- 1Génétique et Physiologie des Microalgues, InBioS/Phytosystems, Institut de Botanique, Université de Liège, Liège, Belgium
- 2Ecophysiologie et Physiologie Animale, InBioS/Phytosystems, Institut de Botanique, Université de Liège, Liège, Belgium
The breakdown of the symbiosis between cnidarians and Symbiodiniaceae often occurs upon periods of elevated sea surface temperature and gives rise to bleaching events that affect coral reefs worldwide. In this respect, an impairment of photosynthesis would be responsible for light-dependent generation of toxic reactive oxygen species putatively contributing to death of symbionts and/or host cells. In some Symbiodiniaceae species, alternative photosynthetic electron flows (AEF) have been documented to occur upon a shift to high temperature, possibly contributing to photoprotection and to the balance of energetic ratio between photoproduced ATP and NADPH. By using a combination of in vivo spectrofluorimetric and oximetric techniques, we studied the capacity for electron rerouting toward oxygen and cyclic electron flow (CEF) around photosystem I in eight Symbiodiniaceae in culture belonging to Symbiodinium and Breviolum genera upon an acute shift from 25 to 33°C. CEF capacity was determined as the kinetic of PSI primary donor P700 re-reduction in the presence of DCMU, a PSII inhibitor. An active oxygen uptake in light was estimated by comparing net oxygen evolution and relative electron transport rate of PSII at different light intensities. Among strains that showed elevated capacity for both AEF, some were thermotolerant while others were thermosensitive. Conversely, in some thermotolerant strains, capacities for these AEF were low upon acute heat stress. A principal component analysis of these results indicates that the long-term heat tolerant/bleached phenotype of cultured Symbiodinium and Breviolum spp. is not correlated with a capacity for different AEF across isolates during early onset of acute heat stress.
Introduction
The symbiosis between reef-building corals and the intracellular dinoflagellates of the family Symbiodiniaceae is of major ecological importance since it allows coral reefs to prosper in tropical oligotrophic waters. However, many corals live very close to their upper thermal threshold and are vulnerable to rising seawater temperature which triggers the breakdown of the mutual relationship between the animal and its photosymbionts (e.g., Suggett and Smith, 2011). Over the last century, global sea surface temperature has increased at an unprecedented rate, and has warmed by almost 1°C (Myhre et al., 2013). This has led to several mass bleaching events that strongly impacted coral reefs health status at regional and global scales (Hughes et al., 2018). About 20% of the world’s coral reefs have already been lost due to global warming over the past 30 years, and climate simulations predict that by the second half of this century, over 80% of the world’s remaining reefs will suffer from annual bleaching events (Frieler et al., 2013; Van Hooidonk et al., 2013).
Coral bleaching is taxonomically and spatially heterogeneous, depending on several factors, including the genetic identity of the host and the symbionts (Baird et al., 2009; Silverstein et al., 2015; Suggett et al., 2017). Until very recently, Symbiodiniaceae were classified into nine phylogenetically distant clades (A–I) which are now proposed to be distinct genera. For instance, representatives of clade A are grouped into the genus Symbiodinium sensus stricto while clade B representatives are part of the genus Breviolum (LaJeunesse et al., 2018). Among the Symbiodiniaceae family, the sensitivity to elevated temperatures has been reported to be highly variable, with for instance, some Symbiodinium or Durusdinium species (previously clade D) being more tolerant to acute heat stress (Abrego et al., 2008; Díaz-Almeyda et al., 2017; Silverstein et al., 2017). While bleaching observations often (but not always) correspond with presence/absence of thermotolerant Symbiodiniaceae taxa, the drivers of functional diversity across broadly phylogenetically diverse taxa is largely unresolved (e.g., Levin et al., 2016; Warner and Suggett, 2016; Goyen et al., 2017; Suggett et al., 2017).
Although the mechanistic details of coral bleaching are rather well studied, the reasons behind the differences in the tolerance to thermal stress in reef-building corals (entire holobiont) are still to be highlighted (e.g., Warner and Suggett, 2016). Thermal stress negatively impacts photosynthesis of endosymbionts by damaging different sites of the photosynthetic apparatus (Venn et al., 2008; Roth, 2014; Suggett et al., 2017), e.g., the D1 protein associated to photosystem II (PSII) and its repair mechanism (Warner et al., 1999; Lesser and Farrell, 2004; Takahashi et al., 2004, 2009; Smith et al., 2005), enzymes of the Calvin-Benson cycle as the ribulose-1,5-bisphosphate carboxylase/oxygenase (rubisco) (Lesser, 1996; Bhagooli and Hidaka, 2003; Leggat et al., 2004; Lilley et al., 2010), and the thylakoid membranes (Tchernov et al., 2004). Overall, these disturbances result in photoinhibition and in accumulation of excess energy, promoting the generation of reactive oxygen species (ROS) or nitric oxide (Bouchard and Yamasaki, 2008) which, if not scavenged by the antioxidant network (e.g., Levin et al., 2016), may trigger a signaling cascade ending with the disruption of the symbiosis (Weis, 2008; Lesser, 2011). Therefore, it is possible to consider that the plasticity of the photosynthetic electron transfer chain, along with the antioxidant network downstream in some particular Symbiodiniaceae species, might contribute to the thermal tolerance of the holobiont.
Plasticity of the photosynthetic apparatus may involve greater ROS tolerance [i.e., higher constitutive antioxidant defenses or higher ability to upregulate components of the antioxidant network (Krueger et al., 2014; Roberty et al., 2016), larger photoprotective capacities (Robison and Warner, 2006; Warner and Berry-Lowe, 2006), and/or some more efficient electron sinks (Warner and Suggett, 2016)]. Photosynthetic alternative electron flows (AEF) such as electron rerouting toward oxygen or cyclic electron flow (CEF) may essentially contribute to ensure photoprotection by decreasing excitation pressure on photosystems and by providing additional ATP to the cellular metabolism (reviewed in Munekage et al., 2008; Cardol et al., 2010; Johnson et al., 2014). In this respect, some Symbiodiniaceae species are known for their high ability to divert photosynthetic electron toward oxygen (Jones et al., 1998; Badger et al., 2000; Tchernov et al., 2004; Ulstrup et al., 2005; Suggett et al., 2008). Previous work showed that this oxygen reduction occurs downstream PSI (i.e., by a Mehler-like reaction), its extent rising with increasing light intensity, and varying among species (Roberty et al., 2014). Upon thermal stress, the capacity of this electron sink is also maintained in temperate Symbiodinium (former clade A) (Roberty et al., 2015), suggesting that this alternative photosynthetic electron flow may contribute to thermal tolerance. Later, it has been shown that the capacity of CEF around Photosystem I upon thermal stress is larger in two Symbiodinium species than in a Breviolum species (former clade B) (Aihara et al., 2016). However, the extent to which the CEF and/or the O2-dependent electron flow occur among Symbiodiniaceae species and how the presence of either mechanism contributes to the thermotolerance of individual species is not known.
Based on the works realized by Aihara et al. (2016) and Roberty et al. (2014), we subjected to an acute thermal stress eight Symbiodiniaceae species belonging to two genera, namely Symbiodinium and Breviolum [previously clades A and B, respectively (LaJeunesse et al., 2018)]. These strains originate from reef-building corals, soft octocorals, sea anemones, and giant clam, and from distant geographic locations (e.g., Hawaii, Panama, Red Sea, Japan). We investigated whether the capacity for different AEF across isolates during early onset of acute heat stress is correlated to long-term tolerant/bleaching phenotypes.
Materials and Methods
Symbiodinium and Breviolum Culturing
Eight cultured strains belonging to two Symbiodiniaceae genera (Symbiodinium and Breviolum) were studied. A detailed description of the strains, their host species, geographic origin and sub-clade is listed in Table 1. By following the protocol described in Hume et al. (2018), the classification of these strains at the cladal scale by sequencing of 5.8S rDNA internal transcribed space 2 regions (ITS2) was confirmed. All strains have been cultured under laboratories conditions for at least 4 years. Cultures were grown semi-continuously in 500 mL Erlenmeyer flasks containing 150 mL F/2 medium based on artificial seawater (Reef Crystals Reef Salt, Instant Ocean, United States) at a salinity of 34 PSU and at pH 8.0–8.2. They were exposed to a light intensity of 75 μmol photons m–2 s–1 (cool white fluorescent lamps, Philips MASTER TL-D Super 80) on a 12h:12 h light:dark cycle at a constant temperature of 25°C in Grobanks (CLF Plant Climatics) plant growth chambers. Exponential growth was maintained by sub-culturing into fresh medium every week at 150 000–200 000 cells mL–1.
