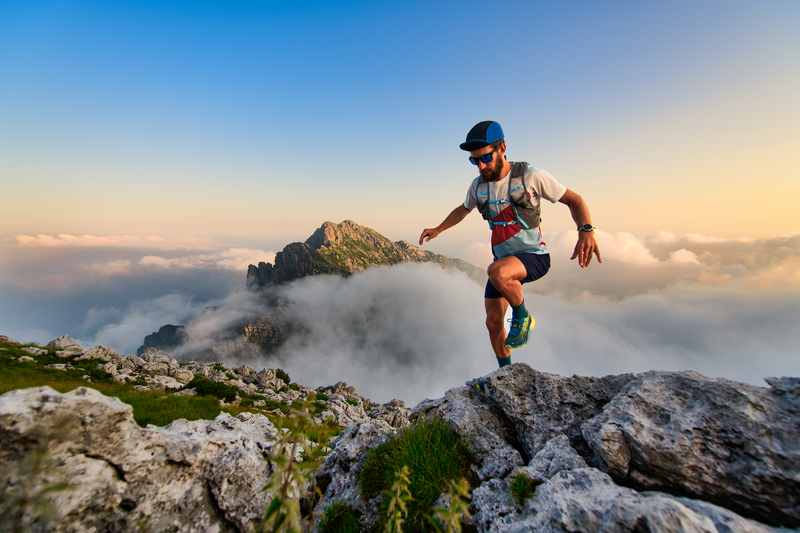
95% of researchers rate our articles as excellent or good
Learn more about the work of our research integrity team to safeguard the quality of each article we publish.
Find out more
ORIGINAL RESEARCH article
Front. Mar. Sci. , 12 November 2019
Sec. Marine Ecosystem Ecology
Volume 6 - 2019 | https://doi.org/10.3389/fmars.2019.00675
This article is part of the Research Topic Seafloor Heterogeneity: Artificial Structures and Marine Ecosystem Dynamics View all 12 articles
Decommissioning of oil and gas infrastructure globally has focused attention on its importance as hard substratum on continental shelf and slope habitats. Observational studies are needed to improve understanding of faunal assemblages supported by offshore infrastructure and better predict the effect of removal. Here, we present results from visual inspection and physical sampling of a small oil and gas industry structure decommissioned from an oil field in the North East Atlantic. This is supported by observations of similar structures nearby and by photographs of the surrounding seabed from environmental baseline surveys. The structure supported a reasonably high biomass and diversity of invertebrates (>10 kg and >39 macrofaunal and 17 megafaunal species) and fishes (>20 kg biomass and >4 species). The invertebrate megafaunal species present on the structure were a sub-set of the hard substratum fauna observed on surrounding seabed. Porifera were absent from the structure. Biological succession in the first 2 years occurred as follows. Sparse colonies of the hydroid Obelia sp. stet were early colonisers then subsequent development of thick hydroid turf (Obelia sp. stet. and Halecium sp. stet.) supported an invertebrate assemblage (2654 individuals kg wet mass–1) dominated by saddle oysters [Pododesmus squama (Gmelin, 1791) and Heteranomia sp. stet.)] and scale worms (Harmothoe spp.). Percentage cover of hydroid turf varied significantly over the structure, with most growth on sections exposed to strongest currents. Commercially important fish species present around the structure included Gadus morhua (Atlantic cod), Pollachius virens (saithe) and Lophius piscatorius (monkfish). Studies of artificial structures such as this provide much needed data to understand their role in the ecology of seafloor habitats and inform environmental decision making on all stages of industry from exploration to decommissioning. We show that the ecological role of the decommissioned three-dimensional structures was to enhance the biomass of a sub-set of epifaunal invertebrates found in the area. This supported diverse associated macrofaunal organisms, providing a food source for motile invertebrates and fishes in an area where background hard substratum can be lost through the impacts of drilling.
Artificial structures in the marine environment alter ecological structure and functioning. They provide habitat for threatened species (Bell and Smith, 1999), contribute reef habitat (Fowler et al., 2018), enhance recruitment of overfished species (Love et al., 2006), increase connectivity (Henry et al., 2018), often produce considerable fish biomass (Claisse et al., 2014) and provide foraging areas for large predators (Todd et al., 2016). These factors may vary over time, relating to environmental conditions and stage of ecological succession (Fujii, 2015). Consequently, artificial structures have a potential role in restoring degraded marine ecosystems such as coral reefs (Rinkevich, 2014), mollusc reefs (Walles et al., 2016), algal forests (Gianni et al., 2013), and have been proposed for restoration of disturbed deep-sea habitats (Cuvelier et al., 2018).
Oil and gas industry infrastructure is an important source of artificial hard substratum on continental shelf and slope habitats globally. Detailed descriptive studies of marine growth (biofouling) on oil and gas structures in the United Kingdom sector of the North Sea were carried out through early inspection and monitoring by oil and gas operators (e.g., Forteath et al., 1982). Oil and gas industry structures are rapidly colonised (Bell and Smith, 1999) and typically develop a highly productive ecosystem, e.g., ∼2700 tons of marine life have been estimated to live on the Shell Brent Alpha platform in the North Sea (Shell UK Ltd., 2017), including conservation priority species such as the reef-forming cold-water coral Desmophyllum pertusum (Linnaeus, 1758) (formerly Lophelia pertusa) (Bell and Smith, 1999). However, research on successional dynamics of organisms living on offshore infrastructure and the impacts to the surrounding benthos is surprisingly rare and generally limited to inaccessible consultancy reports (Gormley et al., 2018).
The imminent decommissioning of oil and gas infrastructure in all major basins has increased attention on their importance as hard substratum in the marine environment. This focus has led to an increase in observational studies on the role of oil and gas infrastructure in local ecosystems (Fowler et al., 2018) demonstrating that they support species of conservation importance (Rouse et al., 2019) including cold-water corals (Gass and Roberts, 2006). Oil and gas infrastructure likely increases, or at least focuses, fish production (Claisse et al., 2014), surrounding benthic biomass, diversity, and connectivity (Macreadie et al., 2011) so their removal may reduce secondary production (Pondella et al., 2015). The particular assemblages supported by these structures varies with structure age, water depth and height on the structure (McLean et al., 2018) and on different timescales (Fujii, 2015; Bond et al., 2018) so there is potentially variation within and between different basins. It is therefore important to develop a better understanding of faunal assemblages supported by offshore infrastructures in order to understand the effect of their removal. This is particularly important as environmental monitoring requirements for decommissioned oil fields are still to be established or are decided on a case-by-case basis (Jones et al., 2019).
In addition to the role of structures created by the oil and gas industry, the industry activities themselves change the surrounding environment. The oil drilling process discharges drill cuttings and drilling mud, which are released into the water and settle to the seafloor, smothering the natural sediments and associated meiofaunal, macrofaunal and megafaunal assemblages (Cordes et al., 2016). The accumulation of this material can lead to direct reductions of faunal standing stocks and biodiversity as well as secondary impacts, such as reducing habitat heterogeneity, further reducing diversity (Jones et al., 2007; Gates and Jones, 2012). Although both physical and biological recovery processes are evident, even in deep-sea ecosystems, there is evidence of persistence of disturbance for at least 10 years (Jones et al., 2012). Faunal assemblages associated with artificial structures may help mitigate or restore biomass lost to other drilling impacts but the net effects of positive and negative impacts to the environment from these anthropogenic activities are not clear.
