: a tool for predicting ecological information? Mar. Biol. 133, 651–657. doi: 10.1007/s002270050505
Bettencourt, V., and Guerra, A. (2001). Age studies based on daily growth increments in statoliths and growth lamellae in cuttlebone of cultured Sepia officinalis. Mar. Biol. 139, 327–334. doi: 10.1007/s002270100582
Birchall, J. D., and Thomas, N. L. (1983). On the architecture and function of cuttlefish bone. J. Mater. Sci. 18, 2081–2086. doi: 10.1007/BF00555001
Carré, M., Bentaleb, I., Bruguier, O., Ordinola, E., Barrett, N. T., and Fontugne, M. (2006). Calcification rate influence on trace element concentrations in aragonitic bivalve shells: evidences and mechanisms. Geochim. Cosmochim. Acta 70, 4906–4920. doi: 10.1016/j.gca.2006.07.019
Chang, M. Y., and Geffen, A. J. (2013). Taxonomic and geographic influences on fish otolith microchemistry. Fish Fish. 14, 458–492. doi: 10.1111/j.1467-2979.2012.00482.x
Chung, M.-T., and Wang, C.-H. (2013). Age validation of the growth lamellae in the cuttlebone from cultured Sepia pharaonis at different stages. J. Exp. Mar. Biol. Ecol. 447, 132–137. doi: 10.1016/j.jembe.2013.02.020
Corrège, T. (2006). Sea surface temperature and salinity reconstruction from coral geochemical tracers. Palaeogeogr. Palaeoclimatol. Palaeoecol. 232, 408–428. doi: 10.1016/j.palaeo.2005.10.014
Denton, E. J., and Gilpin-Brown, J. B. (1961). The distribution of gas and liquid within the cuttlebone. J. Mar. Biol. Assoc. United Kingdom 41, 365–381. doi: 10.1017/S0025315400023973
DiMaria, R. A., Miller, J. A., and Hurst, T. P. (2010). Temperature and growth effects on otolith elemental chemistry of larval Pacific cod, Gadus macrocephalus. Environ. Biol. Fishes 89, 453–462. doi: 10.1007/s10641-010-9665-2
D’Olivo, J. P., Sinclair, D. J., Rankenburg, K., and McCulloch, M. T. (2018). A universal multi-trace element calibration for reconstructing sea surface temperatures from long-lived Porites corals: removing “vital-effects.”. Geochim. Cosmochim. Acta 239, 109–135. doi: 10.1016/j.gca.2018.07.035
Florek, M., Fornal, E., Gómez-Romero, P., Zieba, E., Paszkowicz, W., Lekki, J., et al. (2009). Complementary microstructural and chemical analyses of Sepia officinalis endoskeleton. Mater. Sci. Eng. C 29, 1220–1226. doi: 10.1016/j.msec.2008.09.040
Freitas, P. S., Clarke, L. J., Kennedy, H., Richardson, C. A., and Abrantes, F. (2006). Environmental and biological controls on elemental (Mg/Ca, Sr/Ca and Mn/Ca) ratios in shells of the king scallop Pecten maximus. Geochim. Cosmochim. Acta 70, 5119–5133. doi: 10.1016/j.gca.2006.07.029
Gabitov, R. I., Schmitt, A. K., Rosner, M., McKeegan, K. D., Gaetani, G. A., Cohen, A. L., et al. (2011). In situ δ7Li, Li/Ca, and Mg/Ca analyses of synthetic aragonites. Geochem. Geophys. Geosyst. 12:Q03001. doi: 10.1029/2010GC003322
Gaetani, G. A., and Cohen, A. L. (2006). Element partitioning during precipitation of aragonite from seawater: a framework for understanding paleoproxies. Geochim. Cosmochim. Acta 70, 4617–4634. doi: 10.1016/j.gca.2006.07.008
Gagnon, A. C., Adkins, J. F., Fernandez, D. P., and Robinson, L. F. (2007). Sr/Ca and Mg/Ca vital effects correlated with skeletal architecture in a scleractinian deep-sea coral and the role of Rayleigh fractionation. Earth Planet. Sci. Lett. 261, 280–295. doi: 10.1016/j.epsl.2007.07.013
Gillanders, B. M., Wilkinson, L. M., Munro, A. R., and de Vries, M. C. (2013). Statolith chemistry of two life history stages of cuttlefish: effects of temperature and seawater trace element concentration. Geochim. Cosmochim. Acta 101, 12–23. doi: 10.1016/j.gca.2012.10.005
Gillikin, D. P., Lorrain, A., Navez, J., Taylor, J. W., André, L., Keppens, E., et al. (2005). Strong biological controls on Sr/Ca ratios in aragonitic marine bivalve shells. Geochem. Geophys. Geosyst. 6:Q05009. doi: 10.1029/2004GC000874
Goodkin, N. F., Hughen, K. A., Cohen, A. L., and Smith, S. R. (2005). Record of little ice age sea surface temperatures at Bermuda using a growth-dependent calibration of coral Sr/Ca. Paleoceanography 20:A4016. doi: 10.1029/2005PA001140
Grammer, G. L., Morrongiello, J. R., Izzo, C., Hawthorne, P. J., Middleton, J. F., and Gillanders, B. M. (2017). Coupling biogeochemical tracers with fish growth reveals physiological and environmental controls on otolith chemistry. Ecol. Monogr. 87, 487–507. doi: 10.1002/ecm.1264
Hall, J. M., and Chan, L. H. (2004). Li/Ca in multiple species of benthic and planktonic foraminifera: thermocline, latitudinal, and glacial-interglacial variation. Geochim. Cosmochim. Acta 68, 529–545. doi: 10.1016/S0016-7037(03)00451-4
ICES (2010). Report of the Workshop on Sexual Maturity Staging of Cephalopods (WKMSCEPH). Copenhagen: ICES.