Chlorophylls Content
Cells were harvested and resuspended in 100% methanol and lysed in a TissueLyser II (30 Hz, 5 min, 4°C; Qiagen) in presence of glass beads (710–1,180 μm; Sigma-Aldrich). After debris removal at 4°C, chlorophyll a and c2 concentrations were determined by spectrophotometry according to the equations of Ritchie (2006) for dinophytes.
Heat Treatment
To test the short-term (hours) temperature effects, cells in exponential growth phase were harvested and cell suspensions were adjusted to 10 μg chlorophylls mL–1 with fresh F/2 media. Three biological replicates of each Symbiodiniaceae strains were incubated into a water bath set to 33°C for 24 h. Cultures were sampled and physiological measurements were carried out 15 min, 1, 4, and 24 h after the beginning of the heat treatment. Measurements carried out just before the thermal stress served as controls. For the long-term heat treatment (days), cells were maintained in a growth chamber at 33°C, with a light intensity of 75 μmol photons m–2 s–1 under a light:dark cycle of 12:12 h.
Chlorophyll Fluorescence
Yield of chlorophyll fluorescence emission was measured using a JTS-10 spectrophotometer (Bio-logic, France). Actinic light was provided by LED light sources peaking at 630–640 nm during 2 min for each illumination intensity. Cells were incubated for 2 min in the dark before measurement. The maximum photochemical quantum yield of Photosystem II [Fv/Fm] was calculated as [Fm − Fo]/Fm, where Fo is the minimum fluorescence emission level, and Fm is the maximum fluorescence emission level induced by a 150 ms superimposed pulse of saturating light (3500 μmol photons m–2 s–1) after dark adaptation. The effective photochemical quantum yield of photosystem II (ϕPSII) was calculated as [Fm′ − F′]/Fm′ (Genty et al., 1989), where F′ is the stationary fluorescence emitted by excited actinic light and Fm′ is the maximum fluorescence emission being induced by a 150 ms superimposed pulse of saturating light (3500 μmol photons m–2 s–1) from pre-illuminated cultures.
Oxygen Exchange Measurements
Oxygen uptake in the dark (UOD) and net oxygen exchange rates (VO2) in the light were recorded with an optical oxygen meter – FireStingO2 (Pyro Science) and expressed in pmol O2 s–1 μg–1 chl. Actinic light was provided by LED light sources peaking at 630–640 nm from a JTS-10 spectrophotometer. VO2 is the sum of three terms: EO (gross oxygen evolution by PSII), UOD and UOL (Oxygen uptake in the light). By definition, EO is linearly correlated to ETRPSII, both parameters accounting for PSII activity. Given that UOL is almost null at low light intensities (up to 100 μmol photon m–2 s–1) in all strains investigated in a previous work, including Avir (Roberty et al., 2014), we assumed that it is also the case for all the strains investigated here. Therefore, EO in low light was calculated as VO2 − UOD. And EO value in high light intensity was extrapolated by multiplying rETRPSII in high light by the ratio EO/rETRPSII in low light. Accordingly, UOL in high light was calculated as VO2 − EO − UOD.
P700 Absorption Changes
P700 absorption changes were measured at 705 nm using a JTS-10 spectrophotometer (Bio-logic, France). Actinic light (5 s, 900 μmol photons m–2 s–1) was provided by LED peaking at 660 nm. Samples were supplemented with 10% (w/v) Ficoll to prevent cell sedimentation. Re-reduction rates of P700 in presence of 3-(3,4-dichlorophenyl)-1,1-dimethylurea (DCMU, 40 μM) and hydroxylamine (1 mM) was used for CEF determination. Inhibitors were added 10 min before measurements. Addition of DCMU 40 μM alone had no significant effect on UOD of Avir strain.
Electrochromic Shift
In vivo spectroscopic electrochromic shift measurement was performed with a JTS-10 spectrophotometer (Bio-logic, France). The ratio between active PSI and PSII centers [RCI]/[RCII] was estimated as described in Cardol et al. (2008). Briefly, the amplitude of the fast phase (1 ms) of the ECS signal (at 510–546 nm) was monitored upon excitation with a laser flash (Nd:YAG laser, Minilite II, Continuum). The contribution of PSII was calculated from the decrease in the ECS amplitude after the flash upon the addition of the PSII inhibitors DCMU (40 μM) and hydroxylamine (1 mM), whereas the contribution of PSI corresponded to the amplitude of the ECS that was insensitive to these inhibitors. Inhibitors were added 10 min before measurements.
Statistical Analysis
Statistical analyses of the data were performed in SigmaPlot 11.0 (Systat Software, United States) or in Statistica (StatSoft, United States). Firstly, data were tested for normality and homogeneity of variances before any further analysis, and then they were square-root- or log-transformed when required (i.e., FV/FM). The effects of treatment on the measured variables were examined on each strain by a one-way repeated-measures ANOVA followed by the SNK’s method for all pairwise multiple comparisons. Differences were considered statistically significant when P < 0.05. Principal component analysis (PCA) was performed with XLSTAT 2017 (Addinsoft, Paris, France). PCA was done by eigenvalue decomposition of a data correlation matrix after a normalization step of the initial data. The normalization of each data consisted in computing its Z-score, i.e., subtracting each data value from its variable’s measured mean and then dividing the difference by the standard deviation.
Results
Limited Photoinhibition After 4 h of Acute Heat Stress Contrasts With Different Capacity for Long-Term Thermal Tolerance
We studied here four Symbiodinium and four Breviolum strains in culture originating not only from reef-building corals but also from soft octocorals, sea anemones, and giant clam from distant geographic locations (e.g., Hawaii, Panama, Red sea, Japan; see Table 1). The impact of a long-term acute heat stress at 33°C under day/night (moderate light intensity of 75 μmol photon m–2 s–1) was first evaluated by measuring the number of cells after 12 days (Supplementary Figure 1) and 18 days (Figure 1). Maximum quantum efficiency of PSII (Fv/FM) was also measured after 18 days (Figure 1). Symbiodinium OTcH-1 and Breviolum minutum Rt-351 or SSB01 strains proved to be highly heat-sensitive. In contrast, other strains (e.g., Symbiodinium Avir or Stylodid) were rather tolerant. We then studied different photosynthetic parameters (summarized in Table 2) during the early onset of acute heat stress. Short-term treatment (up to 4 h) had only a limited impact on Fv/FM parameter in all the strains (less than 10% decrease) (Figure 2). Simultaneously, there was no significant change in the stoichiometry of active reaction centers ([RCI]/[RCII]) (Supplementary Figure 2) and in PSII relative electron transport rate (rETRPSII, calculated as the product of PSII quantum yield, ϕPSII, by the light intensity). rETRPSII saturated at the same light intensity in all strains (Supplementary Figure 3). In contrast, after 24 h at 33°C under continuous light, some strains showed a significant decrease by more than 20% in Fv/FM (Symbiodinium KLAp2, B. minutum Rt-351), in [RCI]/[RCII] (e.g., B. minutum SSB01), or in rETRPSII (Symbiodinium OTcH-1, B. minutum Rt-351). In this case, the increased duration of light exposure (24 h) could have an additional impact on the results. In the following experiments, in order to study the nature of early events that may occur at the level of the photosynthetic electron transfer chain upon acute thermal stress, we limited our study to 4 h of heat stress.
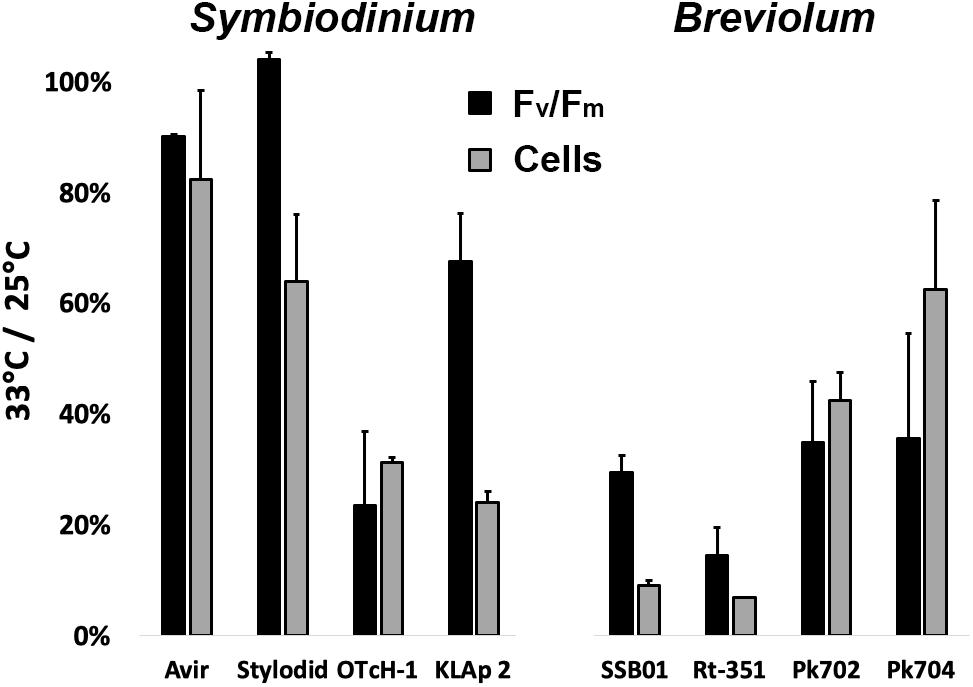
Figure 1. Impact of long-term thermal stress (33°C) on Symbiodinium and Breviolum strains. Changes in the maximum quantum yield (Fv/FM) and in cell number after 18 days of heat treatment at 33°C in 12 h: 12 h light-dark cycle. Results are reported as percentage of the values measured on cells maintained at the control temperature of 25°C. All measurements were performed on three biological replicates and data are presented as means ± SD.