Data from Remotely Operated Vehicle (ROV) video surveys are increasingly being made available by industry (Macreadie et al., 2018) to assess the faunal assemblages associated with subsea structures to understand the effects of decommissioning (e.g., van der Stap et al., 2016) but it is rarely possible to sample such structures. Here, we present results from visual inspection and physical sampling of an oil and gas industry subsea structure that was decommissioned from the Lancaster oil field, west of Shetland in the North East Atlantic in 2016. We aim to identify faunal assemblages associated with the structure and quantify biomass supported by it.
The observations reported here were made at wells drilled as part of a fractured basement exploration (Trice, 2014) of the Lancaster field, west of Shetland in the North East Atlantic (Figure 1). The Lancaster field is now undergoing development (Belaidi et al., 2018). The protective structure was in an area where the majority of the seabed consists of coarse sandy sediment with areas of hard substratum (boulders) to the north and west (Figure 2). The Lancaster field is within the area of an important commercial monkfish (Lophius piscatorius Linnaeus, 1758 and to a lesser extent, L. budegassa Spinola, 1807) fishery (Laurenson et al., 2008). In deeper water to the north west of Lancaster lies the Faroe-Shetland Sponge Belt Nature Conservation MPA and the west Shetland Shelf MPA lies to the south west (Figure 1). During field visits to the site (Table 1) the seabed water temperature ranged from 9 to 11°C. Seabed current direction is tidally reversing (approximately 80–100° to 270–300°, Figure 3C).
Figure 1. Location of the protective structure (Lancaster 205/21a-6) investigated here. Other observations at the location are also shown including extent of drill cuttings around well after drilling Lancaster 205-21a-7z and the location of ROV video observations. Inset: Hurricane Energy well locations west of Shetland and Marine Protected areas including the Faroe-Shetland Sponge belt Nature Conservation Marine Protected Area (NCMPA) http://jncc.defra.gov.uk/page-6479.
Figure 2. Seabed environment and anthropogenic impacts surrounding Protective Structure A at Lancaster-205/21a-6; (a) undisturbed seabed with boulders, (b) boulders impacted by drill cuttings, (c) undisturbed coarse sand, (d) sand covered by drill cuttings, (e) base of the protective structure in contact with the seabed showing no scouring, (f) scoured sediment on the north west corner of the protective structure, (g–i) examples of background hard substratum from the 2011 environmental baseline survey, note Porifera in all images.
Figure 3. (A) Representation of one side of Protective Structure A (not to scale). Panel numbers are labelled. Panels quantified in this study are grey in colour, those included in statistical analysis are darker grey. (B) Representation of the protective structure as it was orientated on the seafloor. Percentage cover by hydroid turf is indicated for each panel included in statistical analysis (diagram not to scale). (C) short-term seafloor current meter deployment with each line representing current speed and direction within a 5-min interval.
The Transocean Sedco-712 semi-submersible drilling rig began drilling the Lancaster-205/21a-6 well in May 2014. On 1st July 2014 the well was suspended and a subsea protective structure (Structure A) was placed on top of the wellhead to prevent damage from trawlers. The protective structure was four sided, each side comprising three large panels of steel grating in two rows below on singe large panel, all panels were approximately 1 m2) (Figures 3a,b). It was approximately a pyramid in shape (Figure 4a). Its seabed footprint was 3.6 × 3.6 m (12.96 m2). In July 2016 the Lancaster-205/21a-7 well was drilled, 25 m from the protective structure, by the Transocean Spitsbergen semi-submersible drilling rig. At this time, inspection of the protective structure revealed significant scouring around its base so the decision was taken to remove it. It had been in place for 860 days before it was decommissioned and recovered to the surface using a riser pipe on 2nd November 2016. Two other almost identical protective structures were observed at nearby well sites. The focus of this work is Structure A and we use observations from structures B and C to provide additional context. The sequence of activities reported in this study are shown in Table 2 and details of the structures are shown in Table 3.
Figure 4. In situ observations of protective structures. Scale bar on images b-l = 35 mm. (a) Structure A and fishes viewed from distance, (b) hydroid growth photographed on structure B, (c) hydroid turf covering a panel on the protective structure, (d) Actinauge richardi (Hormathiidae) and an unidentified flatfish, (e) A. richardi and two unidentified decapods, (f) A. richardi, hydroid turf and Parazoanthus sp., (g) Macropodia tenuispinis and Pollachius virens, (h) hydroid turf and the crab Bathynectes maravigna, (i) Stichastrella rosea, (j) Porania pulvillus, S. rosea and Parazoanthus sp., (k) Atlantic Cod, Gadus morhua and echinoid, (l) European Conger (Conger conger) entering structure through scoured sediment.
Access to the Transocean Spitsbergen was gained through Hurricane Energy’s participation in the SERPENT Project (Gates et al., 2017). In situ observations were made using an Oceaneering Magnum work class Remotely Operated Vehicle (ROV) equipped with standard definition video and a digital stills camera (Kongsberg OE14-208) and strobe (Kongsberg OE11-242). On 2nd October 2016 in situ still images of each of the large individual panels of the protective structure were taken to quantify the sessile invertebrate communities and fish assemblages. Additional close-up images of individual organisms on and around the structure were taken during that visit to the oil rig (26th September to 10th October 2016). To coincide with the recovery of Structure A (2nd November 2016) a further visit was made to the Transocean Spitsbergen (28th October to 3rd November 2016). Additional ROV observations were made of the structure in situ, although time constraints prevented a complete survey. Physical samples were collected when the protective structure arrived on deck. Samples of representative specimens of epifauna were taken for identification and biomass estimation. Five quantitative samples of the epifauna were also taken using either 250 × 250 mm quadrats or areas of the structure of measured dimensions. The time available for sampling was limited by a requirement to remove the structure from the drilling rig. During an earlier visit to the rig, a short seafloor current meter deployment was carried out using an Aanderaa SeaGuard single-point Recording Current Meter from 1st October 2016 10:40:00 to 3rd October 2016 09:30:00.
Structure B was observed in 2010 at the Lancaster-205/21-4C well after it had been on the seabed for 226 days. The Lancaster-205/21-4C well is 1.5 km from the well at Lancaster-205/21a-6. Water depth here was 155 m. It was only observed on a single occasion during the SERPENT project visit to the Borgsten Dolphin drilling rig and although a quantitative survey was not carried out, these observations do support the primary observation reported in this study.
Structure C was deployed at Whirlwind-205/21-A5, 9 km north of Lancaster-205/21a-6 on 16th October 2010 and provides further supporting information. On return to the Whirlwind site in 2011 Structure C was removed from the wellhead and moved 20 m away and placed at the seabed. ROV images were collected of that structure on visits to the Wilphoenix in 2011. These images were opportunistic and neither quantitative survey nor specimen collection were carried out.