Ikeda, Y., Arai, N., Sakamoto, W., Kodokoro, H., and Yoshida, K. (1998). Microchemistry of the Statoliths of the Japanese common squid Todarodes pacificus with special reference to its relation to the vertical temperature profiles of squid habitat. Fish. Sci. 64, 179–184. doi: 10.2331/fishsci.64.179
Ikeda, Y., Arai, N., Sakamoto, W., and Yoshida, K. (1999). Trace elements in cephalopod calcified tissue: cuttlebone as a possible tracer for life historical events of cuttlefish. Int. J. PIXE 09, 335–343. doi: 10.1142/S0129083599000437
Immenhauser, A., Schöne, B. R., Hoffmann, R., and Niedermayr, A. (2016). Mollusc and brachiopod skeletal hard parts: intricate archives of their marine environment. Sedimentology 63, 1–59. doi: 10.1111/sed.12231
Kinsman, D. J. J., and Holland, H. D. (1969). The co-precipitation of cations with CaCO3—IV. The co-precipitation of Sr2+ with aragonite between 16° and 96°C. Geochim. Cosmochim. Acta 33, 1–17. doi: 10.1016/0016-7037(69)90089-1
Klein, R. T., Lohmann, K. C., and Thayer, C. W. (1996). Bivalve skeletons record sea-surface temperature and δ18O via Mg/Ca and 18O/16O ratios. Geology 24, 415–418.
le Goff, R., Gauvrit, E., Pinczon Du Sel, G., and Daguzan, J. (1998). Age group determination by analysis of the cuttlebone of the cuttlefish Sepia Officinalis L. in reproduction in the bay of Biscay. J. Mollus. Stud. 64, 183–193. doi: 10.1093/mollus/64.2.183
Lea, D. W. (2003). “Elemental and isotopic proxies of past ocean temperatures,” in Treatise on Geochemistry, eds H. Holland, and K. Turekian, (Amsterdam: Elsevier), 1–26. doi: 10.1016/B0-08-043751-6/06114-4
Lu, C. C., and Chung, W. S. (2017). Guide to the Cephalopods of Taiwan. Taichung: Huayu Nature Book Trade.
Marriott, C. S., Henderson, G. M., Belshaw, N. S., and Tudhope, A. W. (2004a). Temperature dependence of δ7Li, δ44Ca and Li/Ca during growth of calcium carbonate. Earth Planet. Sci. Lett. 222, 615–624. doi: 10.1016/j.epsl.2004.02.031
Marriott, C. S., Henderson, G. M., Crompton, R., Staubwasser, M., and Shaw, S. (2004b). Effect of mineralogy, salinity, and temperature on Li/Ca and Li isotope composition of calcium carbonate. Chem. Geol. 212, 5–15. doi: 10.1016/j.chemgeo.2004.08.002
Martin, G. B., and Thorrold, S. R. (2005). Temperature and salinity effects on magnesium, manganese, and barium incorporation in otoliths of larval and early juvenile spot Leiostomus xanthurus. Mar. Ecol. Prog. Ser. 293, 223–232. doi: 10.3354/meps293223
Martínez, P., Bettencourt, V., Guerra, A., and Moltschaniwskyj, N. A. (2011). How temperature influences muscle and cuttlebone growth in juvenile cuttlefish (Sepia elliptica) (Mollusca: Cephalopoda) under conditions of food stress. Can. J. Zool. 78, 1855–1861. doi: 10.1139/z00-115
Mehanna, S. F., Al-Kharusi, L., and Al-Habs, S. (2014). Population dynamics of the pharaoh cuttlefish Sepia pharaonis (Mollusca: Cephalopoda) in the Arabian Sea coast of Oman. Indian J. Fish. 61, 7–11.
Minton, J. W. (2004). The pattern of growth in the early life cycle of individual Sepia pharaonis. Mar. Freshw. Res. 55, 415–422.
R Core Team (2018). R: A language and Environment for Statistical Computing. Available at: http://www.r-project.org/ (accessed January 1, 2018).