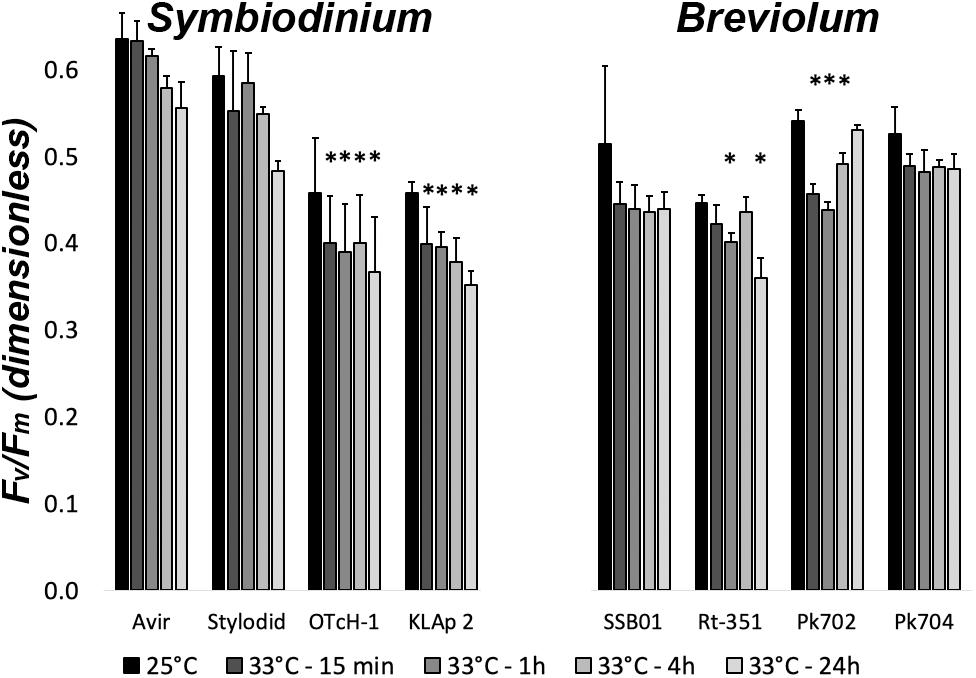
Figure 2. Maximum quantum efficiency of photosystem II (Fv/FM) in Symbiodinium and Breviolum strains upon short-term thermal stress (33°C) under continuous light. Samples were analyzed at time 0 (25°C), after 15 min, 1 h, 4 h and 24 h at 33°C. All measurements were performed on three independent biological replicates and data are presented as means ± SD. ∗value differs significantly (P < 0.05) from the control value at 25°C.
Two Phenotypic Groups With Respect to Oxygen Uptake in the Dark and in the Light Upon Acute Thermal Stress
We first measured net oxygen evolution (VO2) (Supplementary Figure 3) as a function of the light intensity and compared the obtained values to rETRPSII (Supplementary Figure 2) at 25°C and upon heat stress at 33°C. The studied strains could be classified into two distinct groups. In the first group, comprising three Symbiodinium strains (Avir, Stylodid, and OTcH-1), and B. minutum SSB01, the VO2 saturated at lower light intensities than values of rETRPSII in all conditions. Accordingly, a non-linear relationship between both parameters was found (Figure 3). Such a non-linear relationship between both parameters is indicative of a rerouting of photosynthetic electron toward oxygen at high light intensities (Roberty et al., 2014). In contrast, in the second group, comprising three Breviolum strains (Rt-351, Pk702, Pk704), and Symbiodinium KLAp2, the relationship between rETRPSII and VO2 was almost linear in all conditions (Figure 3), UOL in high light was extrapolated from average data presented in Figure 3 (Figure 4A). UOL represents around 50% of rETRPSII in high light in the first group of strains (Avir, Stylodid, OTcH-1, and SSB01), but was almost null, or very low, in the second group (Rt-351, KLAp2, Pk702, Pk704). There was no significant change in this parameter upon heat stress in any strains.
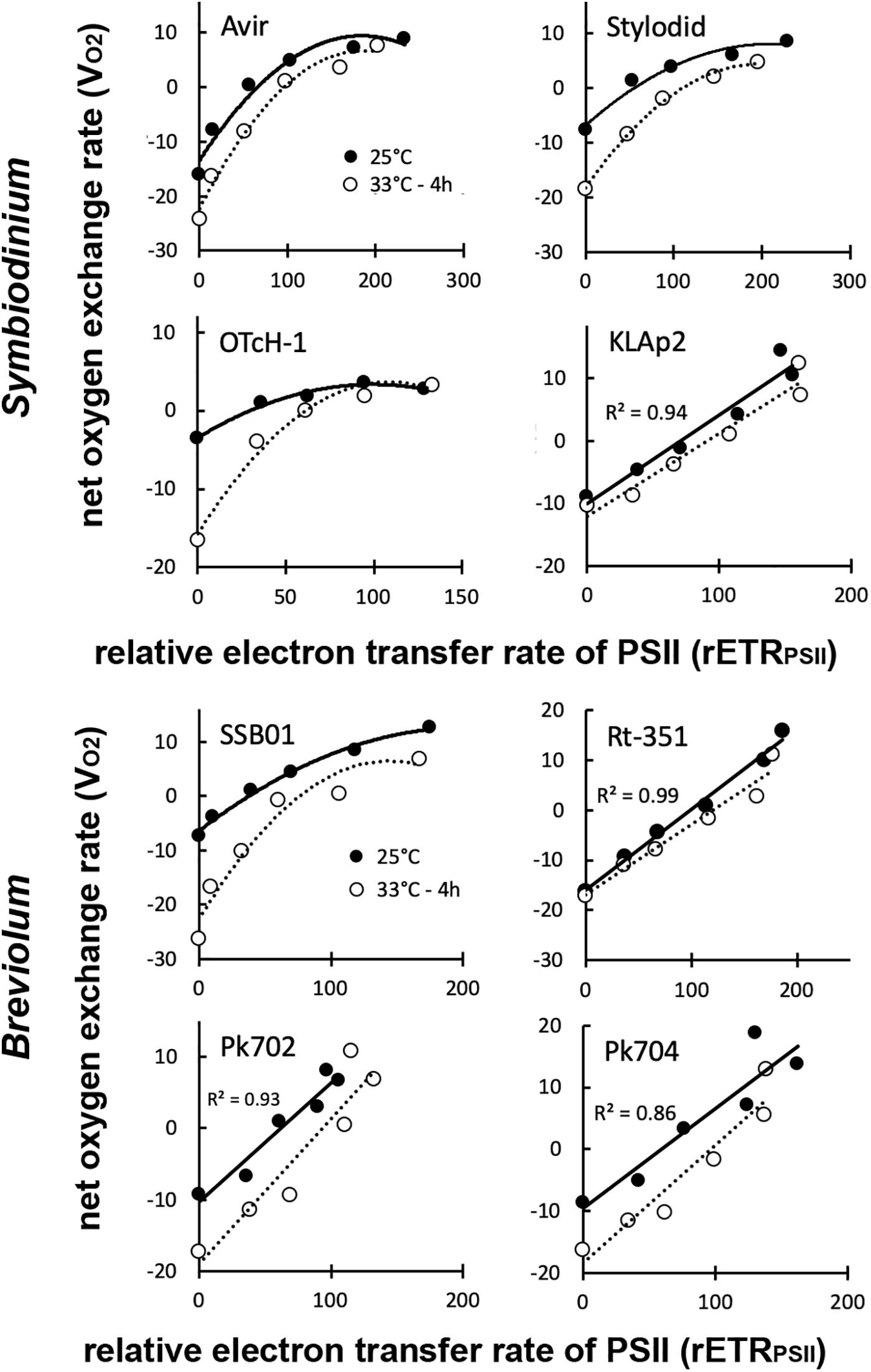
Figure 3. Comparison of net oxygen evolution (VO2) and electron transport rate of photosystem II (rETRPSII) in Symbiodinium and Breviolum strains. Samples were analyzed at time 0 (25°C) and after 4 h at 33°C. All measurements were performed on three biological replicates and data are presented as means. For details about rETRPSII (μmol electrons m–2 s–1) and VO2 (pmol O2 μg chl–1 s–1) measurements, including standard deviations, see Supplementary Figures 2, 3, respectively. Correlation coefficient of linear regression are given only for dataset at 25°C.