Quantitative image analysis was carried out on Structure A only. Seven individual panels of metal grating on each side of the structure were considered samples (Figure 3A). The area of coverage by hydroid turf was quantified using the software ImageJ (Schneider et al., 2012), using known dimensions of the panels. All animals visible in the photographs and in contact with the metal grating were counted. Animals on the painted yellow parts of the structure were not included in the quantitative analysis but are considered in the species list and their counts were used to inform total biomass estimate of epifauna on the structure. Separate colonies of colonial organisms such as Parazoanthus sp. stet. and Filograna implexa Berkeley, 1835 were recorded as individuals. For identification purposes, representative specimens of most taxa encountered in ROV photography were available. In most cases it was not possible to determine whether an individual in a photograph was the same animal as a specimen in the laboratory. Specimen identification from the ROV survey was also aided by using close up in situ images. Organisms were classified to lowest taxonomic level and named according to WoRMS (Horton et al., 2019). Open nomenclature identifiers were used to indicate identifier confidence following the protocol set out for physical specimens (Sigovini et al., 2016). The same approach was used for animals observed in photographs.
To investigate the role of height on the structure and orientation of the side of the structure two-way analysis of variance on logit transformed percentage cover of the panels by hydroid turf was done using the R programing environment (R Development Core Team, 2010). To ensure a balanced design the top panel was excluded from this test (6 panels per side of structure, 3 upper and 3 lower). Two-way analysis of variance was carried out on epibenthic megafaunal invertebrate density on the same panels.
The maximum number of fish (maxN) of each species in a single image was used to estimate total abundance of fishes associated with the structure. Accurate measurements of the total length (TL) of fish were possible because of the well-defined grid pattern of the metal lattice of the protective structure. TL of all individual fish that could be seen in images were taken using ImageJ. The metal lattice of the panels of the structure provided a grid of known scale against which measurements of the fishes were made (the small rectangular sections were 37 × 92 mm, measured at sea by the authors). To reduce measurement error, each fish was measured three times and a mean was taken. Where the same fish was observed in multiple sequential images (<10 s apart) measurements were taken from each image and a mean value presented. Only fishes in close proximity to the panels, whose TL was visible, were measured.
Hydroid biomass was calculated based on the relationship between known area of panel and wet mass of hydroid from material collected on Structure A. This was calculated for one full panel from which all material was removed by scraping the hydroid turf into containers and four 0.25 × 0.25 m quadrats of material removed from the structure (Table 4).
Table 4. Dimension to fresh wet weight relationships from specimens used to calculate biomass on structure.
Wet mass to body dimension conversions were calculated for the asteroids Porania (Porania) pulvillus (Müller, 1776) and Stichastrella rosea (Müller, 1776) (radial length (R), radius from center of disk to the end of arm) and the hormathiid anemone Actinauge richardi (Marion, 1882) (column diameter), to estimate biomass of these more common organisms on the structure (Table 4), following the method of Durden et al. (2016). For less common species, insufficient specimens were collected to calculate body dimension to wet weight ratios. Biomass was therefore estimated as the weight of individual representative specimens, or the mean weight if more than one individual was collected.
Biomass of fish was estimated from Length-Weight ratios in Fishbase (Froese and Pauly, 2019), which were based on measurements in Froese et al. (2014). In the case of Pollachius virens (Linnaeus, 1758), abundance is expected to be considerably higher than estimated by maxN. All individuals for which TL was visible in an image were measured and the mean value multiplied by maxN to estimate total biomass of that species.
Preserved hydroid turf samples (4% borax buffered formaldehyde) were sieved to 250 μm, examined initially in white trays and then by inspection under a stereomicroscope. All specimens were removed, identified and counted. Following quality control of the sample processing it was clear that small bivalves were frequently missed from the sample picking. Therefore, a further 64.0 g (12% of the total) of hydroid turf was re-examined and the small bivalves and some additional polychaetes were enumerated and their total abundance in the overall sample estimated and included in the final count. Owing to insufficient data for meaningful statistical analysis, the five hydroid turf samples were combined to provide one quantitative species list for fauna inhabiting known wet biomass of hydroid turf. Specimens were identified using literature for the north-east Atlantic and all material has been curated and housed in the Discovery Collections at the National Oceanography Centre for future scientific use.
In 2011, an environmental survey contractor surveyed the benthic environment at the Lancaster field. Images of the seafloor were acquired using a drop-down camera to assess the seafloor type. These images were not used for megabenthic image analysis at the time of the survey but have since been analyzed by the authors of this study. A sub-set of 189 images that contain hard substratum were used to assess the fauna associated with naturally occurring hard substratum at Lancaster for comparison with the megafaunal assemblage associated with the protective structure.
Vertical accumulation of drill cuttings at the well was assessed using graduated sediment marker buoys deployed before drilling operations began. These were placed at 5 and 15 m distance from the proposed well location. Spatial coverage of drilling disturbance was assessed by visual observation of the seafloor in quantitative video surveys (outlined below). Physical disturbance of the sediment by smothering with drill cuttings was classified as “complete,” “partial” coverage and “undisturbed sediment” following the methods of Jones et al. (2006). Visual observations were validated using sediment samples and sediment barium concentration used to indicate sediment disturbed by drill cuttings.
On seven occasions, at different phases of the drilling operations, quantitative seafloor ROV video transect surveys were carried out at the Lancaster field (Table 2). Each of the seven surveys comprised eight video transects of approximately 100 m in length, radiating from the well. Video of the seafloor was recorded with standard definition colour video camera on a range of different ROVs depending on the drilling rig. In the transects, every individual whole animal that passed out of shot via the bottom of the frame was counted and their position in relation to the well was recorded. These surveys and subsequent analyses were carried out following the methods of Jones et al. (2006). In this study we present the mean abundance of megafaunal organisms and fishes (individuals m–2) in each of the surveys, where each transect was treated as an individual sample in order to show the shared taxa between the protective structure and surrounding sediments and to highlight a change that occurred during the study period.
After drilling Lancaster-205/21a-6, the surrounding seabed was covered by a layer of drill cuttings (Figures 2b,d) extending to 65 m from the well, after which the sediment resembled the background environment of sandy sediment (Figure 2c) with areas of boulders (Figure 2a). Following drilling of Lancaster-205/21a-7 drill cuttings extended >90 m from the well as indicated in Figure 1. Eight metres north of the Lancaster-205/21a-6 well, drill cuttings had accumulated 55 cm vertically. There was approximately 25 cm of cuttings accumulation at 12 m east of the well. Further from the well at 18 m, accumulation was lower and not measurable on the marker buoys but still visible in photography and in sediment samples. The base of most of the protective structure was in contact with the seabed (Figure 2e). On the southwest of the structure, sediment/drill cuttings had scoured, leaving a gap between the bottom of the structure on Side 2, Panels 4–6, around the corner of the structure to Panel 4 of the northwest facing side (Side 3, Panel 6) (Figure 2h).
After 2 years 4 months (860 days), large areas of the individual panels of Structure A were covered by hydroid turf (Figures 4c,j). The turf comprised the hydroids Obelia sp. stet. and Halecium sp. stet. The growth of hydroid turf varied over the structure. On the upper panels the percentage cover ranged from 30.9 to 63.7% (mean = 44.2%). The lower panels ranged from 1.3 to 47.8% (mean = 15.9%). On all lower panels, other than those on Side 2 (southwest facing), percentage cover was lower than 25% (with 8 of the 9 lower than 9%). On Side 2, the percentage cover of the lower panels ranged from 34–47.8% (Figure 3B). There was a significant difference in percentage cover between the upper and lower panels (F = 21.1, df = 1,20, p < 0.001) but no significant difference (F = 0.1, df = 1,20, p = 0.75) between the percentage cover on each side (once the height was taken into account) and there was no interaction.