Raddatz, J., Liebetrau, V., Rüggeberg, A., Hathorne, E., Krabbenhöft, A., Eisenhauer, A., et al. (2013). Stable Sr-isotope, Sr/Ca, Mg/Ca, Li/Ca and Mg/Li ratios in the scleractinian cold-water coral Lophelia pertusa. Chem. Geol. 352, 143–152. doi: 10.1016/j.chemgeo.2013.06.013
Rexfort, A., and Mutterlose, J. (2006). Stable isotope records from Sepia officinalis—a key to understanding the ecology of belemnites? Earth Planet. Sci. Lett. 247, 212–221. doi: 10.1016/j.epsl.2006.04.025
Reynaud, S., Ferrier-Pagès, C., Meibom, A., Mostefaoui, S., Mortlock, R., Fairbanks, R., et al. (2007). Light and temperature effects on Sr/Ca and Mg/Ca ratios in the scleractinian coral Acropora sp. Geochim. Cosmochim. Acta 71, 354–362. doi: 10.1016/j.gca.2006.09.009
Schöne, B. R., Zhang, Z., Radermacher, P., Thébault, J., Jacob, D. E., Nunn, E. V., et al. (2011). Sr/Ca and Mg/Ca ratios of ontogenetically old, long-lived bivalve shells (Arctica islandica) and their function as paleotemperature proxies. Palaeogeogr. Palaeoclimatol. Palaeoecol. 302, 52–64. doi: 10.1016/j.palaeo.2010.03.016
Sherrard, K. M. (2000). Cuttlebone morphology limits habitat depth in eleven species of Sepia (Cephalopoda: Sepiidae). Biol. Bull. 198, 404–414. doi: 10.2307/1542696
Sturrock, A. M., Hunter, E., Milton, J. A., Johnson, R. C., Waring, C. P., and Trueman, C. N. (2015). Quantifying physiological influences on otolith microchemistry. Methods Ecol. Evol. 6, 806–816. doi: 10.1111/2041-210X.12381
Sturrock, A. M., Trueman, C. N., Darnaude, A. M., and Hunter, E. (2012). Can otolith elemental chemistry retrospectively track migrations in fully marine fishes? J. Fish Biol. 81, 766–795. doi: 10.1111/j.1095-8649.2012.03372.x
Thébault, J., and Chauvaud, L. (2013). Li/Ca enrichments in great scallop shells (Pecten maximus) and their relationship with phytoplankton blooms. Palaeogeogr. Palaeoclimatol. Palaeoecol. 373, 108–122. doi: 10.1016/j.palaeo.2011.12.014
Thébault, J., Schöne, B. R., Hallmann, N., Barth, M., and Nunn, E. V. (2009). Investigation of Li/Ca variations in aragonitic shells of the ocean quahog Arctica islandica, northeast Iceland. Geochem. Geophys. Geosyst. 10:Q12008. doi: 10.1029/2009GC002789
Turan, C., and Yaglioglu, D. (2010). Population identification of common cuttlefish (Sepia officinalis) inferred from genetic, morphometric and cuttlebone chemistry data in the NE Mediterranean Sea. Sci. Mar. 74, 77–86. doi: 10.3989/scimar.2010.74n107
Ullmann, C. V., Campbell, H. J., Frei, R., Hesselbo, S. P., Pogge von Strandmann, P. A., and Korte, C. (2013). Partial diagenetic overprint of Late Jurassic belemnites from New Zealand: implications for the preservation potential of δ7Li values in calcite fossils. Geochim. Cosmochim. Acta 120, 80–96. doi: 10.1016/j.gca.2013.06.029
Wickham, H. (2009). ggplot2: Elegant Graphics for Data Analysis. Berlin: Springer Science & Business Media, 216.
Zhao, L., Schöne, B. R., and Mertz-Kraus, R. (2017). Controls on strontium and barium incorporation into freshwater bivalve shells (Corbicula fluminea). Palaeogeogr. Palaeoclimatol. Palaeoecol. 465, 386–394. doi: 10.1016/j.palaeo.2015.11.040
Keywords: geochemistry, temperature, growth condition, trace elements, biogenic carbonate
Citation: Chung M-T, Huang K-F, You C-F, Chiao C-C and Wang C-H (2020) Elemental Ratios in Cuttlebone Indicate Growth Rates in the Cuttlefish Sepia pharaonis. Front. Mar. Sci. 6:796. doi: 10.3389/fmars.2019.00796
Received: 16 August 2019; Accepted: 10 December 2019;
Published: 10 January 2020
Edited by:
Esteban Avigliano, National Council for Scientific and Technical Research (CONICET), ArgentinaReviewed by:
Roberta Callicó Fortunato, University of Buenos Aires, ArgentinaCristiano Albuquerque, Federal Rural University of the Semi-Arid Region, Brazil
Copyright © 2020 Chung, Huang, You, Chiao and Wang. This is an open-access article distributed under the terms of the Creative Commons Attribution License (CC BY). The use, distribution or reproduction in other forums is permitted, provided the original author(s) and the copyright owner(s) are credited and that the original publication in this journal is cited, in accordance with accepted academic practice. No use, distribution or reproduction is permitted which does not comply with these terms.
*Correspondence: Chia-Hui Wang, Y2h3YW5nOTlAbWFpbC5udG91LmVkdS50dw==