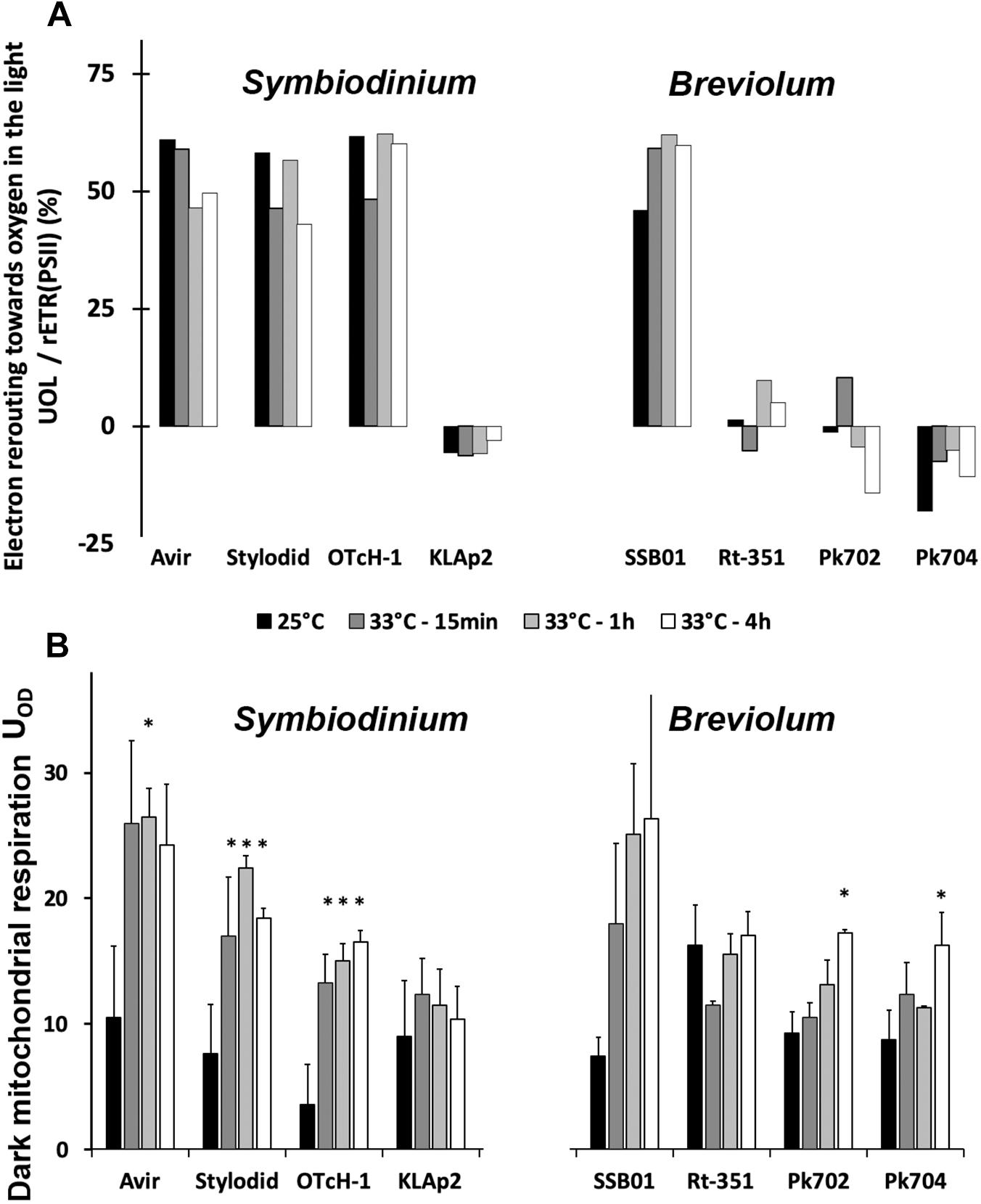
Figure 4. Oxygen exchanges during heat stress in Symbiodinium and Breviolum strains. (A) Fraction of relative electron transport rate of photosystem II (rETRPSII) that is diverted to oxygen reduction (UOL) in saturating light intensity (900 μmol photons m–2 s–1). Values were calculated from the averaged plots in Figure 3. ∗value differs significantly (P < 0.05) from the control value at 25°C. (B) Oxygen uptake in the dark (UOD, pmol O2 μg chl–1 s–1) retrieved out of Supplementary Figure 3. All measurements were performed on three biological replicates and data are presented as means ± SD.
Besides differences in the relationship between VO2 and rETRPSII, species of the two groups showed different responses of their uptake of oxygen in the dark (UOD), a parameter which mainly reflects mitochondrial respiration. For clarity, UOD rates are shown on a separate chart (Figure 4B). Whereas for species of the first group (Avir, Stylodid, OTcH-1, and SSB01) UOD rates increased (2.5–5 times) after 15 min at 33°C, UOD of species of the second group (Rt-351, KLAp2, Pk702, Pk704) did not change significantly or increased to a lesser extent after 4 h.
Cyclic Electron Flow Capacity Upon Acute Heat Stress Is Higher in Symbiodinium Strains Than in Breviolum Strains
We then determined the kinetics (s–1) of PSI primary donor (P700) re-reduction in the presence of DCMU (a PSII inhibitor) in the different cultured strains (Figure 5). The kinetics of P700 re-reduction in the presence of DCMU is usually considered to reflect PSII-independent PSI-dependent CEF capacity (e.g., Alric, 2010). In the control condition (25°C), it was about 10–20 electrons s–1 in all strains, which is in good agreement with previous reports (Roberty et al., 2014; Aihara et al., 2016). Upon heat stress, CEF increased in all of them except in Symbiodinium KLAp2. The largest significant increases were recorded for the three other Symbiodinium strains, with maximal values around 80 electrons s–1. In contrast, Breviolum strains displayed much lower values, with a maximal value of 50 electrons s–1 in Rt-351.
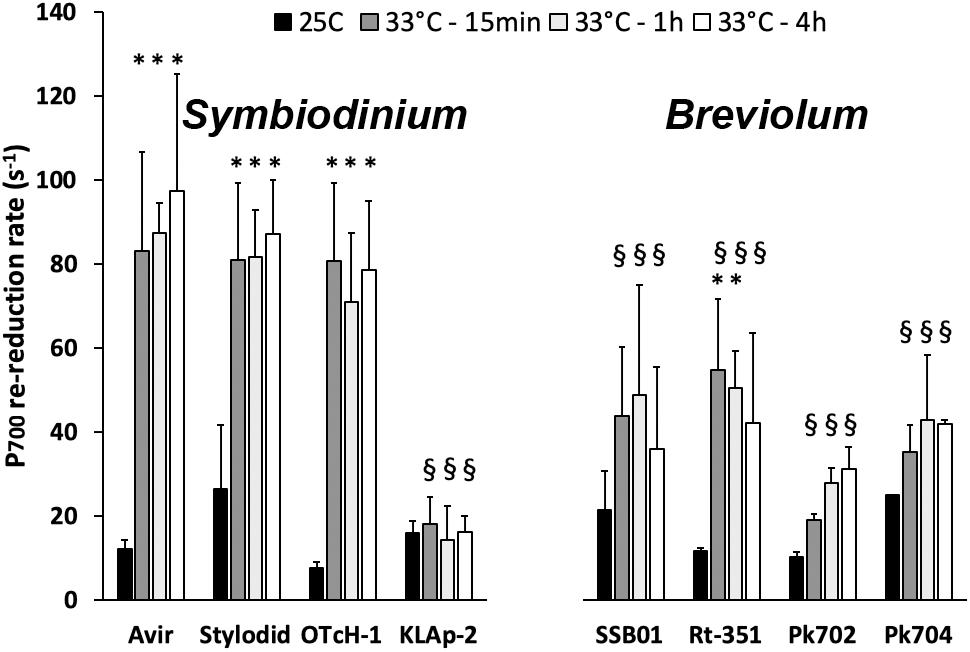
Figure 5. P700 re-reduction rates in presence of DMCU in Symbiodinium and Breviolum strains. Samples were analyzed at time 0 (25°C), after 15 min, 1 h, 4 h and 24 h at 33°C. All measurements were performed on three biological replicates and data are presented as means ± SD. ∗value differs significantly (P < 0.05) from the control value at 25°C. § value differs significantly (P < 0.05) from the value for Avir strain at the same timepoint.