The estimated biomass of hydroid turf on the panels of the protective structure was 6.59 kg. This biomass supported smaller organisms at a density of 2654 individuals kg wet mass–1 of hydroid turf (Table 6). The associated faunal assemblage was dominated by small saddle oysters (Pododesmus squama (Gmelin, 1791) and Heteranomia sp. stet.) and polynoid polychaetes (Harmothoe fraserthomsoni McIntosh, 1897). The majority of Pododesmus squama and Heteranomia sp. were small and attached to the hydroids. Other molluscs included the nudibranch Doto fragilis (Forbes, 1838). The hydroid turf supported suspension feeders such as the terebellid Pista cristata (Müller, 1776) and the serpulids Hydroides norvegica Gunnerus, 1768 and Serpula vermicularis Linnaeus, 1767. Crustaceans included the amphipods Stenothoe marina (Spence Bate, 1857) and Stenopleustes latipes (Sars, 1858) as well as a copepod fish parasite (Caligus sp. stet.). Some of the larger associated fauna were also visible in the photographic surveys reported below.
The quantitative photographic survey revealed the protective structure supported 17 epifaunal invertebrate megafaunal species (Table 5). Of these, all except the gastropod (Calliostoma sp. indet.) and the comatulid crinoid were identified from specimens collected from the structure. There was no significant difference in their density between the upper and lower panels, or the orientation (side) of the structure. In addition to the quantitative analysis of the panels, qualitative observations showed that the yellow painted structural lengths supported polychaetes (Family Serpulidae), larger saddle oysters attached to the surface (Pododesmus squama and Heteranomia sp. stet.) and hormathiid anemones (Actinauge richardi, Figures 4d–f). The serpulid polychaete Filograna implexa Berkeley, 1835 grew to greatest size and abundance on the upright section of the inner part of the structure, but this was not quantified here. The asteroids Porania pulvillus and Stichastrella rosea (Figure 4i) were common on the structure. Bare patches of grating close to these asteroids suggest they were feeding on the hydroid turf (e.g., Figure 4j). Galatheid squat lobsters (Galathea dispersa Bate, 1859) and the inachid crab Macropodia tenuirostris (Leach, 1814) were also observed in images.
Table 5. Hydroid turf percentage cover and epibenthic megafaunal abundance on the visually surveyed sample panels of the protective structure.
In addition to the 6.59 kg of hydroid turf and associated invertebrates on the structure panels, there were a further 1.75 kg of epifaunal invertebrates. These were dominated by Actinauge richardi (0.95 kg) and Porania pulvillus (0.49 kg). Estimated biomass on the panels of the protective structure was therefore 8.34 kg. Addition of the estimated biomass from measurements of 133 large organisms observed on the structure outside of the sample panels (1.76 kg) results in a total estimate of 10.09 kg of epifaunal invertebrate biomass on the protective structure.
There were five species of commercially targeted fish in close association with the protective structure. In greatest abundance were Pollachius virens (saithe), which were observed both outside and within the structure (e.g., Figure 4g). They ranged from 0.50–0.57 m in length; estimated mean biomass was 1.32 kg ind–1 and maxN was eight (total biomass estimate of 10.83 kg). Two (maxN) Molva molva (Linnaeus, 1758) (ling) of 0.62 m and 0.51 m in length were associated with the structure (one inside one just outside). A single Conger conger (Linnaeus, 1758) (European conger) was observed swimming under the scoured sediment beneath the protective structure (estimated biomass = 0.84 kg). Four Gadus morhua Linnaeus, 1758 (Atlantic cod, Figure 4k) were observed swimming around the outside of the structure (estimated biomass = 10.07 kg). A single Lophius piscatorius (monkfish) was lying underneath the structure. The biomass of this individual was not estimated because only the tail could be seen. The estimated total fish biomass in close association with the protective structure was 24.73 kg.
The background hard substratum was assessed using seafloor images classified as “cobbles dominated” or “cobbles and sand.” 34 species were associated with this substratum, dominated by Porifera (50% of observations, Figure 5) including Hymedesmia sp. stet. and an unidentified yellow encrusting sponge (Figures 2g–i). Cnidaria, dominated by an indeterminate solitary coral (Caryophylliidae) comprised 20% of observations and Bryozoa 18%. Of the 17 species (hydroids excluded) observed in the detailed image survey of Structure A, all except Bathynectes maravigna (Prestandrea, 1839) (Figure 4h) were also found in the background hard substratum. Two different crab species were encountered in the baseline survey but characters were not visible to determine if they were B. maravigna. Porifera, Bryozoa and Caryophillidae were not encountered on any of the protective structures. Hydroid turf was not observed in any seafloor images of hard substratum in the 2011 environmental baseline images, despite being distinctive and easily detected in images.
Figure 5. Percentage composition of invertebrate Phyla observed on hard natural substratum at Lancaster and on the Protective Structure A.
The ROV video transect survey shortly before recovery of Protective Structure A (27th September 2016) revealed increased abundance of small Triglidae sp. stet. (gurnard) and Helicolenus sp. stet. (redfish) fishes (Figure 6) as well as large numbers of small indeterminate pelagic fishes (not enumerated). In all the video surveys at the Lancaster field prior to the installation of the structure, fish abundance was lower. In a subsequent ROV survey, 18 months after removal of the structure, fish abundance had reduced to earlier levels (Figure 6). In addition to the increased abundance of small fishes, there were also increased numbers of predators. Eighty-four Lophius piscatorius were counted in the 27th September 2016 ROV survey (density = 0.07 individuals m–2). In contrast only seven L. piscatorius were observed in the six other video surveys at Lancaster (0.001 individuals m–2). Video sequences showed both L. piscatorius and G. morhua feeding on the small pelagic fish (Supplementary Material 1). The increased fish abundance was not recorded in the video surveys carried out in July 2016, despite the presence of the structure.
Figure 6. Time series observations of mean abundance of megafaunal invertebrates and fishes in video transects around wells in the Lancaster field. Green arrows indicate deployment and recovery of Structure A. Red arrows indicate drilling events.
On Structure B, small colonies of the hydroid Obelia sp. stet. had appeared after 9 months (Table 3 and Figure 4b). On Structure C, in the period between the end of operations in 2010 and their restart in 2011 the protective structure had been colonised by a wide variety of organisms. Hydroid turf coverage was patchy on the painted surfaces and thick growth covered the metal. Images suggest that species present were similar to Structure A, but C. conger was more abundant (maxN = 10).
In the 2 years since deployment the structure at Lancaster supported a reasonably high biomass and diversity of invertebrates (>10 kg and >39 macrofaunal and 17 megafaunal species) on a small area of seabed. This in turn supported >4 species of fish (>20 kg biomass). Data obtained from longer-established offshore structures off California, suggested they were some of the most productive habitats in the oceans (Claisse et al., 2014). This productivity is associated with the three dimensional structure of the habitat. It emphasizes the possibly important role that structures, even artificial structures, can play in marine communities.