Principal Component Analysis Indicates That Tolerant/Bleaching Phenotypes Are Uncorrelated With the Photosynthetic Phenotypes
A PCA was then performed on the main dataset presented here above. The two first principal components account for almost 70% of the variance (Figure 6 and Supplementary Table 1). In these results, first principal component has a large positive association with three variables (UOD, UOL, and CEF) so this first component primarily measures strain’s photosynthetic phenotype. The second component has a large positive association with cell survival and photosynthetic efficiency after long exposure at 33°C, reflecting the strain’s tolerant phenotype. The third principal component explained almost 20% of the variance (Supplementary Figure 5) and is positively associated with Fv/FM after 24 h of acute thermal stress. Overall, this analysis shows that the variables accounting for strain’s tolerant/bleaching phenotype are uncorrelated to variables accounting for the photosynthetic phenotypes described here.
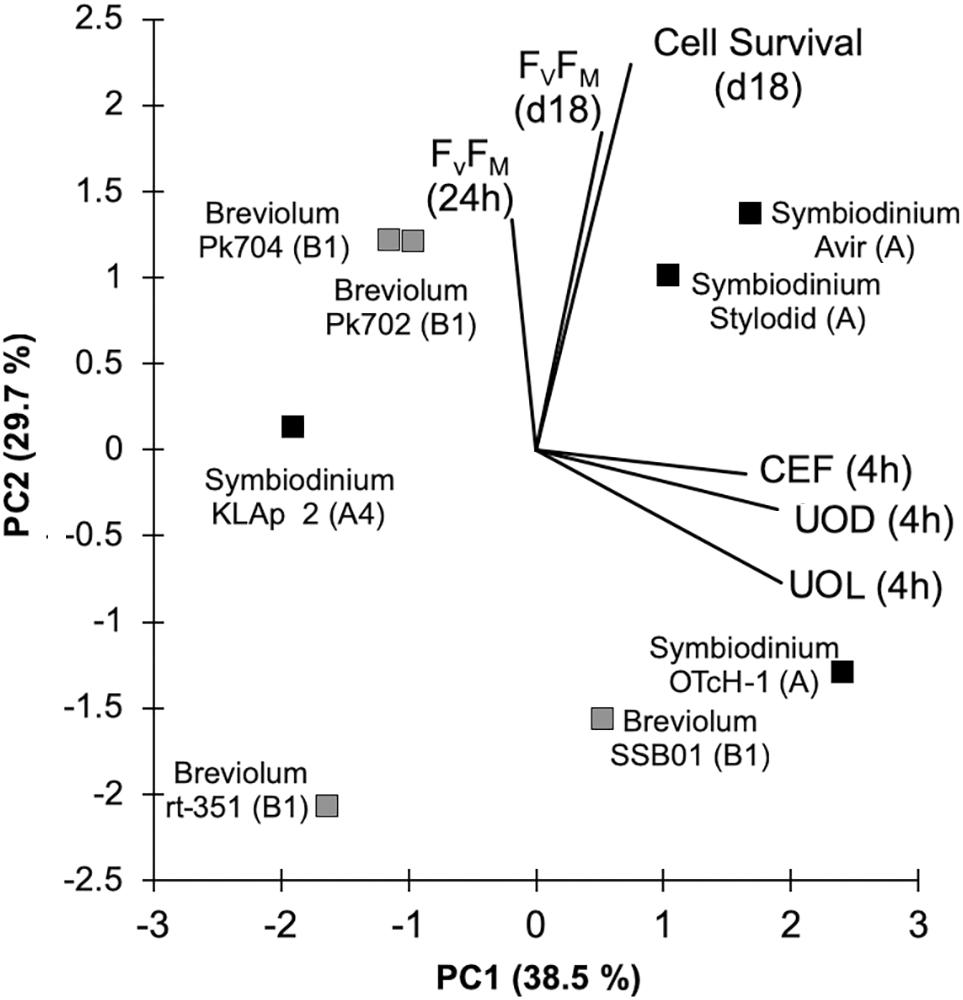
Figure 6. Principal component analysis (PCA) plot showing the grouping of cultured Symbiodinium (black squares) and Breviolum spp. (gray squares) based on the main photosynthetic and physiological traits. Black lines indicate the correlation of the different parameters with PC1 and PC2.
Discussion
After short-term exposure at 33°C (up to 4 h), CEF capacity measured as the rate of P700 re-reduction in presence of the PSII inhibitor DCMU was significantly larger in cultures of Symbiodinium spp. (except for strain KLAp2) than in cultures of Breviolum spp. Aihara et al. (2016) also reported that the kinetics of P700 re-reduction in the presence of DCMU after 15 min at 33°C, is larger in Symbiodinium strain OTcH-1 than in B. minutum (strain Mf1.05b). Reynolds et al. (2008) also concluded that Symbiodinium strains (formerly Clade A) are more prone to perform CEF than Breviolum strains (formerly Clade B), and these authors proposed that the association of Symbiodinium (clade A) strains confers resistance to bleaching. Conversely, because Symbiodinium (clade A) OtcH-1 strain exhibited larger CEF and was more susceptible to elevated temperature than Breviolum Mf1.05b (Clade B), Aihara et al. (2016) concluded that thermal tolerance and CEF capacity are inversely correlated. By examining more strains, we now rather show that there is no correlation between long-term tolerance to an acute increase in temperature and short-term increase of CEF capacity in Symbiodinium and Breviolum strains in culture. Indeed, other Symbiodinium (Avir or Stylodid) strains are thermotolerant and exhibit similar CEF capacity when compared to OtcH-1 strain. Such contrasted response to heat stress among strains of Symbiodinium (Clade A) has been already reported (e.g., Díaz-Almeyda et al., 2017; Goyen et al., 2017). Also, the four Breviolum strains, all exhibiting the same increase in CEF capacity during the first hour at 33°C, displayed different responses to long-term incubation at 33°C, Pk702 and Pk704 being much less impacted than SSB01 or Rt-351. Similarly, Goyen et al. (2017) also concluded on the absence of correlation between the Symbiodiniaceae phylogenetic groups and the distribution of the isolates among photosynthetic functional groups. At this point, we must point out that the method used here to estimate the capacity of the CEF probably does not account for the situation in the absence of PSII inhibitor DCMU (see also discussion in Szabó et al., 2017). A measurement of the activity of PSI in the absence of DCMU is possible but technically more complicated (Roberty et al., 2014; Fan et al., 2016; Szabó et al., 2017) and we have chosen to focus on the method used in Aihara et al. (2016) to be able to compare datasets without ambiguity.
Roberty et al. (2014) showed that high light-dependent oxygen uptake (UOL) values observed in high light regime in many strains of Symbiodiniaceae, including Symbiodinium Avir could be attributed to oxygen reduction downstream of PSI by a Mehler-like activity. Because the UOL capacity was still high under heat stress (Suggett et al., 2008; Roberty et al., 2015), Roberty et al. (2015) suggested that this AEF might be crucial to cope with elevated temperature.
As well as for CEF, there is no correlation between the amplitude of the oxygen uptake in high light (UOL) and the thermotolerance at 33°C. For instance, elevated UOL at 33°C in Symbiodinium OTcH-1 or Breviolum SSB01 is not sufficient to promote long-term thermal resistance. OTcH-1 and SSB01 strains are also more sensitive to heat than other strains (e.g., Breviolum Pk702 and Pk704) apparently lacking this alternative pathway. The method used here to estimate oxygen uptake (UOL) assumes that UOL is very weak in low light. Such a hypothesis should be confirmed by measuring the uptake of oxygen in the light by membrane-inlet mass spectrometry (MIMS). We cannot therefore fully exclude that the linear relationship between VO2 and rETR-PSII of some strains is indicative of an uptake of oxygen in the light which is proportional to rETR-PSII. Nevertheless, the fact remains that the results of such measurements delineate two groups of strains, each group comprising thermosensitive and thermotolerant strains. According to our interpretation of the data, the apparent absence of UOL under high light in four strains, together with the occurrence of CEF (shown by elevated P700 re-reduction rate in presence of DCMU), in the majority of the studied strains, challenges a previous conclusion that electron rerouting toward oxygen is the main alternative electron pathway in Symbiodiniaceae (Roberty et al., 2014). As stated above, the capacity of the CEF estimated as the re-reduction rate of P700 in presence of DCMU gives us only very little information about what happens under normal conditions. However, it is generally assumed that an increase of this parameter is indicative of an accumulation of reducing power (e.g., NADPH or reduced ferredoxin) which feeds in return the electron transfer chain at the level of the PQ or cytochrome b6f complex (Aihara et al., 2016; Nawrocki et al., 2019). Aihara et al. (2016) suggested that NADPH may over accumulate when CO2 fixation is impaired in Symbiodinium, which could occur during thermal stress through the inactivation of the Rubisco (Jones et al., 1998; Lilley et al., 2010). However, the inhibition of CO2 fixation by the addition of glycolaldehyde had only a moderate impact on P700 re-reduction rate in the presence of DCMU in OTcH-1 strain (Aihara et al., 2016) or in SSB01 strain (Supplementary Figure 6). This observation plays in favor of additional metabolic changes at elevated temperature, a conclusion in agreement with those of a previous work on cultured Symbiodinium cells and on nubbins of the coral Pocillopora damicornis (Hill et al., 2014). Because, the rate of most enzymatic reactions increases as the temperature is raised (at least up to a physiological optimum), the increase in P700 re-reduction rate in the presence of DCMU in response to elevated temperature may indicate that the rate of NADPH:PQ oxidoreductase activity is also increased in response to temperature. In addition, the redox poise of the stroma may increase if NAD(P)H-producing pathways (e.g., glycolysis) are favored at the expense of NAD(P)H-consuming enzymes. In this respect, the dark respiratory activity of some strains was found to be higher at 33°C than at 25°C, potentially reflecting a response to ATP deficiency. Mitochondrial respiration is less sensitive to increasing temperature than photosynthesis in Symbiodinium (Iglesias-Prieto et al., 1992). In Cladocopium (formerly Clade C) C1 152 strain (Oakley et al., 2014), the contribution of mitochondrial alternative oxidase to total respiratory rate increased at 32°C. However, total respiratory rate did not increase at short-term or long-term (Oakley et al., 2014). Again, in the present study, there was no direct correlation between changes in respiratory rate upon short-term incubation at 33°C and the long-term response.
It has been postulated in several previous works that the ecological success of some symbiotic Symbiodiniaceae in response to elevated temperature or in highly fluctuating light environment such as in shallow waters is due the occurrence of photosynthetic AEF (Warner and Berry-Lowe, 2006; Reynolds et al., 2008; Roberty et al., 2015). Overall, considered independently, none of the parameters studied upon short-term acute exposure at 33°C (respiration, electron rerouting toward oxygen, activity of photosystem I or II) is correlated with the long-term thermal resistance capacity of the cultured Breviolum and Symbiodinium strains investigated. However, the two most thermotolerant cultured strains (Symbiodinium Avir and Stylodid) share all common photosynthetic features upon short-term exposure to heat, namely capacity to reroute a large fraction of electron to oxygen in high light, increased oxygen uptake in the dark respiratory rate and very fast reduction rate of the primary PSI donor (P700) under conditions where PSII is inhibited. These features indicate a greater plasticity of the metabolism in these strains that may concur to prevent photodamage. Many other parameters may influence thermal sensitivity such as: the ability to detoxify ROS (Krueger et al., 2014; Roberty et al., 2015) or to produce molecular chaperones (i.e., Rosic et al., 2011); the thermal sensitivity of the repair mechanisms of photodamaged photosynthetic components (Takahashi et al., 2009). Stable adaptive changes to increased temperature may also result from genetic mutations or epigenetic modifications of gene expression (Chakravarti and van Oppen, 2018). Finally, it is worth mentioning that corals are subjected in nature to slower shifts in temperature providing time for acclimatization of metabolic apparatus. In this respect, it has been shown that a sliding scale of thermotolerance can be acquired from weeks to seasons (e.g., Takahashi et al., 2013; Chakravarti et al., 2017; Nitschke et al., 2018). At this stage, the possibility that AEF contribute to thermotolerance of holobionts under circumstances of slow changes in temperature remains to be evaluated.
Data Availability Statement
All datasets generated for this study are included in the article/Supplementary Files.
Author Contributions
KD, SR, and PC conceived the original research plans. PC and SR supervised the experiments, conceived the study, wrote the manuscript with contributions of all the authors, and agreed to serve as the authors responsible for contact and ensure communication. KD and MP performed most of the experiments. PC analyzed the data.
Funding
PC acknowledges the financial support from the Belgian Fonds de la Recherche Scientifique (FRS-FNRS) (CDR J.0079) and European Research Council (ERC, H2020-EU BEAL project 682580).
Conflict of Interest
The authors declare that the research was conducted in the absence of any commercial or financial relationships that could be construed as a potential conflict of interest.
Acknowledgments
We thank F. Vega de Luna and J. Minagawa for stimulating discussions during the preparation of this manuscript. We thank J. Minagawa, Annika Guse, and M. Warner for sharing some of the cultures studied. KD and PC are post-doctoral researcher and a Senior Research Associate from F.R.S.-FNRS, respectively.
Supplementary Material
The Supplementary Material for this article can be found online at: https://www.frontiersin.org/articles/10.3389/fmars.2019.00656/full#supplementary-material
References
Abrego, D., Ulstrup, K. E., Willis, B. L., and Van Oppen, M. J. H. (2008). Species-specific interactions between algal endosymbionts and coral hosts define their bleaching response to heat and light stress. Proc. R. Soc. B Biol. Sci. 275, 2273–2282. doi: 10.1098/rspb.2008.0180
Aihara, Y., Takahashi, S., and Minagawa, J. (2016). Heat induction of cyclic electron flow around photosystem I in the symbiotic dinoflagellate Symbiodinium. Plant Physiol. 171, 522–529. doi: 10.1104/pp.15.01886
Alric, J. (2010). Cyclic electron flow around photosystem I in unicellular green algae. Photosynth. Res. 106, 47–56. doi: 10.1007/s11120-010-9566-4
Badger, M. R., Von Caemmerer, S., Ruuska, S., and Nakano, H. (2000). Electron flow to oxygen in higher plants and algae: rates and control of direct photoreduction (mehler reaction) and rubisco oxygenase. Philos. Trans. R. Soc. Lond. Ser. B 355, 1433–1446. doi: 10.1098/rstb.2000.0704
Baird, A. H., Bhagooli, R., Ralph, P. J., and Takahashi, S. (2009). Coral bleaching: the role of the host. Trends Ecol. Evol. 24, 16–20. doi: 10.1016/j.tree.2008.09.005
Baumgarten, S., Simakov, O., Esherick, L. Y., Liew, Y. J., Lehnert, E. M., Michell, C. T., et al. (2015). The genome of Aiptasia, a sea anemone model for coral symbiosis. Proc. Natl. Acad. Sci. U.S.A. 112, 11893–11898. doi: 10.1073/pnas.1513318112
Bhagooli, R., and Hidaka, M. (2003). Comparison of stress susceptibility of in hospite and isolated zooxanthellae among five coral species. J. Exp. Mar. Biol. Ecol. 291, 181–197. doi: 10.1016/s0022-0981(03)00121-7
Bouchard, J. N., and Yamasaki, H. (2008). Heat stress stimulates nitric oxide production in Symbiodinium microadriaticum: a possible linkage between nitric oxide and the coral bleaching phenomenon. Plant Cell Physiol. 49, 641–652. doi: 10.1093/pcp/pcn037
Cardol, P., Bailleul, B., Rappaport, F., Derelle, E., Béal, D., Breyton, C., et al. (2008). An original adaptation of photosynthesis in the marine green alga Ostreococcus. Proc. Natl. Acad. Sci. U.S.A. 105, 7881–7886. doi: 10.1073/pnas.0802762105
Cardol, P., De Paepe, R., Franck, F., Forti, G., and Finazzi, G. (2010). The onset of NPQ and ΔμH+ upon illumination of tobacco plants studied through the influence of mitochondrial electron transport. Biochim. Biophys. Acta Bioenerg. 1797, 177–188. doi: 10.1016/j.bbabio.2009.10.002
Chakravarti, L. J., Beltran, V. H., and van Oppen, M. J. H. (2017). Rapid thermal adaptation in photosymbionts of reef-building corals. Glob. Change Biol. 23, 4675–4688. doi: 10.1111/gcb.13702
Chakravarti, L. J., and van Oppen, M. J. H. (2018). Experimental evolution in coral photosymbionts as a tool to increase thermal tolerance. Front. Mar. Sci. 5:227. doi: 10.3389/fmars.2018.00227
Díaz-Almeyda, E. M., Prada, C., Ohdera, A. H., Moran, H., Civitello, D. J., Iglesias-Prieto, R., et al. (2017). Intraspecific and interspecific variation in thermotolerance and photoacclimation in Symbiodinium dinoflagellates. Proc. R. Soc. B Biol. Sci. 284:20171767. doi: 10.1098/rspb.2017.1767
Fan, D. Y., Fitzpatrick, D., Oguchi, R., Ma, W., Kou, J., and Chow, W. S. (2016). Obstacles in the quantification of the cyclic electron flux around photosystem I in leaves of C3 plants. Photosynth. Res. 129, 239–251. doi: 10.1007/s11120-016-0223-4
Frieler, K., Meinshausen, M., Golly, A., Mengel, M., Lebek, K., Donner, S., et al. (2013). Limiting global warming to 2°C is unlikely to save most coral reefs. Nat. Clim. Change 3, 165–170. doi: 10.1038/nclimate1674
Genty, B., Briantais, J. M., and Baker, N. R. (1989). The relationship between the quantum yield of photosynthetic electron transport and quenching of chlorophyll fluorescence. Biochim. Biophys. Acta Gen.Subjects 990, 87–92. doi: 10.1016/s0304-4165(89)80016-9
Goyen, S., Pernice, M., Szabó, M., Warner, M. E., Ralph, P. J., and Suggett, D. J. (2017). A molecular physiology basis for functional diversity of hydrogen peroxide production amongst Symbiodinium spp.(Dinophyceae). Mar. Biol. 164:46.