Biological succession on the structures observed appears similar to that recorded in the Northern North Sea. With sparse colonies of Obelia sp. stet. (as seen on Structure B after >200 days, Table 3) being early colonisers (Forteath et al., 1982). The subsequent development of, in places, thick hydroid turf, is also typical (Boero, 1984). There were differences in the community development associated with location on Structure A. Coverage of the upper panels was greater than the lower panels and may reflect increased current velocity 2 m above the seabed. The SW facing lower panels (Side 2, 34–48% coverage) and the first panel on Side 3 (25% coverage) were unusual, in having high coverage near the seabed. In these cases, there was notable scouring of the sediment directly underneath the panels indicating that these panels were exposed to greater current velocity. The short-term seafloor current data support greater current velocity on the SW side (Figure 3C) but the currents were tidally reversing so do not explain why there was not greater hydroid coverage on the NE facing side of the structure. Increased current may support the colonisation of the structure through the greater exposure to suspended food particles or through the greater encounters with larvae passing the hard substratum upon which to settle (Wildish and Kristmanson, 1997).
The thick hydroid turf that developed on Structures A and C (Table 3) was unlike other habitat observed at Lancaster or Whirlwind (Figure 5) and it represents an important stage in the ecological succession of the artificial structure. It provides three-dimensional microstructure creating habitat that supports increased biological diversity (Di Camillo et al., 2017). Saddle oysters, amphipods and polynoids (Harmothoe fraserthomsoni) dominated the assemblage associated with the hydroid turf, similar to wind turbine structures and oil rigs in shallower water (Coolen et al., 2018). The genus Harmothoe has previously been reported associated with hydroid turf on artificial structures in the North Sea (Spierings et al., 2017). Juvenile and gravid female amphipods (Stenothoe marina) confirm the hydroid turf supporting production by other species. Specialist hydroid-consuming species such as the nudibranch Doto fragilis, reported to feed on Halecium sp. (Miller, 1961), were also present in the samples.
With the exception of the hydroid turf, the epibenthic megafaunal assemblage associated with Structure A was a subset of the fauna observed in broader surveys in the area. The absence of the most abundant groups on the background hard substratum (Porifera, Bryozoa and Caryophylliidae solitary corals) from Structure A was notable (Figure 5). Sponges contributed 50% of individuals on background hard substratum but were not present on the structure. Their distribution is influenced by various factors (Ramiro-Sánchez et al., 2019) such as current speed (Rice et al., 1990) and larval settlement on appropriate sediment type (Knudby et al., 2013) but it is not clear why they are not present on Structure A.
Actinauge richardi was the most abundant of the larger epifaunal species on Structure A and is common on offshore infrastructure in the North Sea (Rouse et al., 2019) and on a variety of substratum west of Shetland (Jones and Gates, 2010). Filograna implexa was present on the panels of Structure A, but colonies were largest and most abundant on the inner column of the structure, away from potential disturbance. Filograna implexa is part of the climax community of artificial structures (Forteath et al., 1982).
The complex habitat of the hydroid turf supported larger megafaunal invertebrate species. Bare patches of metal around the asteroids Stichastrella rosea and Porania pulvillus suggest they were feeding on the hydroid turf, as previously inferred for P. pulvillus from images taken elsewhere in the Lancaster field (Mah and Foltz, 2014). The faunal assemblage supported by the hydroid turf potentially provide a food source for the fishes observed at the site. This is supported by the fact that the organisms recorded in the hydroid turf in this study are equivalent to the polychaete worms, peracarid crustaceans and anomuran and brachyuran crabs that characterized the stomach contents of fish examined in a study of the feeding ecology around the Miller platform in the northern North Sea (Fujii, 2016).
It is most likely that the fish biomass recorded here was not produced on the structure but rather the commercially important fishes were attracted to it. Gadus morhua of 0.6 m in length are likely around 3–4 years old (Palakovich Carr and Kaufman, 2009) and Pollachius virens of 1 kg are likely 5–6 years old (Mathers et al., 1992). Pollachius virens is reported to aggregate around offshore oil infrastructure (Mathers et al., 1992; Fujii and Jamieson, 2016) and other structures such as fish farms (Otterå and Skilbrei, 2016) where they typically carry out diurnal vertical migrations. Conger conger are well known inhabitants of wrecks and other artificial structures (Steimle and Zetlin, 2000). Nonetheless, the protective structures observed here are clearly providing a service of some importance to the fishes. Many fish species have a tendency to aggregate (Fréon and Dagorn, 2000) as demonstrated by the role of fish aggregating devices in fisheries. In the North Sea, colonisation of a new structure by fish is rapid, with the first individuals arriving within 4 days (Todd et al., 2019). The increased fish abundance around the protective structure at Lancaster may have caused the increase in abundance of “sit and wait” predator L. piscatorius on the sediments up to 100 m from the structure. It is not clear how far the increased abundance of monkfish reached as the surveys were limited by the tether length of the ROVs launched from the drilling rigs. However, this may represent an example of the “ecological halo effect” in which artificial reefs support increased abundance and diversity over a considerably larger area than the reef itself (Reeds et al., 2018). There may also be a refuge effect because the areas around the drilling activity are protected from fishing by vessel exclusion zones.
Structures in the marine environment have long been considered for a role in ecological restoration (Grabowski and Peterson, 2007). Our results certainly suggest that they have a potentially useful role in aggregating or enhancing biomass of both fishes and invertebrates. In this area of the Faroe-Shetland Channel, natural hard substratum is common (Masson, 2001) and many of the same species naturally occurring in the area are attracted to the structures. Hydrocarbon drilling results in smothering of the seabed by drill cuttings and drilling mud, smothering hard substratum as well as causing a reduction in abundance and diversity of a range of size classes of benthic fauna (Netto et al., 2010; Gates and Jones, 2012). The loss of benthic fauna and associated ecosystem functioning from these changes in seabed type may be partially mitigated by the introduction of artificial hard substratum. However, our results suggest, on a short time-scale at least, that several of the most abundant background hard substratum species were absent from the artificial structure.
The structures evaluated here are relatively small, but commonly used in the region. In addition, much larger oil industry structures, including platforms and pipelines are present in large numbers in the North Sea area (Fowler et al., 2018). The effect of the size and type of the structure in controlling colonisation dynamics is unknown. However, the results from fine-scale detailed assessments may be valuable for providing details on the types of assemblage likely to develop on artificial structures and to improve understanding of the role of invasive species.
Environmental monitoring of offshore industries, including the oil and gas industry, generates large quantities of different types of data every year (Bean et al., 2017). Access to these data for scientific study can provide insights into the longer-term effects of industry activity (Henry et al., 2017) but are often inaccessible because they are presented as commercial consultancy reports. Opportunistic studies of seafloor infrastructure like this, and similar observations associated with industry structures such as well heads (Pradella et al., 2014) and pipelines (McLean et al., 2017) have revealed insights into how fishes and invertebrates utilize these structures, including the presence of species and features of conservation importance (Rouse et al., 2019). They provide much needed data to understand the role of these structures in the ecology of poorly studied habitats and inform environmental decision making on all stages of industry from exploration to decommissioning. Beyond understanding the impacts of industry activities in deep water, there is a global need for increased ocean observation to obtain the data needed to address challenges of societal concern (Ruhl et al., 2011). Access to industry datasets may be a way to increase the data available to the ocean observation community in order to drive a better understanding of the changing ocean in areas impacted by anthropogenic activity in addition to well-studied observatory sites (Levin et al., 2019).