Hawkins, T. D., Hagemeyer, J. C., Hoadley, K. D., Marsh, A. G., and Warner, M. E. (2016a). Partitioning of respiration in an animal-algal symbiosis: implications for different aerobic capacity between Symbiodinium spp. Front. Physiol. 7:128. doi: 10.3389/fphys.2016.00128
Hawkins, T. D., Hagemeyer, J. C. G., and Warner, M. E. (2016b). Temperature moderates the infectiousness of two conspecific Symbiodinium strains isolated from the same host population. Environ. Microbiol. 18, 5204–5217. doi: 10.1111/1462-2920.13535
Hill, R., Szabó, M., ur Rehman, A., Vass, I., Ralph, P. J., and Larkum, A. W. D. (2014). Inhibition of photosynthetic CO2 fixation in the coral Pocillopora damicornis and its relationship to thermal bleaching. J. Exp. Biol. 217, 2150–2162. doi: 10.1242/jeb.100578
Hughes, T. P., Anderson, K. D., Connolly, S. R., Heron, S. F., Kerry, J. T., Lough, J. M., et al. (2018). Spatial and temporal patterns of mass bleaching of corals in the Anthropocene. Science 359, 80–83. doi: 10.1126/science.aan8048
Hume, B. C., Ziegler, M., Poulain, J., Pochon, X., Romac, S., Boissin, E., et al. (2018). An improved primer set and amplification protocol with increased specificity and sensitivity targeting the Symbiodinium ITS2 region. PeerJ 6:e4816. doi: 10.7717/peerj.4816
Iglesias-Prieto, R., Matta, J. L., Robins, W. A., and Trench, R. K. (1992). Photosynthetic response to elevated temperature in the symbiotic dinoflagellate Symbiodinium microadriaticum in culture. Proc. Natl. Acad. Sci. U.S.A. 89, 10302–10305. doi: 10.1073/pnas.89.21.10302
Ishikura, M., Hagiwara, K., Takishita, K., Haga, M., Iwai, K., and Maruyama, T. (2004). Isolation of new Symbiodinium strains from tridacnid giant clam (Tridacna crocea) and sea slug (Pteraeolidia ianthina) using culture medium containing giant clam tissue homogenate. Mar. Biotechnol. 6, 378–385. doi: 10.1007/s10126-004-1800-7
Johnson, X., Steinbeck, J., Dent, R. M., Takahashi, H., Richaud, P., Ozawa, S. I., et al. (2014). Proton gradient regulation 5-mediated cyclic electron flow under ATP-or redox-limited conditions: a study of ΔATpase pgr5 and ΔrbcL pgr5 mutants in the green alga Chlamydomonas reinhardtii. Plant Physiol. 165, 438–452. doi: 10.1104/pp.113.233593
Jones, R. J., Hoegh-Guldberg, O., Larkum, A. W. D., and Schreiber, U. (1998). Temperature-induced bleaching of corals begins with impairment of the CO2 fixation mechanism in zooxanthellae. Plant Cell Environ. 21, 1219–1230. doi: 10.1046/j.1365-3040.1998.00345.x
Krueger, T., Becker, S., Pontasch, S., Dove, S., Hoegh-Guldberg, O., Leggat, W., et al. (2014). Antioxidant plasticity and thermal sensitivity in four types of Symbiodinium sp. J. Phycol. 50, 1035–1047. doi: 10.1111/jpy.12232
LaJeunesse, T. C., Parkinson, J. E., Gabrielson, P. W., Jeong, H. J., Reimer, J. D., Voolstra, C. R., et al. (2018). Systematic revision of Symbiodiniaceae highlights the antiquity and diversity of coral endosymbionts. Curr. Biol. 28, 2570–2580. doi: 10.1016/j.cub.2018.07.008
Leggat, W., Whitney, S., and Yellowlees, D. (2004). Is coral bleaching due to the instability of the zooxanthellae dark reactions? Symbiosis 37, 137–153.
Lesser, M. P. (1996). Elevated temperatures and ultraviolet radiation cause oxidative stress and inhibit photosynthesis in symbiotic dinoflagellates. Limnol. Oceanogr. 41, 271–283. doi: 10.4319/lo.1996.41.2.0271
Lesser, M. P., and Farrell, J. H. (2004). Exposure to solar radiation increases damage to both host tissues and algal symbionts of corals during thermal stress. Coral Reefs 23, 367–377. doi: 10.1007/s00338-004-0392-z
Levin, R. A., Beltran, V. H., Hill, R., Kjelleberg, S., McDougald, D., Steinberg, P. D., et al. (2016). Sex, scavengers, and chaperones: transcriptome secrets of divergent Symbiodinium thermal tolerances. Mole. Biol. Evol. 33, 2201–2215. doi: 10.1093/molbev/msw119
Lilley, R. M., Ralph, P. J., and Larkum, A. W. D. (2010). The determination of activity of the enzyme rubisco in cell extracts of the dinoflagellate alga Symbiodinium sp. by manganese chemiluminescence and its response to short-term thermal stress of the alga. Plant Cell Environ. 33, 995–1004. doi: 10.1111/j.1365-3040.2010.02121.x
Munekage, Y. N., Genty, B., and Peltier, G. (2008). Effect of PGR5 impairment on photosynthesis and growth in Arabidopsis thaliana. Plant Cell Physiol. 49, 1688–1698. doi: 10.1093/pcp/pcn140
Myhre, G., Shindell, D., Bréon, F., Collins, W., Fuglestvedt, J., Huang, J., et al. (2013). Climate Change 2013: the Physical Science Basis. Contribution of Working Group I to the Fifth Assessment Report of the Intergovernmental Panel on Climate Change. Cambridge: Cambridge University Press.