All datasets generated for this study are included in the article/Supplementary Material.
Ethical review and approval was not required for the animal study because the research used opportunistic video and photographic observations of fish in their habitat.
AG conceived the idea, secured access to offshore infrastructure, and carried out the fieldwork. AS-S, TH, CC, AB, and AG analyzed the samples and images. DJ, AG, CC, AB, and KR analyzed the data and prepared the figures. AG, LG, CC, TH, AS-S, AB, KR, and DJ conceived the ideas, prepared tables and figures, and wrote the manuscript.
This work was supported in part by Hurricane Energy’s support to the SERPENT Project. AG was also supported by United Kingdom Natural Environment Research Council (NERC) grant NE/S009426/1 “Sustained autonomous environmental monitoring of offshore oil fields.” DJ received funding from United Kingdom Natural Environment Research Council (NERC) grant “Advanced monitoring of marine infrastructure for decommissioning” reference NE/P016561/1 and the European Union’s Horizon 2020 Research and Innovation Program under the MERCES (Marine Ecosystem Restoration in Changing European Seas) project, grant agreement no. 689518. This work was also supported by the NERC National Capability funding to the National Oceanography Centre, as part of the Climate Linked Atlantic Section Science (CLASS) program (Grant Number NE/R015953/1). KR was supported through a Canada Research Chair.
The authors declare that the research was conducted in the absence of any commercial or financial relationships that could be construed as a potential conflict of interest.
This work is an output of the SERPENT Project. We thank R. Trice, C. Slightam, and J. Valentine from Hurricane Energy for providing opportunities to visit their installations. We thank the Offshore Installation Manager and crew of the Transocean Spitsbergen for supporting this work with particular thanks to Stefan Jones, Alan Scott, Chris Stuart, Si Neely, and Jamie Oakley, the Oceaneering International ROV team for their time and expertise during the surveys.
The Supplementary Material for this article can be found online at: https://www.frontiersin.org/articles/10.3389/fmars.2019.00675/full#supplementary-material
Bean, T. P., Greenwood, N., Beckett, R., Biermann, L., Bignell, J. P., Brant, J. L., et al. (2017). A review of the tools used for marine monitoring in the uk: combining historic and contemporary methods with modeling and socioeconomics to fulfill legislative needs and scientific ambitions. Front. Mar. Sci. 4:263. doi: 10.3389/fmars.2017.00263
Belaidi, A., Bonter, D. A., Slightam, C., and Trice, R. C. (2018). The Lancaster Field: Progress in Opening the UK’s Fractured Basement Play. London: Geological Society, 385–398.
Bell, N., and Smith, J. (1999). Coral growing on North Sea oil rigs. Nature 402, 601–601. doi: 10.1038/45127
Boero, F. (1984). The ecology of marine hydroids and effects of environmental factors: a review. Mar. Ecol. 5, 93–118. doi: 10.1111/j.1439-0485.1984.tb00310.x
Bond, T., Langlois, T. J., Partridge, J. C., Birt, M. J., Malseed, B. E., Smith, L., et al. (2018). Diel shifts and habitat associations of fish assemblages on a subsea pipeline. Fish. Res. 206, 220–234. doi: 10.1016/j.fishres.2018.05.011
Claisse, J. T., Pondella, D. J., Love, M., Zahn, L. A., Williams, C. M., Williams, J. P., et al. (2014). Oil platforms off California are among the most productive marine fish habitats globally. Proc. Natl. Acad. Sci. U.S.A. 111, 15462–15467. doi: 10.1073/pnas.1411477111
Coolen, J. W. P., van der Weide, B., Cuperus, J., Blomberg, M., Van Moorsel, G. W. N. M., Faasse, M. A., et al. (2018). Benthic biodiversity on old platforms, young wind farms, and rocky reefs. Ices J. Mar. Sci. fsy092. doi: 10.1093/icesjms/fsy092
Cordes, E., Jones, D., Schlacher, T., Amon, D., Bernardino, A., Brooke, S., et al. (2016). Environmental impacts of the deep-water oil and gas industry: a review to guide management strategies. Front. Environ. Sci. 4:58. doi: 10.3389/fenvs.2016.00058
Cuvelier, D., Gollner, S., Jones, D. O. B., Kaiser, S., Arbizu, P. M., Menzel, L., et al. (2018). Potential mitigation and restoration actions in ecosystems impacted by seabed mining. Front. Mar. Sci. 5:467. doi: 10.3389/fmars.2018.00467
Di Camillo, C. G., Bavestrello, G., Cerrano, C., Gravili, C., Piraino, S., Puce, S., et al. (2017). “Hydroids (Cnidaria, Hydrozoa): a neglected component of animal forests,” in Marine Animal Forests: The Ecology of Benthic Biodiversity Hotspots, eds S. Rossi, L. Bramanti, A. Gori, and C. Orejas (Cham: Springer International Publishing), 397–427. doi: 10.1007/978-3-319-21012-4_11
Durden, J. M., Bett, B. J., Horton, T., Serpell-Stevens, A., Morris, K. J., Billett, D. S. M., et al. (2016). Improving the estimation of deep-sea megabenthos biomass: dimension to wet weight conversions for abyssal invertebrates. Mar. Ecol. Prog. Ser. 552, 71–79. doi: 10.3354/meps11769
Forteath, G. N. R., Picken, G. B., Ralph, R., and Williams, J. (1982). Marine growth-studies on the North Sea oil platform Montrose Alpha. Mar. Ecol. Prog. Ser. 8, 61–68. doi: 10.3354/meps008061
Fowler, A. M., Jørgensen, A.-M., Svendsen, J. C., Macreadie, P. I., Jones, D. O. B., Boon, A. R., et al. (2018). Environmental benefits of leaving offshore infrastructure in the ocean. Front. Ecol. Environ. 16:571–578. doi: 10.1002/fee.1827
Fréon, P., and Dagorn, L. (2000). Review of fish associative behaviour: toward a generalisation of the meeting point hypothesis. Rev. Fish Biol. Fish. 10, 183–207.
Froese, R., and Pauly, D. (eds) (2019). FishBase. World Wide Web Electronic Publication. Available at: www.fishbase.org (accessed February, 2019).
Froese, R., Thorson, J., and Reyes, R. B. Jr. (2014). A Bayesian approach for estimating length-weight relationships in fishes. J. Appl. Ichthyol. 30, 78–85. doi: 10.1111/jai.12299
Fujii, T. (2015). Temporal variation in environmental conditions and the structure of fish assemblages around an offshore oil platform in the North Sea. Mar. Environ. Res. 108, 69–82. doi: 10.1016/j.marenvres.2015.03.013
Fujii, T. (2016). Potential influence of offshore oil and gas platforms on the feeding ecology of fish assemblages in the North Sea. Mar. Ecol. Prog. Ser. 542, 167–186. doi: 10.3354/meps11534
Fujii, T., and Jamieson, A. J. (2016). Fine-scale monitoring of fish movements and multiple environmental parameters around a decommissioned offshore oil platform: a pilot study in the North Sea. Ocean Eng. 126, 481–487. doi: 10.1016/j.oceaneng.2016.09.003
Gass, S. E., and Roberts, J. M. (2006). The occurrence of the cold-water coral Lophelia pertusa (Scleractinia) on oil and gas platforms in the North Sea: colony growth, recruitment and environmental controls on distribution. Mar. Pollut. Bull. 52, 549–559. doi: 10.1016/j.marpolbul.2005.10.002
Gates, A. R., Benfield, M. C., Booth, D. J., Fowler, A. M., Skropeta, D., and Jones, D. O. B. (2017). Deep-sea observations at hydrocarbon drilling locations: contributions from the SERPENT Project after 120 field visits. Deep Sea Res. Part II 137, 463–479. doi: 10.1016/j.dsr2.2016.07.011
Gates, A. R., and Jones, D. O. B. (2012). Recovery of Benthic megafauna from anthropogenic disturbance at a hydrocarbon drilling well (380 m Depth in the Norwegian Sea). PLoS One 7:e44114. doi: 10.1371/journal.pone.0044114
Gianni, F., Bartolini, F., Airoldi, L., Ballesteros, E., Francour, P., Guidetti, P., et al. (2013). Conservation and restoration of marine forests in the mediterranean sea and the potential role of marine protected areas. Adv. Oceanogr. Limnol. 4, 83–101. doi: 10.1080/19475721.2013.845604
Gormley, K., McLellan, F., McCabe, C., Hinton, C., Ferris, J., Kline, D., et al. (2018). Automated image analysis of offshore infrastructure marine biofouling. J. Mar. Sci. Eng. 6:2. doi: 10.3390/jmse6010002
Grabowski, J. H., and Peterson, C. H. (2007). Restoring oyster reefs to recover ecosystem services. Ecosyst. Eng. Plants Protists 4, 281–298. doi: 10.1016/s1875-306x(07)80017-7
Henry, L.-A., Harries, D., Kingston, P., and Roberts, J. M. (2017). Historic scale and persistence of drill cuttings impacts on North Sea benthos. Mar. Environ. Res. 129, 219–228. doi: 10.1016/j.marenvres.2017.05.008
Henry, L. A., Mayorga-Adame, C. G., Fox, A. D., Polton, J. A., Ferris, J. S., McLellan, F., et al. (2018). Ocean sprawl facilitates dispersal and connectivity of protected species. Sci. Rep. 8:11346. doi: 10.1038/s41598-018-29575-4
Horton, T., Kroh, A., Ahyong, S., Bailly, N., Boyko, C. B., Brandão, S. N., et al. (2019). World Register of Marine Species (WoRMS). WoRMS Editorial Board. Available at: http://www.marinespecies.org at VLIZ. (accessed May 14, 2019).
Jones, D. O. B., and Gates, A. R. (2010). Deep-Sea Life of Scotland and Norway. United Kindom: Ophiura press.
Jones, D. O. B., Gates, A. R., Huvenne, V. A. I., Phillips, A. B., and Bett, B. J. (2019). Autonomous marine environmental monitoring: application in decommissioned oil fields. Sci. Total Environ. 668, 835–853. doi: 10.1016/j.scitotenv.2019.02.310
Jones, D. O. B., Gates, A. R., and Lausen, B. (2012). Recovery of deep-water megafaunal assemblages from hydrocarbon drilling disturbance in the Faroe-Shetland Channel. Mar. Ecol. Progress Series 461, 71–82. doi: 10.3354/meps09827
Jones, D. O. B., Hudson, I. R., and Bett, B. J. (2006). Effects of physical disturbance on the cold-water megafaunal communities of the Faroe-Shetland Channel. Mar. Ecol. Prog. Ser. 319, 43–54. doi: 10.3354/meps319043
Jones, D. O. B., Wigham, B. D., Hudson, I. R., and Bett, B. J. (2007). Anthropogenic disturbance of deep-sea megabenthic assemblages: a study with Remotely-Operated Vehicles in the Faroe-Shetland Channel. NE Atlantic. Mar. Biol. 151, 1731–1741. doi: 10.1007/s00227-007-0606-3
Knudby, A., Kenchington, E., and Murillo, F. J. (2013). Modeling the distribution of Geodia sponges and sponge grounds in the northwest Atlantic. PLoS One 8:e82306. doi: 10.1371/journal.pone.0082306
Laurenson, C. H., Dobby, H., and McLay, H. A. (2008). The Lophius budegassa component of monkfish catches in Scottish waters. ICES J. Mar. Sci. 65, 1346–1349. doi: 10.1093/icesjms/fsn100
Levin, L. A., Bett, B. J., Gates, A. R., Heimbach, P., Howe, B. M., Janssen, F., et al. (2019). Global observing needs in the deep ocean. Front. Mar. Sci. 6:241. doi: 10.3389/fmars.2019.00241
Love, M. S., Schroeder, D. M., Lenarz, W., MacCall, A., Bull, A. S., and Thorsteinson, L. (2006). Potential use of offshore marine structures in rebuilding an overfished rockfish species, bocaccio (Sebastes paucispinis). Fish. Bull. 104, 383–390.
Macreadie, P. I., Fowler, A. M., and Booth, D. J. (2011). Rigs-to-reefs: will the deep sea benefit from artificial habitat? Front. Ecol. Environ. 9:455–461. doi: 10.1890/100112
Macreadie, P. I., McLean, D. L., Thomson, P. G., Partridge, J. C., Jones, D. O. B., Gates, A. R., et al. (2018). Eyes in the sea: unlocking the mysteries of the ocean using industrial, remotely operated vehicles (ROVs). Sci. Total Environ. 634, 1077–1091. doi: 10.1016/j.scitotenv.2018.04.049
Mah, C. L., and Foltz, D. W. (2014). New taxa and taxonomic revisions to the Poraniidae (Valvatacea. Asteroidea) with comments on feeding biology. Zootaxa 3795, 327–364. doi: 10.11646/zootaxa.3795.3.7
Masson, D. G. (2001). Sedimentary processes shaping the eastern slope of the Faroe-Shetland Channel. Cont. Shelf Res. 21, 825–857. doi: 10.1016/s0278-4343(00)00115-1
Mathers, E. M., Houlihan, D. F., and Cunningham, M. J. (1992). Estimation of saithe Pollachius virens growth rates around the Beryl oil platforms in the North Sea: a comparison of methods. Mar. Ecol. Prog. Ser. 86, 31–40. doi: 10.3354/meps086031
McLean, D. L., Partridge, J. C., Bond, T., Birt, M. J., Bornt, K. R., and Langlois, T. J. (2017). Using industry ROV videos to assess fish associations with subsea pipelines. Cont. Shelf Res. 141, 76–97. doi: 10.1016/j.csr.2017.05.006
McLean, D. L., Taylor, M. D., Partridge, J. C., Gibbons, B., Langlois, T. J., Malseed, B. E., et al. (2018). Fish and habitats on wellhead infrastructure on the north west shelf of Western Australia. Cont. Shelf Res. 164, 10–27. doi: 10.1016/j.csr.2018.05.007
Miller, M. C. (1961). Distribution and food of the nudibranchiate mollusca of the south of the isle of man. J. Anim. Ecol. 30, 95–116.