Nawrocki, W. J., Bailleul, B., Cardol, P., Rappaport, F., Wollman, F. A., and Joliot, P. (2019). Maximal cyclic electron flow rate is independent of PGRL1 in Chlamydomonas. Biochim. Biophys. Acta Bioenerget. 1860, 425–432. doi: 10.1016/j.bbabio.2019.01.004
Nitschke, M. R., Gardner, S. G., Goyen, S., Fujise, L., Camp, E. F., Ralph, P. J., et al. (2018). Utility of photochemical traits as diagnostics of thermal tolerance amongst great barrier reef corals. Front. Mar. Sci. 5:45. doi: 10.3389/fmars.2018.00045
Oakley, C. A., Schmidt, G. W., and Hopkinson, B. M. (2014). Thermal responses of Symbiodinium photosynthetic carbon assimilation. Coral Reefs 33, 501–512. doi: 10.1007/s00338-014-1130-9
Parkinson, J. E., Baumgarten, S., Michell, C. T., Baums, I. B., LaJeunesse, T. C., and Voolstra, C. R. (2016). Gene expression variation resolves species and individual strains among coral-associated dinoflagellates within the genus Symbiodinium. Genom. Biol. Evol. 8, 665–680. doi: 10.1093/gbe/evw019
Reynolds, J. M., Bruns, B. U., Fitt, W. K., and Schmidt, G. W. (2008). Enhanced photoprotection pathways in symbiotic dinoflagellates of shallow-water corals and other cnidarians. Proc. Natl. Acad. Sci. U.S.A. 105, 13674–13678. doi: 10.1073/pnas.0805187105
Ritchie, R. J. (2006). Consistent sets of spectrophotometric chlorophyll equations for acetone, methanol and ethanol solvents. Photosynth. Res. 89, 27–41. doi: 10.1007/s11120-006-9065-9
Roberty, S., Bailleul, B., Berne, N., Franck, F., and Cardol, P. (2014). PSI Mehler reaction is the main alternative photosynthetic electron pathway in Symbiodinium sp., symbiotic dinoflagellates of cnidarians. New Phytol. 204, 81–91. doi: 10.1111/nph.12903
Roberty, S., Fransolet, D., Cardol, P., Plumier, J. C., and Franck, F. (2015). Imbalance between oxygen photoreduction and antioxidant capacities in Symbiodinium cells exposed to combined heat and high light stress. Coral Reefs 34, 1063–1073. doi: 10.1007/s00338-015-1328-5
Roberty, S., Furla, P., and Plumier, J. C. (2016). Differential antioxidant response between two Symbiodinium species from contrasting environments. Plant Cell Environ. 39, 2713–2724. doi: 10.1111/pce.12825
Robison, J. D., and Warner, M. E. (2006). Differential impacts of photoacclimation and thermal stress on the photobiology of four different phylotypes of Symbiodinium (Pyrrhophyta). J. Phycol. 42, 568–579. doi: 10.1111/j.1529-8817.2006.00232.x
Rosic, N., Pernice, M., Dove, S., Dunn, S., and Hoegh-Guldberg, O. (2011). Gene expression profiles of cytosolic heat shock proteins Hsp70 and Hsp90 from symbiotic dinoflagellates in response to thermal stress: possible implications for coral bleaching. Cell Stress Chaperones 16, 69–80. doi: 10.1007/s12192-010-0222-x
Roth, M. S. (2014). The engine of the reef: photobiology of the coral-algal symbiosis. Front. Microbiol. 5:422. doi: 10.3389/fmicb.2014.00422
Silverstein, R. N., Cunning, R., and Baker, A. C. (2015). Change in algal symbiont communities after bleaching, not prior heat exposure, increases heat tolerance of reef corals. Glob. Change Biol. 21, 236–249. doi: 10.1111/gcb.12706
Silverstein, R. N., Cunning, R., and Baker, A. C. (2017). Tenacious D: Symbiodinium in clade D remain in reef corals at both high and low temperature extremes despite impairment. J. Exp. Biol. 220, 1192–1196. doi: 10.1242/jeb.148239
Smith, D. J., Suggett, D. J., and Baker, N. R. (2005). Is photoinhibition of zooxanthellae photosynthesis the primary cause of thermal bleaching in corals? Glob. Change Biol. 11, 1–11. doi: 10.1111/j.1529-8817.2003.00895.x
Suggett, D. J., and Smith, D. J. (2011). Interpreting the sign of coral bleaching as friend vs. foe. Glob. Change Biol. 17, 45–55. doi: 10.1111/j.1365-2486.2009.02155.x
Suggett, D. J., Warner, M. E., and Leggat, W. (2017). Symbiotic dinoflagellate functional diversity mediates coral survival under ecological crisis. Trends Ecol. Evol. 32, 735–745. doi: 10.1016/j.tree.2017.07.013
Suggett, D. J., Warner, M. E., Smith, D. J., Davey, P., Hennige, S., and Baker, N. R. (2008). Photosynthesis and production of hydrogen peroxide by Symbiodinium (Pyrrhophyta) phylotypes with different thermal tolerances. J. Phycol. 44, 948–956. doi: 10.1111/j.1529-8817.2008.00537.x
Szabó, M., Larkum, A. W. D., Suggett, D. J., Vass, I., Sass, L., Osmond, B., et al. (2017). Non-intrusive assessment of Photosystem II and Photosystem I in whole coral tissues. Front. Mar. Sci. 4:269.
Takahashi, S., Nakamura, T., Sakamizu, M., Van Woesik, R., and Yamasaki, H. (2004). Repair machinery of symbiotic photosynthesis as the primary target of heat stress for reef-building corals. Plant Cell Physiol. 45, 251–255. doi: 10.1093/pcp/pch028
Takahashi, S., Whitney, S. M., and Badger, M. R. (2009). Different thermal sensitivity of the repair of photodamaged photosynthetic machinery in cultured Symbiodinium species. Proc. Natl. Acad. Sci. U.S.A. 106, 3237–3242. doi: 10.1073/pnas.0808363106
Takahashi, S., Yoshioka-Nishimura, M., Nanba, D., and Badger, M. R. (2013). Thermal acclimation of the symbiotic alga Symbiodinium spp. alleviates photobleaching under heat stress. Plant Physiol. 161, 477–485. doi: 10.1104/pp.112.207480
Tchernov, D., Gorbunov, M. Y., De Vargas, C., Yadav, S. N., Milligan, A. J., Haggblom, M., et al. (2004). Membrane lipids of symbiotic algae are diagnostic of sensitivity to thermal bleaching in corals. Proc. Natl. Acad. Sci. U.S.A. 101, 13531–13535. doi: 10.1073/pnas.0402907101
Ulstrup, K. E., Hill, R., and Ralph, P. J. (2005). Photosynthetic impact of hypoxia on in hospite zooxanthellae in the scleractinian coral Pocillopora damicornis. Mar. Ecol. Prog. Ser. 286, 125–132. doi: 10.3354/meps286125
Van Hooidonk, R., Maynard, J., and Planes, S. (2013). Temporary refugia for coral reefs in a warming world. Nat. Clim. Change 3, 508–511. doi: 10.1038/nclimate1829
Venn, A. A., Loram, J. E., and Douglas, A. E. (2008). Photosynthetic symbioses in animals. J. Exp. Bot. 59, 1069–1080. doi: 10.1093/jxb/erm328
Visram, S., Wiedenmann, J., and Douglas, A. E. (2006). Molecular diversity of symbiotic algae of the genus Symbiodinium (Zooxanthellae) in cnidarians of the Mediterranean Sea. J. Mar. Biol. Assoc. U.K. 86, 1281–1283. doi: 10.1017/s0025315406014299
Warner, M. E., and Berry-Lowe, S. (2006). Differential xanthophyll cycling and photochemical activity in symbiotic dinoflagellates in multiple locations of three species of caribbean coral. J. Exp. Mar. Biol. Ecol. 339, 86–95. doi: 10.1016/j.jembe.2006.07.011
Warner, M. E., Fitt, W. K., and Schmidt, G. W. (1999). Damage to photosystem II in symbiotic dinoflagellates: a determinant of coral bleaching. Proc. Natl. Acad. Sci. U.S.A. 96, 8007–8012. doi: 10.1073/pnas.96.14.8007
Warner, M. E., and Suggett, D. J. (2016). “The photobiology of symbiodinium spp.: linking physiological diversity to the implications of stress and resilience,” in The Cnidaria, Past, Present and Future: The world of Medusa and her sisters, eds S. Goffredo, and Z. Dubinsky, (Cham: Springer International Publishing), 489–509. doi: 10.1007/978-3-319-31305-4_30
Weis, V. M. (2008). Cellular mechanisms of Cnidarian bleaching: stress causes the collapse of symbiosis. J. Exp. Biol. 211, 3059–3066. doi: 10.1242/jeb.009597
Keywords: scleractinian corals, cnidaria, photoacclimation, cyclic electron flow, oxygen photoreduction
Citation: Dang KV, Pierangelini M, Roberty S and Cardol P (2019) Alternative Photosynthetic Electron Transfers and Bleaching Phenotypes Upon Acute Heat Stress in Symbiodinium and Breviolum spp. (Symbiodiniaceae) in Culture. Front. Mar. Sci. 6:656. doi: 10.3389/fmars.2019.00656
Received: 19 June 2019; Accepted: 08 October 2019;
Published: 22 October 2019.
Edited by:
Noga Stambler, Bar-Ilan University, IsraelReviewed by:
David Suggett, University of Technology Sydney, AustraliaEmma Mary Gibbin, École Polytechnique Fédérale de Lausanne, Switzerland
Copyright © 2019 Dang, Pierangelini, Roberty and Cardol. This is an open-access article distributed under the terms of the Creative Commons Attribution License (CC BY). The use, distribution or reproduction in other forums is permitted, provided the original author(s) and the copyright owner(s) are credited and that the original publication in this journal is cited, in accordance with accepted academic practice. No use, distribution or reproduction is permitted which does not comply with these terms.
*Correspondence: Stéphane Roberty, c3JvYmVydHlAdWxpZWdlLmJl; Pierre Cardol, cGllcnJlLmNhcmRvbEB1bGllZ2UuYmU=
†These authors share senior authorship