Netto, S. A., Fonseca, G., and Gallucci, F. (2010). Effects of drill cuttings discharge on meiofauna communities of a shelf break site in the southwest Atlantic. Environ. Monit. Assess 167, 49–63. doi: 10.1007/s10661-010-1515-3
Otterå, H., and Skilbrei, O. T. (2016). Vertical distribution of saithe (Pollachius virens) aggregating around fish farms. Ices J. Mar. Sci. 73, 1186–1195. doi: 10.1093/icesjms/fsv261
Palakovich Carr, J., and Kaufman, L. (2009). Estimating the importance of maternal age, size, and spawning experience to recruitment of Atlantic cod (Gadus morhua). Biol. Conserv. 142, 477–487. doi: 10.1016/j.biocon.2008.10.004
Pondella, D. J. II, Zahn, L. A., Love, M. S., Siegel, D., and Bernstein, B. B. (2015). Modeling fish production for southern California’s petroleum platforms. Integr. Environ. Assess. Manag. 11, 584–593. doi: 10.1002/ieam.1689
Pradella, N., Fowler, A. M., Booth, D. J., and Macreadie, P. I. (2014). Fish assemblages associated with oil industry structures on the continental shelf of north-western Australia. J. Fish Biol. 84, 247–255. doi: 10.1111/jfb.12274
R Development Core Team (2010). R: A Language and Environment for Statistical Computing. Vienna: R Foundation for Statistical Computing.
Ramiro-Sánchez, B., González-Irusta, J. M., Henry, L.-A., Cleland, J., Yeo, I., Xavier, J. R., et al. (2019). Characterization and mapping of a deep-sea sponge ground on the tropic seamount (Northeast Tropical Atlantic): implications for spatial management in the high seas. Front. Mar. Sci. 6:278. doi: 10.3389/fmars.2019.00278
Reeds, K. A., Smith, J. A., Suthers, I. M., and Johnston, E. L. (2018). An ecological halo surrounding a large offshore artificial reef: sediments, infauna, and fish foraging. Mar. Environ. Res. 141, 30–38. doi: 10.1016/j.marenvres.2018.07.011
Rice, A., Thurston, M., and New, A. (1990). Dense aggregations of a hexactinellid sponge, Pheronema carpenteri, in the Porcupine Seabight (northeast Atlantic Ocean), and possible causes. Prog. Oceanogr. 24, 179–196. doi: 10.1016/0079-6611(90)90029-2
Rinkevich, B. (2014). Rebuilding coral reefs: does active reef restoration lead to sustainable reefs? Curr. Opin. Environ. Sustain. 7, 28–36. doi: 10.1016/j.cosust.2013.11.018
Rouse, S., Lacey, N. C., Hayes, P., and Wilding, T. A. (2019). Benthic conservation features and species associated with subsea pipelines: considerations for decommissioning. Front. Mar. Sci. 6:200. doi: 10.3389/fmars.2019.00200
Ruhl, H. A., André, M., Beranzoli, L., Namik Çagatay, M., Colaço, A., Cannat, M., et al. (2011). Societal need for improved understanding of climate change, anthropogenic impacts, and geo-hazard warning drive development of ocean observatories in European Seas. Prog. Oceanogr. 91, 1–33. doi: 10.1016/j.pocean.2011.05.001
Schneider, C. A., Rasband, W. S., and Eliceiri, K. W. (2012). NIH Image to ImageJ: 25 years of image analysis. Nat. Methods 9, 671–675. doi: 10.1038/nmeth.2089
Shell UK Ltd. (2017). Brent Field decommissioning programmes. Shell Report Number BDE-F-GEN-AA-5880-00015, Submitted to the UK Department for Business, Energy and Industrial Strategy, Shell UK Ltd., London.
Sigovini, M., Keppel, E., and Tagliapietra, D. (2016). Open Nomenclature in the biodiversity era. Methods Ecol. Evol. 7, 1217–1225. doi: 10.1111/2041-210x.12594
Spierings, M., Dias, I. M., Coolen, J. W. P., van der Weide, B., and Cuperus, J. (2017). First record of Harmothoe aspera (Hansen, 1879) (Polychaeta: Polynoidae) in the Dutch North Sea. Mar. Biodivers. Records 10:29.
Steimle, F. W., and Zetlin, C. (2000). Reef habitats in the middle atlantic bight: abundance, distribution, associated biological communities, and fishery resource use. Mar. Fish. Rev. 62, 24–42.
Todd, V. L. G., Warley, J. C., and Todd, I. B. (2016). Meals on wheels? A decade of megafaunal visual and acoustic observations from offshore oil & gas rigs and platforms in the north and irish seas. PLoS One 11:e0153320. doi: 10.1371/journal.pone.0153320
Todd, V. L. G., Williamson, L. D., Cox, S. E., Todd, I. B., and Macreadie, P. I. (2019). Characterizing the first wave of fish and invertebrate colonization on a new offshore petroleum platform. Ices J. Mar. Sci. fsz077. doi: 10.1093/icesjms/fsz077
Trice, R. (2014). Basement exploration, West of Shetlands: progress in opening a new play on the UKCS. Geol. Soc. 397, 81–105. doi: 10.1144/sp397.3
van der Stap, T., Coolen, J. W. P., and Lindeboom, H. J. (2016). Marine fouling assemblages on offshore gas platforms in the southern north sea: effects of depth and distance from shore on biodiversity. PLoS One 11:e0146324. doi: 10.1371/journal.pone.0146324
Walles, B., Troost, K., van den Ende, D., Nieuwhof, S., Smaal, A. C., and Ysebaert, T. (2016). From artificial structures to self-sustaining oyster reefs. J. Sea Res. 108, 1–9. doi: 10.1016/j.seares.2015.11.007
Keywords: ecosystem restoration, rigs to reef, Gadus morhua (Teleostei), artifical reef, oil and gas activity, decommissioning
Citation: Gates AR, Horton T, Serpell-Stevens A, Chandler C, Grange LJ, Robert K, Bevan A and Jones DOB (2019) Ecological Role of an Offshore Industry Artificial Structure. Front. Mar. Sci. 6:675. doi: 10.3389/fmars.2019.00675
Received: 17 May 2019; Accepted: 17 October 2019;
Published: 12 November 2019.
Edited by:
Toyonobu Fujii, Tohoku University, JapanReviewed by:
Ana Hilário, University of Aveiro, PortugalCopyright © 2019 Gates, Horton, Serpell-Stevens, Chandler, Grange, Robert, Bevan and Jones. This is an open-access article distributed under the terms of the Creative Commons Attribution License (CC BY). The use, distribution or reproduction in other forums is permitted, provided the original author(s) and the copyright owner(s) are credited and that the original publication in this journal is cited, in accordance with accepted academic practice. No use, distribution or reproduction is permitted which does not comply with these terms.
*Correspondence: Andrew R. Gates, YXJnM0Bub2MuYWMudWs=
Disclaimer: All claims expressed in this article are solely those of the authors and do not necessarily represent those of their affiliated organizations, or those of the publisher, the editors and the reviewers. Any product that may be evaluated in this article or claim that may be made by its manufacturer is not guaranteed or endorsed by the publisher.
Research integrity at Frontiers
Learn more about the work of our research integrity team to safeguard the quality of each article we publish.